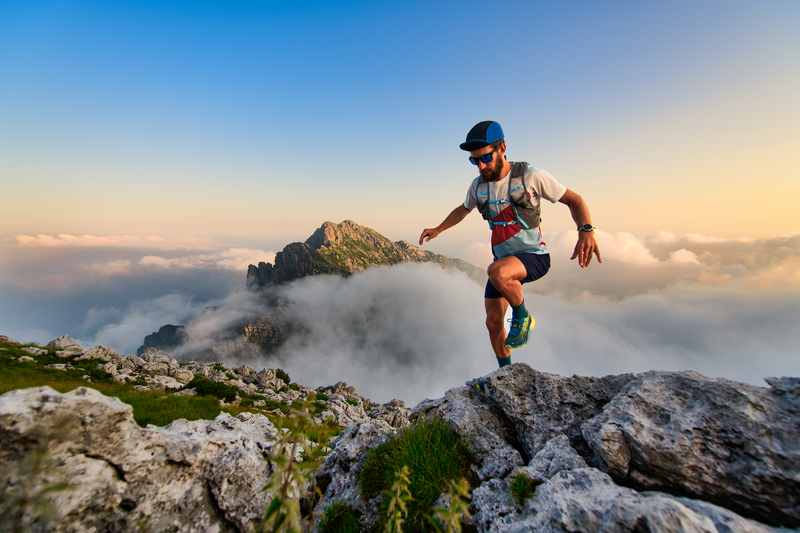
95% of researchers rate our articles as excellent or good
Learn more about the work of our research integrity team to safeguard the quality of each article we publish.
Find out more
ORIGINAL RESEARCH article
Front. Cell. Infect. Microbiol. , 19 October 2021
Sec. Microbiome in Health and Disease
Volume 11 - 2021 | https://doi.org/10.3389/fcimb.2021.752889
This article is part of the Research Topic The Human Microbiome: A New Frontier in Personalized Cancer Therapy View all 6 articles
Background: Body weight (BW) loss is prevalent in patients with pancreatic cancer (PC). Gut microbiota affects BW and is known to directly shape the host immune responses and antitumor immunity. This pilot study evaluated the link between gut microbiota, metabolic parameters and inflammatory/immune parameters, through the fecal material transplantation (FMT) of PC patients and healthy volunteers into germ-free (GF) mice.
Methods: We transplanted the feces from five PC patients and five age- and gender-matched healthy volunteers into two GF mice each. Mouse BW and energy intake were measured every 1-5 days, oral glucose on day 21, insulin tolerance on day 26, fecal bacterial taxonomic profile by 16S rRNA gene sequencing on day 5, 10, 15 and 30, and gut-associated lymphoid tissue T cells, plasma cytokines and weights of fat and muscle mass at sacrifice (day 34). Results are presented as mean ± SD. The continuous parameters of mice groups were compared by linear univariate regressions, and their bacterial communities by Principal Coordinates Analysis (PCoA), Bray-Curtis similarity and ANCOM test.
Results: Recipients of feces from PC patients and healthy volunteers had similar BW gain and food intake. Visceral fat was lower in recipients of feces from PC patients than from healthy individuals (0.72 ± 0.17 vs. 0.92 ± 0.14 g; coeff -0.19, 95% CI -0.38, -0.02, p=0.035). The other non-metataxonomic parameters did not differ between groups. In PCoA, microbiota from PC patients clustered apart from those of healthy volunteers and the same pattern was observed in transplanted mice. The proportions of Clostridium bolteae, Clostridium scindens, Clostridium_g24_unclassified and Phascolarctobacterium faecium were higher, while those of Alistipes obesi, Lachnospiraceae PAC000196_s and Coriobacteriaceae_unclassified species were lower in PC patients and in mice transplanted with the feces from these patients.
Conclusion: In this pilot study, FMT from PC patients was associated with a decrease in visceral fat as compared to FMT from healthy individuals. Some of the differences in fecal microbiota between PC and control samples are common to humans and mice. Further research is required to confirm that feces contain elements involved in metabolic and immune alterations.
Pancreatic cancer (PC) is the 7th cause of death by cancer worldwide (Arnold et al., 2020), the 3rd in the US in the 2021 estimate (https://cancerstatisticscenter.cancer.org/#!/) and is foreseen to be the second cause of cancer death by 2030 (Rahib et al., 2014). It is often detected late, at a metastatic or locally advanced stage, and is associated with a 5-year survival of around 10%. The most commonly identified risk factors are smoking, chronic pancreatitis, type 2 diabetes, obesity and age.
Human studies suggest that microorganisms colonize the pancreas in pancreatic diseases and in the healthy state (Thomas and Jobin, 2020). This could occur via the pancreatic duct or the portal circulation after translocation from the digestive tract through the gut barrier (Thomas and Jobin, 2020). Indeed, the gut microbiome reflects 25% of the tumor microbiome (Riquelme et al., 2019). A few cross-sectional studies described differences in abundance and composition of fecal or rectal bacteria when comparing PC patients with healthy controls, although no consensus could be established between the studies (Ren et al., 2017; Pushalkar et al., 2018; Half et al., 2019).
Animal studies have shown involvement of gut microbiota in tumor progression. Germ-free (GF) mice or mice treated with antibiotics are less prone to tumor growth and oncogenesis (Schwabe and Jobin, 2013; Thomas et al., 2018). In murine models of PC, fecal Actinobacteria, Deferribacteres and Bifidobacterium increase in abundance with time, and bacterial ablation through oral antibiotics limited the tumor growth (Pushalkar et al., 2018). It was suggested that the gut microbiota triggers an inflammatory response promoting pancreatic cancer initiation (Schwabe and Jobin, 2013). Recently, a translational study evaluated the impact of fecal material transplantation (FMT) from surgically-resected PC patients into antibiotic-treated PC-bearing mice (Riquelme et al., 2019). Mice that received the stool of long-term survivors (> 5 years) showed a slower tumor growth, a higher survival, higher serum levels of interferon-γ and interleukin-2 than mice that had received the feces of short-term survivors, and displayed a distinct gut microbiota community. This anti-tumoral effect was mediated by CD8+ T cells. Taken together, these animal studies suggest that the modulation of gut microbiota, whether through FMT or antibiotics, affects the immune and inflammatory parameters and the pancreatic tumor growth. In addition, it has been shown that gut microbiota influences immune responses to cancer in patients, including response to immune checkpoint inhibitors (Routy et al., 2018; Gopalakrishnan et al., 2018). In pancreatic cancer, it was shown that tumors harbor more microbes than the normal pancreatic tissue (Sethi et al., 2019) and that the tumor microbiome promotes immunosuppression at the tumor microenvironment (Pushalkar et al., 2018). Additionally, the PC microbiome composition was shown to influence antitumor immune responses, allowing identification of tumor microbiome signatures predicting of PC patients' outcome (Riquelme et al., 2019).
Although obesity is a risk factor for developing pancreatic cancer, 60 to 70% of the patients suffer of cachexia at diagnosis of PC, defined as a loss of body weight and muscle mass. Cachexia is mediated by anorexia, insulin resistance, systemic inflammation and likely increased brown adipose tissue (Tsoli and Robertson, 2013), and is associated with a high morbidity and mortality (Muscaritoli et al., 2017; Hendifar et al., 2018). Gut microbiota has been associated with body weight and body composition in animal studies. Germ-free mice suffer from muscle atrophy, which improves after FMT from pathogen-free mice (Lahiri et al., 2019). Translational studies have shown that body composition phenotypes, whether obesity or leanness, are transmissible by FMT from humans to GF mice (Ridaura et al., 2013; Blanton et al., 2016). However, it is unknown whether a FMT from PC patients, prone to cachexia, induces changes in body composition of GF mice.
We hypothesized that the gut microbiota of patients with newly diagnosed PC, naïve of any oncologic treatment, induced alterations of metabolic, inflammatory and immune parameters. If these hypotheses were confirmed, they would pave the way for interventional human studies. In this pilot study, we evaluated whether patients with PC displayed a different microbiota composition compared to healthy controls, and whether FMT from these patients into GF mice affected body weight gain, body composition, as well as intestinal immune and inflammatory parameters.
This translational pilot study examined the microbiota alterations in patients with PC compared to healthy volunteers, and the impact of the FMT coming from these patients into GF mice. The human protocol was approved by the local Ethical Committee and the participants signed an informed consent. The animal experiments were approved by the Swiss Federal and the Geneva Cantonal Authorities for animal experimentation.
The patients were recruited at the University Hospitals of Geneva. We included five patients aged ≥ 18 years, newly diagnosed with pancreatic adenocarcinoma, with no tube feeding nor parenteral nutrition, and without treatment with antibiotics in the previous month. We also included 5 healthy volunteers, matched for gender and age (+/- 5 years) with the included patients with PC, aged ≥ 18 years, with a body mass index below 30 kg/m2, no chronic diseases and no treatment with antibiotics in the previous month. The exclusion criteria for all donors were: age < 18 years, cognitive impairment, ongoing oncologic treatment, tube feeding or parenteral nutrition and antibiotic therapy.
For the patients with PC, we collected the following data at the time of inclusion: cancer stage (Kakar et al., 2016), date of diagnosis and results of routine blood samples (complete blood count, urea, creatinine, Na, K, aspartate aminotransferase, alanine aminotransferase, alkaline phosphatase, ɣ-glutamyl-transferase, albumin, C-reactive protein). For patients and volunteers, we reported drugs, co-morbidities and body weight loss over the last 6 months. We measured actual weight with an electronic scale (Seca, Hamburg, Germany) and height with a height gauge. Waist-to-hip ratio was determined with the patient or volunteer in a standing position, legs closed and arms alongside the body, at the level of the umbilicus of the waist and at the largest level of the hip. Body composition was assessed by 50 kHz tetrapolar bioelectrical impedance analysis (Nutriguard®, Data Input GmbH), with the patient or volunteer lying in a supine position, arms and legs abducted from the trunk at about 30 degrees. The measured resistance and reactance were used to calculate fat-free mass by the Geneva formula, validated against dual-energy X-ray absorptiometry in the healthy population living in the Geneva area (Kyle et al., 2001). Fat mass was obtained by subtraction of fat-free mass from body weight. Appetite was evaluated by a visual analogue scale, ranging from 0 (no appetite) to 100 mm (very good appetite) (Blauwhoff-Buskermolen et al., 2016).
Stools were collected within 7 days of the routine consultation during which the clinical assessments were performed. They were collected in a Commode Specimen Container (Covidien, Medline, Arnhem, The Netherlands) placed over the toilet seat opening. The participant put at least a nut-sized section of fecal material immediately into Sarstedt Feces Tube 76x20mm, sealed it in an AnaeroPouch-Bag (Thermo Scientific) with an AnaeroPouch-Anaero gas generator (Thermo Scientific), and the study investigators transported it within 4 h to the laboratory for further processing. About 0.5 mL stool sample was added to 4.5 mL of PBS-CR (phosphate buffer saline containing 0.1% cysteine and 0.3% riboflavin) and homogenized by vortexing during 3 min. To settle large insoluble particles, the sample tubes were allowed to stand for 3 minutes. The supernatant (900 µL) was then mixed with an equal volume of PBS-CRGS (PBS-CR with 30% glycerol and 10% sucrose). The suspension was stored in cryovial 100 µL-aliquots at –80°C until transplantation.
The animal study was performed at the Medical School of the University of Geneva. The fecal suspension of each of the five patients with PC and the five healthy volunteers was transplanted into two GF mice (males, C57BL/6J, 7–8 weeks old, obtained from the University of Bern) by oral gavage on days 0, 2 and 6 (200 µL each day). The two mice that received the feces from the same donor were housed in the same cage. All mice were fed with a standard chow diet (SAFE 150, Safediets, Augy, France) and kept in specific pathogen free (SPF) conditions. The initial colonization of the GF mice with the respective microbiota was done immediately upon arrival, and all transplanted mice were kept in sterilized cages and handled in sterilized hoods using sterile single-use sterile protective gear and coating. Food intake was measured every 3–5 days, by cage.
Body weight was measured every 1 to 5 days. The oral glucose tolerance test (oGTT) was determined on day 21, after a 6-hour fast, by oral gavage of a glucose bolus (2 g/kg body weight). The insulin tolerance test (ITT) was performed on day 26 after a 5-hour fast, with an intraperitoneal injection of 0.75 U/kg insulin (ref. I9278, Sigma-Aldrich). Feces were collected on days 5, 10, 15 and 30 after the first FMT and frozen at –80°C until analysis. At the end of the experiment (day 34), we measured the weight of the subcutaneous, visceral and brown adipose tissue and the skeletal muscle mass. On day 34, the following serum cytokines/chemokines were measured using the 23-plex mouse cytokine & chemokine assay (Bio-Rad) in plasma: interleukin (IL)-1β, IL-2, IL-3, IL-4, IL-5, IL-6, IL-9, IL-10, IL-12 (p40), IL-12(p70), IL-13, IL-17A, eotaxin, G-CSF, GM-CSF, interferon-γ, KC, MCP-1, MIP1α, MIP-1β, RANTES and tumor necrosis factor-α. Finally, numbers of myeloid cells, CD4+ and CD8+ lymphocytes and T regulatory (Treg) cells were measured in the Peyer’s patches, mesenteric lymph nodes and blood by flow cytometry after surface staining using CD45, CD3, CD4, CD8, CD11b, and intracellular staining using FoxP3 and RORγt using the manufacturer’s instructions (Biolegend). Lymphocytes in the Peyer’s patches and mesenteric lymph nodes were isolated as previously described (Genton et al., 2004).
DNA was extracted from 20–30 mg of mouse stools or 100 μL of human stool suspension using ZymoBIOMICS DNA Miniprep Kit (Zymo Research). Purified DNA was quantified using the Qubit dsDNA BR Assay Kit (Thermo Fisher Scientific) and stored at –20°C.
The V3–4 region of the bacterial 16S rRNA genes was amplified using 1 ng of extracted DNA as described previously (Somm et al., 2021). The construction of the sequencing library using the MetaFast protocol, the Illumina MiSeq 2×300 sequencing with MiSeq Reagent Kit v3 and initial sequence processing (demultiplexing and removal of adapter and primer sequences) were carried out at Fasteris (Plan-les-Ouates, Switzerland). For the sequence analysis, paired reads were quality filtered and joined using PEAR v0.9.11 (-m 470, -n 390, -t 150, -v 10, -q 33, -p 0.0001, -u 0) (Zhang et al., 2014). Merged sequence reads were clustered into zero-radius operational taxonomic units (zOTUs) using UNOISE3 from the USEARCH v10.0.240 pipeline (Edgar, 2013; Edgar, 2016). zOTUs were classified using EzBioCloud 16S database (Yoon et al., 2017) via MOTHUR (Schloss et al., 2009) command classify.seqs (method=wang, cutoff=80). We removed the reads corresponding to zOTUs that had < 90% identity to the reference EzBioCloud 16S database as revealed by USEARCH (-id 0.90, -query_cov 0.99) (Edgar, 2010). Sequencing data were submitted to the European Nucleotide Archive (ENA; www.ebi.ac.uk/ena; study number: PRJEB43581).
Results are presented as mean ± SD. Normality of distributions was checked with Shapiro-Wilk tests. As plasma cytokines were not normally distributed, they were normalized using the Stata’s “ladder” command, in order to be used in the mixed regression models. Univariate linear regressions were performed to compare non-metataxonomic data between the animal recipients of feces from PC patients (10 mice) and volunteers (10 mice, reference group). They took into account that 2 mice were transplanted with the feces of the same human donor. For each regression model, we calculated the regression coefficients, standard errors and 95% confidence intervals (CI). Statistics were performed with STATA release 16.1, and significance was set at p<0.05.
Shannon diversity was calculated after rarefaction to 20’900 reads per sample using the rrarefy function of the R vegan package. Principal coordinates analysis (PCoA) of the Bray-Curtis similarity matrix were used to visualize microbiota similarities based on the square-root transformed zOTU and species abundances. Since each human feces was transplanted into two mice (denoted Group A and B), microbiota analyses were, when appropriate, performed twice. To compare the relative abundance of bacterial genera between human donors and corresponding mouse recipients, we used the analysis of composition of microbiomes (ANCOM) v2.1 (Mandal et al., 2015) with the following settings: adjusted = F, repeated = F, multcorr = 2 (“less stringent” multiple comparison correction), sig = 0.05, prev.cut = 0.75 (features not observed in ≥ 75% samples were omitted) and paired.test_ancom = paired. The results that passed the 0.6 threshold were considered significant. Wilcoxon rank sum test was used to compare Bray-Curtis similarities within and between groups defined with respect to the cancer status of human donors.
As this was a pilot study, no sample size calculation was performed. However, a sample size of 6 to 10 mice per group was used in a study which showed statistically significant differences in gut microbiota composition in mouse models of cancer (leukemia) as compared to control mice (Bindels et al., 2012).
Characteristics of human donors are shown in Table 1. Mouse recipients of feces from PC patients tended to have a lower, albeit not significant, BW gain over the whole study than the recipients of healthy volunteer’s feces, (2.4 g vs. 3.3 g; coeff −0.86, 95% CI −1.83 to 0.83, p=0.069), while total food intake was similar between groups (49.1 g vs. 50.3 g, coeff −1.21, 95% CI −5.74 to 3.33, p=0.561). The evolution of BW and energy intake is shown in Figure 1. oGTT and ITT were similar in both groups (Supplemental Figure 1). At sacrifice, visceral fat was lower in recipients of feces from PC patients (Table 2) but the levels of subcutaneous and brown adipose tissue and skeletal muscle mass were similar between groups. There were no significant differences in plasma cytokines and chemokines, myeloid, T or Treg cells and Peyer’s patch numbers were similar between groups (Supplemental Tables 1, 2).
Figure 1 Evolution of body weight (A) and food intake (B) in the mouse recipients of feces from pancreatic cancer (PC) patients as mean – SD (gray line) (n = 10) and volunteers as mean + SD (black line) (n = 10). FMT were performed on day 0, 2 and 6. Body weight at each time point was never significantly different between both groups by linear regression models (all p > 0.5).
Six genera had a relative abundance > 10% in at least one (of 10) human stool samples. Bacteroides, Blautia and Prevotella surpassed the 10%-threshold in 8, 4 and 2 samples, respectively, while Alistipes, Streptococcus and Succinivibrio each surpassed it in one sample (Supplemental Table 3). Principal coordinates analysis (PCoA) of the Bray-Curtis similarity matrix showed a good separation of PC patients’ and volunteers’ microbiota in the first two PCos (Figure 2). Samples from PC patients tended to be displaced rightwards relative to those from controls. Intra-group microbiota similarities, based on relative abundance of species, were more pronounced in controls (median 56.76; interquartile range: 48.80–60.57) than in PC patients (median: 47.53; interquartile range: 44.39–49.69), reaching statistical significance (p=0.01 by Wilcoxon rank sum test) (Figure 3). Comparison of microbiota of PC patients vs. volunteers revealed several differentially abundant taxa (Supplemental Table 4). Of note, Escherichia coli and Streptococcus salivarius were remarkably more abundant (> 147-fold and > 31-fold, respectively, both in median and mean values) in the fecal microbiota of PC patients than in controls. These differences were reflected at the higher levels of the taxonomic hierarchy up to the phylum (Escherichia-Enterobacteriaceae-Enterobacteriales-Gammaproteobacteria-Proteobacteria) and class level (Streptococcus-Lactobacilli-Lactobacillales-Bacilli).
Figure 2 PCoA of human (n = 10) and mouse fecal microbiota (n = 20). The samples from a pair of mice who were transplanted from the same human donor, and collected at four time-points are surrounded by an oval (4 samples per mouse, 8 mouse samples for one human donor). The numbers correspond to human donors. Arrows link the human donor sample to fecal samples from corresponding transplanted mice. The analysis was based on the relative abundance of species.
Figure 3 Boxplots showing Bray-Curtis similarities of fecal microbial communities of mice on day 5, 10, 15 and 30 after transplantation. Since each human feces were transplanted into two mice denoted Group (A, B), microbiota analyses were performed twice.
In the PCoA plot including the first two PCos (Figure 2), mice samples from patient recipients were displaced rightwards relative to those from human donors. Nevertheless, the location of mouse samples on the PCoA correlated with the location of corresponding human donors. Overall, mouse microbiota, compared to that of human donors, was significantly depleted in Lachnospira, Roseburia, Clostridium and Coprococcus_g2, while being enriched in Anaerofilum (Supplemental Table 3). As shown in Supplemental Figure 2, 65 to 99% of recipient mice microbiota was of human donor origin. In contrast, the feces of the human donors shared a lower percentage of microbiota with their corresponding mouse recipients. Shannon diversity index was calculated for the human and mice samples. The diversity index was higher in human samples (cancer patients: 3.59–4.02; controls: 3.43–4.30) than in mice sample (recipients of cancer patients: 2.87–3.79; recipients of volunteers: 3.14–3.92).
Mouse fecal microbiota were clustered according to the cancer/healthy individual status of their respective donors (Figure 2). Samples from mouse recipients of PC patients were displaced rightwards relative to those from mouse recipients of volunteers in the PCoA plot.
These changes were associated with an increased or decreased relative abundance of 43 taxa from phylum to species level, and 260 zOTUs (Supplemental Table 5). Importantly, all bacterial species found to be differentially abundant between PC patients and healthy controls as well as between corresponding transplanted mice groups had the same direction of change: the proportions of Clostridium bolteae, Clostridium scindens, Clostridium_g24_unclassified and Phascolarctobacterium faecium were higher while those of Alistipes obesi, Lachnospiraceae PAC000196_s and Coriobacteriaceae_unclassified species were lower in PC patients and in mice transplanted from these patients. Similarly, of 83 zOTUs differentially abundant between the PC and control groups in both humans and mice, 81 had consisted directions of change (increase or decrease). These zOTUs belong to the species cited above and to 12 additional ones (Supplemental Table 5).
The similarity of bacterial communities tended to be lower, although not significantly, among mice transplanted from PC donors than among those whose donors were healthy volunteers (Figure 3), which was in line with the trend observed for human samples. The similarity between fecal communities of mice transplanted from PC patients tended to increase over time and reach a level observed in the control mice group.
This pilot study showed that: 1) the fecal microbiota of PC patients was different from healthy controls, 2) mouse recipients of feces from PC patients had lower visceral fat than the recipients of volunteer’s feces, but similar immune/inflammatory parameters, and 3) the microbiota of transplanted mice partially reflected the taxonomic composition of bacterial communities of their corresponding human donor.
Some differences of fecal microbiota between patients with PC and healthy controls are in line with previous studies (Ren et al., 2017; Pushalkar et al., 2018; Half et al., 2019). We observed an increased relative abundance of Streptococcus (notably due to S. salivarius) and Escherichia (specifically E. coli) in PC patients as compared to healthy volunteers. Pushalkar et al. (2018) also reported a higher proportion of Streptococcus in the rectal microbiota of PC patients with biliary obstruction and with higher cancer stages (stage 2 vs. stage 1). In addition, PC was associated with an increased proportion of Escherichia, Enterobacteriaceae and Proteobacteria, as it was the case in our study. However, Ren et al. found a higher fecal abundance of Bacteroidetes and decreased abundance of Firmicutes and Proteobacteria (although two Enterobacteriaceae members, Klebsiella and Enterobacter, increased) in PC patients (Ren et al., 2017). Both studies included patients naïve of oncologic treatments, as our study. Differences may be related to the characteristics of the patients (for instance non-oncologic treatments, co-morbidities or genetics), to the diet, to the location of the microbiota sample (rectal vs. fecal) or to the metataxonomic analyses.
In our study, the visceral fat, but not the muscle mass, was lower in the mice transplanted with the feces of PC patients as compared to those transplanted with the feces of healthy volunteers. A low visceral fat, which precedes muscle loss, was described in patients with hepatocellular carcinoma and mouse models of this type of cancer (Erdem et al., 2019). The mechanism of visceral fat loss in cancer involves lipolysis by stimulation of the hormone-sensitive lipase and adipose tissue triglyceride lipase which is mediated by cytokines and other lipolytic factors as for instance zinc alpha2-glycoprotein (Bing, 2011; Das et al., 2011). No study has been performed on the link between fat wasting and microbiota in cancer. By analogy with muscle wasting, we could hypothesize that microbiota may stimulate fat wasting by mechanisms involving pro-inflammatory cytokines, pathogen-associated molecular patterns, as lipopolysaccharides, or microbial metabolites as short-chain fatty acids or bile acids (Bindels and Delzenne, 2013).
In our study, we found that mice transplanted with the feces of PC patients had higher levels of bacteria belonging to the Clostridium genera. High levels of Clostridia have been associated with the leanness phenotype in mice, as reported by Pedersen et al. (Wang and Hooper, 2019; Petersen et al., 2019). They showed that specialized lymphoid T cells (T follicular helper) stimulated the production of IgA by lymphoid B cells which cross the epithelial barrier. In the gut lumen, these IgA could inappropriately target some Clostridia species and promote their proliferation, but antagonize the proliferation of beneficial Clostridia. As a result, the expression of genes involved in lipid synthesis and absorption decreased, which ultimately lead to lower weight and fat mass (Petersen et al., 2019). The authors did not find any impact of T follicular helper cells on cecal short-chain fatty acid, suggesting this effect to occur independently of the short-chain fatty acid production. The impact of other bacteria on body composition has been shown in animal studies. Indeed, certain probiotics decrease the fat mass in mouse models of diet-induced obesity (Everard et al., 2014; Lee et al., 2018). Conversely, some bacteria have been involved in cancer cachexia, which is characterized by muscle wasting with or without fat mass loss. In models of colon carcinoma, Klebsiella oxytoca was a major stimulant of cancer cachexia (Potgens et al., 2018), but Lactobacillus reuteri was beneficial for its prevention (Varian et al., 2016).
The question often arises in what proportion the gut microbiota of human donors engrafts the murine intestine. In our study, the mouse microbiome, when considering zOTUs with a relative abundance > 0.1%, was in large part (between 65 and 99%) of human origin. The fact that it did not reach 100% could be due to zOTUs which were present in donors below the detection levels and/or to contamination, for instance through food, as the mice were raised in SPF conditions. Other studies evaluating the impact of a single human transplantation described a recovery of 85% of human genus-level taxa in GF mice (Turnbaugh et al., 2009), whereas 59% of the fecal microbiota of antibiotic-treated mice were attributable to the donor bacterial community (Staley et al., 2017), several weeks after transplantation. Riquelme et al. focused on PC and transplanted antibiotic-treated mice with fecal samples from patients with PC and healthy controls. The FMT was performed thrice a week for 2 weeks before an orthotopic pancreatic tumor implantation and 1x/week for 5 weeks thereafter. They found that about 40% of the murine fecal microbiome originated from the human donor microbiome, 5 weeks after tumor implantation (Riquelme et al., 2019). The variable FMT protocols and different mouse models may explain partly the different engraftment. A recent study compared the impact of an FMT once a week, with a single FMT or an FMT twice a week, in conventional mice that had underwent bowel cleansing over a duration of 4 weeks. They suggested that the FMT once a week was the best compromise as it led to the highest engraftment of Bacteroidales and Faecalibacterium (Wrzosek et al., 2018). In our study, mice had a higher relative abundance of genera Lachnospira, Roseburia, Clostridium and Coprococcus_g2, and a lower proportion of Anaerofilum than humans.
Although we hypothesized that inflammatory and immune parameters would be differentially influenced by the source of the gut microbiota used for the FMT (i.e. of PC patients vs. healthy donors), we were not able to show that in the present study. Heterogeneity in the microbiome composition between individuals might contribute to hiding differences between the two groups. In addition, the use of GF mice, in which lack of microbiota results in deficient local and systemic immune systems (Round and Mazmanian, 2009), plays a role. Finally, it has been shown that there is a host-specific immune-microbiome interaction (Chung et al., 2012) that might result in a lower ability of human bacteria to influence mouse immune responses.
The strengths of this study are its translational and innovative design, using the feces of PC patients naïve of oncologic treatments and healthy volunteers. The study is limited by the small number of donors, with, by definition, heterogeneous gut microbiota. Despite this, we could demonstrate differences in the gut microbiota in PC patients and healthy volunteers and their corresponding mouse recipients. We found only significant differences in visceral fat mass between mouse recipients of PC patients vs. healthy volunteers. Thus, either the gut microbiota truly does not affect the other measured parameters in this situation, or the feces of the donors and their engraftments in mice were too heterogeneous, or the length of follow-up after FMT was insufficient to induce significant changes. Future studies should observe the gut microbiota in PC patients during the progression of their disease and identify the gut microbiota composition associated with the worst changes in metabolic, intestinal and inflammatory parameters and survival. This gut microbiota composition could then be used for FMT into mice in order to prove causality and potentially pave the way for new interventional studies.
FMT from PC patients into GF mice decreased visceral fat. The resemblance between the microbiota of transplanted mice and their corresponding human donors was partial, but some differences between PC patients and volunteers were common to humans and mice. Further research is required to confirm that feces contain elements involved in metabolic and immune alterations.
The datasets presented in this study can be found in online repositories. The names of the repository/repositories and accession number(s) can be found below: ENA, PRJEB43581.
The studies involving human participants were reviewed and approved by Ethical Committee of Geneva. The patients/participants provided their written informed consent to participate in this study. The animal study was reviewed and approved by Swiss Federal and the Geneva Cantonal Authorities for animal experimentation study.
Conceptualization, LG and JS. Methodology, LG, FH, AM, MT, and JS. Formal analysis, LG, VL, OS, MS, VD, NG, FH, and JS. Investigation, LG, VL, OS, MS, SD, TK, VD, NG, JM, FH, MT, and JS. Resources, LG, AM, MT, and JS. Data curation, VL, OS, MS, SD, and LG. Writing—original draft preparation, LG. Writing—review and editing, VL, OS, MS, SD, TK, VD, NG, JM, AM, FH, MT, and JS. Visualization, LG. Supervision, LG, MT, and JS. Project administration, LG and MT. Funding acquisition, LG. All authors contributed to the article and approved the submitted version.
This research was funded by the « Fondation pour l’innovation sur le cancer et la biologie ».
The authors declare that the research was conducted in the absence of any commercial or financial relationships that could be construed as a potential conflict of interest.
All claims expressed in this article are solely those of the authors and do not necessarily represent those of their affiliated organizations, or those of the publisher, the editors and the reviewers. Any product that may be evaluated in this article, or claim that may be made by its manufacturer, is not guaranteed or endorsed by the publisher.
We thank the nurses of the oncology division for the blood sampling, Mrs Myriam Girard for preparing fecal samples for analyses and transplantation, M. Yves Dupertuis and Mrs Dorothée Rigo for their help during mouse handling.
The Supplementary Material for this article can be found online at: https://www.frontiersin.org/articles/10.3389/fcimb.2021.752889/full#supplementary-material
Arnold, M., Abnet, C. C., Neale, R. E., Vignat, J., Giovannucci, E. L., McGlynn, K. A., et al. (2020). Global Burden of 5 Major Types Of Gastrointestinal Cancer. Gastroenterology 159 (1), 335–349. doi: 10.1053/j.gastro.2020.02.068
Bindels, L. B., Beck, R., Schakman, O., Martin, J. C., De Backer, F., Sohet, F. M., et al. (2012). Restoring Specific Lactobacilli Levels Decreases Inflammation and Muscle Atrophy Markers in an Acute Leukemia Mouse Model. PloS One 7 (6), 1–10. doi: 10.1371/journal.pone.0037971
Bindels, L. B., Delzenne, N. M. (2013). Muscle Wasting: The Gut Microbiota as a New Therapeutic Target? Int. J. Biochem. Cell Biol. 45 (10), 2186–2190. doi: 10.1016/j.biocel.2013.06.021
Bing, C. (2011). Lipid Mobilization in Cachexia: Mechanisms and Mediators. Curr. Opin. Supportive Palliative Care 5 (4), 356–360. doi: 10.1097/SPC.0b013e32834bde0e
Blanton, L. V., Charbonneau, M. R., Salih, T., Barratt, M. J., Venkatesh, S., Ilkaveya, O., et al. (2016). Gut Bacteria That Prevent Growth Impairments Transmitted by Microbiota From Malnourished Children. Science 351 (6275), aad3311–1–aad3311–7. doi: 10.1126/science.aad3311
Blauwhoff-Buskermolen, S., Ruijgrok, C., Ostelo, R. W., de Vet, H. C. W., Verheul, H. M. W., de van der Schueren, M. A. E., et al. (2016). The Assessment of Anorexia in Patients With Cancer: Cut-Off Values for the FAACT-A/CS and the VAS for Appetite. Support Care Cancer 24 (2), 661–666. doi: 10.1007/s00520-015-2826-2
Chung, H., Pamp, S. J., Hill, J. A., Surana, N. K., Edelman, S. M., Troy, E. B., et al. (2012). Gut Immune Maturation Depends on Colonization With a Host-Specific Microbiota. Cell 149 (7), 1578–1593. doi: 10.1016/j.cell.2012.04.037
Das, S. K., Eder, S., Schauer, S., Diwoky, C., Temmel, H., Guertl, B., et al. (2011). Adipose Triglyceride Lipase Contributes to Cancer-Associated Cachexia. Science 333 (6039), 233–238. doi: 10.1126/science.1198973
Edgar, R. C. (2010). Search and Clustering Orders of Magnitude Faster Than BLAST. Bioinformatics 26 (19), 2460–2461. doi: 10.1093/bioinformatics/btq461
Edgar, R. C. (2013). UPARSE: Highly Accurate OTU Sequences From Microbial Amplicon Reads. Nat. Methods 10 (10), 996–998. doi: 10.1038/nmeth.2604
Edgar, R. C. (2016). UNOISE2: Improved Error-Correction for Illumino 16S and ITS Amplicon Sequencing. bioRxiv. doi: 10.1101/081257
Erdem, M., Mockel, D., Jumpertz, S., John, C., Fragoulis, A., Rudolph, I., et al. (2019). Macrophages Protect Against Loss of Adipose Tissue During Cancer Cachexia. J. Cachexia Sarcopenia Muscle 10 (5), 1128–1142. doi: 10.1002/jcsm.12450
Everard, A., Matamoros, S., Geurts, L., Delzenne, N. M., Cani, P. D. (2014). Saccharomyces Boulardii Administration Changes Gut Microbiota and Reduces Hepatic Steatosis, Low-Grade Inflammation, and Fat Mass in Obese and Type 2 Diabetic Db/Db Mice. mBio 5 (3), e01011–e01014. doi: 10.1128/mBio.01011-14
Genton, L., Reese, S. R., Ikeda, S., Le Tho, C., Kudsk, K. A. (2004). The C-Terminal Heptapeptide of Bombesin Reduces the Deleterious Effect of Total Parenteral Nutrition (TPN) on Gut-Associated Lymphoid Tissue (GALT) Mass But Not Intestinal Immunoglobulin A In Vivo. JPEN J. Parenter. Enteral Nutr. 28 (6), 431–434. doi: 10.1177/0148607104028006431
Gopalakrishnan, V., Spencer, C. N., Nezi, L., Reuben, A., Andrews, M. C., Karpinets, T. V., et al. (2018). Gut Microbiome Modulates Response to Anti-PD-1 Immunotherapy in Melanoma Patients. Science 359, 97–193. doi: 10.1126/science.aan4236
Half, E., Keren, N., Reshef, L., Dorfman, T., Lachter, I., Kluger, Y., et al. (2019). Fecal Microbiome Signatures of Pancreatic Cancer Patients. Sci. Rep. 9 (1), 16801. doi: 10.1038/s41598-019-53041-4
Hendifar, A. E., Chang, J. I., Huang, B. Z., Tuli, R., Wu, B. U. (2018). Cachexia, and Not Obesity, Prior to Pancreatic Cancer Diagnosis Worsens Survival and is Negated by Chemotherapy. J. Gastrointestinal Oncol. 9 (1), 17–23. doi: 10.21037/jgo.2017.11.10
Kakar, S., Pawlik, T. M., Allen, P. J., Vauthey, J. N. (2016). “Exocrine Pancreas. Pancreatic Adenocarcinoma,” in AJCC Cancer Staging Manual, 8th ed. Ed. Amin, N. B. (New York, NY: Springer-Verlag).
Kyle, U. G., Genton, L., Karsegard, L., Slosman, D. O., Pichard, C. (2001). Single Prediction Equation for Bioelectrical Impedance Analysis in Adults Aged 20–94 Years. Nutrition 17 (3), 248–253. doi: 10.1016/S0899-9007(00)00553-0
Lahiri, S., Kim, H., Garcia-Perez, I., Reza, M. M., Martin, K. A., Kundu, P., et al. (2019). The Gut Microbiota Influences Skeletal Muscle Mass and Function in Mice. Sci. Transl. Med. 11, eaan5562. doi: 10.1126/scitranslmed.aan5662
Lee, E., Jung, S. R., Lee, S. Y., Lee, N. K., Paik, H. D., Lim, S. I. (2018). Lactobacillus Plantarum Strain Ln4 Attenuates Diet-Induced Obesity, Insulin Resistance, and Changes in Hepatic mRNA Levels Associated With Glucose and Lipid Metabolism. Nutrients 10 (5), 643. doi: 10.3390/nu10050643
Mandal, S., Van Treuren, W., White, R. A., Eggesbo, M., Knight, R., Peddada, S. D. (2015). Analysis of Composition of Microbiomes: A Novel Method for Studying Microbial Composition. Microb. Ecol. Health Dis. 26, 27663. doi: 10.3402/mehd.v26.27663
Muscaritoli, M., Lucia, S., Farcomeni, A., Lorusso, V., Saracino, V., Barone, C., et al. (2017). Prevalence of Malnutrition in Patients at First Medical Oncology Visit: The PreMiO Study. Oncotarget 8 (45), 79884–79896. doi: 10.18632/oncotarget.20168
Petersen, C., Bell, R., Klag, K. A., Lee, S. H., Soto, R., Ghazaryan, A., et al. (2019). T Cell-Mediated Regulation of the Microbiota Protects Against Obesity. Science 365, eaat9351. doi: 10.1126/science.aat9351
Potgens, S. A., Brossel, H., Sboarina, M., Catry, E., Cani, P. D., Neyrinck, A. M., et al. (2018). Klebsiella Oxytoca Expands in Cancer Cachexia and Acts as a Gut Pathobiont Contributing to Intestinal Dysfunction. Sci. Rep. 8 (1), 12321. doi: 10.1038/s41598-018-30569-5
Pushalkar, S., Hundeyin, M., Daley, D., Zambirinis, C. P., Kurz, E., Mishra, A., et al. (2018). The Pancreatic Cancer Microbiome Promotes Oncogenesis by Induction of Innate and Adaptive Immune Suppression. Cancer Discovery 8 (4), 403–416. doi: 10.1158/2159-8290.CD-17-1134
Rahib, L., Smith, B. D., Aizenberg, R., Rosenzweig, A. B., Fleshman, J. M., Matrisian, L. M. (2014). Projecting Cancer Incidence and Deaths to 2030: The Unexpected Burden of Thyroid, Liver, and Pancreas Cancers in the United States. Cancer Res. 74 (11), 2913–2921. doi: 10.1158/0008-5472.CAN-14-0155
Ren, Z., Jiang, J., Xie, H., Li, A., Lu, H., Xu, S., et al. (2017). Gut Microbial Profile Analysis by MiSeq Sequencing of Pancreatic Carcinoma Patients in China. Oncotarget 8 (56), 95176–95191. doi: 10.18632/oncotarget.18820
Ridaura, V. K., Faith, J. J., Rey, F. E., Cheng, J., Duncan, A. E., Kau, A. L., et al. (2013). Gut Microbiota From Twins Discordant for Obesity Modulate Metabolism in Mice. Science 341 (6150), 1241214. doi: 10.1126/science.1241214
Riquelme, E., Zhang, Y., Zhang, L., Montiel, M., Zoltan, M., Dong, W., et al. (2019). Tumor Microbiome Diversity and Composition Influence Pancreatic Cancer Outcomes. Cell 178 (4), 795–806 e12. doi: 10.1016/j.cell.2019.07.008
Round, J. L., Mazmanian, S. K. (2009). The Gut Microbiota Shapes Intestinal Immune Responses During Health and Disease. Nat. Rev. Immunol. 9 (5), 313–323. doi: 10.1038/nri2515
Routy, B., Le Chatelier, E., Derosa, L., Duong, C. P., Alou, M. T., Daillere, R., et al. (2018). Gut Microbiome Influences Efficacy of PD-1-Based Immunotherapy Against Epithelial Tumors. Science 359, 91–97. doi: 10.1126/science.aan3706
Schloss, P. D., Westcott, S. L., Ryabin, T., Hall, J. R., Hartmann, M., Hollister, E. B., et al. (2009). Introducing Mothur: Open-Source, Platform-Independent, Community-Supported Software for Describing and Comparing Microbial Communities. Appl. Environ. Microbiol. 75 (23), 7537–7541. doi: 10.1128/AEM.01541-09
Schwabe, R. F., Jobin, C. (2013). The Microbiome and Cancer. Nat. Rev. Cancer 13 (11), 800–812. doi: 10.1038/nrc3610
Sethi, V., Vitiello, G. A., Saxena, D., Miller, G., Dudeja, V. (2019). The Role of the Microbiome in Immunologic Development and Its Implication For Pancreatic Cancer Immunotherapy. Gastroenterology 156 (7), 2097–2115.e2. doi: 10.1053/j.gastro.2018.12.045
Somm, E., Montandon, S. A., Loizides-Mangold, U., Gaia, N., Lazarevic, V., De Vito, C., et al. (2021). The GLP-1R Agonist Liraglutide Limits Hepatic Lipotoxicity and Inflammatory Response in Mice Fed a Methionine-Choline Deficient Diet. Transl. Res. 227, 75–88. doi: 10.1016/j.trsl.2020.07.008
Staley, C., Kaiser, T., Beura, L. K., Hamilton, M. J., Weingarden, A. R., Bobr, A., et al. (2017). Stable Engraftment of Human Microbiota Into Mice With a Single Oral Gavage Following Antibiotic Conditioning. Microbiome 5 (1), 87. doi: 10.1186/s40168-017-0306-2
Thomas, R. M., Gharaibeh, R. Z., Gauthier, J., Beveridge, M., Pope, J. L., Guijarro, M. V., et al. (2018). Intestinal Microbiota Enhances Pancreatic Carcinogenesis in Preclinical Models. Carcinogenesis 39 (8), 1068–1078. doi: 10.1093/carcin/bgy073
Thomas, R. M., Jobin, C. (2020). Microbiota in Pancreatic Health and Disease: The Next Frontier in Microbiome Research. Nat. Rev. Gastroenterol. Hepatol. 17 (1), 53–64. doi: 10.1038/s41575-019-0242-7
Tsoli, M., Robertson, G. (2013). Cancer Cachexia: Malignant Inflammation, Tumorkines, and Metabolic Mayhem. Trends Endocrinol. Metabolism: TEM 24 (4), 174–183. doi: 10.1016/j.tem.2012.10.006
Turnbaugh, P. J., Ridaura, V. K., Faith, J. J., Rey, F. E., Knight, R., Gordon, J. I. (2009). The Effect of Diet on the Human Gut Microbiome: A Metagenomic Analysis in Humanized Gnotobiotic Mice. Sci. Transl. Med. 1 (6), 6ra14.
Varian, B. J., Gourishetti, S., Poutahidis, T., Lakritz, J. R., Levkovich, T., Kwok, C., et al. (2016). Beneficial Bacteria Inhibit Cachexia. Oncotarget 7 (11), 11803–11816. doi: 10.18632/oncotarget.7730
Wang, Y., Hooper, L. V. (2019). Immune Control of the Microbiota Prevents Obesity. Science 365 (6451), 316–317. doi: 10.1126/science.aay2057
Wrzosek, L., Ciocan, D., Borentain, P., Spatz, M., Puchois, V., Hugot, C., et al. (2018). Transplantation of Human Microbiota Into Conventional Mice Durably Reshapes the Gut Microbiota. Sci. Rep. 8 (1), 6854. doi: 10.1038/s41598-018-25300-3
Yoon, S. H., Ha, S. M., Kwon, S., Lim, J., Kim, Y., Seo, H., et al. (2017). Introducing EzBioCloud: A Taxonomically United Database of 16S rRNA Gene Sequences and Whole-Genome Assemblies. Int. J. Syst. Evol. Microbiol. 67 (5), 1613–1617. doi: 10.1099/ijsem.0.001755
Keywords: microbiota, visceral fat, muscle, weight, fecal material transplantation, pancreatic cancer, mice
Citation: Genton L, Lazarevic V, Stojanovic O, Spiljar M, Djaafar S, Koessler T, Dutoit V, Gaïa N, Mareschal J, Macpherson AJ, Herrmann F, Trajkovski M and Schrenzel J (2021) Metataxonomic and Metabolic Impact of Fecal Microbiota Transplantation From Patients With Pancreatic Cancer Into Germ-Free Mice: A Pilot Study. Front. Cell. Infect. Microbiol. 11:752889. doi: 10.3389/fcimb.2021.752889
Received: 03 August 2021; Accepted: 22 September 2021;
Published: 19 October 2021.
Edited by:
Edwin Ramos Manuel, Beckman Research Institute, City of Hope, United StatesReviewed by:
Valeriy Poroyko, Covance, United StatesCopyright © 2021 Genton, Lazarevic, Stojanovic, Spiljar, Djaafar, Koessler, Dutoit, Gaïa, Mareschal, Macpherson, Herrmann, Trajkovski and Schrenzel. This is an open-access article distributed under the terms of the Creative Commons Attribution License (CC BY). The use, distribution or reproduction in other forums is permitted, provided the original author(s) and the copyright owner(s) are credited and that the original publication in this journal is cited, in accordance with accepted academic practice. No use, distribution or reproduction is permitted which does not comply with these terms.
*Correspondence: Laurence Genton, bGF1cmVuY2UuZ2VudG9uQGhjdWdlLmNo
†These authors share second authorship
Disclaimer: All claims expressed in this article are solely those of the authors and do not necessarily represent those of their affiliated organizations, or those of the publisher, the editors and the reviewers. Any product that may be evaluated in this article or claim that may be made by its manufacturer is not guaranteed or endorsed by the publisher.
Research integrity at Frontiers
Learn more about the work of our research integrity team to safeguard the quality of each article we publish.