- 1Department of Nephrology, Union Hospital, Tongji Medical College, Huazhong University of Science and Technology, Wuhan, China
- 2Department of Surgery, Hubei Provincial Hospital of Traditional Chinese Medicine, Wuhan, China
- 3Hubei Province Academy of Traditional Chinese Medicine, Wuhan, China
The circadian clock regulates numerous key physiological processes and maintains cellular, tissue, and systemic homeostasis. Disruption of circadian clock machinery influences key activities involved in immune response and brain function. Moreover, Immune activation has been closely linked to neurodegeneration. Here, we review the molecular clock machinery and the diurnal variation of immune activity. We summarize the circadian control of immunity in both central and peripheral immune cells, as well as the circadian regulation of brain cells that are implicated in neurodegeneration. We explore the important role of systemic inflammation on neurodegeneration. The circadian clock modulates cellular metabolism, which could be a mechanism underlying circadian control. We also discuss the circadian interventions implicated in inflammation and neurodegeneration. Targeting circadian clocks could be a potential strategy for the prevention and treatment of inflammation and neurodegenerative diseases.
Introduction
Virtually all life has an intrinsic timing system, the so-called biological clock, which coordinates biological processes to adapt to the regular 24-hour light/dark cycles generated by the Earth’s rotation (Bell-Pedersen et al., 2005). In mammals, numerous physiological and behavioral processes exhibit a daily rhythm of approximately 24-hour, such as sleep-wake cycle, locomotor activity, feeding, body temperature, metabolism, immune response, hormone secretion, and cognition (Gerstner and Yin, 2010; Mohawk et al., 2012; Scheiermann et al., 2013; Gnocchi and Bruscalupi, 2017; Mure et al., 2018). These rhythmic activities are driven by cell-autonomous circadian clocks that existed in almost every cell in organisms. However, circadian rhythm can also be affected by external cues and pathological conditions, such as light, diet, feeding pattern, temperature, exercise, oxygen, as well as cancer, cardiovascular disease, inflammation, and neurodegenerative diseases (Cavadini et al., 2007; Kohsaka et al., 2007; Pivovarova et al., 2015; Adamovich et al., 2017; Manoogian and Panda, 2017; Hood and Amir, 2017a; Hood and Amir, 2017b; Crosby et al., 2019; Gabriel and Zierath, 2019).
The circadian system regulates numerous cellular processes, which interact and affect biological activities. For example, circadian clocks modulate cellular metabolism, epigenetic modification, cell cycle, redox homeostasis, gut microbiota, immune response, and cognition. Cellular metabolism is associated with immune activity and neurodegeneration (Camandola and Mattson, 2017; Geltink et al., 2018; Vijayan et al., 2019; Wang et al., 2019). Additionally, both central and peripheral inflammation implicate in neurodegeneration (Trager and Tabrizi, 2013; Chitnis and Weiner, 2017). Disruption of circadian rhythms leads to obesity, diabetes, cancer, immune dysfunction, and cognitive decline. Just as circadian disruption can induce biological disorders, circadian disturbances have also been regarded as the consequences of microbial infection, metabolic disorder, and neurodegenerative diseases. For example, circadian abnormality in sleep/wake cycles is one of the common and earliest signs of neurodegenerative diseases, such as Alzheimer’s disease (AD) and Parkinson’s disease (PD); while the abnormality of circadian rhythms exacerbates the progression of neurodegeneration (Hood and Amir, 2017a). Here, we review the complex circadian network and summarize the circadian control of immune function and neurodegeneration. We explore the complex interplay between systemic inflammation and neurodegeneration and discuss the potential mechanism underlying circadian regulation.
The Circadian Clock in Mammals
The circadian system is an internal timekeeping device, which is hierarchical and organized in multiple oscillators at the organism, cellular, and molecular level in mammals (Reppert and Weaver, 2002; Bell-Pedersen et al., 2005; Saini et al., 2019). The central pacemaker is located in the hypothalamic suprachiasmatic nucleus (SCN) and consists of multiple populations of oscillating neurons and astrocytes; these cells are integrated as a single circadian unit and output a coordinated circadian signal (Herzog et al., 2017; Hastings et al., 2018; Brancaccio et al., 2019). SCN forwards the rhythmic signal via the hypothalamus-pituitary-adrenal (HPA)-axis and the autonomic nervous system, which align the circadian clocks throughout the organism with the external environmental cues, such as light (Kalsbeek et al., 2012; Koronowski and Sassone-Corsi, 2021). At the molecular level, the core clock machinery exists in almost all cells and is a self-sustaining system based on transcription-translation feedback loops (TTFLs); molecular clocks can generate circadian rhythms autonomously without the need of an external signal (Reppert and Weaver, 2001; Dudek and Meng, 2014). The transcriptional factors brain and muscle ARNT-like 1 (Bmal1)/circadian locomotor output cycles Kaput (Clock) heterodimer activate the expression of genes that containing clock regulatory elements (E-box) in their promoters, including Period circadian clock (Per1/2/3), Cryptochrome (Cry1/2), RAR-related orphan receptor alpha (Rorα), Rev-erbα, and D site albumin promoter binding protein (Dbp) genes. Per and Cry, in turn, inhibit the activity of the Bmal1/Clock complex; while the opposing function of nuclear receptors Rorα and Rev-erbα fine-tune the transcription of Bmal1 and nuclear factor interleukin 3 (Nfil3, also known as E4BP4) via activation and repression, respectively (Gekakis et al., 1998; Sato et al., 2004; Ripperger and Schibler, 2006; Haque et al., 2019). DBP and NFIL3 synergistically regulate the expression of Per (Ohno et al., 2007; Yamajuku et al., 2011; Curtis et al., 2014) (Figure 1).
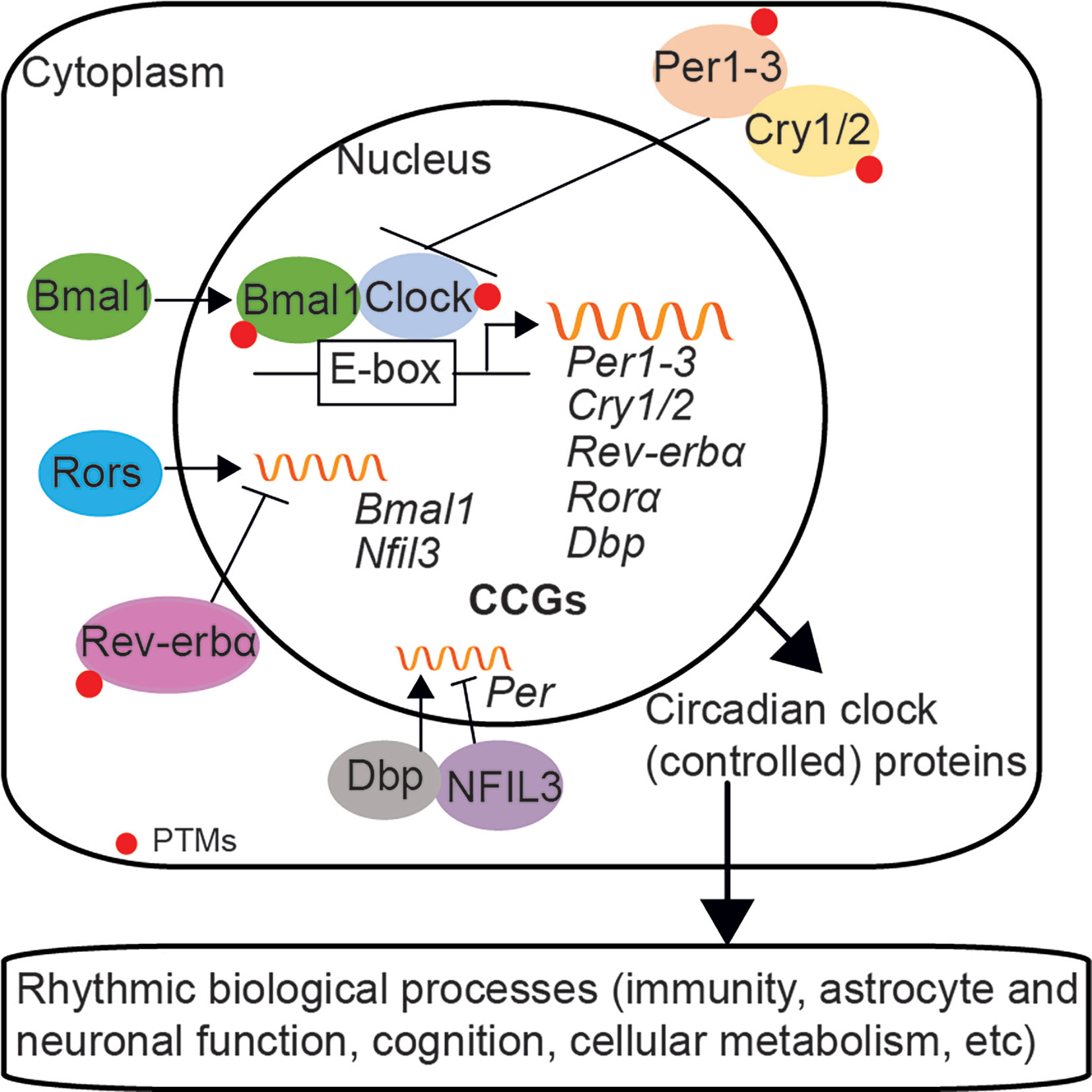
Figure 1 The circadian clock machinery in mammals. The mammalian circadian clock network at the molecular level consists of transcription-translation feedback loops (TTFLs). Bmal1/Clock heterodimer activates the transcription of genes that containing E-box elements in their promoters, such as Per1/2/3, Cry1/2, Rorα, Rev-erbα, and Dbp. Per and Cry proteins translocate back into the nucleus and repress the activity of the Bmal1/Clock complex, which subsequently inhibits their own expression. Rorα and Rev-erbα fine-tune the expression of Bmal1 and Nfil3, via activation and repression, respectively. DBP and NFIL3 regulate the expression of Per. Besides, the stability and nuclear translocation of circadian clock proteins are modulated by post-translational modifications (PTMs). Circadian clocks also control the rhythmic expression of numerous clock-controlled genes (CCGs) and biological processes, such as immunity and brain function.
Except for autoregulation, circadian clocks control the rhythmic expression of widespread genes and proteins throughout the body, thus affect numerous physiological processes (Duffield, 2003; Bass and Takahashi, 2010; Gerstner and Yin, 2010; Curtis et al., 2014; Zhang et al., 2014; Rahman et al., 2015; Man et al., 2016; Loizides-Mangold et al., 2017; Stenvers et al., 2019b; Barca-Mayo et al., 2019). For example, synaptic plasticity and density vary according to time-of-day and circadian clock-Bmal1 deficiency in the brain impairs neuronal function and cognitive performance (Perez-Cruz et al., 2009; Musiek et al., 2013; Jasinska et al., 2015; Krzeptowski et al., 2018; Hasegawa et al., 2019). Transcripts of inflammatory cytokines and host immune responses also show a diurnal variation, while disruption of the circadian clock leads to dysfunction of immunity (Halberg et al., 1960; Bellet et al., 2013; Fonken et al., 2015; Deng et al., 2018). Moreover, circadian clock participates in diverse metabolic processes ranging from glucose transport to gluconeogenesis, lipogenesis, and mitochondrial processes (such as morphological changes, mitochondrial biogenesis, respiration, and oxidative phosphorylation) in both peripheral tissues and the brain (Kohsaka, 2007; Bass and Takahashi, 2010; Kawai et al., 2010; Zhang et al., 2010; Schiaffino et al., 2016; Robles et al., 2017; de Goede et al., 2018). Therefore, disruption of the cell-autonomous clock network has a profound influence on immune responses, energy metabolism, and cognition in mammals (Schiaffino et al., 2016; Stenvers et al., 2019b).
To maintain daily oscillations of circadian clocks to approximately 24 h, the negative-feedback loop of clock network requires a crucial delay between activation and repression of transcription, which is achieved by post-translational modifications (PTMs). For example, transcription repressor Per2 is phosphorylated by casein kinase Iε (CKIε) and targeted for ubiquitin-mediated degradation, while Cry1 is phosphorylated and destabilized by adenosine monophosphate-activated protein kinase (AMPK) (Eide et al., 2005; Lamia et al., 2009). Transcription activator Bmal1 is phosphorylated by mitogen-activated protein kinase (MAPK) and glycogen synthase kinase-3β (GSK3β) (Sanada et al., 2002; Sahar et al., 2010); while Clock possesses intrinsic histone acetyltransferase (HAT) activity indicated a close link between clock machinery and chromatin remolding (Doi et al., 2006). The PTMs modulated by phosphorylation, ubiquitylation, sumoylation, acetylation, and epigenetic modification affect the stability and nuclear translocation of core clock proteins, thus control the time-specific transcription of clock genes (Gallego and Virshup, 2007; Bellet and Sassone-Corsi, 2010; Shi et al., 2016). Protein phosphorylation is the widely reported PTM of the core clocks and plays a key role in generation of circadian rhythms (Reischl and Kramer, 2011). It’s also known that epigenetic modification uses cellular metabolites as a source, which indicates the implication of cellular metabolism in PTMs (Borrelli et al., 2008). Notably, rhythmic oscillations of peroxiredoxins, highly conserved antioxidant proteins, have been observed in both mice and cultured human red blood cells (naturally no nucleus or DNA) (O’Neill and Reddy, 2011; Edgar et al., 2012). These studies indicate the essential role of non-transcriptional dependent redox oscillations in generation of circadian rhythms (Rey and Reddy, 2015). Besides, the redox state can regulate circadian clocks and is also relevant for their functions (Rutter et al., 2001; Fleischhacker et al., 2018; Sundar et al., 2018; Uriz-Huarte et al., 2020). Moreover, the expression pattern of clock genes varies in a tissue-specific manner, which may determine the functional difference of some clock components in different tissues (Ko and Takahashi, 2006).
Effect of Circadian Clock on Peripheral Immune Response
The immune system protects against infections and tissue injury, which has beneficial effects to the host. However, the dysregulated immune response can lead to chronic inflammation, tissue damage, and endotoxin shock (Medzhitov, 2008). While chronic inflammation also presents in tissue stress and malfunction conditions, such as type 2 diabetes, obesity, and neurodegenerative and neuropsychiatric diseases; the persistent low-grade inflammation, in turn, contributes to the further progression of these diseases (DeLegge and Smoke, 2008; Donath and Shoelson, 2011; Cox et al., 2015). Growing evidence shows that circadian clock controls multiple immune functions, such as trafficking of immune cells, pathogen recognition, phagocytic capacity, and secretion of inflammatory cytokines, chemokines, and complement factors (Keller et al., 2009; Curtis et al., 2014; Man et al., 2016; Timmons et al., 2020). Additionally, the mortality caused by lethal bacteria varies according to the time of infection (Halberg et al., 1960; Shackelford and Feigin, 1973; Marpegan et al., 2009). Here, we review the cell-specific disruption of the circadian clock-induced dysfunction of innate and adaptive immunity in peripheral immune cells (Sato et al., 2014; Nakazato et al., 2017; Deng et al., 2018) (Table 1).
In peritoneal macrophages, the transcript of Bmal1 exhibits diurnal rhythm with the peak at the dark phase (Deng et al., 2018; Early et al., 2018; Lee et al., 2020). Bmal1 deficient peritoneal macrophages or bone marrow-derived macrophages (BMDMs) show increased production of IL6, CXCL1, TNFα, MCP1/CCL2, or Il1b expression upon bacterial endotoxin-lipopolysaccharide (LPS) stimulation; both NRF2 and microRNA miR-155 are implicated in Bmal1 control of inflammatory phenotype (Curtis et al., 2015; Early et al., 2018). Myeloid Bmal1 deficient mice show increased IL-1β levels in the serum after LPS administration and increased mortality response to experimental or LPS-induced sepsis (Curtis et al., 2015; Deng et al., 2018; Early et al., 2018). However, deletion of Bmal1 in macrophages protects mice from pneumococcal infection through increased phagocytosis of macrophages and reduced pro-inflammatory cytokines in the lungs (Kitchen et al., 2020). Additionally, Clock deficient BMDMs exhibit reduced expression of proinflammatory genes Il-6, Il1b, Tnfα, Ifn-β, and Ccl2 upon LPS stimulation and decreased secretion of IL-6 and IL-1β after Salmonella infection (Bellet et al., 2013). Per2 mutation reduces the Toll-like receptor 9 (TLR9)-dependent TNFα and IL12 levels in peritoneal macrophages upon challenge with TLR9 ligand (Silver et al., 2012). Cry1 and Cry2 deficiency increases the secretion of TNFα and IL-6 in BMDMs upon LPS stimulation by binding to adenylyl cyclase and subsequently regulating cAMP levels and protein kinase A and NF-κB activity (Narasimamurthy et al., 2012). Moreover, Rev-erbα inhibits the expression of Chemokine (C-C motif) ligand 2 (Ccl2) in peritoneal macrophages and represses the production of IL-6 in human macrophages response to LPS (Gibbs et al., 2012; Sato et al., 2014).
Besides, both clock genes of blood monocytes and Ly6Chi inflammatory monocytes numbers in blood and spleen show a diurnal variation in mice; depletion of Bma1 in myeloid cells disrupts rhythmic trafficking of Ly6Chi monocytes and induces higher expression of inflammatory cytokines and monocyte-attracting chemokines in serum during Listeria monocytogenes infection (Nguyen et al., 2013). Bmal1 is also involved in monocytes and macrophages-related chronic inflammation in diet-induced obesity and atherosclerosis conditions (Nguyen et al., 2013; Huo et al., 2017; Yang et al., 2020). Additionally, Bmal1 regulates the rhythmic expression of chemokine genes-Ccl2, Ccl8, and S100a8 by binding to E-boxes in their promotors in monocytes and peritoneal macrophages (Nguyen et al., 2013). Deletion of Rorα increases the expression of TNFα, IL-1β, and IL-6 in human monocytic cell line (THP-1) (Moharrami et al., 2018). Moreover, NFIL3 is essential for the development of natural killer (NK) cells and CD8α+ conventional dendritic cells (Gascoyne et al., 2009; Kashiwada et al., 2011). It has been reported that approximately 8% of genes are under circadian control in macrophages and more complex molecular mechanisms may be underlying the circadian clock regulation of the innate immune response (Keller et al., 2009).
Furthermore, circadian clock also modulates adaptive immunity (Downton et al., 2020). Rorα and Rorγt regulate the differentiation of pro-inflammatory T helper 17 (Th17) cells and induce the expression of cytokine-interleukin-17 (IL-17) and IL-17F, whereas Rev-erbα antagonizes the function of Rorγt by binding the same DNA motif (Ivanov et al., 2006; Yang et al., 2008; Korn et al., 2009; Amir et al., 2018; Chang et al., 2019). Transcription repressor NFIL3 also suppresses Th17 cell development by directly binding the Rorγt promoter and repressing its activity (Yu et al., 2013). The production of IgE by activated B cells and secretion of cytokines by Th1 and Th2 cells also require the involvement of NFIL3 (Kashiwada et al., 2010; Kashiwada et al., 2011; Motomura et al., 2011; Male et al., 2012). Additionally, lymphocyte migration through lymph nodes and lymph show diurnal variation, which depends on the rhythmic expression of promigratory factors on lymphocytes and can be abolished in lymphocytes loss of Bmal1 (Druzd et al., 2017). However, a study showed that the differentiation of T and B cells and the adaptive immune response are independent of the expression of Bmal1 (Hemmers and Rudensky, 2015). Since the widespread and complex effect of circadian clocks on peripheral immune response, it is necessary to review the role of the molecular clock at the systemic level, especially in circadian disruption and immune dysfunction-related neurodegenerative diseases (Figure 2).
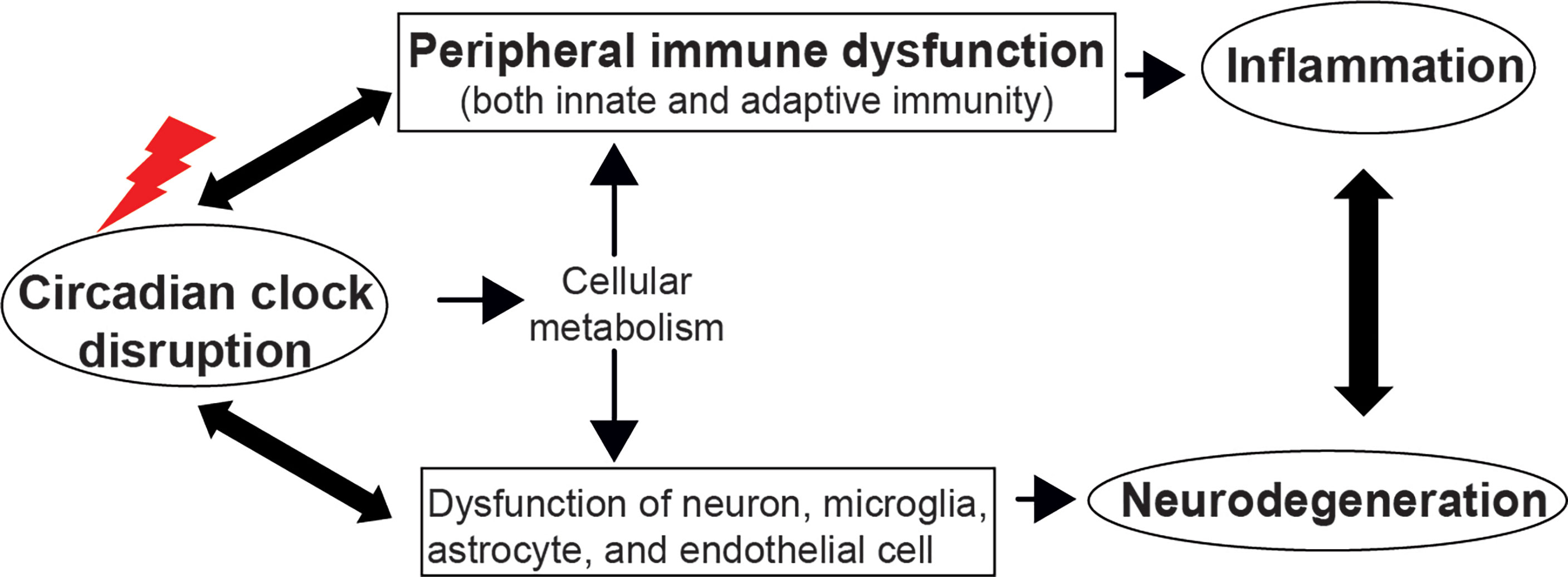
Figure 2 The circadian clock regulates immune function and neurodegeneration. Disruption of the circadian clock machinery leads to immune dysfunction and neurodegeneration, which, in turn, exacerbates the circadian abnormality. The bidirectional communication between systemic inflammation and neurodegeneration deteriorates immune dysfunction and the progression of neurodegenerative diseases. The cellular metabolism pathway could be a mechanism underlying circadian control of immunity and brain function.
Effect of Circadian Clock on Neurodegeneration
Neurodegeneration is mainly characterized by the progressive loss of structure or function of neurons and finally leads to neuronal death. Increasing evidence suggests multiple cell types in the brain are involved in the progression of neurodegeneration (Heneka et al., 2015; Phatnani and Maniatis, 2015; Liddelow et al., 2017; Hickman et al., 2018; Parodi-Rullan et al., 2019). Microglia provide immune surveillance and shape the neural circuits, while astrocytes regulate neuronal signal transmission. Activated microglia and astrocytes induced-chronic neuroinflammation plays a critical role in the development and progression of neurodegeneration (Hickman et al., 2018; Labzin et al., 2018). Thus, maintenance of brain homeostasis is crucial to avoid and prevent the development of neurodegenerative diseases. Compelling studies indicated that circadian clock modulates brain functions and has a direct effect on the function of brain cells, including neurons, astrocytes, microglia, and endothelial cells (Perez-Cruz et al., 2009; Musiek et al., 2013; Jasinska et al., 2015; Barca-Mayo et al., 2017; Krzeptowski et al., 2018; Hasegawa et al., 2019). Circadian clock disruption is an early symptom of neurodegeneration and also could be a risk factor for the development of neurodegenerative diseases (Hood and Amir, 2017a; Leng et al., 2019). AD and PD are the most common neurodegenerative diseases and best studied in relation to circadian rhythms (Breen et al., 2014; Lauretti et al., 2017; Musiek et al., 2018; Leng et al., 2019). Here, we review the effect of disruption of the circadian clock on the function of brain cells and neurodegeneration.
Circadian clock is associated with microglial immune activity. It has been shown that the circadian clock genes are rhythmically expressed in microglial cells isolated from rodents housed under light/dark conditions (Hayashi et al., 2013; Fonken et al., 2015; Wang et al., 2021). Transcripts of pro-inflammatory cytokines-Il1b, Il6, and Tnfa, also show a time-of-day difference in microglia, with elevated expression during the light phase (Fonken et al., 2015; Wang et al., 2020). Additionally, microglial phagocytosis exhibits time-of-day variation (Choudhury et al., 2020; Griffin et al., 2020). Disruption of the circadian function of microglia may sensitize hippocampal inflammatory response in aged rats (Fonken et al., 2016). Notably, the expression of Bmal1 is higher during the light phase compared to the dark phase in microglia isolated from mice, which is consistent with transcripts of pro-inflammatory cytokines (Hayashi et al., 2013; Wang et al., 2020). Microglial Bmal1 deficient mice exhibit less Il6 expression and diminished neuronal damage following middle cerebral artery occlusion than controls (Nakazato et al., 2017). In microglial BV-2 cells, Bmal1 knockdown decreases the expression of pro-inflammatory cytokines (Il1b, Tnfa, and Il6) and increases the expression of anti-inflammatory cytokine-Il10 upon LPS stimulation (Nakazato et al., 2017; Wang et al., 2020). Moreover, lacking Bmal1 increases the microglial phagocytosis in mice on a high-fat diet as well as during the learning process (Wang et al., 2021). Rev-erbα deficient microglia reveal pro-inflammatory phenotypes and elevated NF-kB activation via binding to the promoter regions of related genes; global deletion of Rev-erbα leads to hippocampal microgliosis and neuronal damage in mice (Griffin et al., 2019a). Pharmacologic activation of Rev-erbα inhibits TNFα or LPS-induced pro-inflammatory cytokine expression (Griffin et al., 2019a; Wolff et al., 2020). Furthermore, astrocytes are also involved in innate immunity in the brain and secrete inflammatory mediators. Excessive activation of astrocytes may trigger cell death and neurodegeneration. Rorα deficiency decreases the level of IL-6 at basal conditions in astrocytes, whereas significantly increases IL-6 expression after inflammatory stimulations (Journiac et al., 2009). This study suggests that the circadian clock may exert different effects under different conditions.
In addition, both global Bmal1 knockout and global Rev-erba knockout increase the expression of complement-related genes in the hippocampus; an enhanced transcript of C4b is also observed in the cerebral cortex of neuron and astrocyte Bmal1 knockout mice (Lananna et al., 2018; Griffin et al., 2019b; Griffin et al., 2020). Elevated expression of C4b has also been seen in the neuron-specific Bmal1 knockout and astrocyte-specific Bmal1 knockout mice, but not in microglia-specific Bmal1 knockout mice (Griffin et al., 2020). It’s known that increased C4b and C3 expression is critical for synaptic elimination, which leads to increased microglial phagocytosis and significant synaptic loss in the hippocampal CA3 region of the global Rev-erba mice (Griffin et al., 2020). However, inhibition of Rev-erbα reduces amyloid plaque burden and prevents synaptic loss in 5XFAD mice, an animal model of AD, which may be caused by increased microglial amyloid-β (Aβ) clearance (Lee et al., 2020). Circadian clock also regulates the rhythmic expression of cathepsin S (catS), a microglia-specific lysosomal cysteine protease in the brain, to control the diurnal variation of synaptic strength of the cortical neurons via extracellular proteolysis; Clock deficiency reduces the gene expression of CatS in microglia cells, while deletion of CatS increases spine density and alters synaptic strength, which leads to hyperlocomotor activity in mice (Hayashi et al., 2013).
Global Bmal1 knockout mice are complete loss of locomotor circadian rhythm in constant darkness and display impaired hippocampus-dependent learning and memory (Bunger et al., 2000; Wardlaw et al., 2014). These mice also exhibit age-dependent severe astrocyte activation in the cortex and hippocampus, increased expression of pro-inflammatory cytokines-Tnfa and cyclooxygenase-2 (COX2, Ptghs2), and discrete presynaptic terminal degeneration caused by increased oxidative stress-induced neuronal damage (Musiek et al., 2013; Yang et al., 2016). The astrogliosis and neuropathology are recapitulated in neuron and astrocyte Bmal1 knockout and Clock/neuronal PAS domain protein 2 (NPAS2) double-knockout mice, but not in Per1/Per2 double-knockout mice (Musiek et al., 2013). Neuron and astrocyte Bmal1 knockout mice also exhibit increased total activity in response to novel environment (Musiek et al., 2013). Additionally, global Bmal1 knockout abolished the rhythmic expression of soluble Aβ peptide in hippocampal interstitial fluid and increases amyloid plaque deposition in a mouse model of β-amyloidosis (APPPS1-21), which might be mediated by the increased expression of apolipoprotein E (Apoe) (Kress et al., 2018). Specific-deletion of Bmal1 from forebrain excitatory neurons impairs forebrain dependent spatial memory in mice (Snider et al., 2016). Moreover, in MPTP induced-PD mouse model, lacking Bmal1 exacerbates dopaminergic neuronal loss and glial activation, decreases dopamine transmitter, and aggravates the deficit motor ability (Liu et al., 2020). A study shows that astrocyte-specific Bmal1 deletion induces cell-autonomous astrocyte activation and inflammatory gene expression, which may be partially mediated by inhibition of glutathione signaling; primary cell co-culture experiment shows that Bmal1 deficient astrocytes promote wild-type neuronal death (Lananna et al., 2018). Deletion of Bmal1 from astrocytes affects the vasoactive intestinal polypeptide (VIP) expression in SCN and the oscillation of neuronal clocks through GABA signaling; astrocyte-specific Bmal1 knockout mice display altered circadian locomotor and impaired cognitive phenotypes (Barca-Mayo et al., 2017).
The blood-brain barrier (BBB) isolates the brain from the periphery and is critical for neural function and brain homeostasis. The dysfunction of BBB is implicated in the progression of neurodegeneration in both AD and PD (Kortekaas et al., 2005; Sagare et al., 2013; Parodi-Rullan et al., 2019). The function of BBB is also controlled by circadian clock. Endothelial-specific knockout Bmal1 abolishes the rhythmic efflux by BBB and reduces the efflux from the brain (Zhang et al., 2021). Additionally, Bmal1 deletion from Nestin-positive cells decreases the pericyte coverage of blood vessels and increases the permeability of BBB (Nakazato et al., 2017). Notably, the brain also contains macrophages in perivascular, meningeal, and choroid plexus, which are distinct from microglia and regulate the function of brain barriers (Mendes-Jorge et al., 2009; Bergen et al., 2015). These macrophages may be also regulated by circadian clock and involved in neurodegeneration (Hawkes and McLaurin, 2009; Baruch et al., 2013; Maleki and Rivest, 2019; Ivan et al., 2020). These studies emphasize the importance of circadian clock in maintaining brain homeostasis. Coordinated response of brain cells promotes the progression of neurodegeneration in response to circadian disruption.
Effect of Peripheral Immunity on Neurodegeneration
Systemic inflammation or infection has been regarded as a risk factor for neurodegeneration. Past infections may contribute to cognitive impairment and increase the odds of AD and PD development in later life (Dunn et al., 2005; Katan et al., 2013; Semmler et al., 2013; Widmann and Heneka, 2014; Meng et al., 2019). The low dose of peripheral LPS injection induces sickness behaviors and hippocampal inflammation in rats, which are more severe when the administration during the light (i.e. sleep) phase (Fonken et al., 2015). A single LPS injection induced-severe systemic inflammation leads to persistent microglial activation and inflammatory gene expression, as well as long-term cognitive deficits via remained elevated NOS2 expression (Weberpals et al., 2009). Besides, peripheral LPS challenge results in the persistent increase of TNFα expression in the brain through TNFα receptors, which leads to microglial proliferation and activation, as well as progressive dopamine neuronal loss (Qin et al., 2007). Importantly, numerous studies revealed that LPS administration is able to recapitulate the pathologies and symptoms of PD in animal models (Liu and Bing, 2011; Zhao et al., 2018). Accumulating evidence suggests that low-grade systemic inflammation caused by tissue stress and malfunction conditions, such as high-fat diet consumption, obesity, and diabetes, also leads to cognitive impairment in both adult humans and rodents (Dunn et al., 2005; Murray et al., 2009; Pistell et al., 2010; Edwards et al., 2011; Holloway et al., 2011; Heyward et al., 2012; Pedditzi et al., 2016). The communication between peripheral inflammatory mediators and the brain has been well studied in animal models. It’s shown that the immunological mediators can activate the local afferent vagus pathway; they can also enter into circulation and directly activate macrophages in circumventricular organs where lack a functional BBB (Tracey, 2002; Perry et al., 2007). Additionally, inflammatory mediators interact with endothelial cells and perivascular macrophages, which may cause dysfunction of BBB and peripheral immune cell infiltration (Laflamme and Rivest, 1999; Bohatschek et al., 2001). The peripheral signals and infiltrated immune cells stimulate microglia and astrocyte activation, which subsequently contributes to neuroinflammation and neuronal damage (Puntener et al., 2012; Widmann and Heneka, 2014).
Moreover, systemic inflammation exacerbates the progression of the ongoing neurodegenerative diseases. A single peripheral LPS injection increases the production of IL-1β and transiently elevates the level of amyloid-β in the brain of Tg2576 and APP/PS1 mice (Sly et al., 2001; Tejera et al., 2019). Besides, LPS treatment triggers microglial activation and peripheral myeloid cell infiltration as well as impairs microglial clearance of amyloid-β in APP/PS1 mice (Tejera et al., 2019). Long-term LPS challenge significantly increases the production of IL-1β and deposition of amyloid precursor protein as well as exacerbates the tau phosphorylation in AD mouse models (Sheng et al., 2003; Kitazawa et al., 2005). Notably, neurodegeneration is a systemic disorder. Both human and animal studies reveal the increased levels of peripheral pro-inflammatory factors and immune cells in the circulation of AD and PD (Collins et al., 2012; Chen et al., 2015; Lai et al., 2017; Yang et al., 2017; King et al., 2018). The blood-borne molecules and peripheral immune cells infiltrate into the brain and exacerbate the progression of neurodegeneration (Zenaro et al., 2015; Kempuraj et al., 2017; St-Amour et al., 2019; Cipollini et al., 2019). In periphery, neutrophil migration from blood to tissue follows a diurnal rhythm, which can be abolished by neutrophil-specific Bmal1 deletion (Adrover et al., 2019). Neutrophil invasion has been seen in the brain of both human and mouse models of AD (5xFAD and 3xTg-AD), while depletion of neutrophils improves memory and reduces AD pathology (Zenaro et al., 2015). Infiltrating T lymphocytes have also been observed in post-mortem brains of patients with frontotemporal dementia and PD (Brochard et al., 2009; Laurent et al., 2017). Depletion of the T cell prevents hippocampal T cell infiltration and reverts spatial memory deficits in a mouse model of AD (THY-Tau22) (Laurent et al., 2017). Th17 cells are also involved in the pathogenesis of AD; neutralization of IL17 cytokine produced by Th17 cells rescues cognitive deficits and ameliorates amyloid-β-induced neuroinflammation in mice (Zhang et al., 2013; Zenaro et al., 2015; Cristiano et al., 2019). Deficiency of mature T lymphocytes or lacking CD4+ T cell attenuates dopaminergic neuronal death in the MPTP mouse model of PD (Brochard et al., 2009). Studies also indicated the Th17 cell infiltration in MPTP-treated mice, which exaggerates dopaminergic neuronal loss (Reynolds et al., 2010; Dutta et al., 2019). Moreover, monocytes have been seen in the brain of APP/PS1 mice, but these cells take up Aβ aggregates and transport them back to the bloodstream (Michaud et al., 2013). These infiltrated cell populations have been identified as key players in neurodegenerative diseases. The complex interplay between systemic inflammatory components and dysfunctional brain cells deteriorates the progression of neurodegeneration.
The Cellular Metabolism - A Mechanism Underlying Circadian Control of Immunity and Neurodegeneration
Recent studies have shown that the metabolic pathway plays a central role in modulation of immunity and brain function (Fukuzumi et al., 1996; Kelly and O’Neill, 2015; Man et al., 2016; O’Neill and Kishton, 2016; O’Neill and Pearce, 2016; Geltink et al., 2018; Wang et al., 2019; Bernier et al., 2020). Immune activity is high energy demand and metabolic disorders are associated with immune dysfunction and neurodegeneration (Bird, 2019; Muddapu et al., 2020). Alteration of cellular metabolisms, such as glycolysis, oxidative phosphorylation, fatty acid, and amino acid metabolism, affects the immune cell response (O’Neill and Kishton, 2016). Enhanced glycolysis and fatty acid synthesis are correlated with peripheral immune cell activation, which leads to a more pro-inflammatory state (Doughty et al., 2006; Posokhova et al., 2008; Rodriguez-Prados et al., 2010; Michalek et al., 2011; Everts et al., 2014; Sharma et al., 2020); whereas, oxidative phosphorylation and fatty acid oxidation have been associated with a more anti-inflammatory phenotype (Michalek et al., 2011; Jha et al., 2015; Patsoukis et al., 2015). Besides, cellular metabolism plays a crucial role in regulating the function of brain cells, which is associated with brain homeostasis. Microglial inflammatory response depends on glycolysis for energy production (Orihuela et al., 2016). Inhibition of glucose transporter 1 reduces glucose uptake and glycolysis in microglia under LPS + IFNγ stimulation, which prevents the upregulation of pro-inflammatory cytokines (Wang et al., 2019). Specific knockdown of lipoprotein lipase (Lpl) in microglia reduces lipid uptake and shifts fuel utilization to glutamine, which attenuates microglial immune response under inflammatory stimuli (Gao et al., 2017). Suppression of glutamine synthetase decreases insulin-mediated glucose uptake and increases the expression of inflammatory mediators in activated microglia (Palmieri et al., 2017). In addition, astrocytes regulate the brain glucose metabolism and provide lactate that is converted from glycogen to supply the high energy requirement of neurons (Suzuki et al., 2011; Weber and Barros, 2015). Astrocytes also rapidly uptake glutamate and metabolize to glutamine to maintain brain homeostasis (Schousboe et al., 2014). Metabolic dysfunction of astrocytes contributes to neurodegeneration (Yan et al., 2013; Oksanen et al., 2019; Sonninen et al., 2020).
Numerous transgenic animal studies revealed that circadian clocks control metabolic processes in extensive tissues (Rudic et al., 2004; Hatanaka et al., 2010; Marcheva et al., 2010; Zhang et al., 2010; Sadacca et al., 2011; Marcheva et al., 2013; Dudek and Meng, 2014; Jha et al., 2015; Schiaffino et al., 2016; Dyar et al., 2018; Sussman et al., 2019). Rhythmic expression of metabolic-related genes optimizes the amount of energy consumption (Wang et al., 2015). It has known that the expression of genes related to microglial glucose and fatty acid uptake shows a time-of-day difference; the expression of glucose transporter member 5 (Glut5) and Lpl is increased during the dark phase when microglia are more active (Wang et al., 2020). Thus, oscillation in immunity may be driven by rhythmic changes in cellular metabolism. Circadian disruption impacts cellular metabolism and subsequently drives immune dysfunction and neurodegeneration (Carroll et al., 2019; Bernier et al., 2020; Wang et al., 2020). For example, deletion of Bmal1 in macrophages increases pyruvate kinase M2 expression (PKM2) and lactate production, which is required for expression of the immune checkpoint protein-programmed cell death 1 ligand 1 (PD-L1); mice lacking Bmal1 in myeloid cells are more vulnerable to septic death response to severe infection (Deng et al., 2018). However, knockdown of Bmal1 in microglia decreases expression of Glu5 and Lpl, as well as reduces Il1b (Wang et al., 2020). A study also showed that the protein expression of Clock and Bmal1 in astrocytes is elevated in the human cortex with AD; Overexpression of Clock and Bmal1 in human astrocytes significantly inhibits aerobic glycolysis and lactate production but promotes cytotoxicity and functional impairment (Yoo et al., 2020). Thus, maintenance of the homeostasis of the molecular clock network and cellular metabolism is crucial to proper immune function and prevents neurodegeneration.
Molecule Intervention of Circadian Clock in Inflammation and Neurodegeneration
It’s reported that 82.2% of protein-coding genes identified as druggable targets by the U.S. Food and Drug Administration show rhythmic transcription in at least one human tissue (Mure et al., 2018). Therapeutic targeting circadian core clocks could be a potential strategy to prevent or alleviate inflammation and neurodegenerative diseases (Griffett and Burris, 2013; Ruan et al., 2021) (Table 2). Drug molecules that modulate REV-ERBα have been widely studied in different disease models (Uriz-Huarte et al., 2020; Wang et al., 2020). GSK4112-a agonist of REV-ERBα, suppresses IL-6 protein secretion in human MDMs, primary alveolar macrophages, and THP-1 cells in response to LPS; GSK4112 also represses the gene expression of chemokine (Cxcl11 and Cxcl6), and cytokine (Il19 and Il10) in human primary MDMs (Gibbs et al., 2012). Both Hemin-the endogenous activator of REV-ERB and GSK4112 reduce the transcript of Il6 in THP-1 cells after LPS administration (Raghuram et al., 2007; Gibbs et al., 2012). In contrast, during viral-induced encephalitis, pretreatment of REV-ERBα antagonist-SR8278 significantly reduces the survival rate in mice when infection at the start of the active phase, via an increased transcript of pro-inflammatory chemokine-CCL2 (Gagnidze et al., 2016). Surprisingly, REV-ERBα antagonist-GSK1362 also represses the transcript of LPS-induced inflammatory genes-Il6, Ccl2, and G-csf in alveolar macrophages and inhibits the Il6 gene expression in BMDMs, which may be mediated by stabilizing REV-ERBα protein (Pariollaud et al., 2018). Moreover, inverse agonists of RORα and RORγ, including SR100, ursolic acid, SR2211, SR1555, and ML209, inhibit the development of Th17 cells and reduce associated inflammatory gene expression (Kojetin and Burris, 2014).
Besides, REV-ERBα agonists-GSK4112 and SR9011 suppress microglial activation, reduce iNOS and COX-2 secretion, and transcription of Il6 and Tnfα, which may be mediated by repression of nuclear factor kappa B (NF-κB) pathway in LPS-treated microglial cells (Nakazato et al., 2017; Guo et al., 2019). Moreover, SR9011 reduces phagocytosis, mitochondrial respiration, energy production, and metabolic-related gene expression in primary microglial cells; while under TNFα stimulation, SR9011 decreases the transcripts of Tnfα, Il6, Ccl2, and Il1β, and increases the expression of Il10 in primary microglia (Wolff et al., 2020). Activation of Rev-erbα with agonist-SR9009 suppresses IL-1β secretion and Il6 mRNA expression in primary microglial cells under LPS combined with ATP stimulation, and also significantly reduces the gene expression of Il6, Tnfa, and Il1b in LPS-induced primary astrocytes (Griffin et al., 2019a). SR9009 diminishes LPS-induced transcripts of Il6 and Ccl2 in mice hippocampus (Griffin et al., 2019a). REV-ERB agonists (SR9011, SR9009, and SR10067) increase wakefulness and reduce sleep and anxiety-like behavior in mice; notably, the anxiolytic activity of SR10067 is significantly superior than SR9011 (Banerjee et al., 2014; Chung et al., 2014). Whereas, microinfusion of REV-ERBα antagonist-SR8278 into the ventral midbrain induces Mania-like behavior related to the central hyperdopaminergic state (Chung et al., 2014). Additionally, SR8278 promotes microglial phagocytic capacity and increases fAβ1-42 uptake by BV-2 cells (Lee et al., 2020).
Conclusion
There is clear evidence for the involvement of circadian clock machinery in immune homeostasis and the function of brain cells. Circadian clocks regulate the rhythmic activity of immune cells and govern immune response throughout the body. The function of neuron, astrocyte, and endothelia cells is also regulated by circadian clocks. Circadian disruption leads to immune dysfunction and neurodegeneration. Systemic inflammation is a risk factor and also an accelerator of neurodegenerative diseases, such as AD and PD. Cellular metabolism pathways could be involved in the circadian control of immunity and brain function. Thus, modulation of circadian clocks and cellular metabolism could be a promising therapeutic avenue for inflammatory and neurodegenerative diseases. According to the rhythmic expression of numerous druggable target genes, the time of day for treatment should also be considered to optimize the efficiency. Changing the environment and lifestyle, such as light exposure, exercise, and food intake pattern can entrain the circadian system, which may have a therapeutic effect on inflammation and neurodegeneration (Riemersma-van Der Lek et al., 2008; Figueiro, 2017; Videnovic et al., 2017; Ruan et al., 2021). Moreover, microbial infection, inflammation, and neurodegeneration, in turn, disrupt the clock machinery and subsequently exacerbate the progression of neurodegeneration. Neurodegenerative diseases have a long induction period and no effective therapy. A greater mechanistic understanding of the complex circadian system and the interplay between clock network, inflammation, and neurodegeneration could be critical to identify and manage neurodegenerative diseases in their earliest form. How to pharmacologically target the circadian clock in a cell-type-specific manner from a systemic level needs to be further investigated.
Author Contributions
X-LW and LL conceived the work and wrote the manuscript. All authors contributed to the article and approved the submitted version.
Conflict of Interest
The authors declare that the research was conducted in the absence of any commercial or financial relationships that could be construed as a potential conflict of interest.
Publisher’s Note
All claims expressed in this article are solely those of the authors and do not necessarily represent those of their affiliated organizations, or those of the publisher, the editors and the reviewers. Any product that may be evaluated in this article, or claim that may be made by its manufacturer, is not guaranteed or endorsed by the publisher.
References
Adamovich, Y., Ladeuix, B., Golik, M., Koeners, M. P., Asher, G. (2017). Rhythmic Oxygen Levels Reset Circadian Clocks Through HIF1 Alpha. Cell Metab. 25, 93. doi: 10.1016/j.cmet.2016.09.014
Adrover, J. M., Del Fresno, C., Crainiciuc, G., Cuartero, M. I., Casanova-Acebes, M., Weiss, L. A., et al. (2019). A Neutrophil Timer Coordinates Immune Defense and Vascular Protection. Immunity 50, 390. doi: 10.1016/j.immuni.2019.01.002
Amir, M., Chaudhari, S., Wang, R., Campbell, S., Mosure, S. A., Chopp, L. B., et al. (2018). REV-ERBalpha Regulates TH17 Cell Development and Autoimmunity. Cell Rep. 25, 3733. doi: 10.1016/j.celrep.2018.11.101
Banerjee, S., Wang, Y. J., Solt, L. A., Griffett, K., Kazantzis, M., Amador, A., et al. (2014). Pharmacological Targeting of the Mammalian Clock Regulates Sleep Architecture and Emotional Behaviour. Nat. Commun. 5, 5759. doi: 10.1038/Ncomms6759
Barca-Mayo, O., Boender, A. J., Armirotti, A., De Pietri Tonelli, D. (2019). Deletion of Astrocytic BMAL1 Results in Metabolic Imbalance and Shorter Lifespan in Mice. Glia. 68 (6), 1131–1147 doi: 10.1002/glia.23764
Barca-Mayo, O., Pons-Espinal, M., Follert, P., Armirotti, A., Berdondini, L., De Pietri Tonelli, D. (2017). Astrocyte Deletion of Bmal1 Alters Daily Locomotor Activity and Cognitive Functions via GABA Signalling. Nat. Commun. 8, 14336. doi: 10.1038/ncomms14336
Baruch, K., Ron-Harel, N., Gal, H., Deczkowska, A., Shifrut, E., Ndifon, W., et al. (2013). CNS-Specific Immunity at the Choroid Plexus Shifts Toward Destructive Th2 Inflammation in Brain Aging. Proc. Natl. Acad. Sci. U. S. A. 110, 2264. doi: 10.1073/pnas.1211270110
Bass, J., Takahashi, J. S. (2010). Circadian Integration of Metabolism and Energetics. Science 330, 1349. doi: 10.1126/science.1195027
Bellet, M. M., Deriu, E., Liu, J. Z., Grimaldi, B., Blaschitz, C., Zeller, M., et al. (2013). Circadian Clock Regulates the Host Response to Salmonella. Proc. Natl. Acad. Sci. U. S. A. 110, 9897. doi: 10.1073/pnas.1120636110
Bellet, M. M., Sassone-Corsi, P. (2010). Mammalian Circadian Clock and Metabolism - the Epigenetic Link. J. Cell Sci. 123, 3837. doi: 10.1242/jcs.051649
Bell-Pedersen, D., Cassone, V. M., Earnest, D. J., Golden, S. S., Hardin, P. E., Thomas, T. L., et al. (2005). Circadian Rhythms From Multiple Oscillators: Lessons From Diverse Organisms. Nat. Rev. Genet. 6, 544. doi: 10.1038/nrg1633
Bergen, A. A., Kaing, S., ten Brink, J. B., Gorgels, T. G., Janssen, S. F., Bank, N. B. (2015). Gene Expression and Functional Annotation of Human Choroid Plexus Epithelium Failure in Alzheimer’s Disease. BMC Genomics 16, 956. doi: 10.1186/S12864-015-2159-Z
Bernier, L. P., York, E. M., MacVicar, B. A. (2020). Immunometabolism in the Brain: How Metabolism Shapes Microglial Function. Trends Neurosci. 43, 854. doi: 10.1016/j.tins.2020.08.008
Bird, L. (2019). Getting Enough Energy for Immunity. Nat. Rev. Immunol. 19, 269. doi: 10.1038/s41577-019-0159-y
Bohatschek, M., Werner, A., Raivich, G. (2001). Systemic LPS Injection Leads to Granulocyte Influx Into Normal and Injured Brain: Effects of ICAM-1 Deficiency. Exp. Neurol. 172, 137. doi: 10.1006/exnr.2001.7764
Borrelli, E., Nestler, E. J., Allis, C. D., Sassone-Corsi, P. (2008). Decoding the Epigenetic Language of Neuronal Plasticity. Neuron 60, 961. doi: 10.1016/j.neuron.2008.10.012
Brancaccio, M., Edwards, M. D., Patton, A. P., Smyllie, N. J., Chesham, J. E., Maywood, E. S., et al. (2019). Cell-Autonomous Clock of Astrocytes Drives Circadian Behavior in Mammals. Science 363, 187. doi: 10.1126/science.aat4104
Breen, D. P., Vuono, R., Nawarathna, U., Fisher, K., Shneerson, J. M., Reddy, A. B., et al. (2014). Sleep and Circadian Rhythm Regulation in Early Parkinson Disease. JAMA Neurol. 71, 589. doi: 10.1001/jamaneurol.2014.65
Brochard, V., Combadiere, B., Prigent, A., Laouar, Y., Perrin, A., Beray-Berthat, V., et al. (2009). Infiltration of CD4(+) Lymphocytes Into the Brain Contributes to Neurodegeneration in a Mouse Model of Parkinson Disease. J. Clin. Invest. 119, 182. doi: 10.1172/JCI36470
Bunger, M. K., Wilsbacher, L. D., Moran, S. M., Clendenin, C., Radcliffe, L. A., Hogenesch, J. B., et al. (2000). Mop3 Is an Essential Component of the Master Circadian Pacemaker in Mammals. Cell 103, 1009. doi: 10.1016/S0092-8674(00)00205-1
Camandola, S., Mattson, M. P. (2017). Brain Metabolism in Health, Aging, and Neurodegeneration. EMBO J. 36, 1474. doi: 10.15252/embj.201695810
Carroll, R. G., Timmons, G. A., Cervantes-Silva, M. P., Kennedy, O. D., Curtis, A. M. (2019). Immunometabolism Around the Clock. Trends Mol. Med. 25, 612. doi: 10.1016/j.molmed.2019.04.013
Cavadini, G., Petrzilka, S., Kohler, P., Jud, C., Tobler, I., Birchler, T., et al. (2007). TNF-Alpha Suppresses the Expression of Clock Genes by Interfering With E-Box-Mediated Transcription. Proc. Natl. Acad. Sci. U. S. A. 104, 12843. doi: 10.1073/pnas.0701466104
Chang, C., Loo, C. S., Zhao, X., Solt, L. A., Liang, Y. Q., Bapat, S. P., et al. (2019). The Nuclear Receptor REV-ERB Alpha Modulates Th17 Cell-Mediated Autoimmune Disease. Proc. Natl. Acad. Sci. U. S. A. 116, 18528. doi: 10.1073/pnas.1907563116
Chen, Y. H., Qi, B. Q., Xu, W. F., Ma, B., Li, L., Chen, Q. M., et al. (2015). Clinical Correlation of Peripheral CD4+-Cell Sub-Sets, Their Imbalance and Parkinson’s Disease. Mol. Med. Rep. 12, 6105. doi: 10.3892/mmr.2015.4136
Chitnis, T., Weiner, H. L. (2017). CNS Inflammation and Neurodegeneration. J. Clin. Invest. 127, 3577. doi: 10.1172/JCI90609
Choudhury, M. E., Miyanishi, K., Takeda, H., Islam, A., Matsuoka, N., Kubo, M., et al. (2020). Phagocytic Elimination of Synapses by Microglia During Sleep. Glia 68, 44. doi: 10.1002/glia.23698
Chung, S., Lee, E. J., Yun, S., Choe, H. K., Park, S. B., Son, H. J., et al. (2014). Impact of Circadian Nuclear Receptor REV-ERB Alpha on Midbrain Dopamine Production and Mood Regulation. Cell 157, 858. doi: 10.1016/j.cell.2014.03.039
Cipollini, V., Anrather, J., Orzi, F., Iadecola, C. (2019). Th17 and Cognitive Impairment: Possible Mechanisms of Action. Front. Neuroanat. 13, 95. doi: 10.3389/Fnana.2019.00095
Collins, L. M., Toulouse, A., Connor, T. J., Nolan, Y. M. (2012). Contributions of Central and Systemic Inflammation to the Pathophysiology of Parkinson’s Disease. Neuropharmacology 62, 2154. doi: 10.1016/j.neuropharm.2012.01.028
Cox, A. J., West, N. P., Cripps, A. W. (2015). Obesity, Inflammation, and the Gut Microbiota. Lancet Diabetes Endo. 3, 207. doi: 10.1016/S2213-8587(14)70134-2
Cristiano, C., Volpicelli, F., Lippiello, P., Buono, B., Raucci, F., Piccolo, M., et al. (2019). Neutralization of IL-17 Rescues Amyloid-Beta-Induced Neuroinflammation and Memory Impairment. Brit. J. Pharmacol. 176, 3544. doi: 10.1111/bph.14586
Crosby, P., Hamnett, R., Putker, M., Hoyle, N. P., Reed, M., Karam, C. J., et al. (2019). Insulin/IGF-1 Drives PERIOD Synthesis to Entrain Circadian Rhythms With Feeding Time. Cell 177, 896. doi: 10.1016/j.cell.2019.02.017
Curtis, A. M., Bellet, M. M., Sassone-Corsi, P., O’Neill, L. A. J. (2014). Circadian Clock Proteins and Immunity. Immunity 40, 178. doi: 10.1016/j.immuni.2014.02.002
Curtis, A. M., Fagundes, C. T., Yang, G. R., Palsson-McDermott, E. M., Wochal, P., McGettrick, A. F., et al. (2015). Circadian Control of Innate Immunity in Macrophages by miR-155 Targeting Bmal1. Proc. Natl. Acad. Sci. U. S. A. 112, 7231. doi: 10.1073/pnas.1501327112
de Goede, P., Wefers, J., Brombacher, E. C., Schrauwen, P., Kalsbeek, A. (2018). Circadian Rhythms in Mitochondrial Respiration. J. Mol. Endocrinol. 60, R115. doi: 10.1530/Jme-17-0196
DeLegge, M. H., Smoke, A. (2008). Neurodegeneration and Inflammation. Nutr. Clin. Pract. 23, 35. doi: 10.1177/011542650802300135
Deng, W. J., Zhu, S., Zeng, L., Liu, J., Kang, R., Yang, M. H., et al. (2018). The Circadian Clock Controls Immune Checkpoint Pathway in Sepsis. Cell Rep. 24, 366. doi: 10.1016/j.celrep.2018.06.026
Doi, M., Hirayama, J., Sassone-Corsi, P. (2006). Circadian Regulator CLOCK Is a Histone Acetyltransferase. Cell 125, 497. doi: 10.1016/j.cell.2006.03.033
Donath, M. Y., Shoelson, S. E. (2011). Type 2 Diabetes as an Inflammatory Disease. Nat. Rev. Immunol. 11, 98. doi: 10.1038/nri2925
Doughty, C. A., Bleiman, B. F., Wagner, D. J., Dufort, F. J., Mataraza, J. M., Roberts, M. F., et al. (2006). Antigen Receptor-Mediated Changes in Glucose Metabolism in B Lymphocytes: Role of Phosphatidylinositol 3-Kinase Signaling in the Glycolytic Control of Growth. Blood 107, 4458. doi: 10.1182/blood-2005-12-4788
Downton, P., Early, J. O., Gibbs, J. E. (2020). Circadian Rhythms in Adaptive Immunity. Immunology 161, 268. doi: 10.1111/imm.13167
Druzd, D., Matveeva, O., Ince, L., Harrison, U., He, W. Y., Schmal, C., et al. (2017). Lymphocyte Circadian Clocks Control Lymph Node Trafficking and Adaptive Immune Responses. Immunity 46, 120. doi: 10.1016/j.immuni.2016.12.011
Dudek, M., Meng, Q. J. (2014). Running on Time: The Role of Circadian Clocks in the Musculoskeletal System. Biochem. J. 463, 1. doi: 10.1042/BJ20140700
Duffield, G. E. (2003). DNA Microarray Analyses of Circadian Timing: The Genomic Basis of Biological Time. J. Neuroendocrinol. 15, 991. doi: 10.1046/j.1365-2826.2003.01082.x
Dunn, N., Mullee, M., Perry, V. H., Holmes, C. (2005). Association Between Dementia and Infectious Disease: Evidence From a Case-Control Study. Alzheimer Dis. Assoc. Disord. 19, 91. doi: 10.1097/01.wad.0000165511.52746.1f
Dutta, D., Kundu, M., Mondal, S., Roy, A., Ruehl, S., Hall, D. A., et al. (2019). RANTES-Induced Invasion of Th17 Cells Into Substantia Nigra Potentiates Dopaminergic Cell Loss in MPTP Mouse Model of Parkinson’s Disease. Neurobiol. Dis. 132, 104575. doi: 10.1016/J.Nbd.2019.104575
Dyar, K. A., Hubert, M. J., Mir, A. A., Ciciliot, S., Lutter, D., Greulich, F., et al. (2018). Transcriptional Programming of Lipid and Amino Acid Metabolism by the Skeletal Muscle Circadian Clock. PloS Biol. 16, e2005886. doi: 10.1371/journal.pbio.2005886
Early, J. O., Menon, D., Wyse, C. A., Cervantes-Silva, M. P., Zaslona, Z., Carroll, R. G., et al. (2018). Circadian Clock Protein BMAL1 Regulates IL-1beta in Macrophages via NRF2. Proc. Natl. Acad. Sci. U. S. A. 115, E8460. doi: 10.1073/pnas.1800431115
Edgar, R. S., Green, E. W., Zhao, Y. W., van Ooijen, G., Olmedo, M., Qin, X. M., et al. (2012). Peroxiredoxins Are Conserved Markers of Circadian Rhythms. Nature 485, 459. doi: 10.1038/nature11088
Edwards, L. M., Murray, A. J., Holloway, C. J., Carter, E. E., Kemp, G. J., Codreanu, I., et al. (2011). Short-Term Consumption of a High-Fat Diet Impairs Whole-Body Efficiency and Cognitive Function in Sedentary Men. FASEB J. 25, 1088. doi: 10.1096/fj.10-171983
Eide, E. J., Woolf, M. F., Kang, H., Woolf, P., Hurst, W., Camacho, F., et al. (2005). Control of Mammalian Circadian Rhythm by CKI Epsilon-Regulated Proteasome-Mediated PER2 Degradation. Mol. Cell Biol. 25, 2795. doi: 10.1128/Mcb.25.7.2795-2807.2005
Everts, B., Amiel, E., Huang, S. C. C., Smith, A. M., Chang, C. H., Lam, W. Y., et al. (2014). TLR-Driven Early Glycolytic Reprogramming via the Kinases TBK1-IKK Epsilon Supports the Anabolic Demands of Dendritic Cell Activation. Nat. Immunol. 15, 323. doi: 10.1038/ni.2833
Figueiro, M. G. (2017). Light, Sleep and Circadian Rhythms in Older Adults With Alzheimer’s Disease and Related Dementias. Neurodegener. Dis. Man. 7, 119. doi: 10.2217/nmt-2016-0060
Fleischhacker, A. S., Carter, E. L., Ragsdale, S. W. (2018). Redox Regulation of Heme Oxygenase-2 and the Transcription Factor, Rev-Erb, Through Heme Regulatory Motifs. Antioxid. Redox Signal. 29, 1841. doi: 10.1089/ars.2017.7368
Fonken, L. K., Frank, M. G., Kitt, M. M., Barrientos, R. M., Watkins, L. R., Maier, S. F. (2015). Microglia Inflammatory Responses Are Controlled by an Intrinsic Circadian Clock. Brain Behav. Immun. 45, 171. doi: 10.1016/j.bbi.2014.11.009
Fonken, L. K., Kitt, M. M., Gaudet, A. D., Barrientos, R. M., Watkins, L. R., Maier, S. F. (2016). Diminished Circadian Rhythms in Hippocampal Microglia may Contribute to Age-Related Neuroinflammatory Sensitization. Neurobiol. Aging 47, 102. doi: 10.1016/j.neurobiolaging.2016.07.019
Fukuzumi, M., Shinomiya, H., Shimizu, Y., Ohishi, F., Utsumi, S. (1996). Endotoxin-Induced Enhancement of Glucose Influx Into Murine Peritoneal Macrophages via GLUT1. Infect. Immun. 64, 108. doi: 10.1128/Iai.64.1.108-112.1996
Gabriel, B. M., Zierath, J. R. (2019). Circadian Rhythms and Exercise - Re-Setting the Clock in Metabolic Disease. Nat. Rev. Endocrinol. 15, 197. doi: 10.1038/s41574-018-0150-x
Gagnidze, K., Hajdarovic, K. H., Moskalenko, M., Karatsoreos, I. N., McEwen, B. S., Bulloch, K. (2016). Nuclear Receptor REV-ERB Alpha Mediates Circadian Sensitivity to Mortality in Murine Vesicular Stomatitis Virus-Induced Encephalitis. Proc. Natl. Acad. Sci. U. S. A. 113, 5730. doi: 10.1073/pnas.1520489113
Gallego, M., Virshup, D. M. (2007). Post-Translational Modifications Regulate the Ticking of the Circadian Clock. Nat. Rev. Mol. Cell Bio. 8, 139. doi: 10.1038/nrm2106
Gao, Y. Q., Vidal-Itriago, A., Kalsbeek, M. J., Layritz, C., Garcia-Caceres, C., Tom, R. Z., et al. (2017). Lipoprotein Lipase Maintains Microglial Innate Immunity in Obesity. Cell Rep. 20, 3034. doi: 10.1016/j.celrep.2017.09.008
Gascoyne, D. M., Long, E., Veiga-Fernandes, H., de Boer, J., Williams, O., Seddon, B., et al. (2009). The Basic Leucine Zipper Transcription Factor E4BP4 Is Essential for Natural Killer Cell Development. Nat. Immunol. 10, 1118. doi: 10.1038/ni.1787
Gekakis, N., Staknis, D., Nguyen, H. B., Davis, F. C., Wilsbacher, L. D., King, D. P., et al. (1998). Role of the CLOCK Protein in the Mammalian Circadian Mechanism. Science 280, 1564. doi: 10.1126/science.280.5369.1564
Geltink, R. I. K., Kyle, R. L., Pearce, E. L. (2018). Unraveling the Complex Interplay Between T Cell Metabolism and Function. Annu. Rev. Immunol. 36, 461. doi: 10.1146/annurev-immunol-042617-053019
Gerstner, J. R., Yin, J. C. P. (2010). Circadian Rhythms and Memory Formation. Nat. Rev. Neurosci. 11, 577. doi: 10.1038/nrn2881
Gibbs, J. E., Blaikley, J., Beesley, S., Matthews, L., Simpson, K. D., Boyce, S. H., et al. (2012). The Nuclear Receptor REV-ERB Alpha Mediates Circadian Regulation of Innate Immunity Through Selective Regulation of Inflammatory Cytokines. Proc. Natl. Acad. Sci. U. S. A. 109, 582. doi: 10.1073/pnas.1106750109
Gnocchi, D., Bruscalupi, G. (2017). Circadian Rhythms and Hormonal Homeostasis: Pathophysiological Implications. Biology-Basel 6 (1), 10. doi: 10.3390/Biology6010010
Griffett, K., Burris, T. P. (2013). The Mammalian Clock and Chronopharmacology. Bioorg. Med. Chem. Lett. 23, 1929. doi: 10.1016/j.bmcl.2013.02.015
Griffin, P., Dimitry, J. M., Sheehan, P. W., Lananna, B. V., Guo, C., Robinette, M. L., et al. (2019). Circadian Clock Protein Rev-Erba Regulates Neuroinflammation. Proc. Natl. Acad. Sci. U. S. A. 116, 5102. doi: 10.1073/pnas.1812405116
Griffin, P., Dimitry, J. M., Sheehan, P. W., Lananna, B. V., Guo, C., Robinette, M. L., et al. (2019). Circadian Clock Protein Rev-Erbalpha Regulates Neuroinflammation. Proc. Natl. Acad. Sci. U. S. A. 116, 5102. doi: 10.1073/pnas.1812405116
Griffin, P., Sheehan, P. W., Dimitry, J. M., Guo, C., Kanan, M. F., Lee, J., et al. (2020). REV-ERBalpha Mediates Complement Expression and Diurnal Regulation of Microglial Synaptic Phagocytosis. Elife 9, e58765. doi: 10.7554/eLife.58765
Guo, D. K., Zhu, Y., Sun, H. Y., Xu, X. Y., Zhang, S., Hao, Z. B., et al. (2019). Pharmacological Activation of REV-ERBalpha Represses LPS-Induced Microglial Activation Through the NF-kappaB Pathway. Acta Pharmacol. Sin. 40, 26. doi: 10.1038/s41401-018-0064-0
Halberg, F., Johnson, E. A., Brown, B. W., Bittner, J. J. (1960). Susceptibility Rhythm to E. Coli Endotoxin and Bioassay. Proc. Soc. Exp. Biol. Med. 103, 142. doi: 10.3181/00379727-103-25439
Haque, S. N., Booreddy, S. R., Welsh, D. K. (2019). Effects of BMAL1 Manipulation on the Brain’s Master Circadian Clock and Behavior. Yale J. Biol. Med. 92, 251.
Hasegawa, S., Fukushima, H., Hosoda, H., Serita, T., Ishikawa, R., Rokukawa, T., et al. (2019). Hippocampal Clock Regulates Memory Retrieval via Dopamine and PKA-Induced GluA1 Phosphorylation. Nat. Commun. 10, 5766. doi: 10.1038/s41467-019-13554-y10.1038/s41467-019-13554-y[pii
Hastings, M. H., Maywood, E. S., Brancaccio, M. (2018). Generation of Circadian Rhythms in the Suprachiasmatic Nucleus. Nat. Rev. Neurosci. 19, 453. doi: 10.1038/s41583-018-0026-z
Hatanaka, F., Matsubara, C., Myung, J., Yoritaka, T., Kamimura, N., Tsutsumi, S., et al. (2010). Genome-Wide Profiling of the Core Clock Protein BMAL1 Targets Reveals a Strict Relationship With Metabolism. Mol. Cell Biol. 30, 5636. doi: 10.1128/MCB.00781-10
Hawkes, C. A., McLaurin, J. (2009). Selective Targeting of Perivascular Macrophages for Clearance of Beta-Amyloid in Cerebral Amyloid Angiopathy. Proc. Natl. Acad. Sci. U. S. A. 106, 1261. doi: 10.1073/pnas.0805453106
Hayashi, Y., Koyanagi, S., Kusunose, N., Okada, R., Wu, Z., Tozaki-Saitoh, H., et al. (2013). The Intrinsic Microglial Molecular Clock Controls Synaptic Strength via the Circadian Expression of Cathepsin S. Sci. Rep. 3, 2744. doi: 10.1038/srep02744
Hemmers, S., Rudensky, A. Y. (2015). The Cell-Intrinsic Circadian Clock Is Dispensable for Lymphocyte Differentiation and Function. Cell Rep. 11, 1339. doi: 10.1016/j.celrep.2015.04.058
Heneka, M. T., Carson, M. J., El Khoury, J., Landreth, G. E., Brosseron, F., Feinstein, D. L., et al. (2015). Neuroinflammation in Alzheimer’s Disease. Lancet Neurol. 14, 388. doi: 10.1016/S1474-4422(15)70016-5
Herzog, E. D., Hermanstyne, T., Smyllie, N. J., Hastings, M. H. (2017). Regulating the Suprachiasmatic Nucleus (SCN) Circadian Clockwork: Interplay Between Cell-Autonomous and Circuit-Level Mechanisms. Csh Perspect. Biol. 9 (1), a027706. doi: 10.1101/cshperspect.a027706
Heyward, F. D., Walton, R. G., Carle, M. S., Coleman, M. A., Garvey, W. T., Sweatt, J. D. (2012). Adult Mice Maintained on a High-Fat Diet Exhibit Object Location Memory Deficits and Reduced Hippocampal SIRT1 Gene Expression. Neurobiol. Learn. Memory 98, 25. doi: 10.1016/j.nlm.2012.04.005
Hickman, S., Izzy, S., Sen, P., Morsett, L., El Khoury, J. (2018). Microglia in Neurodegeneration. Nat. Neurosci. 21, 1359. doi: 10.1038/s41593-018-0242-x
Holloway, C. J., Cochlin, L. E., Emmanuel, Y., Murray, A., Codreanu, I., Edwards, L. M., et al. (2011). A High-Fat Diet Impairs Cardiac High-Energy Phosphate Metabolism and Cognitive Function in Healthy Human Subjects. Am. J. Clin. Nutr. 93, 748. doi: 10.3945/ajcn.110.002758
Hood, S., Amir, S. (2017). Neurodegeneration and the Circadian Clock. Front. Aging Neurosci. 9, 170. doi: 10.3389/Fnagi.2017.00170
Hood, S., Amir, S. (2017). The Aging Clock: Circadian Rhythms and Later Life. J. Clin. Invest. 127, 437. doi: 10.1172/JCI90328
Huo, M. Y., Huang, Y. H., Qu, D., Zhang, H. S., Wong, W. T., Chawla, A., et al. (2017). Myeloid Bmal1 Deletion Increases Monocyte Recruitment and Worsens Atherosclerosis. FASEB J. 31, 1097. doi: 10.1096/fj.201601030R
Ivanov, I. I., McKenzie, B. S., Zhou, L., Tadokoro, C. E., Lepelley, A., Lafaille, J. J., et al. (2006). The Orphan Nuclear Receptor RORgammat Directs the Differentiation Program of Proinflammatory IL-17+ T Helper Cells. Cell 126, 1121. doi: 10.1016/j.cell.2006.07.035
Ivan, D. C., Walthert, S., Berve, K., Steudler, J., Locatelli, G. (2020). Dwellers and Trespassers: Mononuclear Phagocytes at the Borders of the Central Nervous System. Front. Immunol. 11, 609921. doi: 10.3389/fimmu.2020.609921
Jasinska, M., Grzegorczyk, A., Woznicka, O., Jasek, E., Kossut, M., Barbacka-Surowiak, G., et al. (2015). Circadian Rhythmicity of Synapses in Mouse Somatosensory Cortex. Eur. J. Neurosci. 42, 2585. doi: 10.1111/ejn.13045
Jha, P. K., Challet, E., Kalsbeek, A. (2015). Circadian Rhythms in Glucose and Lipid Metabolism in Nocturnal and Diurnal Mammals. Mol. Cell. Endocrinol. 418, 74. doi: 10.1016/j.mce.2015.01.024
Jha, A. K., Huang, S. C. C., Sergushichev, A., Lampropoulou, V., Ivanova, Y., Loginicheva, E., et al. (2015). Network Integration of Parallel Metabolic and Transcriptional Data Reveals Metabolic Modules That Regulate Macrophage Polarization. Immunity 42, 419. doi: 10.1016/j.immuni.2015.02.005
Journiac, N., Jolly, S., Jarvis, C., Gautheron, V., Rogard, M., Trembleau, A., et al. (2009). The Nuclear Receptor ROR Alpha Exerts a Bi-Directional Regulation of IL-6 in Resting and Reactive Astrocytes. Proc. Natl. Acad. Sci. U. S. A. 106, 21365. doi: 10.1073/pnas.0911782106
Kalsbeek, A., van der Spek, R., Lei, J., Endert, E., Buijs, R. M., Fliers, E. (2012). Circadian Rhythms in the Hypothalamo-Pituitary-Adrenal (HPA) Axis. Mol. Cell. Endocrinol. 349, 20. doi: 10.1016/j.mce.2011.06.042
Kashiwada, M., Cassel, S. L., Colgan, J. D., Rothman, P. B. (2011). NFIL3/E4BP4 Controls Type 2 T Helper Cell Cytokine Expression. EMBO J. 30, 2071. doi: 10.1038/emboj.2011.111emboj2011111[pii
Kashiwada, M., Levy, D. M., McKeag, L., Murray, K., Schroder, A. J., Canfield, S. M., et al. (2010). IL-4-Induced Transcription Factor NFIL3/E4BP4 Controls IgE Class Switching. Proc. Natl. Acad. Sci. U. S. A. 107, 821. doi: 10.1073/pnas.0909235107
Kashiwada, M., Pham, N. L. L., Pewe, L. L., Harty, J. T., Rothman, P. B. (2011). NFIL3/E4BP4 Is a Key Transcription Factor for CD8 Alpha(+) Dendritic Cell Development. Blood 117, 6193. doi: 10.1182/blood-2010-07-295873
Katan, M., Moon, Y. P., Paik, M. C., Sacco, R. L., Wright, C. B., Elkind, M. S. (2013). Infectious Burden and Cognitive Function: The Northern Manhattan Study. Neurology 80, 1209. doi: 10.1212/WNL.0b013e3182896e7980/13/1209
Kawai, M., Green, C. B., Lecka-Czernik, B., Douris, N., Gilbert, M. R., Kojima, S., et al. (2010). A Circadian-Regulated Gene, Nocturnin, Promotes Adipogenesis by Stimulating PPAR-Gamma Nuclear Translocation. Proc. Natl. Acad. Sci. U. S. A. 107, 10508. doi: 10.1073/pnas.1000788107
Keller, M., Mazuch, J., Abraham, U., Eom, G. D., Herzog, E. D., Volk, H. D., et al. (2009). A Circadian Clock in Macrophages Controls Inflammatory Immune Responses. Proc. Natl. Acad. Sci. U. S. A. 106, 21407. doi: 10.1073/pnas.0906361106
Kelly, B., O’Neill, L. A. J. (2015). Metabolic Reprogramming in Macrophages and Dendritic Cells in Innate Immunity. Cell Res. 25, 771. doi: 10.1038/cr.2015.68
Kempuraj, D., Thangavel, R., Selvakumar, G. P., Zaheer, S., Ahmed, M. E., Raikwar, S. P., et al. (2017). Brain and Peripheral Atypical Inflammatory Mediators Potentiate Neuroinflammation and Neurodegeneration. Front. Cell. Neurosci. 11, 216. doi: 10.3389/Fncel.2017.00216
King, E., O’Brien, J. T., Donaghy, P., Morris, C., Barnett, N., Olsen, K., et al. (2018). Peripheral Inflammation in Prodromal Alzheimer’s and Lewy Body Dementias. J. Neurol. Neurosur. Ps. 89, 339. doi: 10.1136/jnnp-2017-317134
Kitazawa, M., Oddo, S., Yamasaki, T. R., Green, K. N., LaFerla, F. M. (2005). Lipopolysaccharide-Induced Inflammation Exacerbates Tau Pathology by a Cyclin-Dependent Kinase 5-Mediated Pathway in a Transgenic Model of Alzheimer’s Disease. J. Neurosci. 25, 8843. doi: 10.1523/Jneurosci.2868-05.2005
Kitchen, G. B., Cunningham, P. S., Poolman, T. M., Iqbal, M., Maidstone, R., Baxter, M., et al. (2020). The Clock Gene Bmal1 Inhibits Macrophage Motility, Phagocytosis, and Impairs Defense Against Pneumonia. Proc. Natl. Acad. Sci. U. S. A. 117 (3), 1543–1551. doi: 10.1073/pnas.1915932117
Kohsaka, A. (2007). Bass J. A Sense of Time: How Molecular Clocks Organize Metabolism. Trends Endocrin. Met. 18, 4. doi: 10.1016/j.tem.2006.11.005
Kohsaka, A., Laposky, A. D., Ramsey, K. M., Estrada, C., Joshu, C., Kobayashi, Y., et al. (2007). High-Fat Diet Disrupts Behavioral and Molecular Circadian Rhythms in Mice. Cell Metab. 6, 414. doi: 10.1016/j.cmet.2007.09.006
Kojetin, D. J., Burris, T. P. (2014). REV-ERB and ROR Nuclear Receptors as Drug Targets. Nat. Rev. Drug Discov. 13, 197. doi: 10.1038/nrd4100
Korn, T., Bettelli, E., Oukka, M., Kuchroo, V. K. (2009). IL-17 and Th17 Cells. Annu. Rev. Immunol. 27, 485. doi: 10.1146/annurev.immunol.021908.132710
Koronowski, K. B., Sassone-Corsi, P. (2021). Communicating Clocks Shape Circadian Homeostasis. Science 371 (6530), eabd0951. doi: 10.1126/science.abd0951371/6530/eabd0951
Kortekaas, R., Leenders, K. L., van Oostrom, J. C. H., Vaalburg, W., Bart, J., Willemsen, A. T. M., et al. (2005). Blood-Brain Barrier Dysfunction in Parkinsonian Midbrain In Vivo. Ann. Neurol. 57, 176. doi: 10.1002/ana.20369
Ko, C. H., Takahashi, J. S. (2006). Molecular Components of the Mammalian Circadian Clock. Hum. Mol. Genet. 15, R271. doi: 10.1093/hmg/ddl207
Kress, G. J., Liao, F., Dimitry, J., Cedeno, M. R., FitzGerald, G. A., Holtzman, D. M., et al. (2018). Regulation of Amyloid-Beta Dynamics and Pathology by the Circadian Clock. J. Exp. Med. 215, 1059. doi: 10.1084/jem.20172347
Krzeptowski, W., Hess, G., Pyza, E. (2018). Circadian Plasticity in the Brain of Insects and Rodents. Front. Neural Circuit. 12, 32. doi: 10.3389/Fncir.2018.00032
Labzin, L. I., Heneka, M. T., Latz, E. (2018). Innate Immunity and Neurodegeneration. Annu. Rev. Med. 69, 437. doi: 10.1146/annurev-med-050715-104343
Laflamme, N., Rivest, S. (1999). Effects of Systemic Immunogenic Insults and Circulating Proinflammatory Cytokines on the Transcription of the Inhibitory Factor kappaB Alpha Within Specific Cellular Populations of the Rat Brain. J. Neurochem. 73, 309. doi: 10.1046/j.1471-4159.1999.0730309.x
Lai, K. S. P., Liu, C. S., Rau, A., Lanctot, K. L., Kohler, C. A., Pakosh, M., et al. (2017). Peripheral Inflammatory Markers in Alzheimer’s Disease: A Systematic Review and Meta-Analysis of 175 Studies. J. Neurol. Neurosur. Ps. 88, 876. doi: 10.1136/jnnp-2017-316201
Lamia, K. A., Sachdeva, U. M., DiTacchio, L., Williams, E. C., Alvarez, J. G., Egan, D. F., et al. (2009). AMPK Regulates the Circadian Clock by Cryptochrome Phosphorylation and Degradation. Science 326, 437. doi: 10.1126/science.1172156
Lananna, B. V., Nadarajah, C. J., Izumo, M., Cedeno, M. R., Xiong, D. D., Dimitry, J., et al. (2018). Cell-Autonomous Regulation of Astrocyte Activation by the Circadian Clock Protein Bmal1. Cell Rep. 25, 1. doi: 10.1016/j.celrep.2018.09.015
Laurent, C., Dorothee, G., Hunot, S., Martin, E., Monnet, Y., Duchamp, M., et al. (2017). Hippocampal T Cell Infiltration Promotes Neuroinflammation and Cognitive Decline in a Mouse Model of Tauopathy. Brain 140, 184. doi: 10.1093/brain/aww270
Lauretti, E., Di Meco, A., Merali, S., Pratico, D. (2017). Circadian Rhythm Dysfunction: A Novel Environmental Risk Factor for Parkinson’s Disease. Mol. Psychiatr. 22, 280. doi: 10.1038/mp.2016.47
Lee, J., Kim, D. E., Griffin, P., Sheehan, P. W., Kim, D. H., Musiek, E. S., et al. (2020). Inhibition of REV-ERBs Stimulates Microglial Amyloid-Beta Clearance and Reduces Amyloid Plaque Deposition in the 5XFAD Mouse Model of Alzheimer’s Disease. Aging Cell. 19 (2), e13078. doi: 10.1111/acel.13078
Leng, Y., Musiek, E. S., Hu, K., Cappuccio, F. P., Yaffe, K. (2019). Association Between Circadian Rhythms and Neurodegenerative Diseases. Lancet Neurol. 18, 307. doi: 10.1016/S1474-4422(18)30461-7
Liddelow, S. A., Guttenplan, K. A., Larke, L. E. C., Bennett, F. C., Bohlen, C. J., Schirmer, L., et al. (2017). Neurotoxic Reactive Astrocytes Are Induced by Activated Microglia. Nature 541, 481. doi: 10.1038/nature21029
Liu, M., Bing, G. Y. (2011). Lipopolysaccharide Animal Models for Parkinson’s Disease. Parkinsons Dis.-Us. 2011, 327089. doi: 10.4061/2011/327089
Liu, W. W., Wei, S. Z., Huang, G. D., Liu, L. B., Gu, C., Shen, Y., et al. (2020). BMAL1 Regulation of Microglia-Mediated Neuroinflammation in MPTP-Induced Parkinson’s Disease Mouse Model. FASEB J. 34, 6570. doi: 10.1096/fj.201901565RR
Loizides-Mangold, U., Perrin, L., Vandereycken, B., Betts, J. A., Walhin, J. P., Templeman, I., et al. (2017). Lipidomics Reveals Diurnal Lipid Oscillations in Human Skeletal Muscle Persisting in Cellular Myotubes Cultured In Vitro. Proc. Natl. Acad. Sci. U. S. A. 114, E8565. doi: 10.1073/pnas.1705821114
Maleki, A. F., Rivest, S. (2019). Innate Immune Cells: Monocytes, Monocyte-Derived Macrophages and Microglia as Therapeutic Targets for Alzheimer’s Disease and Multiple Sclerosis. Front. Cell. Neurosci. 13, 355. doi: 10.3389/Fncel.2019.00355
Male, V., Nisoli, I., Gascoyne, D. M., Brady, H. J. M. (2012). E4BP4: An Unexpected Player in the Immune Response. Trends Immunol. 33, 98. doi: 10.1016/j.it.2011.10.002
Man, K., Loudon, A., Chawla, A. (2016). Immunity Around the Clock. Science 354, 999. doi: 10.1126/science.aah4966
Manoogian, E. N. C., Panda, S. (2017). Circadian Rhythms, Time-Restricted Feeding, and Healthy Aging. Ageing Res. Rev. 39, 59. doi: 10.1016/j.arr.2016.12.006
Marcheva, B., Ramsey, K. M., Buhr, E. D., Kobayashi, Y., Su, H., Ko, C. H., et al. (2010). Disruption of the Clock Components CLOCK and BMAL1 Leads to Hypoinsulinaemia and Diabetes. Nature 466, 627. doi: 10.1038/nature09253
Marcheva, B., Ramsey, K. M., Peek, C. B., Affinati, A., Maury, E., Bass, J. (2013). Circadian Clocks and Metabolism. Handb. Exp. Pharmacol. 127–155. doi: 10.1007/978-3-642-25950-0_6
Marpegan, L., Leone, M. J., Katz, M. E., Sobrero, P. M., Bekinstein, T. A., Golombek, D. A. (2009). Diurnal Variation in Endotoxin-Induced Mortality in Mice: Correlation With Proinflammatory Factors. Chronobiol. Int. 26, 1430. doi: 10.3109/07420520903408358
Medzhitov, R. (2008). Origin and Physiological Roles of Inflammation. Nature 454, 428. doi: 10.1038/nature07201
Mendes-Jorge, L., Ramos, D., Luppo, M., Llombart, C., Alexandre-Pires, G., Nacher, V., et al. (2009). Scavenger Function of Resident Autofluorescent Perivascular Macrophages and Their Contribution to the Maintenance of the Blood-Retinal Barrier. Invest. Ophth. Vis. Sci. 50, 5997. doi: 10.1167/iovs.09-3515
Meng, L., Shen, L., Ji, H. F. (2019). Impact of Infection on Risk of Parkinson’s Disease: A Quantitative Assessment of Case-Control and Cohort Studies. J. Neurovirol. 25, 221. doi: 10.1007/s13365-018-0707-4
Michalek, R. D., Gerriets, V. A., Jacobs, S. R., Macintyre, A. N., MacIver, N. J., Mason, E. F., et al. (2011). Cutting Edge: Distinct Glycolytic and Lipid Oxidative Metabolic Programs Are Essential for Effector and Regulatory CD4(+) T Cell Subsets. J. Immunol. 186, 3299. doi: 10.4049/jimmunol.1003613
Michaud, J. P., Bellavance, M. A., Prefontaine, P., Rivest, S. (2013). Real-Time In Vivo Imaging Reveals the Ability of Monocytes to Clear Vascular Amyloid Beta. Cell Rep. 5, 646. doi: 10.1016/j.celrep.2013.10.010
Moharrami, N. N., Tande, E. B., Ryan, L., Espevik, T., Boyartchuk, V. (2018). ROR Alpha Controls Inflammatory State of Human Macrophages. PloS One 13. doi: 10.1371/journal.pone.0207374
Mohawk, J. A., Green, C. B., Takahashi, J. S. (2012). Central and Peripheral Circadian Clocks in Mammals. Annu. Rev. Neurosci. 35, 445. doi: 10.1146/annurev-neuro-060909-153128
Motomura, Y., Kitamura, H., Hijikata, A., Matsunaga, Y., Matsumoto, K., Inoue, H., et al. (2011). The Transcription Factor E4BP4 Regulates the Production of IL-10 and IL-13 in CD4+ T Cells. Nat. Immunol. 12, 450. doi: 10.1038/ni.2020ni.2020[pii
Muddapu, V. R., Dharshini, S. A. P., Chakravarthy, V. S., Gromiha, M. M. (2020). Neurodegenerative Diseases - Is Metabolic Deficiency the Root Cause? Front. Neurosci.-Switz. 14, 213. doi: 10.3389/Fnins.2020.00213
Mure, L. S., Le, H. D., Benegiamo, G., Chang, M. W., Rios, L., Jillani, N., et al. (2018). Diurnal Transcriptome Atlas of a Primate Across Major Neural and Peripheral Tissues. Science 359, 1232. doi: 10.1126/science.aao0318
Murray, A. J., Knight, N. S., Cochlin, L. E., McAleese, S., Deacon, R. M. J., Rawlins, J. N. P., et al. (2009). Deterioration of Physical Performance and Cognitive Function in Rats With Short-Term High-Fat Feeding. FASEB J. 23, 4353. doi: 10.1096/fj.09-139691
Musiek, E. S., Bhimasani, M., Zangrilli, M. A., Morris, J. C., Holtzman, D. M., Ju, Y. E. (2018). Circadian Rest-Activity Pattern Changes in Aging and Preclinical Alzheimer Disease. JAMA Neurol. 75, 582. doi: 10.1001/jamaneurol.2017.4719
Musiek, E. S., Lim, M. M., Yang, G. R., Bauer, A. Q., Oi, L., Lee, Y., et al. (2013). Circadian Clock Proteins Regulate Neuronal Redox Homeostasis and Neurodegeneration. J. Clin. Invest. 123, 5389. doi: 10.1172/JCI70317
Nakazato, R., Hotta, S., Yamada, D., Kou, M., Nakamura, S., Takahata, Y., et al. (2017). The Intrinsic Microglial Clock System Regulates Interleukin-6 Expression. Glia 65, 198. doi: 10.1002/glia.23087
Nakazato, R., Kawabe, K., Yamada, D., Ikeno, S., Mieda, M., Shimba, S., et al. (2017). Disruption of Bmal1 Impairs Blood-Brain Barrier Integrity via Pericyte Dysfunction. J. Neurosci. 37, 10052. doi: 10.1523/Jneurosci.3639-16.2017
Narasimamurthy, R., Hatori, M., Nayak, S. K., Liu, F., Panda, S., Verma, I. M. (2012). Circadian Clock Protein Cryptochrome Regulates the Expression of Proinflammatory Cytokines. Proc. Natl. Acad. Sci. U. S. A. 109, 12662. doi: 10.1073/pnas.1209965109
Nguyen, K. D., Fentress, S. J., Qiu, Y. F., Yun, K. R., Cox, J. S., Chawla, A. (2013). Circadian Gene Bmal1 Regulates Diurnal Oscillations of Ly6C(hi) Inflammatory Monocytes. Science 341, 1483. doi: 10.1126/science.1240636
Ohno, T., Onishi, Y., Ishida, N. (2007). A Novel E4BP4 Element Drives Circadian Expression of Mperiod2. Nucleic Acids Res. 35, 648. doi: 10.1093/nar/gkl868
Oksanen, M., Lehtonen, S., Jaronen, M., Goldsteins, G., Hamalainen, R. H., Koistinaho, J. (2019). Astrocyte Alterations in Neurodegenerative Pathologies and Their Modeling in Human Induced Pluripotent Stem Cell Platforms. Cell Mol. Life Sci. 76, 2739. doi: 10.1007/s00018-019-03111-7
O’Neill, L. A. J., Kishton, R. J. (2016). Rathmell J. A Guide to Immunometabolism for Immunologists. Nat. Rev. Immunol. 16, 553. doi: 10.1038/nri.2016.70
O’Neill, L. A. J., Pearce, E. J. (2016). Immunometabolism Governs Dendritic Cell and Macrophage Function. J. Exp. Med. 213, 15. doi: 10.1084/jem.20151570
O’Neill, J. S., Reddy, A. B. (2011). Circadian Clocks in Human Red Blood Cells. Nature 469, 498. doi: 10.1038/nature09702
Orihuela, R., McPherson, C. A., Harry, G. J. (2016). Microglial M1/M2 Polarization and Metabolic States. Brit. J. Pharmacol. 173, 649. doi: 10.1111/bph.13139
Palmieri, E. M., Menga, A., Lebrun, A., Hooper, D. C., Butterfield, D. A., Mazzone, M., et al. (2017). Blockade of Glutamine Synthetase Enhances Inflammatory Response in Microglial Cells. Antioxid. Redox Sign. 26, 351. doi: 10.1089/ars.2016.6715
Pariollaud, M., Gibbs, J. E., Hopwood, T. W., Brown, S., Begley, N., Vonslow, R., et al. (2018). Circadian Clock Component REV-ERB Alpha Controls Homeostatic Regulation of Pulmonary Inflammation. J. Clin. Invest. 128, 2281. doi: 10.1172/JCI93910
Parodi-Rullan, R., Sone, J. Y., Fossati, S. (2019). Endothelial Mitochondrial Dysfunction in Cerebral Amyloid Angiopathy and Alzheimer’s Disease. J. Alzheimers Dis. 72, 1019. doi: 10.3233/Jad-190357
Patsoukis, N., Bardhan, K., Chatterjee, P., Sari, D., Liu, B. L., Bell, L. N., et al. (2015). PD-1 Alters T-Cell Metabolic Reprogramming by Inhibiting Glycolysis and Promoting Lipolysis and Fatty Acid Oxidation. Nat. Commun. 6, 6692. doi: 10.1038/Ncomms7692
Pedditzi, E., Peters, R., Beckett, N. (2016). The Risk of Overweight/Obesity in Mid-Life and Late Life for the Development of Dementia: A Systematic Review and Meta-Analysis of Longitudinal Studies. Age Ageing 45, 737. doi: 10.1093/ageing/afw095
Perez-Cruz, C., Simon, M., Flugge, G., Fuchs, E., Czeh, B. (2009). Diurnal Rhythm and Stress Regulate Dendritic Architecture and Spine Density of Pyramidal Neurons in the Rat Infralimbic Cortex. Behav. Brain Res. 205, 406. doi: 10.1016/j.bbr.2009.07.021
Perry, V. H., Cunningham, C., Holmes, C. (2007). Systemic Infections and Inflammation Affect Chronic Neurodegeneration. Nat. Rev. Immunol. 7, 161. doi: 10.1038/nri2015
Phatnani, H., Maniatis, T. (2015). Astrocytes in Neurodegenerative Disease. Csh Perspect. Biol. 7 (11), e0207374. doi: 10.1101/cshperspect.a020628
Pistell, P. J., Morrison, C. D., Gupta, S., Knight, A. G., Keller, J. N., Ingram, D. K., et al. (2010). Cognitive Impairment Following High Fat Diet Consumption Is Associated With Brain Inflammation. J. Neuroimmunol. 219, 25. doi: 10.1016/j.jneuroim.2009.11.010
Pivovarova, O., Jurchott, K., Rudovich, N., Hornemann, S., Ye, L., Mockel, S., et al. (2015). Changes of Dietary Fat and Carbohydrate Content Alter Central and Peripheral Clock in Humans. J. Clin. Endocr. Metab. 100, 2291. doi: 10.1210/jc.2014-3868
Posokhova, E. N., Khoshchenko, O. M., Chasovskikh, M. I., Pivovarova, E. N., Dushkin, M. I. (2008). Lipid Synthesis in Macrophages During Inflammation In Vivo: Effect of Agonists of Peroxisome Proliferator Activated Receptors Alpha and Gamma and of Retinoid X Receptors. Biochemistry-Moscow+ 73, 296. doi: 10.1134/S0006297908030097
Puntener, U., Booth, S. G., Perry, V. H., Teeling, J. L. (2012). Long-Term Impact of Systemic Bacterial Infection on the Cerebral Vasculature and Microglia. J. Neuroinflamm. 9, 146. doi: 10.1186/1742-2094-9-146
Qin, L. Y., Wu, X. F., Block, M. L., Liu, Y. X., Breese, G. R., Hong, J. S., et al. (2007). Systemic LPS Causes Chronic Neuroinflammation and Progressive Neurodegeneration. Glia 55, 453. doi: 10.1002/glia.20467
Raghuram, S., Stayrook, K. R., Huang, P. X., Rogers, P. M., Nosie, A. K., McClure, D. B., et al. (2007). Identification of Heme as the Ligand for the Orphan Nuclear Receptors REV-ERB Alpha and REV-ERB Beta. Nat. Struct. Mol. Biol. 14, 1207. doi: 10.1038/nsmb1344
Rahman, S. A., Castanon-Cervantes, O., Scheer, F. A., Shea, S. A., Czeisler, C. A., Davidson, A. J., et al. (2015). Endogenous Circadian Regulation of Pro-Inflammatory Cytokines and Chemokines in the Presence of Bacterial Lipopolysaccharide in Humans. Brain Behav. Immun. 47, 4. doi: 10.1016/j.bbi.2014.11.003
Reischl, S., Kramer, A. (2011). Kinases and Phosphatases in the Mammalian Circadian Clock. FEBS Lett. 585, 1393. doi: 10.1016/j.febslet.2011.02.038
Reppert, S. M., Weaver, D. R. (2001). Molecular Analysis of Mammalian Circadian Rhythms. Annu. Rev. Physiol. 63, 647. doi: 10.1146/annurev.physiol.63.1.647
Reppert, S. M., Weaver, D. R. (2002). Coordination of Circadian Timing in Mammals. Nature 418, 935. doi: 10.1038/nature00965
Reynolds, A. D., Stone, D. K., Hutter, J. A. L., Benner, E. J., Mosley, R. L., Gendelman, H. E. (2010). Regulatory T Cells Attenuate Th17 Cell-Mediated Nigrostriatal Dopaminergic Neurodegeneration in a Model of Parkinson’s Disease. J. Immunol. 184, 2261. doi: 10.4049/jimmunol.0901852
Rey, G., Reddy, A. B. (2015). Interplay Between Cellular Redox Oscillations and Circadian Clocks. Diabetes Obes. Metab. 17, 55. doi: 10.1111/dom.12519
Riemersma-van Der Lek, R. F., Swaab, D. F., Twisk, J., Hol, E. M., Hoogendijk, W. J. G., Van Someren, E. J. W. (2008). Effect of Bright Light and Melatonin on Cognitive and Noncognitive Function in Elderly Residents of Group Care Facilities - A Randomized Controlled Trial. Jama-J. Am. Med. Assoc. 299, 2642. doi: 10.1001/jama.299.22.2642
Ripperger, J. R. A., Schibler, U. (2006). Rhythmic CLOCK-BMAL1 Binding to Multiple E-Box Motifs Drives Circadian Dbp Transcription and Chromatin Transitions. Nat. Genet. 38, 369. doi: 10.1038/ng1738
Robles, M. S., Humphrey, S. J., Mann, M. (2017). Phosphorylation Is a Central Mechanism for Circadian Control of Metabolism and Physiology. Cell Metab. 25, 118. doi: 10.1016/j.cmet.2016.10.004
Rodriguez-Prados, J. C., Traves, P. G., Cuenca, J., Rico, D., Aragones, J., Martin-Sanz, P., et al. (2010). Substrate Fate in Activated Macrophages: A Comparison Between Innate, Classic, and Alternative Activation. J. Immunol. 185, 605. doi: 10.4049/jimmunol.0901698
Ruan, W., Yuan, X. Y., Eltzschig, H. K. (2021). Circadian Rhythm as a Therapeutic Target. Nat. Rev. Drug Discov. 20 (4), 287–307. doi: 10.1038/s41573-020-00109-w
Rudic, R. D., McNamara, P., Curtis, A. M., Boston, R. C., Panda, S., Hogenesch, J. B., et al. (2004). BMAL1 and CLOCK, Two Essential Components of the Circadian Clock, Are Involved in Glucose Homeostasis. PloS Biol. 2, e377. doi: 10.1371/journal.pbio.0020377
Rutter, J., Reick, M., Wu, L. C., McKnight, S. L. (2001). Regulation of Clock and NPAS2 DNA Binding by the Redox State of NAD Cofactors. Science 293, 510. doi: 10.1126/science.10606981060698[pii
Sadacca, L. A., Lamia, K. A., deLemos, A. S., Blum, B., Weitz, C. J. (2011). An Intrinsic Circadian Clock of the Pancreas Is Required for Normal Insulin Release and Glucose Homeostasis in Mice. Diabetologia 54, 120. doi: 10.1007/s00125-010-1920-8
Sagare, A. P., Bell, R. D., Zhao, Z., Ma, Q. Y., Winkler, E. A., Ramanathan, A., et al. (2013). Pericyte Loss Influences Alzheimer-Like Neurodegeneration in Mice. Nat. Commun. 4, 2932. doi: 10.1038/Ncomms3932
Sahar, S., Zocchi, L., Kinoshita, C., Borrelli, E., Sassone-Corsi, P. (2010). Regulation of BMAL1 Protein Stability and Circadian Function by GSK3 Beta-Mediated Phosphorylation. PloS One 5 (1), e8561. doi: 10.1371/journal.pone.0008561
Saini, R., Jaskolski, M., Davis, S. J. (2019). Circadian Oscillator Proteins Across the Kingdoms of Life: Structural Aspects. BMC Biol. 17 (1), 13. doi: 10.1186/s12915-018-0623-3
Sanada, K., Okano, T., Fukada, Y. (2002). Mitogen-Activated Protein Kinase Phosphorylates and Negatively Regulates Basic Helix-Loop-Helix-PAS Transcription Factor BMAL1. J. Biol. Chem. 277, 267. doi: 10.1074/jbc.M107850200
Sato, T. K., Panda, S., Miraglia, L. J., Reyes, T. M., Rudic, R. D., McNamara, P., et al. (2004). A Functional Genomics Strategy Reveals Rora as a Component of the Mammalian Circadian Clock. Neuron 43, 527. doi: 10.1016/j.neuron.2004.07.018
Sato, S., Sakurai, T., Ogasawara, J., Takahashi, M., Izawa, T., Imaizumi, K., et al. (2014). A Circadian Clock Gene, Rev-Erbalpha, Modulates the Inflammatory Function of Macrophages Through the Negative Regulation of Ccl2 Expression. J. Immunol. 192, 407. doi: 10.4049/jimmunol.1301982jimmunol.1301982[pii
Scheiermann, C., Kunisaki, Y., Frenette, P. S. (2013). Circadian Control of the Immune System. Nat. Rev. Immunol. 13, 190. doi: 10.1038/nri3386
Schiaffino, S., Blaauw, B., Dyar, K. A. (2016). The Functional Significance of the Skeletal Muscle Clock: Lessons From Bmal1 Knockout Models. Skelet. Muscle 6, 33. doi: 10.1186/s13395-016-0107-5107
Schousboe, A., Scafidi, S., Bak, L. K., Waagepetersen, H. S., McKenna, M. C. (2014). Glutamate Metabolism in the Brain Focusing on Astrocytes. Adv. Neurobiol. 11, 13. doi: 10.1007/978-3-319-08894-5_2
Semmler, A., Widmann, C. N., Okulla, T., Urbach, H., Kaiser, M., Widman, G., et al. (2013). Persistent Cognitive Impairment, Hippocampal Atrophy and EEG Changes in Sepsis Survivors. J. Neurol. Neurosur. Ps. 84, 62. doi: 10.1136/jnnp-2012-302883
Shackelford, P. G., Feigin, R. D. (1973). Periodicity of Susceptibility to Pneumococcal Infection: Influence of Light and Adrenocortical Secretions. Science 182:285. doi: 10.1126/science.182.4109.285
Sharma, M., Boytard, L., Hadi, T., Koelwyn, G., Simon, R., Ouimet, M., et al. (2020). Enhanced Glycolysis and HIF-1 Alpha Activation in Adipose Tissue Macrophages Sustains Local and Systemic Interleukin-1 Beta Production in Obesity. Sci. Rep. 10, 5555. doi: 10.1038/S41598-020-62272-9
Sheng, J. G., Bora, S. H., Xu, G., Borchelt, D. R., Price, D. L., Koliatsos, V. E. (2003). Lipopolysaccharide-Induced-Neuroinflammation Increases Intracellular Accumulation of Amyloid Precursor Protein and Amyloid Beta Peptide in APPswe Transgenic Mice. Neurobiol. Dis. 14, 133. doi: 10.1016/s0969-9961(03)00069-x
Shi, G. S., Xie, P. C., Qu, Z. P., Zhang, Z. H., Dong, Z., An, Y., et al. (2016). Distinct Roles of HDAC3 in the Core Circadian Negative Feedback Loop Are Critical for Clock Function. Cell Rep. 14, 823. doi: 10.1016/j.celrep.2015.12.076
Silver, A. C., Arjona, A., Walker, W. E., Fikrig, E. (2012). The Circadian Clock Controls Toll-Like Receptor 9-Mediated Innate and Adaptive Immunity. Immunity 36, 251. doi: 10.1016/j.immuni.2011.12.017
Sly, L. M., Krzesicki, R. F., Brashler, J. R., Buhl, A. E., McKinley, D. D., Carter, D. B., et al. (2001). Endogenous Brain Cytokine mRNA and Inflammatory Responses to Lipopolysaccharide Are Elevated in the Tg2576 Transgenic Mouse Model of Alzheimer’s Disease. Brain Res. Bull. 56, 581. doi: 10.1016/S0361-9230(01)00730-4
Snider, K. H., Dziema, H., Aten, S., Loeser, J., Norona, F. E., Hoyt, K., et al. (2016). Modulation of Learning and Memory by the Targeted Deletion of the Circadian Clock Gene Bmal1 in Forebrain Circuits. Behav. Brain Res. 308, 222. doi: 10.1016/j.bbr.2016.04.027
Sonninen, T. M., Hamalainen, R. H., Koskuvi, M., Oksanen, M., Shakirzyanova, A., Wojciechowski, S., et al. (2020). Metabolic Alterations in Parkinson’s Disease Astrocytes. Sci. Rep. 10, 14474. doi: 10.1038/S41598-020-71329-8
St-Amour, I., Bosoi, C. R., Pare, I., Doss, P. M. I. A., Rangachari, M., Hebert, S. S., et al. (2019). Peripheral Adaptive Immunity of the Triple Transgenic Mouse Model of Alzheimer’s Disease. J. Neuroinflamm. 16 (1), 3. doi: 10.1186/S12974-018-1380-5
Stenvers, D. J., Scheer, F. A. J. L., Schrauwen, P., la Fleur, S. E., Kalsbeek, A. (2019). Circadian Clocks and Insulin Resistance. Nat. Rev. Endocrinol. 15, 75. doi: 10.1038/s41574-018-0122-1
Sundar, I. K., Sellix, M. T., Rahman, I. (2018). Redox Regulation of Circadian Molecular Clock in Chronic Airway Diseases. Free Radic. Biol. Med. 119, 121. doi: 10.1016/j.freeradbiomed.2017.10.383
Sussman, W., Stevenson, M., Mowdawalla, C., Mota, S., Ragolia, L., Pan, X. Y. (2019). BMAL1 Controls Glucose Uptake Through Paired-Homeodomain Transcription Factor 4 in Differentiated Caco-2 Cells. Am. J. Physiol.-Cell Ph. 317, C492. doi: 10.1152/ajpcell.00058.2019
Suzuki, A., Stern, S. A., Bozdagi, O., Huntley, G. W., Walker, R. H., Magistretti, P. J., et al. (2011). Astrocyte-Neuron Lactate Transport Is Required for Long-Term Memory Formation. Cell 144, 810. doi: 10.1016/j.cell.2011.02.018
Tejera, D., Mercan, D., Sanchez-Caro, J. M., Hanan, M., Greenberg, D., Soreq, H., et al. (2019). Systemic Inflammation Impairs Microglial A Beta Clearance Through NLRP3 Inflammasome. EMBO J. 38, e101064. doi: 10.15252/embj.2018101064
Timmons, G. A., O’Siorain, J. R., Kennedy, O. D., Curtis, A. M., Early, J. O. (2020). Innate Rhythms: Clocks at the Center of Monocyte and Macrophage Function. Front. Immunol. 11, 1743. doi: 10.3389/Fimmu.2020.01743
Tracey, K. J. (2002). The Inflammatory Reflex. Nature 420, 853. doi: 10.1038/nature01321nature01321[pii
Trager, U., Tabrizi, S. J. (2013). Peripheral Inflammation in Neurodegeneration. J. Mol. Med. 91, 673. doi: 10.1007/s00109-013-1026-0
Uriz-Huarte, A., Date, A., Ang, H., Ali, S., Brady, H. J. M., Fuchter, M. J. (2020). The Transcriptional Repressor REV-ERB as a Novel Target for Disease. Bioorg. Med. Chem. Lett. 30, 127395. doi: 10.1016/j.bmcl.2020.127395
Videnovic, A., Klerman, E. B., Wang, W., Marconi, A., Kuhta, T., Zee, P. C. (2017). Timed Light Therapy for Sleep and Daytime Sleepiness Associated With Parkinson Disease A Randomized Clinical Trial. JAMA Neurol. 74, 411. doi: 10.1001/jamaneurol.2016.5192
Vijayan, V., Pradhan, P., Braud, L., Fuchs, H. R., Gueler, F., Motterlini, R., et al. (2019). Human and Murine Macrophages Exhibit Differential Metabolic Responses to Lipopolysaccharide - A Divergent Role for Glycolysis. Redox Biol. 22, 101147. doi: 10.1016/j.redox.2019.101147
Wang, G. Z., Hickey, S. L., Shi, L., Huang, H. C., Nakashe, P., Koike, N., et al. (2015). Cycling Transcriptional Networks Optimize Energy Utilization on a Genome Scale. Cell Rep. 13, 1868. doi: 10.1016/j.celrep.2015.10.043
Wang, X. L., Kooijman, S., Gao, Y., Tzeplaeff, L., Cosquer, B., Milanova, I., et al. (2021). Microglia-Specific Knock-Down of Bmal1 Improves Memory and Protects Mice From High Fat Diet-Induced Obesity. Mol. Psychiatry. doi: 10.1038/s41380-021-01169-z
Wang, S., Li, F., Lin, Y. K., Wu, B. J. (2020). Targeting REV-ERB Alpha for Therapeutic Purposes: Promises and Challenges. Theranostics 10, 4168. doi: 10.7150/thno.43834
Wang, L., Pavlou, S., Du, X., Bhuckory, M., Xu, H., Chen, M. (2019). Glucose Transporter 1 Critically Controls Microglial Activation Through Facilitating Glycolysis. Mol. Neurodegener. 14:2. doi: 10.1186/s13024-019-0305-9
Wang, X. L., Wolff, S. E. C., Korpel, N., Milanova, I., Sandu, C., Rensen, P. C. N., et al. (2020). Deficiency of the Circadian Clock Gene Bmal1 Reduces Microglial Immunometabolism. Front. Immunol. 11, 586399. doi: 10.3389/Fimmu.2020.586399
Wardlaw, S. M., Phan, T. X., Saraf, A., Chen, X. M., Storm, D. R. (2014). Genetic Disruption of the Core Circadian Clock Impairs Hippocampus-Dependent Memory. Learn. Memory 21, 417. doi: 10.1101/lm.035451.114
Weber, B., Barros, L. F. (2015). The Astrocyte: Powerhouse and Recycling Center. Csh Perspect. Biol. 7, a020396. doi: 10.1101/cshperspect.a020396
Weberpals, M., Hermes, M., Hermann, S., Kummer, M. P., Terwel, D., Semmler, A., et al. (2009). NOS2 Gene Deficiency Protects From Sepsis-Induced Long-Term Cognitive Deficits. J. Neurosci. 29, 14177. doi: 10.1523/Jneurosci.3238-09.2009
Widmann, C. N., Heneka, M. T. (2014). Long-Term Cerebral Consequences of Sepsis. Lancet Neurol. 13, 630. doi: 10.1016/S1474-4422(14)70017-1
Wolff, S. E. C., Wang, X. L., Jiao, H., Sun, J., Kalsbeek, A., Yi, C. X., et al. (2020). The Effect of Rev-Erbalpha Agonist SR9011 on the Immune Response and Cell Metabolism of Microglia. Front. Immunol. 11, 550145. doi: 10.3389/fimmu.2020.550145
Yamajuku, D., Shibata, Y., Kitazawa, M., Katakura, T., Urata, H., Kojima, T., et al. (2011). Cellular DBP and E4BP4 Proteins Are Critical for Determining the Period Length of the Circadian Oscillator. FEBS Lett. 585, 2217. doi: 10.1016/j.febslet.2011.05.038
Yang, G. R., Chen, L. H., Grant, G. R., Paschos, G., Song, W. L., Musiek, E. S., et al. (2016). Timing of Expression of the Core Clock Gene Bmal1 Influences Its Effects on Aging and Survival. Sci. Transl. Med. 8 (324), 324ra16. doi: 10.1126/scitranslmed.aad3305
Yang, L. K., Guo, C. F., Zhu, J., Feng, Y., Chen, W. L., Feng, Z. Z., et al. (2017). Increased Levels of Pro-Inflammatory and Anti-Inflammatory Cellular Responses in Parkinson’s Disease Patients: Search for a Disease Indicator. Med. Sci. Monitor. 23, 2972. doi: 10.12659/Msm.904240
Yang, X. O., Pappu, B. P., Nurieva, R., Akimzhanov, A., Kang, H. S., Chung, Y., et al. (2008). T Helper 17 Lineage Differentiation Is Programmed by Orphan Nuclear Receptors ROR Alpha and ROR Gamma. Immunity 28, 29. doi: 10.1016/j.immuni.2007.11.016
Yang, G., Zhang, J., Jiang, T., Monslow, J., Tang, S. Y., Todd, L., et al. (2020). Bmal1 Deletion in Myeloid Cells Attenuates Atherosclerotic Lesion Development and Restrains Abdominal Aortic Aneurysm Formation in Hyperlipidemic Mice. Arterioscler. Thromb. Vasc. Biol. 40, 1523. doi: 10.1161/ATVBAHA.120.314318
Yan, L. J., Xiao, M., Chen, R., Cai, Z. (2013). Metabolic Dysfunction of Astrocyte: An Initiating Factor in Beta-Amyloid Pathology? Aging Neurodegener. 1, 7.
Yoo, I. D., Park, M. W., Cha, H. W., Yoon, S., Boonpraman, N., Yi, S. S., et al. (2020). Elevated CLOCK and BMAL1 Contribute to the Impairment of Aerobic Glycolysis From Astrocytes in Alzheimer’s Disease. Int. J. Mol. Sci. 21, 7862. doi: 10.3390/Ijms21217862
Yu, X. F., Rollins, D., Ruhn, K. A., Stubblefield, J. J., Green, C. B., Kashiwada, M., et al. (2013). T(H)17 Cell Differentiation Is Regulated by the Circadian Clock. Science 342, 727. doi: 10.1126/science.1243884
Zenaro, E., Pietronigro, E., Della Bianca, V., Piacentino, G., Marongiu, L., Budui, S., et al. (2015). Neutrophils Promote Alzheimer’s Disease-Like Pathology and Cognitive Decline via LFA-1 Integrin. Nat. Med. 21, 880. doi: 10.1038/nm.3913
Zhang, J., Ke, K. F., Liu, Z., Qiu, Y. H., Peng, Y. P. (2013). Th17 Cell-Mediated Neuroinflammation Is Involved in Neurodegeneration of Abeta1-42-Induced Alzheimer’s Disease Model Rats. PloS One 8, e75786. doi: 10.1371/journal.pone.0075786
Zhang, R., Lahens, N. F., Ballance, H. I., Hughes, M. E., Hogenesch, J. B. (2014). A Circadian Gene Expression Atlas in Mammals: Implications for Biology and Medicine. Proc. Natl. Acad. Sci. U. S. A. 111, 16219. doi: 10.1073/pnas.1408886111
Zhang, S. L., Lahens, N. F., Yue, Z., Arnold, D. M., Pakstis, P. P., Schwarz, J. E., et al. (2021). A Circadian Clock Regulates Efflux by the Blood-Brain Barrier in Mice and Human Cells. Nat. Commun. 12, 617. doi: 10.1038/s41467-020-20795-9
Zhang, E. E., Liu, Y., Dentin, R., Pongsawakul, P. Y., Liu, A. C., Hirota, T., et al. (2010). Cryptochrome Mediates Circadian Regulation of cAMP Signaling and Hepatic Gluconeogenesis. Nat. Med. 16, 1152. doi: 10.1038/nm.2214
Keywords: circadian clock, immune response, systemic inflammation, neurodegeneration, cellular metabolism
Citation: Wang X-L and Li L (2021) Circadian Clock Regulates Inflammation and the Development of Neurodegeneration. Front. Cell. Infect. Microbiol. 11:696554. doi: 10.3389/fcimb.2021.696554
Received: 19 April 2021; Accepted: 18 August 2021;
Published: 14 September 2021.
Edited by:
Erik Steven Musiek, Saint Louis University, United StatesReviewed by:
Louise Madeleine Ince, University of Texas at Austin, United StatesChristopher S. Colwell, University of California, Los Angeles, United States
Copyright © 2021 Wang and Li. This is an open-access article distributed under the terms of the Creative Commons Attribution License (CC BY). The use, distribution or reproduction in other forums is permitted, provided the original author(s) and the copyright owner(s) are credited and that the original publication in this journal is cited, in accordance with accepted academic practice. No use, distribution or reproduction is permitted which does not comply with these terms.
*Correspondence: Lianjian Li, bGlsaWFuamlhbjExMTVAc2luYS5jb20=
†ORCID: Xiao-Lan Wang, orcid.org/0000-0002-3549-3680