- 1Department of Periodontology, Graduate School of Medical and Dental Sciences, Tokyo Medical and Dental University (TMDU), Tokyo, Japan
- 2Oral Diagnosis and General Dentistry, Division of Clinical Dentistry, Tokyo Medical and Dental University Hospital, Tokyo Medical and Dental University (TMDU), Tokyo, Japan
- 3Division of Molecular Genetics, Research Center for Medical Sciences, The Jikei University School of Medicine, Tokyo, Japan
- 4Department of Bacterial Pathogenesis, Infection and Host Response, Graduate School of Medical and Dental Sciences, Tokyo Medical and Dental University (TMDU), Tokyo, Japan
- 5Comprehensive Reproductive Medicine, Regulation of Internal Environment and Reproduction, Systemic Organ Regulation, Graduate School, Tokyo Medical and Dental University (TMDU), Tokyo, Japan
- 6Division of Metabolism and Endocrinology, Faculty of Medicine, Saga University, Saga, Japan
- 7Liver Center, Saga University Hospital, Faculty of Medicine, Saga University, Saga, Japan
Preventing adverse pregnancy outcomes is crucial for maternal and child health. Periodontal disease is a risk factor for many systemic diseases including adverse pregnancy outcomes, such as preterm birth and low birth weight. In addition, the administration of the periodontopathic bacterium Porphyromonas gingivalis exacerbates obesity, glucose tolerance, and hepatic steatosis and alters endocrine function in the brown adipose tissue (BAT). However, the effects of having periodontal disease during pregnancy remain unclear. Thus, this study investigates the effect of P. gingivalis administration on obesity, liver, and BAT during pregnancy. Sonicated P. gingivalis (Pg) or saline (Co) was injected intravenously and administered orally to pregnant C57BL/6J mice three times per week. Maternal body weight and fetal body weight on embryonic day (ED) 18 were evaluated. Microarray analysis and qPCR in the liver and BAT and hepatic and plasma triglyceride quantification were performed on dams at ED 18. The body weight of Pg dams was heavier than that of Co dams; however, the fetal body weight was decreased in the offspring of Pg dams. Microarray analysis revealed 254 and 53 differentially expressed genes in the liver and BAT, respectively. Gene set enrichment analysis exhibited the downregulation of fatty acid metabolism gene set in the liver and estrogen response early/late gene sets in the BAT, whereas inflammatory response and IL6/JAK/STAT3 signaling gene sets were upregulated both in the liver and BAT. The downregulation of expression levels of Lpin1, Lpin2, and Lxra in the liver, which are associated with triglyceride synthesis, and a decreasing trend in hepatic triglyceride of Pg dams were observed. P. gingivalis administration may alter lipid metabolism in the liver. Overall, the intravenous and oral administration of sonicated P. gingivalis-induced obesity and modified gene expression in the liver and BAT in pregnant mice and caused fetuses to be underweight.
Introduction
Adverse pregnancy outcomes, such as preterm birth, small/large for gestational age, gestational diabetes, and hypertensive disorders, are serious health concerns, as they threaten the lives of both the mother and baby (Say et al., 2014; Liu et al., 2016). Particularly, preterm birth complications are the leading cause of death of infants under the age of five (Liu et al., 2016). Furthermore, obesity and severe liver dysfunction in pregnant mothers increase the risks of maternal and perinatal complications (Poston et al., 2016; Westbrook et al., 2016; Catalano and Shankar, 2017).
Periodontal disease is an infectious disease caused by periodontal bacteria; it triggers chronic inflammation and the destruction of tooth-supporting structures (Pihlstrom et al., 2005; Hajishengallis, 2015). Many systemic diseases, including diabetes and metabolic disorders, are epidemiologically associated with periodontal disease (Saito et al., 1998; Kocher et al., 2018; Genco and Borgnakke, 2020; Polak et al., 2020), and evidence supporting this claim has been reported in animal studies (Blasco-Baque et al., 2017; Komazaki et al., 2017; Polak et al., 2020; Watanabe et al., 2021). Systemic bacterial dissemination, swallowing bacteria, and systemic inflammation associated with periodontal disease are plausible pathways to systemic diseases (Hajishengallis, 2015; Olsen and Yamazaki, 2019).
A significant association between periodontal disease and preterm birth and low birth weight was found in multiple systematic reviews with meta-analysis (Vergnes and Sixou, 2007; Ide and Papapanou, 2013; Corbella et al., 2016; Vivares-Builes et al., 2018). In addition, periodontitis increases the risk for gestational diabetes (Abariga and Whitcomb, 2016). Administration of Porphyromonas gingivalis, a major periodontopathic bacterium, has been reported to cause obesity, impaired glucose tolerance, and aggravation of steatosis of the liver in mice after they were fed a high-fat diet (Sasaki et al., 2018). Furthermore, P. gingivalis administration altered endocrine function in the brown adipose tissue (BAT) (Hatasa et al., 2020). However, the effects of P. gingivalis on maternal obesity as well as liver and BAT functions under gestational conditions remain unclear.
Therefore, in the present study, we investigated the effects of the administration of P. gingivalis on pregnant mice, focusing on maternal obesity, fetal growth, and comprehensive gene expression in the liver and BAT.
Materials and Methods
Cultivation of P. gingivalis
P. gingivalis (ATCC 33277) was cultivated as described previously (Sasaki et al., 2018; Udagawa et al., 2018). Briefly, the bacteria were maintained on trypticase soy agar (Difco Laboratories, Detroit, MI, USA) supplemented with 10% defibrinated horse blood, hemin, and menadione at 37°C under anaerobic conditions. After 48 h, the bacteria were inoculated in trypticase soy broth and cultured at 37°C to the mid-log phase under anaerobic conditions. Subsequently, 109 CFU/mL of the bacterial suspension was sonicated at an amplitude of 20 kHz for 5 min on ice using a Qsonica Q700 sonicator (Waken Btech, Kyoto, Japan). To confirm the viability of the sonicated bacteria suspension, it was cultivated to ensure that no colony was detected. The presence of endotoxin in the sonicated P. gingivalis suspension was confirmed in a previous study (Sasaki et al., 2018).
Animals
Pregnant C57BL/6J mice (Embryonic day [ED] one; Sankyo Laboratory, Tokyo, Japan) were used in this study. The mice were provided ad libitum access to normal chow and water throughout the study and housed under standard conditions with a 12-h light/dark (light: 8:00 to 20:00) cycle. The mice were randomly divided into two groups: those administered with sonicated P. gingivalis suspension in the saline (Pg group, n = 10) and those receiving only saline (control [Co] group, n = 10). The administration was performed by both oral gavage and intravenous injection for each group. Sonicated P. gingivalis (108 CFU) suspension in 100 μL of saline or 100 μL of saline only (control) was administered by two different methods, respectively. In total, 200 μL of saline containing sonicated P. gingivalis or 200 μL of only saline was administered to each mouse every round. Administration was performed three times a week from ED 2, followed by the evaluation of the body weight of the mice. The liver, BAT from interscapular fat, and plasma were harvested from the mothers on ED 18 after they had been fasted for 6 h. The body weight of the surviving fetuses and the survival rate of fetuses were evaluated. The experimental design and time schedule of the study are shown in the Figure 1. All protocols associated with animal use and euthanasia were reviewed and approved by the Animal Care Committee of the Experimental Animal Center at Tokyo Medical and Dental University (A2020-157A, 2021-107A).
RNA Preparation
Extraction of total RNA from the liver and BAT samples (n = 10) were performed as described previously (Komazaki et al., 2017; Hatasa et al., 2021). In brief, the tissues were lysed and the aqueous layer containing RNA was separated using Trizol reagent (Invitrogen, Carlsbad, CA, USA). Then, total RNA was extracted from the layer using NucleoSpin® RNA kit (TaKaRa Bio, Shiga, Japan).
Microarray and Data Analysis
The quality of total RNA extracted from the liver and BAT were verified using an Agilent 2100 Bioanalyzer (Agilent Technologies, Santa Clara, CA). The Agilent Low Input Quick Amp Labeling kit (Agilent Technologies, Santa Clara, CA, USA) was used to generate complementary RNA (cRNA) from 200 ng of total RNA for single-color (Cy3) microarray analysis (n = 4), following the manufacturer’s instructions. Afterward, the cRNAs were hybridized onto an Agilent SurePrint G3 Unrestricted Gene Expression 8 × 60 K Microarray (Agilent Technologies). Fluorescence signals were detected using the Agilent Microarray Scanner System (Agilent Technologies). Raw microarray data were extracted using the Feature Extraction Software (ver. 11.0.1.1; Agilent Technologies).
Quantitative Reverse-Transcription PCR Analysis
Reverse-transcription and real-time PCR were performed (n = 10) as described previously (Hatasa et al., 2021). Briefly, 500 ng of total RNA was reverse-transcribed to cDNA using the PrimeScriptTM RT Master Mix (TaKaRa Bio). Real-time PCR was performed using the Thermal Cycler Dice® Real Time System II (TaKaRa Bio). PCR mixtures were prepared using TB Green Premix Ex TaqTM II (TaKaRa Bio). All procedures were performed following the manufacturer’s instructions. Rn18s was used as the reference gene for normalization. The PCR primers used in this study are listed in Supplementary Table S1.
Hepatic Triglyceride Measurements
Triglyceride in the liver was measured as previously described (Le Marchand et al., 1973; Fujii et al., 2008; Komazaki et al., 2017; Sasaki et al., 2018). Briefly, the aliquots of liver lysates were added to a microcentrifuge tubes containing 37.5% KOH and heated at 70°C for 30 min. The tubes were placed in 55°C water bath overnight (n = 4). Subsequently, 50% ethanol was added, and the tubes were centrifuged. The supernatants were separated, treated with MgCl2, left on ice for 10 min, and then centrifuged again. The supernatants and a triglyceride standard (Sigma Aldrich [St. Louis, MO, USA]) were placed in a 96 well black plate with a clear flat bottom, and triglyceride levels were measured using a commercially available kit (Triglyceride quantification kit, Sigma Aldrich [St. Louis, MO, USA]). Preparation was performed by following the manufacturer’s instruction. Fluorescence intensity (λex = 540 nm/λem = 590 nm) was measured with a plate reader (BMG Labtech FLUOstar OPTIMA-6).
Plasma Triglyceride Level Measurements
Blood samples with heparin were centrifuged and plasma was extracted. Commercially available kits were used to measure the plasma levels of triglycerides (n = 10) (Lab assay total triglyceride kit [290-63701], Wako [Osaka, Japan]) with a plate reader (Molecular devices SpectraMax ABS Plus). The measurement was carried out according to the manufacturer’s protocols.
Statistical Analysis
Data distributions were analyzed using the Shapiro–Wilk test. The unpaired t-test was performed to compare the data from the two groups; differences with P < 0.05 were considered statistically significant. Data were analyzed using the R software (ver. 4.0.2). The microarray data were quantile-normalized and log2-transformed, and differentially expressed genes (DEGs) from these data were identified using R with the Limma Bioconductor package (ver. 3.40.6) (Ritchie et al., 2015). Benjamin and Hochberg’s false discovery rate (FDR) was applied for multiple testing. DEGs were defined in accordance with the following criteria: an FDR q < 0.1 and a |fold change| > 1.5. Overrepresentation enrichment analyses for the DEGs were performed using the WEB-based Gene SeT AnaLysis Toolkit (http://www.webgestalt.org) (Wang et al., 2013) and the Database for Annotation, Visualization, and Integrated Discovery (DAVID) (http://david.abcc.ncifcrf.gov/), along with the Gene Ontology (GO) and Kyoto encyclopedia of genes and genomes (KEGG) pathway databases. Gene set enrichment analysis (GSEA) (http://software.broadinstitute.org/gsea/index.jsp) (Subramanian et al., 2005) was performed using hallmark gene sets (Liberzon et al., 2015).
Results
Significant weight gain and loss in the dams and fetuses, respectively, were observed and compared. The gene expression patterns in the liver and BATs associated with lipid synthesis and metabolism were studied to explain the weight gain and related impacts of P. gingivalis in dams.
P. gingivalis Administration to Pregnant Mice Increased the Body Weight of Mothers but Decreased the Body Weight of Fetuses
The body weight of the Pg dams was increased significantly from ED 14 to ED 18 after both oral gavage and intravenous administration of sonicated P. gingivalis thrice per week. The differences in body weight between Co and Pg dams increased throughout the experimental period (Figure 2A). A significant decrease in the body weight of fetuses from the Pg dams was observed at ED 18 (Figures 2B, C). Comparing dams with the same number of fetuses, the body weight of fetuses from Pg dams significantly decreased in dams with five, seven, and nine pups (Figures 2D, E, G) and that tended to decrease in dams with eight pups (P = 0.069, Figure 2F). There were no significant differences in the number of fetuses (Figure 2H) obtained from, or the fetus survival rate (Figure 2I) of, the Co and Pg dams at ED 18.
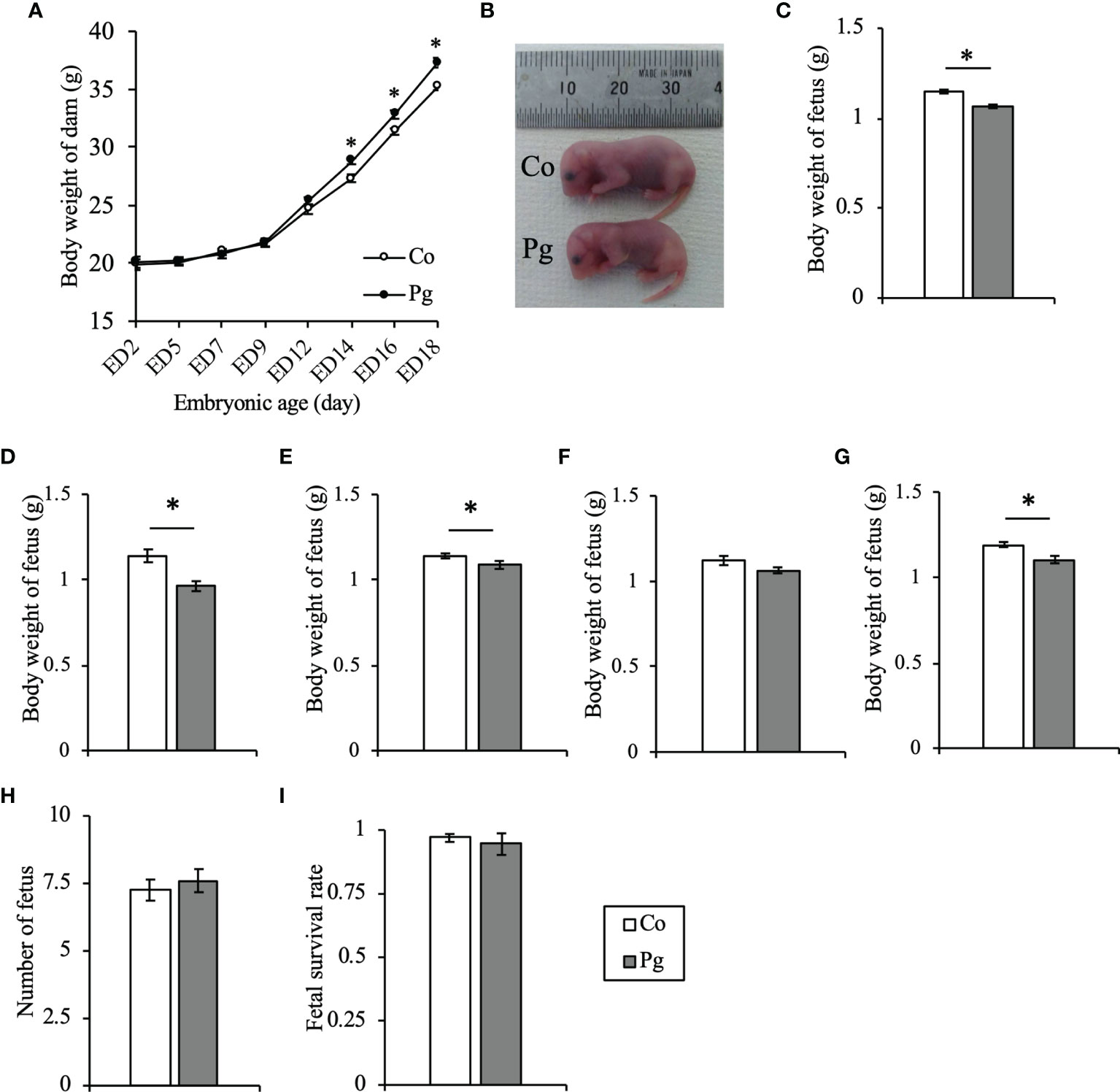
Figure 2 Sonicated P. gingivalis intravenous injection and oral administration induced maternal obesity and reduced body weight of fetuses. (A) Body weight of dams (n = 10, means ± SE, *P < 0.05). (B) Photograph of fetuses from Co and Pg dams at ED 18. (C) Body weight of fetuses at ED 18 (Co; n = 84, Pg; n = 76, means ± SE, *P < 0.05). (D) Body weight of fetuses at ED 18 from dams with five fetuses (Co; n = 5, Pg; n = 5, means ± SE, *P < 0.05). (E) Body weight of fetuses at ED 18 from dams with seven fetuses (Co; n = 55, Pg; n = 14, means ± SE, *P < 0.05). (F) Body weight of fetuses at ED 18 from dams with eight fetuses (Co; n = 8, Pg; n = 32, means ± SE, *P < 0.05). (G) Body weight of fetuses at ED 18 from dams with nine fetuses (Co; n = 9, Pg; n = 9, means ± SE, *P < 0.05). (H) Number of fetuses per dam (n = 10, means ± SE, *P < 0.05). (I) Fetal survival rate (n = 10, means ± SE, *P < 0.05).
P. gingivalis Administration Altered the Gene Expression Patterns in the Liver of Dams
To investigate gene expression in the liver following P. gingivalis administration during pregnancy, microarray analysis was performed to obtain a comprehensive overview of the gene expression profiles. All microarray data herein are available in the Gene Expression Omnibus (GEO) database (www.ncbi.nlm.nih.gov/geo) under GSE180189. As shown in Figure 3A, among 254 DEGs (|fold change| > 1.5 and q < 0.1), 248 genes were upregulated, and six genes were downregulated. The gene expression patterns differed substantially (Figure 3B). GO slim overviewed the ontology content in upregulated DEGs; interestingly, 42% of the upregulated DEGs with GO terms were classified as “metabolic process” in the biological process category (Figure 3C).
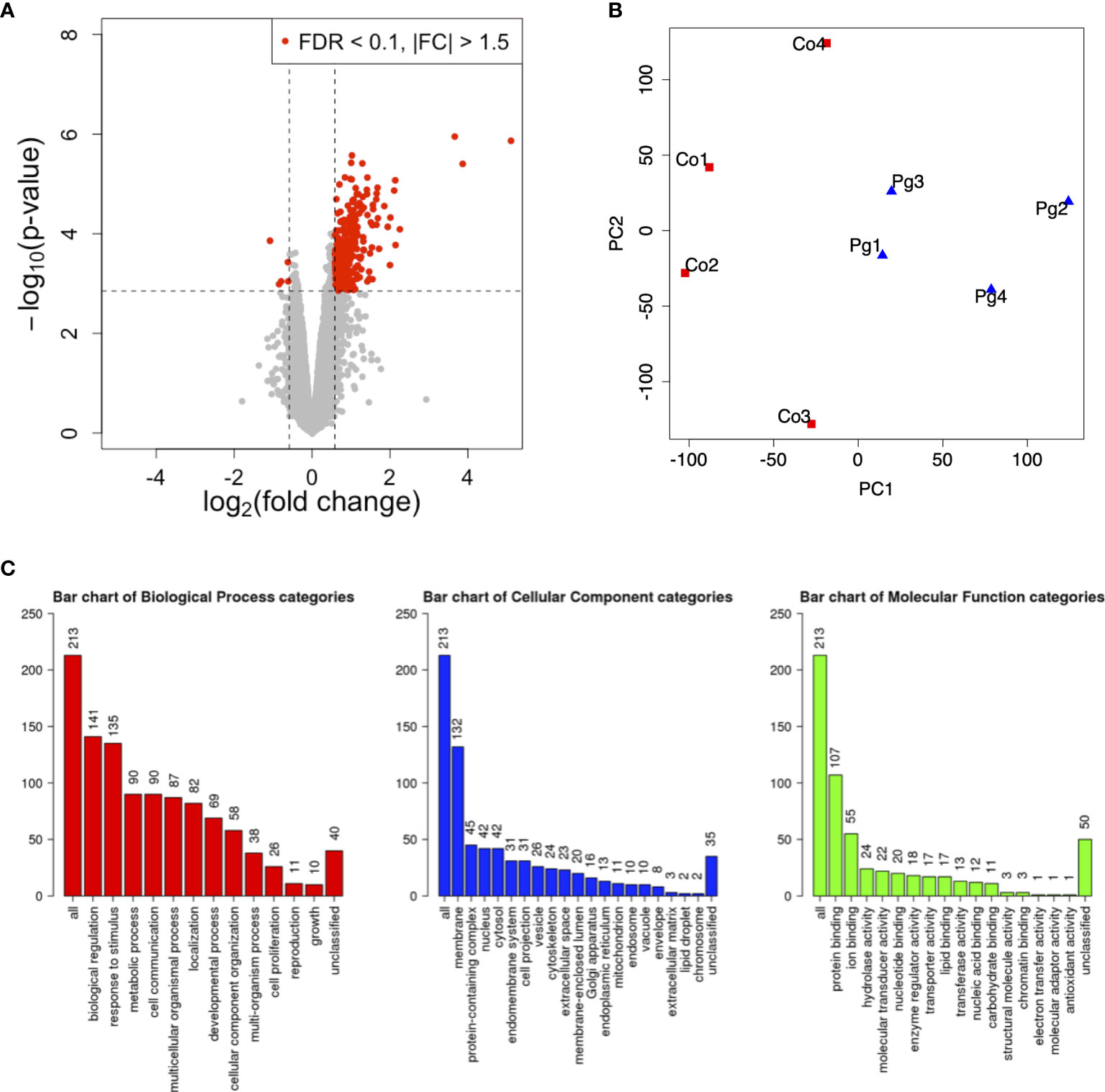
Figure 3 Microarray analysis in the liver between Co and Pg mice at ED 18 (n = 4). (A) Volcano plot; red plots show genes with FDR q < 0.1 and |fold change| > 1.5. (B) Principal component analysis. (C) Gene ontology in the upregulated DEGs.
KEGG pathway analysis from upregulated DEGs was then performed; significantly enriched pathways in the liver (P < 0.05, Bonferroni q < 0.1) are listed in Table 1. Notably, “Natural killer cell-mediated cytotoxicity” and “B cell receptor signaling pathways” were included. There was no significantly enriched pathway associated with the downregulated DEGs in the liver.
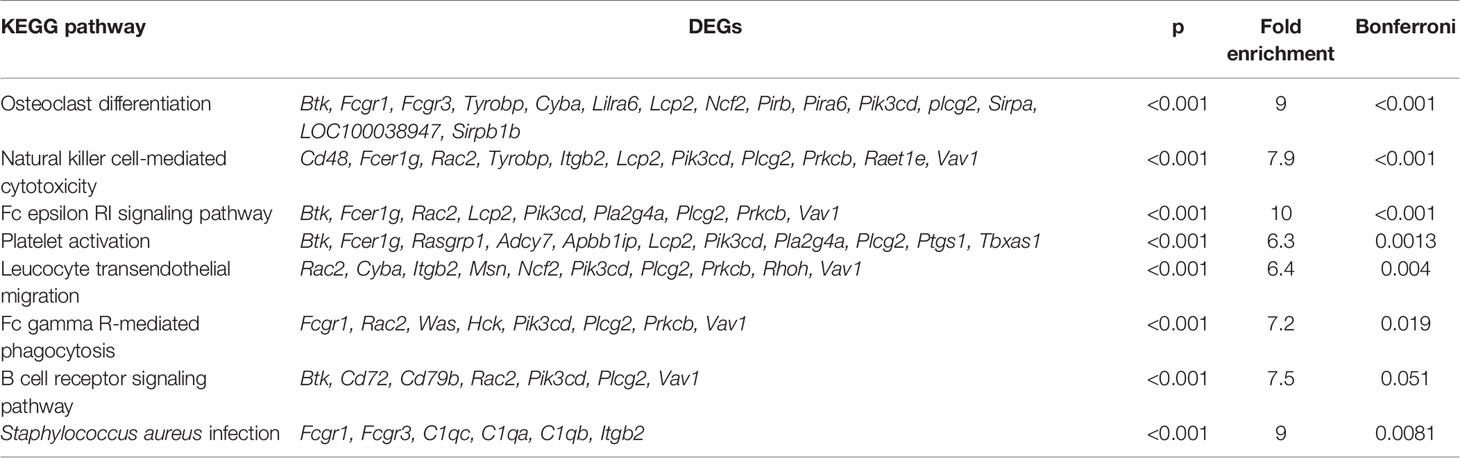
Table 1 Kyoto encyclopedia of genes and genomes pathways in the upregulated differentially expressed genes in the liver of Pg dams at ED 18.
The GO terms associated with the upregulated DEGs (P < 0.05 and Bonferroni q < 0.1) are listed in Table 2. Interestingly, many of these GO terms were related to the immune system, such as “Immune system process”, “Innate immune response”, “Adaptive immune response”, and “Acute-phase response”.
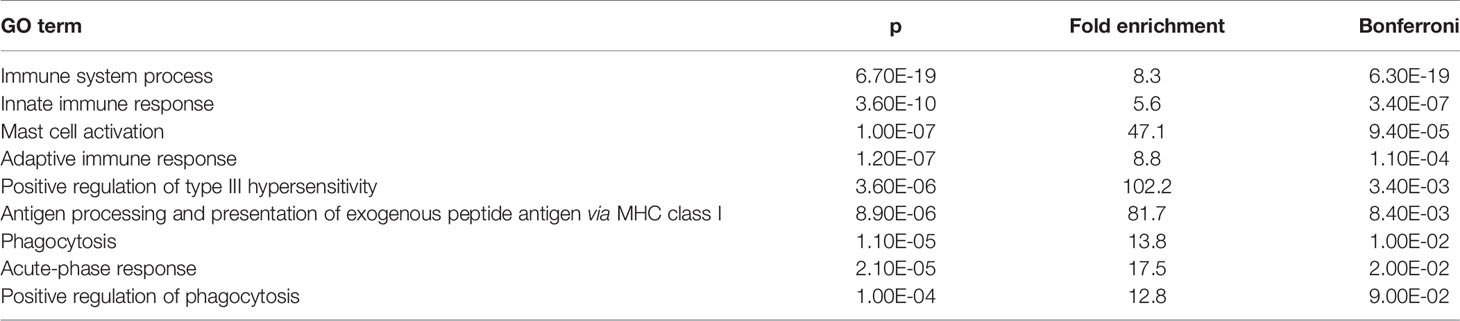
Table 2 Gene ontology terms in upregulated differentially expressed genes in the liver of Pg dams at ED 18.
GSEA was performed using hallmark gene sets to evaluate differences in the mRNA expression levels of various genes in the livers of the Co and Pg dams. Table 3 indicates the upregulated and downregulated gene sets with FDR q < 0.1. Several upregulated gene sets were related to inflammation, including inflammatory response set (Figure 4A, NES = 1.97, q < 0.001) and IL6/JAK/STAT3 signaling set (Figure 4B, NES = 1.53, q = 0.044). Interestingly, fatty acid metabolism gene set (Figure 5A, NES = -1.74, q = 0.011) and bile acid metabolism gene set (Figure 5B, NES = -1.64, q = 0.027) were downregulated.
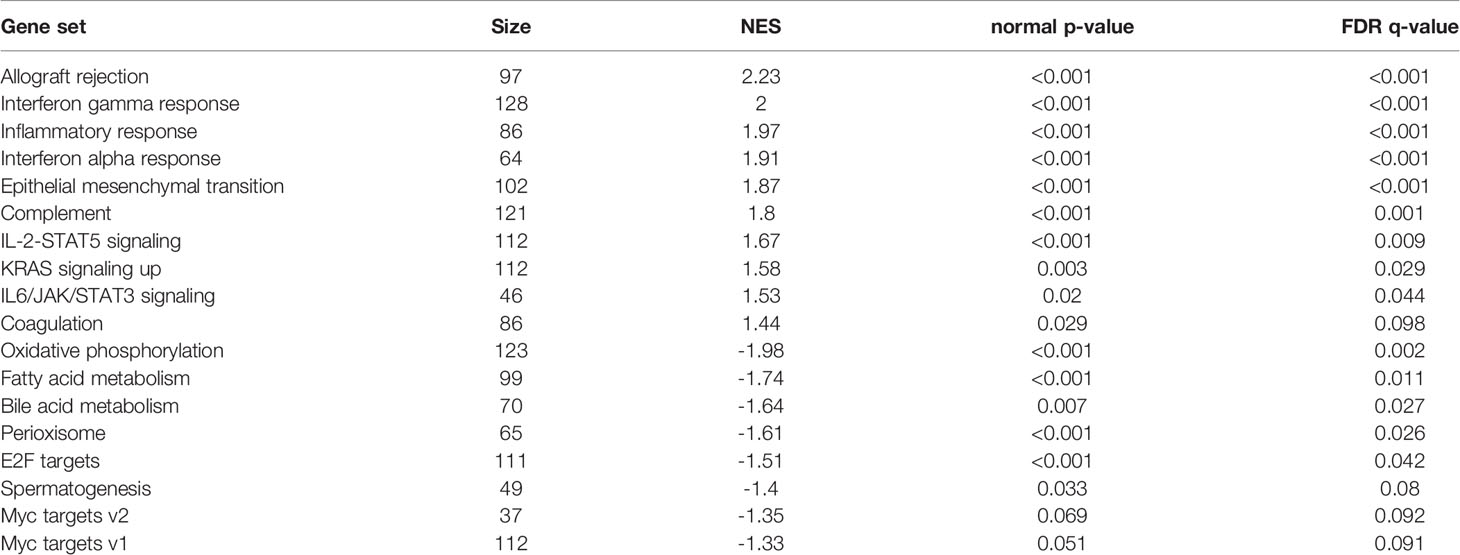
Table 3 Gene set enrichment analysis with hallmark gene sets enriched and downregulated in the liver of Pg dams at ED 18.
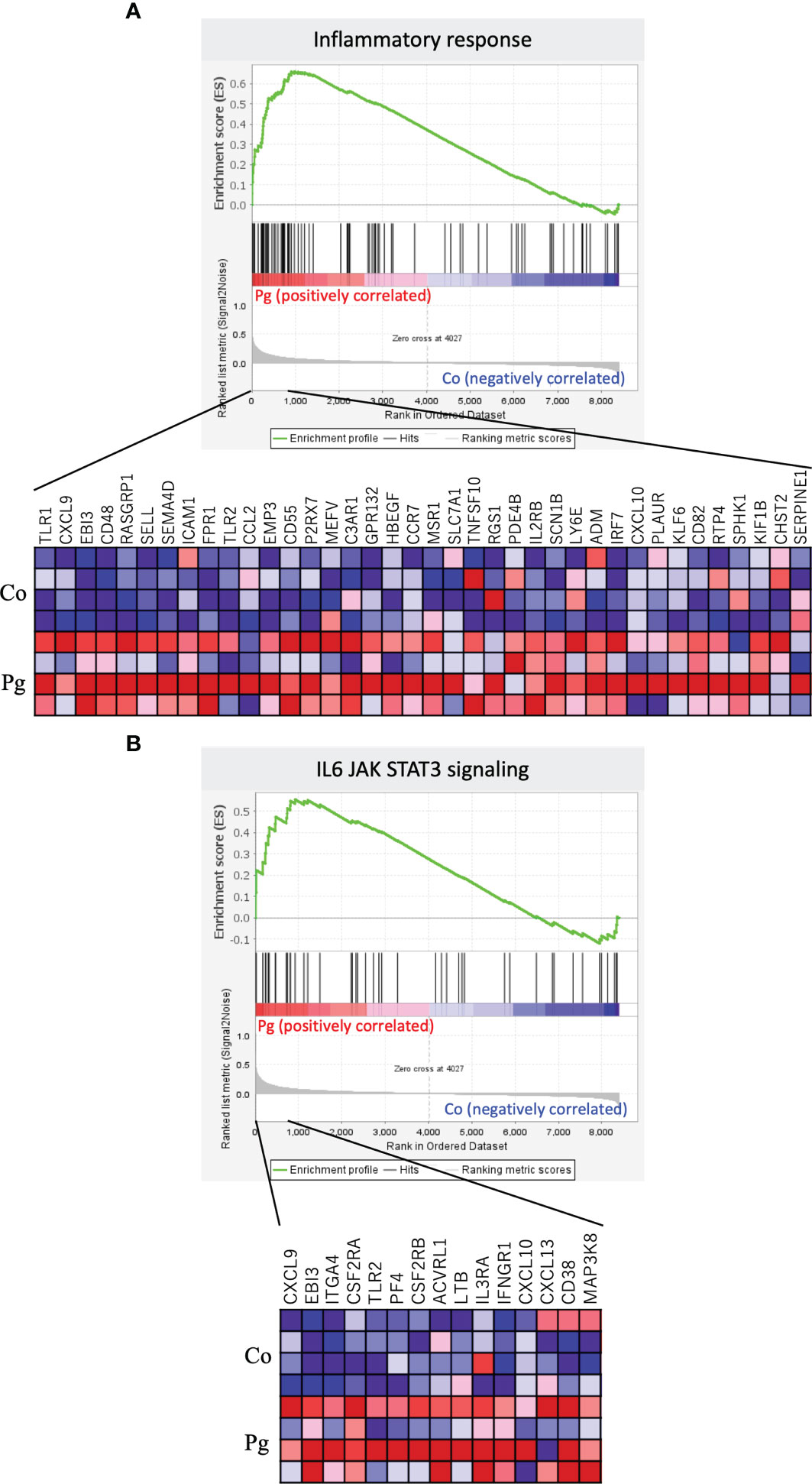
Figure 4 Notable gene sets enriched in the liver of Pg mice at ED 18 (n = 4). Gene sets about (A) Inflammatory response (NES = 1.97, q < 0.001) and (B) IL6/JAK/STAT3 signaling (NES = 1.53, q = 0.044). A heatmap provided illustrating gene expression levels for each gene in the core enrichment subset (blue: low, red: high).
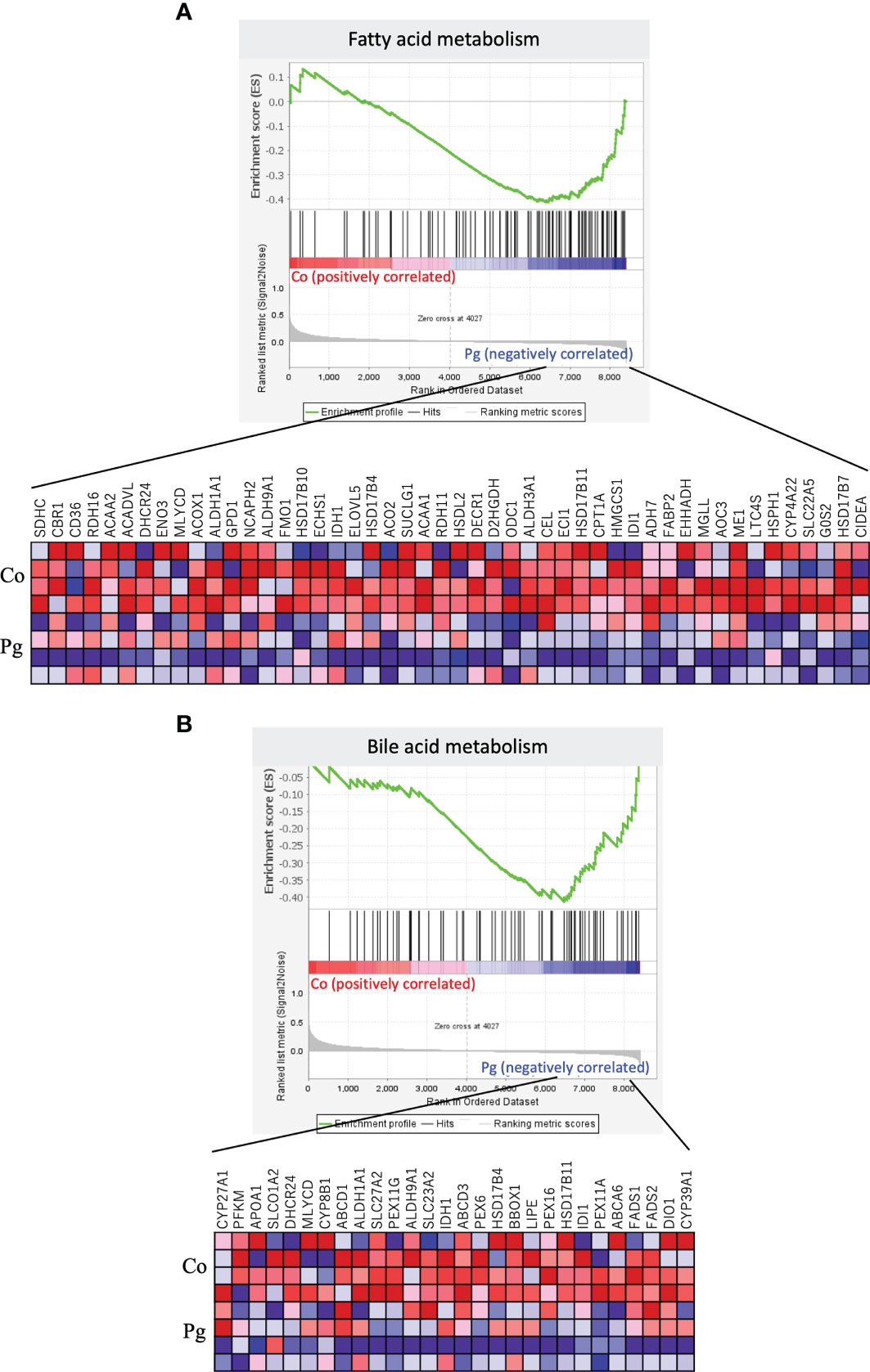
Figure 5 Notable gene sets downregulated in the liver of Pg mice at ED 18 (n = 4). Gene sets about (A) Fatty acid metabolism (NES = -1.74, q = 0.011) and (B) Bile acid metabolism (NES = -1.64, q = 0.027). A heatmap provided illustrating gene expression levels for each gene in the core enrichment subset (blue: low, red: high).
P. gingivalis Administration Altered the Gene Expression Patterns in the BAT of Dams
Microarray analysis was also performed in the BAT from pregnant mice at ED 18. All microarray data presented herein are available in the GEO database under GSE180115. Only 53 DEGs (|fold change| > 1.5 and q < 0.1) were found (14 DEGs, upregulated; 39 DEGs, downregulated; Figure 6A). However, the gene expression patterns differed substantially (Figure 6B). The GO term “Lipid catabolic process” was found in downregulated DEGs showing a P < 0.05 and Bonferroni q < 0.1 (Table 4).
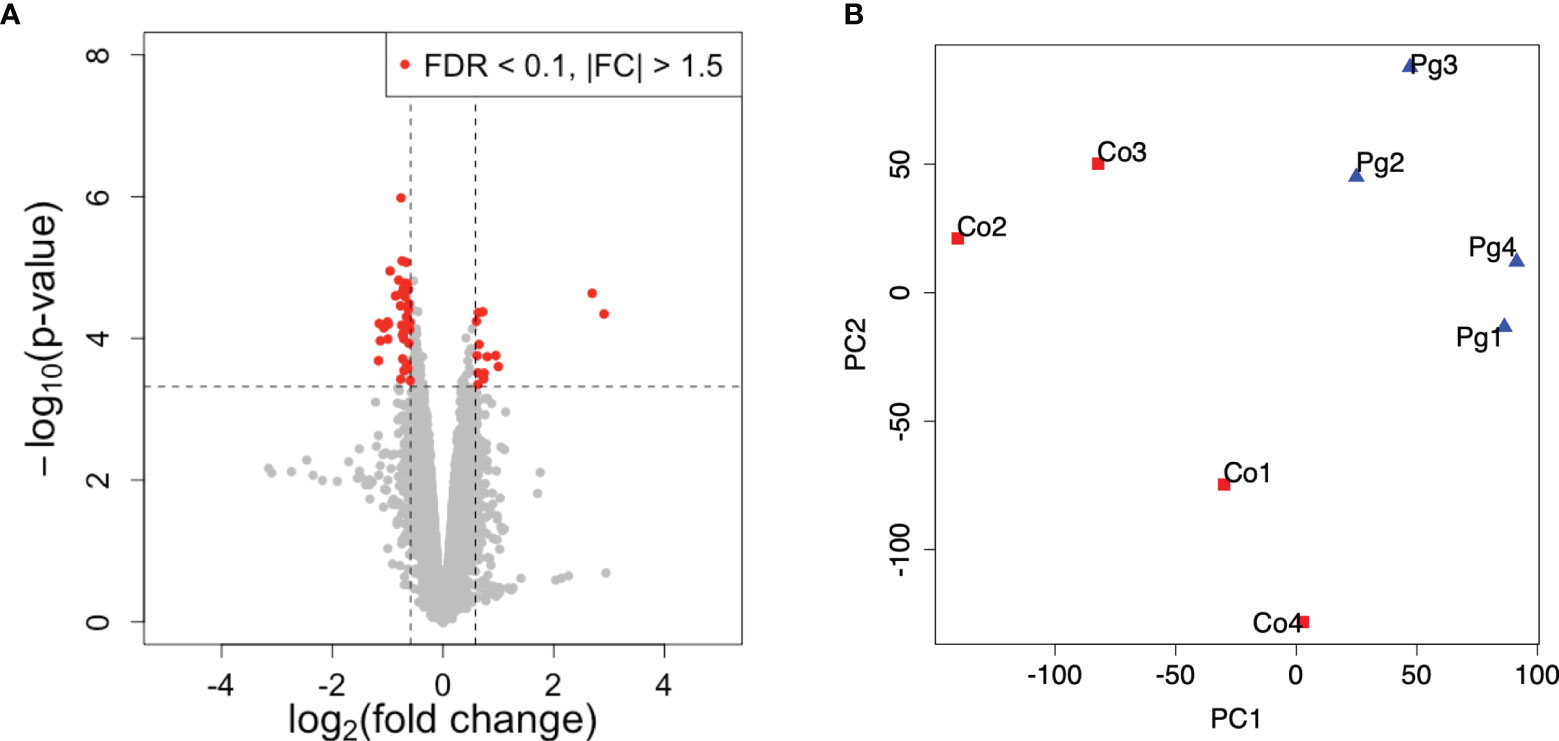
Figure 6 Microarray analysis in the brown adipose tissue between Co and Pg mice at ED 18 (n = 4). (A) Volcano plot; red plots show genes with FDR q < 0.1 and |fold change| > 1.5. (B) Principal component analysis.
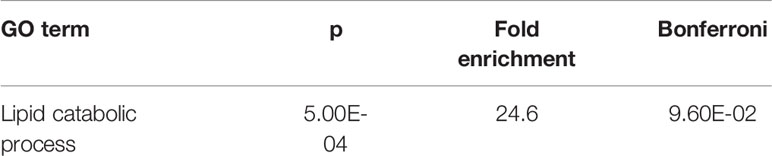
Table 4 Gene ontology terms in downregulated differentially expressed genes in BAT of Pg dams at ED 18.
GSEA was performed using hallmark gene sets to evaluate the differences between the mRNA expression patterns in the BAT of the Co and Pg dams. Enriched gene sets with FDR q < 0.1 are noted in Table 5. In accordance with the results of GSEA for the liver samples, the BAT of the Pg dams also showed the upregulation of gene sets associated with inflammation, including the inflammatory response gene set (Figure 7A, NES = 2.06, q < 0.001), the TNF-α signaling via NF-κB gene set (Figure 7B, NES = 1.70, q = 0.009), and the IL6/JAK/STAT3 signaling gene set (Figure 7C, NES = 1.53, q = 0.023). Surprisingly, the members of the estrogen response early/late gene sets were upregulated in the BAT of Pg dams (Figure 8A, NES = 1.71, q = 0.009; Figure 8B, NES = 1.71, q = 0.008).
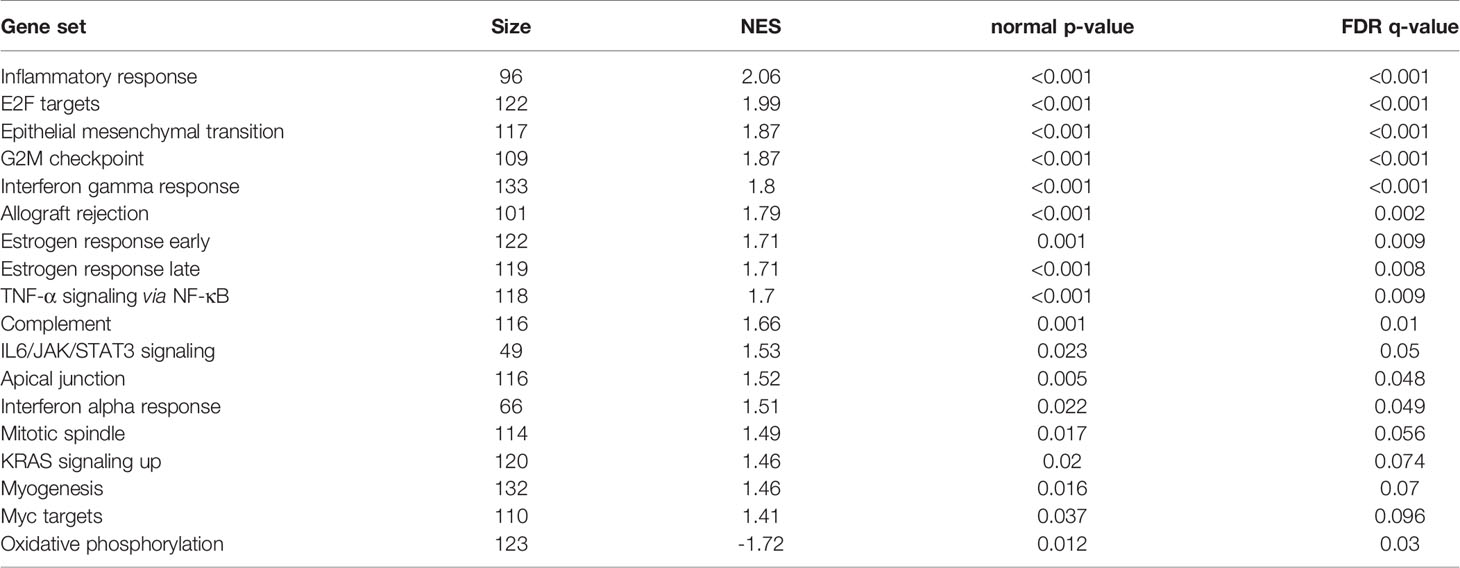
Table 5 Gene set enrichment analysis with hallmark gene sets enriched and downregulated in BAT of Pg dams at ED 18.
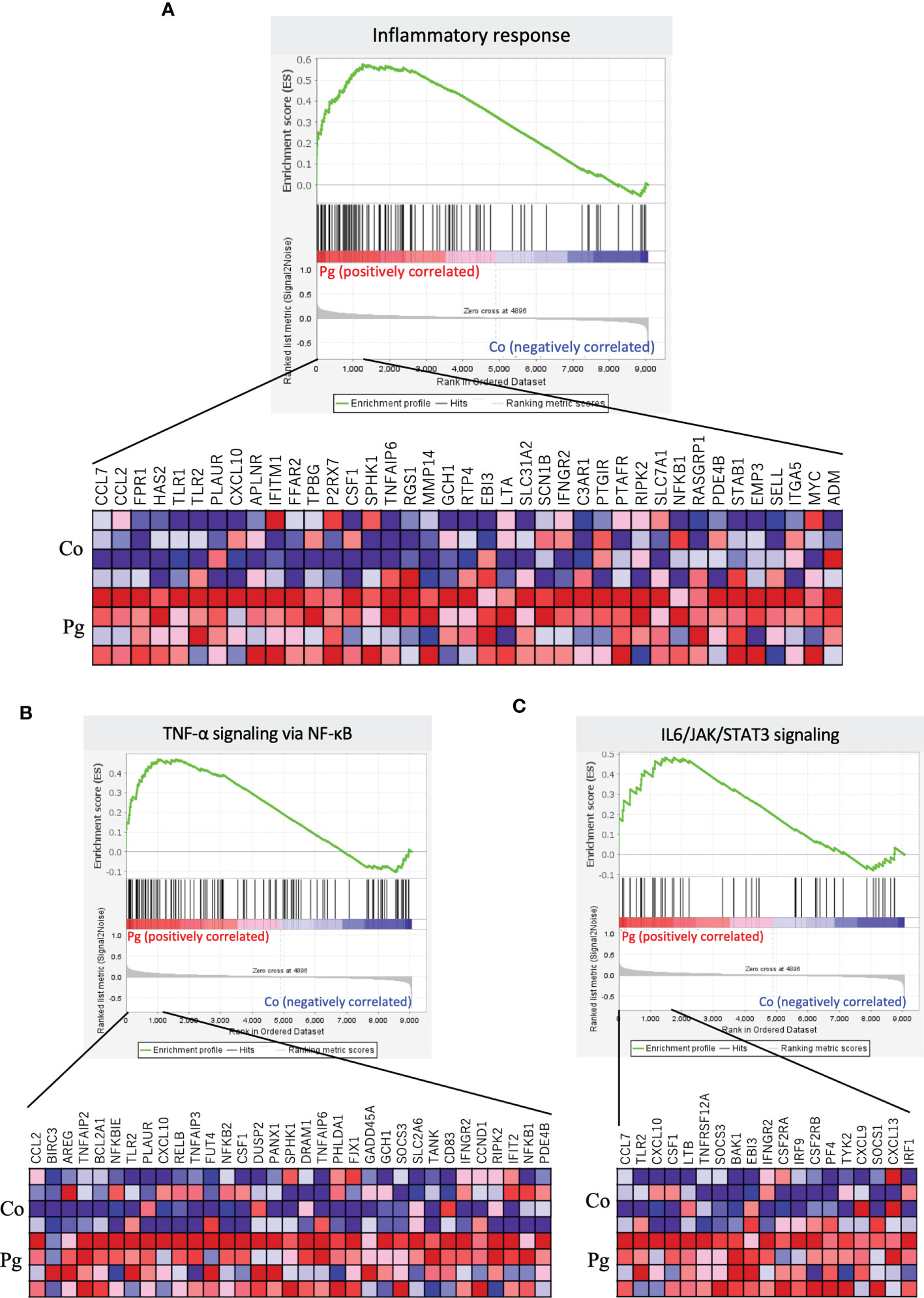
Figure 7 Notable gene sets enriched in the BAT of Pg mice at ED 18 (n = 4). Gene sets about (A) Inflammatory response (NES = 2.06, q < 0.001), (B) TNF-α signaling via NF-κB (NES = 1.70, q = 0.009), (C) IL6/JAK/STAT3 signaling (NES = 1.53, q = 0.023). A heatmap provided illustrating gene expression levels for each gene in the core enrichment subset (blue: low, red: high).
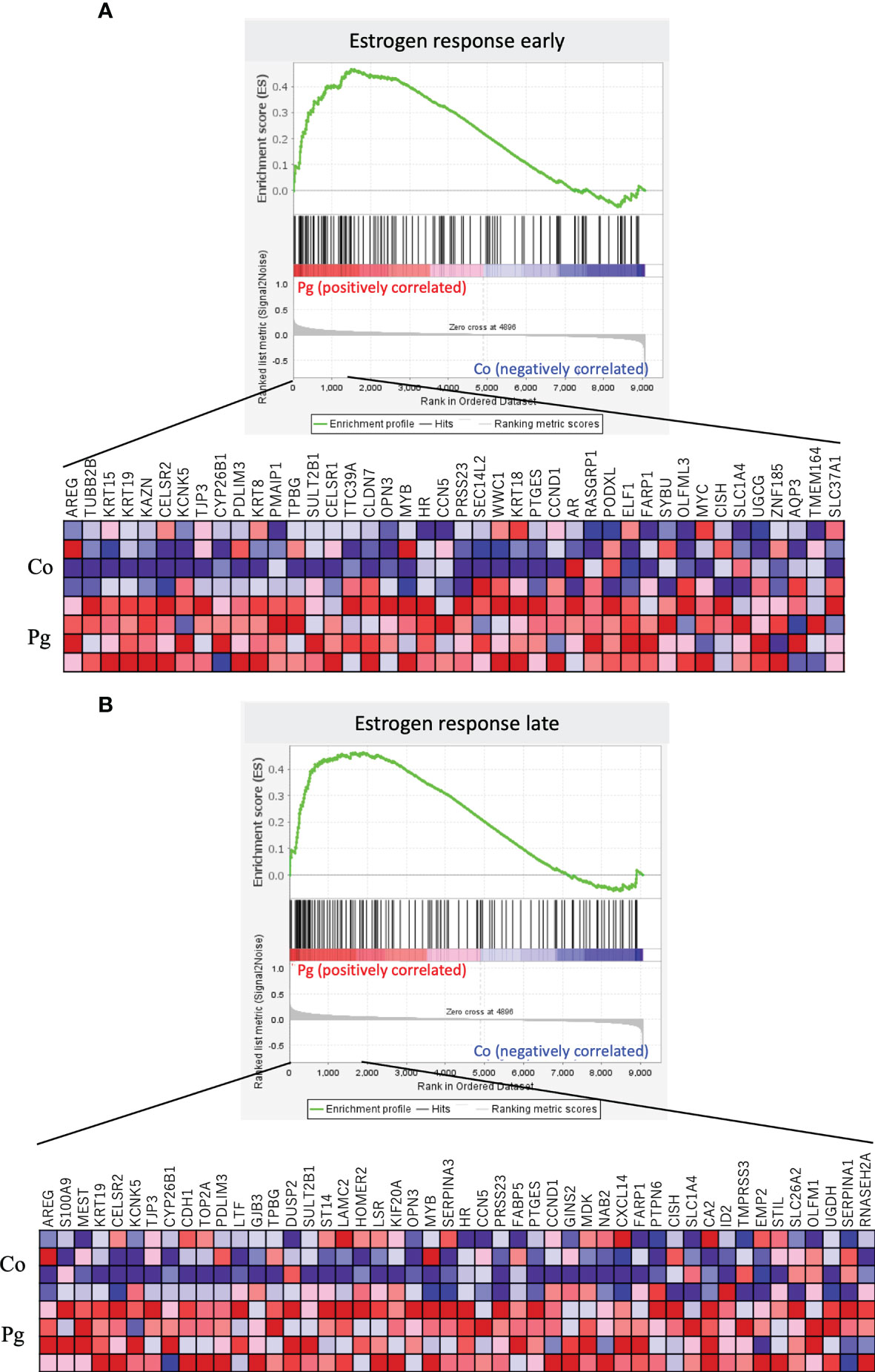
Figure 8 Notable gene sets enriched in the BAT of Pg mice at ED 18 (n = 4). Gene sets about (A) Estrogen response early (NES = 1.71, q = 0.009) and (B) Estrogen response late (NES = 1.71, q = 0.008). A heatmap provided illustrating gene expression levels for each gene in the core enrichment subset (blue: low, red: high).
P. gingivalis Administration Downregulated the Transcript Levels of Genes Involved in Lipid Synthesis and Metabolism
Genes encoding lipins, which are involved in lipid synthesis via their phosphatidate phosphatase activity and act as transcriptional coactivators to regulate fatty acid oxidation, were among the DEGs that were downregulated in the liver of the Pg dams. Therefore, the transcript levels of lipin protein-encoding genes and other related genes were investigated. The expression levels of the Lipin 1 (Lpin1) and Lipin 2 genes (Lpin2) were significantly downregulated in the liver of Pg dams. Similarly, the expression of another lipin protein-encoding gene, Lpin3, was decreased in the liver of Pg dams. Moreover, the expression level of the peroxisome proliferator-activated receptor-gamma coactivator 1-alpha (Ppargc1a), which is the master regulator of mitochondrial biogenesis and induces lipin expression, decreased in the liver of Pg dams (p = 0.054). Furthermore, the administration of P. gingivalis significantly decreased the gene expression of Lxra in the liver, which is related to triglyceride synthesis. Other key genes for triglyceride synthesis (Lxrb, Fasn, and Dgat2) were downregulated in the liver of Pg dams (p = 0.077, p = 0.088, p = 0.057, respectively, Figure 9A). Liver triglyceride was evaluated for the validation of results from the microarray analysis and qPCR. Liver triglyceride from Pg dams tended to be decreased (Figure 9B). Contrastingly, in the BAT of Pg mice, there was no significant change in the transcript levels of the genes encoding lipins or peroxisome proliferator-activated receptor-gamma coactivator 1-alpha (Figure 9C). However, there were no significant differences between the concentrations of plasma triglycerides in the Co and Pg dams (Figure 9D).
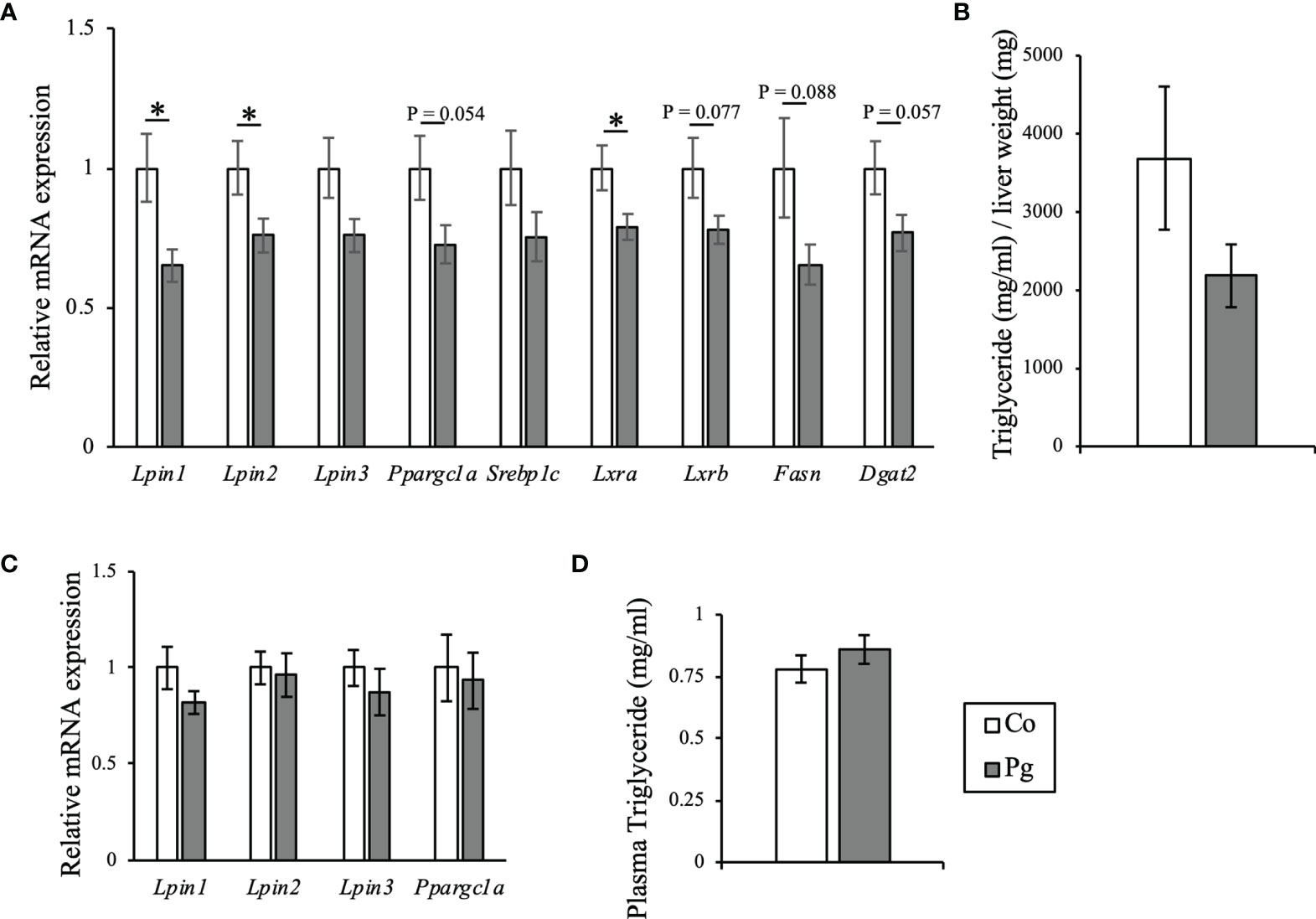
Figure 9 Evaluation of lipid metabolism in dams at ED 18. (A) Quantitative reverse-transcription PCR analysis in the liver. Lpin1, Lpin2, Lpin3, Ppargc1a, Srebp1c, Lxra, Lxrb, Fasn, and Dgat2 expression (n = 10, means ± SE, *P < 0.05). (B) Hepatic triglyceride of dams at ED 18 (n = 4, means ± SE). (C) Quantitative reverse-transcription PCR analysis in BAT. Lpin1, Lpin2, Lpin3 and Ppargc1a expression (n = 10, means ± SE). (D) Plasma triglyceride concentration of dams at ED 18 (n = 10, means ± SE, *P < 0.05).
Discussion
Maternal periodontal disease has been reported to be associated with adverse pregnancy outcomes (Offenbacher et al., 1996; Vergnes and Sixou, 2007; Ide and Papapanou, 2013; Corbella et al., 2016; Figuero et al., 2020). A previous clinical study reported that threatened preterm labor and preterm birth were notably associated with the periodontal disease (Ye et al., 2013). In addition, another previous study have shown that sonicated P. gingivalis intravenous injection induced low birth weight in mice (Udagawa et al., 2018). However, the physiological changes in P. gingivalis administered dams remain unclear; ascertaining this was the target of the present study.
In this study, pregnant mice were subjected to both the intravenous injection and oral administration of sonicated P. gingivalis from ED 2. As periodontal disease features both endotoxiemia and the swallowing of periodontal bacteria (Hajishengallis, 2015), the administration of P. gingivalis was performed both orally and intravenously to mimic the clinical condition in this study. In addition, because the gestational period in mice is short, the effect of P. gingivalis administration had to be induced within the limited period of administration. Thus, administering both orally and intravenously is suitable for experimental design. The detection rate of P. gingivalis from plaque or saliva increased markedly in the mothers diagnosed with threatened preterm labor (Ye et al., 2013). In addition, the anti-P. gingivalis IgG titer in serum has been significantly higher in mothers with threatened preterm labor with small-for-gestational-age offspring than in those with threatened preterm labor with appropriate-for-gestational-age offspring (Ye et al., 2020).
In the present study, maternal obesity was also induced by the intravenous and oral administration of sonicated P. gingivalis. Excessive weight gain during pregnancy represents a risk for adverse pregnancy and neonatal outcomes (Stotland et al., 2006; Voerman et al., 2019). A previous study wherein endotoxemia was induced by administering P. gingivalis to pregnant mice via the dental pulp chamber did not report a change in the body weight of mothers (Ao et al., 2015). However, in an epidemiologic study, maternal overweight/obesity was reported to be associated with periodontitis and insufficient birth weight (Foratori-Junior et al., 2020). It is plausible that endotoxemia or oral administration of P. gingivalis induces obesity; this has been well reported in many previous studies. Endotoxemia induced by the administration of sonicated P. gingivalis after feeding mice with high-fat diets increased the body weight and visceral/subcutaneous fat mass, aggravated liver steatosis, and caused impaired glucose tolerance and insulin resistance (Sasaki et al., 2018). Furthermore, just a single intravenous injection of the sonicated P. gingivalis altered the endocrine activities of BAT (Hatasa et al., 2021). Oral administration of P. gingivalis also caused impaired glucose tolerance and insulin resistance (Arimatsu et al., 2014; Watanabe et al., 2021). Therefore, the maternal weight gain observed herein suggests that similar alterations in metabolism were induced during pregnancy after P. gingivalis administration. Thus, increased visceral and subcutaneous fat mass may be leading causes of weight gain.
Gestational weight gain is regarded as a high-risk factor of large-for-gestational-age or macrosomia (Goldstein et al., 2017; Goldstein et al., 2018). Interestingly, despite the gestational weight gain observed among the dams in this study, the fetal body weight was lesser after P. gingivalis administration. Previous studies on the maternal administration of P. gingivalis also reported a low birth weight and the birth of small-for-gestational-age pups (Ao et al., 2015; Udagawa et al., 2018); this is consistent with the results of the present study. All three studies, including this study, conducted multiple or continuous administration of P. gingivalis through almost the entire gestational period, which resulted in causing a lower fetal weight (Ao et al., 2015; Udagawa et al., 2018).
Microarray analysis in the liver and BAT was performed to evaluate the gene expression and compared these results with those from previous studies conducted using male mice (Sasaki et al., 2018; Hatasa et al., 2021). In the liver, the number of DEGs, particularly downregulated DEGs, were lesser than those in a previous study; this may have resulted from the shorter period of bacterial administration (16 days vs. 12 weeks) and the state of pregnancy in the current study. In the GO analysis of the present study, 42% of upregulated DEGs in the liver enriched with GO terms were classified as “metabolic process” in the biological process category; the DEGs from the male mice in the previous study showed a similar tendency (Sasaki et al., 2018). In the liver, pathways and GO terms related to the innate immune system were upregulated, such as the KEGG pathway “Natural killer cell-mediated cytotoxicity”, and the GO terms “Innate system process”, “Innate immune response”, and “Acute-phase response”. Natural killer cells, which are activated by dendritic cells, may be the source of interferon-γ, and may thus, be responsible for the P. gingivalis-specific IgG2 production in the gingival crevicular fluid and serum (Kikuchi et al., 2005). Interestingly, in the present study, the members from the interferon-gamma response gene set were also upregulated in the liver. Neutrophils are important for the maintenance of periodontal tissues. However, periodontal bacteria can interfere with the complement function and neutrophil-mediated killing in periodontal tissues (Hajishengallis, 2015). The results of the present study suggest that the innate immune system was disrupted by P. gingivalis administration. With regard to the adaptive immune system, the KEGG pathway “B cell receptor signaling pathway” and the GO term “Adaptive immune response” were enriched in the liver from Pg dams. As the plasma anti-P. gingivalis IgG antibody titer was increased in dams with P. gingivalis-induced endotoxemia (Udagawa et al., 2018), B cells may be activated by P. gingivalis in the present model. The members from the inflammatory response and IL6/JAK/STAT3 signaling gene sets were upregulated in the liver, suggesting that the inflammation of the liver is induced as a response to bacterial administration. Systemic inflammation caused by periodontitis is regarded as one of the possible mechanisms underlying adverse pregnancy outcomes (Madianos et al., 2013; Figuero et al., 2020). Periodontal disease may mediate systemic inflammatory pathologies (Hajishengallis, 2015) and eventually trigger pregnancy-related complications. Interestingly, members from the fatty acid metabolism gene set were downregulated in the liver; these were reported to be upregulated in the aforementioned previous study conducted using male mice (Sasaki et al., 2018). The concentration of maternal blood lipids and the blood glucose level are important factors that influence fetal growth (Kulkarni et al., 2013). Maternal lipid metabolism changes drastically during pregnancy, as highlighted by multiple changes in lipoprotein metabolism caused by elevated maternal insulin resistance and estrogen levels in the third trimester, which is critical to fetal growth (Herrera, 2002; Lain and Catalano, 2007; Barrett et al., 2014). In addition, members of the bile acid metabolism gene set were also upregulated in the liver. Maternal bile acid metabolism is important for fetal development (Brouwers et al., 2015; Pataia et al., 2017); it enables the absorption and metabolism of nutrients and fat-soluble vitamins and regulates the body’s sugar levels, lipid levels, energy metabolism, and endocrine and detoxification effects (Li and Chiang, 2014). As the disorder of bile acid metabolism can be induced by the injection of lipopolysaccharides from Escherichia coli in pregnant mice (Zhang et al., 2020), sonicated P. gingivalis injection may also affect fetal growth via altered bile acid metabolism.
Unlike the white adipose tissue, BAT contributes to thermogenesis by regulating the consumption of glucose and fatty acids (Nedergaard and Cannon, 2010). Interestingly, the loss of phenotype, whitening, and lipid accumulation of BAT are important for the maintenance of the gestational metabolic environment and fetal growth (McIlvride et al., 2017). In the GO analysis, members from the lipid catabolism gene set were downregulated in the BAT. This suggests that the alteration of the BAT phenotype may have been caused, which in turn, resulted in metabolic disorders in the mother and impaired fetal development. Furthermore, members of gene sets associated with responses to estrogen, the hormone that shows elevated levels in the third trimester and causes insulin tolerance and hyperglycemia (Baz et al., 2016), were upregulated in the BAT. Similar to the findings of a previous study that used male mice (Hatasa et al., 2020), in the present study, the BAT of pregnant mice also showed the upregulation of gene sets associated with inflammatory responses, such as the inflammatory response gene set, TNF-α signaling via NF-κB gene set, and IL6/JAK/STAT3 signaling gene set, suggesting that inflammation was induced in the BAT after P. gingivalis administration.
To detect the key genes responsible for altering maternal metabolism and weight gain, DEGs in both tissues were examined. Lpin2, which was listed as a DEG in the liver, plays a crucial role in lipid metabolism, along with the other lipin protein family genes Lpin1 and Lpin3 (Reue and Zhang, 2008). Lpin1 mutation is responsible for lipodystrophy in fatty liver dystrophic mice (Péterfy et al., 2001). Lipin proteins function as lipid phosphatase enzymes to form diacylglycerol from phosphatidic acid for triglyceride synthesis (Han et al., 2006). Notably, Lpin1 also plays a critical role as a transcriptional coactivator for Ppargc1a, and activates fatty acid oxidation and mitochondrial oxidative phosphorylation while suppressing lipogenesis and lipoprotein secretion. The upregulation of Ppargc1a expression activates Lpin1, and Lpin1, in turn, increases peroxisome proliferator-activated receptor alpha (PPARa) activity via the transcriptional activation of the PPARa gene and the direct coactivation of PPARa in cooperation with Ppargc1a and p300 (Finck et al., 2006). The expression levels of Lpin1 and Lpin2 were significantly downregulated in the liver of Pg dams, along with the downregulation of Ppargc1a; this implies that P. gingivalis administration may alter lipid homeostasis. Lipopolysaccharide and inflammatory cytokines reduce the expression of Lpin1 (Lu et al., 2008; Meana et al., 2014); thus, the downregulation of Lpin1 in this study may have been induced by the lipopolysaccharides derived from P. gingivalis and/or inflammation. Along with Lpin 1 and Lpin 2, other key genes of triglyceride synthesis, Lxra, Lxrb, Fasn, and Dgat2 were also downregulated in Pg administered dams. Furthermore, hepatic triglyceride tended to be decreased in Pg dams. A drastic change in maternal lipid metabolism is vital for fetal growth and development. Body fat accumulation is increased in the early stage of pregnancy. Conversely, during the third trimester, the catabolic process is accelerated, thereby elevating serum triglyceride cholesterol level (Herrera, 2002). The increase in plasma triglyceride corresponds to triglycerides synthesized in the liver (Wasfi et al., 1980). Moreover, the alteration of Liver X receptors signaling in the liver, which has key roles in the regulation of lipid metabolism, influences pregnancy adaptations in lipogenesis and protects against dysregulated fetoplacental lipid homeostasis (Nikolova et al., 2017). In the present study, although the plasma concentration of triglyceride of Pg dams was not significantly altered, significant downregulation of gene expression of triglyceride synthesis and decreasing trend of triglyceride in the liver of Pg dams were observed. The alteration of lipid metabolism in the liver induced by P. gingivalis administration may influence fetal growth and development.
However, in the BAT, there was no difference in the expression levels of any lipin genes or Ppargc1a. In addition, fewer DEGs were detected in the BAT than in the liver. These results suggest that the effects of P. gingivalis administration may be critical to the liver, which primarily performs detoxification and is connected directly to the portal vein.
In conclusion, the administration of P. gingivalis to pregnant mice causes gestational weight gain in dams and causes lower body weight in fetuses. Moreover, P. gingivalis administration altered the gene expression profiles in the liver and BAT of dams, suggesting the presence of inflammatory responses, immune responses, and altered metabolism. To the best of our knowledge, this is the first study reporting maternal obesity in pregnant mice administered with sonicated P. gingivalis and providing a comprehensive evaluation of gene expression in the liver and BAT of pregnant mice administered with P. gingivalis.
Data Availability Statement
The original contributions presented in the study are included in the article/Supplementary Material. Further inquiries can be directed to the corresponding author.
Ethics Statement
The animal study was reviewed and approved by the Animal Care Committee of the Experimental Animal Center at Tokyo Medical and Dental University.
Author Contributions
SY performed most of the experiments and wrote the 1st draft of the manuscript. MH, YO, YTs, AL, HNii, KM, TShim, NS, SM, TShib, TH, TO, AH, RI, KN, YTo, HNit, TSu, HT, NM, and TI assisted in some studies and reviewed the manuscript. SK supervised all the studies and the writing of the manuscript. All authors contributed to the article and approved the submitted version.
Funding
This work was supported by the Japan Society for the Promotion of Science (20H03863 to SK, 20K23020 to YTs, 19K24062 to NS, 19K18989 to SM, and 19K19016 to TShib).
Conflict of Interest
The authors declare that the research was conducted in the absence of any commercial or financial relationships that could be construed as a potential conflict of interest.
Publisher’s Note
All claims expressed in this article are solely those of the authors and do not necessarily represent those of their affiliated organizations, or those of the publisher, the editors and the reviewers. Any product that may be evaluated in this article, or claim that may be made by its manufacturer, is not guaranteed or endorsed by the publisher.
Acknowledgments
The authors would like to thank Prof. Masami Kanai, as well as the staff at the TMDU’s experimental animal center for their support.
Supplementary Material
The Supplementary Material for this article can be found online at: https://www.frontiersin.org/articles/10.3389/fcimb.2021.745117/full#supplementary-material
References
Abariga, S. A., Whitcomb, B. W. (2016). Periodontitis and Gestational Diabetes Mellitus: A Systematic Review and Meta-Analysis of Observational Studies. BMC Pregnancy. Childbirth. 16 (1), 344. doi: 10.1186/s12884-016-1145-z
Ao, M., Miyauchi, M., Furusho, H., Inubushi, T., Kitagawa, M., Nagasaki, A., et al. (2015). Dental Infection of Porphyromonas Gingivalis Induces Preterm Birth in Mice. PloS One 10 (8), e0137249. doi: 10.1371/journal.pone.0137249
Arimatsu, K., Yamada, H., Miyazawa, H., Minagawa, T., Nakajima, M., Ryder, M. I., et al. (2014). Oral Pathobiont Induces Systemic Inflammation and Metabolic Changes Associated With Alteration of Gut Microbiota. Sci. Rep. 4, 4828. doi: 10.1038/srep04828
Barrett, H. L., Dekker Nitert, M., McIntyre, H. D., Callaway, L. K. (2014). Normalizing Metabolism in Diabetic Pregnancy: Is it Time to Target Lipids? Diabetes Care 37 (5), 1484–1493. doi: 10.2337/dc13-1934
Baz, B., Riveline, J. P., Gautier, J. F. (2016). ENDOCRINOLOGY OF PREGNANCY: Gestational Diabetes Mellitus: Definition, Aetiological and Clinical Aspects. Eur. J. Endocrinol. 174 (2), R43–R51. doi: 10.1530/eje-15-0378
Blasco-Baque, V., Garidou, L., Pomié, C., Escoula, Q., Loubieres, P., Le Gall-David, S., et al. (2017). Periodontitis Induced by Porphyromonas Gingivalis Drives Periodontal Microbiota Dysbiosis and Insulin Resistance via an Impaired Adaptive Immune Response. Gut 66 (5), 872–885. doi: 10.1136/gutjnl-2015-309897
Brouwers, L., Koster, M. P., Page-Christiaens, G. C., Kemperman, H., Boon, J., Evers, I. M., et al. (2015). Intrahepatic Cholestasis of Pregnancy: Maternal and Fetal Outcomes Associated With Elevated Bile Acid Levels. Am. J. Obstet. Gynecol. 212 (1), 100.e101–107. doi: 10.1016/j.ajog.2014.07.026
Catalano, P. M., Shankar, K. (2017). Obesity and Pregnancy: Mechanisms of Short Term and Long Term Adverse Consequences for Mother and Child. Bmj 356, j1. doi: 10.1136/bmj.j1
Corbella, S., Taschieri, S., Del Fabbro, M., Francetti, L., Weinstein, R., Ferrazzi, E. (2016). Adverse Pregnancy Outcomes and Periodontitis: A Systematic Review and Meta-Analysis Exploring Potential Association. Quintessence. Int. 47 (3), 193–204. doi: 10.3290/j.qi.a34980
Figuero, E., Han, Y. W., Furuichi, Y. (2020). Periodontal Diseases and Adverse Pregnancy Outcomes: Mechanisms. Periodontol. 2000. 83 (1), 175–188. doi: 10.1111/prd.12295
Finck, B. N., Gropler, M. C., Chen, Z., Leone, T. C., Croce, M. A., Harris, T. E., et al. (2006). Lipin 1 Is an Inducible Amplifier of the Hepatic PGC-1alpha/PPARalpha Regulatory Pathway. Cell Metab. 4 (3), 199–210. doi: 10.1016/j.cmet.2006.08.005
Foratori-Junior, G. A., Jesuino, B. G., Caracho, R. A., Orenha, E. S., Groppo, F. C., Sales-Peres, S. H. C. (2020). Association Between Excessive Maternal Weight, Periodontitis During the Third Trimester of Pregnancy, and Infants’ Health at Birth. J. Appl. Oral. Sci. 28, e20190351. doi: 10.1590/1678-7757-2019-0351
Fujii, N., Ho, R. C., Manabe, Y., Jessen, N., Toyoda, T., Holland, W. L., et al. (2008). Ablation of AMP-Activated Protein Kinase Alpha2 Activity Exacerbates Insulin Resistance Induced by High-Fat Feeding of Mice. Diabetes 57 (11), 2958–2966. doi: 10.2337/db07-1187
Genco, R. J., Borgnakke, W. S. (2020). Diabetes as a Potential Risk for Periodontitis: Association Studies. Periodontol. 2000. 83 (1), 40–45. doi: 10.1111/prd.12270
Goldstein, R. F., Abell, S. K., Ranasinha, S., Misso, M., Boyle, J. A., Black, M. H., et al. (2017). Association of Gestational Weight Gain With Maternal and Infant Outcomes: A Systematic Review and Meta-Analysis. Jama 317 (21), 2207–2225. doi: 10.1001/jama.2017.3635
Goldstein, R. F., Abell, S. K., Ranasinha, S., Misso, M. L., Boyle, J. A., Harrison, C. L., et al. (2018). Gestational Weight Gain Across Continents and Ethnicity: Systematic Review and Meta-Analysis of Maternal and Infant Outcomes in More Than One Million Women. BMC Med. 16 (1), 153. doi: 10.1186/s12916-018-1128-1
Hajishengallis, G. (2015). Periodontitis: From Microbial Immune Subversion to Systemic Inflammation. Nat. Rev. Immunol. 15 (1), 30–44. doi: 10.1038/nri3785
Han, G. S., Wu, W. I., Carman, G. M. (2006). The Saccharomyces Cerevisiae Lipin Homolog Is a Mg2+-Dependent Phosphatidate Phosphatase Enzyme. J. Biol. Chem. 281 (14), 9210–9218. doi: 10.1074/jbc.M600425200
Hatasa, M., Ohsugi, Y., Katagiri, S., Yoshida, S., Niimi, H., Morita, K., et al. (2020). Endotoxemia by Porphyromonas Gingivalis Alters Endocrine Functions in Brown Adipose Tissue. Front. Cell Infect. Microbiol. 10, 580577. doi: 10.3389/fcimb.2020.580577
Hatasa, M., Yoshida, S., Takahashi, H., Tanaka, K., Kubotsu, Y., Ohsugi, Y., et al. (2021). Relationship Between NAFLD and Periodontal Disease From the View of Clinical and Basic Research, and Immunological Response. Int. J. Mol. Sci. 22 (7), 3728. doi: 10.3390/ijms22073728
Herrera, E. (2002). Lipid Metabolism in Pregnancy and its Consequences in the Fetus and Newborn. Endocrine 19 (1), 43–55. doi: 10.1385/endo:19:1:43
Ide, M., Papapanou, P. N. (2013>). Epidemiology of Association Between Maternal Periodontal Disease and Adverse Pregnancy Outcomes–Systematic Review. J. Clin. Periodontol. 40 (Suppl 14), S181–S194. doi: 10.1111/jcpe.12063
Kikuchi, T., Willis, D. L., Liu, M., Purkall, D. B., Sukumar, S., Barbour, S. E., et al. (2005). Dendritic-NK Cell Interactions in P. Gingivalis-Specific Responses. J. Dent. Res. 84 (9), 858–862. doi: 10.1177/154405910508400915
Kocher, T., König, J., Borgnakke, W. S., Pink, C., Meisel, P. (2018). Periodontal Complications of Hyperglycemia/Diabetes Mellitus: Epidemiologic Complexity and Clinical Challenge. Periodontol. 2000. 78 (1), 59–97. doi: 10.1111/prd.12235
Komazaki, R., Katagiri, S., Takahashi, H., Maekawa, S., Shiba, T., Takeuchi, Y., et al. (2017). Periodontal Pathogenic Bacteria, Aggregatibacter Actinomycetemcomitans Affect Non-Alcoholic Fatty Liver Disease by Altering Gut Microbiota and Glucose Metabolism. Sci. Rep. 7 (1), 13950. doi: 10.1038/s41598-017-14260-9
Kulkarni, S. R., Kumaran, K., Rao, S. R., Chougule, S. D., Deokar, T. M., Bhalerao, A. J., et al. (2013). Maternal Lipids Are as Important as Glucose for Fetal Growth: Findings From the Pune Maternal Nutrition Study. Diabetes Care 36 (9), 2706–2713. doi: 10.2337/dc12-2445
Lain, K. Y., Catalano, P. M. (2007). Metabolic Changes in Pregnancy. Clin. Obstet. Gynecol. 50 (4), 938–948. doi: 10.1097/GRF.0b013e31815a5494
Le Marchand, Y., Singh, A., Assimacopoulos-Jeannet, F., Orci, L., Rouiller, C., Jeanrenaud, B. (1973). A Role for the Microtubular System in the Release of Very Low Density Lipoproteins by Perfused Mouse Livers. J. Biol. Chem. 248 (19), 6862–6870. doi: 10.1016/S0021-9258(19)43430-3
Li, T., Chiang, J. Y. (2014). Bile Acid Signaling in Metabolic Disease and Drug Therapy. Pharmacol. Rev. 66 (4), 948–983. doi: 10.1124/pr.113.008201
Liberzon, A., Birger, C., Thorvaldsdottir, H., Ghandi, M., Mesirov, J. P., Tamayo, P. (2015). The Molecular Signatures Database (MSigDB) Hallmark Gene Set Collection. Cell Syst. 1 (6), 417–425. doi: 10.1016/j.cels.2015.12.004
Liu, L., Oza, S., Hogan, D., Chu, Y., Perin, J., Zhu, J., et al. (2016). Global, Regional, and National Causes of Under-5 Mortality in 2000–15: An Updated Systematic Analysis With Implications for the Sustainable Development Goals. Lancet 388 (10063), 3027–3035. doi: 10.1016/S0140-6736(16)31593-8
Lu, B., Lu, Y., Moser, A. H., Shigenaga, J. K., Grunfeld, C., Feingold, K. R. (2008). LPS and Proinflammatory Cytokines Decrease Lipin-1 in Mouse Adipose Tissue and 3T3-L1 Adipocytes. Am. J. Physiol. Endocrinol. Metab. 295 (6), E1502–E1509. doi: 10.1152/ajpendo.90323.2008
Madianos, P. N., Bobetsis, Y. A., Offenbacher, S. (2013). Adverse Pregnancy Outcomes (APOs) and Periodontal Disease: Pathogenic Mechanisms. J. Periodontol. 84 (4 Suppl), S170–S180. doi: 10.1902/jop.2013.1340015
McIlvride, S., Mushtaq, A., Papacleovoulou, G., Hurling, C., Steel, J., Jansen, E., et al. (2017). A Progesterone-Brown Fat Axis Is Involved in Regulating Fetal Growth. Sci. Rep. 7 (1), 10671. doi: 10.1038/s41598-017-10979-7
Meana, C., Peña, L., Lordén, G., Esquinas, E., Guijas, C., Valdearcos, M., et al. (2014). Lipin-1 Integrates Lipid Synthesis With Proinflammatory Responses During TLR Activation in Macrophages. J. Immunol. 193 (9), 4614–4622. doi: 10.4049/jimmunol.1400238
Nedergaard, J., Cannon, B. (2010). The Changed Metabolic World With Human Brown Adipose Tissue: Therapeutic Visions. Cell Metab. 11 (4), 268–272. doi: 10.1016/j.cmet.2010.03.007
Nikolova, V., Papacleovoulou, G., Bellafante, E., Manna, L. B., Jansen, E., Baron, S., et al. (2017). Changes in LXR Signaling Influence Early-Pregnancy Lipogenesis and Protect Against Dysregulated Fetoplacental Lipid Homeostasis. Am. J. Physiology-Endocrinol. Metab. 313 (4), E463–E472. doi: 10.1152/ajpendo.00449.2016
Offenbacher, S., Katz, V., Fertik, G., Collins, J., Boyd, D., Maynor, G., et al. (1996). Periodontal Infection as a Possible Risk Factor for Preterm Low Birth Weight. J. Periodontol. 67 Suppl 10S, 1103–1113. doi: 10.1902/jop.1996.67.10s.1103
Olsen, I., Yamazaki, K. (2019). Can Oral Bacteria Affect the Microbiome of the Gut? J. Oral. Microbiol. 11 (1), 1586422. doi: 10.1080/20002297.2019.1586422
Pataia, V., Dixon, P. H., Williamson, C. (2017). Pregnancy and Bile Acid Disorders. Am. J. Physiol. Gastrointest. Liver. Physiol. 313 (1), G1–g6. doi: 10.1152/ajpgi.00028.2017
Péterfy, M., Phan, J., Xu, P., Reue, K. (2001). Lipodystrophy in the Fld Mouse Results From Mutation of a New Gene Encoding a Nuclear Protein, Lipin. Nat. Genet. 27 (1), 121–124. doi: 10.1038/83685
Pihlstrom, B. L., Michalowicz, B. S., Johnson, N. W. (2005). Periodontal Diseases. Lancet 366 (9499), 1809–1820. doi: 10.1016/s0140-6736(05)67728-8
Polak, D., Sanui, T., Nishimura, F., Shapira, L. (2020). Diabetes as a Risk Factor for Periodontal Disease-Plausible Mechanisms. Periodontol. 2000. 83 (1), 46–58. doi: 10.1111/prd.12298
Poston, L., Caleyachetty, R., Cnattingius, S., Corvalán, C., Uauy, R., Herring, S., et al. (2016). Preconceptional and Maternal Obesity: Epidemiology and Health Consequences. Lancet Diabetes Endocrinol. 4 (12), 1025–1036. doi: 10.1016/s2213-8587(16)30217-0
Reue, K., Zhang, P. (2008). The Lipin Protein Family: Dual Roles in Lipid Biosynthesis and Gene Expression. FEBS Lett. 582 (1), 90–96. doi: 10.1016/j.febslet.2007.11.014
Ritchie, M. E, Phipson, B., Wu, D., Hu, Y., Law, C. W., Shi, W., et al. (2015). Limma Powers Differential Expression Analyses for RNA-Sequencing and Microarray Studies. Nucleic Acids Res. 43 (7), e47. doi: 10.1093/nar/gkv007
Saito, T., Shimazaki, Y., Sakamoto, M. (1998). Obesity and Periodontitis. N. Engl. J. Med. 339 (7), 482–483. doi: 10.1056/nejm199808133390717
Sasaki, N., Katagiri, S., Komazaki, R., Watanabe, K., Maekawa, S., Shiba, T., et al. (2018). Endotoxemia by Porphyromonas Gingivalis Injection Aggravates Non-Alcoholic Fatty Liver Disease, Disrupts Glucose/Lipid Metabolism, and Alters Gut Microbiota in Mice. Front. Microbiol. 9, 2470. doi: 10.3389/fmicb.2018.02470
Say, L., Chou, D., Gemmill, A., Tunçalp, Ö., Moller, A.-B., Daniels, J., et al. (2014). Global Causes of Maternal Death: A WHO Systematic Analysis. Lancet Global Health 2 (6), e323–e333. doi: 10.1016/S2214-109X(14)70227-X
Stotland, N. E., Cheng, Y. W., Hopkins, L. M., Caughey, A. B. (2006). Gestational Weight Gain and Adverse Neonatal Outcome Among Term Infants. Obstet. Gynecol. 108 (3 Pt 1), 635–643. doi: 10.1097/01.AOG.0000228960.16678.bd
Subramanian, A., Tamayo, P., Mootha, V. K., Mukherjee, S., Ebert, B. L., Gillette, M. A., et al. (2005). Gene Set Enrichment Analysis: a Knowledge-Based Approach for Interpreting Genome-Wide Expression Profiles. Proc. Natl. Acad. Sci. U. S. A 102 (43), 15545–15550. doi: 10.1073/pnas.0506580102
Udagawa, S., Katagiri, S., Maekawa, S., Takeuchi, Y., Komazaki, R., Ohtsu, A., et al. (2018). Effect of Porphyromonas Gingivalis Infection in the Placenta and Umbilical Cord in Pregnant Mice With Low Birth Weight. Acta Odontol. Scand. 76 (6), 433–441. doi: 10.1080/00016357.2018.1426876
Vergnes, J. N., Sixou, M. (2007). Preterm Low Birth Weight and Maternal Periodontal Status: A Meta-Analysis. Am. J. Obstet. Gynecol. 196 (2), 135.e131–137. doi: 10.1016/j.ajog.2006.09.028
Vivares-Builes, A. M., Rangel-Rincón, L. J., Botero, J. E., Agudelo-Suárez, A. A. (2018). Gaps in Knowledge About the Association Between Maternal Periodontitis and Adverse Obstetric Outcomes: An Umbrella Review. J. Evid. Based. Dent. Pract. 18 (1), 1–27. doi: 10.1016/j.jebdp.2017.07.006
Voerman, E., Santos, S., Inskip, H., Amiano, P., Barros, H., Charles, M. A., et al. (2019). Association of Gestational Weight Gain With Adverse Maternal and Infant Outcomes. JAMA 321 (17), 1702–1715. doi: 10.1001/jama.2019.3820
Wang, J., Duncan, D., Shi, Z., Zhang, B. (2013). WEB-Based GEne SeT AnaLysis Toolkit (WebGestalt): Update 2013. Nucleic Acids Res. 41, W77–W83. doi: 10.1093/nar/gkt439
Wasfi, I., Weinstein, I., Heimberg, M. (1980). Increased Formation of Triglyceride From Oleate in Perfused Livers From Pregnant Rats*. Endocrinology 107 (2), 584–590. doi: 10.1210/endo-107-2-584
Watanabe, K., Katagiri, S., Takahashi, H., Sasaki, N., Maekawa, S., Komazaki, R., et al. (2021). Porphyromonas Gingivalis Impairs Glucose Uptake in Skeletal Muscle Associated With Altering Gut Microbiota. FASEB J. 35 (2), e21171. doi: 10.1096/fj.202001158R
Westbrook, R. H., Dusheiko, G., Williamson, C. (2016). Pregnancy and Liver Disease. J. Hepatol. 64 (4), 933–945. doi: 10.1016/j.jhep.2015.11.030
Ye, C., Katagiri, S., Miyasaka, N., Bharti, P., Kobayashi, H., Takeuchi, Y., et al. (2013). The Anti-Phospholipid Antibody-Dependent and Independent Effects of Periodontopathic Bacteria on Threatened Preterm Labor and Preterm Birth. Arch. Gynecol. Obstet. 288 (1), 65–72. doi: 10.1007/s00404-013-2741-z
Ye, C., Katagiri, S., Miyasaka, N., Kobayashi, H., Khemwong, T., Nagasawa, T., et al. (2020). The Periodontopathic Bacteria in Placenta, Saliva and Subgingival Plaque of Threatened Preterm Labor and Preterm Low Birth Weight Cases: A Longitudinal Study in Japanese Pregnant Women. Clin. Oral. Investig. 24 (12), 4261–4270. doi: 10.1007/s00784-020-03287-4
Keywords: periodontal disease, Porphyromonas gingivalis, small for gestational age, gestational obesity, liver, brown adipose tissue
Citation: Yoshida S, Hatasa M, Ohsugi Y, Tsuchiya Y, Liu A, Niimi H, Morita K, Shimohira T, Sasaki N, Maekawa S, Shiba T, Hirota T, Okano T, Hirose A, Ibi R, Noritake K, Tomiga Y, Nitta H, Suzuki T, Takahashi H, Miyasaka N, Iwata T and Katagiri S (2022) Porphyromonas gingivalis Administration Induces Gestational Obesity, Alters Gene Expression in the Liver and Brown Adipose Tissue in Pregnant Mice, and Causes Underweight in Fetuses. Front. Cell. Infect. Microbiol. 11:745117. doi: 10.3389/fcimb.2021.745117
Received: 21 July 2021; Accepted: 07 December 2021;
Published: 13 January 2022.
Edited by:
Natarajaseenivasan Kalimuthusamy, Bharathidasan University, IndiaReviewed by:
Aruni Wilson, Loma Linda University, United StatesRaja Veerapandian, Texas Tech University Health Sciences Center El Paso, United States
Copyright © 2022 Yoshida, Hatasa, Ohsugi, Tsuchiya, Liu, Niimi, Morita, Shimohira, Sasaki, Maekawa, Shiba, Hirota, Okano, Hirose, Ibi, Noritake, Tomiga, Nitta, Suzuki, Takahashi, Miyasaka, Iwata and Katagiri. This is an open-access article distributed under the terms of the Creative Commons Attribution License (CC BY). The use, distribution or reproduction in other forums is permitted, provided the original author(s) and the copyright owner(s) are credited and that the original publication in this journal is cited, in accordance with accepted academic practice. No use, distribution or reproduction is permitted which does not comply with these terms.
*Correspondence: Sayaka Katagiri, a2F0YWdpcmkucGVyaUB0bWQuYWMuanA=