- 1Frontier Research Center, Toyota Motor Corporation, Toyota, Japan
- 2Department of Dermatology, Kyoto University Graduate School of Medicine, Kyoto, Japan
- 3Department of Microbiology, Kyoto University Graduate School of Medicine, Kyoto, Japan
The skin microbiota has been recognized to play an integral role in the physiology and pathology of the skin. The crosstalk between skin and the resident microbes has been extensively investigated using two-dimensional (2D) and three-dimensional (3D) cell cultures in vitro; however, skin colonization by multiple species and the effects of interspecific interactions on the structure and function of skin remains to be elucidated. This study reports the establishment of a mixed infection model, incorporating both commensal (Staphylococcus epidermidis) and pathogenic (Staphylococcus aureus) bacteria, based on a 3D human epidermal model. We observed that co-infecting the 3D epidermal model with S. aureus and S. epidermidis restricted the growth of S. aureus. In addition, S. aureus induced epidermal cytotoxicity, and the release of proinflammatory cytokines was attenuated by the S. aureus-S. epidermidis mixed infection model. S. epidermidis also inhibited the invasion of the deeper epidermis by S. aureus, eliciting protective effects on the integrity of the epidermal barrier. This 3D culture-based mixed infection model would be an effective replacement for existing animal models and 2D cell culture approaches for the evaluation of diverse biotic and abiotic factors involved in maintaining skin health.
Introduction
On the skin of humans and other mammals there resides an enormously diverse microbial community, known as the skin microbiota, which encompasses more than 1000 bacterial species belonging to 19 different phyla (Grice et al., 2009). Coagulase-negative staphylococci (CoNS), which comprise a diverse group of staphylococcal species, such as Staphylococcus epidermidis and Staphylococcus hominis, predominantly and commensally colonize the normal skin by adeptly inducing host tolerance (Chen et al., 2018). In contrast, Staphylococcus aureus, although persistently present in the skin of 10-20% of healthy individuals (Lowy, 1998), is a leading cause of skin and soft tissue infections and related to disease flares in inflammatory skin diseases, such as atopic dermatitis (AD). In AD patients, disease severity is positively associated with the abundance of S. aureus, whereas a predominance of S. epidermidis underlies less severe disease states (Byrd et al., 2017). Commensal staphylococci and S. aureus share similar ecological niches, and thus, they compete for surface adhesion and host colonization.
The skin microbiota plays crucial roles in maintaining skin homeostasis by interacting reciprocally with tissues and mediating immune responses, contributing not only to local homeostasis but also to the overall wellbeing of the organism. As the primary interface between the host and external pathogens, skin is opportunely positioned to act as the first barrier in the host defense mechanism. Epidermal keratinocytes and Langerhans cells, along with the resident immune cells in the dermis, orchestrate early immune responses against external pathogens by inducing both innate and acquired immune responses (Kupper and Fuhlbrigge, 2004). Interleukin (IL)-1, a member of proinflammatory cytokines, is centrally involved in the innate immune response against S. aureus infection. The release of the mature form of IL-1α from keratinocytes provides a rapid response against invasion by S. aureus by inducing the production of various chemokines that promote neutrophil recruitment for pathogen clearance (Olaru and Jensen, 2010). In contrast, the response mediated by IL-1β is stimulated by an intradermal S. aureus infection. IL-1β is synthesized in keratinocytes; however, it is an inactive precursor that is processed by caspase-1 only after leaving the cell, in a manner that depends on the assembly of inflammasomes (Franchi et al., 2009; Schroder and Tschopp, 2010; Shimada et al., 2010; Czachor et al., 2015).
Commensal staphylococci are capable of counteracting the pathogenic effects of S. aureus, conferring the skin with considerable resilience to dysbiosis and consequent barrier impairment and inflammation (Iwase et al., 2010; Janek et al., 2016; Byrd et al., 2017; Nakatsuji et al., 2017; Domenico et al., 2019). Persistent skin colonization of both S. aureus and commensal staphylococci is enabled and sustained by the formation of biofilms. S. aureus biofilms have been found to occur in the eccrine ducts of AD skin, influencing the secretion of keratinocyte-derived cytokines and inducing the differentiation and apoptosis of keratinocytes (Allen et al., 2014; Tankersley et al., 2014). These activities may act to disrupt barrier function and promote disease pathogenesis as well as allergen sensitization. The biofilm matrix also makes bacteria less susceptible to phagocytosis, which sets the stage for adaptive immune responses. Recent evidence suggests that skin commensal-related factors may directly affect the capacity of S. aureus to adhere and colonize the epidermis, thereby altering its pathogenic behavior by targeting biofilms. For instance, S. epidermidis secretes high levels of extracellular serine protease (Esp), which degrades the ligand protein for S. aureus adhesion, and other proteins required for biofilm formation, thereby inhibiting S. aureus colonization (Iwase et al., 2010; Sugimoto et al., 2013; Vandecandelaere et al., 2014). S. epidermidis-derived Esp also increases the susceptibility of S. aureus biofilms to immune system effector mechanisms. In this context, human β-defensin 2 (hBD2), an antimicrobial peptide (AMP) produced by epidermal keratinocytes, has been found to efficiently eradicate S. aureus biofilms when acting synergistically with Esp (Iwase et al., 2010). In addition, some CoNS species, particularly S. epidermidis and S. hominis, have been reported to selectively eliminate S. aureus by producing AMPs that act synergistically with the human AMP LL-37 (Nakatsuji et al., 2017). These findings support the notion that S. aureus, other staphylococci, and phylogenetically similar species, compete to gain their own ecological niche on the skin surface by interacting with each other.
The crosstalk between the skin and resident microbes has been investigated in vitro; however, potential interactions between species have remained inadequately evaluated. Recently, Jordana-Lluch et al. (2020) explored interspecific and microbe-keratinocyte interactions using two-dimensional (2D) cultures of immobilized keratinocytes colonized by a polymicrobial community that consisted of both commensals and pathogens. They demonstrated that the presence of commensals could limit the growth and biofilm production of the pathogens, thereby reducing pathogen-induced damage to the keratinocytes. Three-dimensional (3D) epidermal models have been established as a valuable alternative to 2D keratinocyte cultures, as they more realistically mimic the morphology and physiology of normal human epidermis as well as the liquid-air interface microenvironment of the skin surface. In particular, cells in 3D epidermal models, unlike those in 2D cultures, can differentiate and develop into subsets of cells with different functional states and form tissue structures (Chen et al., 2019). 3D epidermal models have also been shown to be appropriate for assessing bacterial transmigration and cell death over extended periods of time (Barua, 2019). Indeed, 3D epidermal models have been used to study the adherence, growth, and localization of microbes and their implications in epidermal immune responses; however, these studies have been limited to single-species colonization (Rademacher et al., 2018).
In this study, we aimed to develop and validate an epidermal mixed infection model using S. aureus and S. epidermidis with a 3D human epidermal model. The established 3D epidermal mixed infection model, involving both commensal and pathogenic staphylococci, could provide a platform for investigating the commensal-pathogen-epidermis trialogue under diverse colonization scenarios as well as identifying environmental factors or therapeutic candidates capable of improving skin eubiosis and health.
Materials and Methods
Bacterial Strains
Staphylococcus aureus (strain USA300, ATCC BAA-1717) and Staphylococcus epidermidis (ATCC 35984 or RP62A, biofilm positive) were obtained from American Type Culture Collection (ATCC, Manassas, VA). Bacterial strains were preserved as 15% glycerol stocks at -80°C and grown on tryptic soy agar (TSA) plates (BD Biosciences, Franklin Lakes, NJ) at 37°C.
3D Epidermal Equivalent
LabCyte EPI-MODEL (12 inserts) and assay medium were purchased from Japan Tissues Engineering (Aichi, Japan). The LabCyte EPI-MODEL consisted of normal human epidermal keratinocytes whose biological origin is human neonatal foreskin. This 3D skin model consists of multiple layers similar to the structure of native human epidermis (Katoh et al., 2009). The LabCyte EPI-MODEL was incubated according to the manufacturer’s instructions. Specifically, incubation with 1 mL of pre-warmed medium per well at 37°C in a humidified atmosphere with 5% CO2 was performed overnight, prior to the colonization experiments.
Colonization of the 3D Epidermal Equivalent
S. aureus and S. epidermidis were streaked on TSA plates and grown overnight at 37°C. For each strain, a single colony was transferred to a liquid medium of Brain Heart Infusion (BHI) (Nissui Pharmaceutical, Tokyo, Japan) and incubated overnight (16-20 h) at 37°C with reciprocal shaking at 130 rpm. The bacterial cells were pelleted at 5000 ×g for 5 min, washed twice to remove residual medium, and resuspended in normal saline. At this point, the concentration of bacterial cells as colony forming units per mL (CFU/mL) for each strain was estimated by measuring the optical density at 600 nm (OD600). Subsequently, the suspension of S. aureus was diluted with normal saline to 4 × 104 CFU/mL, and the suspension of S. epidermidis was diluted to 4 × 105 and 4 × 106 CFU/mL. The suspensions of S. aureus and S. epidermidis were also diluted and mixed appropriately to yield a final concentration of 4 × 104 CFU/mL for S. aureus and 4 × 105/4 × 106 CFU/mL for S. epidermidis. Given that 25 μL of each bacterial preparation was applied onto the surface of the 3D epidermal equivalent, the final bacterial cell counts per well (CFU/well) were 103 CFU/mL for S. aureus, which has been previously used to generate a surgical wound infection in mice (McLoughlin et al., 2006), 104 and 105 CFU/mL for S. epidermidis, and S. aureus vs. S. epidermidis = 103:104/105 CFU/mL, respectively. After inoculation, the 3D epidermal tissues were incubated at 37°C in a humidified atmosphere with 5% CO2 for 48 h, with the medium changed after 24 h. The colonization protocols are schematically presented in Figure 1.
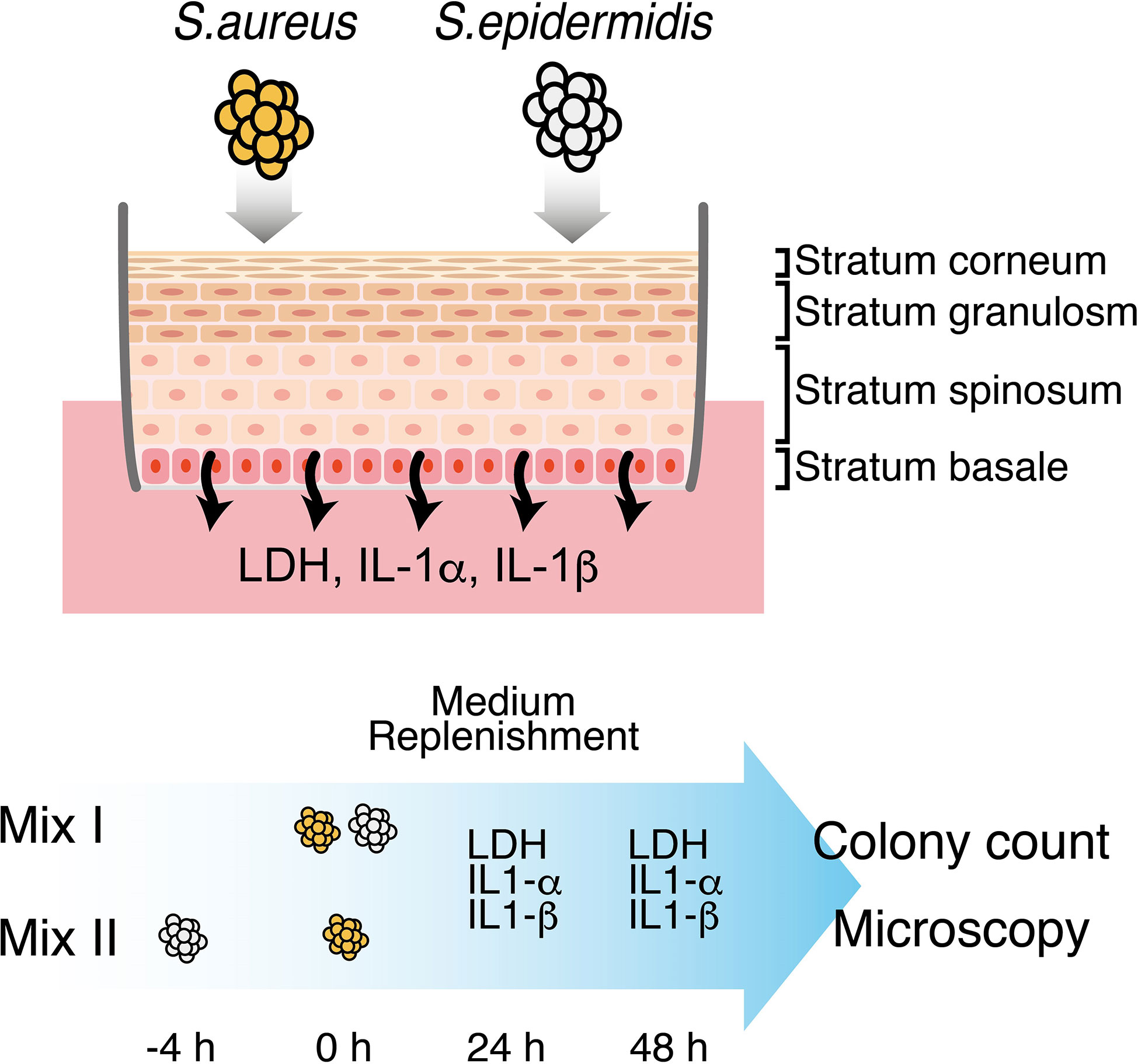
Figure 1 Schematic representation of establishing the mixed infection model of S. aureus and S. epidermidis. S. aureus and S. epidermidis were inoculated onto the surface of a fully-differentiated, stratified 3D epidermal model following two different protocols: (I) S. aureus and S. epidermidis were pre-mixed and inoculated simultaneously; (II) S. epidermidis was inoculated 4h prior to S. aureus. Culture media were replenished at 24h and collected at 24h and 48h to monitor the extracellular levels of LDH, IL-1α and IL-1β. At 48h, S. aureus and S. epidermidis on the surface of the 3D epidermal model were harvested and then enumerated by plate count. Intact epidermal tissues along with the Staphylococci were also preserved and processed for the analysis of bacterial distribution and migration using confocal fluorescence microscopy.
Cytotoxicity
The culture media were collected at 24 h and 48 h, and the cytotoxicity was determined using the Cytotoxicity Assay Kit-WST (Dojindo, Kumamoto, Japan), a colorimetric assay based on the activity of lactate dehydrogenase (LDH) released due to cell damage (Figure 1). The level of LDH was quantified by measuring the absorbance at 490 nm. The absorbance data were normalized to those of the control condition (i.e., incubation of the 3D epidermal equivalent with normal saline) (Kaja et al., 2017).
Cytokines
The culture media collected at 24 h and 48 h were analyzed for the presence of IL-1α and IL-1β using enzyme-linked immunosorbent assay (ELISA) kits (Proteintech, Chicago, IL) according to the manufacturer’s instructions (Figure 1).
Determination of Viable Bacterial Numbers
Following colonization experiments, the bacterial cells in each well were collected by washing the epidermal tissue surface with 200 μL of normal saline with 0.1% Triton® X-100 (Nacalai Tesque, Kyoto, Japan). The bacterial cells were serially diluted and 100 μL of each sample was plated onto a selective agar for the isolation and differentiation of pathogenic staphylococci (Staphylococcus agar #110, consisted of 10 g/L pancreatic digest of Casein, 2.5 g/L yeast extract, 30 g/L gelatin, 2 g/L lactose, 10 g/L D-mannitol, 75 g/L NaCl, 5 g/L K2HPO4, 15 g/L agar, Nissui Pharmaceutical, Tokyo, Japan) and incubated at 37°C for 24-48 h for the final enumeration of viable S. aureus and S. epidermidis (Figure 1).
Confocal Fluorescence Microscopy
After 48 h of cultivation, intact epidermal tissues with colonizing staphylococci were collected by cutting with a scalpel and embedding them perpendicularly in the optimal cutting temperature™ compound (Tissue-Tek; Sakura Finetechnical Co., Ltd., Tokyo, Japan) in the specimen holder of a microtome-cryostat, prior to frozen sectioning. The tissues were cut into ten-micrometer thick cryo-sections and mounted on gelatin-coated slides, which were then stored at -80°C until further analysis. The sections were immunostained after being fixed for 15 min with 100% methanol chilled on ice. For blocking, the sections were incubated with 5% (v/v) normal Goat serum (Vector Laboratories, Burlingame, CA) or 5% (w/v) bovine serum albumin (Biosearch Technologies, Petaluma, CA) in phosphate-buffered saline (PBS) for 60 min at room temperature. The sections were subsequently incubated overnight at 4°C with anti-Staphylococcus aureus (ab20920, Abcam, Cambridge, UK), anti-gram-positive bacteria (ab20344, ab267414, Abcam), and anti-claudin 1 antibodies (ab15098, Abcam) for the specific staining of S. aureus, S. epidermidis, and claudin-1, respectively, followed by washing three times for 5 min with PBS. The sections were further incubated with 1:100 dilutions of goat anti-rabbit IgG (H+L) Alexa Fluor 647 (A21244, Thermo Fisher Scientific, Waltham, MA) and goat anti-mouse IgG Alexa Fluor 546 (H+L) (A11003, Thermo Fisher Scientific) at room temperature for 60 min. The nuclei were stained with 4’,6-diamidino-2-phenylindole (DAPI) (Sigma-Aldrich, St. Louis, MO). The stained sections were washed three times with PBS, mounted in ProLong Diamond Antifade Mountant without DAPI (Thermo Fisher Scientific), and visualized using a confocal scanning laser microscope (Nikon A1 confocal microscope, Nikon, Tokyo, Japan).
Statistical Analysis
The data were analyzed using one-way analysis of variance and the Tukey-Kramer post hoc test for all pairs. Data are presented as the mean ± standard error of the mean (S.E.), and two-sided P values less than 0.05 were considered statistically significant.
Results
S. epidermidis Restricted the Colonization of S. aureus on the Epidermal Mixed Infection Model
S. aureus and S. epidermidis mixed infection was established by following one of the two protocols: (1) colonizing the 3D human epidermal model simultaneously with 103 CFU/well of S. aureus and 104 or 105 CFU/well of S. epidermidis or (2) colonizing the epidermal model with 104 or 105 CFU/well of S. epidermidis 4 h prior to colonization with 103 CFU/well of S. aureus, which was intended to allow S. epidermidis to propagate before the rapidly growing and highly virulent S. aureus was introduced into the same niche. After 48 h of colonization, bacterial cells were harvested from the surfaces and viable bacteria were enumerated (Figure 1). For both protocols, given a fixed infective dose of S. aureus (i.e., 103 CFU/well), colonizing with S. aureus and S. epidermidis resulted in significantly reduced total bacterial load and abundance of S. aureus compared with S. aureus infection alone (Figure 2). Expectedly, inoculating S. epidermidis 4 h prior to S. aureus inoculation led to a reduced abundance of S. aureus compared with inoculating the two staphylococci at the same time (Figure 2). In the context of the simultaneous protocol, a further decrease in the abundance of S. aureus was observed in response to a 10-fold increase in the infective dose of S. epidermidis. Similarly, when S. epidermidis was inoculated 4 h prior to S. aureus, increasing the infective dose of S. epidermidis from 104 to 105 CFU/well further reduced the abundance of S. aureus; however, no statistically significant difference was observed. These observations suggest that the presence of S. epidermidis significantly restricted the colonization of the epidermal tissue by S. aureus compared with colonization with S. aureus alone for both inoculation protocols.
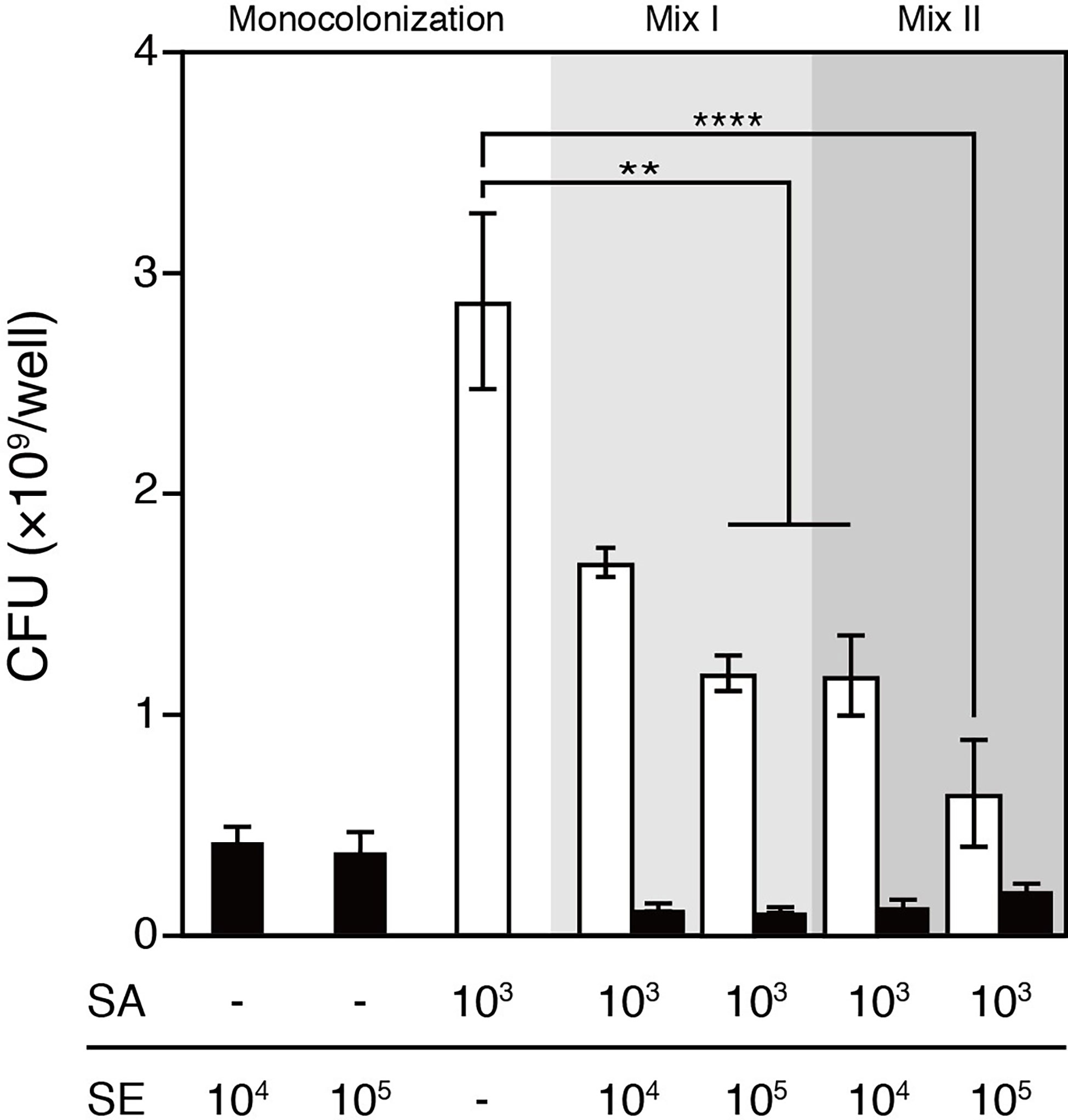
Figure 2 Viable counts of S. aureus and/or S. epidermidis that colonized the surface of the 3D epidermal model after 48h cultivation. The viable counts of S. aureus were significantly lower in the coculture with S epidermidis than in S. aureus monoculture. Increasing the infective dose of S. epidermidis from 104 to 105 CFU/well or inoculating S. epidermidis 4h prior to S. aureus further reduced the viable counts of S. aureus that colonized the epidermal surface. SE, S. epidermidis (black bars); SA, S. aureus (open bars); Mix I, S. epidermidis and S. aureus were inoculated simultaneously; Mix II, S. epidermidis was inoculated 4h prior to S. aureus. Data are presented as mean ± S.E. (n = 3-6). **p ≤ 0.01, ****p ≤ 0.0001. Tukey–Kramer Post Hoc test after One-way analysis of variance (ANOVA).
S. epidermidis Alleviated the Cytotoxic Effects of S. aureus on Epidermal Keratinocytes
The epidermal keratinocyte cytotoxicity induced by staphylococcal infection was assessed based on the activity of LDH released into the culture medium by damaged cells at 24 h and 48 h after inoculation (Figure 3). At the 24 h time point, significant cytotoxic responses were detected in keratinocytes exposed to S. aureus and to the bacterial mixture of 103 CFU/well of S. aureus and 104 CFU/well of S. epidermidis (Figure 3A). After an additional 24 h, the cytotoxic effects of S. aureus colonization and differences between colonization groups became more prominent, while the commensal S. epidermidis maintained low cytotoxicity, which was comparable to that of the saline control (Figure 3B). Notably, co-colonizing the epidermis with S. epidermidis and S. aureus significantly reduced S. aureus-induced keratinocyte cytotoxicity. The attenuating effects of S. epidermidis on S. aureus-induced cytotoxicity were further enhanced following a 10-fold increase in the infective dose of S. epidermidis for both colonization protocols, suggesting that inoculating S. epidermidis prior to S. aureus was effective in further reducing S. aureus-induced cytotoxicity.
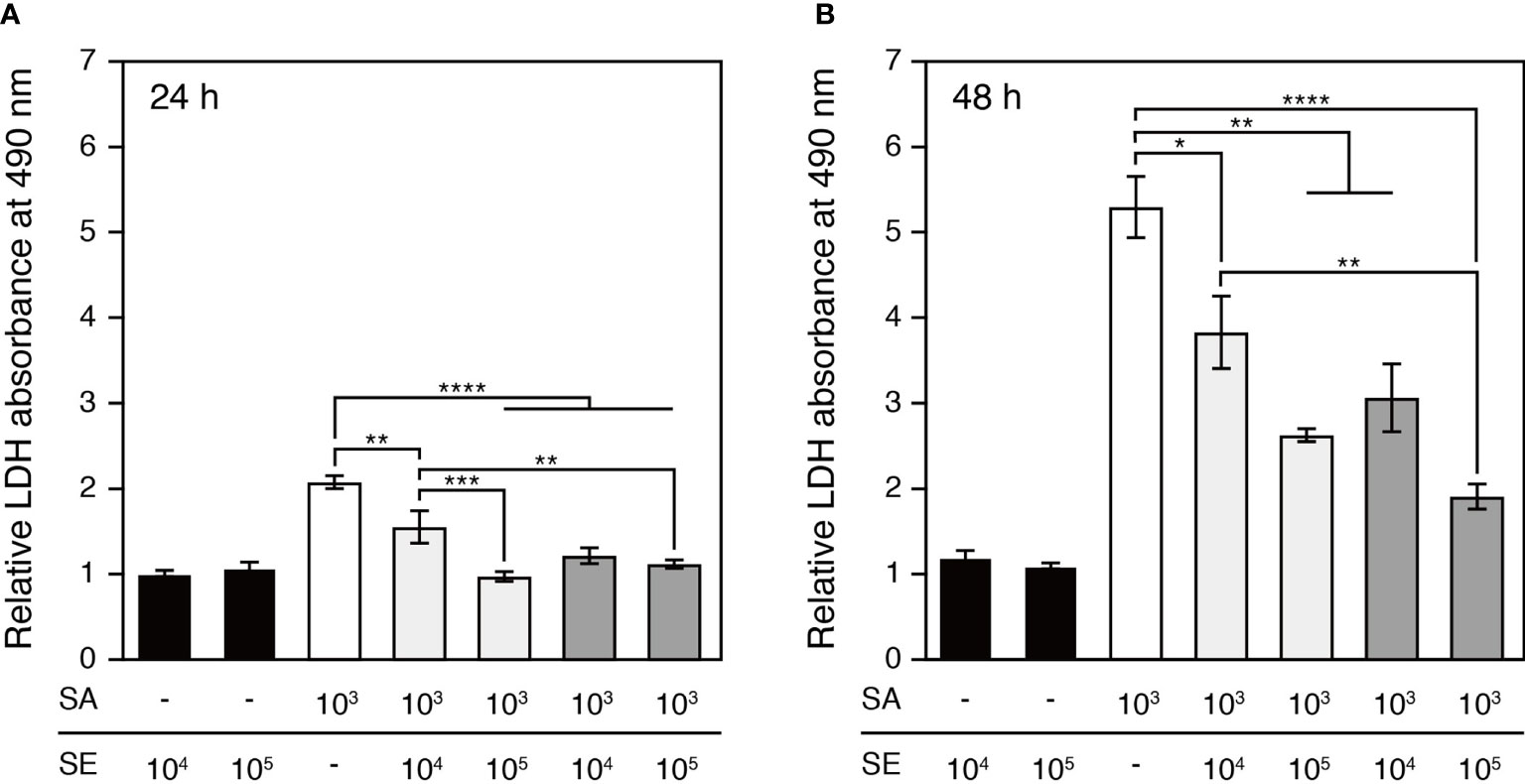
Figure 3 Cytotoxicity elicited by the colonization of S. aureus and/or S. epidermidis after 24h (A) and 48h (B) cultivation. Cytotoxicity was quantified based on the activity of LDH released by the damaged keratinocytes. The S. aureus-colonized epidermal tissue exhibited a significantly elevated level of cytotoxicity in comparison to the S. epidermidis-colonized tissues. The S. aureus-induced cytotoxicity was attenuated by the colonization of S. epidermidis to an increasing degree as S. epidermidis was inoculated at a greater infective dose or prior to S. aureus. SE, S. epidermidis (black bars); SA, S. aureus (open bars). Light grey bars: S. epidermidis and S. aureus were inoculated simultaneously; dark grey bars: S. epidermidis was inoculated 4h prior to S. aureus. Data are presented as mean ± S.E. (n = 3-6). *p ≤ 0.05, **p ≤ 0.01, ***p ≤ 0.001, ****p ≤ 0.0001. Tukey–Kramer Post Hoc test after One-way analysis of variance (ANOVA).
S. epidermidis Suppressed IL-1 Secretion Induced by S. aureus
We demonstrated that S. epidermidis restricted not only the colonization of S. aureus but also reduced its cytotoxicity in the mixed infection model. Next, we sought to evaluate the mechanism through which cytotoxicity was induced in this model. To this end, we focused on IL-1, which is a member of the proinflammatory cytokine family mainly involved in innate immune responses against S. aureus infection (Olaru and Jensen, 2010).
The culture medium was analyzed 48 h after colonization for both IL-1 protein forms: IL-1α (Figure 4A) and IL-1β (Figure 4B). At this time point, the epidermis colonized by S. aureus was characterized by elevated extracellular release of IL-1α and IL-1β (at 821.1 ± 45.1 pg/mL and 37.1 ± 3.1 pg/mL, respectively) compared with the tissue colonized by S. epidermidis (at 23.2 ± 14.7 pg/mL and 2.2 ± 1.5 pg/mL, respectively). In contrast, co-colonizing the epidermis with S. aureus and S. epidermidis decreased the levels of both cytokines, which were further reduced as the infective dose of S. epidermidis increased, and the cytokines were nearly neutralized by pre-colonizing the epidermis with this commensal at a concentration of 105 CFU/well.
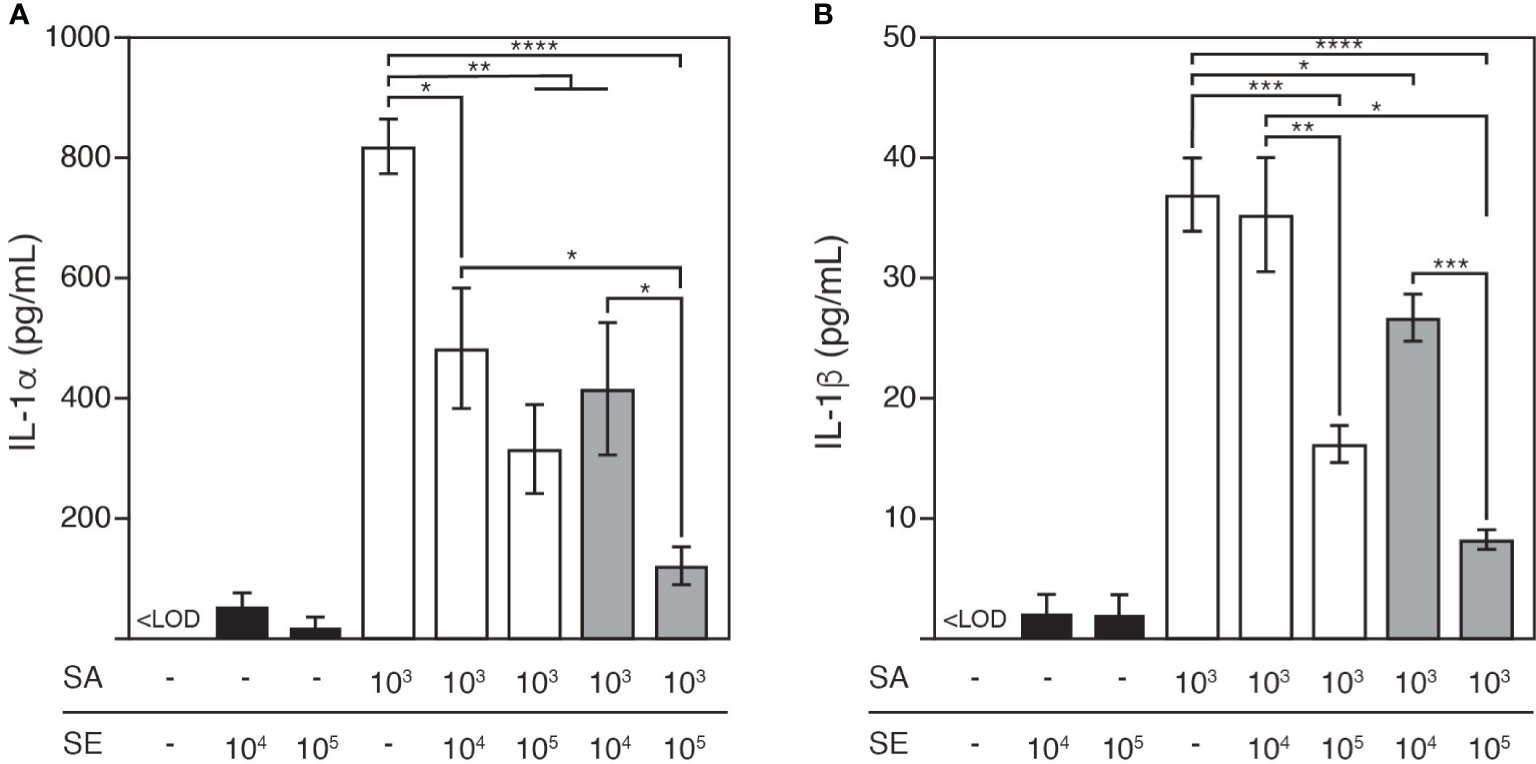
Figure 4 Release of pro-inflammatory cytokines, IL-1α (A) and IL-1β (B) in response to S. aureus and/or S. epidermidis colonization after 48h cultivation. S. aureus colonization led to significant release of IL-1α and IL-1β from the keratinocytes, which was reduced by the colonization of S. epidermidis to an increasing degree as S. epidermidis was inoculated at a greater infective dose or prior to S. aureus. LOQ, limit of quantification; SE, S. epidermidis (black bars); SA, S. aureus (open bars). Light grey bars: S. epidermidis and S. aureus were inoculated simultaneously; dark grey bars: S. epidermidis was inoculated 4h prior to S. aureus. Data are presented as mean ± S.E. (n = 3-6). *p ≤ 0.05, **p ≤ 0.01, ***p ≤ 0.001, ****p ≤ 0.0001. Tukey–Kramer Post Hoc test after One-way analysis of variance (ANOVA).
S. epidermidis Prevented S. aureus From Invading Deeper Tissues, Contributing to the Maintenance of Epidermal Barrier Integrity
Having demonstrated that S. aureus could infect epidermal keratinocytes and induce the secretion of proinflammatory cytokines, we further hypothesized that these skin infections may disrupt the barrier integrity, contributing to the release of proinflammatory cytokines by epidermal keratinocytes. To explore this hypothesis, we examined the bacterial distribution associated with different inoculation protocols in the 3D epidermal model. After 48 h of colonization, the distribution and migration of S. aureus and S. epidermidis in the stratified 3D epidermal model (Figure 5A) was visually inspected by using confocal microscopy. While S. epidermidis largely remained on the surface of stratum corneum (Figure 5B), the epidermis colonized by S. aureus was found to have S. aureus residing on the surface and penetrating the tissue, with a considerable proportion of S. aureus migrating to the bottom of the basal layer (Figure 5C). For the epidermis co-colonized with S. aureus and S. epidermidis, S. aureus was observed to be confined to the surface along with S. epidermidis, leaving the deeper tissue largely free of bacteria (Figures 5D, E). These observations provide strong evidence that the presence of S. epidermidis in the cutaneous microbial community prevents S. aureus from penetrating the epidermal layers and invading the deeper epidermis. Moreover, the effects of staphylococcal colonization on epidermal integrity were assessed by examining claudin-1, a tight junction protein that contributes to the physiological barrier in the skin. Microscopy images of the epidermis colonized by S. aureus revealed significantly intensified fluorescence associated with claudin-1 along with the invading S. aureus (Supplemental Figure 1). This result suggested that S. aureus could penetrate the tight junction and possibly affect epidermal barrier integrity.
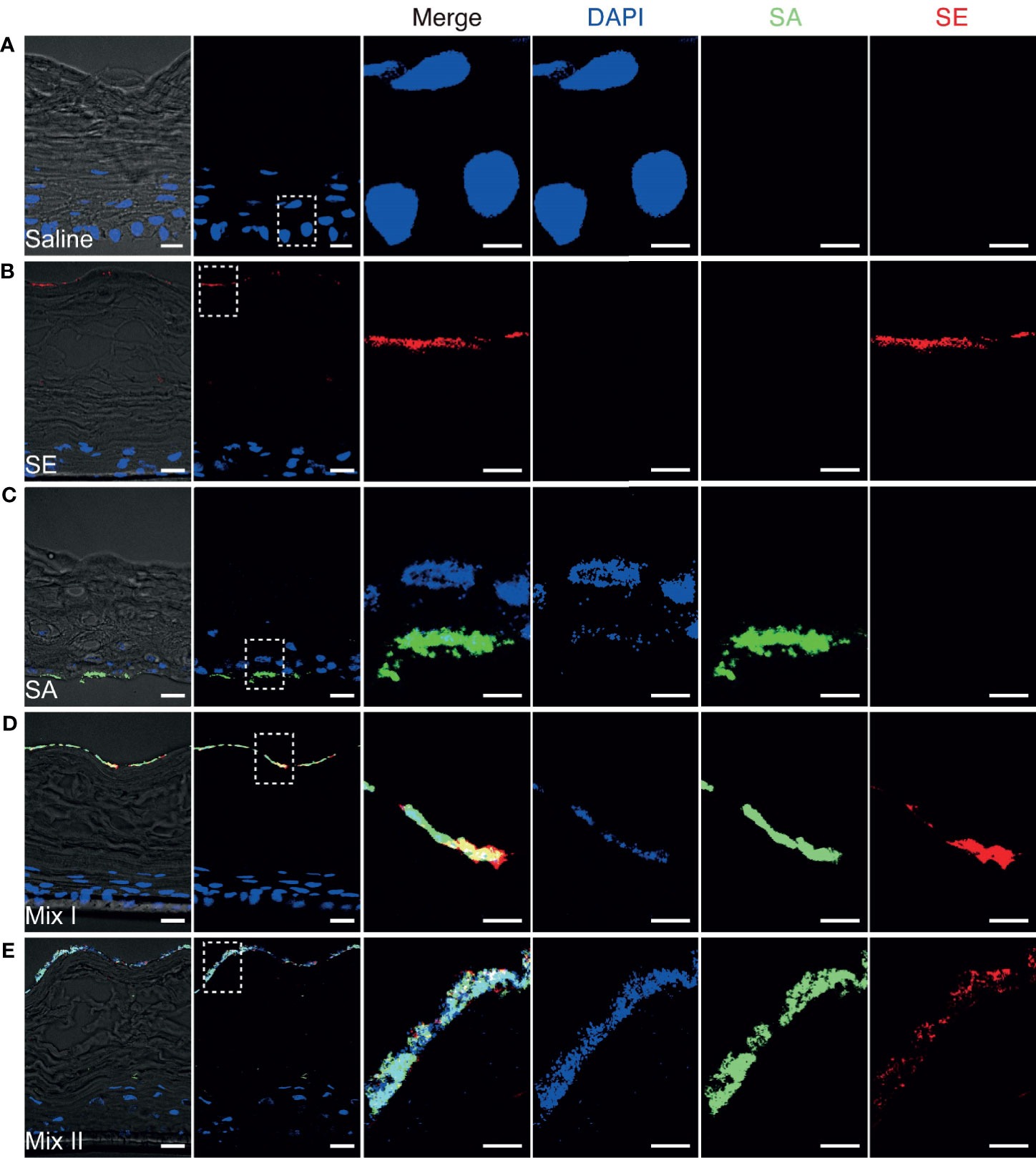
Figure 5 Confocal fluorescence microscopy illustrating the distribution and migration of the Staphylococci after 48h colonization. (A) The 3D human epidermal model exhibits differentiated, stratified layers; (B) S. epidermidis remained on the surface of the 3D epidermal model; (C) S. aureus penetrated through the epidermal layers and invaded the bottom layer of the epidermal tissue; (D, E) The presence of S. epidermidis prevented S. aureus from invading the deeper epidermis. SA, S. aureus was inoculated at an infective dose of 103 CFU/well; Mix I, S. epidermidis and S. aureus were inoculated simultaneously at infective dose of 103 and 105 CFU/well, respectively; Mix II, S. epidermidis was inoculated at an infective dose of 105 CFU/well and 4h prior to 105 CFU/well of S. aureus. Bar: 20 μm for the original view and 10 μm for the enlarged view.
Discussion
To the best of our knowledge, this is the first study to report the establishment of an epidermal mixed infection model consisting of S. epidermidis, S. aureus, and a 3D human epidermal model to mimic a microbial community comprising both commensals and pathogens sharing the same ecological niche on the skin. We first established the model by optimizing the infective dose and inoculation timing required for both bacteria to propagate and reach coexistence. We demonstrated that the bacterial burden exhibited a 105-fold change over a two-day period of colonization, as enumerated by counting viable bacteria. Given an infective dose of 103 CFU/well for S. aureus, we found that a minimal load of 104 CFU/well for S. epidermidis was required to sustain its propagation and biofilm formation during the crucial, initial phase of colonization in competition with S. aureus under the conditions used. We also demonstrated that the propagation of S. aureus induced measurable cell damage and inflammatory responses, which were evaluated by quantifying the elevated levels of extracellular LDH and proinflammatory cytokines.
In this model, S. epidermidis restricted the growth of S. aureus and attenuated S. aureus-induced cytotoxicity and proinflammatory responses, which provides evidence for the protective role of S. epidermidis in host defense by shaping the resident microbial community. Accumulating evidence suggests that several S. epidermidis extracellular products disrupt S. aureus biofilm formation, thereby affecting the capacity of S. aureus to adhere and colonize the epidermis (Iwase et al., 2010; Sugimoto et al., 2013; Vandecandelaere et al., 2014; Nakatsuji et al., 2017). Moreover, some S. epidermidis strains, including the strain RP62A used in this study, have been reported to produce agr type I autoinducing peptide that inhibits the S. aureus agr system, thus reducing the expression of proteases and phenol-soluble modulin α (PSMα), which disrupts the skin barrier and causes inflammation (Olson et al., 2014; Williams et al., 2019). These results provide an explanation for the reduction in cytotoxicity and proinflammatory cytokine release associated with mixed S. aureus and S. epidermidis infection compared with S. aureus infection alone.
IL-1α and IL-1β are the two primary cytokines that mediate early inflammatory responses in the epidermis. In the present study, IL-1α was detected at a markedly higher concentration than IL-1β. This is consistent with the findings of previous studies, which reported that IL-1α is constitutively synthesized as a biologically active precursor protein and released passively in response to cell injury (Dinarello, 2009), while IL-1β exists as an inactive precursor that requires processing by the inflammasome and caspase-1 before being secreted and is only induced upon the invasion of deeper tissue by S. aureus (Franchi et al., 2009).
By using immunostaining and confocal fluorescence microscopy, we visually inspected the colonization of staphylococci and their impact on the epidermal tissue. We demonstrated that S. epidermidis limited both the growth of S. aureus on the epidermal surface and its invasion of deeper tissue through the tight junction. To the best of our knowledge, this is the first study to report the microscopic characterization of the invasion of deeper epidermis by S. aureus as well as the consequent disruption of tight junctions in 3D organotypic cultures of the human epidermis. In contrast to S. aureus, S. epidermidis colonization was restricted to the epidermal surface. This result is consistent with that of a previous study, which reported that S. epidermidis is unable to penetrate the stratum corneum under normal physiological conditions (Percoco et al., 2013). Indeed, in multispecies communities, S. epidermidis is capable of growing a more confluent biofilm when the addition of S. aureus is delayed by 4 to 6 h, as reported by Stewart et al. (2017). A well-developed S. epidermidis biofilm may exert constraints on the overgrowth and migration of S. aureus, thereby serving as a “buffer zone” between the skin and invading pathogen. This may at least partially explain the significant improvement in the protective effects of S. epidermidis against S. aureus-induced cell damage and inflammation when it was inoculated 4 h prior to its pathogenic competitor.
Collectively, the present study represents a proof of concept for the construction of a model of a skin microbial community using 3D human epidermal model. Since the 3D epidermal model lacks skin appendages and resident immune cells, this mixed infection model has limitations in simulating or predicting the in vivo responses of the human skin. However, unlike 2D cell cultures, this 3D mixed infection model facilitates the status of microbial colonization and invasion to be determined; in addition, it allows the associated epidermal cytotoxicity and inflammatory responses to be more realistically evaluated. This model could serve as a powerful tool for revealing the mechanisms underlying the growth, migration, and activity of multiple skin commensals sharing the same ecological niche to collectively shape the microbial community and affect the immune responses of the skin. This mixed infection model would be an effective replacement for existing animal models and 2D cell cultures for the evaluation of diverse biotic and abiotic factors related to skin health and disease pathogenesis.
Data Availability Statement
The original contributions presented in the study are included in the article/Supplementary Material. Further inquiries can be directed to the corresponding author.
Author Contributions
AI and KK conceived the study. AI, KK, NS and XL designed the experiments. XL, KK and RN performed the experiments. XL and NS analyzed the data. XL wrote the manuscript. TD performed the immunostaining and microscopic analyses. AI, KK, XL, NS, MI, IN, and SN critically reviewed the manuscript. All authors contributed to the article and approved the submitted version.
Conflict of Interest
Author KK, XL, NS, RN, MI, and AI were employed by company Toyota Motor Corporation.
The remaining authors declare that the research was conducted in the absence of any commercial or financial relationships that could be construed as a potential conflict of interest.
Publisher’s Note
All claims expressed in this article are solely those of the authors and do not necessarily represent those of their affiliated organizations, or those of the publisher, the editors and the reviewers. Any product that may be evaluated in this article, or claim that may be made by its manufacturer, is not guaranteed or endorsed by the publisher.
Supplementary Material
The Supplementary Material for this article can be found online at: https://www.frontiersin.org/articles/10.3389/fcimb.2021.712360/full#supplementary-material
Supplementary Figure 1 | Microscopy images of claudin-1 and the epidermis colonized by S. aureus. S. aureus did not affect the expression of barrier protein claudin-1 but penetrated the epidermal tight junction. Saline, control group. SA, S. aureus was inoculated at an infective dose of 103 CFU/well. Bar: 10 μm.
References
Allen, H., Vaze, N., Choi, C., Hailu, T., Tulbert, B., Cusack, C., et al. (2014). The Presence and Impact of Biofilm-Producing Staphylococci in Atopic Dermatitis. JAMA Dermatol. 150 (3), 260–265. doi: 10.1001/jamadermatol.2013.8627
Barua, N. (2019). Comparison of MRSA Skin Infection Models With HaCaT Keratinocytes and a 3D-Organotypic Skin Model. Access Microbiol. 1 (1A). doi: 10.1099/acmi.ac2019.po0351
Byrd, A., Deming, C., Cassidy, S., Harrison, O., Ng, W., Conlan, S., et al. (2017). Staphylococcus Aureus and Staphylococcus Epidermidis Strain Diversity Underlying Pediatric Atopic Dermatitis. Sci. Transl. Med. 9 (397), eaal4651. doi: 10.1126/scitranslmed.aal4651
Chen, Y., Fischbach, M., Belkaid, Y. (2018). Skin Microbiota-Host Interactions. Nature 553 (7689), 427–436. doi: 10.1038/nature25177
Chen, L., Wu, M., Jiang, S., Zhang, Y., Li, R., Lu, Y., et al. (2019). Skin Toxicity Assessment of Silver Nanoparticles in a 3D Epidermal Model Compared to 2D Keratinocytes. Int. J. Nanomed. 14, 9707–9719. doi: 10.2147/IJN.S225451
Czachor, A., Cho, Y., Lockey, R., Kolliputi, N. (2015). Kinases: A Remote Control in Inflammasome Activity. Cell Commun. Signal 9 (3), 285–287. doi: 10.1007/s12079-015-0287-5
Dinarello, C. A. (2009). Immunological and Inflammatory Functions of the Interleukin-1 Family. Annu. Rev. Immunol 27, 519–550. doi: 10.1146/annurev.immunol.021908.132612
Domenico, E. G. D., Cavallo, I., Captanio, B., Ascenzioni, F., Pimpinelli, F., Morrone, A., et al. (2019). Staphylococcus Aureus and the Cutaneous Microbiota Biofilms in the Pathogenesis of Atopic Dermatitis. Microorganisms 7, 301. doi: 10.3390/microorganisms7090301
Franchi, L., Eigenbrod, T., Muñoz-Planillo, R., Nuñez, G. (2009). The Inflammasome: A Caspase-1-Activation Platform That Regulates Immune Responses and Disease Pathogenesis. Nat. Immunol. 10 (3), 241–247. doi: 10.1038/ni.1703
Grice, E., Kong, H., Conlan, S., Deming, C., Davis, J., Young, A., et al. (2009). Topographical and Temporal Diversity of the Human Skin Microbiome. Science 324 (5931), 1190–1192. doi: 10.1126/science.1171700
Iwase, T., Uehara, Y., Shinji, H., Tajima, A., Seo, H., Takada, K., et al. (2010). Staphylococcus Epidermidis Esp Inhibits Staphylococcus Aureus Biofilm Formation and Nasal Colonization. Nature 465 (7296), 346–349. doi: 10.1038/nature09074
Janek, D., Zipperer, A., Kulik, A., Krismer, B., Peschel, A. (2016). High Frequency and Diversity of Antimicrobial Activities Produced by Nasal Staphylococcus Strains Against Bacterial Competitors. PloS Pathog. 12, e1005812. doi: 10.1371/journal.ppat.1005812s
Jordana-Lluch, E., Garcia, V., Kingdon, A., Singh, N., Alexander, C., Williams, P., et al. (2020). A Simple Polymicrobial Biofilm Keratinocyte Colonization Model for Exploring Interactions Between Commensals, Pathogens and Antimicrobials. Front. Microbiol. 11, 291. doi: 10.3389/fmicb.2020.00291
Kaja, S., Payne, A., Naumchuk, Y., Koulen, P. (2017). Quantification of Lactate Dehydrogenase for Cell Viability Testing Using Cell Lines and Primary Cultured Astrocytes. Curr. Protoc. Toxicol. 72, 2.26.1–2.26.10. doi: 10.1002/cptx.21
Katoh, M., Hamajima, F., Ogasawara, T., Hata, K.-I. (2009). Assessment of Human Epidermal Model LabCyte EPI-MODEL for In Vitro Skin Irritation Testing According to European Centre for the Validation of Alternative Methods (ECVAM)-Validated Protocol. J. Toxicol. Sci. 34 (3), 327–334. doi: 10.2131/jts.34.327
Kupper, T., Fuhlbrigge, R. (2004). Immune Surveillance in the Skin: Mechanisms and Clinical Consequences. Nat. Rev. Immunol. 4 (3), 211–222. doi: 10.1038/nri1310
Lowy, F. D. (1998). Staphylococcus Aureus Infections. N. Engl. J. Med. 339 (27), 2025–2027. doi: 10.1056/nejm199812313392716
McLoughlin, R., Solinga, R., Rich, J., Zaleski, K., Cocchiaro, J., Risley, A., et al. (2006). CD4+ T Cells and CXC Chemokines Modulate the Pathogenesis of Staphylococcus Aureus Wound Infections. Proc. Natl. Acad. Sci. 103 (27), 10408–10413. doi: 10.1073/pnas.0508961103
Nakatsuji, T., Chen, T., Narala, S., Chun, K., Two, A., Yun, T., et al. (2017). Antimicrobials From Human Skin Commensal Bacteria Protect Against Staphylococcus Aureus and Are Deficient in Atopic Dermatitis. Sci. Transl. Med. 9 (378), eaah4680. doi: 10.1126/scitranslmed.aah4680
Olaru, F., Jensen, L. (2010). Staphylococcus Aureus Stimulates Neutrophil Targeting Chemokine Expression in Keratinocytes Through an Autocrine IL-1α Signaling Loop. J. Invest. Dermatol. 130 (7), 1866–1876. doi: 10.1038/jid.2010.37
Olson, M., Todd, D., Schaeffer, C., Paharik, A., Van Dyke, M., Buttner, H., et al. (2014). Staphylococcus Epidermidis Agr Quorum-Sensing System: Signal Identification, Cross Talk, and Importance in Colonization. J. Bacteriol. 196 (19), 3482–3493. doi: 10.1128/JB.01882-14
Percoco, G., Merle, C., Jaouen, T., Ramdani, Y., Bénard, M., Hillion, M., et al. (2013). Antimicrobial Peptides and Pro-Inflammatory Cytokines Are Differentially Regulated Across Epidermal Layers Following Bacterial Stimuli. Exp. Dermatol. 22 (12), 800–806. doi: 10.1111/exd.12259
Rademacher, F., Simanski, M., Gläser, R., Harder, J. (2018). Skin Microbiota and Human 3D Skin Models. Exp. Dermatol. 27 (5), 489–494. doi: 10.1111/exd.13517
Schroder, K., Tschopp, J. (2010). The Inflammasomes. Cell 140 (6), 821–832. doi: 10.1016/j.cell.2010.01.040
Shimada, T., Park, B., Wolf, A., Brikos, C., Goodridge, H., Becker, C., et al. (2010). Staphylococcus Aureus Evades Lysozyme-Based Peptidoglycan Digestion That Links Phagocytosis, Inflammasome Activation, and IL-1β Secretion. Cell Host Microbe 7 (1), 38–49. doi: 10.1016/j.chom.2009.12.008
Stewart, E. J., Payne, D. E., Ma, T. M., Van Epps, J. S., Boles, B. R., Younger, Y. G., et al. (2017). Effect of Antimicrobial and Physical Treatments on Growth of Multispecies Staphylococcal Biofilms. Appl. Environ. Microbiol. 83 (12), e03483–16. doi: 10.1128/AEM.03483-16
Sugimoto, S., Iwamoto, T., Takada, K., Okuda, K., Tajima, A., Iwase, T., et al. (2013). Staphylococcus Epidermidis Esp Degrades Specific Proteins Associated With Staphylococcus Aureus Biofilm Formation and Host-Pathogen Interaction. J. Bacteriol. 195 (8), 1645–1655. doi: 10.1128/jb.01672-12
Tankersley, A., Frank, M., Bebak, M., Brennan, R. (2014). Early Effects of Staphylococcus Aureus Biofilm Secreted Products on Inflammatory Responses of Human Epithelial Keratinocytes. J. Inflammation 11, 17. doi: 10.1186/1476-9255-11-17
Vandecandelaere, I., Depuydt, P., Nelis, H., Coenye, T. (2014). Protease Production by Staphylococcus Epidermidis and Its Effect on Staphylococcus Aureus Biofilms. Pathog. Dis. 70 (3), 321–331. doi: 10.1111/2049-632x.12133
Keywords: skin microbiota, 3D human epidermal equivalent, Staphylococcus aureus, Staphylococcus epidermidis, cytotoxicity, skin inflammation
Citation: Kohda K, Li X, Soga N, Nagura R, Duerna T, Nakajima S, Nakagawa I, Ito M and Ikeuchi A (2021) An In Vitro Mixed Infection Model With Commensal and Pathogenic Staphylococci for the Exploration of Interspecific Interactions and Their Impacts on Skin Physiology. Front. Cell. Infect. Microbiol. 11:712360. doi: 10.3389/fcimb.2021.712360
Received: 20 May 2021; Accepted: 16 August 2021;
Published: 16 September 2021.
Edited by:
Vera Kozjak-Pavlovic, Julius Maximilian University of Würzburg, GermanyReviewed by:
Sarah Maddocks, Cardiff Metropolitan University, United KingdomSudip Das, University of Lausanne, Switzerland
Knut Ohlsen, Julius Maximilian University of Würzburg, Germany
Copyright © 2021 Kohda, Li, Soga, Nagura, Duerna, Nakajima, Nakagawa, Ito and Ikeuchi. This is an open-access article distributed under the terms of the Creative Commons Attribution License (CC BY). The use, distribution or reproduction in other forums is permitted, provided the original author(s) and the copyright owner(s) are credited and that the original publication in this journal is cited, in accordance with accepted academic practice. No use, distribution or reproduction is permitted which does not comply with these terms.
*Correspondence: Akinori Ikeuchi, YWtpbm9yaV9pa2V1Y2hpQG1haWwudG95b3RhLmNvLmpw
†These authors have contributed equally to this work and share first authorship