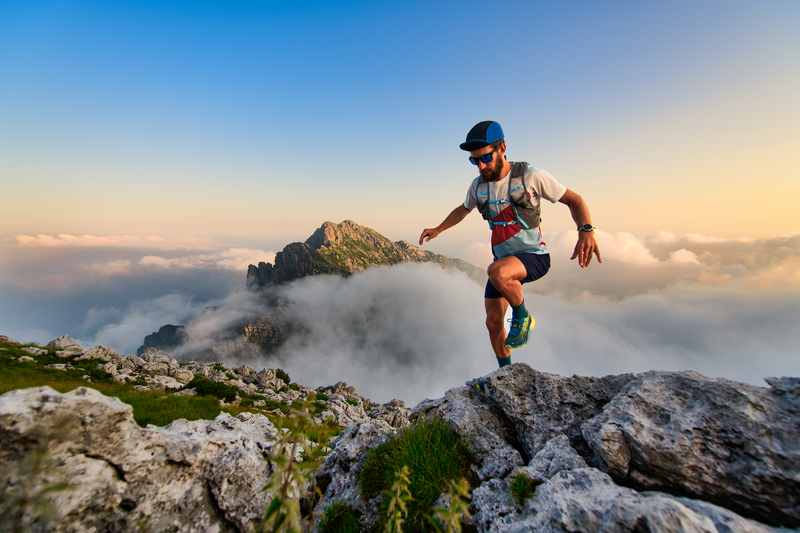
95% of researchers rate our articles as excellent or good
Learn more about the work of our research integrity team to safeguard the quality of each article we publish.
Find out more
REVIEW article
Front. Cell. Infect. Microbiol. , 14 October 2021
Sec. Microbiome in Health and Disease
Volume 11 - 2021 | https://doi.org/10.3389/fcimb.2021.710945
This article is part of the Research Topic The Human Microbiome: A New Frontier in Personalized Cancer Therapy View all 6 articles
Irinotecan (CPT11) and its active metabolite ethyl-10-hydroxy-camptothecin (SN38) are broad-spectrum cytotoxic anticancer agents. Both cause cell death in rapidly dividing cells (e.g., cancer cells, epithelial cells, hematopoietic cells) and commensal bacteria. Therefore, CPT11 can induce a series of toxic side-effects, of which the most conspicuous is gastrointestinal toxicity (nausea, vomiting, diarrhea). Studies have shown that the gut microbiota modulates the host response to chemotherapeutic drugs. Targeting the gut microbiota influences the efficacy and toxicity of CPT11 chemotherapy through three key mechanisms: microbial ecocline, catalysis of microbial enzymes, and immunoregulation. This review summarizes and explores how the gut microbiota participates in CPT11 metabolism and mediates host immune dynamics to affect the toxicity and efficacy of CPT11 chemotherapy, thus introducing a new concept that is called “microbiota-host-irinotecan axis”. Also, we emphasize the utilization of bacterial β-glucuronidase-specific inhibitor, dietary interventions, probiotics and strain-engineered interventions as emergent microbiota-targeting strategies for the purpose of improving CPT11 chemotherapy efficiency and alleviating toxicity.
Surgery, radiotherapy and chemotherapy are there main treatments for cancer treatment. For most cancers, surgery is still the primary and possible cure, while radiation and chemotherapy are mostly auxiliary or palliative treatments for advanced cancers (Furue, 2003). However, some of the cancers, such as endometrial cancer (Taylor et al., 2020) and testicular cancer (Baird et al., 2018), can be healed up by chemotherapy. Additionally, with the comparison of the local treatment of surgery and radiotherapy, chemotherapy works on the whole body as it can reach all organs and systems of the body by intravenous injection, thus it is possible to comprehensively kill cancer cells (Berman et al., 1993). Therefore, chemotherapeutic agents continue to be the backbone of oncotherapy for advanced cancer (Wallington et al., 2016).
As a topoisomerase I (TOP1) inhibitor, irinotecan (CPT11) was first approved in Japan for cancer treatment in 1995 (Bailly, 2019). Since then, CPT11 has been used widely in the treatment of metastatic or advanced solid tumors (e.g., gastric, pancreatic, ovarian, colorectal and other tumors). Up until now, CPT11 and its derivatives remain the primary anticancer drugs used in the clinic (Kciuk et al., 2020).
CPT11 kills cancer cells and normal proliferating cells indiscriminately. Hence, severe toxicity is induced in the form of myelosuppression, nausea, vomiting, diarrhea and peripheral neuropathy (Eng, 2009). All these adverse factors can incur treatment interruption/cessation, thereby imperiling the prognosis and quality of life of patients (Bailly, 2019). Furthermore, severe diarrhea and neutropenia symptoms are associated with a greater risk of dying (Campbell et al., 2017; Paulik et al., 2020). CPT11 use is correlated with diarrhea in 50–80% patients if used individually or in combination with other chemotherapy drugs (Gelibter et al., 2019). Moreover, the prevalence of severe diarrhea (defined as grade 3 or 4) caused by CPT11 can reach up to 22–44% (de Man et al., 2018). Studies have shown that CPT11 is detoxified mainly through uridine diphosphate glucuronosyltransferase 1A1 (UGT1A1)-catalyzed glucuronidation (Bruera and Ricevuto, 2020). UGT1A1 is a crucial phase-II enzyme that can metabolize CPT11 into inactive metabolites. Hence, medication guides for CPT11 were labeled by the US Food and Drug Administration (FDA) in 2005 and 2010. Patients carrying the homozygous UGT1A1*28 allele (known as Gilbert’s syndrome) have decreased hepatic expression of UGT1A1 enzyme, and a lower initial dose is recommended (Hulshof et al., 2020). However, this recommendation has been challenged because patients with Gilbert’s syndrome do not seem to be more sensitive when undergoing CPT11 chemotherapy than anticipated previously (Toffoli et al., 2006; Palomaki et al., 2009).
Thus, the metabolism and elimination of CPT11 does not appear to be a simple detoxification reaction. Other metabolic pathways may also be involved in this metabolic process. With the rapid development and innovation of multi-omics (e.g., microbial metabonomics, genomics, and immunomics), increasing numbers of researchers have found that the gut microbiota (GM) may be an important consideration in clinical oncology (Yachida et al., 2019; Dohlman et al., 2021). The human gut is colonized by ~1013 bacterial cells, which is defined as the GM (Ma et al., 2021). In addition, the genome of the GM is 100-fold larger than that of humans, which can encode various types of metabolic enzymes specific to microbiota (Xie et al., 2020). This phenomenon expands the metabolic capacity of the host and influences chemotherapy for patients in terms of efficacy, toxicity, and bioavailability (Ervin et al., 2020).
In this review, we focus on the complex metabolic processes of CPT11. We discuss in-depth how the GM is involved in this process, explaining and illustrating how the GM modulates CPT11 chemotherapy through three key mechanisms: microbial ecocline, catalysis of microbial enzymes, and microbial-mediated immunoregulation.
CPT11 is a semi-synthetic and water-soluble analog of camptothecin (CPT). The latter is a pentacyclic alkaloid isolated from Camptotheca acuminata, and is a TOPI inhibitor (Li et al., 2017). CPT11 has been used widely in the treatment of colon cancer (Reita et al., 2019), non-small-cell lung cancer (Zhang et al., 2015), pancreatic cancer (Glassman et al., 2018), gastric cancer (Fukuchi et al., 2020), and other cancer types (Mabuchi et al., 2019; Mizrahi et al., 2020). The primary antitumor mechanism of CPT11 is to inhibit DNA TOPI specifically (Pommier, 2006). TOPI modulates the DNA topology and “twists” DNA into a specific spatial structure during replication and transcription with its nuclear enzymatic activity (Gentry et al., 2011). CPT11 as well as its active metabolite ethyl-10-hydroxy-camptothecin (SN38) can bind to TOPI. The formation of a CPT11–TOPI–DNA complex blocks the DNA replication fork, the single strand of DNA breaks, and thereby leads to cell-cycle arrest and apoptosis (Bailly, 2019). Studies have shown that TOPI expression in cancer cells may be 14–16-times higher than that of normal cells surrounding the tumor. Owing to the dose-dependent inhibition of the enzyme, cancer cells are more susceptible to TOP toxicity (Burris and Fields, 1994).
Recent studies have found that CPT11 has other targets besides TOPI. The active metabolite ethyl-10-hydroxy-camptothecin (SN38) has been shown to significantly increase expression of p53 protein and pro-apoptosis proteins Bax, caspase-3, and caspase-9 in human hepatocellular carcinoma cell lines, and meanwhile decrease expression of the anti-apoptosis protein B-cell lymphoma (Bcl)-xL (Takeba et al., 2007). In addition, studies using nuclear magnetic resonance have demonstrated that CPT11 can bind directly to mouse double minute 2 homolog (MDM2), a ligase of tumor suppressor p53, and Bcl-xL. Moreover, p53 expression is increased by CPT11 only in the presence of MDM2 (Takeba et al., 2007). CPT11 has also been shown to activate p38. Early and short-duration activation of p38 is induced by a higher CPT11 concentration but, with a lower CPT11 concentration, the activation is delayed and sustained (Rudolf et al., 2013).
CPT11 can be used individually but it is more frequently combined with other cytotoxic drugs (e.g., 5-fluorouracil, oxaliplatin), monoclonal antibodies (e.g., cetuximab, bevacizumab) or with kinase inhibitors (Di Desidero et al., 2017; Chen and Jiang, 2019). Recent experimental and clinical studies have indicated that inhibitors of DNA repair, epigenetic modifications, signaling modulators, and immunotherapy can also be combined with CPT11 (Liu et al., 2021). Several CPT derivatives have been developed over the past two decades. They have been developed to show different activity for a given tumor type in the clinic, such as rubitecan, lurtotecan, difflomotecan, lurtotecan and others (Li et al., 2017). Such findings indicate that CPT11 is a major anticancer drug and may exert its effect through multiple pathways. Nevertheless, the detailed mechanism has not been clarified.
Various metabolic enzymes and drug transporters can participate in CPT11 metabolism directly and indirectly: human carboxylesterase 2 (CES2) (Xu et al., 2019), UGT1A1 (Hulshof et al., 2020), cytochrome P450 (CYP)3A4 (Riera et al., 2018), butyrylcholinesterase (Hyatt et al., 2005), adenosine triphosphate-binding cassette B1 (Garcia et al., 2018), and microbial β-glucuronidase (Chamseddine et al., 2019). Elaborating the complex metabolic processes of CPT11 (Figure 1) may offer a unique opportunity to understand its efficacy and toxicity, and promote “individualized” chemotherapy regimens.
Figure 1 CPT11 metabolism and excretion in the human body. The deactivation and activation of compounds (CPT11 or SN38) are represented by blue and red arrows, respectively. CPT11 induced delayed diarrhea are represented by red stellate symbol. The black solid arrows indicate the antineoplastic activity of CPT11 and SN38. The transport of CPT11 and its metabolites (SN38 and SN38G) between the blood circulation system, liver and gut lumen are pointed out by dashed-line arrows. CES1/2, carboxylesterase 1/2; APC, 7-ethyl-10-(4-N-(5-aminopentanoic acid)-1-piperidino) carbonyloxy-camptothecin; NPC, 7-ethyl-10-(4-amino-1-piperidino) carbonyloxy-camptothecin; CYP3A4, cytochrome P450; UGT, UDP-glucuronosyltransferase; β-Gus, β-glucuronidase.
CES1 and CES2 are the two major carboxylesterases in metabolizing endogenous and exogenous chemicals distributed in the blood, colon, kidney, and liver of humans. CES2 is primarily responsible for the metabolic activation of various prodrugs, including CPT11 (the affinity of CES2 for CPT11 is as high as that for CES1) (Xu et al., 2019). After intravenous injection, CPT11 flows through peripheral blood to the liver. Then, this agent can be metabolized to a more efficient compound, SN38, by hepatic CES enzymes (CES1 and CES2) (Alimonti et al., 2004). However, SN38 can also be generated by butyrylcholinesterase (hBChE) and CES in blood plasma, and the metabolic activity of hBChE is 6-times higher than that of CES (Morton et al., 1999; Rudakova et al., 2011). Subsequently, SN38 can be metabolized rapidly to inactive O-glucuronide (SN38G) by hepatic microsomal enzymes (UGT1A1 and UGT1A9), as well as UGT1A7 and UGT1A10 secreted by bile (de Man et al., 2018). Most notably, SN38G is generated almost immediately after SN38 production (Rivory et al., 1997).
Furthermore, intrahepatic CYP enzymes such as CYP3A4 and CYP3A5 also participate in the metabolism of CPT11. They convert CPT11 to additional inactivated metabolites, including 7-ethyl-10-[4-N-(5-aminopentanoic acid)-1-piperidino] carbonyloxy-camptothecin (APC) and 7-ethyl-10-(4-amino-1-piperidino) carbonyloxy-camptothecin (NPC) (Kuhn, 1998; Santos et al., 2000). The latter can be converted to SN38 by CES1 and CES2 enzymes (Sanghani et al., 2004).
Humans are colonized by 10–100 trillion interconnected microbial organisms, most of which are positioned on the gut (Adak and Khan, 2019). Moreover, the microbial genomes are about 100-fold greater than that of the human host, so the metabolic repertoire of theGM is larger than that of humans. Distinct from host genomes, the GM encodes various enzymes serving the function of metabolism and detoxification in unique and unnoticed ways (Zimmermann et al., 2019). Notably, >60 bioactive compounds undergo direct and indirect GM modifications. Specifically, the microbial transformations of chemical components often counteract the host metabolism by changing the pharmacokinetics parameters and pharmacodynamics characteristics of exogenous compounds (Spanogiannopoulos et al., 2016; Koppel et al., 2017).
As a microbiome-encoded enzyme, β-glucuronidase plays an important part in human health by metabolizing exogenous compounds in the intestinal tract. An elegant study profiled an atlas of human GM-encoded enzymes, and found that β-glucuronidase is derived mainly from four major microbial phyla: Bacteroidetes, Firmicutes, Verrucomicrobia, and Proteobacteria (Pollet et al., 2017). Furthermore, β-glucuronidase can beget drug-originated intestinal toxicity. Examples of such drugs include CPT11, of which the inactive metabolin SN38G is produced after complex metabolism in the liver. In the intestinal lumen, however, bacterial β-glucuronidase expressed by the host microbiota regenerate the active ingredient (SN38) and might induce severe diarrhea in a dose-limiting manner. Studies have shown that selective and highly effective inhibition of bacterial β-glucuronidase recedes the intestinal destruction induced by CPT11 without eliciting abnormal disturbance of serum pharmacokinetics (Wallace et al., 2010; Wallace et al., 2015). Moreover, reducing or eliminating the GM by broad-spectrum antibiotics shows lower intestinal toxicity in mice, and the same as that in germ-free mice (Pedroso et al., 2015; Mody et al., 2017). Taken together, the evidence mentioned above emphasizes the crucial role that bacterial β-glucuronidase has in affecting CPT11 metabolism and influencing chemotherapy toxicity.
CPT11 chemotherapy is administered to cancer patients. The physiology and ecology of the GM of patients given CPT11 tend to be disturbed and immature compared with that of healthy individuals (Hofseth et al., 2020). In addition, dysbiosis might occur after CPT11 treatment and further exacerbate the influence of harmful bacteria, thereby reducing efficacy or aggravating chemotherapy toxicity (Alexander et al., 2017). A “vicious circle” may be formed because CPT11 might further aggravate this disturbed status instead of redressing it. These inharmonious state can be called “microbiota-host-irinotecan axis dysregulation”. The microbiota-host-irinotecan axis refers to the intercommunication system between gut microbiota, host immune microenvironment and host drug metabolism after CPT11 chemotherapy. Understanding the mechanisms by which CPT11 alters the diversity, catalysis and metabolism of microbiota is crucial for real-time monitoring the dynamic variation of host immune response and CPT11 toxicity after CPT11 chemotherapy. Furthermore, in-depth discussion on the dynamics of the bacterial community and immunological condition in patients receiving CPT11 chemotherapy is needed, because it may provide a new opportunity for individualized cancer chemotherapy. Moreover, cancer chemotherapy combined with a “dynamics atlas” of the GM may be an exciting new strategy for cancer treatment.
The first stage in illustrating the interactive relationships and estimating the curative effect of CPT11 chemotherapy is to detail the variation in the diversity and ecology of microbiota. Some studies have shown how CPT11 affects microbial ecocline. After 3 days of CPT11 (125 mg/kg bodyweight) treatment in rats, Lin et al. observed an obvious change in microbiota composition, including an increased abundance of intestinal Enterobacteriaceae spp. and Clostridium cluster XL (Lin et al., 2012). Another study noted a prominent reduction of diversity in the microbiota community in colitis-associated mice with colon cancer upon 5-fluorouracil (25 mg/kg)/CPT11 (25 mg/kg) treatment (Wang et al., 2020b). Wang et al. found that the GM was enriched in mice suffering from intestinal mucositis induced by 5-fluorouracil (25 mg/kg)/CPT11 (25 mg/kg); the enriched bacterial species were from the genera Escherichia, Shigella, Clostridium, Parasutterella, Streptococcus, Lactococcus, Staphylococcus, and Enterococcus (Wang et al., 2020a). Wang et al. showed that CPT11 (150 mg/kg) administration decreased the richness of the GM markedly compared with that in control mice, but the level of bacteria of the phylum Proteobacteria and class Porphyromonadaceae and Mogibacteriaceae increased significantly (Wang et al., 2019). Several recent studies have indicated that CPT11 triggers the innate immune response to cause the secretion and release of proinflammatory cytokines such as interleukin (IL)-18, IL-1β, IL-6, and tumor necrosis factor-α (Stringer, 2013; Lian et al., 2017). In addition, the increase in the level of proinflammatory cytokines accelerates the discharge of mucin stored in goblet cells. There actions induce vacuole formation, which further influences intestinal microbial ecocline by reducing the number of adhesion sites and decreasing nutrition. Those actions cause a reduction in the number of symbiotic bacteria (e.g., Lactobacillus spp.) and an increase in the number of opportunistic pathogens (e.g., Escherichia coli) (Stringer, 2013). Notably, these changes in the GM are strikingly similar to those observed in intestinal inflammatory diseases such as ulcerative colitis, Crohn’s disease, and proctitis (Shivaji, 2021).
Changes in the composition of the intestinal microbiota caused by CPT11 have garnered interest. Studies have shown that intestinal dysbacteriosis might not be the leading cause of CPT11 toxicity. However, microbial metabolites [e.g., short-chain fatty acids (SCFAs)] and enzyme catalysis (e.g., β-glucuronidase) are correlated with the intestinal mucosal barrier and CPT11 detoxication, respectively (Lin et al., 2014; Gallotti et al., 2020; Hueso et al., 2020). As ubiquitous bacterial metabolites, SCFAs are generated mainly by fermentative bacteria. They maintain the intestinal epithelial barrier, inhibit the growth of colorectal cancer cells, and modulate the immune response (Cani and Jordan, 2018; Singh et al., 2014). The abundance of bacteria of the genera Lactobacillus and Bifidobacterium is decreased after CPT11 treatment. Moreover, these two species of bacteria generate or contribute to SCFA creation (LeBlanc et al., 2017; Markowiak-Kopec and Slizewska, 2020). Therefore, a vicious cycle arises whereby the decreased level of SCFAs induced by CPT11 therapy can aggravate its toxicity further.
Bacterial β-glucuronidase (or putative β-glucuronidase) has been identified from 43% of species in the Human Microbiome Database (Morkunas et al., 2020). However, a discrepant GM possesses a structurally diverse assortment of bacterial β-glucuronidase enzymes (Pollet et al., 2017). A study based on mouse-gut microbiome-encoded β-glucuronidase found that the latter were largely encoded by bacteria in the GM of the phyla Firmicutes (60%) and Bacteroidetes (21%), but the taxonomy for ~20% of the GM was not defined (Creekmore et al., 2019). β-glucuronidase from bacteria in mice or humans maintain a portion of an active site named the “bacterial loop” (Wallace et al., 2015; Creekmore et al., 2019). CPT11 can induce changes in microbial ecocline that are correlated with augmentation in bacteria from the family Enterobacteriaceae and E. coli, thereby leading to a higher level of β-glucuronidase in the intestine, and resulting in enhanced toxicity from β-glucuronidase (Stringer et al., 2008; Fujimura et al., 2010; Lin et al., 2012).
Immune-based therapeutic strategies for cancer have become increasingly attractive to researchers. Several immune-checkpoint inhibitors have been developed over the past decade (Meric-Bernstam et al., 2020). The importance of the immune-checkpoint inhibitor programmed cell death-1 was recognized with the award of the 2018 Nobel Prize in Physiology or Medicine to Professor James Allison. Therefore, researchers must monitor the vital functions of the immune system during oncotherapy. Conventional chemotherapy (including CPT11) operates mainly by blocking TOPI, thereby exerting a highly anti-proliferation effect upon cancer cells. However meanwhile, an antinomy may be involved that this aggravate cytotoxicity probably brings ‘off target’ effect responding to immune cells and the inflammatory microenvironment (Duffy and Greten, 2014).
Direct impact on the immune system. Xue et al. were the first to investigate the effects of CPT11 on the gut and systemic immune environment in tumor-bearing rats. They discovered that CPT11 facilitates a preponderance of activated T cells but induces hypo-reactivity in spleen cells (Xue et al., 2009). One study involving 133 patients showed that CPT11 administration led to 28% of patients experiencing severe neutropenia and 10% of patients suffering febrile neutropenia (van der Bol et al., 2010). A review by Logan et al. noted that cancer treatment using CPT11 resulted in (Logan et al., 2007). Those data suggest changes in the immunological micro-environment the activation of mitogen-activated protein kinase and nuclear factor kappa-B (NF-κB) signaling pathways which, ultimately, caused apoptosis of intestinal cells upon CPT11 treatment.
Indirect impact on immune cells mediated by the microbiota. An intact commensal microbiota contributes to cancer treatment and orients the therapeutic outcome from chemotherapy (Iida et al., 2013). The intestinal commensal microbiota is vital for the maintenance of the epithelial barrier and immune functions (Mukaida, 2014). Schluter et al. disclosed a fascinating association between gut bacteria and immune-cell dynamics; Faecalibacterium, Ruminococcus and Akkermansia were the taxa with the strongest association with immune-cell dynamics in the gut (Schluter et al., 2020). Bifidobacteriaceae are the only family of bacteria in the order of Bifidobacteriales. Bifidobacteriaceae are one of the preponderant bacteria of the GM, accounting for >1% of total intestinal bacteria (Picard et al., 2005; Russell et al., 2011; Bottacini et al., 2017). López et al. reported that dendritic cells (DCs) exposed to Bifidobacterium bifidum LMG 13195 stimulated the polarization of naïve T cells into functional T regulatory cells. Meanwhile, the IL-10 level was increased significantly after DCs were exposed to B. bifidum LMG13195 membrane vesicles in vitro (Lopez et al., 2012). This action could trigger a “domino effect” of local immunological suppression in the gut. Moreover, study showed that oral supplementation with B. infantis and B. bifidum in Balb/c mice reduced levels of endotoxins and inflammation (Griffiths et al., 2004).
Another probiotic, Lactobacillus salivarius LI01, shows immune modulation that can restore the levels of serum biomarkers (IL-1α, IL-5, IL-10) before the differentiation of naïve T cells towards immune homeostasis in germ-free Sprague–Dawley rats (Xia et al., 2021). A transcriptome study using a germfree murine mode revealed that Lactobacillus acidophilus NCFM (as immunostimulatory components) could coordinately “educate” the immune system without eliciting a detrimental immune response in the host (Goh et al., 2021). Furthermore, studies also showed that Lactobacillus spp. initiate apoptosis of cancer cells (especially colorectal cancer cells) by mediating apoptotic signals. Exopolysaccharides (EPS) generated by Lactobacillus plantarum NCU116 restrain the proliferation and induce the apoptosis in the mouse epithelial colorectal carcinoma cell line CT26 via toll-like receptor 2 and activation of the death receptor Jun (Zhou et al., 2017). Use of the human colorectal cancer line HT29 in vitro indicated that EPS from nine strains of Lactobacillus spp. significantly suppressed cell-cycle arrest at the G0/G1 phase and apoptosis (Di et al., 2018). Nevertheless, Nowak et al. discussed in detail the anti-proliferative and pro-apoptotic activity of Lactobacillus spp. and Bifidobacterium spp. in a review (Nowak et al., 2019). As mentioned above, CPT11 can cause a lower abundance of Bifidobacterium spp. and Lactobacillus spp. in the intestinal tract, which entails initiation of destabilization in the tumor immune environment.
CPT11 administration resulting in augmentation of the number of opportunistic pathogens can also lead to disturbance of the host immunologic state. As non-spore-forming Gram-negative bacteria, Escherichia spp. and Shigella spp. can spark acute mucosal inflammation. Escherichia spp. and Shigella spp. elicit a series of acute inflammation responses, including activation of caspase-1, to release IL-1β and IL-18 through infection of macrophages and epithelial cells (Sansonetti et al., 2000). Moreover, Escherichia spp. and Shigella spp. possessing the Shigella type-III secretion system generate various effector molecules (e.g., OspI, IpaH9.8, and PtdIns5P) through which Escherichia spp. and Shigella spp. use the NF-κB signaling pathway and thereby mediate expression of some proinflammatory cytokines (Okuda et al., 2005; Sperandio et al., 2008; Sanada et al., 2012; Puhar et al., 2013). Furthermore, Escherichia spp. and Shigella spp. induce a deviant adaptive immunity response. One study using a murine infection model revealed that Shigella flexneri mainly initiates a Th17-cell response to produce IL-17 and IL-22 (Sellge et al., 2010). Besides, the apoptotic death rate of DCs is augmented after treatment with the effectors (OspF) of Shigella spp. (Kim et al., 2008). Moreover, studies have shown that Shigella spp. intrude into T cells and inhibit migration of chemokines and chemokine receptor-induced lymphocytes, thereby affecting the function and dynamics of lymph nodes (Konradt et al., 2011; Salgado-Pabon et al., 2013). In particular, the chronic inflammatory status presenting in mice with deficiency of DNA-mismatch repair is often accompanied by enrichment of Escherichia spp. and Shigella spp. in the fecal sample, which aggravates colonic tumorigenesis (Lang et al., 2020). In conclusion, opportunistic pathogens trigger an anomalously local immunological micro-environment in the host, which can aggravate adverse reactions in the body and which may be new focus of ongoing research.
A cutting-edge research topic in regard to the GM is mediation of the efficacy and toxicity of CPT11 chemotherapy (Alexander et al., 2017) (Figure 2). As stated above, the GM impacts the host immunological state and pharmacodynamic metabolism pathways of CPT11. Thus, the GM may have a dual role in carcinogenesis as an oncogene and tumor suppressor (Bhatt et al., 2017). By regulation of intestinal microorganisms as an auxiliary strategy or by utilizing inhibitors that target microbial enzymes, the GM can improve CPT11 chemotherapy and alleviate toxicity (Figure 3).
Figure 2 Effects of microbiota on the trend of CPT11 chemotherapy efficacy and toxicity. Schematic presents the dualism’s functional role of microbiota in CPT11 chemotherapy. Lactobacillus spp, bifidobacterium spp, oncolytic viruses, and azide-modified phages can cooperate with CPT11, respectively, and exert immune activation, synergetic anti-proliferative and pro-apoptosis effect on tumor. That eventually enhances the anti-tumor efficacy on tumor. However, some microbes, such as Escherichia-shigella, Parasutterella, Streptococcus, Lactococcus, Staphylococcus and Clostridium difficile elicit a series of inflammation response by activating NF-κB pathway after infection of macrophages and epithelial cells, which aggravate the toxic reactions induced by CPT11, and impair chemotherapeutic outcome.
Figure 3 Manipulation of microbiota improve the outcomes of CPT11 chemotherapy. SN38 active), regenerated by microbial β-glucuronidases in the intestinal tract from SN38G (inactive), primarily targets topoisomerase 1, thereby causing breaks in DNA strands. SN38-induced DNA lesions lead to the apoptosis of intestinal epithelial cells, which destroys the epithelial barrier. Then, local inflammation and dysbiosis are triggered. In addition, shortening of intestinal villi and bacterial translocation can amplify this damage, and lead eventually to CPT11-induced diarrhea. Moreover, microbial dysbiosis can affect the development and progression of cancer by activating inflammatory signaling pathways. Antibiotics, β-glucuronidase inhibitors, and herbal medicines can be used to inhibit β-glucuronidases to alleviate intestinal symptoms. Probiotics mediate microbial metabolism and immunologic homeostasis by reconstruction of the intestinal microbiota, thereby synergizing with CPT11 chemotherapy. SCFAs, short-chain fatty acids; PAMP, pathogen-associated molecular patterns; IL-10, interleukin 10; TNF, tumor necrosis factor; β-Gus, β-glucuronidases; Th17, T helper 17; Top1, Topoisomerase I; NF-κB, nuclear factor-kappa B; Bax, BCL2-associated X; Bcl2, BCL2 apoptosis regulator.
Bacterial β-glucuronidase is a lysosomal exoglycosidase enzyme. It can cleave the glucuronic moiety to glucuronic acid and an aglycone, a process termed “deglucuronidation”. As a glucuronide prodrug, SN38G is non-toxic with highly hydrophilic characteristics, and can be subject to fast renal clearance so that it cannot to enter cells. However, SN38G enters the gut through the enterohepatic circulation, and is reactivated by β-glucuronidase generated from the GM (Pusztaszeri et al., 2007). Hence, β-glucuronidase prolongs the clearance time of CPT11 within the body and is, therefore, considered to be a direct promotor of CPT11-induced intestinal toxicity and delayed diarrhea (Wallace et al., 2010). Moreover, several investigations have demonstrated that the extent of intestinal damage induced by CPT11 is highly and positively correlated with the level of intestinal β-glucuronidase activity (Nishiike et al., 2013; Roberts et al., 2013). Targeted inhibition of intestinal bacterial β-glucuronidase has shown to be a promising therapeutic strategy for relieving the toxicity induced by CPT11 chemotherapy (Chamseddine et al., 2019). Most strikingly, a study conducted by Cheng et al. indicated that pharmacological inhibition of β-glucuronidase alleviates the intestinal injury caused by CPT11 without diminishing its anti-tumor effect (Cheng et al., 2019).
However, one study cast doubts on the crucial role of bacterial β-glucuronidase in CPT11-induced diarrhea; streptomycin ablated intestinal toxicity but did not restrain β-glucuronidase activity (Kurita et al., 2011). The reason for this result is probably because β-glucuronidase from different bacterial phyla has distinct differences with one another in terms of catalytic efficiency, substrate binding, and reaction rates (Wallace et al., 2015). Subsequently, an innovative study using a novel activity-based protein profiling platform identified various specific bacterial β-glucuronidase enzymes that could regenerate SN38 (Jariwala et al., 2020).
Explorations of inhibitors of bacterial β-glucuronidase to relieve CPT11-induced intestinal damage have increased in recent years. Initially, Ahmad et al. screened marketed drugs from the US FDA using purified β-glucuronidase from E. coli. They found that nialamide, isocarboxazid, phenelzine, amoxapine, and mefloquine had significant inhibitory activity in vitro, respectively (Ahmad et al., 2012). Three older drugs (aspartame, N-desmethylclozapine and gemifloxacin) have also been shown to have β-glucuronidase inhibitory activity using enzyme-based assays (Chen et al., 2020). In vivo studies have indicated that amoxapine alleviates CPT11 toxicity and enhances the anti-tumor efficacy (to a certain extent) in tumor-bearing mice, and that the pharmaceutical activity of CPT11 is associated with its inhibitory impact on β-glucuronidase from E. coli, Enterococcus spp., Streptococcus spp., Escherichia spp., and Staphylococcus spp. (Kong et al., 2014; Yang et al., 2018). The specificity of inhibitors against β-glucuronidase from E. coli was designed and exhibited activity in protecting mice from CPT11-induced toxicity, but did not kill bacteria or harm mammalian cells (Roberts et al., 2013; Pollet et al., 2017). Another E. coli β-glucuronidase-specific inhibitor, the pyrazolo[4,3-c]quinoline derivative TCH-3562, also exhibited inhibitory activity against endogenous β-glucuronidase from the anaerobes Eubacterium spp. and Peptostreptococcus anaerobius (Cheng et al., 2019). As a uniquely covalent inhibitor, UNC102016524 is combined with β-glucuronidase from Clostridium perfringens after conjugation at the active site of β-glucuronidase, and prevents intestinal toxicity while retaining the anti-tumor efficacy of CPT11 (Bhatt et al., 2020). Besides synthetic compounds, natural products derived from herbs and fruits (e.g., flavonoids and cinnamic-acid derivatives) exhibit β-glucuronidase inhibitory activity (Li et al., 2020; Sun et al., 2020; Yang et al., 2020). Nevertheless, almost all the discovered inhibitors based on β-glucuronidase have been designed to reduce the toxicity of CPT11 chemotherapy rather than enhance its efficacy.
Maintenance of a normal structure and diversity of a microbial community is crucial for continuous routine CPT11 chemotherapy and its curative effect. However, intervention measures to regulate the GM are suboptimal, such as dietary and probiotics interventions, which are not adequate for microbiota adjustment and are controversial.
Antibiotic treatment. Clinical guidelines state that antibiotics should be used for the management of CPT11 chemotherapy-induced diarrhea. As a broad-spectrum antibiotic, neomycin can relieve CPT11-induced diarrhea in patients by reduction in the activity of fecal β-glucuronidase (Sharma et al., 2005). A combination of neomycin and bacitracin also appeared to affect treatment efficacy in one small study (Alimonti et al., 2003). A phase-I clinical trial revealed that cefixime significantly decreased the dose-limiting toxicity induced by CPT11 treatment in pediatric patients with refractory solid tumors (Furman et al., 2006). Moreover, preventative ciprofloxacin treatment lowered CPT11 chemotherapy-related mortality in tumor-bearing rats (Xue et al., 2009). Nevertheless, the usefulness of antibiotics for the duration of CPT11 chemotherapy is controversial. Retrospective analyses conducted at Osaka National Hospital (Osaka, Japan) revealed no difference in the prevalence of grade-3–4 diarrhea between the co-administration group (clarithromycin and CPT11) and the CPT11-monotherapy group in patients with colorectal cancer (Makihara et al., 2017). Another retrospective study found that antibiotics did not improve the therapeutic efficacy of CPT11 in advanced colorectal cancer (Imai et al., 2020). Bacterial resistance is another serious issue. A literature review and modeling study revealed that in the USA, 26.8% of pathogens causing infections are resistant to standard prophylactic antibiotics after chemotherapy (Teillant et al., 2015). Use of antibiotics in immunocompromised patients may provoke a Clostridium difficile infection, thereby increasing the risk of diarrhea (Kim et al., 2013). Due to the diverse roles of the GM, non-selective killing of all bacteria by antibiotics elicits only limited benefits for CPT11 chemotherapy.
Dietary interventions. Dietary interventions can improve the efficacy of CPT11 chemotherapy, but their role in regulation of the GM during CPT11 chemotherapy is incompletely understood. However, evidence from animal studies is emerging. Mammals fed a high-fiber diet showed lighter symptoms of CPT11-induced mucositis, which is related to a decrease in abundance of Enterobacteriaceae, and increasing numbers of Lactobacillus spp. and Bifidobaterium spp., as well as increasing cecal production of butyrate (Lin et al., 2014; Gallotti et al., 2020). Other dietary interventions, including glutamine supplementation (Gaurav et al., 2012), supplementation with n-3 fatty acids (Hardman et al., 2002; Xue et al., 2007), protein/calorie restriction (de Man et al., 2020), fasting (Huisman et al., 2016), butyrate supplementation (Encarnacao et al., 2018), and ketogenic diets (Wang Y. et al., 2020), have also shown the usefulness for CPT11 chemotherapy (Table 1). These approaches have limited advantages in combination with concurrent CPT11 chemotherapy (and are deficient in comprehension of diet–microbiota–chemotherapy interactions in cancer). However, evidence from the effect of dietary components on cancer as well the GM studies have shown that dietary components affect the efficacy of cancer chemotherapy by targeting the GM (Kanarek et al., 2020; Tao et al., 2020). Therefore, the GM is likely conditioned by dietary interventions and, in turn, is a crucial regulator of the outcome of CPT11 chemotherapy.
Probiotics and bacterial strain-engineered interventions. Studies in animals and humans have suggested that probiotics based on Bifidobacterium spp., Lactobacillus spp., and Saccharomyces spp. can prevent intestinal mucositis and promote curative effects (Pico-Monllor and Mingot-Ascencao, 2019). VSL#3 comprises freeze-dried living bacteria: four strains of Lactobacilli spp., three strains of Bifidobacteria spp., and one strain of Streptococcus spp. VSL#3 can prevent CPT11-induced weight loss and diarrhea in rats (Bowen et al., 2007). Qiu et al. investigated the efficacy of selenium-enriched Bifidobacterium longum (Se-B. longum) on CPT11-induced intestinal mucositis. They found that Se-B. longum significantly reduced the prevalence of diarrhea in mice (Qiu et al., 2019). In preliminary clinical studies, Se-B. longum also presented positive outcomes if used as a probiotic. Mego et al. conducted a randomized double-blind, placebo-controlled pilot study and showed that oral probiotic supplements composed mainly of Bifidobacterium spp., Lactobacillus spp., and Streptococcus spp. decreased the prevalence and severity of intestinal toxicity induced by CPT11 (Mego et al., 2015). According to a recent prospective observational study, Lactobacillus kefiri LKF01 (Fefibios®) can relieve CPT11-induced severe diarrhea in cancer patients (Ghidini et al., 2021). Notably, a phase II/III, randomized, double blind, placebo controlled study conducted by Sharma et al. showed that a high-concentration multi-strain probiotic supplements (consist of 4 strains of Lactobacillus, 3 strains of Bifidobacteria and 1 strain of Streptococcus thermophilus) showed a relieved effect for grade 3 and grade 4 diarrhea induced by CPT11 (Sharma et al., 2018). All preclinical studies are summarized in Table 2.
Besides probiotics, several commensal bacteria, as well as genetically engineered bacteria and viruses, can also be used to alleviate intestinal toxicity or improve the efficacy of CPT11 chemotherapy. As a Gram-negative bacteria, E. coli Nissle 1917 relieves GM dysbiosis and promotes the intestinal barrier in CPT11-induced intestinal injury (Wang et al., 2019). In recent years, studies on oncolytic viruses in combination with chemotherapy have been undertaken (Zhang and Cheng, 2020). The consensus is that oncolytic viruses infect and lyse tumor cells, as well as activate innate and adaptive immunity through antigen presentation (Lawler et al., 2017). An in vitro study indicated that combination of ReoT3D (Dearing strain of oncolytic viruses), CPT11, and napabucasin (inhibitor of signal transducer and activator of transcription 3) induced the apoptosis of murine colorectal cancer cells (CT26) (Babaei et al., 2020). It has been reported that, as an intravenously delivered oncolytic reovirus, pelareorep can activate the immune response in the host. In a phase-Ib study, Mahalingam et al. revealed that pelareorep adjuvanted with CPT11 showed encouraging efficacy without additional toxicity in patients with an advanced pancreatic adenocarcinoma (Mahalingam et al., 2020). As a newly discovered bacterium, Fusobacterium nucleatum has been implicated in driving formation of a pro-tumoral microenvironment, as well as inducing chemoresistance and immunosuppression (Kostic et al., 2013; Mima et al., 2015). However, Zheng et al. revealed that F. nucleatum rescued the inhibitory effect of CPT11 on colorectal cancer cells (HCT116 and CT26) (Zheng et al., 2019). Nevertheless, the emergence of bioengineered phages could reduce the unfavorable effects of bacteria (Kannen et al., 2019). One study showed that azide-modified phages linked covalently to CPT11-loaded dextran nanoparticles could significantly inhibit F. nucleatum growth and strengthen the therapeutic effect of CPT11 against colorectal cancer (Zheng et al., 2019).
Germ-free mice have been shown to be more resistant to CPT11-induced intestinal toxicity than holoxenic mice (Brandi et al., 2006). Hence, researchers have demonstrated the benefits of applying antibiotics for prevention of the intestinal injury induced by CPT11. The GM has diverse roles in hosts. Antibiotics used for non-selective elimination of pro-tumoral and antineoplastic bacteria show only limited advantages during CPT11 chemotherapy in cancer patients. One study revealed that combination of metronidazole and ciprofloxacin increased the occurrence of breast cancer in proto-neu transgenic mice (Rossini et al., 2006). Besides, indiscriminate removal of gut microbes can reduce microbial diversity, increase the infection risk, and induce antibiotic resistance (Murphy et al., 2019). Prolonged use of antibiotics in cancer patients treated with CPT11 may cause a particularly stubborn C. difficile infection (Principi et al., 2020). Moreover, a recurrent C. difficile infection may induce severe diarrhea and cause electrolyte imbalances, toxic megacolon, shock, and even death, and thereby lead to the failure of CPT11 chemotherapy (Abu-Sbeih et al., 2019; Aziz et al., 2019).
Application of dietary and probiotics interventions seems to open new perspectives for a better pharmacological action of CPT11. CPT11 metabolism involves multiple activation and deactivation pathways. Most notably, inhibition of β-glucuronidase blunts the stark shifts in GM composition induced by CPT11 (Bhatt et al., 2020). Nevertheless, an alternative strategy to reduce CPT11-related intestinal toxicity that, by contrast, requires active biotransformation via bacterial β-glucuronidase to exert its protective effect on the intestinal epithelium, is provided by the Chinese herbal formulation PHY906. This is achieved through regeneration of intestinal stem cells and potentiation of Wnt signaling (Lam et al., 2010). Besides, various herbs have been used as adjuvants in animal and clinical studies for assisting CPT11 chemotherapy through other mechanisms, and are summarized in Table 3.
The biological complexity of various signaling pathways in the GM is an obstacle for understanding how host–microbial reciprocal actions meditate the outcomes of CPT11 chemotherapy. However, several emerging interventions have been studied in the preclinical setting to assist in CPT11 chemotherapy for cancer patients. Microbial diversity impacts upon cancer chemotherapy. Hence, the research focus should shift from a “one-size-fits-all” antibacterial pattern to maintenance of a diverse microbiota. Studies on how GM components act together to mediate the metabolism, bioavailability, efficacy, and toxicity of CPT11 will be of enormous value. GM manipulation by dietary, herbal, probiotic, or bacterial strain-engineered strains is likely to become an integral part of cancer treatment regimens.
Both BY and RG equally contributed to writing the manuscript. ZW and WD revised the manuscript and provided critical input. All listed authors participated in critical discussions and have approved the final version of the manuscript.
This work was supported by grants from the National Natural Science Foundation of China (81920108033, 81530096) and Natural Science Foundation of Shanghai (20ZR1458000).
The authors declare that the research was conducted in the absence of any commercial or financial relationships that could be construed as a potential conflict of interest.
All claims expressed in this article are solely those of the authors and do not necessarily represent those of their affiliated organizations, or those of the publisher, the editors and the reviewers. Any product that may be evaluated in this article, or claim that may be made by its manufacturer, is not guaranteed or endorsed by the publisher.
Abu-Sbeih, H., Choi, K., Tran, C. N., Wang, X., Lum, P., Shuttlesworth, G., et al. (2019). Recurrent Clostridium Difficile Infection is Associated With Treatment Failure and Prolonged Illness in Cancer Patients. Eur. J. Gastroenterol. Hepatol. 31, 128–134. doi: 10.1097/MEG.0000000000001288
Adak, A., Khan, M. R. (2019). An Insight Into Gut Microbiota and its Functionalities. Cell. Mol. Life Sci. 76, 473–493. doi: 10.1007/s00018-018-2943-4
Ahmad, S., Hughes, M. A., Yeh, L. A., Scott, J. E. (2012). Potential Repurposing of Known Drugs as Potent Bacterial Beta-Glucuronidase Inhibitors. J. Biomol. Screen 17, 957–965. doi: 10.1177/1087057112444927
Alexander, J. L., Wilson, I. D., Teare, J., Marchesi, J. R., Nicholson, J. K., Kinross, J. M. (2017). Gut Microbiota Modulation of Chemotherapy Efficacy and Toxicity. Nat. Rev. Gastroenterol. Hepatol. 14, 356–365. doi: 10.1038/nrgastro.2017.20
Alimonti, A., Gelibter, A., Pavese, I., Satta, F., Cognetti, F., Ferretti, G., et al. (2004). New Approaches to Prevent Intestinal Toxicity of Irinotecan-Based Regimens. Cancer Treat. Rev. 30, 555–562. doi: 10.1016/j.ctrv.2004.05.002
Alimonti, A., Satta, F., Pavese, I., Burattini, E., Zoffoli, V., Vecchione, A. (2003). Prevention of Irinotecan Plus 5-Fluorouracil/Leucovorin-Induced Diarrhoea by Oral Administration of Neomycin Plus Bacitracin in First-Line Treatment of Advanced Colorectal Cancer. Ann. Oncol. 14, 805–806. doi: 10.1093/annonc/mdg193
Almasud, A. A., Giles, K. H., Miklavcic, J. J., Martins, K., Baracos, V. E., Putman, C. T., et al. (2017). Fish Oil Mitigates Myosteatosis and Improves Chemotherapy Efficacy in a Preclinical Model of Colon Cancer. PloS One 12, e183576. doi: 10.1371/journal.pone.0183576
Aziz, M., Fatima, R., Douglass, L. N., Abughanimeh, O., Raza, S. (2019). Current Updates in Management of Clostridium Difficile Infection in Cancer Patients. Curr. Med. Res. Opin. 35, 473–478. doi: 10.1080/03007995.2018.1487389
Babaei, A., Soleimanjahi, H., Soleimani, M., Arefian, E. (2020). The Synergistic Anticancer Effects of ReoT3D, CPT-11, and BBI608 on Murine Colorectal Cancer Cells. Daru 28, 555–565. doi: 10.1007/s40199-020-00361-w
Bailly, C. (2019). Irinotecan: 25 Years of Cancer Treatment. Pharmacol. Res. 148, 104398. doi: 10.1016/j.phrs.2019.104398
Baird, D. C., Meyers, G. J., Hu, J. S. (2018). Testicular Cancer: Diagnosis and Treatment. Am. Fam. Physician 97, 261–268.
Bastos, R. W., Pedroso, S. H., Vieira, A. T., Moreira, L. M., Franca, C. S., Cartelle, C. T., et al. (2016). Saccharomyces Cerevisiae UFMG A-905 Treatment Reduces Intestinal Damage in a Murine Model of Irinotecan-Induced Mucositis. Benef. Microbes 7, 549–557. doi: 10.3920/BM2015.0190
Berman, A., Chisholm, L., de Carvalho, M., Piemme, J. A., Gorrell, C. R. (1993). Cancer Chemotherapy: Intravenous Administration. Cancer Nurs. 16, 145–158. doi: 10.1097/00002820-199304000-00010
Bhatt, A. P., Pellock, S. J., Biernat, K. A., Walton, W. G., Wallace, B. D., Creekmore, B. C., et al. (2020). Targeted Inhibition of Gut Bacterial Beta-Glucuronidase Activity Enhances Anticancer Drug Efficacy. Proc. Natl. Acad. Sci. U. S. A. 117, 7374–7381. doi: 10.1073/pnas.1918095117
Bhatt, A. P., Redinbo, M. R., Bultman, S. J. (2017). The Role of the Microbiome in Cancer Development and Therapy. CA Cancer J. Clin. 67, 326–344. doi: 10.3322/caac.21398
Bottacini, F., van Sinderen, D., Ventura, M. (2017). Omics of Bifidobacteria: Research and Insights Into Their Health-Promoting Activities. Biochem. J. 474, 4137–4152. doi: 10.1042/BCJ20160756
Bowen, J. M., Stringer, A. M., Gibson, R. J., Yeoh, A. S., Hannam, S., Keefe, D. (2007). VSL3 Probiotic Treatment Reduces Chemotherapy-Induced Diarrhea and Weight Loss. M Cancer Biol. Ther. 6, 1449–1454. doi: 10.4161/cbt.6.9.4622
Brandi, G., Dabard, J., Raibaud, P., Di Battista, M., Bridonneau, C., Pisi, A. M., et al. (2006). Intestinal Microflora and Digestive Toxicity of Irinotecan in Mice. Clin. Cancer Res. 12, 1299–1307. doi: 10.1158/1078-0432.CCR-05-0750
Bruera, G., Ricevuto, E. (2020). Pharmacogenomic Assessment of Patients With Colorectal Cancer and Potential Treatments. Pharmgenom. Pers. Med. 13, 601–617. doi: 10.2147/PGPM.S253586
Burris, H. R., Fields, S. M. (1994). Topoisomerase I Inhibitors. An Overview of the Camptothecin Analogs. Hematol. Oncol. Clin. North Am. 8, 333–355. doi: 10.1016/S0889-8588(18)30176-X
Campbell, J. M., Stephenson, M. D., Bateman, E., Peters, M. D., Keefe, D. M., Bowen, J. M. (2017). Irinotecan-Induced Toxicity Pharmacogenetics: An Umbrella Review of Systematic Reviews and Meta-Analyses. Pharmacogenom. J. 17, 21–28. doi: 10.1038/tpj.2016.58
Cani, P. D., Jordan, B. F. (2018). Gut Microbiota-Mediated Inflammation in Obesity: A Link With Gastrointestinal Cancer. Nat. Rev. Gastroenterol. Hepatol. 15, 671–682. doi: 10.1038/s41575-018-0025-6
Chamseddine, A. N., Ducreux, M., Armand, J. P., Paoletti, X., Satar, T., Paci, A., et al. (2019). Intestinal Bacterial Beta-Glucuronidase as a Possible Predictive Biomarker of Irinotecan-Induced Diarrhea Severity. Pharmacol. Ther. 199, 1–15. doi: 10.1016/j.pharmthera.2019.03.002
Chan, Y. T., Cheung, F., Zhang, C., Fu, B., Tan, H. Y., Norimoto, H., et al. (2020). Ancient Chinese Medicine Herbal Formula Huanglian Jiedu Decoction as a Neoadjuvant Treatment of Chemotherapy by Improving Diarrhea and Tumor Response. Front. Pharmacol. 11, 252. doi: 10.3389/fphar.2020.00252
Chen, Z., Jiang, L. (2019). The Clinical Application of Fruquintinib on Colorectal Cancer. Expert Rev. Clin. Pharmacol. 12, 713–721. doi: 10.1080/17512433.2019.1630272
Chen, Z., Xu, X., Piao, L., Chang, S., Liu, J., Kong, R. (2020). Identify Old Drugs as Selective Bacterial Beta-GUS Inhibitors by Structural-Based Virtual Screening and Bio-Evaluations. Chem. Biol. Drug Des. 95, 368–379. doi: 10.1111/cbdd.13655
Cheng, K. W., Tseng, C. H., Tzeng, C. C., Leu, Y. L., Cheng, T. C., Wang, J. Y., et al. (2019). Pharmacological Inhibition of Bacterial Beta-Glucuronidase Prevents Irinotecan-Induced Diarrhea Without Impairing its Antitumor Efficacy In Vivo. Pharmacol. Res. 139, 41–49. doi: 10.1016/j.phrs.2018.10.029
Creekmore, B. C., Gray, J. H., Walton, W. G., Biernat, K. A., Little, M. S., Xu, Y., et al. (2019). Mouse Gut Microbiome-Encoded Beta-Glucuronidases Identified Using Metagenome Analysis Guided by Protein Structure. mSystems 4, e00452-19. doi: 10.1128/mSystems.00452-19
de Man, F. M., Goey, A., van Schaik, R., Mathijssen, R., Bins, S. (2018). Individualization of Irinotecan Treatment: A Review of Pharmacokinetics, Pharmacodynamics, and Pharmacogenetics. Clin. Pharmacokinet. 57, 1229–1254. doi: 10.1007/s40262-018-0644-7
de Man, F. M., van Eerden, R., van Doorn, G. M., Oomen-de, H. E., Koolen, S., Olieman, J. F., et al. (2020). Effects of Protein and Calorie Restriction on the Metabolism and Toxicity Profile of Irinotecan in Cancer Patients. Clin. Pharmacol. Ther. 109, 1304–1313. doi: 10.1002/cpt.2094
Di Desidero, T., Antonelli, A., Orlandi, P., Ferrari, S. M., Fioravanti, A., Ali, G., et al. (2017). Synergistic Efficacy of Irinotecan and Sunitinib Combination in Preclinical Models of Anaplastic Thyroid Cancer. Cancer Lett. 411, 35–43. doi: 10.1016/j.canlet.2017.09.032
Di, W., Zhang, L., Yi, H., Han, X., Zhang, Y., Xin, L. (2018). Exopolysaccharides Produced by Lactobacillus Strains Suppress HT-29 Cell Growth via Induction of G0/G1 Cell Cycle Arrest and Apoptosis. Oncol. Lett. 16, 3577–3586. doi: 10.3892/ol.2018.9129
Dohlman, A. B., Arguijo, M. D., Ding, S., Gao, M., Dressman, H., Iliev, I. D., et al. (2021). The Cancer Microbiome Atlas: A Pan-Cancer Comparative Analysis to Distinguish Tissue-Resident Microbiota From Contaminants. Cell Host Microbe 29, 281–298. doi: 10.1016/j.chom.2020.12.001
Duffy, A. G., Greten, T. F. (2014). Immunological Off-Target Effects of Standard Treatments in Gastrointestinal Cancers. Ann. Oncol. 25, 24–32. doi: 10.1093/annonc/mdt349
Encarnacao, J. C., Pires, A. S., Amaral, R. A., Goncalves, T. J., Laranjo, M., Casalta-Lopes, J. E., et al. (2018). Butyrate, a Dietary Fiber Derivative That Improves Irinotecan Effect in Colon Cancer Cells. J. Nutr. Biochem. 56, 183–192. doi: 10.1016/j.jnutbio.2018.02.018
Eng, C. (2009). Toxic Effects and Their Management: Daily Clinical Challenges in the Treatment of Colorectal Cancer. Nat. Rev. Clin. Oncol. 6, 207–218. doi: 10.1038/nrclinonc.2009.16
Ervin, S. M., Ramanan, S. V., Bhatt, A. P. (2020). Relationship Between the Gut Microbiome and Systemic Chemotherapy. Dig Dis. Sci. 65, 874–884. doi: 10.1007/s10620-020-06119-3
Fujimura, K. E., Slusher, N. A., Cabana, M. D., Lynch, S. V. (2010). Role of the Gut Microbiota in Defining Human Health. Expert Rev. Anti Infect. Ther. 8, 435–454. doi: 10.1586/eri.10.14
Fukuchi, M., Kuwabara, K., Ishiguro, T., Kumagai, Y., Ishibashi, K., Mochiki, E., et al. (2020). Efficacy of Irinotecan as Third-Line Chemotherapy for Unresectable or Recurrent Gastric Cancer. In Vivo 34, 903–908. doi: 10.21873/invivo.11856
Furman, W. L., Crews, K. R., Billups, C., Wu, J., Gajjar, A. J., Daw, N. C., et al. (2006). Cefixime Allows Greater Dose Escalation of Oral Irinotecan: A Phase I Study in Pediatric Patients With Refractory Solid Tumors. J. Clin. Oncol. 24, 563–570. doi: 10.1200/JCO.2005.03.2847
Furue, H. (2003). Chemotherapy Cancer Treatment During the Past Sixty Years. Gan To Kagaku Ryoho 30, 1404–1411.
Gallotti, B., Galvao, I., Leles, G., Quintanilha, M. F., Souza, R. O., Miranda, V. C., et al. (2020). Effects of Dietary Fibre Intake in Chemotherapy-Induced Mucositis in Murine Model. Br. J. Nutr. 126, 853–864. doi: 10.1017/S0007114520004924
Garcia, G. S., Ramos, D. R., Nazco, C. G., Llanos, M. M., Vina, R. M., Martin, C. B., et al. (2018). Effect of UGT, SLCO, ABCB and ABCC Polymorphisms on Irinotecan Toxicity. Med. Clin. (Barc). 151, 425–430. doi: 10.1016/j.medcli.2018.01.016
Gaurav, K., Goel, R. K., Shukla, M., Pandey, M. (2012). Glutamine: A Novel Approach to Chemotherapy-Induced Toxicity. Indian J. Med. Paediatr. Oncol. 33, 13–20. doi: 10.4103/0971-5851.96962
Gelibter, A. J., Caponnetto, S., Urbano, F., Emiliani, A., Scagnoli, S., Sirgiovanni, G., et al. (2019). Adjuvant Chemotherapy in Resected Colon Cancer: When, How and How Long? Surg. Oncol. 30, 100–107. doi: 10.1016/j.suronc.2019.06.003
Gentry, A. C., Juul, S., Veigaard, C., Knudsen, B. R., Osheroff, N. (2011). The Geometry of DNA Supercoils Modulates the DNA Cleavage Activity of Human Topoisomerase I. Nucleic Acids Res. 39, 1014–1022. doi: 10.1093/nar/gkq822
Ghidini, M., Nicoletti, M., Ratti, M., Tomasello, G., Lonati, V., Ghilardi, M., et al. (2021). Lactobacillus Kefiri LKF01 (Kefibios((R))) for Prevention of Diarrhoea in Cancer Patients Treated With Chemotherapy: A Prospective Study. Nutrients 13, 385. doi: 10.3390/nu13020385
Glassman, D. C., Palmaira, R. L., Covington, C. M., Desai, A. M., Ku, G. Y., Li, J., et al. (2018). Nanoliposomal Irinotecan With Fluorouracil for the Treatment of Advanced Pancreatic Cancer, a Single Institution Experience. BMC Cancer. 18, 693. doi: 10.1186/s12885-018-4605-1
Goh, Y. J., Barrangou, R., Klaenhammer, T. R. (2021). In Vivo Transcriptome of Lactobacillus Acidophilus and Colonization Impact on Murine Host Intestinal Gene Expression. mBio 12, e03399–20. doi: 10.1128/mBio.03399-20
Griffiths, E. A., Duffy, L. C., Schanbacher, F. L., Qiao, H., Dryja, D., Leavens, A., et al. (2004). In Vivo Effects of Bifidobacteria and Lactoferrin on Gut Endotoxin Concentration and Mucosal Immunity in Balb/c Mice. Dig Dis. Sci. 49, 579–589. doi: 10.1023/b:ddas.0000026302.92898.ae
Guan, H. Y., Li, P. F., Wang, X. M., Yue, J. J., He, Y., Luo, X. M., et al. (2017). Shengjiang Xiexin Decoction Alters Pharmacokinetics of Irinotecan by Regulating Metabolic Enzymes and Transporters: A Multi-Target Therapy for Alleviating the Gastrointestinal Toxicity. Front. Pharmacol. 8, 769. doi: 10.3389/fphar.2017.00769
Hardman, W. E., Moyer, M. P., Cameron, I. L. (2002). Consumption of an Omega-3 Fatty Acids Product, INCELL AAFA, Reduced Side-Effects Of CPT-11 (Irinotecan) in Mice. Br. J. Cancer. 86, 983–988. doi: 10.1038/sj.bjc.6600175
Hofseth, L. J., Hebert, J. R., Chanda, A., Chen, H., Love, B. L., Pena, M. M., et al. (2020). Early-Onset Colorectal Cancer: Initial Clues and Current Views. Nat. Rev. Gastroenterol. Hepatol. 17, 352–364. doi: 10.1038/s41575-019-0253-4
Hueso, T., Ekpe, K., Mayeur, C., Gatse, A., Joncquel-Chevallier, C. M., Gricourt, G., et al. (2020). Impact and Consequences of Intensive Chemotherapy on Intestinal Barrier and Microbiota in Acute Myeloid Leukemia: The Role of Mucosal Strengthening. Gut Microbes 12, 1800897. doi: 10.1080/19490976.2020.1800897
Huisman, S. A., de Bruijn, P., Ghobadi, M. I., IJzermans, J. N., Wiemer, E. A., Mathijssen, R. H., et al. (2016). Fasting Protects Against the Side Effects of Irinotecan Treatment But Does Not Affect Anti-Tumour Activity in Mice. Br. J. Pharmacol. 173, 804–814. doi: 10.1111/bph.13317
Hulshof, E. C., Deenen, M. J., Guchelaar, H. J., Gelderblom, H. (2020). Pre-Therapeutic UGT1A1 Genotyping to Reduce the Risk of Irinotecan-Induced Severe Toxicity: Ready for Prime Time. Eur. J. Cancer. 141, 9–20. doi: 10.1016/j.ejca.2020.09.007
Hyatt, J. L., Tsurkan, L., Morton, C. L., Yoon, K. J., Harel, M., Brumshtein, B., et al. (2005). Inhibition of Acetylcholinesterase by the Anticancer Prodrug CPT-11. Chem. Biol. Interact. 157–158, 247–252. doi: 10.1016/j.cbi.2005.10.033
Iida, N., Dzutsev, A., Stewart, C. A., Smith, L., Bouladoux, N., Weingarten, R. A., et al. (2013). Commensal Bacteria Control Cancer Response to Therapy by Modulating the Tumor Microenvironment. Science 342, 967–970. doi: 10.1126/science.1240527
Imai, H., Saijo, K., Komine, K., Yoshida, Y., Sasaki, K., Suzuki, A., et al. (2020). Antibiotics Improve the Treatment Efficacy of Oxaliplatin-Based But Not Irinotecan-Based Therapy in Advanced Colorectal Cancer Patients. J. Oncol. 2020, 1701326. doi: 10.1155/2020/1701326
Jariwala, P. B., Pellock, S. J., Goldfarb, D., Cloer, E. W., Artola, M., Simpson, J. B., et al. (2020). Discovering the Microbial Enzymes Driving Drug Toxicity With Activity-Based Protein Profiling. ACS Chem. Biol. 15, 217–225. doi: 10.1021/acschembio.9b00788
Kanarek, N., Petrova, B., Sabatini, D. M. (2020). Dietary Modifications for Enhanced Cancer Therapy. Nature 579, 507–517. doi: 10.1038/s41586-020-2124-0
Kannen, V., Parry, L., Martin, F. L. (2019). Phages Enter the Fight Against Colorectal Cancer. Trends Cancer. 5, 577–579. doi: 10.1016/j.trecan.2019.08.002
Kciuk, M., Marciniak, B., Kontek, R. (2020). Irinotecan-Still an Important Player in Cancer Chemotherapy: A Comprehensive Overview. Int. J. Mol. Sci. 21, 4919. doi: 10.3390/ijms21144919
Kim, D. W., Chu, H., Joo, D. H., Jang, M. S., Choi, J. H., Park, S. M., et al. (2008). OspF Directly Attenuates the Activity of Extracellular Signal-Regulated Kinase During Invasion by Shigella Flexneri in Human Dendritic Cells. Mol. Immunol. 45, 3295–3301. doi: 10.1016/j.molimm.2008.02.013
Kim, J., Kim, H. Y., Hong, S., Shin, S., Kim, Y. A., Kim, N. S., et al. (2019). A New Herbal Formula BP10A Exerted an Antitumor Effect and Enhanced Anticancer Effect of Irinotecan and Oxaliplatin in the Colon Cancer PDTX Model. Biomed. Pharmacother. 116, 108987. doi: 10.1016/j.biopha.2019.108987
Kim, J. S., Ward, K. K., Shah, N. R., Saenz, C. C., McHale, M. T., Plaxe, S. C. (2013). Excess Risk of Clostridium Difficile Infection in Ovarian Cancer is Related to Exposure to Broad-Spectrum Antibiotics. Support. Care Cancer. 21, 3103–3107. doi: 10.1007/s00520-013-1888-2
Kong, R., Liu, T., Zhu, X., Ahmad, S., Williams, A. L., Phan, A. T., et al. (2014). Old Drug New Use–Amoxapine and its Metabolites as Potent Bacterial Beta-Glucuronidase Inhibitors for Alleviating Cancer Drug Toxicity. Clin. Cancer Res. 20, 3521–3530. doi: 10.1158/1078-0432.CCR-14-0395
Konradt, C., Frigimelica, E., Nothelfer, K., Puhar, A., Salgado-Pabon, W., di Bartolo, V., et al. (2011). The Shigella Flexneri Type Three Secretion System Effector IpgD Inhibits T Cell Migration by Manipulating Host Phosphoinositide Metabolism. Cell Host Microbe 9, 263–272. doi: 10.1016/j.chom.2011.03.010
Koppel, N., Maini, R. V., Balskus, E. P. (2017). Chemical Transformation of Xenobiotics by the Human Gut Microbiota. Science 356, eaag2770. doi: 10.1126/science.aag2770
Kostic, A. D., Chun, E., Robertson, L., Glickman, J. N., Gallini, C. A., Michaud, M., et al. (2013). Fusobacterium Nucleatum Potentiates Intestinal Tumorigenesis and Modulates the Tumor-Immune Microenvironment. Cell Host Microbe 14, 207–215. doi: 10.1016/j.chom.2013.07.007
Kuhn, J. G. (1998). Pharmacology of Irinotecan. Oncol. (Williston Park) 12, 39–42. doi: 10.1358/dot.1998.34.9.485276
Kurita, A., Kado, S., Matsumoto, T., Asakawa, N., Kaneda, N., Kato, I., et al. (2011). Streptomycin Alleviates Irinotecan-Induced Delayed-Onset Diarrhea in Rats by a Mechanism Other Than Inhibition of Beta-Glucuronidase Activity in Intestinal Lumen. Cancer Chemother. Pharmacol. 67, 201–213. doi: 10.1007/s00280-010-1310-4
Lam, W., Bussom, S., Guan, F., Jiang, Z., Zhang, W., Gullen, E. A., et al. (2010). The Four-Herb Chinese Medicine PHY906 Reduces Chemotherapy-Induced Gastrointestinal Toxicity. Sci. Transl. Med. 2, 45r–59r. doi: 10.1126/scitranslmed.3001270
Lam, W., Jiang, Z., Guan, F., Hu, R., Liu, S. H., Chu, E., et al. (2014). The Number of Intestinal Bacteria is Not Critical for the Enhancement of Antitumor Activity and Reduction of Intestinal Toxicity of Irinotecan by the Chinese Herbal Medicine PHY906 (Kd018). BMC Complement Altern. Med. 14, 490. doi: 10.1186/1472-6882-14-490
Lang, M., Baumgartner, M., Rozalska, A., Frick, A., Riva, A., Jarek, M., et al. (2020). Crypt Residing Bacteria and Proximal Colonic Carcinogenesis in a Mouse Model of Lynch Syndrome. Int. J. Cancer. 147, 2316–2326. doi: 10.1002/ijc.33028
Lawler, S. E., Speranza, M. C., Cho, C. F., Chiocca, E. A. (2017). Oncolytic Viruses in Cancer Treatment: A Review. JAMA Oncol. 3, 841–849. doi: 10.1001/jamaoncol.2016.2064
LeBlanc, J. G., Chain, F., Martin, R., Bermudez-Humaran, L. G., Courau, S., Langella, P. (2017). Beneficial Effects on Host Energy Metabolism of Short-Chain Fatty Acids and Vitamins Produced by Commensal and Probiotic Bacteria. Microb. Cell Fact. 16, 79. doi: 10.1186/s12934-017-0691-z
Lian, Q., Xu, J., Yan, S., Huang, M., Ding, H., Sun, X., et al. (2017). Chemotherapy-Induced Intestinal Inflammatory Responses are Mediated by Exosome Secretion of Double-Strand DNA via AIM2 Inflammasome Activation. Cell Res. 27, 784–800. doi: 10.1038/cr.2017.54
Li, X. N., Hua, L. X., Zhou, T. S., Wang, K. B., Wu, Y. Y., Emam, M., et al. (2020). Cinnamic Acid Derivatives: Inhibitory Activity Against Escherichia Coli Beta-Glucuronidase and Structure-Activity Relationships. J. Enzyme Inhib. Med. Chem. 35, 1372–1378. doi: 10.1080/14756366.2020.1780225
Li, F., Jiang, T., Li, Q., Ling, X. (2017). Camptothecin (CPT) and its Derivatives are Known to Target Topoisomerase I (Top1) as Their Mechanism of Action: Did We Miss Something in CPT Analogue Molecular Targets for Treating Human Disease Such as Cancer? Am. J. Cancer Res. 7, 2350–2394.
Lin, X. B., Dieleman, L. A., Ketabi, A., Bibova, I., Sawyer, M. B., Xue, H., et al. (2012). Irinotecan (CPT-11) Chemotherapy Alters Intestinal Microbiota in Tumour Bearing Rats. PloS One 7, e39764. doi: 10.1371/journal.pone.0039764
Lin, X. B., Farhangfar, A., Valcheva, R., Sawyer, M. B., Dieleman, L., Schieber, A., et al. (2014). The Role of Intestinal Microbiota in Development of Irinotecan Toxicity and in Toxicity Reduction Through Dietary Fibres in Rats. PloS One 9, e83644. doi: 10.1371/journal.pone.0083644
Liu, Q., Hua, S., Wang, X., Chen, F., Gou, S. (2021). The Introduction of Immunosuppressor (TDO Inhibitor) Significantly Improved the Efficacy of Irinotecan in Treating Hepatocellular Carcinoma. Cancer Immunol. Immunother. 70, 497–508. doi: 10.1007/s00262-020-02697-3
Logan, R. M., Stringer, A. M., Bowen, J. M., Yeoh, A. S., Gibson, R. J., Sonis, S. T., et al. (2007). The Role of Pro-Inflammatory Cytokines in Cancer Treatment-Induced Alimentary Tract Mucositis: Pathobiology, Animal Models and Cytotoxic Drugs. Cancer Treat. Rev. 33, 448–460. doi: 10.1016/j.ctrv.2007.03.001
Lopez, P., Gonzalez-Rodriguez, I., Sanchez, B., Gueimonde, M., Margolles, A., Suarez, A. (2012). Treg-Inducing Membrane Vesicles From Bifidobacterium Bifidum LMG13195 as Potential Adjuvants in Immunotherapy. Vaccine 30, 825–829. doi: 10.1016/j.vaccine.2011.11.115
Lu, H., Qin, J., Han, N., Xie, F., Gong, L., Li, C. (2018). Banxia Xiexin Decoction is Effective to Prevent and Control Irinotecan-Induced Delayed Diarrhea in Recurrent Small Cell Lung Cancer. Integr. Cancer Ther. 17, 1109–1114. doi: 10.1177/1534735418801532
Ma, J., Zhu, W., Liu, B. (2021). Role of Gut Microbiome in the Outcome of Cancer Immunotherapy. Int. J. Cancer 149, 760–768. doi: 10.1002/ijc.33524
Mabuchi, S., Yokoi, E., Shimura, K., Komura, N., Matsumoto, Y., Sawada, K., et al. (2019). A Phase II Study of Irinotecan Combined With S-1 in Patients With Advanced or Recurrent Cervical Cancer Previously Treated With Platinum Based Chemotherapy. Int. J. Gynecol. Cancer. 29, 474–479. doi: 10.1136/ijgc-2018-000070
Mahalingam, D., Wilkinson, G. A., Eng, K. H., Fields, P., Raber, P., Moseley, J. L., et al. (2020). Pembrolizumab in Combination With the Oncolytic Virus Pelareorep and Chemotherapy in Patients With Advanced Pancreatic Adenocarcinoma: A Phase Ib Study. Clin. Cancer Res. 26, 71–81. doi: 10.1158/1078-0432.CCR-19-2078
Makihara, K., Nakamura, S., Miyagi, K., Ueno, H., Nakata, I. (2017). Clarithromycin Co-Administration Does Not Increase Irinotecan (CPT-11) Toxicity in Colorectal Cancer Patients. Cancer Chemother. Pharmacol. 80, 527–533. doi: 10.1007/s00280-017-3388-4
Markowiak-Kopec, P., Slizewska, K. (2020). The Effect of Probiotics on the Production of Short-Chain Fatty Acids by Human Intestinal Microbiome. Nutrients 12, 1107. doi: 10.3390/nu12041107
Mego, M., Chovanec, J., Vochyanova-Andrezalova, I., Konkolovsky, P., Mikulova, M., Reckova, M., et al. (2015). Prevention of Irinotecan Induced Diarrhea by Probiotics: A Randomized Double Blind, Placebo Controlled Pilot Study. Complement. Ther. Med. 23, 356–362. doi: 10.1016/j.ctim.2015.03.008
Meric-Bernstam, F., Larkin, J., Tabernero, J., Bonini, C. (2020). Enhancing Anti-Tumour Efficacy With Immunotherapy Combinations. Lancet. 397, 1010–1022. doi: 10.1016/S0140-6736(20)32598-8
Mima, K., Sukawa, Y., Nishihara, R., Qian, Z. R., Yamauchi, M., Inamura, K., et al. (2015). Fusobacterium Nucleatum and T Cells in Colorectal Carcinoma. JAMA Oncol. 1, 653–661. doi: 10.1001/jamaoncol.2015.1377
Mizrahi, J. D., Gunchick, V., Mody, K., Xiao, L., Surapaneni, P., Shroff, R. T., et al. (2020). Multi-Institutional Retrospective Analysis of FOLFIRI in Patients With Advanced Biliary Tract Cancers. World J. Gastrointest Oncol. 12, 83–91. doi: 10.4251/wjgo.v12.i1.83
Mody, R., Naranjo, A., Van Ryn, C., Yu, A. L., London, W. B., Shulkin, B. L., et al. (2017). Irinotecan-Temozolomide With Temsirolimus or Dinutuximab in Children With Refractory or Relapsed Neuroblastoma (COG ANBL1221): An Open-Label, Randomised, Phase 2 Trial. Lancet Oncol. 18, 946–957. doi: 10.1016/S1470-2045(17)30355-8
Morkunas, E., Skieceviciene, J., Kupcinskas, J. (2020). The Impact of Modulating the Gastrointestinal Microbiota in Cancer Patients. Best Pract. Res. Clin. Gastroenterol. 48–49, 101700. doi: 10.1016/j.bpg.2020.101700
Morton, C. L., Wadkins, R. M., Danks, M. K., Potter, P. M. (1999). The Anticancer Prodrug CPT-11 Is a Potent Inhibitor of Acetylcholinesterase But is Rapidly Catalyzed to SN-38 by Butyrylcholinesterase. Cancer Res. 59, 1458–1463.
Mukaida, N. (2014). Intestinal Microbiota: Unexpected Alliance With Tumor Therapy. Immunotherapy-UK 6, 231–233. doi: 10.2217/imt.13.170
Murphy, C. L., O’Toole, P. W., Shanahan, F. (2019). The Gut Microbiota in Causation, Detection, and Treatment of Cancer. Am. J. Gastroenterol. 114, 1036–1042. doi: 10.14309/ajg.0000000000000075
Nishiike, S., Okazaki, S., Watanabe, H., Akizuki, H., Imai, T., Uno, A., et al. (2013). The Effect of Visual-Vestibulosomatosensory Conflict Induced by Virtual Reality on Postural Stability in Humans. J. Med. Invest 60, 236–239. doi: 10.2152/jmi.60.236
Nowak, A., Paliwoda, A., Blasiak, J. (2019). Anti-Proliferative, Pro-Apoptotic and Anti-Oxidative Activity of Lactobacillus and Bifidobacterium Strains: A Review of Mechanisms and Therapeutic Perspectives. Crit. Rev. Food Sci. Nutr. 59, 3456–3467. doi: 10.1080/10408398.2018.1494539
Okuda, J., Toyotome, T., Kataoka, N., Ohno, M., Abe, H., Shimura, Y., et al. (2005). Shigella Effector IpaH9.8 Binds to a Splicing Factor U2AF(35) to Modulate Host Immune Responses. Biochem. Biophys. Res. Commun. 333, 531–539. doi: 10.1016/j.bbrc.2005.05.145
Palomaki, G. E., Bradley, L. A., Douglas, M. P., Kolor, K., Dotson, W. D. (2009). Can UGT1A1 Genotyping Reduce Morbidity and Mortality in Patients With Metastatic Colorectal Cancer Treated With Irinotecan? An Evidence-Based Review. Genet. Med. 11, 21–34. doi: 10.1097/GIM.0b013e31818efd77
Paulik, A., Nekvindova, J., Filip, S. (2020). Irinotecan Toxicity During Treatment of Metastatic Colorectal Cancer: Focus on Pharmacogenomics and Personalized Medicine. Tumori 106, 87–94. doi: 10.1177/0300891618811283
Pedroso, S., Vieira, A. T., Bastos, R. W., Oliveira, J. S., Cartelle, C. T., Arantes, R., et al. (2015). Evaluation of Mucositis Induced by Irinotecan After Microbial Colonization in Germ-Free Mice. Microbiol. (Reading) 161, 1950–1960. doi: 10.1099/mic.0.000149
Picard, C., Fioramonti, J., Francois, A., Robinson, T., Neant, F., Matuchansky, C. (2005). Review Article: Bifidobacteria as Probiotic Agents – Physiological Effects and Clinical Benefits. Aliment Pharmacol. Ther. 22, 495–512. doi: 10.1111/j.1365-2036.2005.02615.x
Pico-Monllor, J. A., Mingot-Ascencao, J. M. (2019). Search and Selection of Probiotics That Improve Mucositis Symptoms in Oncologic Patients. A Systematic Review. Nutrients 11, 2322. doi: 10.3390/nu11102322
Pollet, R. M., D’Agostino, E. H., Walton, W. G., Xu, Y., Little, M. S., Biernat, K. A., et al. (2017). An Atlas of Beta-Glucuronidases in the Human Intestinal Microbiome. Structure 25, 967–977. doi: 10.1016/j.str.2017.05.003
Pommier, Y. (2006). Topoisomerase I Inhibitors: Camptothecins and Beyond. Nat. Rev. Cancer. 6, 789–802. doi: 10.1038/nrc1977
Principi, N., Gnocchi, M., Gagliardi, M., Argentiero, A., Neglia, C., Esposito, S. (2020). Prevention of Clostridium Difficile Infection and Associated Diarrhea: An Unsolved Problem. Microorganisms 8, 1640. doi: 10.3390/microorganisms8111640
Puhar, A., Tronchere, H., Payrastre, B., Nhieu, G. T., Sansonetti, P. J. (2013). A Shigella Effector Dampens Inflammation by Regulating Epithelial Release of Danger Signal ATP Through Production of the Lipid Mediator PtdIns5P. Immunity 39, 1121–1131. doi: 10.1016/j.immuni.2013.11.013
Pusztaszeri, M. P., Genta, R. M., Cryer, B. L. (2007). Drug-Induced Injury in the Gastrointestinal Tract: Clinical and Pathologic Considerations. Nat. Clin. Pract. Gastroenterol. Hepatol. 4, 442–453. doi: 10.1038/ncpgasthep0896
Qiu, Y., Zhang, J., Ji, R., Zhou, Y., Shao, L., Chen, D., et al. (2019). Preventative Effects of Selenium-Enriched Bifidobacterium Longum on Irinotecan-Induced Small Intestinal Mucositis in Mice. Benef. Microbes 10, 569–577. doi: 10.3920/BM2018.0096
Reita, D., Bour, C., Benbrika, R., Groh, A., Pencreach, E., Guerin, E., et al. (2019). Synergistic Anti-Tumor Effect of mTOR Inhibitors With Irinotecan on Colon Cancer Cells. Cancers (Basel) 11, 1581. doi: 10.3390/cancers11101581
Riera, P., Salazar, J., Virgili, A. C., Tobena, M., Sebio, A., Gallano, P., et al. (2018). Relevance of CYP3A4*20, UGT1A1*37 and UGT1A1*28 Variants in Irinotecan-Induced Severe Toxicity. Br. J. Clin. Pharmacol. 84, 1389–1392. doi: 10.1111/bcp.13574
Rivory, L. P., Haaz, M. C., Canal, P., Lokiec, F., Armand, J. P., Robert, J. (1997). Pharmacokinetic Interrelationships of Irinotecan (CPT-11) and its Three Major Plasma Metabolites in Patients Enrolled in Phase I/II Trials. Clin. Cancer Res. 3, 1261–1266.
Roberts, A. B., Wallace, B. D., Venkatesh, M. K., Mani, S., Redinbo, M. R. (2013). Molecular Insights Into Microbial Beta-Glucuronidase Inhibition to Abrogate CPT-11 Toxicity. Mol. Pharmacol. 84, 208–217. doi: 10.1124/mol.113.085852
Rossini, A., Rumio, C., Sfondrini, L., Tagliabue, E., Morelli, D., Miceli, R., et al. (2006). Influence of Antibiotic Treatment on Breast Carcinoma Development in Proto-Neu Transgenic Mice. Cancer Res. 66, 6219–6224. doi: 10.1158/0008-5472.CAN-05-4592
Rudakova, E. V., Boltneva, N. P., Makhaeva, G. F. (2011). Comparative Analysis of Esterase Activities of Human, Mouse, and Rat Blood. Bull. Exp. Biol. Med. 152, 73–75. doi: 10.1007/s10517-011-1457-y
Rudolf, E., Kralova, V., Rudolf, K., John, S. (2013). The Role of P38 in Irinotecan-Induced DNA Damage and Apoptosis of Colon Cancer Cells. Mutat. Res. 741–742, 27–34. doi: 10.1016/j.mrfmmm.2013.02.002
Russell, D. A., Ross, R. P., Fitzgerald, G. F., Stanton, C. (2011). Metabolic Activities and Probiotic Potential of Bifidobacteria. Int. J. Food Microbiol. 149, 88–105. doi: 10.1016/j.ijfoodmicro.2011.06.003
Salgado-Pabon, W., Celli, S., Arena, E. T., Nothelfer, K., Roux, P., Sellge, G., et al. (2013). Shigella Impairs T Lymphocyte Dynamics In Vivo. Proc. Natl. Acad. Sci. U. S. A. 110, 4458–4463. doi: 10.1073/pnas.1300981110
Sanada, T., Kim, M., Mimuro, H., Suzuki, M., Ogawa, M., Oyama, A., et al. (2012). The Shigella Flexneri Effector OspI Deamidates UBC13 to Dampen the Inflammatory Response. Nature 483, 623–626. doi: 10.1038/nature10894
Sanghani, S. P., Quinney, S. K., Fredenburg, T. B., Davis, W. I., Murry, D. J., Bosron, W. F. (2004). Hydrolysis of Irinotecan and its Oxidative Metabolites, 7-Ethyl-10-[4-N-(5-Aminopentanoic Acid)-1-Piperidino] Carbonyloxycamptothecin and 7-Ethyl-10-[4-(1-Piperidino)-1-Amino]-Carbonyloxycamptothecin, by Human Carboxylesterases CES1A1, CES2, and a Newly Expressed Carboxylesterase Isoenzyme, CES3. Drug Metab. Dispos. 32, 505–511. doi: 10.1124/dmd.32.5.505
Sansonetti, P. J., Phalipon, A., Arondel, J., Thirumalai, K., Banerjee, S., Akira, S., et al. (2000). Caspase-1 Activation of IL-1beta and IL-18 are Essential for Shigella Flexneri-Induced Inflammation. Immunity 12, 581–590. doi: 10.1016/s1074-7613(00)80209-5
Santos, A., Zanetta, S., Cresteil, T., Deroussent, A., Pein, F., Raymond, E., et al. (2000). Metabolism of Irinotecan (CPT-11) by CYP3A4 and CYP3A5 in Humans. Clin. Cancer Res. 6, 2012–2020.
Schluter, J., Peled, J. U., Taylor, B. P., Markey, K. A., Smith, M., Taur, Y., et al. (2020). The Gut Microbiota is Associated With Immune Cell Dynamics in Humans. Nature 588, 303–307. doi: 10.1038/s41586-020-2971-8
Sellge, G., Magalhaes, J. G., Konradt, C., Fritz, J. H., Salgado-Pabon, W., Eberl, G., et al. (2010). Th17 Cells are the Dominant T Cell Subtype Primed by Shigella Flexneri Mediating Protective Immunity. J. Immunol. 184, 2076–2085. doi: 10.4049/jimmunol.0900978
Sharma, A., Chaudhary, S. P., Raina, V., Shukla, N. K., Sreenivas, V., Prakash, S., et al. (2018). Final Results of a Phase II/III, Randomized, Double Blind, Placebo Controlled Study to Investigate the Efficacy of a High Potency Multistrain Probiotic, on Chemotherapy Induced Diarrhea in Cancer Patients Receiving Fluropyrimidines and/or Irinotecanbased Therapy. Ann. Oncol. 29, viii755. doi: 10.1093/annonc/mdy424.085
Sharma, R., Tobin, P., Clarke, S. J. (2005). Management of Chemotherapy-Induced Nausea, Vomiting, Oral Mucositis, and Diarrhoea. Lancet Oncol. 6, 93–102. doi: 10.1016/S1470-2045(05)01735-3
Shivaji, S. (2021). A Systematic Review of Gut Microbiome and Ocular Inflammatory Diseases: Are They Associated? Indian J. Ophthalmol. 69, 535–542. doi: 10.4103/ijo.IJO_1362_20
Singh, N., Gurav, A., Sivaprakasam, S., Brady, E., Padia, R., Shi, H., et al. (2014). Activation of Gpr109a, Receptor for Niacin and the Commensal Metabolite Butyrate, Suppresses Colonic Inflammation and Carcinogenesis. Immunity 40, 128–139. doi: 10.1016/j.immuni.2013.12.007
Spanogiannopoulos, P., Bess, E. N., Carmody, R. N., Turnbaugh, P. J. (2016). The Microbial Pharmacists Within Us: A Metagenomic View of Xenobiotic Metabolism. Nat. Rev. Microbiol. 14, 273–287. doi: 10.1038/nrmicro.2016.17
Sperandio, B., Regnault, B., Guo, J., Zhang, Z., Stanley, S. J., Sansonetti, P. J., et al. (2008). Virulent Shigella Flexneri Subverts the Host Innate Immune Response Through Manipulation of Antimicrobial Peptide Gene Expression. J. Exp. Med. 205, 1121–1132. doi: 10.1084/jem.20071698
Stringer, A. M. (2013). Interaction Between Host Cells and Microbes in Chemotherapy-Induced Mucositis. Nutrients 5, 1488–1499. doi: 10.3390/nu5051488
Stringer, A. M., Gibson, R. J., Logan, R. M., Bowen, J. M., Yeoh, A. S., Keefe, D. M. (2008). Faecal Microflora and Beta-Glucuronidase Expression are Altered in an Irinotecan-Induced Diarrhea Model in Rats. Cancer Biol. Ther. 7, 1919–1925. doi: 10.4161/cbt.7.12.6940
Sun, R., Basu, S., Zeng, M., Sunsong, R., Li, L., Ghose, R., et al. (2019). Xiao-Chai-Hu-Tang (XCHT) Intervening Irinotecan’s Disposition: The Potential of XCHT in Alleviating Irinotecan-Induced Diarrhea. Curr. Cancer Drug Targets 19, 551–560. doi: 10.2174/1568009618666181029153255
Sun, C. P., Yan, J. K., Yi, J., Zhang, X. Y., Yu, Z. L., Huo, X. K., et al. (2020). The Study of Inhibitory Effect of Natural Flavonoids Toward Beta-Glucuronidase and Interaction of Flavonoids With Beta-Glucuronidase. Int. J. Biol. Macromol. 143, 349–358. doi: 10.1016/j.ijbiomac.2019.12.057
Takeba, Y., Kumai, T., Matsumoto, N., Nakaya, S., Tsuzuki, Y., Yanagida, Y., et al. (2007). Irinotecan Activates P53 With its Active Metabolite, Resulting in Human Hepatocellular Carcinoma Apoptosis. J. Pharmacol. Sci. 104, 232–242. doi: 10.1254/jphs.fp0070442
Tao, J., Li, S., Gan, R. Y., Zhao, C. N., Meng, X., Li, H. B. (2020). Targeting Gut Microbiota With Dietary Components on Cancer: Effects and Potential Mechanisms of Action. Crit. Rev. Food Sci. Nutr. 60, 1025–1037. doi: 10.1080/10408398.2018.1555789
Taylor, M. H., Lee, C. H., Makker, V., Rasco, D., Dutcus, C. E., Wu, J., et al. (2020). Phase IB/II Trial of Lenvatinib Plus Pembrolizumab in Patients With Advanced Renal Cell Carcinoma, Endometrial Cancer, and Other Selected Advanced Solid Tumors. J. Clin. Oncol. 38, 1154–1163. doi: 10.1200/JCO.19.01598
Teillant, A., Gandra, S., Barter, D., Morgan, D. J., Laxminarayan, R. (2015). Potential Burden of Antibiotic Resistance on Surgery and Cancer Chemotherapy Antibiotic Prophylaxis in the USA: A Literature Review and Modelling Study. Lancet Infect. Dis. 15, 1429–1437. doi: 10.1016/S1473-3099(15)00270-4
Toffoli, G., Cecchin, E., Corona, G., Russo, A., Buonadonna, A., D’Andrea, M., et al. (2006). The Role of UGT1A1*28 Polymorphism in the Pharmacodynamics and Pharmacokinetics of Irinotecan in Patients With Metastatic Colorectal Cancer. J. Clin. Oncol. 24, 3061–3068. doi: 10.1200/JCO.2005.05.5400
Urushiyama, H., Jo, T., Yasunaga, H., Michihata, N., Yamana, H., Matsui, H., et al. (2018). Effect of Hangeshashin-To (Japanese Herbal Medicine Tj-14) on Tolerability of Irinotecan: Propensity Score and Instrumental Variable Analyses. J. Clin. Med. 7, 246. doi: 10.3390/jcm7090246
van der Bol, J. M., de Jong, F. A., van Schaik, R. H., Sparreboom, A., van Fessem, M. A., van de Geijn, F. E., et al. (2010). Effects of Mannose-Binding Lectin Polymorphisms on Irinotecan-Induced Febrile Neutropenia. Oncologist 15, 1063–1072. doi: 10.1634/theoncologist.2010-0033
Wallace, B. D., Roberts, A. B., Pollet, R. M., Ingle, J. D., Biernat, K. A., Pellock, S. J., et al. (2015). Structure and Inhibition of Microbiome Beta-Glucuronidases Essential to the Alleviation of Cancer Drug Toxicity. Chem. Biol. 22, 1238–1249. doi: 10.1016/j.chembiol.2015.08.005
Wallace, B. D., Wang, H., Lane, K. T., Scott, J. E., Orans, J., Koo, J. S., et al. (2010). Alleviating Cancer Drug Toxicity by Inhibiting a Bacterial Enzyme. Science 330, 831–835. doi: 10.1126/science.1191175
Wallington, M., Saxon, E. B., Bomb, M., Smittenaar, R., Wickenden, M., McPhail, S., et al. (2016). 30-Day Mortality After Systemic Anticancer Treatment for Breast and Lung Cancer in England: A Population-Based, Observational Study. Lancet Oncol. 17, 1203–1216. doi: 10.1016/S1470-2045(16)30383-7
Wang, Y., Jing, M. X., Jiang, L., Jia, Y. F., Ying, E., Cao, H., et al. (2020). Does a Ketogenic Diet as an Adjuvant Therapy for Drug Treatment Enhance Chemotherapy Sensitivity and Reduce Target Lesions in Patients With Locally Recurrent or Metastatic Her-2-Negative Breast Cancer? Study Protocol for a Randomized Controlled Trial. Trials 21, 487. doi: 10.1186/s13063-020-04429-5
Wang, Y., Sun, L., Chen, S., Guo, S., Yue, T., Hou, Q., et al. (2019). The Administration of Escherichia Coli Nissle 1917 Ameliorates Irinotecan-Induced Intestinal Barrier Dysfunction and Gut Microbial Dysbiosis in Mice. Life Sci. 231, 116529. doi: 10.1016/j.lfs.2019.06.004
Wang, L., Wang, R., Wei, G. Y., Wang, S. M., Du, G. H. (2020a). Dihydrotanshinone Attenuates Chemotherapy-Induced Intestinal Mucositis and Alters Fecal Microbiota in Mice. Biomed. Pharmacother. 128, 110262. doi: 10.1016/j.biopha.2020.110262
Wang, L., Wang, R., Wei, G. Y., Zhang, R. P., Zhu, Y., Wang, Z., et al. (2020b). Cryptotanshinone Alleviates Chemotherapy-Induced Colitis in Mice With Colon Cancer via Regulating Fecal-Bacteria-Related Lipid Metabolism. Pharmacol. Res. 105232. doi: 10.1016/j.phrs.2020.105232
Wu, Y., Wang, D., Yang, X., Fu, C., Zou, L., Zhang, J. (2019a). Traditional Chinese Medicine Gegen Qinlian Decoction Ameliorates Irinotecan Chemotherapy-Induced Gut Toxicity in Mice. Biomed. Pharmacother. 109, 2252–2261. doi: 10.1016/j.biopha.2018.11.095
Wu, K., Zhu, Z., He, Y., Huang, L., Yan, X., Wang, D. (2019b). Efficacy and Safety of Xiao Ai Ping Injection Combined With Chemotherapy in Advanced Gastric Cancer: A Systematic Review and Meta-Analysis. Evid. Based. Complement Alternat. Med. 2019, 3821053. doi: 10.1155/2019/3821053
Xia, J., Jiang, S., Lv, L., Wu, W., Wang, Q., Xu, Q., et al. (2021). Modulation of the Immune Response and Metabolism in Germ-Free Rats Colonized by the Probiotic Lactobacillus Salivarius LI01. Appl. Microbiol. Biotechnol. 105, 1629–1645. doi: 10.1007/s00253-021-11099-z
Xie, Y., Hu, F., Xiang, D., Lu, H., Li, W., Zhao, A., et al. (2020). The Metabolic Effect of Gut Microbiota on Drugs. Drug Metab. Rev. 52, 139–156. doi: 10.1080/03602532.2020.1718691
Xue, H., Field, C. J., Sawyer, M. B., Dieleman, L. A., Baracos, V. E. (2009). Prophylactic Ciprofloxacin Treatment Prevented High Mortality, and Modified Systemic and Intestinal Immune Function in Tumour-Bearing Rats Receiving Dose-Intensive CPT-11 Chemotherapy. Br. J. Cancer. 100, 1581–1588. doi: 10.1038/sj.bjc.6605051
Xue, H., Sawyer, M. B., Field, C. J., Dieleman, L. A., Baracos, V. E. (2007). Nutritional Modulation of Antitumor Efficacy and Diarrhea Toxicity Related to Irinotecan Chemotherapy in Rats Bearing the Ward Colon Tumor. Clin. Cancer Res. 13, 7146–7154. doi: 10.1158/1078-0432.CCR-07-0823
Xu, J., Qiu, J. C., Ji, X., Guo, H. L., Wang, X., Zhang, B., et al. (2019). Potential Pharmacokinetic Herb-Drug Interactions: Have We Overlooked the Importance of Human Carboxylesterases 1 and 2? Curr. Drug Metab. 20, 130–137. doi: 10.2174/1389200219666180330124050
Yachida, S., Mizutani, S., Shiroma, H., Shiba, S., Nakajima, T., Sakamoto, T., et al. (2019). Metagenomic and Metabolomic Analyses Reveal Distinct Stage-Specific Phenotypes of the Gut Microbiota in Colorectal Cancer. Nat. Med. 25, 968–976. doi: 10.1038/s41591-019-0458-7
Yang, W., Wei, B., Yan, R. (2018). Amoxapine Demonstrates Incomplete Inhibition of Beta-Glucuronidase Activity From Human Gut Microbiota. SLAS Discov. 23, 76–83. doi: 10.1177/2472555217725264
Yang, F., Zhu, W., Sun, S., Ai, Q., Edirisuriya, P., Zhou, K. (2020). Isolation and Structural Characterization of Specific Bacterial Beta-Glucuronidase Inhibitors From Noni (Morinda Citrifolia) Fruits. J. Nat. Prod. 83, 825–833. doi: 10.1021/acs.jnatprod.9b00279
Zhang, B., Cheng, P. (2020). Improving Antitumor Efficacy via Combinatorial Regimens of Oncolytic Virotherapy. Mol. Cancer. 19, 158. doi: 10.1186/s12943-020-01275-6
Zhang, M. Q., Lin, X., Li, Y., Lu, S. (2015). Irinotecan as a Second-Line Chemotherapy for Small Cell Lung Cancer: A Systemic Analysis. Asian Pac J. Cancer Prev. 16, 1993–1995. doi: 10.7314/apjcp.2015.16.5.1993
Zheng, D. W., Dong, X., Pan, P., Chen, K. W., Fan, J. X., Cheng, S. X., et al. (2019). Phage-Guided Modulation of the Gut Microbiota of Mouse Models of Colorectal Cancer Augments Their Responses to Chemotherapy. Nat. BioMed. Eng. 3, 717–728. doi: 10.1038/s41551-019-0423-2
Zhou, X., Hong, T., Yu, Q., Nie, S., Gong, D., Xiong, T., et al. (2017). Exopolysaccharides From Lactobacillus Plantarum NCU116 Induce C-Jun Dependent Fas/Fasl-Mediated Apoptosis via TLR2 in Mouse Intestinal Epithelial Cancer Cells. Sci. Rep. 7, 14247. doi: 10.1038/s41598-017-14178-2
Zhu, X. H., Lang, H. D., Wang, X. L., Hui, S. C., Zhou, M., Kang, C., et al. (2019). Synergy Between Dihydromyricetin Intervention and Irinotecan Chemotherapy Delays The Progression of Colon Cancer in Mouse Models. Food Funct. 10, 2040–2049. doi: 10.1039/c8fo01756e
Keywords: irinotecan, gut microbiota, immunoregulation, chemotherapy, toxicity
Citation: Yue B, Gao R, Wang Z and Dou W (2021) Microbiota-Host-Irinotecan Axis: A New Insight Toward Irinotecan Chemotherapy. Front. Cell. Infect. Microbiol. 11:710945. doi: 10.3389/fcimb.2021.710945
Received: 05 June 2021; Accepted: 23 September 2021;
Published: 14 October 2021.
Edited by:
Valeriy Poroyko, Covance, United StatesReviewed by:
Bin Wei, Zhejiang University of Technology, ChinaCopyright © 2021 Yue, Gao, Wang and Dou. This is an open-access article distributed under the terms of the Creative Commons Attribution License (CC BY). The use, distribution or reproduction in other forums is permitted, provided the original author(s) and the copyright owner(s) are credited and that the original publication in this journal is cited, in accordance with accepted academic practice. No use, distribution or reproduction is permitted which does not comply with these terms.
*Correspondence: Wei Dou, ZG91d2VpMTIzNDU2QDEyNi5jb20=; Zhengtao Wang, enR3YW5nQHNodXRjbS5lZHUuY24=
†These authors have contributed equally to this work
Disclaimer: All claims expressed in this article are solely those of the authors and do not necessarily represent those of their affiliated organizations, or those of the publisher, the editors and the reviewers. Any product that may be evaluated in this article or claim that may be made by its manufacturer is not guaranteed or endorsed by the publisher.
Research integrity at Frontiers
Learn more about the work of our research integrity team to safeguard the quality of each article we publish.