- 1National Engineering Research Center for Non-Food Biorefinery, Guangxi Academy of Sciences, Nanning, China
- 2College of Life Science and Technology, Guangxi University, Nanning, China
- 3Department of Microbiology, University of Nigeria, Nsukka, Nigeria
- 4Department of Microbiology, University of Jos, Jos, Nigeria
- 5State Key Laboratory of Non-Food Biomass and Enzyme Technology, Guangxi Academy of Sciences, Nanning, China
The threat burden from pathogenic fungi is universal and increasing with alarming high mortality and morbidity rates from invasive fungal infections. Understanding the virulence factors of these fungi, screening effective antifungal agents and exploring appropriate treatment approaches in in vivo modeling organisms are vital research projects for controlling mycoses. Caenorhabditis elegans has been proven to be a valuable tool in studies of most clinically relevant dimorphic fungi, helping to identify a number of virulence factors and immune-regulators and screen effective antifungal agents without cytotoxic effects. However, little has been achieved and reported with regard to pathogenic filamentous fungi (molds) in the nematode model. In this review, we have summarized the enormous breakthrough of applying a C. elegans infection model for dimorphic fungi studies and the very few reports for filamentous fungi. We have also identified and discussed the challenges in C. elegans-mold modeling applications as well as the possible approaches to conquer these challenges from our practical knowledge in C. elegans-Aspergillus fumigatus model.
Introduction
Pathogenic fungi pose an enormous global threat to humanity, leading to millions of deaths and substantial financial losses annually (Fisher et al., 2012; Rhodes, 2019). Morbidity and mortality rates from opportunistic fungal pathogens, such as Candida albicans, Aspergillus fumigatus, and Cryptococcus neoformans, have been increasing for some years, especially in immunocompromised patients (Pal, 2017; Linder et al., 2019; de Sousa-Neto et al., 2020). Addressing the pathogenesis of these fungal pathogens and finding controllable strategies are crucial and urgent. To tackle this threat, model organisms are required to conduct research focusing on the identification of virulence factors, screening of effective antifungal agents, and exploring appropriate treatment approaches.
Several model organisms have been adopted for studying of dimorphic and filamentous pathogenic fungi, including invertebrate models such as Drosophila melanogaster (Lamaris et al., 2008; Regulin and Kempken, 2018; Sampaio et al., 2018; Wurster et al., 2019), Galleria mellonella (Gomez-Lopez et al., 2014; Long et al., 2018; Silva et al., 2018; Staniszewska et al., 2020), Bombyx mori (Matsumoto et al., 2013; Uchida et al., 2016; Nakamura et al., 2017; Matsumoto and Sekimizu, 2019), Caenorhabditis elegans (Okoli and Bignell, 2015; Song et al., 2019; Wong et al., 2019; Ahamefule et al., 2020a), and vertebrate models such as mice (Fakhim et al., 2018; Skalski et al., 2018; Wang et al., 2018; Mueller et al., 2019), guinea pigs (Vallor et al., 2008; Nadăş et al., 2013; Garvey et al., 2015), and zebrafish (Chen et al., 2015; Knox et al., 2017; Koch et al., 2019; Kulatunga et al., 2019).
C. elegans is a microscopic multicellular nematode that lives freely in soil (Muhammed et al., 2012; Kim et al., 2017). Advantages, such as short life cycle, physiological simplicity, transparent body, complete sequenced genome, mature genetic manipulation system, and no requirement for ethical license, have greatly encouraged the wide adoption of this nematode as a model organism in scientific research with assorted applications across several research fields (Okoli et al., 2009; Ballestriero et al., 2010; Huang et al., 2014; Jiang and Wang, 2018). Some of these applications have been established for decades now whereas others are still in their nascent stages undergoing several studies. Nematode infection by the natural nematophagous obligate filamentous fungus Drechmeria coniospora is a common incidence in nature. C. elegans is usually applied for studying the innate immunity of nematodes to this fungus (Engelmann et al., 2011; Couillault et al., 2012; Zugasti et al., 2016). This nematode model has also been explored as an in vivo model for studying infections of human pathogenic filamentous fungi (Okoli and Bignell, 2015; Ahamefule et al., 2020a).
Application of the nematode model for dimorphic pathogenic fungi studies has resulted in numerous publications whereas only a few publications thus far have been recorded for human filamentous pathogenic fungi studies, such as A. fumigatus (Okoli and Bignell, 2015; Ahamefule et al., 2020a; Eldesouky et al., 2020a). Here, we have extensively portrayed C. elegans-dimorphic fungi (in particular Candida spp.) infection models for determining virulence factors (reported within the last decade) and evaluated the effectiveness of anticandidal agents, including drugs, bioactive compounds, and live biotherapeutic products (reported within the last 5 years). The practical challenges constraining the applications of the C. elegans model for filamentous fungi are elaborated, and possible solutions are raised for future improvement.
Application of C. elegans for Dimorphic Fungi Studies
C. elegans has been extensively used for studying several dimorphic fungi of clinical relevance. The most devastating and pathogenic dimorphic fungus that has been adequately explored with this nematode model is Candida albicans (Hans et al., 2019a; Hans et al., 2019b; Song et al., 2019; Venkata et al., 2020) and a few other non-albicans species such as C. tropicalis (Brilhante et al., 2016; Feistel et al., 2019; Pedroso et al., 2019), C. krusei (De Aguiar Cordeiro et al., 2018; Kunyeit et al., 2019), and C. auris (Eldesouky et al., 2018a; Mohammad et al., 2019). Another important clinical dimorphic fungus, Taloromyces (Penicillium) marneffei, has also been studied in a C. elegans model for both virulence tests and antifungal agent efficacy evaluations (Huang et al., 2014; Sangkanu et al., 2021).
Virulence factors of C. albicans such as genes involved in hyphal filamentation and biofilm formation (Romanowski et al., 2012; Sun et al., 2015; Holt et al., 2017), intestinal adhesion and colonization (Rane et al., 2014a; Muthamil et al., 2018; Priya and Pandian, 2020), important virulence enzymes (Ortega-Riveros et al., 2017; Song et al., 2019), transcription factors (Jain et al., 2013; Hans et al., 2019a), and environmental and nutrient factors (Hammond et al., 2013; Lopes et al., 2018; Hans et al., 2019b; Wong et al., 2019) have been identified in a C. elegans model to strengthen our understanding of the in vivo pathogenesis of this important fungal pathogen (Table 1). The virulence traits of some other non-albicans species (both dimorphic and nondimorphic) have also been investigated with this nematode model (Table 1). Similarly, virulence factors such as pigmentation and hyphal filamentation have been demonstrated to be critical pathogenic features of T. marneffei in a C. elegans infection model (Huang et al., 2014; Sangkanu et al., 2021). C. elegans glp-4; sek-1 worms have mostly been used in these studies (aside from the wild-type strain, N2) because of their inability to produce progeny at 25°C due to the glp-4 mutation and their susceptibility to pathogens due to sek-1 mutation, thus making the worms immunocompromised for infection by opportunistic human fungi (Huang et al., 2014; Okoli and Bignell, 2015; Ahamefule et al., 2020a)
Moreover, the adoption of a C. elegans model for searching and screening of effective bioactive compounds against several species of Candida has also received much attention. Effective bioactive compounds from marine habitats (Subramenium et al., 2017; Ganesh Kumar et al., 2019), plant parts (Shu et al., 2016; Pedroso et al., 2019), and other sources (Table 2) have been discovered because of their in vivo efficacies against several Candida species and were simultaneously evaluated for their cytotoxicity in a C. elegans model. Compounds such as alizarin, chrysazin, sesquiterpene, and purpurin were discovered to be quite effective in in vivo assays with effective doses ranging from 1 to 10 µg/ml (Table 2), indicating potential future prospects for antifungal drug research and discovery. Other compounds such as thymol (Shu et al., 2016), coumarin (Xu et al., 2019), and theophylline (Singh et al., 2020), were only effective at high concentrations of 64, 2, and 1.6 mg/ml, respectively (Table 2). Most of these compounds were certified as nontoxic at such effective concentrations as they were able to rescue infected nematodes and significantly elongated their lifespan (Table 2).
The drug resistance threat of Candida species, similar to most other pathogens, is constantly increasing, leading to increased incidences of mortality and morbidity (Sanguinetti et al., 2015; Popp et al., 2017; Popp et al., 2019; Prasad et al., 2019). C. elegans has also proven to be an effective in vivo model for studying the infection of several azole-resistant C. albicans (Chang et al., 2015; Sun et al., 2018) and C. auris (Eldesouky et al., 2018a; Eldesouky et al., 2018b) strains. Studies have demonstrated the in vivo efficacy of some bioactive compounds applied singly or in combination with initially resistant antifungal drugs in the treatment of infected nematodes (Table 3).
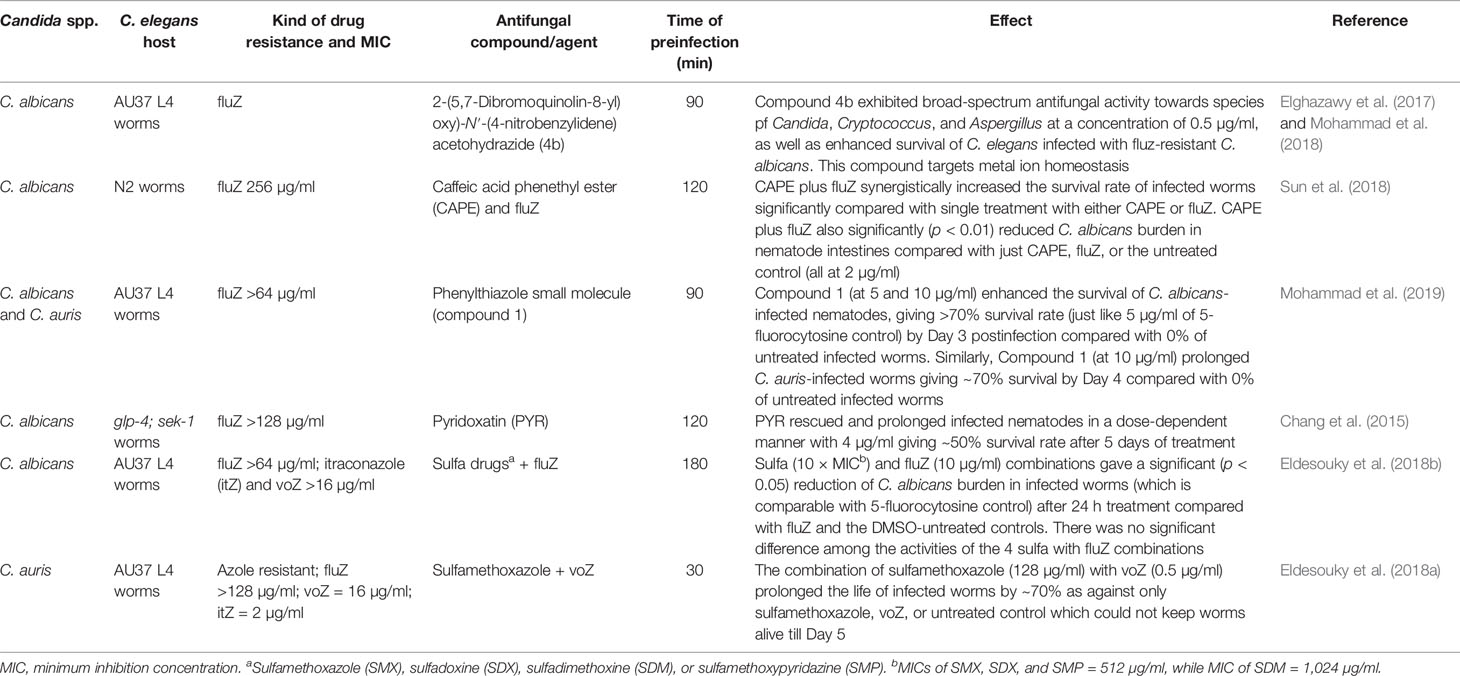
Table 3 Evaluation of effective agents against drug-resistant Candida species in a C. elegans model.
Compounds such as 2-(5,7-dibromoquinolin-8-yl)oxy)-N′-(4-nitrobenzylidene) acetohydrazide (Elghazawy et al., 2017; Mohammad et al., 2018) and phenylthiazole small molecules (Mohammad et al., 2019) are among the recently demonstrated effective compounds with good outcomes in nematode candidiasis (with effective dose concentrations of ≥4 and ≥5 µg/ml, respectively) against fluZ-resistant C. albicans and/or C. auris (Table 3). The combination of caffeic acid phenethyl ester (CAPE) and fluZ (Sun et al., 2018) as well as the sulfamethoxazole and voriconazole (voZ) combination (Eldesouky et al., 2018a) effectively rescued C. elegans worms infected by azole-resistant C. albicans and C. auris, respectively (Table 3).
The search for alternative treatment drugs with new inhibition mechanisms against pathogenic fungi such as C. albicans is a pressing need. Obtaining effective compounds that may not necessarily have a direct effect on Candida planktonic cells but affect critical virulence factors has recently been made possible by evaluating the efficacy of the compounds in a C. elegans infection model (Graham et al., 2017; Subramenium et al., 2017; Manoharan et al., 2018) (Table 4).
Remarkably, some compounds such as loureirin A (Lin et al., 2019), camphor, and fenchyl alcohol (Manoharan et al., 2017b) are effective compounds protecting infected worms at concentration doses less than the in vitro MICs (Table 4). Cascarilla bark oil, α-longipinene, linalool (Manoharan et al., 2018), and Enterococcus faecalis bacteriocin (EntV) (Graham et al., 2017) were reported to be quite potent in rescuing infected worms at low effective concentration doses, such as ≥0.001% for cascarilla bark oil, α-longipinene and linalool and 0.1 nM for EntV (Table 4).
These compounds usually rescue infected nematodes through other pathways such as direct effects on cardinal virulence factors and/or by stimulating/enhancing the immune responses of the host against pathogens (Okoli et al., 2009; Peterson and Pukkila-Worley, 2018; Ahamefule et al., 2020b). Such compounds may only be screened and identified through in vivo assays since they usually show little or no antimicrobial activities in in vitro assays. The adoption of simple in vivo models such as C. elegans significantly supports the screening and identification of more such compounds, which may expand the narrative of the usual antifungal therapies that primarily address direct effects on causative pathogens.
The application of live biotherapeutic products (LBPs) consisting mainly of probiotics is another alternative approach for the treatment of nematode candidiasis. Such alternative therapy is an interesting and promising option since pathogenic fungi are currently developing resistance to the few clinically available antifungal drugs (Sanguinetti et al., 2015; Prasad et al., 2019). Several species of Lactobacillus such as L. rhamnosus (Poupet et al., 2019a; Poupet et al., 2019b) and L. paracasei (de Barros et al., 2018) as well as probiotic yeasts—Saccharomyces cerevisiae and Issatchenkia occidentalis (Kunyeit et al., 2019)—have demonstrated efficient rescue of worms infected with a number of Candida species. These therapeutic microorganisms drastically reduced the burden of the pathogens in the C. elegans intestine approximately 2 to 4 h postinfection treatment (Table 5).
The efficacy of these LBPs in reducing and/or eliminating fungal burden implies the future potential of LBPs in addressing the fungal menace. The demonstrated significant increase (p < 2 × 10−16) in worm mean lifespan (Poupet et al., 2019a; Poupet et al., 2019b) is so high that it has not been reported in any potent bioactive compounds or even established antifungal drugs. The fact that most of these LBPs are already established probiotics is yet another important parameter that would advance future research beyond nematode models.
The in vivo efficacy of known antifungal drugs and a number of repurposed drugs have also been applied in the treatment of nematode candidiasis. Several azoles (Souza et al., 2018; Hernando-Ortiz et al., 2020), echinocandins (Souza et al., 2018), polyenes—particularly amphotericin B (Hernando-Ortiz et al., 2020), and β-lactam antibiotics (in combination with vancomycin) (De Aguiar Cordeiro et al., 2018) have been evaluated for their in vivo efficacy at varying effective concentrations in rescuing worms infected with Candida species (Table 6). Synthesized azole drugs, such as 1-(4-cyclopropyl-1H-1,2,3-triazol-1-yl)-2-(2,4-difluorophenyl)-3-(1H-1,2,4-triazol-1-yl) propan-2-ol, have also been evaluated for both efficacy and cytotoxicity in a C. elegans model (Chen et al., 2017).
Given that decades of searching for new antifungal agents have not truly resulted in new antifungal drugs, drug repurposing is a less expensive and welcome research prospect. The C. elegans infection model for evaluating the efficacy of repurposed drugs on candidiasis has attracted attention (Eldesouky et al., 2020b; Singh et al., 2020) (Table 6) due to the advantages of saving extensive time, cumbersome labor, and enormous cost of searching and obtaining new antifungal drugs.
C. elegans and Pathogenic Molds
The deadly opportunistic mold pathogen, A. fumigatus, ranks as the number 1 aetiological agent for aspergilloses in immunocompromised patients (Snelders et al., 2009; Fang and Latgé, 2018; Geißel et al., 2018) with an almost 100% mortality rate in some groups of patients (Darling and Milder, 2018; Geißel et al., 2018; Linder et al., 2019). This pathogen had not been well studied in C. elegans until recently. Okoli and Bignell (2015) were the first to demonstrate the possibility of adopting C. elegans for A. fumigatus infection. They set up the nematode model to study the pathogenicity of the clinical strain A. fumigatus Af293 for 72 h postinfection after an initial preinfection of 12 h. We recently reported a breakthrough in overcoming some of the challenges usually encountered in the C. elegans-mold infection system, one of which is removing spores that were not ingested by worms through a hand-made filter with a membrane-attached-on-tube. We were able to develop a stable and consistent C. elegans model for evaluating the virulence of A. fumigatus mutant strains that had previously been studied in other established models, including mice and insects. We also successfully demonstrated the possibility of in vivo testing of antifungal agents on nematode aspergillosis using the established model (Ahamefule et al., 2020a).
The established C. elegans-A. fumigatus model clearly demonstrated the progression of aspergillosis infection in nematodes using the A. fumigatus fluorescence strain, Af293-dsRed, showing that hyphal filamentation could actually emanate from any part of the infected worms against the previously reported concept of mainly the tail region (Okoli and Bignell, 2015; Ahamefule et al., 2020a). Our worm model was able to identify important virulence factors of A. fumigatus such as α-(1,3)-glucan synthase, melanin pigmentation, iron transporter, Zn2Cys6-type transcription factor, and mitochondrial thiamine pyrophosphate transporter, as mutant strains without these components (triple agsΔ, pksPΔ, ΔmrsA, ΔleuB, and ΔtptA, respectively), all of which gave significantly attenuated virulence compared with the A. fumigatus parent strain KU80Δ. These reduced virulence patterns obtained by our C. elegans model were similar to previously reported attenuated virulence patterns of these A. fumigatus mutants in both vertebrate and insect models. The nematode model was also demonstrated to be an easy in vivo system to evaluate antifungal drug efficacy thus presenting the model as a desired platform for screening antifungal agents against A. fumigatus in the future (Ahamefule et al., 2020a).
Challenges of C. elegans Applications in Modeling Pathogenic Mold
One of the biggest challenges usually encountered in the applications of the C. elegans model for filamentous fungal infection is the difficulty in infecting the worms through conidia. Worms usually avoid eating conidia unless they starve with no other option (Okoli and Bignell, 2015). This avoidance is unlike the case of dimorphic fungal and bacterial pathogens, where infection is never much of a problem as worms easily feed on the cells of these pathogens when they replace or are mixed up with nematode choice food (E. coli OP50 or HB101) (Breger et al., 2007; Johnson et al., 2009; Kirienko et al., 2013; Okoli and Bignell, 2015).
Giving the worms more time to starve and more access to the conidia (placed at four cardinal points) for ingestion is very important for establishing mold preinfection assays. Okoli and Bignell (2015) adopted a 12-h preinfection technique, while we modified to 16 h (Ahamefule et al., 2020a). The fact is that worms must be given such ample time to “force” them to ingest the mold conidia in a preinfection system since coinfection approach (which is usually adopted for most dimorphic fungi modeling) cannot work well for mold pathogens (Okoli et al., 2009; Okoli and Bignell, 2015; Ahamefule et al., 2020a). As conidia germinate very fast even before the worms have ingested enough spores in killing assay medium, a relatively less nutritious medium was adopted for pre-infection assay to avoid the quick growth and flooding of hyphal filaments in the rich killing assay medium (brain heart infusion medium); otherwise later experimental procedures will be severely limited (Okoli and Bignell, 2015; Ahamefule et al., 2020a).
Another challenging aspect in setting up the C. elegans-mold model is the separation of noningested conidia from worms after pre-infection stage. Failure at this stage leads to the germination of unseparated spores in killing or antifungal screening media thus obstructing experimental progress. Although our designed membrane-attached-on-tube filter (with a 35-µm pore diameter) was able to remove a great deal of noningested conidia, the separation was not 100% efficient. Modifying the membrane pore size to an appropriate diameter should help improve the filtration efficiency by allowing faster and better removal of conidia while keeping the preinfected L4/young adult worms (Figure 1). Even though the separation efficiency of noningested spores becomes 100% or close to it, hyphae growth in killing medium would still not be completely eliminated, particularly if the experiment is scheduled to go beyond 72 h postinfection. This is because we have discovered that some conidia could be egested out of the nematode intestine into the killing medium and still retain their viability of germinating to hyphae, which is a big challenge to tackle and severely affect the experiment.
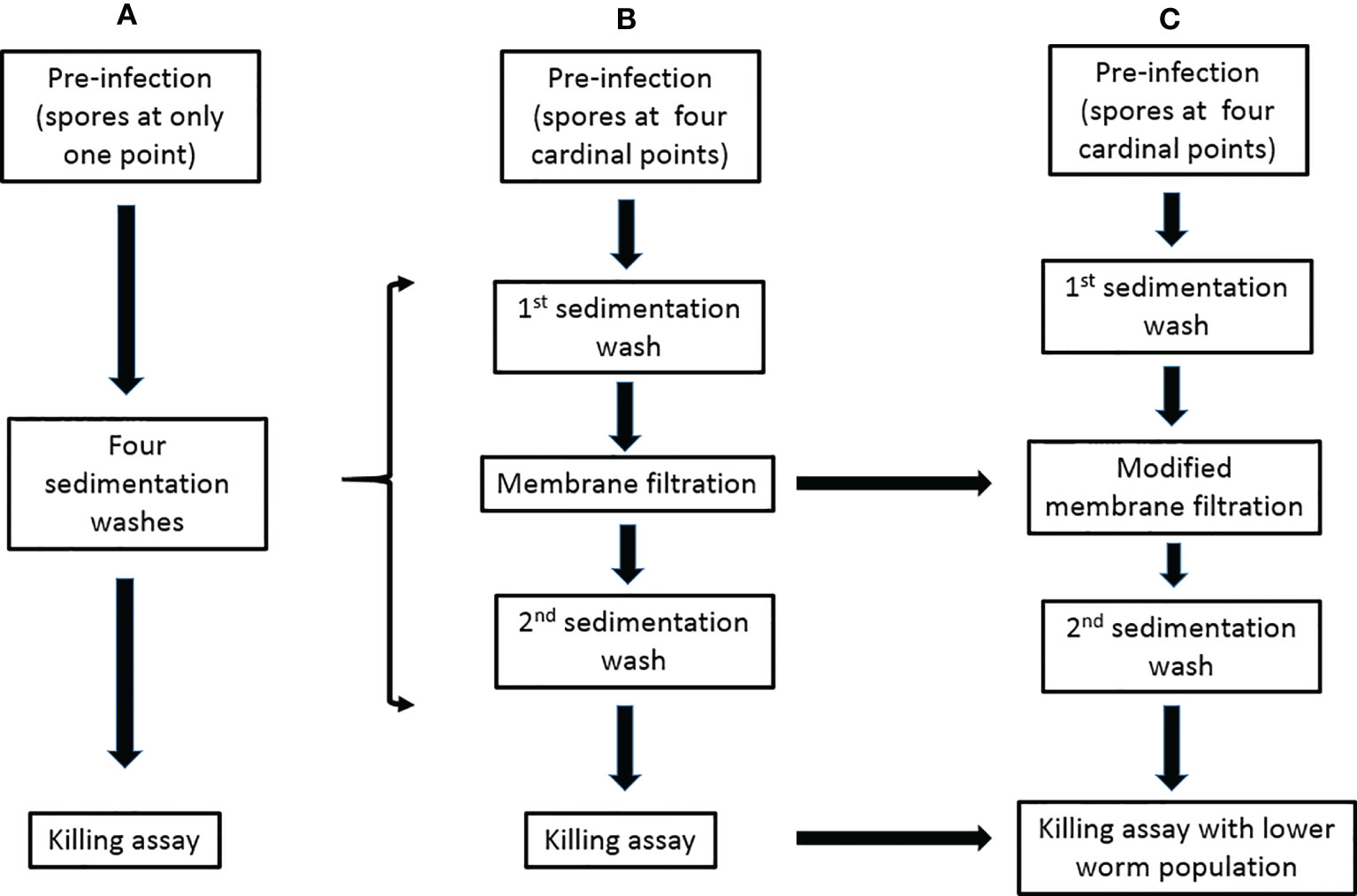
Figure 1 Modifications of the preinfection to killing assays for the C. elegans-mold infection model. (A) The previously described procedure (Okoli and Bignell, 2015). (B) The procedure in our publication (Ahamefule et al., 2020a). (C) Our proposed modifications.
Hyphal filamentation usually occurs in infected worms. Unlike most studied dimorphic fungi whose external hyphae protrude when worms were already dead (and could therefore be easily transferred), numerous worms infected with filamentous fungi such as A. fumigatus (Ahamefule et al., 2020a), A. flavus, and some strains of Penicillium (that we have studied in our laboratory), were discovered to still be alive with protruded hyphae. This makes these worms stuck to the killing assay plates and therefore difficult to remove (Ahamefule et al., 2020a). Such filamentation usually becomes profuse, growing and spreading very fast and may eventually obstruct visibility and affect the experimental results. Regulating the number of immunocompromised worms in killing assays, especially for highly virulent pathogenic molds, is an option to ameliorate this menace (Figure 1).
Conclusions
The tremendous health hazards of pathogenic fungi cannot be overemphasized. Better understanding of in vivo pathogeneses and identification of virulence factors are urgent and imperative to fight against these fungi. Screening, identifying and repurposing effective compounds/drugs against them as well as obtaining and optimizing effective treatment alternatives are desirable at this time. Therefore, developing, optimizing and applying better modelling organisms such as C. elegans is meaningful not only for dimorphic fungi but also for mold pathogens. Our review of the breakthrough applications of C. elegans for dimorphic fungi studies and progress/modifications of the C. elegans-mold infection model will provide a reference for studying fungal infections and developing antifungal agents.
Author Contributions
CA and BE wrote the initial manuscript. JO, AM, AI, BW, CJ, and WF revised the manuscript. WF supervised the manuscript. All authors have read and agreed to the published version of the manuscript.
Funding
This work was supported by National Natural Science Foundation of China (31960032, 32071279), Guangxi Natural Science Foundation (2020GXNSFDA238008) to WF, Research Start-up Funding of Guangxi Academy of Sciences (2017YJJ026) to BW, and Bagui Scholar Program Fund (2016A24) of Guangxi Zhuang Autonomous Region to CJ.
Conflict of Interest
The authors declare that the research was conducted in the absence of any commercial or financial relationships that could be construed as a potential conflict of interest.
Publisher’s Note
All claims expressed in this article are solely those of the authors and do not necessarily represent those of their affiliated organizations, or those of the publisher, the editors and the reviewers. Any product that may be evaluated in this article, or claim that may be made by its manufacturer, is not guaranteed or endorsed by the publisher.
References
Ahamefule, C. S., Ezeuduji, B. C., Ogbonna, J. C., Moneke, A. N., Ike, A. C., Wang, B., et al. (2020b). Marine Bioactive Compounds AgainstAspergillus Fumigatus: Challenges and Future Prospects. Antibiotics 9 (11), 813. doi: 10.3390/antibiotics9110813
Ahamefule, C. S., Qin, Q., Odiba, A. S., Li, S., Moneke, A. N., Ogbonna, J. C., et al. (2020a). Caenorhabditis Elegans-Based Aspergillus Fumigatus Infection Model for Evaluating Pathogenicity and Drug Efficacy. Front. Cell. Infection Microbiol. 10, 320. doi: 10.3389/fcimb.2020.00320
Ansari, M. A., Fatima, Z., Ahmad, K., Hameed, S. (2018). Monoterpenoid Perillyl Alcohol Impairs Metabolic Flexibility of Candida Albicans by Inhibiting Glyoxylate Cycle. Biochem. Biophys. Res. Commun. 495 (1), 560–566. doi: 10.1016/j.bbrc.2017.11.064
Ballestriero, F., Thomas, T., Burke, C., Egan, S., Kjelleberg, S. (2010). Identification of Compounds With Bioactivity Against the Nematode Caenorhabditis Elegans by a Screen Based on the Functional Genomics of the Marine Bacterium Pseudoalteromonas Tunicate D2. Appl. Environ. Microbiol. 76 (17), 5710–5717. doi: 10.1128/AEM.00695-10
Bi, S., Lv, Q. Z., Wang, T. T., Fuchs, B. B., Hu, D.-D., Anastassopoulou, C. G., et al. (2018). SDH2 Is Involved in Proper Hypha Formation and Virulence in Candida Albicans. Future Microbiol. 13 (10), 1141–1156. doi: 10.2217/fmb-2018-0033
Breger, J., Fuchs, B. B., Aperis, G., Moy, T. I., Asbel, F. M., Mylonakis, E. (2007). Antifungal Chemical Compounds Identified Using a C. Elegans Pathogenicity Assay. PloS Pathog. 3 (2), e18. doi: 10.1371/journal.ppat.0030018
Brilhante, R. S. N., Oliveira, J. S., Evangelista, A. J. J., Serpa, R., Silva, A. L. D., Aguiar, F. R. M., et al. (2016). Candida Tropicalis From Veterinary and Human Sources Shows Similar In Vitro Hemolytic Activity, Antifungal Biofilm Susceptibility and Pathogenesis Against Caenorhabditis Elegans. Veterinary Microbiol. 30 (192), 213–219. doi: 10.1016/j.vetmic.2016.07.022
Chang, W., Zhang, M., Li, Y., Li, X., Gao, Y., Xie, Z., et al. (2015). Lichen Endophyte Derived Pyridoxatin Inactivates Candida Growth by Interfering With Ergosterol Biosynthesis. Biochim. Biophys. Acta 1850 (9), 1762–1771. doi: 10.1016/j.bbagen.2015.05.005
Chen, H.-J., Jiang, Y.-J., Zhang, Y.-Q., Jing, Q.-W., Liu, N., Wang, Y. (2017). New Triazole Derivatives Containing Substituted 1,2,3-Triazole Side Chains: Design, Synthesis and Antifungal Activity. Chin. Chem. Lett. 28, 913–918. doi: 10.1016/j.cclet.2016.11.027
Chen, Y. Z., Yang, Y. L., Chu, W. L., You, M. S., Lo, H. J. (2015). Zebrafish Egg Infection Model for Studying Candida Albicans Adhesion Factors. PloS One 10 (11), e0143048. doi: 10.1371/journal.pone.0143048
Couillault, C., Fourquet, P., Pophillat, M., Ewbank, J. J. (2012). A UPR-Independent Infection-Specific Role for a BiP/GRP78 Protein in the Control of Antimicrobial Peptide Expression in C. Elegans Epidermis. Virulence 3 (3), 299–308. doi: 10.4161/viru.20384
Darling, B. A., Milder, E. A. (2018). Invasive Aspergillosis. Pediatr. Rev. 39 (9), 476–478. doi: 10.1542/pir.2017-0129
De Aguiar Cordeiro, R., de Jesus Evangelista, A. J., Serpa, R., Colares de Andrade, A. R., Leite Mendes, P. B., Silva Franco, J. D., et al. (2018). β-Lactam Antibiotics & Vancomycin Increase the Growth & Virulence of Candida Spp. Future Microbiol. 13, 869–875. doi: 10.2217/fmb-2018-0019
de Barros, P. P., Scorzoni, L., Ribeiro, F. C., Fugisaki, L. R. O., Fuchs, B. B., Mylonakis, E., et al. (2018). Lactobacillus Paracasei 28.4 Reduces In Vitro Hyphae Formation of Candida Albicans and Prevents the Filamentation in an Experimental Model of Caenorhabditis Elegans. Microbial Pathogenesis 117, 80–87. doi: 10.1016/j.micpath.2018.02.019
de Sá, N. P., de Paula, L. F. J., Lopes, L. F. F., Cruz, L. I. B., Matos, T. T. S., Lino, C. I., et al. (2018). In Vivo and In Vitro Activity of a Bis-Arylidenecyclo-Alkanone Against Fluconazole-Susceptible and -Resistant Isolates of Candida Albicans. J. Global Antimicrobial Resistance 14, 287–293. doi: 10.1016/j.jgar.2018.04.012
de Sousa-Neto, A., de Brito Röder, D., Pedroso, R. (2020). Invasive Fungal Infections in People Living With HIV/AIDS. J. Biosci. Med. 8, 15–26. doi: 10.4236/jbm.2020.89002
Eldesouky, H. E., Li, X., Abutaleb, N. S., Mohammad, H., Seleem, M. N. (2018a). Synergistic Interactions of Sulfamethoxazole and Azole Antifungal Drugs Against Emerging Multidrug-Resistant Candida Auris. Int. J. Antimicrobial Agents 52 (6), 754–761. doi: 10.1016/j.ijantimicag.2018.08.016
Eldesouky, H. E., Mayhoub, A., Hazbun, T. R., Seleem, M. N. (2018b). Reversal of Azole Resistance in Candida Albicans by Sulfa Antibacterial Drugs. Antimicrobial Agents Chemotherapy 62 (3), e00701–e00717. doi: 10.1128/AAC.00701-17
Eldesouky, H. E., Salama, E. A., Hazbun, T. R., Mayhoub, A. S., Seleem, M. N. (2020a). Ospemifene Displays Broad-Spectrum Synergistic Interactions With Itraconazole Through Potent Interference With Fungal Efflux Activities. Sci. Rep. 10 (1), 6089. doi: 10.1038/s41598-020-62976-y
Eldesouky, H. E., Salama, E. A., Li, X., Hazbun, T. R., Mayhoub, A. S., Seleem, M. N. (2020b). Repurposing Approach Identifies Pitavastatin as a Potent Azole Chemosensitizing Agent Effective Against Azole-Resistant Candida Species. Sci. Rep. 10 (1), 7525. doi: 10.1038/s41598-020-64571-7
Elghazawy, N. H., Hefnawy, A., Sedky, N. K., El-Sherbiny, I. M., Arafa, R. K. (2017). Preparation and Nanoformulation of New Quinolone Scaffold-Based Anticancer Agents: Enhancing Solubility for Better Cellular Delivery. Eur. J. Pharm. Sci. 105, 203–211. doi: 10.1016/j.ejps.2017.05.036
Engelmann, I., Griffon, A., Tichit, L., Montañana-Sanchis, F., Wang, G., Reinke, V., et al. (2011). A Comprehensive Analysis of Gene Expression Changes Provoked by Bacterial and Fungal Infection in C. Elegans. PloS One 6 (5), e19055. doi: 10.1371/journal.pone.0019055
Fakhim, H., Vaezi, A., Dannaoui, E., Chowdhary, A., Nasiry, D., Faeli, L., et al. (2018). Comparative Virulence of Candida Auris With Candida Haemulonii, Candida Glabrata and Candida Albicans in a Murine Model. Mycoses 61 (6), 377–382. doi: 10.1111/myc.12754
Fang, W., Latgé, J. P. (2018). Microbe Profile: Aspergillus Fumigatus: A Saprotrophic and Opportunistic Fungal Pathogen. Microbiology 164 (8), 1009–1011. doi: 10.1099/mic.0.000651
Feistel, D. J., Elmostafa, R., Nguyen, N., Penley, M., Morran, L., Hickman, M. A., et al. (2019). A Novel Virulence Phenotype Rapidly Assesses Candida Fungal Pathogenesis in Healthy and Immunocompromised Caenorhabditis Elegans Hosts. mSphere 4 (2), e00697–e00618. doi: 10.1128/mSphere.00697-18
Fiori, A., Kucharíková, S., Govaert, G., Cammue, B. P., Thevissen, K., Van Dijck, P. (2012). The Heat-Induced Molecular Disaggregase Hsp104 of Candida Albicans Plays a Role in Biofilm Formation and Pathogenicity in a Worm Infection Model. Eukaryotic Cell 11 (8), 1012–1020. doi: 10.1128/EC.00147-12
Fisher, M. C., Henk, D. A., Briggs, C. J., Brownstein, J. S., Madoff, L. C., McCraw, S. L., et al. (2012). Emerging Fungal Threats to Animal, Plant and Ecosystem Health. Nature 484 (7393), 186–194. doi: 10.1038/nature10947
Ganesh Kumar, A., Balamurugan, K., Vijaya Raghavan, R., Dharani, G., Kirubagaran, R. (2019). Studies on the Antifungal and Serotonin Receptor Agonist Activities of the Secondary Metabolites From Piezotolerant Deep-Sea Fungus Ascotricha Sp. Mycology 10 (2), 92–108. doi: 10.1080/21501203.2018.1541934
Garvey, E. P., Hoekstra, W. J., Moore, W. R., Schotzinger, R. J., Long, L., Ghannoum, M. A. (2015). VT-1161 Dosed Once Daily or Once Weekly Exhibits Potent Efficacy in Treatment of Dermatophytosis in a Guinea Pig Model. Antimicrobial Agents Chemotherapy 59 (4), 1992–1997. doi: 10.1128/AAC.04902-14
Geißel, B., Loiko, V., Klugherz, I., Zhu, Z., Wagener, N., Kurzai, O., et al. (2018). Azole-Induced Cell Wall Carbohydrate Patches Kill Aspergillus Fumigatus. Nat. Commun. 9 (1), 3098. doi: 10.1038/s41467-018-05497-7
Gomez-Lopez, A., Forastiero, A., Cendejas-Bueno, E., Gregson, L., Mellado, E., Howard, S. J., et al. (2014). An Invertebrate Model to Evaluate Virulence in Aspergillus Fumigatus: The Role of Azole Resistance. Med. Mycology 52 (3), 311–319. doi: 10.1093/mmy/myt022
Graham, C. E., Cruz, M. R., Garsin, D. A., Lorenz, M. C. (2017). Enterococcus Faecalis Bacteriocin EntV Inhibits Hyphal Morphogenesis, Biofilm Formation, and Virulence of Candida Albicans. Proc. Natl. Acad. Sci. United States America 25 (114), 4507–4512. doi: 10.1073/pnas.1620432114
Hammond, T. G., Stodieck, L., Birdsall, H. H., Becker, J. L., Koenig, P., Hammond, J. S., et al. (2013). Effects of Microgravity on the Virulence of Listeria Monocytogenes, Enterococcus Faecalis, Candida Albicans, and Methicillin-Resistant Staphylococcus Aureus. Astrobiology 13 (11), 1081–1090. doi: 10.1089/ast.2013.0986
Hans, S., Fatima, Z., Hameed, S. (2019a). Magnesium Deprivation Affects Cellular Circuitry Involved in Drug Resistance and Virulence in Candida Albicans. J. Global Antimicrobial Resistance 17, 263–275. doi: 10.1016/j.jgar.2019.01.011
Hans, S., Fatima, Z., Hameed, S. (2019b). Retrograde Signaling Disruption Influences ABC Superfamily Transporter, Ergosterol and Chitin Levels Along With Biofilm Formation in Candida Albicans. J. Mycologie Medicale 29 (3), 210–218. doi: 10.1016/j.mycmed.2019.07.003
Hernando-Ortiz, A., Mateo, E., Ortega-Riveros, M., De-la-Pinta, I., Quindós, G., Eraso, E. (2020). Caenorhabditis elegans as a Model System to Assess Candida glabrata, Candida nivariensis, and Candida bracarensis Virulence and Antifungal Efficacy. Antimicrob. Agents Chemother. 64 (10), e00824-20. doi: 10.1128/AAC.00824-20
Holt, J. E., Houston, A., Adams, C., Edwards, S., Kjellerup, B. V. (2017). Role of Extracellular Polymeric Substances in Polymicrobial Biofilm Infections of Staphylococcus Epidermidis and Candida Albicans Modelled in the Nematode Caenorhabditis Elegans. Pathog. Dis. 75 (5), ftx052. doi: 10.1093/femspd/ftx052
Huang, X., Li, D., Xi, L., Mylonakis, E. (2014). Caenorhabditis Elegans: A Simple Nematode Infection Model for Penicillium Marneffei. PloS One 9 (9), e108764. doi: 10.1371/journal.pone.0108764
Jain, C., Pastor, K., Gonzalez, A. Y., Lorenz, M. C., Rao, R. P. (2013). The Role of Candida Albicans AP-1 Protein Against Host Derived ROS in In Vivo Models of Infection. Virulence 4 (1), 67–76. doi: 10.4161/viru.22700
Jiang, H., Wang, D. (2018). The Microbial Zoo in the C. Elegans Intestine: Bacteria, Fungi and Viruses. Viruses 10 (2), 85. doi: 10.3390/v10020085
Johnson, C. H., Ayyadevara, S., McEwen, J. E., Shmookler Reis, R. J. (2009). Histoplasma Capsulatum and Caenorhabditis Elegans: A Simple Nematode Model for an Innate Immune Response to Fungal Infection. Med. Mycology 47, 808–813. doi: 10.3109/13693780802660532
Kim, W., Hendricks, G. L., Lee, K., Mylonakis, E. (2017). An Update on the Use of C. Elegans for Preclinical Drug Discovery: Screening and Identifying Anti-Infective Drugs. Expert Opin. Drug Discov. 12 (6), 625–633. doi: 10.1080/17460441.2017.1319358
Kirienko, N. V., Kirienko, D. R., Larkins-Ford, J., Wahlby, C., Ruvkun, G., Ausubel, F. M. (2013). Pseudomonas Aeruginosa Disrupts Caenorhabditis Elegans Iron Homeostasis, Causing a Hypoxic Response and Death. Cell Host Microbe 13 (4), 406–416. doi: 10.1016/j.chom.2013.03.003
Knox, B. P., Huttenlocher, A., Keller, N. P. (2017). Real-Time Visualization of Immune Cell Clearance of Aspergillus Fumigatus Spores and Hyphae. Fungal Genet. Biol. 105, 52–54. doi: 10.1016/j.fgb.2017.05.005
Koch, B. E. V., Hajdamowicz, N. H., Lagendijk, E., Ram, A. F. J., Meijer, A. H. (2019). Aspergillus Fumigatus Establishes Infection in Zebrafish by Germination of Phagocytized Conidia, While Aspergillus Niger Relies on Extracellular Germination. Sci. Rep. 9 (1), 12791. doi: 10.1038/s41598-019-49284-w
Kulatunga, D. C. M., Dananjaya, S. H. S., Nikapitiya, C., Kim, C. H., Lee, J., De Zoysa, M. (2019). Candida Albicans Infection Model in Zebrafish (Danio Rerio) for Screening Anticandidal Drugs. Mycopathologia 184 (5), 559–572. doi: 10.1007/s11046-019-00378-z
Kunyeit, L., Kurrey, N. K., Anu-Appaiah, K. A., Rao, R. P. (2019). Probiotic Yeasts Inhibit Virulence of Non-Albicans Candida Species. mBio 10 (5), e02307–e02319. doi: 10.1128/mBio.02307-19
Lamaris, G. A., Ben-Ami, R., Lewis, R. E., Kontoyiannis, D. P. (2008). Does Pre-Exposure of Aspergillus Fumigatus to Voriconazole or Posaconazole In Vitro Affect its Virulence and the In Vivo Activity of Subsequent Posaconazole or Voriconazole, Respectively? A Study in a Fly Model of Aspergillosis. J. Antimicrob. Chemother. 62 (3), 539–542. doi: 10.1093/jac/dkn224
Li, Y., Chang, W., Zhang, M., Ying, Z., Lou, H. (2015). Natural Product Solasodine-3-O-β-D-Glucopyranoside Inhibits the Virulence Factors of Candida Albicans. FEMS Yeast Res. 15 (6), fov060. doi: 10.1093/femsyr/fov060
Linder, K. A., McDonald, P. J., Kauffman, C. A., Revankar, S. G., Chandrasekar, P. H., Miceli, M. H. (2019). Invasive Aspergillosis in Patients Following Umbilical Cord Blood Transplant. Bone Marrow Transplant. 54, 308–311. doi: 10.1038/s41409-018-0230-5
Lin, M.-Y., Yuan, Z.-L., Hu, D.-D., Hu, G.-H., Zhang, R.-L., Zhong, H., et al. (2019). Effect of Loureirin A Against Candida Albicans Biofilms. Chin. J. Natural Med. 17 (8), 616–623. doi: 10.1016/S1875-5364(19)30064-0
Li, Y., Shan, M., Yan, M., Yao, H., Wang, Y., Gu, B., et al. (2019). Anticandidal Activity of Kalopanaxsaponin A: Effect on Proliferation, Cell Morphology, and Key Virulence Attributes of Candida Albicans. Front. Microbiol. 10, 2844. doi: 10.3389/fmicb.2019.02844
Long, N., Orasch, T., Zhang, S., Gao, L., Xu, X., Hortschansky, P., et al. (2018). The Zn2Cys6-Type Transcription Factor LeuB Cross-Links Regulation of Leucine Biosynthesis and Iron Acquisition in Aspergillus Fumigatus. PloS Genet. 14 (10), e1007762. doi: 10.1371/journal.pgen.1007762
Lopes, J. P., Stylianou, M., Backman, E., Holmberg, S., Jass, J., Claesson, R., et al. (2018). Evasion of Immune Surveillance in Low Oxygen Environments Enhances Candida Albicans Virulence. mBio 9, e02120–e02118. doi: 10.1128/mBio.02120-18
Manoharan, R. K., Lee, J. H., Kim, Y. G., Kim, S. I., Lee, J. (2017c). Inhibitory Effects of the Essential Oils α-Longipinene and Linalool on Biofilm Formation and Hyphal Growth of Candida Albicans. Biofouling 33 (2), 143–155. doi: 10.1080/08927014.2017.1280731
Manoharan, R. K., Lee, J.-H., Kim, Y.-G., Lee, J. (2017a). Alizarin and Chrysazin Inhibit Biofilm and Hyphal Formation by Candida Albicans. Front. Cell. Infection Microbiol. 7, 447. doi: 10.3389/fcimb.2017.00447
Manoharan, R. K., Lee, J. H., Lee, J. (2017b). Antibiofilm and Antihyphal Activities of Cedar Leaf Essential Oil, Camphor, and Fenchone Derivatives Against Candida Albicans. Front. Microbiol. 8, 1476. doi: 10.3389/fmicb.2017.01476
Manoharan, R. K., Lee, J. H., Lee, J. (2018). Efficacy of 7-Benzyloxyindole and Other Halogenated Indoles to Inhibit Candida Albicans Biofilm and Hyphal Formation. Microbial Biotechnol. 11 (6), 1060–1069. doi: 10.1111/1751-7915.13268
Matsumoto, H., Nagao, J., Cho, T., Kodama, J. (2013). Evaluation of Pathogenicity of Candida Albicans in Germination-Ready States Using a Silkworm Infection Model. Med. Mycology J. 54 (2), 131–140. doi: 10.3314/mmj.54.131
Matsumoto, Y., Sekimizu, K. (2019). Silkworm as an Experimental Animal for Research on Fungal Infections. Microbiol. Immunol. 63 (2), 41–50. doi: 10.1111/1348-0421.12668
Mohammad, H., Eldesouky, H. E., Hazbun, T., Mayhoub, A. S., Seleem, M. N. (2019). Identification of a Phenylthiazole Small Molecule With Dual Antifungal and Antibiofilm Activity Against Candida Albicans and Candida Auris. Sci. Rep. 9 (1), 18941. doi: 10.1038/s41598-019-55379-1
Mohammad, H., Elghazawy, N. H., Eldesouky, H. E., Hegazy, Y. A., Younis, W., Avrimova, L., et al. (2018). Discovery of a Novel Dibromoquinoline Compound Exhibiting Potent Antifungal and Antivirulence Activity That Targets Metal Ion Homeostasis. ACS Infect. Dis. 4 (3), 403–414. doi: 10.1021/acsinfecdis.7b00215
Mueller, K. D., Zhang, H., Serrano, C. R., Billmyre, R. B., Huh, Y. H., Wiemann, P., et al. (2019). Gastrointestinal Microbiota Alteration Induced by Mucor Circinelloides in a Murine Model. J. Microbiol. 57 (6), 509–520. doi: 10.1007/s12275-019-8682-x
Muhammed, M., Coleman, J. J., Mylonakis, E. (2012). “Caenorhabditis Elegans: A Nematode Infection Model for Pathogenic Fungi,” in Host-Fungus Interactions. Methods in Molecular Biology (Methods and Protocols). Eds. Brand, A. C., MacCallum, D. M. (New Jersey, USA: Humana Press), 447–454. doi: 10.1007/978-1-61779-539-8_31
Muthamil, S., Balasubramaniam, B., Balamurugan, K., Pandian, S. K. (2018). Synergistic Effect of Quinic Acid Derived From Syzygium Cumini and Undecanoic Acid Against Candida Spp. Biofilm and Virulence. Front. Microbiol. 9, 2835. doi: 10.3389/fmicb.2018.02835
Nadăş, G. C., Taulescu, M. A., Ciobanu, L., Fiţ, N. I., Flore, C., Răpuntean, S., et al. (2013). The Interplay Between NSAIDs and Candida Albicans on the Gastrointestinal Tract of Guinea Pigs. Mycopathologia 175 (3-4), 221–230. doi: 10.1007/s11046-013-9613-8
Nakamura, I., Kanasaki, R., Yoshikawa, K., Furukawa, S., Fujie, A., Hamamoto, H., et al. (2017). Discovery of a New Antifungal Agent ASP2397 Using a Silkworm Model of Aspergillus Fumigatus Infection. J. Antibiotics 70 (1), 41–44. doi: 10.1038/ja.2016.106
Okoli, I., Bignell, E. M. (2015). Caenorhabditis Elegans-Aspergillus Fumigatus (Nematode-Mould) Model for Study of Fungal Pathogenesis. Br. Microbiol. Res. J. 7 (2), 93–99. doi: 10.9734/BMRJ/2015/15838
Okoli, I., Coleman, J. J., Tempakakis, E., An, W. F., Holson, E., Wagner, F., et al. (2009). Identification of Antifungal Compounds Active Against Candida Albicans Using an Improved High-Throughput Caenorhabditis Elegans Assay. PloS One 4 (9), e7025. doi: 10.1371/journal.pone.0007025
Ortega-Riveros, M., De-la-Pinta, I., Marcos-Arias, C., Ezpeleta, G., Quindós, G., Eraso, E. (2017). Usefulness of the Non-Conventional Caenorhabditis Elegans Model to Assess Candida Virulence. Mycopathologia 182 (9-10), 785–795. doi: 10.1007/s11046-017-0142-8
Pal, M. (2018). Morbidity and Mortality due to Fungal Infections. J. Appl. Microbiol. Biochem. 1 (1), 1–3. doi: 10.21767/2576-1412.100002
Pedroso, R. D. S., Balbino, B. L., Andrade, G., Dias, M. C. P. S., Alvarenga, T. A., Pedroso, R. C. N., et al. (2019). In Vitro and In Vivo Anti-Candida Spp. Activity of Plant-Derived Products. Plants (Basel) 8 (11), 494. doi: 10.3390/plants8110494
Peterson, N. D., Pukkila-Worley, R. (2018). Caenorhabditis Elegans in High-Throughput Screens for Anti-Infective Compounds. Curr. Opin. Immunol. 54, 59–65. doi: 10.1016/j.coi.2018.06.003
Popp, C., Hampe, I., Hertlein, T., Ohlsen, K., Rogers, P. D., Morschhäuser, J. (2017). Competitive Fitness of Fluconazole-Resistant Clinical Candida Albicans Strains. Antimicrobial Agents chemotherapy 61 (7), e00584–e00517. doi: 10.1128/AAC.00584-17
Popp, C., Ramírez-Zavala, B., Schwanfelder, S., Krüger, I., Morschhäuser, J. (2019). Evolution of Fluconazole-Resistant Candida Albicans Strains by Drug-Induced Mating Competence and Parasexual Recombination. mBio 10 (1), e02740–e02718. doi: 10.1128/mBio.02740-18
Poupet, C., Saraoui, T., Veisseire, P., Bonnet, M., Dausset, C., Gachinat, M., et al. (2019b). Lactobacillus Rhamnosus Lcr35 as an Effective Treatment for Preventing Candida Albicans Infection in the Invertebrate Model Caenorhabditis Elegans: First Mechanistic Insights. PloS One 14 (11), e0216184. doi: 10.1371/journal.pone.0216184
Poupet, C., Veisseire, P., Bonnet, M., Camarès, O., Gachinat, M., Dausset, C., et al. (2019a). Curative Treatment of Candidiasis by the Live Biotherapeutic Microorganism Lactobacillus Rhamnosus Lcr35® in the Invertebrate Model Caenorhabditis Elegans: First Mechanistic Insights. Microorganisms 8 (1), 34. doi: 10.3390/microorganisms8010034
Prasad, R., Nair, R., Banerjee, A. (2019). Emerging Mechanisms of Drug Resistance in Candida Albicans. Prog. Mol. Subcellular Biol. 58, 135–153. doi: 10.1007/978-3-030-13035-0_6
Priya, A., Pandian and, S. K. (2020). Piperine Impedes Biofilm Formation and Hyphal Morphogenesis of Candida Albicans. Front. Microbiol. 11, 756. doi: 10.3389/fmicb.2020.00756
Rane, H. S., Bernardo, S. M., Hayek, S. R., Binder, J. L., Parra, K. J., Lee, S. A. (2014b). The Contribution of Candida Albicans Vacuolar ATPase Subunit V₁B, Encoded by VMA2, to Stress Response, Autophagy, and Virulence Is Independent of Environmental pH. Eukaryotic Cell 13 (9), 1207–1221. doi: 10.1128/EC.00135-14
Rane, H.,. S., Hardison, S., Botelho, C., Bernardo, S. M., Wormley, J. F., Lee, S. A. (2014a). Candida Albicans VPS4 Contributes Differentially to Epithelial and Mucosal Pathogenesis. Virulence 5 (8), 810—818. doi: 10.4161/21505594.2014.956648
Regulin, A., Kempken, F. (2018). Fungal Genotype Determines Survival of Drosophila Melanogaster When Competing With Aspergillus Nidulans. PloS One 13 (1), e0190543. doi: 10.1371/journal.pone.0190543
Rhodes, J. (2019). Rapid Worldwide Emergence of Pathogenic Fungi. Cell Host Microbe 26 (1), 12–14. doi: 10.1016/j.chom.2019.06.009
Romanowski, K., Zaborin, A., Valuckaite, V., Rolfes, R. J., Babrowski, T., Bethel, C., et al. (2012). Candida Albicans Isolates From the Gut of Critically Ill Patients Respond to Phosphate Limitation by Expressing Filaments and a Lethal Phenotype. PloS One 7 (1), e30119. doi: 10.1371/journal.pone.0030119
Sampaio, A. D. G., Gontijo, A. V. L., Araujo, H. M., Koga-Ito, C. Y. (2018). In Vivo Efficacy of Ellagic Acid Against Candida Albicans in a Drosophila Melanogaster Infection Model. Antimicrobial Agents Chemotherapy 62 (12), e01716–e01718. doi: 10.1128/AAC.01716-18
Sangkanu, S., Rukachaisirikul, V., Suriyachadkun, C., Phongpaichit, S. (2021). Antifungal Activity of Marine-Derived Actinomycetes Against Talaromyces Marneffei. J. Appl. Microbiol. 130, 1508–1522. doi: 10.1111/jam.14877
Sanguinetti, M., Posteraro, B., Lass-Flörl, C. (2015). Antifungal Drug Resistance Among Candida Species: Mechanisms and Clinical Impact. Mycoses 58 (Suppl 2), 2–13. doi: 10.1111/myc.12330
Shu, C., Sun, L., Zhang, W. (2016). Thymol has Antifungal Activity Against Candida Albicans During Infection and Maintains the Innate Immune Response Required for Function of the P38 MAPK Signaling Pathway in Caenorhabditis Elegans. Immunologic Res. 64 (4), 1013–1024. doi: 10.1007/s12026-016-8785-y
Silva, L. N., Campos-Silva, R., Ramos, L. S., Trentin, D. S., Macedo, A. J., Branquinha, M. H., et al. (2018). Virulence of Candida Haemulonii Complex in Galleria Mellonella and Efficacy of Classical Antifungal Drugs: A Comparative Study With Other Clinically Relevant Non-Albicans Candida Species. FEMS Yeast Res. 18 (7), foy082. doi: 10.1093/femsyr/foy082
Singh, S., Fatima, Z., Ahmad, K., Hameed, S. (2018). Fungicidal Action of Geraniol Against Candida Albicans Is Potentiated by Abrogated CaCdr1p Drug Efflux and Fluconazole Synergism. PloS One 13 (8), e0203079. doi: 10.1371/journal.pone.0203079
Singh, S., Fatima, Z., Ahmad, K., Hameed, S. (2020). Repurposing of Respiratory Drug Theophylline Against Candida Albicans: Mechanistic Insights Unveil Alterations in Membrane Properties and Metabolic Fitness. J. Appl. Microbiol. 129 (4), 860–875. doi: 10.1111/jam.14669
Singulani, J. L., Scorzoni, L., Gomes, P. C., Nazaré, A. C., Polaquini, C. R., Regasini, L. O., et al. (2017). Activity of Gallic Acid and Its Ester Derivatives in Caenorhabditis Elegans and Zebrafish (Danio Rerio) Models. Future Medicinal Chem. 9 (16), 1863–1872. doi: 10.4155/fmc-2017-0096
Skalski, J. H., Limon, J. J., Sharma, P., Gargus, M. D., Nguyen, C., Tang, J., et al. (2018). Expansion of Commensal Fungus Wallemia Mellicola in the Gastrointestinal Mycobiota Enhances the Severity of Allergic Airway Disease in Mice. PloS Pathogen 14 (9), e1007260. doi: 10.1371/journal.ppat.1007260
Snelders, E., Huisin’t Veld, R. A. G., Rijs, A. J. M. M., Kema, G. H. J., Melchers, W. J. G., Verweij, P. E. (2009). Possible Environmental Origin of Resistance of Aspergillus Fumigatus to Medical Triazoles. Appl. Environ. Microbiol. 75 (12), 4053–4057. doi: 10.1128/AEM.00231-09
Song, Y., Li, S., Zhao, Y., Zhang, Y., Lv, Y., Jiang, Y., et al. (2019). ADH1 Promotes Candida Albicans Pathogenicity by Stimulating Oxidative Phosphorylation. Int. J. Med. Microbiol. 309, 151330. doi: 10.1016/j.ijmm.2019.151330
Souza, A. C. R., Fuchs, B. B., Alves, V. S., Jayamani, E., Colombo, A. L., Mylonakis, E. (2018). Pathogenesis of the Candida Parapsilosis Complex in the Model Host Caenorhabditis Elegans. Genes (Basel) 9 (8), 401. doi: 10.3390/genes9080401
Staniszewska, M., Gizińska, M., Kazek, M., de Jesús González-Hernández, R., Ochal, Z., Mora-Montes, H. M. (2020). New Antifungal 4-Chloro-3-Itrophenyldifluoroiodomethyl Sulfone Reduces the Candida Albicans Pathogenicity in the Galleria Mellonella Model Organism. Braz. J. Microbiol. 51 (1), 5–14. doi: 10.1007/s42770-019-00140-z
Subramenium, G. A., Swetha, T. K., Iyer, P. M., Balamurugan, K., Pandian, S. K. (2017). 5-Hydroxymethyl-2-Furaldehyde From Marine Bacterium Bacillus Subtilis Inhibits Biofilm and Virulence of Candida Albicans. Microbiological Res. 207, 19–32. doi: 10.1016/j.micres.2017.11.002
Sun, L., Liao, K., Hang, C. (2018). Caffeic Acid Phenethyl Ester Synergistically Enhances the Antifungal Activity of Fluconazole Against Resistant Candida Albicans. Phytomedicine 40, 55–58. doi: 10.1016/j.phymed.2017.12.033
Sun, L., Liao, K., Wang, D. (2015). Effects of Magnolol and Honokiol on Adhesion, Yeast-Hyphal Transition, and Formation of Biofilm by Candida Albicans. PloS One 10 (2), e0117695. doi: 10.1371/journal.pone.0117695
Tan, X., Fuchs, B. B., Wang, Y., Chen, W., Yuen, G. J., Chen, R. B., et al. (2014). The Role of Candida Albicans SPT20 in Filamentation, Biofilm Formation and Pathogenesis. PloS One 9 (4), e94468. doi: 10.1371/journal.pone.0094468
Thangamani, S., Eldesouky, H. E., Mohammad, H., Pascuzzi, P. E., Avramova, L., Hazbun, T. R., et al. (2017). Ebselen Exerts Antifungal Activity by Regulating Glutathione (GSH) and Reactive Oxygen Species (ROS) Production in Fungal Cells. Biochim. Biophys. Acta 1861 (1PtA), 3002–3010. doi: 10.1016/j.bbagen.2016.09.029
Uchida, R., Namiguchi, S., Ishijima, H., Tomoda, H. (2016). Therapeutic Effects of Three Trichothecenes in the Silkworm Infection Assay With Candida Albicans. Drug Discoveries Ther. 10 (1), 44–48. doi: 10.5582/ddt.2016.01013
Vallor, A. C., Kirkpatrick, W. R., Najvar, L. K., Bocarnegra, R., Kinney, M. C., Fothergill, A. W., et al. (2008). Assessment of Aspergillus Fumigatus Burden in Pulmonary Tissue of Guinea Pigs by Quantitative PCR, Galactomannan Enzyme Immunoassay, and Quantitative Culture. Antimicrobial Agents Chemotherapy 52 (7), 2593–2598. doi: 10.1128/AAC.00276-08
Venkata, S., Zeeshan, F., Kamal, A., Luqman, A. K., Saif, H. (2020). Efficiency of Vanillin in Impeding Metabolic Adaptability and Virulence of Candida Albicans by Inhibiting Glyoxylate Cycle, Morphogenesis, and Biofilm Formation. Curr. Med. Mycology 6 (1), 1–8. doi: 10.18502/cmm.6.1.2501
Wang, X., Bing, J., Zheng, Q., Zhang, F., Liu, J., Yue, H., et al. (2018). The First Isolate of Candida Auris in China: Clinical and Biological Aspects. Emerging Microbes Infection 187 (1), 93. doi: 10.1038/s41426-018-0095-0
Wong, D., Plumb, J., Talab, H., Kurdi, M., Pokhrel, K., Oelkers, P. (2019). Genetically Compromising Phospholipid Metabolism Limits Candida Albicans’ Virulence. Mycopathologia 184 (2), 213–226. doi: 10.1007/s11046-019-00320-3
Wurster, S., Bandi, A., Beyda, N. D., Albert, N. D., Raman, N. M., Raad, I. I., et al. (2019). Drosophila Melanogaster as a Model to Study Virulence and Azole Treatment of the Emerging Pathogen Candida Auris. J. Antimicrobial Chemotherapy 74 (7), 1904–1910. doi: 10.1093/jac/dkz100
Xu, K., Wang, J. L., Chu, M. P., Jia, C. (2019). Activity of Coumarin Against Candida Albicans Biofilms. J. Mycologie Medicale 29 (1), 28–34. doi: 10.1016/j.mycmed.2018.12.003
Zhang, M., Chang, W., Shi, H., Li, Y., Zheng, S., Li, W., et al. (2018). Floricolin C Elicits Intracellular Reactive Oxygen Species Accumulation and Disrupts Mitochondria to Exert Fungicidal Action. FEMS Yeast Res. 18 (1), foy002. doi: 10.1093/femsyr/foy002
Zheng, S., Chang, W., Zhang, M., Shi, H., Lou, H. (2018). Chiloscyphenol A Derived From Chinese Liverworts Exerts Fungicidal Action by Eliciting Both Mitochondrial Dysfunction and Plasma Membrane Destruction. Sci. Rep. 8 (1), 326. doi: 10.1038/s41598-017-18717-9
Keywords: Caenorhabditis elegans, dimorphic fungi, filamentous fungi, in vivo model, pathogenicity, high-throughput screening
Citation: Ahamefule CS, Ezeuduji BC, Ogbonna JC, Moneke AN, Ike AC, Jin C, Wang B and Fang W (2021) Caenorhabditis elegans as an Infection Model for Pathogenic Mold and Dimorphic Fungi: Applications and Challenges. Front. Cell. Infect. Microbiol. 11:751947. doi: 10.3389/fcimb.2021.751947
Received: 02 August 2021; Accepted: 28 September 2021;
Published: 15 October 2021.
Edited by:
Carlos Pelleschi Taborda, University of São Paulo, BrazilReviewed by:
Yen-Ping Hsueh, Academia Sinica, TaiwanHelen Fuchs, Rhode Island Hospital, United States
Copyright © 2021 Ahamefule, Ezeuduji, Ogbonna, Moneke, Ike, Jin, Wang and Fang. This is an open-access article distributed under the terms of the Creative Commons Attribution License (CC BY). The use, distribution or reproduction in other forums is permitted, provided the original author(s) and the copyright owner(s) are credited and that the original publication in this journal is cited, in accordance with accepted academic practice. No use, distribution or reproduction is permitted which does not comply with these terms.
*Correspondence: Bin Wang, YndhbmdAZ3hhcy5jbg==; Wenxia Fang, d2ZhbmdAZ3hhcy5jbg==