- 1Mikrobiologisches Institut - Klinische Mikrobiologie, Immunologie und Hygiene, Universitätsklinikum Erlangen and Friedrich-Alexander Universität (FAU) Erlangen-Nürnberg, Erlangen, Germany
- 2Medical Immunology Campus Erlangen, FAU Erlangen-Nürnberg, Erlangen, Germany
Innate (-like) T lymphocytes such as natural killer T (NKT) cells play a pivotal role in the recognition of microbial infections and their subsequent elimination. They frequently localize to potential sites of pathogen entry at which they survey extracellular and intracellular tissue spaces for microbial antigens. Engagement of their T cell receptors (TCRs) induces an explosive release of different cytokines and chemokines, which often pre-exist as constitutively expressed gene transcripts in NKT cells and underlie their poised effector state. Thus, NKT cells regulate immune cell migration and activation and subsequently, bridge innate and adaptive immune responses. In contrast to conventional T cells, which react to peptide antigens, NKT cells recognize lipids presented by the MHC class I like CD1d molecule on antigen presenting cells (APCs). Furthermore, each NKT cell TCR can recognize various antigen specificities, whereas a conventional T lymphocyte TCR reacts mostly only to one single antigen. These lipid antigens are either intermediates of the intracellular APC`s-own metabolism or originate from the cell wall of different bacteria, fungi or protozoan parasites. The best-characterized subset, the type 1 NKT cell subset expresses a semi-invariant TCR. In contrast, the TCR repertoire of type 2 NKT cells is diverse. Furthermore, NKT cells express a panoply of inhibitory and activating NK cell receptors (NKRs) that contribute to their primarily TCR-mediated rapid, innate like immune activation and even allow an adaption of their immune response in an adoptive like manner. Dueto their primary localization at host-environment interfaces, NKT cells are one of the first immune cells that interact with signals from different microbial pathogens. Vice versa, the mutual exchange with local commensal microbiota shapes also the biology of NKT cells, predominantly in the gastrointestinal tract. Following infection, two main signals drive the activation of NKT cells: first, cognate activation upon TCR ligation by microbial or endogenous lipid antigens; and second, bystander activation due to cytokines. Here we will discuss the role of NKT cells in the control of different microbial infections comparing pathogens expressing lipid ligands in their cell walls to infectious agents inducing endogenous lipid antigen presentation by APCs.
1 Introduction
Innate (-like) or unconventional T lymphocytes consist of a highly diverse group of cells. These include subsets of γ/δ T cells, mucosal-associated invariant T (MAIT) cells and natural killer T (NKT) cells (Godfrey et al., 2015; Legoux et al., 2017). During maturation in the thymus, they acquire characteristics specific for memory cells and exhibit unique trafficking patterns into peripheral, frequently non-lymphoid barrier tissues including the gut, the skin, the liver or the lung (Godfrey et al., 2015). There, innate (-like) T lymphocytes become resident, regulate tissue homeostasis or fight infections (Legoux et al., 2017). In contrast to conventional T lymphocytes, these innate (-like) T cell subsets express TCRs that are not restricted to the MHC-mediated presentation of peptide antigens (Legoux et al., 2017). Instead, MAIT cells, for example, recognize bacterial metabolite intermediates (Legoux et al., 2020) whereas NKT cells react to a variety of different self- or microbial lipid antigens, presented by the MHC-like molecules, MR1 or CD1, respectively (Godfrey et al., 2004; Bendelac et al., 2007; Singh et al., 2018). Following cognate TCR engagement, all three unconventional T cell subsets rapidly release copious amounts of cytokines and chemokines (Godfrey et al., 2004; Bendelac et al., 2007). Indeed, one single unconventional T cell clone can respond to various ligands, in contrast to the single antigen specificity of conventional T cells. Thus, the TCRs of innate (-like) T lymphocytes act rather as a pattern recognition receptor of the innate arm of the immune system than as highly diverse antigen recognition receptors of the adaptive arm of the immune system. Being frequently localized at interfaces of the host with its environment, innate (-like) T cells sense infections, form the first line of defense against invading pathogens and boost the subsequent innate and adaptive immune response.
In this review, we will focus on NKT cells (Godfrey et al., 2004; Bendelac et al., 2007; Godfrey et al., 2015; Singh et al., 2018).
2 NKT Cells
Natural killer T (NKT) cells belong to a subset of innate-like T lymphocytes that share properties of both, T and natural killer (NK) cells (Lantz and Bendelac, 1994; Bendelac et al., 1995; Godfrey et al., 2004). There are two main NKT cell subsets, which both react to a broad variety of endogenous and microbial, lipid-based antigens presented by the atypical MHC-I (-like) molecule CD1d on antigen presenting cells (APCs) (Bendelac et al., 2007; Birkholz and Kronenberg, 2015). Indeed, the best-characterized NKT cell subset are type 1 NKT cells, which express a semi-invariant TCR that combines Vα14-Jα18 with Vβ2, 7, 8 in mice and Vα24-Jα18 with Vβ11 in humans. Furthermore, all type 1 NKT cells react to the glycosphingolipid antigen alpha-galactosylceramide (α-GalCer) (Bendelac et al., 2007; Godfrey et al., 2018). In contrast, type 2 NKT cells exhibit a diverse TCR repertoire, do not react to α-GalCer and are more abundantly present in humans than in mice (Exley et al., 2001; Godfrey et al., 2018; Singh et al., 2018). Like other types of innate-like T lymphocytes, NKT cells are reactive to both, foreign and self-antigens (Bendelac et al., 2001). Following TCR engagement NKT cells can rapidly secrete high amounts of several cytokines and chemokines (Bendelac et al., 2007; Coquet et al., 2008). Second, NKT cells also express a wide range of activating and inhibitory NK cell receptors (Bendelac et al., 2007). While the role of activating NK cell receptors on NKT cell biology is only partially understood the inhibitory NK cell receptors control the self-reactivity of NKT cells and thus, avoid autoimmune activation (Bendelac et al., 2001; Kronenberg and Rudensky, 2005). Conversely, the NKT cell TCR also shapes the distribution of NK cell receptors (Skold et al., 2003; Voyle et al., 2003).
Following selection, maturation, cytokine polarization and egress from the thymus [for further reading please refer to (Kronenberg and Gapin, 2002; Bendelac et al., 2007; Constantinides and Bendelac, 2013)], NKT cells home to several lymphoid and non-lymphoid organs, including the adipose tissue, intestines, liver, lungs, lymph nodes and the spleen (Crosby and Kronenberg, 2018). NKT cells can exit the thymus either already as pre-committed subsets or can also acquire polarized functions in the periphery (Cameron and Godfrey, 2018). Once arrived at these distinct tissue sites, NKT cells usually reside there as non-circulating long-term residents and release a Th1, Th2, Th10 or Th17 polarized cytokine pattern following activation (Constantinides and Bendelac, 2013; Lee et al., 2013; Sag et al., 2014; Cameron and Godfrey, 2018). Although the substantial functional heterogeneity of polarized NKT cell subsets is well studied (Constantinides and Bendelac, 2013; Cameron and Godfrey, 2018; Crosby and Kronenberg, 2018), the factors and mechanisms underlying their recruitment to different tissues have not been characterized yet.
In humans, there exist large inter-individual variations in NKT cell numbers, ranging usually from 0.01% up to 1%, and in rare cases for up to 5% of the total T cell population in human blood, for example (Mattner, 2013; Godfrey et al., 2015). NKT cells comprise about 0.5% of the local T cell population in the blood and peripheral lymph nodes, around 2% in the spleen and up to 30% in the livers in mice (Benlagha et al., 2000; Matsuda et al., 2000; Hammond et al., 2001; Bendelac et al., 2007). Particularly, NKT cells accumulate in the livers of mice; there they patrol liver sinusoids for microbial and nutritional antigens (Geissmann et al., 2005) floating in from the portal vein system that drains the gastrointestinal tract. Although NKT cells contribute to the control of microbial infections, their aberrant activation under certain circumstances can also perpetuate tissue damage (Mattner, 2013).
3 NKT Cell Antigens
In contrast to conventional T cells, which recognize peptides bound to major histocompatibility complex (MHC) proteins, NKT cells react to lipid antigens presented by the MHC-like protein CD1d (Beckman et al., 1994; Brigl and Brenner, 2004). CD1d is a member of the CD1 family, which comprises five CD1 genes, CD1a, CD1b, CD1c, CD1d and CD1e in humans (Bradbury et al., 1988; Godfrey et al., 2018) with mice containing two orthologues of the CD1d gene, CD1d1 and CD1d2 (Bradbury et al., 1988; Balk et al., 1991). Both encode CD1d proteins, which are assembled in the endoplasmic reticulum, loaded with different microbial or self-antigens in the late endosome and delivered to the cell surface (Bricard and Porcelli, 2007; Cohen et al., 2009). There CD1d presents these antigens to NKT cells. Isoglobotrihexosylceramide (iGb3) (Zhou et al., 2004; Zajonc et al., 2008) and α-GalCer (Kain et al., 2014) have been suggested as endogenous ligands that are pivotal for the selection and differentiation of NKT cells in the thymus and their function in the periphery (Sundararaj et al., 2018). Furthermore, the CD1-restricted TCRs of NKT cells are autoreactive to CD1 expressing APCs (Cardell et al., 1995; Skold et al., 2003; Almeida et al., 2019). Indeed, endoplasmic reticulum (ER) stress in APCs is a potent inducer of CD1d-dependent NKT cell auto-reactivity (Govindarajan et al., 2020).
Synthetic α-GalCer was the first known antigen presented by CD1d that could stimulate the invariant TCR expressed by NKT cells (Kawano et al., 1997). This prototypical NKT cell ligand is a very close structural analog of several agelasphins, which have been isolated from extracts of the Agelus genus of marine sponges in the Okinawan sea because of their anti-tumor properties (Kobayashi et al., 1995; Morita et al., 1995). Importantly, α-GalCer is even active in picomolar concentration ranges. The physiological relevance of α-GalCer, however, was not well understood for many years. Nowadays, however, it is clear that α-GalCer or related structures are not only detected in marine sponges, but also in commensal or environmental bacteria (Kinjo et al., 2005; Mattner et al., 2005; Wieland Brown et al., 2013). Similarly, related structures have been identified in mammalian tissues or cow´s milk including α-psychosines (Kain et al., 2014; Kain et al., 2015; Deng et al., 2017) or α-glucosylceramides (GlcCers) (Brennan et al., 2014; Brennan et al., 2017). Many research groups have identified other microbial and endogenous NKT cell antigens. These include glycolipids, glycosphingolipids, diacylglycerols, glycerophospholipids, lysophospholipids and cholesterol esters (Mattner, 2013).
3.1 Microbial Antigens
3.1.1 Bacterial Antigens
One of the bacteria in whose cell wall antigens for NKT cells have been detected were Sphingomonas spp. (Kinjo et al., 2005; Mattner et al., 2005; Sriram et al., 2005). The genus Sphingomonas and the three closely related new genera, Sphingobium, Novosphingobium and Sphingopyxis contain more than 30 different species (Takeuchi et al., 2001). All four Sphingomonas genera are ubiquitous α-proteobacteria, commonly found in the environment including soil, sediments, plants and water (Barbeau et al., 1996; Cavicchioli et al., 1999; Brodie et al., 2007). Furthermore, Sphingomonas spp. can colonize mucosal surfaces of humans and mice (Selmi et al., 2003; Ivanov et al., 2009; Wei et al., 2010; Huang et al., 2011). Opportunistic nosocomial and even community-acquired infections, albeit rarely have been reported for these non-fermentative, aerobic, gram-negative bacilli (Crane et al., 1981; Freney et al., 1987; Reina et al., 1991; Hsueh et al., 1998; Marinella, 2002; Kampfer et al., 2005; Kilic et al., 2007; Ryan and Adley, 2010; Lin et al., 2010; Toh et al., 2011; Lanoix et al., 2012). Furthermore, Sphingomonas spp. have been associated with primary biliary cirrhosis (Selmi et al., 2003; Kaplan, 2004; Padgett et al., 2005; Mattner et al., 2008; Mohammed and Mattner, 2009; Mohammed et al., 2011), a chronic cholestatic liver disease characterized by a T cell mediated destruction of small bile ducts and autoantibodies targeting the E2 subunit of the mitochondrial pyruvate dehydrogenase complex (PDC-E2) (Gershwin et al., 2000; Kaplan and Gershwin, 2005).
Instead of expressing lipopolysaccharide (LPS) in their cell wall, these Gram-negative bacteria abundantly express glycosphingolipids (GSLs) which exhibit strong structural similarities with α-GalCer when present as monosaccharides (GSL-1´) (Kawahara et al., 1990; Kawahara et al., 1991; Kawahara et al., 1999; Kawahara et al., 2000; Kawahara et al., 2001; Kawahara et al., 2002; Kawahara et al., 2006). Thus, it is not surprising, that NKT cell-deficient mice clear infections with Sphingomonas spp. less efficiently compared to respective littermate controls (Kinjo et al., 2005; Mattner et al., 2005). Dependent on the sugar moiety linked to the ceramide portion, these GSL-1s contain a glucuronic acid or a galacturonic acid and thus, are called α- glucuronosylceramide (GSL-1) or α-galacturonosylceramide (GSL-1´). Besides of being linked to one sugar, GSLs of Sphingomonas can also contain additional saccharide groups. These include tri-and tetrasaccharides entitled as GSL-3 and GSL-4, respectively. While GSL-1 and GSL-1´ activate NKT cells in vitro and in vivo (Kinjo et al., 2005; Mattner et al., 2005; Sriram et al., 2005), GSL-3 and GSL-4 are not antigenic (Long et al., 2007; Kinjo et al., 2008). As bacterial symbionts frequently colonize the Agelus genus of marine sponges (Dieckmann et al., 2005), the prototypical ligand α-GalCer itself might have originated from Sphingomonas or related bacteria.
Other bacterial NKT cell antigens are diacylglycerols (DAGs) which are expressed in the cell wall of Borrelia burgdorferi, the causative agent of Lyme disease, or Streptococcus pneumonia, a Gram-positive bacterium triggering meningitis or pneumonia (Kinjo et al., 2006; Kinjo et al., 2011). Indeed, DAGs from both bacteria directly stimulated NKT cells in a CD1d-retricted manner. Consistently, the anti-microbial defense against both pathogens in vivo was impaired in the absence of NKT cells (Kumar et al., 2000; Nakamatsu et al., 2007; Christaki et al., 2015). Moreover, NKT cells modulate also the severity of Lyme disease, even after the infection has been cleared (Tupin et al., 2008; Olson et al., 2009). Other diacylglycerols (DAGs) are found in the cell walls of Corynebacterium glutamicum, an uniquitous soil bacterium without clinical relevance, Mycobacterium tuberculosis, Listeria monocytogenes and Mycobacterium smegmatis, a fast-growing, non-pathogenic bacterial species usually detected in urogenital secretions. Interestingly, α-glucuronosyl diacylglycerol (α-GlcA-DAG) derived from Mycobacterium smegmatis stimulated a NKT cell subset carrying a semi-invariant α-chain Vα10-Jα50+ (Uldrich et al., 2011; Burugupalli et al., 2020) as well as type 2 NKT cells (Almeida et al., 2019). Although Vα10-Jα50+ NKT cells recognized α-GalCer presented by CD1d, they neither fit in the type 1 nor the type 2 NKT cell category. Interestingly, Vα10-Jα50+ NKT cells produced 10- to 100-fold more IL-4, IL-13 and IL-17 compared to type 1 NKT cells following stimulation with α-GlcA–DAG (Uldrich et al., 2011). Furthermore, the TCRs of Vα10-Jα50+ and type 2 NKT cells can bind with diverse docking modes to CD1d-antigen complexes (Almeida et al., 2019).
Bacterial derived phospolipids also stimulate NKT cells with diverse TCRs (Wolucka et al., 1993; Cao et al., 2013). For example, phosphatidylglycerol (PG), diphosphatidylglycerol (DPG) and phosphatidylinositol from Mycobacterium tuberculosis, Listeria monocytogenes or Corynebacterium glutamicum stimulated type 2 NKT cells in a CD1d-restriced manner (Tatituri et al., 2013; Wolf et al., 2015). Type 2 NKT cells protected also against Methicillin-resistant Staphylococcus aureus infections due to the recognition of polar bacterial lipid species containing both PG and lyso-PG (Genardi et al., 2020).
Furthermore, phosphatidylinositolmannoside PIM4 in Mycobacterium tuberculosis (Fischer et al., 2004) and cholesteryl α-glucosides in Helicobacter pylori (Chang et al., 2011; Ito et al., 2013), a causative agent of gastritis and malignant tissue transformation, have been suggested as NKT cell antigens. However, only purified, but not synthetic PIM4 of Mycobacterium tuberculosis activated NKT cells (Fischer et al., 2004; Kinjo et al., 2006). In addition, NKT cells played no significant beneficial role in clearing both bacteria (Behar et al., 1999; Chang et al., 2011). Thus, the biological relevance of both antigens remains unclear. Compared to other antigens, the abundance of NKT cell ligands in the cell wall of both bacteria might be lower. Moreover, the stimulatory capacity of PIM4 and cholesteryl α-glucosides has not been compared yet with the exception of a study testing few NKT cell clones (Burugupalli et al., 2020) to the one of GSLs or DAGs found in other bacteria. Nonetheless, we speculate that the NKT cell TCR might have evolved as a pattern recognition receptor for distinct classes of bacteria carrying GSLs or DAGs in their cell walls.
Interestingly, bacteria, such as Bacteroides spp., physiologic commensals of mucosal surfaces can also evade the recognition by NKT cells. Following trauma or surgery, this predominant genus of the gastrointestinal tract can convert into pathobionts and can also cause life-threatening infections. First, Bacteroides spp., prominent members of the human gut microbiota, produce α-GalCer (Wieland Brown et al., 2013). Compared to marine-sponge derived α-GalCer, however, this bacterial α-GalCer triggered significantly less IFN-γ release, suggesting that molecular differences in the respective ligand determine the level and type of NKT cell activation. Furthermore, Bacteroides spp. contain unusual membrane glycosphingolipids (GSLs) (Kato et al., 1995), which among other functions (An et al., 2011) impede the activation of NKT cells in vitro and in vivo, in particular, GSL-Bf717 of Bacteroides >fragilis (An et al., 2014). Thus, the blockade of NKT cell activation likely hampers the detection of this opportunistic pathogen. Furthermore, this unique ligand also shapes the homeostasis of intestinal NKT cells during neonatal development (An et al., 2014) suggesting that intestinal microbiota significantly impact NKT cell biology.
Indeed, there is evidence that intestinal microbiota and NKT cells mutually interact with each other (Nieuwenhuis et al., 2009; Wei et al., 2010; Olszak et al., 2012; Wingender et al., 2012). Relative and absolute numbers of NKT cells specifically increase in intestinal tissues of germ free (GF) mice (Olszak et al., 2012; Wingender et al., 2012). The accumulation of NKT cells in the absence of intestinal microbiota, particularly in the colonic mucosa, is due to an increased expression of CXCL16, the cell surface ligand for the chemokine receptor CXCR6 (Olszak et al., 2012) expressed on NKT cells (Johnston et al., 2003; Geissmann et al., 2005). Moreover, NKT cells from GF mice were hyperreactive following induction of experimental colitis and perpetuated intestinal pathology (Olszak et al., 2012). Subsequently, mutual interactions between microbiota and NKT cells maintain intestinal immune homeostasis and prevent mucosal damage (Zeissig and Blumberg, 2013). Interestingly, albeit CXCR6-/- mice exhibit selectively reduced NKT cell numbers in the liver (Geissmann et al., 2005) and microbial antigens from the gut also circulate through the portal vein system into hepatic tissues, the absence of the intestinal microbiota did not significantly alter the expression of CXCL16 in hepatic tissues and thus, the accumulation of NKT cells there.
3.1.2 Fungal Antigens
In addition to bacterial cell wall antigens, NKT cells also directly detect fungal GSLs. In contrast to the α-linked bacterial ligands, however, the prototype of a fungal GSL is a β-linked glucosylceramide, asperamide B. Asperamide B has been identified in Aspergillus (Albacker et al., 2013), a saprophytic mold that causes invasive aspergillosis in immunocompromised patients (Dagenais and Keller, 2009) and allergic sensitization in predisposed, but otherwise healthy individuals (Knutsen et al., 2012). Indeed, purified and synthetic asperamide B directly activate mouse and human NKT cells in a CD1d-restricted manner. Furthermore, mice experienced airway hyperreactivity, a characteristic feature of asthma, following exposure to asperamide B in vivo (Albacker et al., 2013). Thus, as most protein allergens can bind lipids (Thomas et al., 2005; Karp, 2010), NKT cells activated by the GSLs of Aspergillus likely enhance an allergic sensitization to accompanying proteins and the subsequent adaptive Th2 responses. In addition, alpha-glycosyl diacylglyceride of Aspergillus fumigatus (Fontaine et al., 2009) are also recognized by type 1 and type 2 NKT cells (Burugupalli et al., 2020).
Next to the cognate recognition of fungal cell wall antigens, bystander mechanisms trigger also NKT cell activation during fungal infections. For example, major fungal cell-wall polysaccharides such as β-1,3 glucans trigger IL-12 production in a dectin-1 and MyD88-dependent manner by APCs. This drives the self-reactive iNKT activation and the release of IFN-γ in response to Aspergillus, Candida, Histoplasma and Alternaria (Cohen et al., 2011). Furthermore, CD1d-deficient mice poorly control infections with Aspergillus fumigatus (Cohen et al., 2011) reflecting the need for NKT cells in the control of fungal infections. In addition, human NKT cells exhibit immunomodulatory effects following exposure to Aspergillus spp (Beitzen-Heineke et al., 2016).
3.1.3 Protozoan Parasite Antigens
The third kingdom of microorganisms from which NKT cell antigens have been isolated from were protozoan parasites. For example, inositol and its derivatives have been proposed as NKT cell antigens. For example, the glycosylphosphatidylinositol (GPI) anchors of the surface proteins of Plasmodium falciparum or Trypanosoma brucei, the causative agents of malaria or African sleeping sickness, respectively, expanded NKT cells in a CD1d dependent manner (Schofield et al., 1999). These data, however, are controversial, as a subsequent study failed to induce NKT cell activation by GPI anchors (Molano et al., 2000). Furthermore, phosphoinositol (PI) antigens of Entamoeba histolytica, the causative agent of amoebiasis, also activated NKT cells (Lotter et al., 2009). In addition, the surface glycoconjugate lipophosphoglycan as well as related glycoinositol phospholipids of Leishmania, the protozoan parasite causing cutaneous und mucosal leishmaniasis, bind with high affinity to CD1d and induce a CD1d-dependent release of IFN-γ in subsets of intrahepatic lymphocytes (Amprey et al., 2004).
3.2 Endogenous Antigens
NKT cells are autoreactive towards CD1d-expressing cells (Brigl and Brenner, 2004). Endogenous ligands presented by CD1d presumably underlie this low-level auto-reactivity of NKT cells (Cardell et al., 1995; Burdin et al., 1998; Behar et al., 1999; Brossay and Kronenberg, 1999; Zhou et al., 2004; Chang et al., 2008). Since these ligands are potentially weaker agonists than microbial antigens, NKT cells require additional signals from other receptors and/or cell subsets for optimal activation when exposed to endogenous, CD1d presented ligands. Alternatively, the infection of APCs or different pathologic conditions increase or decrease the expression of CD1d molecules on their surfaces and subsequently, the amount and frequency of self-antigens presented to NKT cells resulting in altered NKT cell activation (Durante-Mangoni et al., 2004; Falcone et al., 2004; Yuan et al., 2006). Importantly, in contrast to the α-linked antigens from bacteria and protozoan parasites, a β-linkage of the sugar head to the ceramide lipid portion naturally occurs in mammals. Although several antigens have been described as endogenous ligands (Birkholz and Kronenberg, 2015), particularly isoglobotrihexosylceramide (iGb3) (Zhou et al., 2004; Zajonc et al., 2008) and β-glucosylceramide (Stanic et al., 2003; Brennan et al., 2011) that might be involved in NKT cell activation during bacterial infection (Mattner et al., 2005; Brennan et al., 2011). There is also evidence that infections lead to an accumulation self-lipid reactive type 1 and type 2 NKT cells (Mattner et al., 2005; Chang et al., 2008; Nair et al., 2015). However, whether one or both of these endogenous antigens or other ligands drive autoreactive NKT cell responses in infections with viruses, fungi or protozoan parasites is still largely unknown. Thus, the exact context in which endogenous ligand(s) trigger NKT cell activation still require definition. Furthermore, dependent on the tissue and the (patho-) physiological context, different self-antigens might be presented to NKT cells that likely affect their biology and subsequent functional response.
4 Mechanisms of NKT Cell Activation During Infection
Following phagocytosis and degradation of microbial pathogens by different APCs and phagocytes, microbial and endogenous antigens and ligands are released which engage their respective receptors. Among those, lipid antigens are loaded onto CD1d molecules in the late endosome, which recycle afterwards back to the cell surface. There, NKT cells survey CD1d molecules for antigens as well as for additional accessory signals provided by APCs, which allow subsequent NKT cell activation. Based on the nature of these interactions, there are three major mechanisms driving NKT cell activation during microbial infection (Figure 1): A) NKT cells directly recognize due to cognate TCR engagement microbial lipid antigens presented by CD1d (Mattner et al., 2005; La Gruta et al., 2018); B) NKT cell TCRs react to endogenous ligands incorporated into CD1d; the availability of endogenous lipid antigens can be induced by pattern recognition receptor- (PRR-) driven APC activation (Mattner et al., 2005; Zeissig et al., 2012); C) engagement of toll-like receptors (TLRs) and Dectin by LPS, CpG or β-1,3-glucan drives the release of IL-12 and IL-18 by APCs, which leads to a TCR-independent activation of NKT cells (bystander activation) (Leite-De-Moraes et al., 1999; Brigl et al., 2011; Cohen et al., 2011; Moran et al., 2011; Holzapfel et al., 2014). This mechanism can also enhance the cognate TCR activation by exogenous or endogenous antigens (mechanisms A & B). However, IL-12, IL-18, and TLRs are completely dispensable for the TCR activation pathway of NKT cells when a strong TCR agonist is used (Anderson et al., 2021).
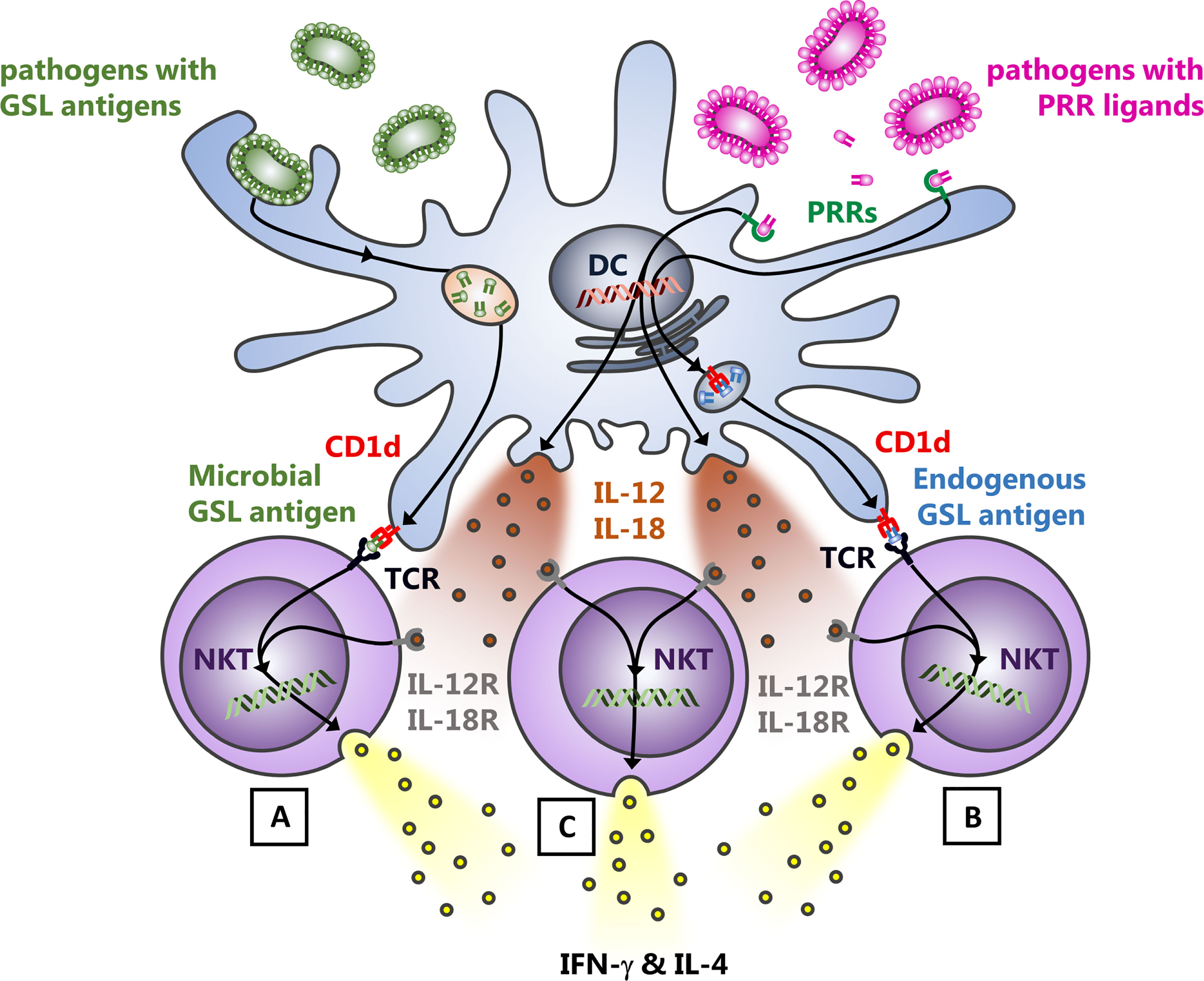
Figure 1 Modes of NKT cell activation upon bacterial infection. Following the uptake and digestion of bacteria by myeloid cells, three mechanisms predominantly drive the activation of NKT cells: (A), bacterial cell wall glycosphingolipid (GSL) antigens presented by APCs are sufficient to induce the release of IFN-γ and IL-4 by NKT cells due to the CD1d mediated presentation of the ligand to the NKT cell TCR. (B), endogenous GSL presentation via CD1d to the NKT cell TCR in response to infection and subsequent augmented NKT cell auto-reactivity. The identity of the lipid ligands underlying the auto-reactivity of NKT cells in different tissues and under distinct (patho-) physiological circumstances, however, still requires definition. (C), the cytokine driven, TCR-independent activation of NKT cells for which TLR- oder Dectin triggered IL-12 and IL-18 release by APCs is responsible. This also further enhances the release of cytokines by NKT cells, activated by cognate TCR engagement [mechanisms (A, B)]. Thus, NKT cells are activated during infection with bacteria that do not express themselves NKT cell antigens in their cell walls or unable to induce endogenous antigens.
Accordingly, NKT cells activated by microbial glycolipid antigens contribute to bacterial clearance (cognate activation) (Kinjo et al., 2005; Mattner et al., 2005; Kinjo et al., 2006; Kinjo et al., 2011). However, the TLR-elicited cytokine responses of myeloid cells infected by bacteria that do not contain lipids in their cell wall also activate and recruit NKT cells as part of an inflammatory cellular network (bystander activation) (Brigl et al., 2003; Mattner et al., 2005). Furthermore, these myeloid cell-derived cytokines also enhance the TCR-mediated activation of NKT cells. Thus, an absence of NKT cells has not only been associated with an enhanced susceptibility to infectious diseases in mice (Kinjo et al., 2005; Mattner et al., 2005; Kinjo et al., 2006; Kinjo et al., 2008; Cohen et al., 2011), but also in humans. Indeed, genetic mutations resulting in low NKT cell numbers and decreased innate IFN-γ release have been associated with mycobacterial diseases (Yang et al., 2020) or with an enhanced susceptibility to infections with the Epstein Barr Virus (EBV) (Rigaud et al., 2006).
Following NKT cell activation, many other cell populations subsequently respond to the released cytokines, including different myeloid cell subsets, NK cells, T- and B-lymphocytes (Godfrey et al., 2004; Bendelac et al., 2007). This has been associated with immune cell activation and the augmentation of respective immune responses. Thus, lipid antigens activating NKT cells are utilized as adjuvants in various vaccination strategies. However, dependent on the expression of CD1d and the cytokine receptor expression profile (Figure 2), NKT cells can also suppress the downstream cellular network. For example, NKT cells interact with B cells or DCs through cognate or bystander interactions (Mattner et al., 2008; Chang et al., 2011; King et al., 2011) (Figures 2A, B). Dominant influence of one or the other pathway might contribute to the observation that NKT cells can serve both as helpers for effector B-lymphocytes and negatively regulate autoreactive B cell responses (Sedimbi et al., 2020). Furthermore, an application of glyco-lipid containing nanoparticles enhances humoral immunity, but abrogates T cell - independent vaccine responses (Shute et al., 2021). IFN-γ released by NKT cells is also critical for the cross-activation of NK cells one the one hand (Carnaud et al., 1999) and the subsequent activation of CD8+ T cells. Indeed, NK cells are even the dominant cellular source for IFN-γ following NKT cell activation (Hoeksema et al., 2015; Kim et al., 2020). However, on the other hand, the release of cytokines by NKT cells interfering with NK cell responses can contribute also to immunosuppression due to the upregulation of mTOR (Figure 2C), as observed, for example, in bacterial sepsis (Kim et al., 2020).
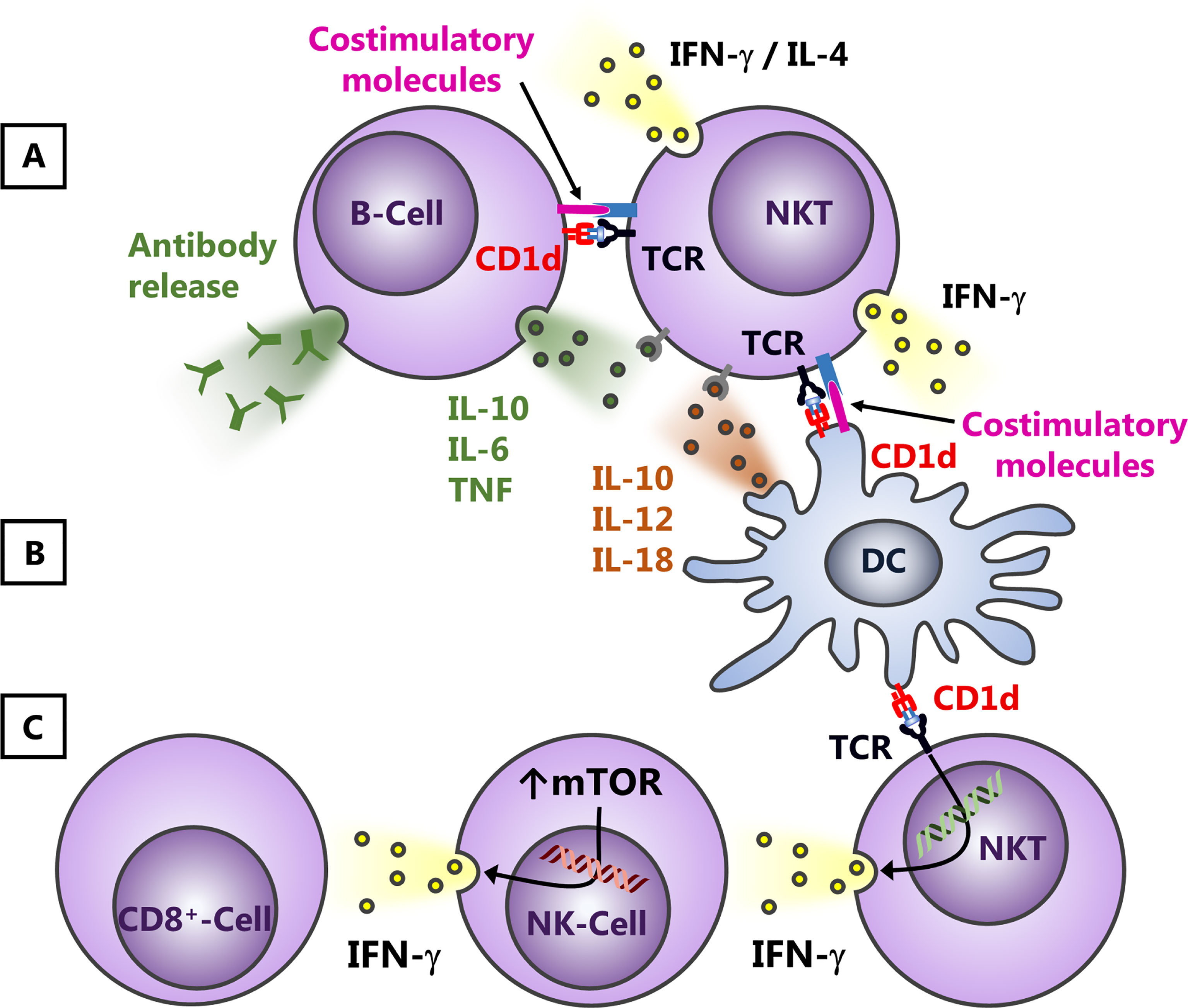
Figure 2 Effects of NKT cell activation on B-lymphocytes and NK cells. NKT cells and antigen presenting cells such as B-lymphocytes (A) or DCs (B) influence each other due to cognate and bystander activation. Thus, on the one hand, B-lymphocytes and DCs can modify NKT cell responses due to variations in the presentation of lipid antigens and/or an altered release of cytokines and/or changes in the expression of costimulatory molecules. B cells can promote thereby also Th2 responses (A), whereas DCs presumably trigger predominantly the release of Th1 cytokines (B). Vice versa, dependent on the interaction with cytokines, costimulatory molecules and/or the lipid antigens presented, NKT cells, for example, can alter their cytokine profile (Th1 and Th2) and subsequently suppress or augment B cell responses. This can affect the release and/or the class switch of antibodies by B-lymphocytes (A) or the cytokine profile and/or expression of costimulatory molecules by DCs (B). Within a more Th1-dominated cytokine milieu, NKT cells can cross-activate NK cells and CD8+ T-lymphocytes (C), a process that involves IFN-γ. This release of cytokines by NKT cells, however, does not only augment subsequent immune responses, but can also contribute to immunosuppression, for example, due to the upregulation of mTOR.
5 Conclusion
In summary, several bacteria including Sphingomonas, Borrelia, Streptococcus, Mycobacterium, Helicobacter, Corynebacterium and Bacteroides express NKT cell antigens. These include GSLs and DAGs, which predominantly trigger the activation of NKT cells in a TCR-dependent manner when presented on CD1d. As these antigens are either highly immunogenic and/or present in large numbers in the bacterial cell wall, NKT cells can mediate a pivotal role for the clearance of these bacteria. Moreover, Sphingomonas spp., which are Gram-negative bacteria, express GSLs instead of LPS in their cell walls. Interestingly, one antigen of Bacteroides fragilis seems to inhibit the activation of NKT cells, which might contribute to bacterial immune evasion or reflect a mutual tolerance mechanism of NKT cells and commensal bacteria. As the TCR signal strength can also influence the polarization of NKT cell subsets (Tuttle et al., 2018), the functional phenotype of NKT cells, which tends to be imprinted in the thymus, might change even during the course of infection, particularly during chronic infections. Indeed, a functional polarization of NKT cells, similar to conventional T cells, can also occur during peripheral activation depending on the cytokine milieu characteristic of the specific activation context (Monteiro et al., 2010; Monteiro et al., 2013; Monteiro et al., 2015; Cameron and Godfrey, 2018). Akin to the more adaptive T cell response, NKT cells can expand and antigen-specific NKT cell clones can be generated that will assist in the clearance of infection. Thus, vice versa, microbial signals can also influence the functionality of NKT cells. Subsequently, further studies need to characterize the phenotypical changes of NKT cells in the respective infections and to delineate their functional consequences for bacterial clearance.
Author Contributions
SV prepared the figure and added comments to the manuscript. JM wrote the manuscript. All authors contributed to the article and approved the submitted version.
Funding
This study was supported by the Staedtler Stiftung (to JM), the German Research Foundation DFG (grant MA 2621/4-1 to JM, grant MA 2621/5-1 to JM and DFG-CRC 1181 - project number C04 to JM).
Conflict of Interest
The authors declare that the research was conducted in the absence of any commercial or financial relationships that could be construed as a potential conflict of interest.
Publisher’s Note
All claims expressed in this article are solely those of the authors and do not necessarily represent those of their affiliated organizations, or those of the publisher, the editors and the reviewers. Any product that may be evaluated in this article, or claim that may be made by its manufacturer, is not guaranteed or endorsed by the publisher.
Acknowledgments
We thank all the present members of our lab for their support and their contributions.
References
Albacker, L. A., Chaudhary, V., Chang, Y. J., Kim, H. Y., Chuang, Y. T., Pichavant, M., et al. (2013). Invariant Natural Killer T Cells Recognize a Fungal Glycosphingolipid That Can Induce Airway Hyperreactivity. Nat. Med. 19, 1297–1304. doi: 10.1038/nm.3321
Almeida, C. F., Sundararaj, S., Le Nours, J., Praveena, T., Cao, B., Burugupalli, S., et al. (2019). Distinct CD1d Docking Strategies Exhibited by Diverse Type II NKT Cell Receptors. Nat. Commun. 10, 5242. doi: 10.1038/s41467-019-12941-9
Amprey, J. L., Im, J. S., Turco, S. J., Murray, H. W., Illarionov, P. A., Besra, G. S., et al. (2004). A Subset of Liver NK T Cells Is Activated During Leishmania Donovani Infection by CD1d-Bound Lipophosphoglycan. J. Exp. Med. 200, 895–904. doi: 10.1084/jem.20040704
Anderson, C. K., Reilly, S. P., Brossay, L. (2021). The Invariant NKT Cell Response Has Differential Signaling Requirements During Antigen-Dependent and Antigen-Independent Activation. J. Immunol. 206, 132–140. doi: 10.4049/jimmunol.2000870
An, D., Na, C., Bielawski, J., Hannun, Y. A., Kasper, D. L. (2011). Membrane Sphingolipids as Essential Molecular Signals for Bacteroides Survival in the Intestine. Proc. Natl. Acad. Sci. U. S. A. 108 Suppl 1, 4666–4671. doi: 10.1073/pnas.1001501107
An, D., Oh, S. F., Olszak, T., Neves, J. F., Avci, F. Y., Erturk-Hasdemir, D., et al. (2014). Sphingolipids From a Symbiotic Microbe Regulate Homeostasis of Host Intestinal Natural Killer T Cells. Cell 156, 123–133. doi: 10.1016/j.cell.2013.11.042
Balk, S. P., Bleicher, P. A., Terhorst, C. (1991). Isolation and Expression of cDNA Encoding the Murine Homologues of CD1. J. Immunol. 146, 768–774.
Barbeau, J., Tanguay, R., Faucher, E., Avezard, C., Trudel, L., Cote, L., et al. (1996). Multiparametric Analysis of Waterline Contamination in Dental Units. Appl. Environ. Microbiol. 62, 3954–3959. doi: 10.1128/aem.62.11.3954-3959.1996
Beckman, E. M., Porcelli, S. A., Morita, C. T., Behar, S. M., Furlong, S. T., Brenner, M. B. (1994). Recognition of a Lipid Antigen by CD1-Restricted Alpha Beta+ T Cells. Nature 372, 691–694. doi: 10.1038/372691a0
Behar, S. M., Dascher, C. C., Grusby, M. J., Wang, C. R., Brenner, M. B. (1999). Susceptibility of Mice Deficient in CD1D or TAP1 to Infection With Mycobacterium Tuberculosis. J. Exp. Med. 189, 1973–1980. doi: 10.1084/jem.189.12.1973
Beitzen-Heineke, A., Bouzani, M., Schmitt, A. L., Kurzai, O., Hunniger, K., Einsele, H., et al. (2016). Human Invariant Natural Killer T Cells Possess Immune-Modulating Functions During Aspergillus Infection. Med. Mycol 54, 169–176. doi: 10.1093/mmy/myv074
Bendelac, A., Bonneville, M., Kearney, J. F. (2001). Autoreactivity by Design: Innate B and T Lymphocytes. Nat. Rev. Immunol. 1, 177–186. doi: 10.1038/35105052
Bendelac, A., Lantz, O., Quimby, M. E., Yewdell, J. W., Bennink, J. R., Brutkiewicz, R. R. (1995). CD1 Recognition by Mouse NK1+ T Lymphocytes. Science 268, 863–865. doi: 10.1126/science.7538697
Bendelac, A., Savage, P. B., Teyton, L. (2007). The Biology of NKT Cells. Annu. Rev. Immunol. 25, 297–336. doi: 10.1146/annurev.immunol.25.022106.141711
Benlagha, K., Weiss, A., Beavis, A., Teyton, L., Bendelac, A. (2000). In Vivo Identification of Glycolipid Antigen-Specific T Cells Using Fluorescent CD1d Tetramers. J. Exp. Med. 191, 1895–1903. doi: 10.1084/jem.191.11.1895
Birkholz, A. M., Kronenberg, M. (2015). Antigen Specificity of Invariant Natural Killer T-Cells. BioMed. J. 38, 470–483. doi: 10.1016/j.bj.2016.01.003
Bradbury, A., Belt, K. T., Neri, T. M., Milstein, C., Calabi, F. (1988). Mouse CD1 Is Distinct From and Co-Exists With TL in the Same Thymus. EMBO J. 7, 3081–3086. doi: 10.1002/j.1460-2075.1988.tb03173.x
Brennan, P. J., Cheng, T. Y., Pellicci, D. G., Watts, G. F. M., Veerapen, N., Young, D. C., et al. (2017). Structural Determination of Lipid Antigens Captured at the CD1d-T-Cell Receptor Interface. Proc. Natl. Acad. Sci. U. S. A. 114, 8348–8353. doi: 10.1073/pnas.1705882114
Brennan, P. J., Tatituri, R. V., Brigl, M., Kim, E. Y., Tuli, A., Sanderson, J. P., et al. (2011). Invariant Natural Killer T Cells Recognize Lipid Self Antigen Induced by Microbial Danger Signals. Nat. Immunol. 12, 1202–1211. doi: 10.1038/ni.2143
Brennan, P. J., Tatituri, R. V., Heiss, C., Watts, G. F., Hsu, F. F., Veerapen, N., et al. (2014). Activation of iNKT Cells by a Distinct Constituent of the Endogenous Glucosylceramide Fraction. Proc. Natl. Acad. Sci. U. S. A. 111, 13433–13438. doi: 10.1073/pnas.1415357111
Bricard, G., Porcelli, S. A. (2007). Antigen Presentation by CD1 Molecules and the Generation of Lipid-Specific T Cell Immunity. Cell Mol. Life Sci. 64, 1824–1840. doi: 10.1007/s00018-007-7007-0
Brigl, M., Brenner, M. B. (2004). CD1: Antigen Presentation and T Cell Function. Annu. Rev. Immunol. 22, 817–890. doi: 10.1146/annurev.immunol.22.012703.104608
Brigl, M., Bry, L., Kent, S. C., Gumperz, J. E., Brenner, M. B. (2003). Mechanism of CD1d-Restricted Natural Killer T Cell Activation During Microbial Infection. Nat. Immunol. 4, 1230–1237. doi: 10.1038/ni1002
Brigl, M., Tatituri, R. V., Watts, G. F., Bhowruth, V., Leadbetter, E. A., Barton, N., et al. (2011). Innate and Cytokine-Driven Signals, Rather Than Microbial Antigens, Dominate in Natural Killer T Cell Activation During Microbial Infection. J. Exp. Med. 208, 1163–1177. doi: 10.1084/jem.20102555
Brodie, E. L., DeSantis, T. Z., Parker, J. P., Zubietta, I. X., Piceno, Y. M., Andersen, G. L. (2007). Urban Aerosols Harbor Diverse and Dynamic Bacterial Populations. Proc. Natl. Acad. Sci. U. S. A. 104, 299–304. doi: 10.1073/pnas.0608255104
Brossay, L., Kronenberg, M. (1999). Highly Conserved Antigen-Presenting Function of CD1d Molecules. Immunogenetics 50, 146–151. doi: 10.1007/s002510050590
Burdin, N., Brossay, L., Koezuka, Y., Smiley, S. T., Grusby, M. J., Gui, M., et al. (1998). Selective Ability of Mouse CD1 to Present Glycolipids: Alpha-Galactosylceramide Specifically Stimulates V Alpha 14+ NK T Lymphocytes. J. Immunol. 161, 3271–3281.
Burugupalli, S., Almeida, C. F., Smith, D. G. M., Shah, S., Patel, O., Rossjohn, J., et al. (2020). Alpha-Glucuronosyl and Alpha-Glucosyl Diacylglycerides, Natural Killer T Cell-Activating Lipids From Bacteria and Fungi. Chem. Sci. 11, 2161–2168. doi: 10.1039/C9SC05248H
Cameron, G., Godfrey, D. I. (2018). Differential Surface Phenotype and Context-Dependent Reactivity of Functionally Diverse NKT Cells. Immunol. Cell Biol. 96, 759–771. doi: 10.1111/imcb.12034
Cao, B., Chen, X., Yamaryo-Botte, Y., Richardson, M. B., Martin, K. L., Khairallah, G. N., et al. (2013). Synthesis, Structural Elucidation, and Biochemical Analysis of Immunoactive Glucuronosyl Diacylglycerides of Mycobacteria and Corynebacteria. J. Org Chem. 78, 2175–2190. doi: 10.1021/jo302508e
Cardell, S., Tangri, S., Chan, S., Kronenberg, M., Benoist, C., Mathis, D. (1995). CD1-Restricted CD4+ T Cells in Major Histocompatibility Complex Class II-Deficient Mice. J. Exp. Med. 182, 993–1004. doi: 10.1084/jem.182.4.993
Carnaud, C., Lee, D., Donnars, O., Park, S. H., Beavis, A., Koezuka, Y., et al. (1999). Cutting Edge: Cross-Talk Between Cells of the Innate Immune System: NKT Cells Rapidly Activate NK Cells. J. Immunol. 163, 4647–4650.
Cavicchioli, R., Fegatella, F., Ostrowski, M., Eguchi, M., Gottschal, J. (1999). Sphingomonads From Marine Environments. J. Ind. Microbiol. Biotechnol. 23, 268–272. doi: 10.1038/sj.jim.2900732
Chang, P. P., Barral, P., Fitch, J., Pratama, A., Ma, C. S., Kallies, A., et al. (2011). Identification of Bcl-6-Dependent Follicular Helper NKT Cells That Provide Cognate Help for B Cell Responses. Nat. Immunol. 13, 35–43. doi: 10.1038/ni.2166
Chang, Y. J., Kim, H. Y., Albacker, L. A., Lee, H. H., Baumgarth, N., Akira, S., et al. (2011). Influenza Infection in Suckling Mice Expands an NKT Cell Subset That Protects Against Airway Hyperreactivity. J. Clin. Invest. 121, 57–69. doi: 10.1172/JCI44845
Chang, J. H., Lee, J. M., Youn, H. J., Lee, K. A., Chung, Y., Lee, A. Y., et al. (2008). Functional Maturation of Lamina Propria Dendritic Cells by Activation of NKT Cells Mediates the Abrogation of Oral Tolerance. Eur. J. Immunol. 38, 2727–2739. doi: 10.1002/eji.200838159
Christaki, E., Diza, E., Giamarellos-Bourboulis, E. J., Papadopoulou, N., Pistiki, A., Droggiti, D. I., et al. (2015). NK and NKT Cell Depletion Alters the Outcome of Experimental Pneumococcal Pneumonia: Relationship With Regulation of Interferon-Gamma Production. J. Immunol. Res. 2015, 532717. doi: 10.1155/2015/532717
Cohen, N. R., Garg, S., Brenner, M. B. (2009). Antigen Presentation by CD1 Lipidsand NKT Cells in Microbial Immunity. Adv. Immunol. 102, 1–94. doi: 10.1016/S0065-2776(09)01201-2
Cohen, N. R., Tatituri, R. V., Rivera, A., Watts, G. F., Kim, E. Y., Chiba, A., et al. (2011). Innate Recognition of Cell Wall Beta-Glucans Drives Invariant Natural Killer T Cell Responses Against Fungi. Cell Host Microbe 10, 437–450. doi: 10.1016/j.chom.2011.09.011
Constantinides, M. G., Bendelac, A. (2013). Transcriptional Regulation of the NKT Cell Lineage. Curr. Opin. Immunol. 25, 161–167. doi: 10.1016/j.coi.2013.01.003
Coquet, J. M., Chakravarti, S., Kyparissoudis, K., McNab, F. W., Pitt, L. A., McKenzie, B. S., et al. (2008). Diverse Cytokine Production by NKT Cell Subsets and Identification of an IL-17-Producing CD4-Nk1.1- NKT cell population. Proc. Natl. Acad. Sci. U. S. A. 105, 11287–11292. doi: 10.1073/pnas.0801631105
Crane, L. R., Tagle, L. C., Palutke, W. A. (1981). Outbreak of Pseudomonas Paucimobilis in an Intensive Care Facility. JAMA 246, 985–987. doi: 10.1001/jama.1981.03320090047030
Crosby, C. M., Kronenberg, M. (2018). Tissue-Specific Functions of Invariant Natural Killer T Cells. Nat. Rev. Immunol. 18, 559–574. doi: 10.1038/s41577-018-0034-2
Dagenais, T. R., Keller, N. P. (2009). Pathogenesis of Aspergillus Fumigatus in Invasive Aspergillosis. Clin. Microbiol. Rev. 22, 447–465. doi: 10.1128/CMR.00055-08
Deng, S., Kain, L., Pereira, C. S., Mata, S., Macedo, M. F., Bendelac, A., et al. (2017). Psychosine Variants as Antigens for Natural Killer T Cells. Chem. Sci. 8, 2204–2208. doi: 10.1039/C6SC04218J
Dieckmann, R., Graeber, I., Kaesler, I., Szewzyk, U., von Dohren, H. (2005). Rapid Screening and Dereplication of Bacterial Isolates From Marine Sponges of the Sula Ridge by Intact-Cell-MALDI-TOF Mass Spectrometry (ICM-Ms). Appl. Microbiol. Biotechnol. 67, 539–548. doi: 10.1007/s00253-004-1812-2
Durante-Mangoni, E., Wang, R., Shaulov, A., He, Q., Nasser, I., Afdhal, N., et al. (2004). Hepatic CD1d Expression in Hepatitis C Virus Infection and Recognition by Resident Proinflammatory CD1d-Reactive T Cells. J. Immunol. 173, 2159–2166. doi: 10.4049/jimmunol.173.3.2159
Exley, M. A., Tahir, S. M., Cheng, O., Shaulov, A., Joyce, R., Avigan, D., et al. (2001). A Major Fraction of Human Bone Marrow Lymphocytes Are Th2-Like CD1d-Reactive T Cells That can Suppress Mixed Lymphocyte Responses. J. Immunol. 167, 5531–5534. doi: 10.4049/jimmunol.167.10.5531
Falcone, M., Facciotti, F., Ghidoli, N., Monti, P., Olivieri, S., Zaccagnino, L., et al. (2004). Up-Regulation of CD1d Expression Restores the Immunoregulatory Function of NKT Cells and Prevents Autoimmune Diabetes in Nonobese Diabetic Mice. J. Immunol. 172, 5908–5916. doi: 10.4049/jimmunol.172.10.5908
Fischer, K., Scotet, E., Niemeyer, M., Koebernick, H., Zerrahn, J., Maillet, S., et al. (2004). Mycobacterial Phosphatidylinositol Mannoside Is a Natural Antigen for CD1d-Restricted T Cells. Proc. Natl. Acad. Sci. U. S. A. 101, 10685–10690. doi: 10.1073/pnas.0403787101
Fontaine, T., Lamarre, C., Simenel, C., Lambou, K., Coddeville, B., Delepierre, M., et al. (2009). Characterization of Glucuronic Acid Containing Glycolipid in Aspergillus Fumigatus Mycelium. Carbohydr Res. 344, 1960–1967. doi: 10.1016/j.carres.2009.07.012
Freney, J., Hansen, W., Ploton, C., Meugnier, H., Madier, S., Bornstein, N., et al. (1987). Septicemia Caused by Sphingobacterium Multivorum. J. Clin. Microbiol. 25, 1126–1128. doi: 10.1128/jcm.25.6.1126-1128.1987
Geissmann, F., Cameron, T. O., Sidobre, S., Manlongat, N., Kronenberg, M., Briskin, M. J., et al. (2005). Intravascular Immune Surveillance by CXCR6+ NKT Cells Patrolling Liver Sinusoids. PloS Biol. 3, e113. doi: 10.1371/journal.pbio.0030113
Genardi, S., Visvabharathy, L., Cao, L., Morgun, E., Cui, Y., Qi, C., et al. (2020). Type II Natural Killer T Cells Contribute to Protection Against Systemic Methicillin-Resistant Staphylococcus Aureus Infection. Front. Immunol. 11, 610010. doi: 10.3389/fimmu.2020.610010
Gershwin, M. E., Ansari, A. A., Mackay, I. R., Nakanuma, Y., Nishio, A., Rowley, M. J., et al. (2000). Primary Biliary Cirrhosis: An Orchestrated Immune Response Against Epithelial Cells. Immunol. Rev. 174, 210–225. doi: 10.1034/j.1600-0528.2002.017402.x
Godfrey, D. I., Le Nours, J., Andrews, D. M., Uldrich, A. P., Rossjohn, J. (2018). Unconventional T Cell Targets for Cancer Immunotherapy. Immunity 48, 453–473. doi: 10.1016/j.immuni.2018.03.009
Godfrey, D. I., MacDonald, H. R., Kronenberg, M., Smyth, M. J., Van Kaer, L. (2004). NKT Cells: What's in a Name? Nat. Rev. Immunol. 4, 231–237. doi: 10.1038/nri1309
Godfrey, D. I., Uldrich, A. P., McCluskey, J., Rossjohn, J., Moody, D. B. (2015). The Burgeoning Family of Unconventional T Cells. Nat. Immunol. 16, 1114–1123. doi: 10.1038/ni.3298
Govindarajan, S., Verheugen, E., Venken, K., Gaublomme, D., Maelegheer, M., Cloots, E., et al. (2020). ER Stress in Antigen-Presenting Cells Promotes NKT Cell Activation Through Endogenous Neutral Lipids. EMBO Rep. 21, e48927. doi: 10.15252/embr.201948927
Hammond, K. J., Pellicci, D. G., Poulton, L. D., Naidenko, O. V., Scalzo, A. A., Baxter, A. G., et al. (2001). CD1d-Restricted NKT Cells: An Interstrain Comparison. J. Immunol. 167, 1164–1173. doi: 10.4049/jimmunol.167.3.1164
Hoeksema, M. A., Scicluna, B. P., Boshuizen, M. C., van der Velden, S., Neele, A. E., Van den Bossche, J., et al. (2015). IFN-Gamma Priming of Macrophages Represses a Part of the Inflammatory Program and Attenuates Neutrophil Recruitment. J. Immunol. 194, 3909–3916. doi: 10.4049/jimmunol.1402077
Holzapfel, K. L., Tyznik, A. J., Kronenberg, M., Hogquist, K. A. (2014). Antigen-Dependent Versus -Independent Activation of Invariant NKT Cells During Infection. J. Immunol. 192, 5490–5498. doi: 10.4049/jimmunol.1400722
Hsueh, P. R., Teng, L. J., Yang, P. C., Chen, Y. C., Pan, H. J., Ho, S. W., et al. (1998). Nosocomial Infections Caused by Sphingomonas Paucimobilis: Clinical Features and Microbiological Characteristics. Clin. Infect. Dis. 26, 676–681. doi: 10.1086/514595
Huang, Y. J., Nelson, C. E., Brodie, E. L., Desantis, T. Z., Baek, M. S., Liu, J., et al. (2011). Airway Microbiota and Bronchial Hyperresponsiveness in Patients With Suboptimally Controlled Asthma. J. Allergy Clin. Immunol. 127, 372–381.e1–3. doi: 10.1016/j.jaci.2010.10.048
Ito, Y., Vela, J. L., Matsumura, F., Hoshino, H., Tyznik, A., Lee, H., et al. (2013). Helicobacter Pylori Cholesteryl Alpha-Glucosides Contribute to Its Pathogenicity and Immune Response by Natural Killer T Cells. PloS One 8, e78191. doi: 10.1371/journal.pone.0078191
Ivanov, I. I., Atarashi, K., Manel, N., Brodie, E. L., Shima, T., Karaoz, U., et al. (2009). Induction of Intestinal Th17 Cells by Segmented Filamentous Bacteria. Cell 139, 485–498. doi: 10.1016/j.cell.2009.09.033
Johnston, B., Kim, C. H., Soler, D., Emoto, M., Butcher, E. C. (2003). Differential Chemokine Responses and Homing Patterns of Murine TCR Alpha Beta NKT Cell Subsets. J. Immunol. 171, 2960–2969. doi: 10.4049/jimmunol.171.6.2960
Kain, L., Costanzo, A., Webb, B., Holt, M., Bendelac, A., Savage, P. B., et al. (2015). Endogenous Ligands of Natural Killer T Cells Are Alpha-Linked Glycosylceramides. Mol. Immunol. 68, 94–97. doi: 10.1016/j.molimm.2015.06.009
Kain, L., Webb, B., Anderson, B. L., Deng, S., Holt, M., Costanzo, A., et al. (2014). The Identification of the Endogenous Ligands of Natural Killer T Cells Reveals the Presence of Mammalian Alpha-Linked Glycosylceramides. Immunity 41, 543–554. doi: 10.1016/j.immuni.2014.08.017
Kampfer, P., Engelhart, S., Rolke, M., Sennekamp, J. (2005). Extrinsic Allergic Alveolitis (Hypersensitivity Pneumonitis) Caused by Sphingobacterium Spiritivorum From the Water Reservoir of a Steam Iron. J. Clin. Microbiol. 43, 4908–4910. doi: 10.1128/JCM.43.9.4908-4910.2005
Kaplan, M. M. (2004). Novosphingobium Aromaticivorans: A Potential Initiator of Primary Biliary Cirrhosis. Am. J. Gastroenterol. 99, 2147–2149. doi: 10.1111/j.1572-0241.2004.41121.x
Kaplan, M. M., Gershwin, M. E. (2005). Primary Biliary Cirrhosis. N. Engl. J. Med. 353, 1261–1273. doi: 10.1056/NEJMra043898
Karp, C. L. (2010). Guilt by Intimate Association: What Makes an Allergen an Allergen? J. Allergy Clin. Immunol. 125, 955–60; quiz 961–2. doi: 10.1016/j.jaci.2010.03.002
Kato, M., Muto, Y., Tanaka-Bandoh, K., Watanabe, K., Ueno, K. (1995). Sphingolipid Composition in Bacteroides Species. Anaerobe 1, 135–139. doi: 10.1006/anae.1995.1009
Kawahara, K., Kubota, M., Sato, N., Tsuge, K., Seto, Y. (2002). Occurrence of an Alpha-Galacturonosyl-Ceramide in the Dioxin-Degrading Bacterium Sphingomonas Wittichii. FEMS Microbiol. Lett. 214, 289–294. doi: 10.1111/j.1574-6968.2002.tb11361.x
Kawahara, K., Kuraishi, H., Zahringer, U. (1999). Chemical Structure and Function of Glycosphingolipids of Sphingomonas Spp and Their Distribution Among Members of the Alpha-4 Subclass of Proteobacteria. J. Ind. Microbiol. Biotechnol. 23, 408–413. doi: 10.1038/sj.jim.2900708
Kawahara, K., Lindner, B., Isshiki, Y., Jakob, K., Knirel, Y. A., Zahringer, U. (2001). Structural Analysis of a New Glycosphingolipid From the Lipopolysaccharide-Lacking Bacterium Sphingomonas Adhaesiva. Carbohydr Res. 333, 87–93. doi: 10.1016/S0008-6215(01)00111-2
Kawahara, K., Matsuura, M., Danbara, H. (1990). Chemical Structure and Biological Activity of Lipooligosaccharide Isolated From Sphingomonas Paucimobilis, A Gram-Negative Bacterium Lacking Usual Lipopolysaccharide. Jpn J. Med. Sci. Biol. 43, 250.
Kawahara, K., Moll, H., Knirel, Y. A., Seydel, U., Zahringer, U. (2000). Structural Analysis of Two Glycosphingolipids From the Lipopolysaccharide-Lacking Bacterium Sphingomonas Capsulata. Eur. J. Biochem. 267, 1837–1846. doi: 10.1046/j.1432-1327.2000.01189.x
Kawahara, K., Sato, N., Tsuge, K., Seto, Y. (2006). Confirmation of the Anomeric Structure of Galacturonic Acid in the Galacturonosyl-Ceramide of Sphingomonas Yanoikuyae. Microbiol. Immunol. 50, 67–71. doi: 10.1111/j.1348-0421.2006.tb03763.x
Kawahara, K., Seydel, U., Matsuura, M., Danbara, H., Rietschel, E. T., Zahringer, U. (1991). Chemical Structure of Glycosphingolipids Isolated From Sphingomonas Paucimobilis. FEBS Lett. 292, 107–110. doi: 10.1016/0014-5793(91)80845-T
Kawano, T., Cui, J., Koezuka, Y., Toura, I., Kaneko, Y., Motoki, K., et al. (1997). CD1d-Restricted and TCR-Mediated Activation of Valpha14 NKT Cells by Glycosylceramides. Science 278, 1626–1629. doi: 10.1126/science.278.5343.1626
Kilic, A., Senses, Z., Kurekci, A. E., Aydogan, H., Sener, K., Kismet, E., et al. (2007). Nosocomial Outbreak of Sphingomonas Paucimobilis Bacteremia in a Hemato/Oncology Unit. Jpn J. Infect. Dis. 60, 394–396.
Kim, E. Y., Ner-Gaon, H., Varon, J., Cullen, A. M., Guo, J., Choi, J., et al. (2020). Post-Sepsis Immunosuppression Depends on NKT Cell Regulation of mTOR/IFN-Gamma in NK Cells. J. Clin. Invest. 130, 3238–3252. doi: 10.1172/JCI128075
King, I. L., Fortier, A., Tighe, M., Dibble, J., Watts, G. F., Veerapen, N., et al. (2011). Invariant Natural Killer T Cells Direct B Cell Responses to Cognate Lipid Antigen in an IL-21-Dependent Manner. Nat. Immunol. 13, 44–50. doi: 10.1038/ni.2172
Kinjo, Y., Illarionov, P., Vela, J. L., Pei, B., Girardi, E., Li, X., et al. (2011). Invariant Natural Killer T Cells Recognize Glycolipids From Pathogenic Gram-Positive Bacteria. Nat. Immunol. 12, 966–974. doi: 10.1038/ni.2096
Kinjo, Y., Pei, B., Bufali, S., Raju, R., Richardson, S. K., Imamura, M., et al. (2008). Natural Sphingomonas Glycolipids Vary Greatly in Their Ability to Activate Natural Killer T Cells. Chem. Biol. 15, 654–664. doi: 10.1016/j.chembiol.2008.05.012
Kinjo, Y., Tupin, E., Wu, D., Fujio, M., Garcia-Navarro, R., Benhnia, M. R., et al. (2006). Natural Killer T Cells Recognize Diacylglycerol Antigens From Pathogenic Bacteria. Nat. Immunol. 7, 978–986. doi: 10.1038/ni1380
Kinjo, Y., Wu, D., Kim, G., Xing, G. W., Poles, M. A., Ho, D. D., et al. (2005). Recognition of Bacterial Glycosphingolipids by Natural Killer T Cells. Nature 434, 520–525. doi: 10.1038/nature03407
Knutsen, A. P., Bush, R. K., Demain, J. G., Denning, D. W., Dixit, A., Fairs, A., et al. (2012). Fungi and Allergic Lower Respiratory Tract Diseases. J. Allergy Clin. Immunol. 129, 280–91; quiz 292-3. doi: 10.1016/j.jaci.2011.12.970
Kobayashi, E., Motoki, K., Uchida, T., Fukushima, H., Koezuka, Y. (1995). KRN7000, A Novel Immunomodulator, and Its Antitumor Activities. Oncol. Res. 7, 529–534.
Kronenberg, M., Gapin, L. (2002). The Unconventional Lifestyle of NKT Cells. Nat. Rev. Immunol. 2, 557–568. doi: 10.1038/nri854
Kronenberg, M., Rudensky, A. (2005). Regulation of Immunity by Self-Reactive T Cells. Nature 435, 598–604. doi: 10.1038/nature03725
Kumar, H., Belperron, A., Barthold, S. W., Bockenstedt, L. K. (2000). Cutting Edge: CD1d Deficiency Impairs Murine Host Defense Against the Spirochete, Borrelia Burgdorferi. J. Immunol. 165, 4797–4801. doi: 10.4049/jimmunol.165.9.4797
La Gruta, N. L., Gras, S., Daley, S. R., Thomas, P. G., Rossjohn, J. (2018). Understanding the Drivers of MHC Restriction of T Cell Receptors. Nat. Rev. Immunol. 18, 467–478. doi: 10.1038/s41577-018-0007-5
Lanoix, J. P., Hamdad, F., Borel, A., Thomas, D., Salle, V., Smail, A., et al. (2012). Sphingomonas Paucimobilis Bacteremia Related to Intravenous Human Immunoglobulin Injections. Med. Mal Infect. 42, 37–39. doi: 10.1016/j.medmal.2011.10.002
Lantz, O., Bendelac, A. (1994). An Invariant T Cell Receptor Alpha Chain Is Used by a Unique Subset of Major Histocompatibility Complex Class I-Specific CD4+ and CD4-8- T Cells in Mice and Humans. J. Exp. Med. 180, 1097–1106. doi: 10.1084/jem.180.3.1097
Lee, Y. J., Holzapfel, K. L., Zhu, J., Jameson, S. C., Hogquist, K. A. (2013). Steady-State Production of IL-4 Modulates Immunity in Mouse Strains and Is Determined by Lineage Diversity of iNKT Cells. Nat. Immunol. 14, 1146–1154. doi: 10.1038/ni.2731
Legoux, F., Salou, M., Lantz, O. (2017). Unconventional or Preset Alphabeta T Cells: Evolutionarily Conserved Tissue-Resident T Cells Recognizing Nonpeptidic Ligands. Annu. Rev. Cell Dev. Biol. 33, 511–535. doi: 10.1146/annurev-cellbio-100616-060725
Legoux, F., Salou, M., Lantz, O. (2020). MAIT Cell Development and Functions: The Microbial Connection. Immunity 53, 710–723. doi: 10.1016/j.immuni.2020.09.009
Leite-De-Moraes, M. C., Hameg, A., Arnould, A., Machavoine, F., Koezuka, Y., Schneider, E., et al. (1999). A Distinct IL-18-Induced Pathway to Fully Activate NK T Lymphocytes Independently From TCR Engagement. J. Immunol. 163, 5871–5876.
Lin, J. N., Lai, C. H., Chen, Y. H., Lin, H. L., Huang, C. K., Chen, W. F., et al. (2010). Sphingomonas Paucimobilis Bacteremia in Humans: 16 Case Reports and a Literature Review. J. Microbiol. Immunol. Infect. 43, 35–42. doi: 10.1016/S1684-1182(10)60005-9
Long, X., Deng, S., Mattner, J., Zang, Z., Zhou, D., McNary, N., et al. (2007). Synthesis and Evaluation of Stimulatory Properties of Sphingomonadaceae Glycolipids. Nat. Chem. Biol. 3, 559–564. doi: 10.1038/nchembio.2007.19
Lotter, H., Gonzalez-Roldan, N., Lindner, B., Winau, F., Isibasi, A., Moreno-Lafont, M., et al. (2009). Natural Killer T Cells Activated by a Lipopeptidophosphoglycan From Entamoeba Histolytica Are Critically Important to Control Amebic Liver Abscess. PloS Pathog. 5, e1000434. doi: 10.1371/journal.ppat.1000434
Marinella, M. A. (2002). Cellulitis and Sepsis Due to Sphingobacterium. JAMA 288, 1985. doi: 10.1001/jama.288.16.1985-a
Matsuda, J. L., Naidenko, O. V., Gapin, L., Nakayama, T., Taniguchi, M., Wang, C. R., et al. (2000). Tracking the Response of Natural Killer T Cells to a Glycolipid Antigen Using CD1d Tetramers. J. Exp. Med. 192, 741–754. doi: 10.1084/jem.192.5.741
Mattner, J. (2013). Natural killer T (NKT) Cells in Autoimmune Hepatitis. Curr. Opin. Immunol. 25, 697–703. doi: 10.1016/j.coi.2013.09.008
Mattner, J., Debord, K. L., Ismail, N., Goff, R. D., Cantu, C., 3rd, Zhou, D., et al. (2005). Exogenous and Endogenous Glycolipid Antigens Activate NKT Cells During Microbial Infections. Nature 434, 525–529. doi: 10.1038/nature03408
Mattner, J., Savage, P. B., Leung, P., Oertelt, S. S., Wang, V., Trivedi, O., et al. (2008). Liver Autoimmunity Triggered by Microbial Activation of Natural Killer T Cells. Cell Host Microbe 3, 304–315. doi: 10.1016/j.chom.2008.03.009
Mohammed, J. P., Fusakio, M. E., Rainbow, D. B., Moule, C., Fraser, H. I., Clark, J., et al. (2011). Identification of Cd101 as a Susceptibility Gene for Novosphingobium Aromaticivorans-Induced Liver Autoimmunity. J. Immunol. 187, 337–349. doi: 10.4049/jimmunol.1003525
Mohammed, J. P., Mattner, J. (2009). Autoimmune Disease Triggered by Infection With Alphaproteobacteria. Expert Rev. Clin. Immunol. 5, 369–379. doi: 10.1586/eci.09.23
Molano, A., Park, S. H., Chiu, Y. H., Nosseir, S., Bendelac, A., Tsuji, M. (2000). Cutting Edge: The IgG Response to the Circumsporozoite Protein Is MHC Class II-Dependent and CD1d-Independent: Exploring the Role of GPIs in NK T Cell Activation and Antimalarial Responses. J. Immunol. 164, 5005–5009. doi: 10.4049/jimmunol.164.10.5005
Monteiro, M., Agua-Doce, A., Almeida, C. F., Fonseca-Pereira, D., Veiga-Fernandes, H., Graca, L. (2015). IL-9 Expression by Invariant NKT Cells Is Not Imprinted During Thymic Development. J. Immunol. 195, 3463–3471. doi: 10.4049/jimmunol.1403170
Monteiro, M., Almeida, C. F., Agua-Doce, A., Graca, L. (2013). Induced IL-17-Producing Invariant NKT Cells Require Activation in Presence of TGF-Beta and IL-1beta. J. Immunol. 190, 805–811. doi: 10.4049/jimmunol.1201010
Monteiro, M., Almeida, C. F., Caridade, M., Ribot, J. C., Duarte, J., Agua-Doce, A., et al. (2010). Identification of Regulatory Foxp3+ Invariant NKT Cells Induced by TGF-Beta. J. Immunol. 185, 2157–2163. doi: 10.4049/jimmunol.1000359
Moran, A. E., Holzapfel, K. L., Xing, Y., Cunningham, N. R., Maltzman, J. S., Punt, J., et al. (2011). T Cell Receptor Signal Strength in Treg and iNKT Cell Development Demonstrated by a Novel Fluorescent Reporter Mouse. J. Exp. Med. 208, 1279–1289. doi: 10.1084/jem.20110308
Morita, M., Motoki, K., Akimoto, K., Natori, T., Sakai, T., Sawa, E., et al. (1995). Structure-Activity Relationship of Alpha-Galactosylceramides Against B16-Bearing Mice. J. Med. Chem. 38, 2176–2187. doi: 10.1021/jm00012a018
Nair, S., Boddupalli, C. S., Verma, R., Liu, J., Yang, R., Pastores, G. M., et al. (2015). Type II NKT-TFH Cells Against Gaucher Lipids Regulate B-Cell Immunity and Inflammation. Blood 125, 1256–1271. doi: 10.1182/blood-2014-09-600270
Nakamatsu, M., Yamamoto, N., Hatta, M., Nakasone, C., Kinjo, T., Miyagi, K., et al. (2007). Role of Interferon-Gamma in Valpha14+ Natural Killer T Cell-Mediated Host Defense Against Streptococcus Pneumoniae Infection in Murine Lungs. Microbes Infect. 9, 364–374. doi: 10.1016/j.micinf.2006.12.003
Nieuwenhuis, E. E., Matsumoto, T., Lindenbergh, D., Willemsen, R., Kaser, A., Simons-Oosterhuis, Y., et al. (2009). Cd1d-Dependent Regulation of Bacterial Colonization in the Intestine of Mice. J. Clin. Invest. 119, 1241–1250. doi: 10.1172/JCI36509
Olson, C. M., Jr., Bates, T. C., Izadi, H., Radolf, J. D., Huber, S. A., Boyson, J. E., et al. (2009). Local Production of IFN-Gamma by Invariant NKT Cells Modulates Acute Lyme Carditis. J. Immunol. 182, 3728–3734. doi: 10.4049/jimmunol.0804111
Olszak, T., An, D., Zeissig, S., Vera, M. P., Richter, J., Franke, A., et al. (2012). Microbial Exposure During Early Life has Persistent Effects on Natural Killer T Cell Function. Science 336, 489–493. doi: 10.1126/science.1219328
Padgett, K. A., Selmi, C., Kenny, T. P., Leung, P. S., Balkwill, D. L., Ansari, A. A., et al. (2005). Phylogenetic and Immunological Definition of Four Lipoylated Proteins From Novosphingobium Aromaticivorans, Implications for Primary Biliary Cirrhosis. J. Autoimmun 24, 209–219. doi: 10.1016/j.jaut.2005.01.012
Reina, J., Bassa, A., Llompart, I., Portela, D., Borrell, N. (1991). Infections With Pseudomonas Paucimobilis: Report of Four Cases and Review. Rev. Infect. Dis. 13, 1072–1076. doi: 10.1093/clinids/13.6.1072
Rigaud, S., Fondaneche, M. C., Lambert, N., Pasquier, B., Mateo, V., Soulas, P., et al. (2006). XIAP Deficiency in Humans Causes an X-Linked Lymphoproliferative Syndrome. Nature 444, 110–114. doi: 10.1038/nature05257
Ryan, M. P., Adley, C. C. (2010). Sphingomonas Paucimobilis: A Persistent Gram-Negative Nosocomial Infectious Organism. J. Hosp Infect. 75, 153–157. doi: 10.1016/j.jhin.2010.03.007
Sag, D., Krause, P., Hedrick, C. C., Kronenberg, M., Wingender, G. (2014). IL-10-Producing NKT10 Cells Are a Distinct Regulatory Invariant NKT Cell Subset. J. Clin. Invest. 124, 3725–3740. doi: 10.1172/JCI72308
Schofield, L., McConville, M. J., Hansen, D., Campbell, A. S., Fraser-Reid, B., Grusby, M. J., et al. (1999). CD1d-Restricted Immunoglobulin G Formation to GPI-Anchored Antigens Mediated by NKT Cells. Science 283, 225–229. doi: 10.1126/science.283.5399.225
Sedimbi, S. K., Hagglof, T., Garimella, M. G., Wang, S., Duhlin, A., Coelho, A., et al. (2020). Combined Proinflammatory Cytokine and Cognate Activation of Invariant Natural Killer T Cells Enhances Anti-DNA Antibody Responses. Proc. Natl. Acad. Sci. U. S. A. 117, 9054–9063. doi: 10.1073/pnas.1920463117
Selmi, C., Balkwill, D. L., Invernizzi, P., Ansari, A. A., Coppel, R. L., Podda, M., et al. (2003). Patients With Primary Biliary Cirrhosis React Against a Ubiquitous Xenobiotic-Metabolizing Bacterium. Hepatology 38, 1250–1257. doi: 10.1053/jhep.2003.50446
Shute, T., Amiel, E., Alam, N., Yates, J. L., Mohrs, K., Dudley, E., et al. (2021). Glycolipid-Containing Nanoparticle Vaccine Engages Invariant NKT Cells to Enhance Humoral Protection Against Systemic Bacterial Infection But Abrogates T-Independent Vaccine Responses. J. Immunol. 206, 1806–1816. doi: 10.4049/jimmunol.2001283
Singh, A. K., Tripathi, P., Cardell, S. L. (2018). Type II NKT Cells: An Elusive Population With Immunoregulatory Properties. Front. Immunol. 9, 1969. doi: 10.3389/fimmu.2018.01969
Skold, M., Stenstrom, M., Sidobre, S., Hoglund, P., Kronenberg, M., Cardell, S. (2003). MHC-Dependent and -Independent Modulation of Endogenous Ly49 Receptors on NK1.1+ T Lymphocytes Directed by T-Cell Receptor Type. Immunology 110, 313–321. doi: 10.1046/j.1365-2567.2003.01741.x
Sriram, V., Du, W., Gervay-Hague, J., Brutkiewicz, R. R. (2005). Cell Wall Glycosphingolipids of Sphingomonas Paucimobilis Are CD1d-Specific Ligands for NKT Cells. Eur. J. Immunol. 35, 1692–1701. doi: 10.1002/eji.200526157
Stanic, A. K., De Silva, A. D., Park, J. J., Sriram, V., Ichikawa, S., Hirabyashi, Y., et al. (2003). Defective Presentation of the CD1d1-Restricted Natural Va14Ja18 NKT Lymphocyte Antigen Caused by Beta-D-Glucosylceramide Synthase Deficiency. Proc. Natl. Acad. Sci. U. S. A. 100, 1849–1854. doi: 10.1073/pnas.0430327100
Sundararaj, S., Zhang, J., Krovi, S. H., Bedel, R., Tuttle, K. D., Veerapen, N., et al. (2018). Differing Roles of CD1d2 and CD1d1 Proteins in Type I Natural Killer T Cell Development and Function. Proc. Natl. Acad. Sci. U. S. A. 115, E1204–E1213. doi: 10.1073/pnas.1716669115
Takeuchi, M., Hamana, K., Hiraishi, A. (2001). Proposal of the Genus Sphingomonas Sensu Stricto and Three New Genera, Sphingobium, Novosphingobium and Sphingopyxis, on the Basis of Phylogenetic and Chemotaxonomic Analyses. Int. J. Syst. Evol. Microbiol. 51, 1405–1417. doi: 10.1099/00207713-51-4-1405
Tatituri, R. V., Watts, G. F., Bhowruth, V., Barton, N., Rothchild, A., Hsu, F. F., et al. (2013). Recognition of Microbial and Mammalian Phospholipid Antigens by NKT Cells With Diverse TCRs. Proc. Natl. Acad. Sci. U. S. A. 110, 1827–1832. doi: 10.1073/pnas.1220601110
Thomas, W. R., Hales, B. J., Smith, W. A. (2005). Structural Biology of Allergens. Curr. Allergy Asthma Rep. 5, 388–393. doi: 10.1007/s11882-005-0012-1
Toh, H. S., Tay, H. T., Kuar, W. K., Weng, T. C., Tang, H. J., Tan, C. K. (2011). Risk Factors Associated With Sphingomonas Paucimobilis Infection. J. Microbiol. Immunol. Infect. 44, 289–295. doi: 10.1016/j.jmii.2010.08.007
Tupin, E., Benhnia, M. R., Kinjo, Y., Patsey, R., Lena, C. J., Haller, M. C., et al. (2008). NKT Cells Prevent Chronic Joint Inflammation After Infection With Borrelia Burgdorferi. Proc. Natl. Acad. Sci. U. S. A. 105, 19863–19868. doi: 10.1073/pnas.0810519105
Tuttle, K. D., Krovi, S. H., Zhang, J., Bedel, R., Harmacek, L., Peterson, L. K., et al. (2018). TCR Signal Strength Controls Thymic Differentiation of iNKT Cell Subsets. Nat. Commun. 9, 2650. doi: 10.1038/s41467-018-05026-6
Uldrich, A. P., Patel, O., Cameron, G., Pellicci, D. G., Day, E. B., Sullivan, L. C., et al. (2011). A Semi-Invariant Valpha10+ T Cell Antigen Receptor Defines a Population of Natural Killer T Cells With Distinct Glycolipid Antigen-Recognition Properties. Nat. Immunol. 12, 616–623. doi: 10.1038/ni.2051
Voyle, R. B., Beermann, F., Lees, R. K., Schumann, J., Zimmer, J., Held, W., et al. (2003). Ligand-Dependent Inhibition of CD1d-Restricted NKT Cell Development in Mice Transgenic for the Activating Receptor Ly49D. J. Exp. Med. 197, 919–925. doi: 10.1084/jem.20021615
Wei, B., Wingender, G., Fujiwara, D., Chen, D. Y., McPherson, M., Brewer, S., et al. (2010). Commensal Microbiota and CD8+ T Cells Shape the Formation of Invariant NKT Cells. J. Immunol. 184, 1218–1226. doi: 10.4049/jimmunol.0902620
Wieland Brown, L. C., Penaranda, C., Kashyap, P. C., Williams, B. B., Clardy, J., Kronenberg, M., et al. (2013). Production of Alpha-Galactosylceramide by a Prominent Member of the Human Gut Microbiota. PloS Biol. 11, e1001610. doi: 10.1371/journal.pbio.1001610
Wingender, G., Stepniak, D., Krebs, P., Lin, L., McBride, S., Wei, B., et al. (2012). Intestinal Microbes Affect Phenotypes and Functions of Invariant Natural Killer T Cells in Mice. Gastroenterology 143, 418–428. doi: 10.1053/j.gastro.2012.04.017
Wolf, B. J., Tatituri, R. V., Almeida, C. F., Le Nours, J., Bhowruth, V., Johnson, D., et al. (2015). Identification of a Potent Microbial Lipid Antigen for Diverse NKT Cells. J. Immunol. 195, 2540–2551. doi: 10.4049/jimmunol.1501019
Wolucka, B. A., McNeil, M. R., Kalbe, L., Cocito, C., Brennan, P. J. (1993). Isolation and Characterization of a Novel Glucuronosyl Diacylglycerol From Mycobacterium Smegmatis. Biochim. Biophys. Acta 1170, 131–136. doi: 10.1016/0005-2760(93)90062-E
Yang, R., Mele, F., Worley, L., Langlais, D., Rosain, J., Benhsaien, I., et al. (2020). Human T-Bet Governs Innate and Innate-Like Adaptive IFN-Gamma Immunity Against Mycobacteria. Cell 183, 1826–1847.e31. doi: 10.1016/j.cell.2020.10.046
Yuan, W., Dasgupta, A., Cresswell, P. (2006). Herpes Simplex Virus Evades Natural Killer T Cell Recognition by Suppressing CD1d Recycling. Nat. Immunol. 7, 835–842. doi: 10.1038/ni1364
Zajonc, D. M., Savage, P. B., Bendelac, A., Wilson, I. A., Teyton, L. (2008). Crystal Structures of Mouse CD1d-Igb3 Complex and Its Cognate Valpha14 T Cell Receptor Suggest a Model for Dual Recognition of Foreign and Self Glycolipids. J. Mol. Biol. 377, 1104–1116. doi: 10.1016/j.jmb.2008.01.061
Zeissig, S., Blumberg, R. S. (2013). Commensal Microbiota and NKT Cells in the Control of Inflammatory Diseases at Mucosal Surfaces. Curr. Opin. Immunol. 25, 690–696. doi: 10.1016/j.coi.2013.09.012
Zeissig, S., Murata, K., Sweet, L., Publicover, J., Hu, Z., Kaser, A., et al. (2012). Hepatitis B Virus-Induced Lipid Alterations Contribute to Natural Killer T Cell-Dependent Protective Immunity. Nat. Med. 18, 1060–1068. doi: 10.1038/nm.2811
Keywords: NKT cells, lipid antigens, cognate activation, tissue homeostasis, microbial infection, bystander activation
Citation: Vogt S and Mattner J (2021) NKT Cells Contribute to the Control of Microbial Infections. Front. Cell. Infect. Microbiol. 11:718350. doi: 10.3389/fcimb.2021.718350
Received: 31 May 2021; Accepted: 25 August 2021;
Published: 14 September 2021.
Edited by:
Luciana Balboa, Academia Nacional de Medicina (CONICET), ArgentinaReviewed by:
Catarina Almeida, The University of Melbourne, AustraliaAlvaro De Mingo Pulido, Moffitt Cancer Center, United States
Copyright © 2021 Vogt and Mattner. This is an open-access article distributed under the terms of the Creative Commons Attribution License (CC BY). The use, distribution or reproduction in other forums is permitted, provided the original author(s) and the copyright owner(s) are credited and that the original publication in this journal is cited, in accordance with accepted academic practice. No use, distribution or reproduction is permitted which does not comply with these terms.
*Correspondence: Jochen Mattner, am9jaGVuLm1hdHRuZXJAdWstZXJsYW5nZW4uZGU=