- 1Centre for Urological Biology, Department of Renal Medicine, University College London, London, United Kingdom
- 2Department of Bioengineering, Charles Stark Draper Laboratory, Inc., Cambridge, MA, United States
Urinary tract infections (UTIs) are among the most common infectious diseases worldwide but are significantly understudied. Uropathogenic E. coli (UPEC) accounts for a significant proportion of UTI, but a large number of other species can infect the urinary tract, each of which will have unique host-pathogen interactions with the bladder environment. Given the substantial economic burden of UTI and its increasing antibiotic resistance, there is an urgent need to better understand UTI pathophysiology – especially its tendency to relapse and recur. Most models developed to date use murine infection; few human-relevant models exist. Of these, the majority of in vitro UTI models have utilized cells in static culture, but UTI needs to be studied in the context of the unique aspects of the bladder’s biophysical environment (e.g., tissue architecture, urine, fluid flow, and stretch). In this review, we summarize the complexities of recurrent UTI, critically assess current infection models and discuss potential improvements. More advanced human cell-based in vitro models have the potential to enable a better understanding of the etiology of UTI disease and to provide a complementary platform alongside animals for drug screening and the search for better treatments.
Introduction: Urinary Tract Infection – A Globally Important Disease in Need of Better Human-Based Model Systems
UTIs are among the most common infectious diseases worldwide, causing approximately 150 million cases per annum (Stamm and Norrby, 2001), but are significantly understudied (Losada et al., 2016). Although much more common in women, UTI can affect men and children (Foxman, 2010) and after respiratory infection, it is the most common infectious disease of our ageing population (Rowe and Juthani-Mehta, 2013). In care homes, UTI is the most common infectious disease of all (Rowe and Juthani-Mehta, 2013). What’s more, UTI is one of the most frequent healthcare-acquired infections, and it is particularly problematic for people with multiple sclerosis, spinal injuries, pregnant women and patients requiring urinary catheters (Foxman, 2003). As a result, UTI imposes a substantial economic and healthcare burden (Foxman, 2010). In addition, because of the vast number of individual treatments required, the World Health Organization has described antibiotic resistance in uropathogens as a key pressure point in the growing global antimicrobial resistance crisis (WHO, 2014). As a number of the bacteria involved also infect different bodily niches, resistance can affect treatment of other diseases, including those requiring surgery. There is therefore an urgent need to better understand UTI pathophysiology, so that alternatives to antibiotics can be developed.
Uropathogenic E. coli (UPEC) accounts for about 80% of community-acquired UTI in otherwise healthy people (Foxman, 2003), but a large number of other bacterial species can cause a UTI (Foxman, 2010). One of the biggest concerns about UTI is its tendency to recur. While there have been advances in new treatments and vaccines (reviewed in O’Brien et al., 2016), antibiotics remain the mainstay of therapy. Even despite treatment, up to 25% of women experience a relapse within six months (Foxman et al., 2000); in one large study, 2% had six or more episodes within a two-year period (Laupland et al., 2007). Some patients experience recurrent UTI for years, necessitating prophylactic antibiotics that only increase the risk of antimicrobial resistance (Selekman et al., 2018). Although the pathophysiology of UTI – primarily of UPEC-associated disease – has been extensively researched in animal models (Hunstad and Justice, 2010), relatively little is understood about the infection cycle in humans. In particular, the behavior of E. coli pathogens in the human bladder lumen is understudied, and the situation for other bacterial species is even hazier.
To develop new treatment strategies for UTI, it is imperative that researchers understand the pathophysiology. Although careful studies in UTI patients have been greatly illustrative, as with most diseases, there are limits to what can be understood in this context. The study of UTI with small animal models and cell culture systems, while also incredibly valuable, still have limitations. Further progress in improving the lot of UTI patients will require advances in human-based model systems.
In this review, we will discuss the proposed mechanisms of UTI pathogenesis and recurrence, and highlight the unknowns that remain. Next, we will discuss the animal models used to study UTI, followed by the main in vitro human-cell-based models, examining their strengths and limitations. Then we will review the key features of human bladder physiology that ideally would be present in an improved in vitro model system. Finally, we will discuss state-of-the-art platforms and solutions to these challenges, and conclude with our perspectives on where the field is headed.
The Mechanisms of Recurrent UTI: A Multi-Faceted Problem
Recurrent urinary tract infection (rUTI) represents a massive burden for the economy, for healthcare systems and for the patients who suffer from them. Several hypotheses for the mechanism of rUTI have emerged over the past decades – which are not necessarily mutually exclusive. Indeed, different – and likely multiple – scenarios will exist within different patients and across different species of uropathogen, as shown recently (Thänert et al., 2019). We argue that better human-based infection models will be required to elucidate them fully.
Microbiota, Dysbiosis, and Distal Reservoirs
One of the most long-standing theories for recurrence of UTI is that the gastrointestinal tract functions as a reservoir for uropathogens, which are repeatedly reintroduced into the urinary tract via contamination of the periurethral surface and subsequent retrograde ascension. Indeed, common uropathogens are abundant as gut commensals, and there are many papers supporting this mechanism (reviewed recently in (Jones-Freeman et al., 2021). In agreement, recent studies with patients experiencing rUTI revealed that re-infection was usually preceded by a bloom of uropathogens in the intestine (Thänert et al., 2019), and that gut abundance of Escherichia and Enterococcus served as an independent risk factor for late problematic urine colonization in kidney transplant recipients, with genomic strain analysis supporting the association (Magruder et al., 2019). Also in support of this hypothesis, molecules designed to selectively deplete uropathogenic E. coli in the gut also decrease the incidence of UTIs in mice [e.g. (Spaulding et al., 2017)].
The gut is not the only proximal niche that might harbor uropathogens, however. A crosstalk between the vaginal and urinary microbiomes has been reported based on both clinical correlations and experimental models, and the data strongly suggest that this interconnection affects the recurrence of UTIs (Komesu et al., 2020; Lewis and Gilbert, 2020). Moreover, the now well-established observation that healthy urinary tracts are not sterile and that their microbiota is distinct from those of chronic UTI patients has highlighted the potential relationship between bladder dysbiosis and rUTI (Khasriya et al., 2013; Hilt et al., 2014; Neugent et al., 2020). Dysbiosis, defined as an imbalance in the natural microbial community, may even affect several host protective mechanisms, such as those mediated by commensals. Also, alteration of the urinary microbiota through the introduction of native microbes from either the gut or the vagina may potentiate the recurrence of UTI. For example, the vaginal commensal Gardnerella vaginalis was reported in the urinary tract of both human males and females (Fairley and Birch, 1983; Lam et al., 1988). More recently, the inoculation of Gardnerella into the bladders of mice that had recovered from UPEC infection caused urothelium exfoliation and facilitated the exposure of intracellular UPEC reservoirs in the bladder (Gilbert et al., 2017). In addition, this exposure increased the severity of infection compared with control mice. Of note, a small clinical trial showed that the introduction of a probiotic Lactobacillus species capable of outcompeting uropathogens into the vagina of women with rUTI was able to modestly reduce the incidence of UTI (Stapleton et al., 2011). Reciprocally, commensal Lactobacillus in the vagina establish a low pH that is non-permissive to uropathogenic species such as E. coli; if these commensals are outcompeted, uropathogens can become the dominant vaginal species, which would facilitate their eventual transit to the urethra (Brannon et al., 2020; Lewis and Gilbert, 2020).
Antibiotics are another important factor affecting rUTI, not only those taken to treat UTI, but also other indications. Antibiotics favor the development and proliferation of multidrug-resistant organisms (either in the bladder or in the gut/vagina), as well as increase the availability of niches that are no longer inhabited by commensals. As a result, these niches can become dysbiotic and more easily colonized by more virulent and persistent bacteria (Chen et al., 2013).
Taken together, these various strands of evidence have boosted the search for probiotic approaches and other therapies focused on the modulation of microbiota in the UTI context. However, a human cell-based model that could accurately mimic the human urinary microbiota and its interactions with other microbiota would be a very helpful tool in this endeavor.
Bacterial Virulence and Bladder Reservoirs
Many strain-specific bacterial virulence factors may contribute to the recurrence of UTI, such as flagella/pili, adhesins, extracellular polysaccharides, lipopolysaccharides, toxins, ureases, proteases and iron-scavenging siderophores. These factors allow uropathogens to survive during long periods in a nutrient-limited habitat, helping them to adhere, colonize, damage and invade host cells, as well as to evade host defenses, ultimately increasing their persistence in the urinary tract. For instance, a common uropathogenic strategy is the formation of biofilms, either directly on the urothelial surface or on indwelling devices such as catheters (Jansen et al., 2004; Jacobsen and Shirtliff, 2011). This behavior has been reported in Klebsiella pneumoniae (Reid et al., 1992; Stahlhut et al., 2012), Pseudomonas aeruginosa (Ivanova et al., 2015; Saini et al., 2015), UPEC (Ferrières et al., 2007) and Enterococci (Sillanpää et al., 2010), and may even be facilitated by polymicrobial interactions during infection (Gaston et al., 2020). The biofilm provides an ideal physicochemical barrier that protects uropathogens from antimicrobial agents, host immunity and other stresses, allowing them to persist and reinfect the urinary tract. Furthermore, a subpopulation of bacterial cells in biofilms, the so-called persistors, are known to reversibly reduce their metabolic activity, adopting a dormant state that can evade host defenses as well as treatments that target active metabolic pathways or activities such as cell division (Wood et al., 2013). Another strategy is the adhesion of bacteria either to the host cell surface and/or the extracellular matrix (ECM), through the use of a vast arsenal of virulence factors. Among the most common are curli proteins (similar to amyloid fibers), which are major facilitators of UPEC colonization (Luna-Pineda et al., 2019). These proteins allow bacterial binding to ECM and serum proteins such as fibronectin, laminin and plasminogen, and are highly expressed among Enterobacteriaceae. P-type pilus and the FimH fimbrial adhesin (part of the Type-1 pilus) have also received attention due to their high binding affinity with urothelial receptors, being important players for UPEC colonization and/or invasion in several UTI models (Mulvey et al., 1998; Zhou et al., 2001; Bishop et al., 2007; Rosen et al., 2008; Feenstra et al., 2017). Therefore, a number of therapeutic approaches against rUTI target specific virulence factors, mainly blocking adhesion and/or biofilm formation (Liao et al., 2012; Flores-Mireles et al., 2014; Mike et al., 2016).
Another bacterial mechanism implicated in rUTI is the ability of bacteria to invade host urothelial cells and establish intracellular bacterial communities (IBCs). This was first discovered in the early 2000s in a UPEC mouse model of induced UTI, in which the bacteria subverted host defenses, invaded urothelial cells and formed IBCs that could later erupt and re-establish UTI (Anderson et al., 2003; Justice et al., 2004). In this exciting model, after invasion, bacteria rapidly multiply in the cytoplasm of superficial bladder epithelial cells, where they form “pods” that can expand, blister-like, into the lumen of the bladder as the community grows (Anderson et al., 2003). These communities are embedded in a biofilm-like matrix, which may confer a protective effect similar to that seen in more conventional biofilms. In other cases, UPEC may even invade deeper layers of the urothelium, remaining in a membrane-bound compartment with little to no metabolic activity. These “quiescent intracellular reservoirs” (QIR) can be latent for months in mice, and can be re-activated after the exfoliation of the upper urothelium cell layers (Mulvey et al., 2001; Mysorekar and Hultgren, 2006).
While IBCs have also been experimentally demonstrated in human cancer cell lines (e.g. (Bishop et al., 2007; González et al., 2020), nearly two decades on from the original IBC discovery in mice, surprisingly few papers have reported the existence of UPEC IBCs in patients. A handful show IBC using exfoliated urothelial cells from the urine of human UTI patients (Rosen et al., 2007; Robino et al., 2013; Robino et al., 2014; Cheng et al., 2016), although only some (Robino et al., 2013; Robino et al., 2014) used imaging resolution with sufficient discriminatory power to distinguish intracellular bacteria from those on the surface of these notoriously flat cells. To our knowledge, only one group has studied human biopsies and reported the existence of IBC and QIR (De Nisco et al., 2019). In contrast, in a recent study with a porcine model, which has a more similar urogenital anatomy, physiology and immune response to human compared with small-animal models, IBCs were not observed in the bladder after UPEC infection, although high loads of bacteria were detected after prolonged infection, some forming biofilm-like extracellular aggregates (Nielsen et al., 2019). Therefore, more studies on the role of IBC and QIR in rUTI in humans or human bladder model systems would be welcome.
Although intracellular lifestyle stages have been observed mainly in UPEC infection, other uropathogens, such as Klebsiella pneumoniae (Rosen et al., 2008), Staphylococcus saprophyticus (Szabados et al., 2008) and Salmonella enterica (Bishop et al., 2007) might also display them, at least in murine models and/or cell lines. More recently, Enterococcus faecalis was shown to reside inside urothelial cells shed from patients with chronic UTI (Horsley et al., 2013), and to invade a human organoid model (Horsley et al., 2018). It remains to be seen whether other uropathogens invade human cells, and how widespread the phenomenon is in patients.
Morphological Alterations
Recurrent infection may also be facilitated by the ability of some uropathogens to alter their morphology to evade the host immune system and recolonize naïve regions in the urinary tract more easily. One well-studied example is UPEC cell filamentation, a process which occurs when bacterial cells emerge from IBCs (Justice et al., 2006). This filamentous form confers resistance to phagocytic engulfment (Justice et al., 2006) and may provide enhanced adhesive properties (Andersen et al., 2012). Another example is Proteus mirabilis, which can increase flagellar density (Armbruster and Mobley, 2012). Recently, Mickiewicz et al. reported that UPEC may also acquire a reversible “L-form”, a cell-wall deficient phase that can evade antibiotic treatments targeting the bacterial cell wall. In both urine and a zebrafish model, UPEC could rapidly switch into this form; the L-form was also the most prevalent in urine from older rUTI patients (Mickiewicz et al., 2019).
Bacterial Resistance and Resilience
Antibiotic resistance is a key issue in recurrent UTI, and is the most well-studied mode of treatment failure generally (Ho et al., 2019; Yelin et al., 2019; Mattoo and Asmar, 2020). The overuse of antibiotics has positively selected for strains with specific genetic traits allowing them to survive and proliferate in the presence of a single or even a class of antimicrobial compounds. In addition, most of these bacteria have the necessary machinery for intra- and inter-species transmission (e.g. through horizontal gene transfer mechanisms), which can spread the selected traits rapidly and boost the generation of multidrug-resistant uropathogens (Ho et al., 2019; Mattoo and Asmar, 2020).
On the other hand, a much less well-understood phenomenon involves resilience behaviors that provide temporary antibiotic evasion in a sub-population of bacterial cells. The emergence of resilient bacteria in an overall susceptible bacterial population might therefore play an important role in the selection of fitter uropathogens and subsequent recurrence. Several genetic and non-genetic mechanisms might be involved in the development of resilient profiles, which can be generally categorized as tolerance, persistence and heteroresistance phenotypes (Carvalho et al., 2019).
One of the best studied bacterial resilient mechanisms involved in rUTI are biofilms, which provide tolerance to external stresses, such as antibiotic treatments and host defenses (Olsen, 2015). Bacteria in polymicrobial UTI also have the ability to protect one other from clinically relevant antibiotics through the increase of tolerant/resilient phenotypes in the bacterial community (de Vos et al., 2017). In contrast, the mechanisms of persistence and heteroresistance are still largely unexplored in the UTI context, as well as their impact in clinical settings. However, the development of bacterial subpopulations that are dormant/less metabolically active and/or display a heterogenous (more resistant) phenotype might be crucial for the success of a pathogenic community facing inconsistent and unexpected environmental challenges (Kussell et al., 2005; Carvalho et al., 2019). Although much remains to be learned about this interesting class of treatment failure, bacterial resilience may have a role in the development of chronic UTIs, while resistance may play a more prominent role in recurrent acute infections (Olsen, 2015). It is important to note that the host context is likely to influence bacterial resilience phenotypes, so studying this phenomenon in a human-cell (and one day, patient-specific) environment will be important.
Host Factors
Bacteria are not the only players to alter their properties during the host/pathogen interaction. Host urothelial cells can also change upon first infection, a phenomenon observed in experimental mouse models of chronic UPEC infection and in cell lines. These changes influence host cell gene expression, shape, size, growth and proliferation, and depending upon what is changed, may make the urothelium more resilient, or more susceptible, to re-infection (Mysorekar et al., 2009; Shin et al., 2011; O’Brien et al., 2016).
Other host factors, mainly immune-related, also play a prominent role in the chronicity of rUTI, as the recurrence of infection is frequently associated with a disturbed innate immune response and/or insufficient adaptive immunity (recently reviewed in (Lacerda Mariano and Ingersoll, 2020). The reasons behind these events are still unclear and largely unexplored due to the lack of knowledge about how the immune response is triggered and develops in human UTIs, which can differ significantly from the murine context. Indeed, up until recently it was assumed that adaptive immunity was not involved at all, due to early cumulative evidence from immune-deficient mouse models lacking interleukins, immunoglobulins and/or T lymphocytes, that could nevertheless resolve UTI as well as their wild-type counterparts or even be resistant to infection (Svanborg Edén et al., 1984; Ragnarsdóttir et al., 2008). A revision of this view has paved the way for the development of vaccines against UTI, some of which are showing promising results (Prattley et al., 2020).
It has been increasingly reported, mainly in children, that a genetic component might be involved, as polymorphisms and mutations in innate immunity-related genes can increase susceptibility to UTIs (Karoly et al., 2007; Tabel et al., 2007). Additionally, an impaired immune response (Thumbikat et al., 2006) as well as an exacerbated pro-inflammatory response to a primary infection (Hannan et al., 2014), might favor recurrence of infection. In fact, the activation of host immunity frequently leads to severe injuries in the urinary mucosa/urothelium, which become more prone to subsequent infections. In one way or another, this common suboptimal and non-sterilizing immune response may create the perfect environment for the occurrence of rUTIs in an experienced host.
Animal Models for Studying UTI
Brief History of Animals in UTI Research
Animals of many species were essential for biological scientific experiments as early as the late 19th century, furthering our understanding of physiology and human diseases. The earliest mention of establishing infection in animal bladders was in 1873, when Fels and Ritter inoculated canine bladders to induce cystitis using urethral ligation (Keyes, 1894). Many tried to induce infection in animals using pure cultures of microorganisms, but ligation or a wound to the bladder was always necessary to establish cystitis. In 1890, Schnitzler managed to induce cystitis in rabbits without ligation using ‘Urobacillus liquefaciens septicus’ [later defined as a member of the Bacillus cloacae group (Archer, 1931)]. Later, it was reported that ‘coliform bacillus’ could produce cystitis in rabbit kidneys and an inflammatory response in the bladder mucosa (Lepper, 1921). Animals continued to be important for UTI research, mainly rats and rabbits, until the first murine model of UTI was described in 1967, as an experimental infection model for pyelonephritis (Keane and Freedman, 1967).
Murine models eventually became established as a valuable tool to study UTI, a trend which continues to this day. Mice were considered superior to rats because they were slightly more relevant to humans, with a greater abundance of globoseries glycolipid receptors on urothelial cells for attachment (Hagberg et al., 1983). Similar to humans, mice do not have a natural vesicoureteral reflux, unlike other rodent models, so would be a more physiologically accurate model for pyelonephritis (Hvidberg et al., 2000). Mouse and human bladders also have highly conserved uroplakins, which aid type 1 fimbriae adherence and invasion by UPEC (Zhou et al., 2001). Recently, female C57BL/6 mice urothelial cells were analyzed and classified into eight clusters dependent on expression of cell-specific markers. A novel urothelial cell type expressing Plxna4 was discovered in the mouse bladder (Li et al., 2021) which is conserved in humans and may play a role in host immune response (Wen et al., 2010).
As mice do not naturally develop urinary infections, instillation techniques were developed. Hagberg et al. described the ascending, unobstructed UTI instillation in female CBA mice with E. coli (Hagberg et al., 1983) which has since been adapted for other uropathogens such as Proteus mirabilis and Enterococcus faecalis (Jones et al., 1990; Shankar et al., 2001). This instillation method helped replicate the attachment of human-derived UTI isolates to murine urothelial cells which was considered essential for understanding human infection. CBA mice were preferred due to better bacterial attachment and colonization; female CBA were favored as the anatomy of male mice presented challenges for urethral inoculation (Hagberg et al., 1983). Although UTI is more prevalent in females than males (Foxman, 2010), little is known about sex differences in UTI pathophysiology. To address this, a protocol for inducing UTI in male mice using transurethral catheterization allowed direct comparison between male and female C57B1/6 mice and their host response (Zychlinsky Scharff et al., 2017). However, all instillations via catheterization bypass the biology of ascending UTI via the urethra (Barber et al., 2016).
Many different mouse models have been used for UTI (Table 1). The mouse model offers systemic context and a diverse range of genetic variability, allowing researchers to test specific host factors and immune responses in transgenic and knockdown mutants (Barber et al., 2016). ‘Germ free’ murine models are also potentially an attractive option for modeling UTI and the gut microbiota/UTI axis, allowing exclusive colonization of certain pathogens or purposefully introduced commensal species. For immunological purposes, naturalizing mouse models can also be used to help translate research to humans (Graham, 2021). The immune profiles of laboratory mice are different from that of human as they have a low density of mature T cells (Beura et al., 2016) and lower LPS sensitivity which could lead to differences in pathogenesis and treatment responses in humans (Graham, 2021). However, germ-free mice are more expensive and need specialized equipment and training (Kennedy et al., 2018). To our knowledge, however, there have been no reports of naturalized mice used for UTI research.
Differences Between Mouse and Human Models
Although murine models remain incredibly valuable for UTI, they are expensive and labor-intensive to maintain and breed. This precludes their use for high-throughput analysis, particularly for drug screening and testing. Ethical guidelines and applications have also slowed down animal research, although scientists can navigate these obstacles with a little patience.
Aside from these logistical issues, there is a deeper concern that animal models do not always recapitulate the human environment well enough to predict how disease physiology works, nor how prospective treatments might behave in human patients (Herati and Wherry, 2018), especially as mice do not naturally acquire UTI. Accordingly, the use and accuracy of animal models is a frequent discussion point among UTI researchers, with Barber et al. reviewing their strengths and limitations (Barber et al., 2016). Since then, further questions about the accuracy of animal models, especially mice, have arisen.
Host Bladder Physiological Differences
Murine and human bladders differ in certain anatomical features and expression of biomarkers on the epithelial surface (Figure 1). The mouse urothelium is typically 3-4 cells thick, making it thinner compared with human, which is 5-7 cells thick. The difference is due to the number of intermediate cells (Khandelwal et al., 2009), which could create differences in physiology and microenvironment between the upper and lower intermediate cells. In addition, the basal cells of the human urothelium have a higher expression of cytokeratin (CK) 5, 13, 14 & 17 compared with mice, whereas human umbrella cells have a higher expression of CK7, 8, 18 & 20 (Laguna et al., 2006).
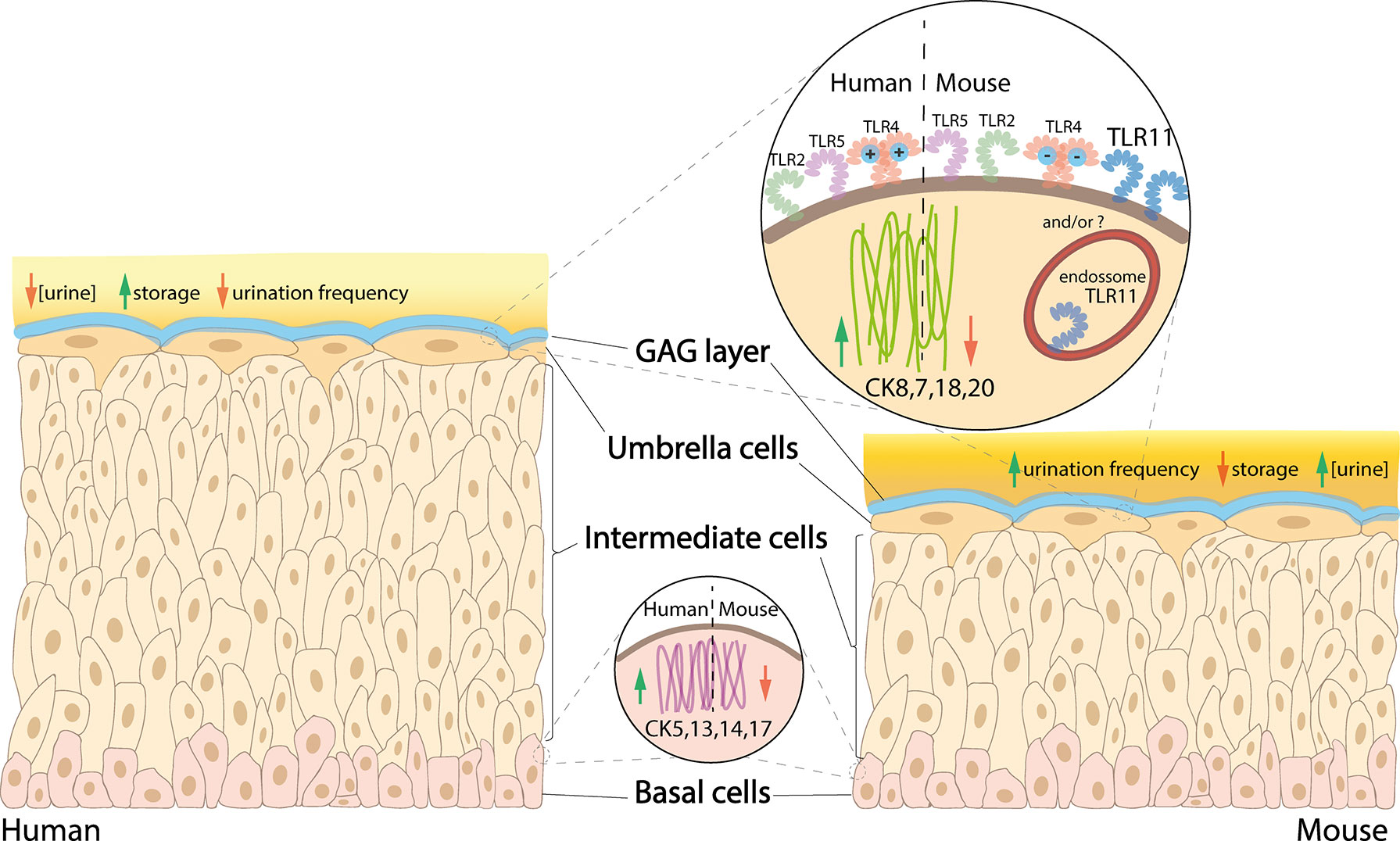
Figure 1 Comparison between human versus mouse urothelium. In human bladder, basal cells have higher expression of CK5, 13, 14 and 17, intermediate cells are stacked in 5-7 layers (vs 3-4 in mice), and umbrella cells have higher expression of CK8, 7, 18 and 20, as well as a more cationic MD-2 protein associated with the TLR4, while mice have a high expression of TLR11 (which is not present in humans). Urine is less concentrated in humans, which also have higher storage capacity and lower urination frequency compared with mice.
As the upper intermediate cells are more competent to differentiate into umbrella cells compared with the basal-like lower intermediate cells, there may be increased expression of umbrella cell cytokeratins within the upper intermediate cells compared with those below. Although studies are lacking, as mice only have 1 or 2 intermediate cell layers, it is possible they do not possess the full array of cell-specific biomarkers present in human. Studying their differences could help establish whether murine bladders can accurately recapitulate human UTI. Lui et al. found differences in CD markers in the different layers of the human urothelium, with CD271 in basal cells and CD227 in umbrella cells; however, there was no specific CD marker for intermediate cells (Liu et al., 2012). The analogous situation in murine urothelium has not been explored.
Another species difference involves the interstitial cells (IC), which are recently described specialized cells residing in the lamina propria and detrusor muscle associated with nerves (McCloskey, 2010). Yu et al. found a high similarity in single-cell types between human and mice bladders, but single-cell transcriptomic mapping highlighted two novel IC expression types specific to human bladders, namely ADRA2A+ and HRH2+. These ICs may play a role in allergic reactions and nerve conduction (Yu et al., 2019). Gavaert et al. also reported a difference between mouse and human IC, with human ICs manifesting increased contractile microfilaments, versus a fibroblast phenotype in murine ICs (Gevaert et al., 2017). The role of ICs in the host response during UTI is unknown but could inform new therapeutic targets.
Urodynamics and voiding patterns also are different between humans and mice. Both sexes of 10-week-old mice void more frequently than humans, ~10 times a day vs ~6 times a day (Chung and van Mastrigt, 2009; Aizawa et al., 2013), and also more frequently at night (Ito et al., 2017). Unlike animals over 3 kg, including humans, which possess a scalable urinary capacity and similar voiding durations, Yang et al. reported that mice scarcely store urine at all, which would affect the shape and stretching parameters of their bladders (Yang et al., 2014). Contraction of the detrusor muscle in mammalian bladders usually depends on activation of muscarinic-3 (M3) receptors, whereas mice use activation of muscarinic-2 (M2) receptors via an indirect mechanism (Ehlert et al., 2005; Zhou et al., 2010), which could be important for accurate disease modeling. Age may also play a role; e.g. differences were reported in voiding and storage with 12 week “mature” mice compared to “aged” 27-30 month C57BL/6 mice, with more severe bladder dysfunction in aged male mice compared with female. M3 receptor expression was downregulated in aged male mice, whereas β2-adrenoceptor was downregulated in aged females (Kamei et al., 2018). As aging is a risk factor in developing UTIs (Foxman, 2010) yet younger mice are mostly selected for UTI research, it is possible that youthful mouse models are inaccurate for modeling UTI in our aging population.
Host Pathogen Interactions and Immunological Differences
Humans and mice also differ in some innate immunological parameters. In UTI, the innate immune system is particularly important for host recognition of pathogen-associated molecular patterns (PAMPs) via pathogen recognition receptors (PRRs). PRRs recognize pathogens and help regulate a quick pro-inflammatory immune response, the mechanisms of which have been extensively reviewed (Takeuchi and Akira, 2010; Abraham and Miao, 2015). The predominant group of PRRs are Toll-like receptors (TLRs). Differences in TLR structure and function between humans and mice could influence how the innate immune system responds to a particular UTI pathogen, which could influence model accuracy.
There are 13 members of TLRs and between humans and mice; TLR1-9 are present in both, TLR10 is found in only in humans and TLR11-13, only in mice (Behzadi and Behzadi, 2016). In the urothelium, TLR2, 4, 5 and 11 (in mice) are the most competent TLRs against UTIs (Song and Abraham, 2008; Behzadi and Behzadi, 2016).
TLR4 is the most well-studied of the TLRs. TLR4 forms complexes with CD14 and MD-2 which help bind bacterial lipopolysaccharide (LPS) to initiate signaling for innate immune response (Vaure and Liu, 2014). LPS is displayed abundantly on the outside of Gram-negative bacteria such as UPEC, highlighting the importance of bacterial TLR4 interactions in the urinary tract. Despite TLR4 being present in both species, noticeable differences exist (reviewed by Vaure and Liu, 2014), with only a 57% similarity between their MD-2 proteins; this makes human MD-2 more cationic than mouse MD-2 which further affects the activation of TLR4 signaling (Vasl et al., 2009). The hypervariable region of TLR4, where MD-2 complexes with TLR4, has only 48% similarity between humans and mice, which again might lead to differences in how the host responds to infections. To date, the impact of these differences in TLR4 in human and mouse UTI has not been investigated.
TLR2, expressed in both species, has been shown to be a signaling receptor for bacterial peptidoglycan, important for recognition of Gram-positive bacteria (Takeuchi et al., 1999), and its ability to form heterodimer complexes with TLR1 and 6 broadens its spectrum to recognize other PAMPs (reviewed in Behzadi and Behzadi, 2016). TLR5 and 11 are similar in that they recognize UPEC PAMP flagellin (Hatai et al., 2016). As TLR11 is only expressed in mice, mice might have a higher capacity to recognize flagellin from UPEC, which should be kept in mind when studying this bacterial parameter.
Bacterial Differences
Bacterial phenotypes differ between mouse and human infections, likely influenced by differences in environment, bacterial development and growth. For example, the importance of some bacterial virulence factors seems to be completely different between human cell lines and animal models (Alamuri et al., 2010). A recent paper explored the gene expression of three UPEC strains isolated from cases of uncomplicated UTI; gene expression between human and mouse UTI was highly correlated, but 5.4% of analyzed genes were differentially regulated with 30 genes upregulated and 145 downregulated in human UTI (Frick-Cheng et al., 2020). Most of the downregulated genes were involved in anaerobic metabolism, so the authors hypothesized that the human bladder is more oxygenated than the murine. The presence and full effect of this physiological difference on bacterial phenotypes has not been explored.
Species differences in urine composition might also contribute to differences in bacterial behavior. Mouse urine has been shown to change the gene expression of UPEC when compared with human urine (Hagan et al., 2010). This study found that type 1 fimbrial genes – essential for bacterial adherence in murine models – were not expressed in 6 of 8 clinical isolates in human urine, suggesting that murine models impart a different expression phenotype. Mouse urine is more concentrated than that of larger mammals, which can affect UPEC biofilm production and cell morphology. FliC, a virulence factor encoding UPEC flagellin, was shown to be downregulated in mouse urine and upregulated in human urine (Snyder et al., 2004; Berry et al., 2009). On the other hand, filamentation, which is important for UPEC virulence, occurred in both highly concentrated human and mouse urine (Klein et al., 2015).
Murine models have been used to study antibiotic efficacy. Chockalingham et al. infected immunocompetent Balb/C mice with UPEC strain CFT073 to investigate resistance development against ampicillin, ciprofloxacin and fosfomycin, concluding that the mouse model was not suitable for studying resistance patterns, but could be useful for studying persistence (Chockalingam et al., 2019).
Other Animal Models for UTI
Porcine models have recently been mooted as an attractive species to model pathogenesis of UTI. They have more conserved homology and structural motifs to humans compared with mice, which may make it a more accurate model particularly for immunological studies (Dawson et al., 2017). Thus far pigs have been mainly used for research into pyelonephritis and renal damage, especially vesicoureteric reflux and renal scarring in infants and establishment of upper UTI (Coulthard et al., 2002). However, until recently pigs had not been used to model cystitis. In 2019, Nielsen et al. reported a UPEC model of infection using a clinical isolate UTI89 to sustain an infection for up to 23 days in female pigs (Nielsen et al., 2019). Interestingly, as mentioned above, no intracellular UPEC were seen as has been widely reported for UTI89 in mice. The porcine model, as a large mammal, shares similarities in urine density and anatomy with humans (Nielsen et al., 2019). However, large animal models are particularly expensive. What’s more, the scope for genetic manipulation in pigs has yet to match that of mice (Barber et al., 2016).
The nematode Caenorhabditis elegans has been used for in vivo infection studies that include various UTI pathogens (Lavigne et al., 2008; Szabados et al., 2013; Engelsöy et al., 2019). Very recently, Hashimoto et al. demonstrated that UPEC mutants with defective iron acquisition-related virulence factors were a significant factor in survival of C. elegans (Hashimoto et al., 2021). However, as a model, C. elegans has only been useful for survival assays and cannot be explored as a physiological model as it lacks a urinary system. Zebrafish, a popular model for real-time visualization of infections, also lack a urinary system, but has recently been used to determine in vivo L-form switching of UPEC and its role in recurrent UTI (Mickiewicz et al., 2019).
Non-human primate (NHP) models benefit from their high similarity with humans. To our knowledge there are no papers describing an NHP model for UTI (Chen et al., 2019), but they have been used in the past for UTI vaccine studies (reviewed in (O’Brien et al., 2016) and viral studies (reviewed in (Estes et al., 2018). However, their expense, ethical issues and specialized facilities pose significant drawbacks.
In summary, animal models have enabled crucial advances, but relevant human-related models are still needed to fully understand UTI in patients. These models should provide complementary valuable insights into the host-pathogen interactions and allow the development of more human-relevant therapeutic approaches.
In Vitro Human Cell-Based Models
The in vitro recapitulation of the human bladder environment and the reconstitution of the urothelium (or even the organ) is highly desirable because of the advantages such models present compared with animal models (see Section 3) and their potential for studying a wide range of conditions, from UTIs to bladder cancer, tissue regeneration/transplant and the effect of drugs and xenobiotics that contact the urinary tract. Three major strategies have been employed: i) cell monolayers, using commercially available cell lines, cells recovered from urine or derived from biopsies/explant cultures; ii) tissue/organ cultures from patients; and iii) 3D structures/organoids that mimic the urothelium after induced cell stratification and differentiation. An overview of the different bladder models used in UTI and non-UTI related research is presented in Tables 2, 3, respectively.
As human bladder cell lines are easy to maintain in 2D and to manipulate using standard techniques, cell monolayer culture remains one of the most attractive methodologies. There are also an increasing variety of well-established human bladder cell lines that can be purchased; e.g. the American Type Culture Collection currently provides 14 certified human bladder cell lines (4 normal and 10 cancer). Those from cancer sources are among the most popular, mainly HTB-9 and HTB-4, due to their fast growth and easy manipulation. Apart from their obvious relevance for cancer studies, the data obtained with these cells in UTI research should be carefully interpreted due to the differences between normal and cancer cells. Moreover, these cells cannot acquire a native three-dimensional urothelial architecture. On the other hand, normal cells are either immortalized, which may compromise cell differentiation (Georgopoulos et al., 2011), or are derived from human samples (urine or biopsies), maintaining their ability to stratify and differentiate. In the latter case, cells can only be used during a limited number of passages so their routine use requires a large amount of material. Also, donor variation may affect reproducibility.
In general, the previously mentioned models generate one of two extremes, either highly differentiated primary cultures with lower serial growth potential, or undifferentiated cultures with higher serial growth potential. Importantly, regardless of the cell source, cells cultured in monolayers do not display growth, architecture and physiology comparable to the human urothelium, limitations that are particularly relevant to UTI (Smith et al., 2011).
In contrast, a more biologically relevant model is organ/explant culture, where intact specimens of tissues are cultured for a time. While these may maintain an accurate 3D cell architecture, stratification and differentiation status, the method is time-consuming, requires a ready availability of fresh tissue, and again, may exhibit inter-sample variability. Also, there is an increasing probability that stromal and urothelial cells will undergo unwanted mixing over culturing time, with the overgrowth of stromal cells relative to urothelial (Baker et al., 2014). Moreover, the lumen of these models is commonly exposed to air, which does not happen in the bladder in situ, and may affect the maintenance of cell differentiation and/or polarization (Višnjar and Kreft, 2013).
Due to the drawbacks of these strategies, other 3D experimental bladder models are emerging which attempt to biomimic the human urothelium using stem- or stem-like cells subjected to phases of propagation/expansion and stratification/differentiation. Some of them were even patented (Cross, 2003). Recently, Kim et al. reported the most complex 3D bladder multilayered model, mimicking an organized architecture of a mature organ through the assembly of a urothelium surrounding stroma and an outer muscle layer (Kim et al., 2020).
Interestingly, apart from the 3D organoid described by Horsley et al. in 2018, all the aforementioned in vitro models were not exposed to urine, or if so, only for a few hours, up to a day – although a few studies report the use of urine components (e.g. ammonia, urea or water). This is important, since the exposure of the apical urothelial surface to urine seems to be essential for proper differentiation and adequate mucopolysaccharide production (Horsley et al., 2018). Also, bacterial behavior and gene expression is affected by the presence of urine (Hagan et al., 2010; Reitzer and Zimmern, 2019); as urine is the natural context in which these microorganisms operate, models that do not include urine will be incomplete.
Notwithstanding the lack of a cellular immune response and the systemic host background that only in vivo studies can provide, it is expected that human-based in vitro biomimetic urothelium constructs with more physiological aspects will accelerate drug discovery for bladder diseases and even reduce the use of animal models (Baker et al., 2014). In a UTI context, promising platforms already exist for the study of host-pathogen interactions, but they lack key parameters which have a profound impact on normal bladder physiology and may dramatically influence the experimental results obtained. Below we review these key aspects and why they should be included in the next generation of models.
Key Features of the Human Bladder That Should Be Recapitulated in Advanced Human-Cell Models, and Models That Attempt Them
Advanced in vitro models more closely approximating the human urothelium would be valuable for assessing the cellular changes that occur with infection. Such models would ideally incorporate at least four unique structural and biophysical aspects theorized to be important for host-pathogen interactions including: (1) tissue architecture; (2) apical urine exposure; (3) dynamic fluid flow; and (4) urothelial stretch. These key features are described in further detail below, in the context of unique bladder physiology, relevance to UTI, and current models or platforms of relevance.
Tissue Architecture
As described above, the human bladder is lined by a unique transitional urothelium formed of approximately 5-7 layers of cells (Figure 1) (reviewed in Khandelwal et al., 2009). Terminally differentiated umbrella or facet cells top the intermediate cell layer and face outward into the bladder lumen in contact with urine; these unique and specialized cells are formed by fusion of intermediate layers below, are very large but flattened when stretched, and form a barrier to urine despite their highly dynamic environment. Turnover is slow under healthy conditions (on the order of weeks), but when an umbrella cell is exfoliated, the urothelium ensures replacement through the fusing and differentiation of intermediate cells, which can be replaced in turn via the basal progenitor layer. Umbrella cells deploy a protective apical array of asymmetric unit membrane plaques comprised of uroplakin proteins (Wu et al., 2009). The urothelium also elaborates a mucopolysaccharide-rich layer of glycosaminoglycans (GAG) which offers additional protection, enhancing the urothelial barrier against urine (Janssen et al., 2013). Indeed, the urothelium is one of the strongest epithelial barriers in vivo (Eaton et al., 2019) with native tissue trans-epithelial electrical resistance (TEER) values exceeding 3000 Ω*cm2 (Cross et al., 2005). The urothelium is not a passive barrier but is responsive to biomechanical cues, serving as a “mechanosensory conductor” as distention of the bladder stretches the urothelium during filling (Winder et al., 2014).
Given that the urothelium exhibits differential expression of specific markers throughout its layers, recapitulating the stratified human urothelium with appropriate markers and functions is critical for in vitro models of human bladder health and disease, and has particular relevance for UTI. The hypothesis that deep “quiescent intracellular reservoirs” in the intermediate cell layer may act as long-term reservoirs for recurrence requires a thicker multi-layer structure for studying this phenomenon. Moreover, as infection causes copious apical shedding, more than three layers are useful for retaining urothelial structure during bacterial insult. At the same time, studying adhesion properties of uropathogens, as well as innate epithelial response, ideally requires a human umbrella cell-bacteria interface.
Strategies to Induce or Improve Differentiated Multicellular Architecture
Much of our understanding of stratified urothelial architecture comes from animal models and human bladder biopsies (Jost et al., 1989), but recreating this architecture in vitro is not trivial. The following trends emerged as we surveyed the literature to elucidate what factors are critical for achieving multiple cell layers alongside umbrella cell differentiation in human-cell models.
Cell Source
Three-dimensional, multilayer urothelial organoid development in vitro has been reported using various cell sources: patient-derived cells from biopsies and surgical specimens, either by enzymatic dissociation or explant (e.g., Southgate et al., 1994; Daher et al., 2004; Cross et al., 2005; Zhang and Atala, 2013) immortalized cell lines (e.g., UROtsa used by Rossi et al., 2001); commercial primary cells or spontaneously immortalized cells (HBEP and HBLAK used by Horsley et al., 2018); human urine stem cells (Lang et al., 2013); and iPS cells (Suzuki et al., 2019). However, not all recapitulate the proper stratification and necessary hallmarks of the apical-most layer of umbrella cells, nor were used for infection. Most models report at least some important features, such as uroplakins, cytokeratins, and/or junctions, and include some form of visual evidence of a multilayer structure (e.g., electron or confocal microscopy). It remains unclear whether one cell source is superior to another, since there is no standard approach to urothelial differentiation either in terms of method or assessment. The ideal cell source would be readily available (either commercially and/or through well-described isolation techniques) and lead to reproducible differentiation in culture under defined conditions.
Organoid vs. Spheroid Culture
Although there is some confusion about the terms, organoids are usually defined as in vitro culture models that replicate features of native tissue organization, whereas spheroids are a specific, spherical form of organoid (Fang and Eglen, 2017; Vasyutin et al., 2019). While each approach has its advantages and limitations, a flattened organoid approach may be more desirable for UTI models, as the lumen of spheroid cultures would be difficult to access for inducing infection and readouts of interest beyond imaging (e.g., secreted factors, barrier function assays). Flattened organoids, on the other hand, can establish 3D tissue architecture on a 2D surface allowing for ease of infection, imaging, sampling of media, barrier function (permeability assays or TEER), and the addition of other biophysical cues such as flow and/or stretch as described below.
Manipulation of Soluble Factors and Media Additives
It is well-established that “high” calcium (2-4 mM) is a major factor for in vitro stratification and differentiation of human urothelial models (e.g., (Southgate et al., 1994; Rossi et al., 2001; Daher et al., 2004). Other additives have been used, such as FGF-7 (Tash et al., 2001), FGF-10, PPAR-γ agonists, and/or EGFR inhibitors (Suzuki et al., 2019). Urine exposure may also be required for umbrella cell differentiation (Horsley et al., 2018). Additional systematic studies of necessary factors may facilitate convergence towards a standardized media for urothelial models.
Culture Platform
Beyond the liquid environment, the solid substrate on which cells are cultured can greatly influence 3D tissue architecture. Substrate mechanical properties have been shown to strongly affect cell structure and function, which may influence the optimal choice of materials (Janmey et al., 2020). The most prevalent platforms have been tissue culture plastic (e.g., well plates) and Transwell® inserts or similar porous membrane culture systems. The pore size typically used, when reported, is 0.4-0.45 µm diameter. Pore size and pore density may be critical factors in urothelial stratification and differentiation, but to our knowledge have not been systematically studied. Stratification is enhanced on porous membrane substrates, leading to increased urothelial tissue thickness and more uniform stratification (Cross et al., 2005; Suzuki et al., 2019) compared with well plates. In addition to providing nutrients to both sides of the tissue which could allow thicker layer formation, polarization can be achieved by providing differential cues on each side of the microporous membrane. Thus, a microporous substrate may be a requirement for advanced 3D urothelial organoid models. However, Transwells and similar platforms are static cultures not readily adaptable to dynamic biomechanical cues such as fluid flow and stretch. These advanced platforms will be described later.
Extracellular Matrix
The extracellular matrix (ECM) can influence cell adhesion, proliferation, differentiation and function (Hynes, 2009; Yue, 2014; Chaudhuri et al., 2020). Collagen IV is the ECM coating of choice across multiple models, and bestowed accelerated outgrowth of urothelial explants compared with laminin or fibronectin (Daher et al., 2004). Beyond substrate coatings, more complex ECM scaffolds may be beneficial for 3D urothelial model development. The Atala lab has performed extensive studies of decellularized scaffolds, including the bladder, and has described several approaches to 3D urothelial culture on ECM scaffolds (Zhang and Atala, 2013). Such scaffolds are naturally porous and provide more complex environmental cues such as multiple ECM components and the topography present in native tissue. Whether decellularized bladder or similar scaffolds enhance urothelial stratification and differentiation compared with other culture substrates remains to be determined.
Co-Culture
While many urothelial models are derived from a single cell source, some studies have co-cultured other cell types. The most common addition is fibroblasts (Vasyutin et al., 2019), although endothelial cells (Sharma et al., 2021) and smooth muscle cells have also been used (Zhang and Atala, 2013). Additional cell types found in the underlying stroma may provide important cues that influence urothelial proliferation, architecture and differentiation (Southgate et al., 1994). A challenge with co-culture is the inevitable increase in biological variability; alternative approaches such as defined soluble factors may be preferred when cross-talk between cell types is not critical to the study.
Apical Urine Exposure
The urine microenvironment is an important consideration for UTI models from both the host and pathogen side. To our knowledge only two studies report the use of long-term urine exposure (>24 h) in urothelial models. One model implemented commercially available cells (primary HBEC and spontaneously immortalized HBLAK), differentiation media, and pooled urine in Millicell Transwell inserts (Horsley et al., 2018). Urine was introduced into the insert (apical side of the cell culture) 24 hr after initiating differentiation of the confluent monolayer and maintained for several weeks. The authors reported that urine was necessary for stratification, differentiation of the umbrella cell layer and GAG elaboration. This model was also used to study infection with Enterococcus faecalis, resulting in urothelial sloughing and formation of intracellular colonies previously observed in human patient cells. Second, a recent pre-print described a bladder-on-chip system with human bladder epithelial cells, bladder microvascular cells and neutrophils in the Emulate microfluidic platform with flow and mechanical stretch (Sharma et al., 2021). The bladder epithelium was exposed to diluted urine in the co-culture, including during infection. However, the effect of urine on model development and infection was not explicitly studied. In another recent example, a microfluidic platform with the human bladder epithelial cell line PD07i was used in experiments to infect UPEC with short-term (20 h) urine exposure under flow (Iosifidis and Duggin, 2020).
Given that few human urothelial models use urine, much remains to be learned about this key variable. In vivo, the umbrella cells are exposed to urine and form a tight barrier against it, while being nourished by the underlying vasculature and other cells/tissue in close proximity. Although urine may be necessary for umbrella cell differentiation in vitro, it is a harsh environment and cannot be the sole fluid used, even short term. For an ideal in vitro model, the apical surface of the urothelium would be exposed to urine while the basolateral compartment would be supported by an appropriate differentiation media. This is why platforms incorporating a microporous substrate with apical and basal compartmentalization will be more suitable for advanced urothelial models. The simplest implementation would be a Transwell® or similar permeable membrane systems, such as described by Horsley et al., 2018. Other parameters to consider are the timing of urine introduction, acclimation to increasing concentrations of urine over time, and donor characteristics (sex, age, any diseases or conditions that may alter urine composition). More advanced models may implement platforms with fluid flow, such as systems described in the following sections.
Although existing static organoid models can capture some of human urothelial physiology, many biological questions probably can be answered only with a model incorporating additional biophysical aspects, including fluid flow and stretch, which are present in the human bladder and likely affect both normal physiology and infection dynamics. In vitro models that incorporate flow and/or stretch have been developed, but very few for the human bladder. We describe below how flow and stretch are important for both normal bladder function and UTI, and how incorporating these biomechanical cues into an in vitro model would be a valuable addition.
Dynamic Fluid Flow
Fluid Flow in the Bladder
Given the shape and compliance of the bladder, fluid flow during voiding is extremely complex and non-uniform. Computational fluid dynamic (CFD) simulations of flow in the bladder-urethra system revealed that the peak shear stress experienced by cells is approximately 3 dyn/cm2 (Jin et al., 2010) – a moderate level compared with that found in the vascular system, for example. However, this level is only encountered by cells in the urethra, whereas the bladder umbrella cells experience extremely low shear, perhaps well below the levels required to activate shear-induced bacterial adhesion mechanisms. While this does not discount the hypothesis that flow-induced bacterial adhesion plays a role in bladder UTI, it does suggest that umbrella cells are perhaps less affected by this process in vivo, although it may be a likely mechanism in the urethra. A CFD-based study by (Ateşçi et al., 2014) produced similar results but in terms of velocity profiles within the bladder and urethra. Although this simulation data cannot be directly extrapolated to assign shear stress values experienced at the bladder wall by umbrella cells, it indicates that it is lower than in the urethra.
Aside from fluid flow possibly affecting uropathogenic bacterial adherence, it may also influence other virulence behaviors including the propensity of UPEC to take on a filamentous form that increases virulence, adhesion, invasion and escape from immune surveillance (Justice et al., 2006; Andersen et al., 2012). This may be particularly relevant for biofilm formation (Weaver et al., 2012). In addition, a number of studies have shown that microfluidic flow provides physiological cues that guide tissue architecture; as one example, in an organotypic kidney model, fluid flow altered cell shape, protein expression and transport to better approximate in vivo organization (Jang et al., 2013). Although currently under-studied in the context of bladder cell culture models, flow even with low shear stress could be an important cue to enhance urothelial organoid development in vitro as well as subsequent studies of infection. Models of the urethra with higher shear could provide insights into mechanisms of bacterial adhesion and how uropathogens migrate into the bladder. Examples of models incorporating fluid flow are provided below.
Models Incorporating Fluid Flow
Fluid flow has been incorporated into numerous in vitro model organ systems as a means for fluid and nutrient exchange, waste removal, to maintain cellular survival and tissue architecture, or to induce cellular differentiation (Baudoin et al., 2007; Carraro et al., 2008; Fritsche et al., 2009; Douville et al., 2011; Cheng et al., 2012; Park et al., 2012; Agarwal et al., 2013; Kim and Ingber, 2013; Torisawa et al., 2014; Zhang et al., 2014; Henry et al., 2017; Kasendra et al., 2018; Shin et al., 2019; Sidar et al., 2019; Azizipour et al., 2020). Many liver and kidney models use fluid flow to maintain tissue structure, deliver drugs, measure reabsorption, or carry out metabolites for ADME-tox studies (Sin et al., 2004; Sivaraman et al., 2005; Kane et al., 2006; Lee et al., 2007; Chao et al., 2009; Mahler et al., 2009; Toh et al., 2009; Jang and Suh, 2010; Novik et al., 2010; Snouber et al., 2012; Legendre et al., 2013; Esch et al., 2015; Lee et al., 2017; Maschmeyer et al., 2015; Vedula et al., 2017; Zhang et al., 2017; Bale et al., 2019; Homan et al., 2019; Jalili-Firoozinezhad et al., 2019; Tan et al., 2020). Disrupted flow patterns have been applied to induce damage that mimics tissue injury in lung and heart models (Huh et al., 2007; Giridharan et al., 2010; Huh et al., 2010; Khanal et al., 2011; Tavana et al., 2011; Nguyen et al., 2015), and microfluidic flow systems have been created to study muscle contractility (Grosberg et al., 2012), endothelial vascularization (Shin et al., 2004), pulmonary thrombosis (Jain et al., 2018), tumor-vascular invasion (Nguyen et al., 2019), blood-brain barrier (Booth and Kim, 2012), nerve injury (Park et al., 2013; Tsantoulas et al., 2013) and neuronal networks (Shi et al., 2013; Xiao et al., 2013; van de Wijdeven et al., 2018).
Microfluidic lung and gut models have also been used to study infection by bacteria and viruses. For example, fluid flow, either alone or together with cyclical strain, enabled not only proper tissue differentiation but also enhanced invasion by the infecting organism (Zhu et al., 2009; Kang et al., 2015; Barr et al., 2015; Benam et al., 2016; Villenave et al., 2017; Ortega-Prieto et al., 2018; Sunuwar et al., 2020; Tang et al., 2020; Thacker et al., 2020; Baddal and Marrazzo, 2021). Fluid flow led to upregulated invasion of colonic epithelium by Shigella bacteria (in the presence of peristalsis-like mechanical strain) (Grassart et al., 2019), increased epithelial barrier function in a lung epithelium/macrophage influenza virus co-infection model (Deinhardt-Emmer et al., 2020), and enhanced infection of alveolar or airway epithelial cells by SARS-CoV-2 (Si et al., 2020; Zhang et al., 2021). Finally, Kim et al. used a microfluidic gut chip to study the contributions of the microbiome to intestinal pathophysiology and showed that flow plus peristalsis-like mechanical deformations were necessary to protect against bacterial overgrowth reminiscent of inflammatory bowel disease (Kim et al., 2016); other studies have used microfluidic systems to investigate host-microbe interactions (Kim et al., 2012; Shah et al., 2016) and microbial diversity (Tovaglieri et al., 2019; Jalili-Firoozinezhad et al., 2019).
Despite its potential relevance, few in vitro bladder models have incorporated flow. One study used a Cellix chip to show that flow of UPEC in cell culture media enabled their adhesion to vascular endothelial cells (mimicking blood-borne dissemination) but had minimal effect on binding to bladder epithelial cells compared with static conditions (Feenstra et al., 2017). However, this study was performed in 2D culture in the absence of urine, and the primary goal was to investigate blood-borne dissemination of UPEC rather than invasion into bladder cells per se. Another study demonstrated filamentous rod formation and secondary infection of bladder cells by UPEC under conditions of urine flow using a flow-cell chamber (Andersen et al., 2012). The degree of filamentation was dependent on urine concentration, supporting the idea that flow can affect the infection behavior of UPEC in human cells. However, infection was tested in bladder cell cultures, not a fully differentiated urothelium. As mentioned above, a microfluidic platform with urine flow was used to study UPEC behavior in a human bladder epithelial cell line (Iosifidis and Duggin, 2020). In a recent pre-print, Sharma et al., 2021 reported a bladder-on-chip incorporating fluid flow, mechanical strain and urine exposure applied to differentiated bladder cell monolayers co-cultured with microvascular cells (see Section 5.4.3) (Sharma et al., 2021). Finally, attachment of E. coli to bladder cell line monolayers was found to be highest at low shear stress, allowing for maximal initial attachment of bacterial Fim H receptors via a slip-bond mechanism (Zalewska-Piątek et al., 2020). Thus, how the low level of shear experienced by umbrella cells in vivo (Jin et al., 2010; Ateşçi et al., 2014) affects their physiology and interaction with invading pathogens remains unclear. The above studies suggest the potential for uncovering further benefits of flow in UTI bladder models, including (but not limited to) maintenance of bladder epithelial differentiation and promotion of bacterial-epithelial interactions in a more physiological context.
Platforms/Strategies to Study the Effects of Flow
To study the role of fluid flow in UTI, the ideal platform would provide user control over flow (for example, to mimic urination patterns, to provide perfusion or nutrient replenishment); have the potential to include stretch or other biophysical and biomechanical cues (e.g., ECM); maintain two fluid compartments to aid polarization of the urothelial organoid; incorporate capabilities to interrogate the tissue using various assays; and ideally scale to a throughput allowing experimental replicates while also accommodating multiple variables such bacterial strains and therapeutic dosing. Microfluidic-based approaches such as “microphysiological systems” or “organ-on-chip” platforms have been deployed extensively for other tissue models (recent reviews include (Zhang et al., 2018; Low et al., 2020; Peterson et al., 2020) and could be an equally promising strategy for UTI models. Bacterial biofilms and antibiotic therapies have been studied in cell-free systems with flow, where flow within a microfluidic configuration allowed analysis of biofilm growth and infection potential (Terry and Neethirajan, 2014; Wright et al., 2015). As described above, bacterial infection of human cells has also responded to flow in an in vitro system capable of stretch (Grassart et al., 2019). Such systems demonstrate the utility and impact of flow on bacterial infection in vitro, but have yet to be merged with throughput. Given the timeframe of urothelial organoid differentiation in vitro (2-3 weeks), throughput will be essential for advanced models seeking to study multiple variables or for drug discovery efforts. Flow has been employed in high throughput, although not in an infection context, and the flow mechanism in those studies afforded limited control of flow parameters (Vormann et al., 2021). Pump-controlled flow has been deployed in high throughput in a microfluidic system with two fluid compartments for a liver model (Tan et al., 2020), but the system lacked a stretch component. A logical next step would be to incorporate flow into a high throughput system, while retaining the ability to actuate stretch for a two-fluid compartment system for advanced UTI models.
Urothelial Stretch
Many tissues in the human body undergo modest dynamic mechanical deformation, but the bladder expands drastically during the micturition cycle. Unlike the heart and blood vessels which are constantly under pressure from the blood, the bladder experiences the extremes of being completely empty and completely full, with maximum volume capacity of the human bladder ranging from 300-500 ml. Pressures experienced within the bladder range from 0-34 cmH2O (0-25 mmHg) while filling and can be as high as 200 cmH2O (100-150 mmHg) when full (Wolfe et al., 2015). In vivo, pressure and stretch are coupled by the physiological processes of gradual urine filling, storage and sudden emptying, but these biomechanical cues can be studied independently ex vivo or in vitro.
Given the ability of the urothelium to increase its surface area in response to pressure or stretch, proscribing a mechanical strain experienced by the tissue is not a simple calculation, and data are difficult to obtain for humans. The urothelium can increase in apical surface area by ~50% within hours of experiencing stretch (Wang et al., 2003). Bladder tissue capacitance, a measure of umbrella cell surface area, increases over approximately 5 hr regardless of the filling rate at a pressure differential of 8 cmH2O (Truschel et al., 2002; Carattino et al., 2013), which represents the pressure during the extended storage phase in rabbits (Levin and Wein, 1982). Studies of rat bladders suggest than under normal filling loads, the bladder wall itself passively stretches 10-20% (Gloeckner et al., 2002). Calculations based on responses of rabbit bladder tissue to pressure suggest that umbrella cells can respond to strains in this same range (Carattino et al., 2013).
Biological Effects of Stretch
Cells in other mechanically active tissues have well-developed mechano-sensing properties; the bladder urothelium is no exception. Several signaling pathways and urothelial receptors contribute to bladder sensing of stretch, and are described in detail in (Janssen et al., 2017). Biomechanical stretch is known to affect a variety of cellular behaviors, such as proliferation, differentiation, maturation, and tissue-specific functions (Zimmermann, 2013; Yu et al., 2016). Limited published data exist regarding the effects of stretch on human urothelial cell behavior, although a few are highlighted below. As such, most of our understanding is derived from animal studies, typically rodent and rabbit, where species differences likely exist.
A primary urothelial function is to maintain the urine-blood barrier (Kreft et al., 2010), no small biological feat during the dramatic and dynamic mechanical fluctuations that occur with bladder filling and voiding. It has been reported that TEER initially dips and then increases during prolonged stretch (Truschel et al., 2002). Although TEER may drop with stretch, tight junctions are nevertheless maintained, as determined by immunofluorescent staining for claudins and ZO-1 and the limited permeability of tracer molecules (Carattino et al., 2013). Another group studied human urothelial cell response to various strains and noted increased proliferation under 5% strain (Gao et al., 2018).
During bladder filling and extended storage phases, umbrella cells undergo remarkable changes in surface area mediated by endo- and exocytosis (Truschel et al., 2002). Changes in these rates are triggered by mechanical stretch, but not pressure, and begin within seconds of inducing stretch at pressures as low as 2 cmH2O (Yu et al., 2009). This behavior was demonstrated ex vivo using rabbit bladder tissue by measured alterations in tissue capacitance, indicating changes in surface area, which increased up to 50% after 5 hr of prolonged stretch. Endocytosis was also remarkably upregulated with stretch as assayed by biotin-labeled membrane internalization and imaging of intracellular FITC-labeled wheat germ agglutinin. Prolonged, rather than transient or short-term, stretch is required for observed changes in surface area. However, increased endocytosis occurs in as little as 5 min after initiation of stretched conditions (Truschel et al., 2002).
Another study showed that mechanical stretch associated with filling and voiding increased the endo/exocytosis behavior of bladder epithelial cells, which use fusiform endocytic vesicle transport to increase and decrease surface membrane area during bladder expansion and contraction, respectively. This vesicle trafficking is of considerable interest because it is hypothesized to be a mechanism hijacked by certain bacterial strains that form intracellular colonies (Bishop et al., 2007).
Models Incorporating Urothelial Stretch
Despite the critical physiological relevance, an integrated stretch platform to support complex differentiation of urothelial organoids along with fluidics and polarized urine exposure has only begun to be explored. But at a more basic level, researchers have used multiple strategies to study stretch in urothelial cells, ranging from whole bladder distension to urothelial monolayer culture on commercially available stretch platforms. Most such studies have focused on the impact of mechanical stretch on ATP release, though few have evaluated human cells. ATP activity is linked to exocytosis and endocytosis of the mucosal surface through binding of umbrella P2 receptors; thus, the impact of stretch on ATP secretion inevitably affects membrane transport mechanisms (Wang et al., 2005) which, as pointed out previously, is potentially important for uropathogen invasion.
Tanaka et al. used an organ bath to demonstrate the impact of stretch on ATP and prostaglandin E2 release, which was dependent on volume-based rat bladder distension (Tanaka et al., 2011). To interrogate the impact of stretch on ATP exocytosis, Mochizuki et al. developed elastic silicone chambers mounted on glass coverslips seeded with primary mouse urothelial cells for simultaneous stretch and in situ Ca2+ imaging. They demonstrated that the TRPV4 cation channel mediates the release of ATP and the influx of Ca2+ via a stretch-dependent mechanism (Mochizuki et al., 2009). This stretch methodology was also subsequently used to analyze mechanosensation in the bladder through piezo channels (Miyamoto et al., 2014).
The importance of stretch on barrier function and tight junction proteins was demonstrated by mounting circular excised sections of rabbit urothelia in modified Ussing chambers and utilizing hydrostatic pressure-induced stretch (Truschel et al., 2002; Carattino et al., 2013). Multiple studies used a similar system to study the polarization of stretch-induced ATP secretion, demonstrating ten times more concentrated ATP supernatant on the mucosal surface versus the basal surface in rabbits (Yu, 2015). Although these models are fascinating, all results discussed thus far have been in non-human urothelial cell models and tissues.
A small subset of studies focusing on patient samples has demonstrated that mechanical stretch also affects primary human urothelial cells cultured in vitro. Using commercial stretch platforms, primary human cells isolated from patients with interstitial cystitis (IC), a chronic disease of unknown etiology, were shown to have significantly higher supernatant levels of ATP under stretch compared with stretched healthy control samples (Sun et al., 2001). Similarly, stretched IC patient cells demonstrated higher levels of purinergic receptor subtype P2X3, which plays a role in transmitting pain signals to the central nervous system (SUN and CHAI, 2004). This finding supports the hypothesis that urothelial cells can phenotypically mimic sensory neurons, with this phenotype driven by the presence of mechanical stretch. Therefore, primary human urothelial cells in stretch models can address crucial questions in urothelial biology.
In addition to the urothelium, the impact of mechanical stretch on other cell types such as smooth muscle cells (SMCs) isolated from the bladder has been explored. Bu et al. demonstrated that stretch-induced proliferation of SMCs correlated with the upregulation of metalloproteinases (MMPs) MMP-1, 2, 3, and 7 under 10% and 15% stretch conditions in a stretch-dependent manner (Bu et al., 2014). Other studies have explored the impact of stretch on SMCs, demonstrating the importance of this mechanical stimulus for aspects of the bladder beyond the urothelium (McDermott et al., 2013; Zheng et al., 2016; Pingyu et al., 2019).
Platforms/Strategies to Study the Effects of Stretch
Multiple commercial platforms provide the capability to study various in vitro tissues under mechanical stretch conditions, though certain parameters discussed in this review have yet to be incorporated into a platform capable of supporting a complete bladder infection model. Ideally, an in vitro system capable of uniform, biaxial strain within the range of 10-50% strain is desirable. To support a pragmatic and robust workflow, the ability to experiment with multiple replicates and image experimental samples in situ is vital; however, frequently used stretch platforms in their current forms are not likely to support the complexity of a fully differentiated bladder UTI model.
Existing commercial options achieve multiple-device throughput by implementing stretch on silicone-based substrates such as the uniaxial MCFX (CellScale) and STB-1400 (Strex Inc.) systems, achieving maximum strains of 12.5% and 20%, respectively. These companies also offer models that achieve biaxial stretch up to 20% in the XY plane, but most commercial biaxial models are only offered as single-well systems, such as the MCB1 (CellScale), or the STB-190-XY (Strex Inc.), which sacrifices throughput for the additional strain dimension. The most impressive system to date able to implement biaxial strain in relatively high throughput, the HT BioFlex® (FlexCell), can actuate 24 wells at once, though the increased throughput sacrifices the maximum strain that the system can achieve, topping out at 8%. The company offers a lower throughput BioFlex® model that achieves biaxial 20% strain in 6 devices simultaneously (FlexCell).
The ability to provide multi-faceted stimuli for cell-type differentiation is favorable; the C-Stretch system (IonOptix LLC) incorporates electrical stimulation alongside stretch to assist differentiation of naïve cultured cardiomyocytes. The ability to incorporate both fluid flow and stretch in these platforms would be widely beneficial for bladder and other in vitro models. Unfortunately, none of these systems incorporate them simultaneously, and all of the options discussed do not implement permeable substrates compatible with urine exposure differentiation strategies.
Figure 2 depicts examples of various platforms used for in vitro bladder studies. To date, the closest relevant platform capable of integrating multiple mechanical stimuli is the Chip S-1® system (Emulate Inc.), which utilizes microfluidic flow paths and flexible side walls exposed to vacuum to deliver simultaneous flow up to 0.3 dynes/cm2, and demonstrated uniaxial strain of 10%. In a recent pre-print, this platform modeled infection in differentiated HTB9 bladder cancer cell monolayers over a 6-hour voiding cycle, incorporating both flow and mechanical stretch with a porous membrane allowing for fluid transport between channels; neutrophils were recruited to sites of infection, and UPEC IBCs formed and persisted in the presence of antibiotics, supporting the hypothesis that IBCs play a role in recurrent infection (Sharma et al., 2021). Despite these strengths, areas for improvement include urothelial stratification, throughput capacity, and membrane properties, specifically pore size, pore density and drug sorption issues in PDMS (Shirure and George, 2017).
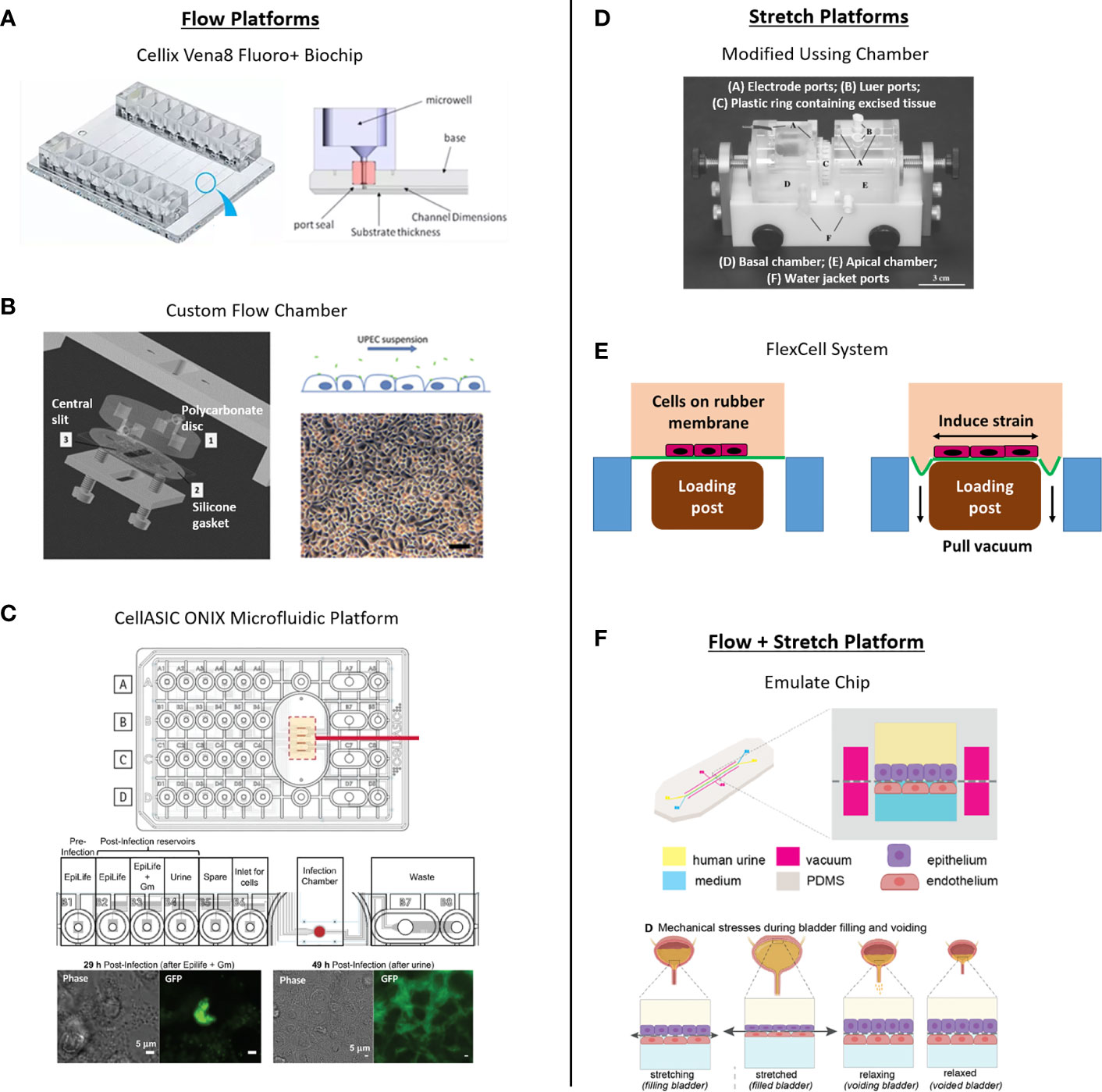
Figure 2 Platforms with fluid flow and/or mechanical stretch used for in vitro bladder studies. (A) The Cellix Vena8 Fluoro+ Biochip was used by Feenstra et al., 2017 to study E. coli adhesion to human microvascular endothelial cells and bladder epithelial cell lines. Figures modified from Cellix company website, with permission. (B) Custom flow chambers were used by Andersen et al., 2012 and Zalewska-Piatek et al., 2020 to study the role of flow in E. coli adhesion to human bladder epithelial cells. Example shown is from Andersen et al., 2012 reproduced with permission from the American Society for Microbiology. (C) The CellASIC ONIX platform was used by Iosifidis and Duggin, 2020 to study the role of urine composition and pH on UPEC infection of a bladder epithelial cell line. Top schematic of plate from Lee et al., reproduced with permission from Springer Nature. Bottom panel from Iosifidis and Duggin, 2020 reproduced with permission from the American Society for Microbiology. (D) Truschel et al., 2002 used modified Ussing chambers to induce stretch of excised rabbit bladder tissue. Figure reproduced in compliance with the Creative Commons Non-Commercial Share Alike 3.0 Uported license agreement. (E) FlexCell systems were used by Sun et al., 2001 and 2004 studies to investigate ATP release from primary human bladder urothelial cells from healthy and IC patients. Author schematic depicting the platform’s function. (F) Recently, Sharma et al., 2021 used Emulate’s organ-on-chip platform that incorporates both fluid flow and stretch in a bladder chip model of infection. Figure modified from Sharma et al., 2021 pre-print in compliance with the Creative Commons CC-BY-NC-ND 4.0 International License.
To our knowledge the field still lacks a combined stretch- and flow-based platform with some degree of throughput to support a truly differentiated and stratified human urothelial organoid, fulfilling all the criteria necessary for exploring the complex mechanisms involved in human bladder UTIs (Figure 3).
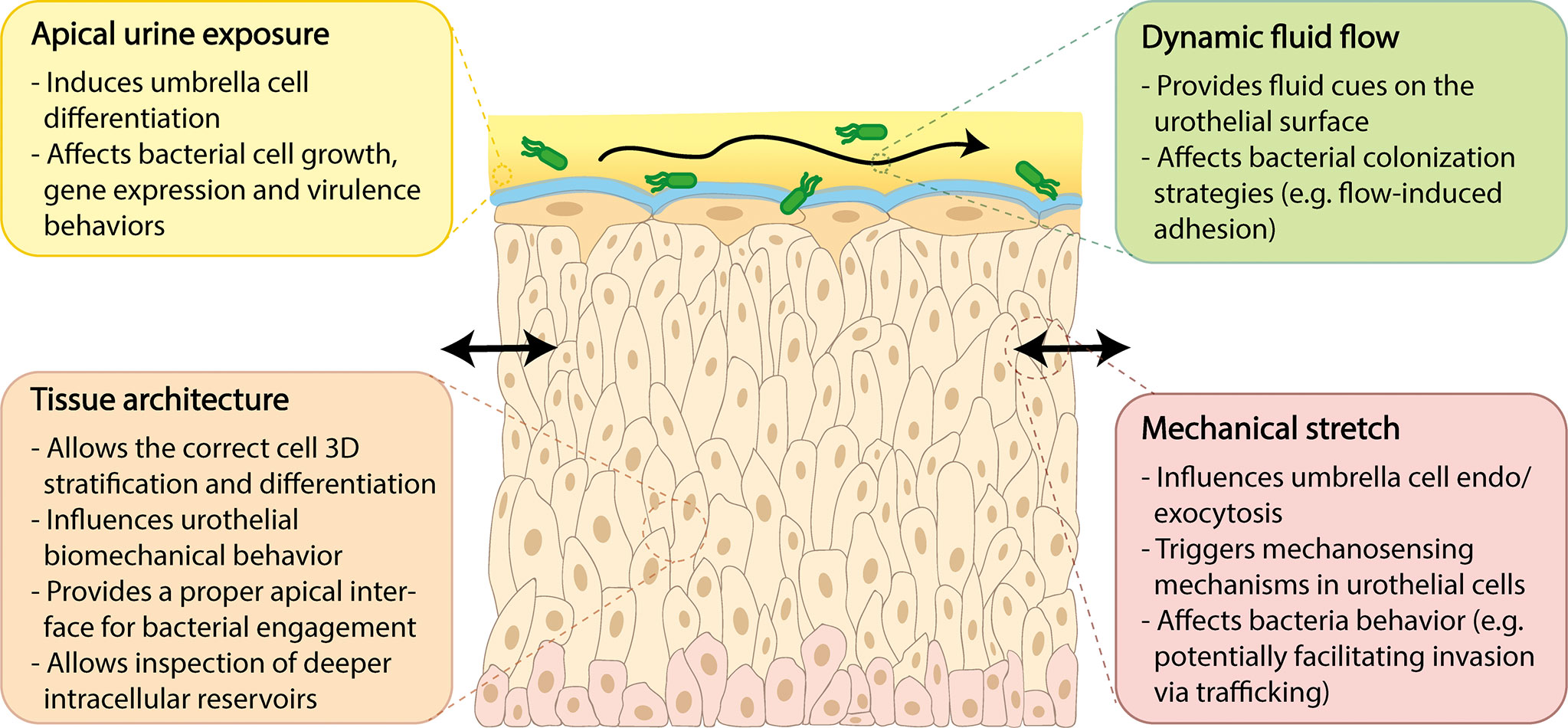
Figure 3 Key features required for advanced human urothelial models and their importance for UTI research.
Future directions
Many mysteries still remain regarding the host/pathogen interactions of UPEC, to say nothing of the wide array of other understudied species that infect the urinary tract. On the horizon, technological advancements promise an exciting array of improvements to in vitro human models both generally, and for studying the infection biology of UTI in particular. The use of human cells also opens up the possibility of precision medicine by allowing the interrogation of UTI in the context of individual patients.
It is important to be aware of the limitations of in vitro systems. For instance, since most human cell-based models are closed structures, they still lack tissue-tissue interfaces, vascularization and circulation of immune cells. Therefore, beyond making the necessary improvements described in this review to isolated urothelial systems, we expect that linking up the bladder environment to its adjacent niches such as gut and kidney using “body-on-chip” platforms will add further nuance. Similarly, co-culturing human immune cells and/or underlying human muscle cells, fibroblasts and stroma could breathe further life into existing models. Currently, however, the recapitulation of some crucial physiology is difficult, such as the transport and/or absorption of drugs, nutrients, oxygen, inflammatory molecules and the immune cells themselves. However, significant breakthroughs have occurred recently (reviewed in Ingber, 2020). Once advanced strategies can be introduced into human-based models, such as the integration of self-organized capillary networks, a complex surrounding ECM, sensors for real-time functional measurements, and the long-term maintenance of co-cultures with different tissue types and their microbiota, the study of human pathophysiological events may well be more accurate and controllable than in animal models.
While animals will continue to reveal crucial insights about human infection biology, we must acknowledge their own limitations openly. We support a strategy whereby human cell model systems are used alongside animals to provide complementary information. It is no secret that paper referees and grant reviewers are quick to demand animal studies or to discount meticulous results gathered using even advanced human in vitro systems, and we quite agree with those (Ingber, 2020) who argue that the scientific community should consider whether such dampening activities are in the best spirit of scientific inquiry.
Author Contributions
BM, CF, JR, CW and DF conceived the overall conception and design of the review. All authors contributed to writing various sections of the manuscript. All authors contributed to the article and approved the submitted version.
Funding
This work was supported by the UK Engineering and Physical Sciences Research Council (UCL Grant Number 554581).
Conflict of Interest
Authors CW, DF, EM, BC, and JC were or are currently employed by The Charles Stark Draper Laboratory, Inc., a not-for-profit research and development organization that develops hardware for advanced biological models. JR and BM have received research funding from AtoCap Ltd., a University College London spinoff company, to develop novel cures for urinary tract infection and bladder cancer, and JR has share options in the company. JR and CF have also received basic research funding from Pfizer.
The remaining authors declare that the research was conducted in the absence of any commercial or financial relationships that could be construed as a potential conflict of interest.
Acknowledgments
We thank Dr. Jeffrey Borenstein (Draper) for his critical review of the manuscript as well as members of the Rohn lab (University College London) for their scientific insight and discussions. Content contained in Figure 2 previously appeared online and are used with permission.
References
Abraham, S. N., Miao, Y. (2015). The Nature of Immune Responses to Urinary Tract Infections. Nat. Rev. Immunol. 15, 655–663. doi: 10.1038/nri3887
Agarwal, A., Goss, J. A., Cho, A., McCain, M. L., Parker, K. K. (2013). Microfluidic Heart on a Chip for Higher Throughput Pharmacological Studies. Lab. Chip 13, 3599–3608. doi: 10.1039/c3lc50350j
Aizawa, N., Homma, Y., Igawa, Y. (2013). Influence of High Fat Diet Feeding for 20 Weeks on Lower Urinary Tract Function in Mice. LUTS: Lower Urinary Tract Symptoms 5, 101–108. doi: 10.1111/j.1757-5672.2012.00172.x
Alamuri, P., Löwer, M., Hiss, J. A., Himpsl, S. D., Schneider, G., Mobley, H. L.T. (2010). Adhesion, Invasion, and Agglutination Mediated by Two Trimeric Autotransporters in the Human Uropathogen Proteus Mirabilis. Infection Immun. 78, 4882. doi: 10.1128/IAI.00718-10
Andersen, T. E., Khandige, S., Madelung, M., Brewer, J., Kolmos, H. J., Møller-Jensen, J. (2012). Escherichia Coli Uropathogenesis In Vitro: Invasion, Cellular Escape, and Secondary Infection Analyzed in a Human Bladder Cell Infection Model. Infection Immun. 80, 1858–1867. doi: 10.1128/IAI.06075-11
Anderson, G. G., Palermo, J. J., Schilling, J. D., Roth, R., Heuser, J., Hultgren, S. J. (2003). Intracellular Bacterial Biofilm-Like Pods in Urinary Tract Infections’. Science 301, 105. doi: 10.1126/science.1084550
Archer, G. T. L. (1931). Notes on the Proteus Group of Organisms With Special Reference to a Case of Renal Infection by a Member of This Group. J. R. Army Med. Corps 57, 241. doi: 10.1136/jramc-57-04-01
Armbruster, C. E., Mobley, H. L.T. (2012). Merging Mythology and Morphology: The Multifaceted Lifestyle of Proteus Mirabilis. Nat. Rev. Microbiol. 10, 743–754. doi: 10.1038/nrmicro2890
Ateşçi, Y. Z., Aydoğdu, Özgü, Karaköse, A., Pekedis, M., Karal, Ömer, Şentürk, U., et al. (2014). Erratum to “Does Urinary Bladder Shape Affect Urinary Flow Rate in Men With Lower Urinary Tract Symptoms?” Sci. World J. 2014, 462623. doi: 10.1155/2014/462623
Azizipour, N., Avazpour, R., Rosenzweig, D. H., Sawan, M., Ajji, A. (2020). Evolution of Biochip Technology: A Review From Lab-on-a-Chip to Organ-on-a-Chip. Micromachines 11, 599. doi: 10.3390/mi11060599
Baddal, B., Marrazzo, P. (2021). Refining Host-Pathogen Interactions: Organ-on-Chip Side of the Coin. Pathogens 10, 203. doi: 10.3390/pathogens10020203
Baker, S. C., Shabir, S., Southgate, J. (2014). Biomimetic Urothelial Tissue Models for the in Vitro Evaluation of Barrier Physiology and Bladder Drug Efficacy. Mol. Pharmaceut. 11, 1964–1970. doi: 10.1021/mp500065m
Bale, S. S., Manoppo, A., Thompson, R., Markoski, A., Coppeta, J., Cain, B., et al. (2019). A Thermoplastic Microfluidic Microphysiological System to Recapitulate Hepatic Function and Multicellular Interactions. Biotechnol. Bioengineer. 116, 3409–3420. doi: 10.1002/bit.26986
Barber, A. E., Norton, J. P., Wiles, T. J., Mulvey, M. A. (2016). Strengths and Limitations of Model Systems for the Study of Urinary Tract Infections and Related Pathologies. Microbiol. Mol. Biol. Rev. 80, 351. doi: 10.1128/MMBR.00067-15
Barr, J. J., Auro, R., Sam-Soon, N., Kassegne, S., Peters, G., Bonilla, N., et al. (2015). Subdiffusive Motion of Bacteriophage in Mucosal Surfaces Increases the Frequency of Bacterial Encounters. Proc. Natl. Acad. Sci. 112, 13675. doi: 10.1073/pnas.1508355112
Baudoin, R, Griscom, L., Monge, M., Legallais, C, Leclerc, E. (2007). Development of a Renal Microchip for In Vitro Distal Tubule Models. Biotechnol. Prog. 23, 1245–1253. doi: 10.1021/bp0603513
Beatson, S. A., Zakour, N. L.B., Totsika, M., Forde, B. M., Watts, R. E., Mabbett, A. N., et al. (2015). Molecular Analysis of Asymptomatic Bacteriuria Escherichia Coli Strain VR50 Reveals Adaptation to the Urinary Tract by Gene Acquisition. Infection Immun. 83, 1749–1764. doi: 10.1128/IAI.02810-14
Becknell, B., Eichler, T. E., Beceiro, S., Li, B., Easterling, R. S., Carpenter, A. R., et al. (2015). Ribonucleases 6 and 7 Have Antimicrobial Function in the Human and Murine Urinary Tract. Kidney Int. 87, 151–161. doi: 10.1038/ki.2014.268
Belik, R., Föllmann, W., Degen, G. H., Roos, P. H., Blaszkewicz, M., Knopf, H. J., et al (2008). Improvements in Culturing Exfoliated Urothelial Cells In Vitro from Human Urine. J. Toxicol. Environ. Health Part 71, 13–14. doi: 10.1080/15287390801988871
Behzadi, E., Behzadi, P. (2016). The Role of Toll-Like Receptors (Tlrs) in Urinary Tract Infections (Utis). Cent. Eur. J. Urol. 69, 404–410. doi: 10.5173/ceju.2016.871
Benam, K. H., Villenave, R., Lucchesi, C., Varone, A., Hubeau, C., Lee, H.-H., et al. (2016). Small Airway-on-a-Chip Enables Analysis of Human Lung Inflammation and Drug Responses In Vitro. Nat. Methods 13, 151–157. doi: 10.1038/nmeth.3697
Berry, R. E., Klumpp, D. J., Schaeffer, A. J. (2009). Urothelial Cultures Support Intracellular Bacterial Community Formation by Uropathogenic Escherichia Coli. Infection Immun. 77, 2762–2772. doi: 10.1128/IAI.00323-09
Beura, L. K., Hamilton, S. E., Bi, K., Schenkel, J. M., Odumade, O. A., Casey, K. A., et al. (2016). Normalizing the Environment Recapitulates Adult Human Immune Traits in Laboratory Mice. Nature 532, 512–516. doi: 10.1038/nature17655
Billips, B. K., Forrestal, S. G., Rycyk, M. T., Johnson, J. R., Klumpp, D. J., Schaeffer, A. J. (2007). Modulation of Host Innate Immune Response in the Bladder by Uropathogenic Escherichia Coli. Infection Immun. 75, 5353–5360. doi: 10.1128/IAI.00922-07
Billips, B. K., Schaeffer, A. J., Klumpp, D. J. (2008). Molecular Basis of Uropathogenic ≪Em<Escherichia Coli≪/Em< Evasion of the Innate Immune Response in the Bladder. Infection Immun. 76, 3891. doi: 10.1128/IAI.00069-08
Bishop, B. L., Duncan, M. J., Song, J., Li, G., Zaas, D., Abraham, S. N. (2007). Cyclic AMP–regulated Exocytosis of Escherichia Coli From Infected Bladder Epithelial Cells. Nat. Med. 13, 625–630. doi: 10.1038/nm1572
Booth, R., Kim, H. (2012). Characterization of a Microfluidic In Vitro Model of the Blood-Brain Barrier (μbbb). Lab. Chip 12, 1784–1792. doi: 10.1039/c2lc40094d
Brannon, J. R., Dunigan, T. L., Beebout, C. J., Ross, T., Wiebe, M. A., Reynolds, W. S., et al. (2020). Invasion of Vaginal Epithelial Cells by Uropathogenic Escherichia Coli. Nat. Commun. 11, 2803. doi: 10.1038/s41467-020-16627-5
Bu, S., Zhu, Y., Peng, C., Cai, X., Cao, C., Tan, H., et al. (2014). Simulated Physiological Stretch-Induced Proliferation of Human Bladder Smooth Muscle Cells is Regulated by Mmps. Arch. Biochem. Biophysics 564, 197–202. doi: 10.1016/j.abb.2014.09.012
Carattino, M. D., Prakasam, H. S., Ruiz, W. G., Clayton, D. R., McGuire, M., Gallo, L. I., et al. (2013). Bladder Filling and Voiding Affect Umbrella Cell Tight Junction Organization and Function. Am. J. Physiol. Renal Physiol. 305, F1158–F1F68. doi: 10.1152/ajprenal.00282.2013
Carraro, A., Hsu, W.-M., Kulig, K. M., Cheung, W. S., Miller, M. L., Weinberg, E. J., et al. (2008). In Vitro Analysis of a Hepatic Device With Intrinsic Microvascular-Based Channels. Biomed. Microdevices 10, 795–805. doi: 10.1007/s10544-008-9194-3
Carvalho, G., Forestier, C., Mathias, J.-D. (2019). Antibiotic Resilience: A Necessary Concept to Complement Antibiotic Resistance? Proc. R. Soc. B: Biol. Sci. 286, 20192408. doi: 10.1098/rspb.2019.2408
Chao, P., Maguire, T., Novik, E., Cheng, K. C., Yarmush, M. L. (2009). Evaluation of a Microfluidic Based Cell Culture Platform With Primary Human Hepatocytes for the Prediction of Hepatic Clearance in Human. Biochem. Pharmacol. 78, 625–632. doi: 10.1016/j.bcp.2009.05.013
Chaudhuri, O., Cooper-White, J., Janmey, P. A., Mooney, D. J., Shenoy, V. B. (2020). Effects of Extracellular Matrix Viscoelasticity on Cellular Behaviour’. Nature 584, 535–546. doi: 10.1038/s41586-020-2612-2
Cheng, Y., Chen, Z., Gawthorne, J. A., Mukerjee, C., Varettas, K., Mansfield, K. J., et al. (2016). ‘Detection of Intracellular Bacteria in Exfoliated Urothelial Cells From Women With Urge Incontinence’. Pathog. Dis. 74. doi: 10.1093/femspd/ftw067
Cheng, S., Prot, J.-M., Leclerc, E., Bois, FY (2012). Zonation Related Function and Ubiquitination Regulation in Human Hepatocellular Carcinoma Cells in Dynamic vs. Static Culture Conditions. BMC Genomics 13, 54–54. doi: 10.1186/1471-2164-13-54
Chen, Y.-H., Ko, W.-C., Hsueh, P.-R. (2013). Emerging Resistance Problems and Future Perspectives in Pharmacotherapy for Complicated Urinary Tract Infections. Expert Opin. Pharmacother. 14, 587–596. doi: 10.1517/14656566.2013.778827
Chen, L., Welty-Wolf, K. E., Kraft, B. D. (2019). Nonhuman Primate Species as Models of Human Bacterial Sepsis. Lab. Anim. 48, 57–65. doi: 10.1038/s41684-018-0217-2
Chia, R., Achilli, F., Festing, M. F.W., Fisher, E. M.C. (2005). ‘The Origins and Uses of Mouse Outbred Stocks’. Nat. Genet. 37, 1181–1186. doi: 10.1038/ng1665
Chockalingam, A., Stewart, S., Xu, L., Gandhi, A., Matta, M. K., Patel, V., et al. (2019). ‘Evaluation of Immunocompetent Urinary Tract Infected Balb/C Mouse Model for the Study of Antibiotic Resistance Development Using Escherichia Coli CFT073 Infection’. Antibiotics (Basel Switzerland) 8, 170. doi: 10.3390/antibiotics8040170
Chung, J. W. N. C. H. F., van Mastrigt, R. (2009). Age and Volume Dependent Normal Frequency Volume Charts for Healthy Males. J. Urol. 182, 210–214. doi: 10.1016/j.juro.2009.02.113
Cieślik, M., Bagińska, N., Górski, A., Jończyk-Matysiak, E. (2021). Animal Models in the Evaluation of the Effectiveness of Phage Therapy for Infections Caused by Gram-Negative Bacteria From the ESKAPE Group and the Reliability of Its Use in Humans. Microorganisms 9, 206. doi: 10.3390/microorganisms9020206
Coady, A., Ramos, A. R., Olson, J., Nizet, V., Patras, K. A. (2018). Tamm-Horsfall Protein Protects the Urinary Tract Against Candida Albicans. Infection Immun. 86, e00451–e00418. doi: 10.1128/IAI.00451-18
Coulthard, M. G., Flecknell, P., Orr, H., Manas, D., O’Donnell, M. (2002). Renal Scarring Caused by Vesicoureteric Reflux and Urinary Infection: A Study in Pigs. Pediatr. Nephrol. 17, 481–484. doi: 10.1007/s00467-002-0878-2
Cross, W. R., Eardley, I., Leese, H. J., Southgate, J. (2005). A Biomimetic Tissue From Cultured Normal Human Urothelial Cells: Analysis of Physiological Function. Am. J. Physiol. Renal Physiol. 289, F459–FF68. doi: 10.1152/ajprenal.00040.2005
Croxford, A. L., Kurschus, F. C., Waisman, A. (2011). Mouse Models for Multiple Sclerosis: Historical Facts and Future Implications. Biochim. Biophys. Acta (BBA) Mol. Basis Dis. 1812, 177–183. doi: 10.1016/j.bbadis.2010.06.010
Daher, A., de Boer, W. I., Le Frère-Belda, M.-A., Kheuang, L., Abbou, C. C., Radvanyi, F, et al. (2004). Growth, Differentiation and Senescence of Normal Human Urothelium in an Organ-Like Culture. Eur. Urol. 45, 799–805. doi: 10.1016/j.eururo.2004.01.002
Dawson, H. D., Smith, A. D., Chen, C., Urban, J. F. (2017). An in-Depth Comparison of the Porcine, Murine and Human Inflammasomes; Lessons From the Porcine Genome and Transcriptome. Veterinary Microbiol. 202, 2–15. doi: 10.1016/j.vetmic.2016.05.013
Deinhardt-Emmer, S., Rennert, K., Schicke, E., Cseresnyés, Z, Windolph, M., Nietzsche, S., et al. (2020). Co-Infection With Staphylococcus Aureus After Primary Influenza Virus Infection Leads to Damage of the Endothelium in a Human Alveolus-on-a-Chip Model. Biofabrication 12, 025012. doi: 10.1088/1758-5090/ab7073
De Nisco, N. J., Neugent, M., Mull, J., Chen, L., Kuprasertkul, A., de Souza Santos, M., et al. (2019). Direct Detection of Tissue-Resident Bacteria and Chronic Inflammation in the Bladder Wall of Postmenopausal Women With Recurrent Urinary Tract Infection. J. Mol. Biol. 431, 4368–4379. doi: 10.1016/j.jmb.2019.04.008
de Vos, M. G.J., Zagorski, M., McNally, A., Bollenbach, T. (2017). Interaction Networks, Ecological Stability, and Collective Antibiotic Tolerance in Polymicrobial Infections. Proc. Natl. Acad. Sci. 114, 10666. doi: 10.1073/pnas.1713372114
Dörrenhaus, A., Müller, J. I. F., Golka, K., Jedrusik, P., Schulze, H., Föllmann, W. (2000). Cultures of exfoliated epithelial cells from different locations of the human urinary tract and the renal tubular system. Arch. Toxicol. 74, 618–626. doi: 10.1007/s002040000173
Douville, N. J., Zamankhan, P., Tung, Y.-C., Li, R., Vaughan, B. L., Tai, C.-F., et al. (2011). Combination of Fluid and Solid Mechanical Stresses Contribute to Cell Death and Detachment in a Microfluidic Alveolar Model. Lab. Chip 11, 609–619. doi: 10.1039/C0LC00251H
Eaton, A. F., Clayton, D. R., Ruiz, W. G., Griffiths, S. E., Rubio, M. E., Apodaca, G. (2019). Expansion and Contraction of the Umbrella Cell Apical Junctional Ring in Response to Bladder Filling and Voiding. Mol. Biol. Cell 30, 2037–2052. doi: 10.1091/mbc.E19-02-0115
Ehlert, F. J., Griffin, M. T., Abe, D. M., Vo, T. H., Taketo, M. M., Manabe, T., et al. (2005). The M2 Muscarinic Receptor Mediates Contraction Through Indirect Mechanisms in Mouse Urinary Bladder. J. Pharmacol. Exp. Ther. 313, 368. doi: 10.1124/jpet.104.077909
EMBL-EBI, Ontology Lookup Service (2021) ‘Akr/J’. Available at: https://www.ebi.ac.uk/ols/ontologies/efo/terms?short_form=EFO_0007726.
Engelsöy, U., Rangel, I., Demirel, I. (2019). Impact of Proinflammatory Cytokines on the Virulence of Uropathogenic Escherichia Coli. Front. Microbiol. 10, 1051.
Esch, M. B., Prot, J.-M., Wang, Y. I., Miller, P., Llamas-Vidales, J. R., Naughton, B. A., et al. (2015). Multi-Cellular 3D Human Primary Liver Cell Culture Elevates Metabolic Activity Under Fluidic Flow. Lab. Chip 15, 2269–2277. doi: 10.1039/C5LC00237K
Estes, J. D., Wong, S. W., Brenchley, J. M. (2018). Nonhuman Primate Models of Human Viral Infections. Nat. Rev. Immunol. 18, 390–404. doi: 10.1038/s41577-018-0005-7
Eto, D. S., Sundsbak, J. L., Mulvey, M. A. (2006). Actin-Gated Intracellular Growth and Resurgence of Uropathogenic Escherichia Coli. Cell. Microbiol. 8, 704–717. doi: 10.1111/j.1462-5822.2006.00691.x
Fairley, K. F., Birch, D. F. (1983). Unconventional Bacteria in Urinary Tract Disease: Gardnerella Vaginalis. Kidney Int. 23, 862–865. doi: 10.1038/ki.1983.107
Fang, Y, Eglen, R. M. (2017). Three-Dimensional Cell Cultures in Drug Discovery and Development. SLAS Discovery Advancing Life Sci. R D 22, 456–472. doi: 10.1177/1087057117696795
Feenstra, T., Thøgersen, M. S., Wieser, E., Peschel, A., Ball, M. J., Brandes, R., et al. (2017). Adhesion of Escherichia Coli Under Flow Conditions Reveals Potential Novel Effects of FimH Mutations. Eur. J. Clin. Microbiol. Infect. Dis. 36, 467–478. doi: 10.1007/s10096-016-2820-8
Feil, G., Maurer, S., Nagele, U., Krug, J., Bock, C., Sievert, K.-D., et al. (2008). Immunoreactivity of p63 in Monolayered and In Vitro Stratified Human Urothelial Cell Cultures Compared With Native Urothelial Tissue. Eur. Urol. 53, 1066–1072. doi: 10.1016/j.eururo.2007.10.026
Ferrières, L., Hancock, V., Klemm, P. (2007). Specific Selection for Virulent Urinary Tract Infectious Escherichia Coli Strains During Catheter-Associated Biofilm Formation. FEMS Immunol. Med. Microbiol. 51, 212–219. doi: 10.1111/j.1574-695X.2007.00296.x
Flieger, A., Golka, K., Schulze, H., Föllmann, W. (2008). Primary Cultures of Human Urothelial Cells for Genotoxicity Testing. J. Toxicol. Environ. Health Part A 71, 930–935. doi: 10.1080/15287390801988939
Flores-Mireles, A. L., Pinkner, J. S., Caparon, M. G., Hultgren, S. J. (2014). Ebpa Vaccine Antibodies Block Binding of Enterococcus Faecalis to Fibrinogen to Prevent Catheter-Associated Bladder Infection in Mice. Sci. Trans. Med. 6, 254ra127. doi: 10.1126/scitranslmed.3009384
Foxman, B. (2003). Epidemiology of Urinary Tract Infections: Incidence, Morbidity, and Economic Costs. Disease-a-Month 49, 53–70. doi: 10.1067/mda.2003.7
Foxman, B. (2010). The Epidemiology of Urinary Tract Infection. Nat. Rev. Urol. 7, 653–660. doi: 10.1038/nrurol.2010.190
Foxman, B., Gillespie, B., Koopman, J., Zhang, L., Palin, K., Tallman, P., et al. (2000). Risk Factors for Second Urinary Tract Infection Among College Women. Am. J. Epidemiol. 151, 1194–1205. doi: 10.1093/oxfordjournals.aje.a010170
Frick-Cheng, A. E., Sintsova, A., Smith, S. N., Krauthammer, M., Eaton, K. A., Mobley, H. L.T. (2020). The Gene Expression Profile of Uropathogenic Escherichia Coli in Women With Uncomplicated Urinary Tract Infections Is Recapitulated in the Mouse Model. mBio 11, e01412–e01420. doi: 10.1128/mBio.01412-20
Fritsche, C. S., Simsch, O., Weinberg, E. J., Orrick, B., Stamm, C., Kaazempur-Mofrad, M. R., et al. (2009). Pulmonary Tissue Engineering Using Dual-Compartment Polymer Scaffolds With Integrated Vascular Tree. Int. J. Artif. Organs 32, 701–710. doi: 10.1177/039139880903201001
Gao, X., Wei, T., Liao, B., Ai, J., Zhou, L., Gong, L., et al. (2018). Physiological Stretch Induced Proliferation of Human Urothelial Cells Via Integrin α6-FAK Signaling Pathway. Neurourol. Urodynamics 37, 2114–2120. doi: 10.1002/nau.23572
Garofalo, C. K., Hooton, T. M., Martin, S. M., Stamm, W. E., Palermo, J. J., Gordon, J. I., et al. (2007). Escherichia Coli From Urine of Female Patients With Urinary Tract Infections is Competent for Intracellular Bacterial Community Formation. Infection Immun. 75, 52. doi: 10.1128/IAI.01123-06
Garthwaite, M., Hinley, J., Cross, W., Warwick, R. M., Ambrose, A., Hardaker, H., et al. (2014). Use of Donor Bladder Tissues for In Vitro Research. BJU Int. 113, 160–166. doi: 10.1111/bju.12285
Gaston, J. R., Andersen, M. J., Johnson, A. O., Bair, K. L., Sullivan, C. M., Guterman, L. B., et al. (2020). Enterococcus Faecalis Polymicrobial Interactions Facilitate Biofilm Formation, Antibiotic Recalcitrance, and Persistent Colonization of the Catheterized Urinary Tract. Pathogens 9, 835. doi: 10.3390/pathogens9100835
Georgopoulos, N. T., Kirkwood, L. A., Varley, C. L., MacLaine, N. J., Aziz, N., Southgate, J. (2011). Immortalisation of Normal Human Urothelial Cells Compromises Differentiation Capacity. Eur. Urol. 60, 141–149. doi: 10.1016/j.eururo.2011.02.022
Gevaert, T., Neuhaus, J., Vanstreels, E., Daelemans, D., Everaerts, W., Van Der Aa, F., et al. (2017). Comparative Study of the Organisation and Phenotypes of Bladder Interstitial Cells in Human, Mouse and Rat. Cell Tissue Res. 370, 403–416. doi: 10.1007/s00441-017-2694-9
Gilbert, N. M., O’Brien, V. P., Lewis, A. L. (2017). Transient Microbiota Exposures Activate Dormant Escherichia Coli Infection in the Bladder and Drive Severe Outcomes of Recurrent Disease. PloS Pathog. 13, e1006238. doi: 10.1371/journal.ppat.1006238
Giridharan, G. A., Nguyen, M.-D., Estrada, R., Parichehreh, V., Hamid, T., Ameen Ismahil, M., et al. (2010). Microfluidic Cardiac Cell Culture Model (μcccm). Analytical Chem. 82, 7581–7587. doi: 10.1021/ac1012893
Gloeckner, D. C., Sacks, M. S., Fraser, M. O., Somogyi, G. T., de Groat, W. C., Chancellor, M. B. (2002). Passive Biaxial Mechanical Properties of the Rat Bladder Wall After Spinal Cord Injury. J. Urol. 167, 2247–2252. doi: 10.1016/S0022-5347(05)65137-3
González, MJ, Zunino, P., Scavone, P., Robino, L. (2020). Selection of Effective Antibiotics for Uropathogenic Escherichia Coli Intracellular Bacteria Reduction. Front. Cell. Infection Microbiol. 10, 542755.
Graham, A. L. (2021). Naturalizing Mouse Models for Immunology. Nat. Immunol. 22, 111–117. doi: 10.1038/s41590-020-00857-2
Grassart, A., Malardé, V, Gobaa, S., Sartori-Rupp, A., Kerns, J., Karalis, K., et al. (2019). Bioengineered Human Organ-on-Chip Reveals Intestinal Microenvironment and Mechanical Forces Impacting Shigella Infection. Cell Host Microbe 26, 565. doi: 10.1016/j.chom.2019.09.007
Grimm, D., Staeheli, P., Hufbauer, M., Koerner, I., Martínez-Sobrido, L., Solórzano, A., et al. (2007). Replication Fitness Determines High Virulence of Influenza A Virus in Mice Carrying Functional Mx1 Resistance Gene. Proc. Natl. Acad. Sci. 104, 6806. doi: 10.1073/pnas.0701849104
Grosberg, A., Nesmith, A. P., Goss, J. A., Brigham, M. D., McCain, M. L., Parker, K. K. (2012). Muscle on a Chip: In Vitro Contractility Assays for Smooth and Striated Muscle. J. Pharmacol. Toxicol. Methods 65, 126–135. doi: 10.1016/j.vascn.2012.04.001
Gunther, N. W., Lockatell, V., Johnson, D. E., Mobley, H. L.T. (2001). In Vivo Dynamics of Type 1 Fimbria Regulation in Uropathogenic Escherichia Coli During Experimental Urinary Tract Infection. Infection Immun. 69, 2838. doi: 10.1128/IAI.69.5.2838-2846.2001
Guze, P. A., Kalmanson, G. M., Ishida, K., Guze, L. B. (1987). Strain-Dependent Difference in Susceptibility of Mice to Experimental Ascending Pyelonephritis. J. Infect. Dis. 156, 523–525. doi: 10.1093/infdis/156.3.523
Guze, P. A., Karavodin, L. M., Bonavida, B., Kalmanson, G. M., Ishida, K., Targan, S., et al. (1985). Strain-Dependent Differences in Susceptibility of Mice to Experimental Pyelonephritis. J. Infect. Dis. 152, 416–419. doi: 10.1093/infdis/152.2.416
Hagan, E. C., Lloyd, A. L., Rasko, D. A., Faerber, G. J., Mobley, H. L.T. (2010). Escherichia Coli Global Gene Expression in Urine From Women With Urinary Tract Infection. PloS Pathog. 6, e1001187. doi: 10.1371/journal.ppat.1001187
Hagberg, L., Engberg, I., Freter, R., Lam, J., Olling, S., Svanborg Edén, C. (1983). Ascending, Unobstructed Urinary Tract Infection in Mice Caused by Pyelonephritogenic Escherichia Coli of Human Origin. Infection Immun. 40, 273–283. doi: 10.1128/IAI.40.1.273-283.1983
Hagberg, L., Hull, R., Hull, S., Falkow, S., Freter, R., Svanborg Edén, C. (1983). Contribution of Adhesion to Bacterial Persistence in the Mouse Urinary Tract. Infection Immun. 40, 265–272. doi: 10.1128/IAI.40.1.265-272.1983
Hagberg, L., Hull, R., Hull, S., McGhee, J. R., Michalek, S. M., Svanborg Edén, C. (1984). Difference in Susceptibility to Gram-Negative Urinary Tract Infection Between C3H/HeJ and C3H/HeN Mice. Infection Immun. 46, 839. doi: 10.1128/IAI.46.3.839-844.1984
Hannan, T. J., Roberts, P. L., Riehl, T. E., van der Post, S., Binkley, J. M., Schwartz, D. J., et al. (2014). Inhibition of Cyclooxygenase-2 Prevents Chronic and Recurrent Cystitis. EBioMedicine 1, 46–57. doi: 10.1016/j.ebiom.2014.10.011
Hashimoto, M., Ma, Y.-F., Wang, S.-T., Chen, C.-S., Teng, C.-H. (2021). Iron Acquisition of Urinary Tract Infection Escherichia Coli Involves Pathogenicity in Caenorhabditis Elegans. Microorganisms 9, 310. doi: 10.3390/microorganisms9020310
Hatai, H., Lepelley, A., Zeng, W., Hayden, M. S., Ghosh, S. (2016). Toll-Like Receptor 11 (Tlr11) Interacts With Flagellin and Profilin Through Disparate Mechanisms. PloS One 11, e0148987–e87. doi: 10.1371/journal.pone.0148987
Henry, O. Y.F., Villenave, R., Cronce, M. J., Leineweber, W. D., Benz, M. A., Ingber, D. E. (2017). Organs-on-Chips With Integrated Electrodes for Trans-Epithelial Electrical Resistance (TEER) Measurements of Human Epithelial Barrier Function. Lab. Chip 17, 2264–2271. doi: 10.1039/C7LC00155J
Herati, R. S., Wherry, E. J. (2018). “What Is the Predictive Value of Animal Models for Vaccine Efficacy in Humans? Consideration of Strategies to Improve the Value of Animal Models,” in Cold Spring Harbor Perspectives in Biology 10 (4), a031583. doi: 10.1101/cshperspect.a031583.
Hilt, E. E., McKinley, K., Pearce, M. M., Rosenfeld, A. B., Zilliox, M. J., Mueller, E. R., et al. (2014). Urine Is Not Sterile: Use of Enhanced Urine Culture Techniques To Detect Resident Bacterial Flora in the Adult Female Bladder. J. Clin. Microbiol. 52, 871. doi: 10.1128/JCM.02876-13
Homan, K. A., Gupta, N., Kroll, K. T., Kolesky, D. B., Skylar-Scott, M., Miyoshi, T., et al. (2019). Flow-Enhanced Vascularization and Maturation of Kidney Organoids In Vitro. Nat. Methods 16, 255–262. doi: 10.1038/s41592-019-0325-y
Hopkins, W. J., Gendron-Fitzpatrick, A., Balish, E., Uehling, D. T. (1998). Time Course and Host Responses to Escherichia Coli Urinary Tract Infection in Genetically Distinct Mouse Strains. Infection Immun. 66, 2798–2802. doi: 10.1128/IAI.66.6.2798-2802.1998
Hopkins, W., Gendron-Fitzpatrick, A., McCarthy, D. O., Haine, J. E., Uehling, D. T. (1996). Lipopolysaccharide-Responder and Nonresponder C3H Mouse Strains are Equally Susceptible to an Induced Escherichia Coli Urinary Tract Infection. Infection Immun. 64, 1369. doi: 10.1128/IAI.64.4.1369-1372.1996
Horsley, H., Dharmasena, D., Malone-Lee, J., Rohn, J. L. (2018). A Urine-Dependent Human Urothelial Organoid Offers a Potential Alternative to Rodent Models of Infection. Sci. Rep. 8, 1238. doi: 10.1038/s41598-018-19690-7
Horsley, H., Malone-Lee, J., Holland, D., Tuz, M., Hibbert, A., Kelsey, M., et al. (2013). Enterococcus Faecalis Subverts and Invades the Host Urothelium in Patients With Chronic Urinary Tract Infection. PloS One 8, e83637. doi: 10.1371/journal.pone.0083637
Ho, H. J., Tan, M. X., Chen, M. I., Tan, T. Y., Koo, S. H., Koong, A. Y.L., et al. (2019). Interaction Between Antibiotic Resistance, Resistance Genes, and Treatment Response for Urinary Tract Infections in Primary Care. J. Clin. Microbiol. 57, e00143–e00119. doi: 10.1128/JCM.00143-19
Huh, D., Fujioka, H., Tung, Y.-C., Futai, N., Paine, R., Grotberg, J. B., et al. (2007). Acoustically Detectable Cellular-Level Lung Injury Induced by Fluid Mechanical Stresses in Microfluidic Airway Systems. Proc. Natl. Acad. Sci. 104, 18886. doi: 10.1073/pnas.0610868104
Huh, D., Matthews, B. D., Mammoto, A., Montoya-Zavala, M, Yuan Hsin, H., Ingber, D. E. (2010). Reconstituting Organ-Level Lung Functions on a Chip. Science 328, 1662. doi: 10.1126/science.1188302
Hunstad, D. A., Justice, S. S. (2010). Intracellular Lifestyles and Immune Evasion Strategies of Uropathogenic Escherichia Coli. Annu. Rev. Microbiol. 64, 203–221. doi: 10.1146/annurev.micro.112408.134258
Hutton, K. A. R., Trejdosiewicz, L. K., Thomas, D. F. M., Southgate, J. (1993). Urothelial Tissue Culture for Bladder Reconstruction: An Experimental Study. J. Urol. 150, 721–725. doi: 10.1016/S0022-5347(17)35597-0
Hvidberg, H., Struve, C., Krogfelt, K. A., Christensen, N., Rasmussen, S. N., Frimodt-Møller, N. (2000). Development of a Long-Term Ascending Urinary Tract Infection Mouse Model for Antibiotic Treatment Studies. Antimicrobial Agents Chemother. 44, 156–163. doi: 10.1128/AAC.44.1.156-163.2000
Hynes, R. O. (2009). The Extracellular Matrix: Not Just Pretty Fibrils. Science 326, 1216. doi: 10.1126/science.1176009
Ingber, D. E. (2020). Is it Time for Reviewer 3 to Request Human Organ Chip Experiments Instead of Animal Validation Studies? Advanced Sci. 7, 2002030. doi: 10.1002/advs.202002030
Iosifidis, G., Duggin, I. G. (2020). Distinct Morphological Fates of Uropathogenic Escherichia Coli Intracellular Bacterial Communities: Dependency on Urine Composition and pH. Infection Immun. 88, e00884–e00819. doi: 10.1128/IAI.00884-19
Ito, H., Pickering, A., Igawa, Y., Kanai, A., Fry, C., Drake, M. (2017). Muro-Neuro-Urodynamics ; a Review of the Functional Assessment of Mouse Lower Urinary Tract Function. Front. Physiol. 8, 49. doi: 10.3389/fphys.2017.00049
Ivanova, K., Fernandes, M. M., Mendoza, E., Tzanov, T. (2015). Enzyme Multilayer Coatings Inhibit Pseudomonas Aeruginosa Biofilm Formation on Urinary Catheters. Appl. Microbiol. Biotechnol. 99, 4373–4385. doi: 10.1007/s00253-015-6378-7
Jacobsen, S. M., Shirtliff, M. E. (2011). Proteus Mirabilis Biofilms and Catheter-Associated Urinary Tract Infections. Virulence 2, 460–465. doi: 10.4161/viru.2.5.17783
Jain, A., Barrile, R., van der Meer, A. D., Mammoto, A., Mammoto, T., De Ceunynck, K., et al. (2018). Primary Human Lung Alveolus-on-a-chip Model of Intravascular Thrombosis for Assessment of Therapeutics. Clin. Pharmacol. Ther. 103, 332–340. doi: 10.1002/cpt.742
Jain, S., Chen, J., Nicolae, A., Wang, H., Shin, D.-M., Adkins, E. B., et al. (2015). Il-21–Driven Neoplasms in SJL Mice Mimic Some Key Features of Human Angioimmunoblastic T-Cell Lymphoma. Am. J. Pathol. 185, 3102–3114. doi: 10.1016/j.ajpath.2015.07.021
Jalili-Firoozinezhad, S., Gazzaniga, F. S., Calamari, E. L., Camacho, D. M., Fadel, C. W., Bein, A., et al. (2019). A Complex Human Gut Microbiome Cultured in an Anaerobic Intestine-on-a-Chip. Nat. Biomed. Eng. 3, 520–531. doi: 10.1038/s41551-019-0397-0
Jang, K.-J., Mehr, A. P., Hamilton, G. A., McPartlin, L. A., Chung, S., Suh, K.-Y., et al. (2013). Human Kidney Proximal Tubule-on-a-Chip for Drug Transport and Nephrotoxicity Assessment. Integr. Biol. 5, 1119–1129. doi: 10.1039/c3ib40049b
Jang, K.-J., Suh, K.-Y. (2010). A Multi-Layer Microfluidic Device for Efficient Culture and Analysis of Renal Tubular Cells. Lab. Chip 10, 36–42. doi: 10.1039/B907515A
Janmey, P. A., Fletcher, D. A., Reinhart-King, C. A. (2020). Stiffness Sensing by Cells. Physiol. Rev. 100, 695–724. doi: 10.1152/physrev.00013.2019
Jansen, A. M., Lockatell, V., Johnson, D. E., Mobley, H. L.T. (2004). Mannose-Resistant Proteus-Like Fimbriae Are Produced by Most Proteus Mirabilis Strains Infecting the Urinary Tract, Dictate the In Vivo Localization of Bacteria, and Contribute to Biofilm Formation. Infection Immun. 72, 7294. doi: 10.1128/IAI.72.12.7294-7305.2004
Janssen, D. A. W., Schalken, J. A., Heesakkers, J. P. F. A. (2017). Urothelium Update: How the Bladder Mucosa Measures Bladder Filling. Acta Physiol. 220, 201–217. doi: 10.1111/apha.12824
Janssen, D. A. W., van Wijk, X. M. R., Jansen, K. C. F. J., van Kuppevelt, T. H., Heesakkers, J. P. F. A., Schalken, J. A. (2013). The Distribution and Function of Chondroitin Sulfate and Other Sulfated Glycosaminoglycans in the Human Bladder and Their Contribution to the Protective Bladder Barrier. J. Urol. 189, 336–342. doi: 10.1016/j.juro.2012.09.022
Jin, Q., Zhang, X., Li, X., Wang, J. (2010). Dynamics Analysis of Bladder-Urethra System Based on CFD. Front. Mechanical Eng. China 5, 336–340. doi: 10.1007/s11465-010-0027-8
Jones-Freeman, B., Chonwerawong, M., Marcelino, V. R., Deshpande, A. V., Forster, S. C., Starkey, M. R. (2021). The Microbiome and Host Mucosal Interactions in Urinary Tract Diseases. Mucosal Immunol. doi: 10.1038/s41385-020-00372-5
Jones, B. D., Lockatell, C. V., Johnson, D. E., Warren, J. W., Mobley, H. L. (1990). Construction of a Urease-Negative Mutant of Proteus Mirabilis: Analysis of Virulence in a Mouse Model of Ascending Urinary Tract Infection. Infection Immun. 58, 1120–1123. doi: 10.1128/IAI.58.4.1120-1123.1990
Jost, S. P., Gosling, J. A., Dixon, J. S. (1989). The Morphology of Normal Human Bladder Urothelium. J. Anat. 167, 103–115.
Justice, S. S., Hung, C., Theriot, J. A., Fletcher, D. A., Anderson, G. G., Footer, M. J., et al. (2004). Differentiation and Developmental Pathways of Uropathogenic Escherichia Coli in Urinary Tract Pathogenesis. Proc. Natl. Acad. Sci. U. S. A. 101, 1333. doi: 10.1073/pnas.0308125100
Justice, S. S., Hunstad, D. A., Seed, P. C., Hultgren, S. J. (2006). Filamentation by Escherichia Coli Subverts Innate Defenses During Urinary Tract Infection. Proc. Natl. Acad. Sci. 103, 19884. doi: 10.1073/pnas.0606329104
Kamei, J., Ito, H., Aizawa, N., Hotta, H., Kojima, T., Fujita, Y., et al. (2018). Age-Related Changes in Function and Gene Expression of the Male and Female Mouse Bladder. Sci. Rep. 8, 2089. doi: 10.1038/s41598-018-20406-0
Kane, B. J., Zinner, M. J., Yarmush, M. L., Toner, M. (2006). Liver-Specific Functional Studies in a Microfluidic Array of Primary Mammalian Hepatocytes. Analytical Chem. 78, 4291–4298. doi: 10.1021/ac051856v
Kang, Y. B., Sodunke, T. R., Lamontagne, J., Cirillo, J., Rajiv, C., Bouchard, M. J., et al. (2015). Liver Sinusoid on a Chip: Long-term Layered Co-Culture of Primary Rat Hepatocytes and Endothelial Cells in Microfluidic Platforms. Biotechnol. Bioengineer. 112, 2571–2582. doi: 10.1002/bit.25659
Karoly, E., Fekete, A., Banki, N. F., Szebeni, B., Vannay, A., Szabo, A. J., et al. (2007). Heat Shock Protein 72 (Hspa1b) Gene Polymorphism and Toll-Like Receptor (Tlr) 4 Mutation are Associated With Increased Risk of Urinary Tract Infection in Children. Pediatr. Res. 61, 371–374. doi: 10.1203/pdr.0b013e318030d1f4
Kasendra, M., Tovaglieri, A., Sontheimer-Phelps, A., Jalili-Firoozinezhad, S., Bein, A., Chalkiadaki, A., et al. (2018). Development of a Primary Human Small Intestine-on-a-Chip Using Biopsy-Derived Organoids. Sci. Rep. 8, 2871. doi: 10.1038/s41598-018-21201-7
Keane, W. F., Freedman, L. R. (1967). Experimental Pyelonephritis. XIV. Pyelonephritis in Normal Mice Produced by Inoculation of E. Coli Into the Bladder Lumen During Water Diuresis. Yale J. Biol. Med. 40, 231–237.
Kelmenson, P. (2016). “There IS No SUCH Thing as A C57bl/6 Mouse!,” in The Jackson Laboratory https://www.jax.org/news-and-insights/jax-blog/2016/june/there-is-no-such-thing-as-a-b6-mouse# [Accessed May 12, 2021].
Kennedy, E. A., King, K. Y., Baldridge, M. T. (2018). Mouse Microbiota Models: Comparing Germ-Free Mice and Antibiotics Treatment as Tools for Modifying Gut Bacteria. Front. Physiol. 9, 1534. doi: 10.3389/fphys.2018.01534
Keyes, E. L. (1894). - Nephritis in Its Surgical Aspects.1: Part I.–Introductory. Part Ii.–Practical Application of This Resume. Part Iii.–the Bacteriological Study. Report on a Series of Experiments to Determine the Value of Certain Solutions as Germicides, Made for Dr. E. L. Keyes in October and Novembeby Edward K. Dunham. Observations on Samples of Glycerin and Vaseline. Bibliography. Am. J. Med. Sci. (1827-1924) 107, – 613.
Khanal, G., Chung, K., Solis-Wever, X., Johnson, B., Pappas, D. (2011). Ischemia/Reperfusion Injury of Primary Porcine Cardiomyocytes in a Low-Shear Microfluidic Culture and Analysis Device. Analyst 136, 3519–3526. doi: 10.1039/c0an00845a
Khandelwal, P., Abraham, S. N., Apodaca, G. (2009). Cell Biology and Physiology of the Uroepithelium. Am. J. Physiol. Renal Physiol. 297, F1477–FF501. doi: 10.1152/ajprenal.00327.2009
Khasriya, R., Sathiananthamoorthy, S., Ismail, S., Kelsey, M., Wilson, M., Rohn, J. L., et al. (2013). Spectrum of Bacterial Colonization Associated With Urothelial Cells From Patients With Chronic Lower Urinary Tract Symptoms. J. Clin. Microbiol. 51, 2054. doi: 10.1128/JCM.03314-12
Kim, E., Choi, S., Kang, B., Kong, J., Kim, Y., Yoon, W. H., et al. (2020). Creation of Bladder Assembloids Mimicking Tissue Regeneration and Cancer. Nature 588, 664–669. doi: 10.1038/s41586-020-3034-x
Kim, H. J., Huh, D., Hamilton, G., Ingber, D. E. (2012). Human Gut-on-a-Chip Inhabited by Microbial Flora That Experiences Intestinal Peristalsis-Like Motions and Flow. Lab. Chip 12, 2165–2174. doi: 10.1039/c2lc40074j
Kim, H. J., Ingber, D. E. (2013). Gut-on-a-Chip Microenvironment Induces Human Intestinal Cells to Undergo Villus Differentiation. Integr. Biol. 5, 1130–1140. doi: 10.1039/c3ib40126j
Kim, H. J., Li, H, Collins, J. J., Ingber, D. E. (2016). Contributions of Microbiome and Mechanical Deformation to Intestinal Bacterial Overgrowth and Inflammation in a Human Gut-on-a-Chip. Proc. Natl. Acad. Sci. 113, E7. doi: 10.1073/pnas.1522193112
Klein, K., Palarasah, Y., Kolmos, H. J, Møller-Jensen, J., Andersen, T. E. (2015). Quantification of Filamentation by Uropathogenic Escherichia Coli During Experimental Bladder Cell Infection by Using Semi-Automated Image Analysis. J. Microbiol. Methods 109, 110–116. doi: 10.1016/j.mimet.2014.12.017
Klumpp, D. J., Rycyk, M. T., Chen, M. C., Thumbikat, P., Sengupta, S., Schaeffer, A. J. (2006). Uropathogenic Escherichia Coli Induces Extrinsic and Intrinsic Cascades To Initiate Urothelial Apoptosis. Infection Immun. 74, 5106. doi: 10.1128/IAI.00376-06
Klumpp, D. J., Weiser, A. C., Sengupta, S., Forrestal, S. G., Batler, R. A., Schaeffer, A. J. (2001). Uropathogenic Escherichia Coli Potentiates Type 1 Pilus-Induced Apoptosis by Suppressing NF-Kappab. Infection Immun. 69, 6689–6695. doi: 10.1128/IAI.69.11.6689-6695.2001
Knowles, M. A., Finesilver, A., Harvey, A. E., Berry, R. J., Hicks, R. M. (1983). Long-Term Organ Culture of Normal Human Bladder. Cancer Res. 43, 374.
Komesu, Y. M., Dinwiddie, D. L., Richter, H. E., Lukacz, E. S., Sung, V. W., Siddiqui, N. Y., et al. (2020). Defining the Relationship Between Vaginal and Urinary Microbiomes. Am. J. Obstetr. Gynecol 222, 154.e1–54.e10. doi: 10.1016/j.ajog.2019.08.011
Kreft, M. E., Hudoklin, S., Jezernik, K., Romih, R. (2010). Formation and Maintenance of Blood–Urine Barrier in Urothelium. Protoplasma 246, 3–14. doi: 10.1007/s00709-010-0112-1
Kussell, E., Kishony, R., Balaban, N. Q., Leibler, S. (2005). Bacterial Persistence: A Model of Survival in Changing Environments. Genetics 169, 1807–1814. doi: 10.1534/genetics.104.035352
Kutscher, C. L., Miller, D. G. (1974). Age-Dependent Polydipsia in the SWR/J Mouse. Physiol. Behav. 13, 71–79. doi: 10.1016/0031-9384(74)90308-4
Kutscher, C. L., Miller, M., Schmalbach, N. L. (1975). Renal Deficiency Associated With Diabetes Insipidus in the SWR/J Mouse. Physiol. Behav. 14, 815–818. doi: 10.1016/0031-9384(75)90075-X
Lacerda Mariano, L., Ingersoll, M. A. (2020). The Immune Response to Infection in the Bladder. Nat. Rev. Urol. 17, 439–458. doi: 10.1038/s41585-020-0350-8
Laguna, P., Smedts, F., Nordling, J, Horn, T., Bouchelouche, K., Hopman, A., et al. (2006). Keratin Expression Profiling of Transitional Epithelium in the Painful Bladder Syndrome/Interstitial Cystitis. Am. J. Clin. Pathol. 125, 105–110. doi: 10.1309/W342BWMDMDDBCTVH
Lam, M. H., Birch, D. F., Fairley, K. F. (1988). Prevalence of Gardnerella Vaginalis in the Urinary Tract. J. Clin. Microbiol. 26, 1130. doi: 10.1128/JCM.26.6.1130-1133.1988
Langermann, S., Palaszynski, S., Barnhart, M., Auguste, G., Pinkner, J. S., Burlein, J., et al. (1997). Prevention of Mucosal Escherichia Coli Infection by FimH-Adhesin-Based Systemic Vaccination. Science 276, 607. doi: 10.1126/science.276.5312.607
Lang, R., Liu, G., Shi, Y., Bharadwaj, S., Leng, X., Zhou, X., et al. (2013). Self-Renewal and Differentiation Capacity of Urine-Derived Stem Cells After Urine Preservation for 24 Hours. PloS One 8, e53980. doi: 10.1371/journal.pone.0053980
Laupland, K. B., Ross, T., Pitout, J. D. D., Church, D. L., Gregson, D. B. (2007). Community-Onset Urinary Tract Infections: A Population-Based Assessment. Infection 35, 150. doi: 10.1007/s15010-007-6180-2
Lavigne, J.-P., Nicolas-Chanoine, M-H, Bourg, G, Moreau, J, Sotto, A. (2008). Virulent Synergistic Effect Between Enterococcus Faecalis and Escherichia Coli Assayed by Using the Caenorhabditis Elegans Model. PloS One 3, e3370. doi: 10.1371/journal.pone.0003370
Lee, D. W., Ha, S. K., Choi, I., Sung, J. H. (2017). 3D Gut-Liver Chip With a PK Model for Prediction of First-Pass Metabolism. Biomed. Microdevices 19, 100. doi: 10.1007/s10544-017-0242-8
Lee, P. J., Hung, P. J., Lee, L. P. (2007). An Artificial Liver Sinusoid With a Microfluidic Endothelial-Like Barrier for Primary Hepatocyte Culture. Biotechnol. Bioengineer. 97, 1340–1346. doi: 10.1002/bit.21360
Legendre, A., Baudoin, R, Alberto, G., Paullier, P., Naudot, M., Bricks, T., et al. (2013). Metabolic Characterization of Primary Rat Hepatocytes Cultivated in Parallel Microfluidic Biochips. J. Pharm. Sci. 102, 3264–3276. doi: 10.1002/jps.23466
Leibowitz, S. F., Alexander, J., Dourmashkin, J. T., Hill, J. O., Gayles, E. C., Chang, G.-Q. (2005). Phenotypic Profile of SWR/J and A/J Mice Compared to Control Strains: Possible Mechanisms Underlying Resistance to Obesity on a High-Fat Diet. Brain Res. 1047, 137–147. doi: 10.1016/j.brainres.2005.03.047
Lepper, E. H. (1921). The Production of Coliform Infection in the Urinary Tract of Rabbits. J. Pathol. Bacteriol. 24, 192–204. doi: 10.1002/path.1700240211
Levin, R. M., Wein, A. J. (1982). Response of the In Vitro Whole Bladder (Rabbit) Preparation to Autonomic Agonists. J. Urol. 128, 1087–1090. doi: 10.1016/S0022-5347(17)53350-9
Lewis, A. L., Gilbert, N. M. (2020). Roles of the Vagina and the Vaginal Microbiota in Urinary Tract Infection: Evidence From Clinical Correlations and Experimental Models. GMS Infect. Dis. 8, Doc02–Doc02. doi: 10.3205/id000046
Liao, K. S., Lehman, S. M., Tweardy, D. J., Donlan, R. M., Trautner, B. W. (2012). Bacteriophages are Synergistic With Bacterial Interference for the Prevention of Pseudomonas Aeruginosa Biofilm Formation on Urinary Catheters. J. Appl. Microbiol. 113, 1530–1539. doi: 10.1111/j.1365-2672.2012.05432.x
Li, Y., Liu, Y., Gao, Z., Zhang, L., Chen, L., Wu, Z., et al. (2021). Single-Cell Transcriptomes of Mouse Bladder Urothelium Uncover Novel Cell Type Markers and Urothelial Differentiation Characteristics. Cell Proliferation 54(4), e13007. doi: 10.1111/cpr.13007
Li, X., Pei, G., Zhang, L., Cao, Y., Wang, J., Yu, L, et al. (2019). Compounds Targeting YadC of Uropathogenic Escherichia Coli and its Host Receptor Annexin A2 Decrease Bacterial Colonization in Bladder. EBioMedicine 50, 23–33. doi: 10.1016/j.ebiom.2019.11.014
Liu, A. Y., Vêncio, R. Z.N., Page, L. S., Ho, M. E., Loprieno, M. A., True, L. D. (2012). Bladder Expression of CD Cell Surface Antigens and Cell-Type-Specific Transcriptomes. Cell Tissue Res. 348, 589–600. doi: 10.1007/s00441-012-1383-y
Losada, L., Amundsen, C. L., Ashton-Miller, J., Chai, T., Close, C., Damaser, M., et al. (2016). Expert Panel Recommendations on Lower Urinary Tract Health of Women Across Their Life Span. J. Women’s Health (2002) 25, 1086–1096. doi: 10.1089/jwh.2016.5895
Low, L. A., Mummery, C., Berridge, B. R., Austin, C. P., Tagle, D. A. (2020). Organs-on-Chips: Into the Next Decade. Nat. Rev. Drug Discov. 20, 345–361. doi: 10.1038/s41573-020-0079-3
Luna-Pineda, V. M., Moreno-Fierros, L., Cázares-Domínguez, V., Ilhuicatzi-Alvarado, D., Ochoa, S. A., Cruz-Córdova, A., et al. (2019). Curli of Uropathogenic Escherichia Coli Enhance Urinary Tract Colonization as a Fitness Factor. Front. Microbiol. 10, 2063. doi: 10.3389/fmicb.2019.02063
Magruder, M., Sholi, A. N., Gong, C., Zhang, L., Edusei, E., Huang, J., et al. (2019). Gut Uropathogen Abundance is a Risk Factor for Development of Bacteriuria and Urinary Tract Infection. Nat. Commun. 10, 5521. doi: 10.1038/s41467-019-13467-w
Mahler, G. J., Esch, M. B., Glahn, R. P., Shuler, M. L. (2009). Characterization of a Gastrointestinal Tract Microscale Cell Culture Analog Used to Predict Drug Toxicity. Biotechnol. Bioengineer. 104, 193–205. doi: 10.1002/bit.22366
Maier, S. M., Gross, J. K., Hamlin, K. L., Maier, J. L., Workman, J. L., Kim-Howard, X. R., et al. (2007). Proteinuria of Nonautoimmune Origin in Wild-type Fvb/Nj Mice. Comp. Med. 57, 255–266.
Martínez-Figueroa, C., Cortés-Sarabia, K., del Carmen Alarcón-Romero, L., Catalán-Nájera, H. G., Martínez-Alarcón, M., Vences-Velázquez, A. (2020). Observation of Intracellular Bacterial Communities in Urinary Sediment Using Brightfield Microscopy; a Case Report. BMC Urol. 20, 89. doi: 10.1186/s12894-020-00661-y
Maschmeyer, I., Lorenz, A. K., Schimek, K., Hasenberg, T., Ramme, A. P., Hübner, J., et al. (2015). A Four-Organ-Chip for Interconnected Long-Term Co-Culture of Human Intestine, Liver, Skin and Kidney Equivalents. Lab. Chip 15, 2688–2699. doi: 10.1039/C5LC00392J
Mattoo, T. K., Asmar, B. I. (2020). Annotations on Emerging Concerns About Antibiotic-Resistant Urinary Tract Infection. Pediatrics 145, e20193512. doi: 10.1542/peds.2019-3512
McCloskey, K. D. (2010). Interstitial Cells in the Urinary Bladder—Localization and Function. Neurourol. Urodynamics 29, 82–87. doi: 10.1002/nau.20739
McDermott, C., Chess-Williams, R., Mills, K. A., Kang, S. H., Farr, S. E., Grant, G. D., et al. (2013). Alterations in Acetylcholine, PGE2 and IL6 Release From Urothelial Cells Following Treatment With Pyocyanin and Lipopolysaccharide. Toxicol. Vitro 27, 1693–1698. doi: 10.1016/j.tiv.2013.04.015
Miao, Y., Bist, P., Wu, J., Zhao, Q., Li, Q.-j., Wan, Y., et al. (2017). Collaboration Between Distinct Rab Small Gtpase Trafficking Circuits Mediates Bacterial Clearance From the Bladder Epithelium. Cell Host Microbe 22, 330–42.e4. doi: 10.1016/j.chom.2017.08.002
Miao, Y., Li, G., Zhang, X., Xu, H., Abraham, S. N. (2015). ‘A TRP Channel Senses Lysosome Neutralization by Pathogens to Trigger Their Expulsion’. Cell 161, 1306–1319. doi: 10.1016/j.cell.2015.05.009
Mickiewicz, K. M., Kawai, Y., Drage, L., Gomes, M. C., Davison, F., Pickard, R., et al. (2019). Possible Role of L-form Switching in Recurrent Urinary Tract Infection. Nat. Commun. 10, 4379. doi: 10.1038/s41467-019-12359-3
Mike, L. A., Smith, S. N., Sumner, C. A., Eaton, K. A., Mobley, H. L.T. (2016). Siderophore Vaccine Conjugates Protect Against Uropathogenic Escherichia Coli Urinary Tract Infection. Proc. Natl. Acad. Sci. 113, 13468. doi: 10.1073/pnas.1606324113
Miyamoto, T., Mochizuki, T., Nakagomi, H., Kira, S., Watanabe, M., Takayama, Y., et al. (2014). Functional Role for Piezo1 in Stretch-evoked Ca2+ Influx and ATP Release in Urothelial Cell Cultures*. J. Biol. Chem. 289, 16565–16575. doi: 10.1074/jbc.M113.528638
Mochizuki, T., Sokabe, T., Araki, I., Fujishita, K., Shibasaki, K., Uchida, K., et al. (2009). The TRPV4 Cation Channel Mediates Stretch-evoked Ca2+ Influx and ATP Release in Primary Urothelial Cell Cultures. J. Biol. Chem. 284, 21257–21264. doi: 10.1074/jbc.M109.020206
Mulvey, M. A., Lopez-Boado, Y. S., Wilson, C. L., Roth, R., Parks, W. C., Heuser, J., et al. (1998). Induction and Evasion of Host Defenses by Type 1-Piliated Uropathogenic Escherichia Coli. Science 282, 1494. doi: 10.1126/science.282.5393.1494
Mulvey, M. A., Schilling, J. D., Hultgren, S. J. (2001). Establishment of a Persistent Escherichia Coli Reservoir During the Acute Phase of a Bladder Infection. Infection Immun. 69, 4572. doi: 10.1128/IAI.69.7.4572-4579.2001
Mysorekar, I. U., Hultgren, S. J. (2006). Mechanisms of Uropathogenic Escherichia Coli Persistence and Eradication From the Urinary Tract. Proc. Natl. Acad. Sci. 103, 14170. doi: 10.1073/pnas.0602136103
Mysorekar, I. U., Isaacson-Schmid, M., Walker, J. N., Mills, J. C., Hultgren, S. J. (2009). Bone Morphogenetic Protein 4 Signaling Regulates Epithelial Renewal in the Urinary Tract in Response to Uropathogenic Infection. Cell Host Microbe 5, 463–475. doi: 10.1016/j.chom.2009.04.005
Nagele, U., Maurer, S., Feil, G., Bock, C., Krug, J., Sievert, K.-D., et al. (2008). In Vitro Investigations of Tissue-Engineered Multilayered Urothelium Established From Bladder Washings. Eur. Urol. 54, 1414–1422. doi: 10.1016/j.eururo.2008.01.072
Neugent, M. L., Hulyalkar, N. V., Nguyen, V. H., Zimmern, P. E., De Nisco, N. J. (2020). Advances in Understanding the Human Urinary Microbiome and Its Potential Role in Urinary Tract Infection. mBio 11, e00218–e00220. doi: 10.1128/mBio.00218-20
Nguyen, D.-H. T., Lee, E., Alimperti, S., Norgard, R. J., Wong, A., Lee, J. J.-K., et al. (2019). A Biomimetic Pancreatic Cancer on-Chip Reveals Endothelial Ablation Via ALK7 Signaling. Sci. Adv. 5, eaav6789. doi: 10.1126/sciadv.aav6789
Nguyen, M.-D., Tinney, J. P., Ye, F., Elnakib, A. A., Yuan, F., El-Baz, A., et al. (2015). Effects of Physiologic Mechanical Stimulation on Embryonic Chick Cardiomyocytes Using a Microfluidic Cardiac Cell Culture Model. Analytical Chem. 87, 2107–2113. doi: 10.1021/ac503716z
Nielsen, T. K., Petersen, N. A., Stærk, K., Grønnemose, R. B., Palarasah, Y., Nielsen, L. F., et al. (2019). A Porcine Model for Urinary Tract Infection. Front. Microbiol. 10, 2564–2564. doi: 10.3389/fmicb.2019.02564
Noronha, R. F.X. (1977). Decreased Incidence of Renal Tumors in DMN Treated Balb/C Mice After Orchiectomy. J. Surg. Oncol. 9, 463–468. doi: 10.1002/jso.2930090508
Novik, E., Maguire, T. J., Chao, P., Cheng, K. C., Yarmush, M. L. (2010). A Microfluidic Hepatic Coculture Platform for Cell-Based Drug Metabolism Studies. Biochem. Pharmacol. 79, 1036–1044. doi: 10.1016/j.bcp.2009.11.010
O’Brien, V. P., Hannan, T. J., Nielsen, H. V., Hultgren, S. J. (2016). Drug and Vaccine Development for the Treatment and Prevention of Urinary Tract Infections. Microbiol. Spectr. 4. doi: 10.1128/microbiolspec.UTI-0013-2012
O’Brien, V. P., Hannan, T. J., Yu, L, Livny, J., Roberson, E. D.O., Schwartz, D. J., et al. (2016). A Mucosal Imprint Left by Prior Escherichia Coli Bladder Infection Sensitizes to Recurrent Disease. Nat. Microbiol. 2, 16196. doi: 10.1038/nmicrobiol.2016.196
Olsen, I. (2015). Biofilm-Specific Antibiotic Tolerance and Resistance. Eur. J. Clin. Microbiol. Infect. Dis. 34, 877–886. doi: 10.1007/s10096-015-2323-z
Olson, P. D., Hruska, K. A., Hunstad, D. A. (2016). Androgens Enhance Male Urinary Tract Infection Severity in a New Model. J. Am. Soc. Nephrol. JASN 27, 1625–1634. doi: 10.1681/ASN.2015030327
Olson, P. D., McLellan, L. K., Liu, A., Briden, K. E., Tiemann, K. M., Daugherty, A. L., et al. (2017). Renal Scar Formation and Kidney Function Following Antibiotic-Treated Murine Pyelonephritis. Dis. Models Mech. 10, 1371–1379. doi: 10.1242/dmm.030130
Ortega-Prieto, A. M., Skelton, J. K., Wai, S. N., Large, E., Lussignol, M., Vizcay-Barrena, G., et al. (2018). 3D Microfluidic Liver Cultures as a Physiological Preclinical Tool for Hepatitis B Virus Infection. Nat. Commun. 9, 682. doi: 10.1038/s41467-018-02969-8
Park, H. S., Liu, S., McDonald, J., Thakor, N., Yang, I. H. (2013). “Neuromuscular Junction in a Microfluidic Device,” in 2013 35th Annual International Conference of the IEEE Engineering in Medicine and Biology Society (Embc), 2833–2835. doi: 10.1109/EMBC.2013.6610130
Park, S.-H., Sim, W. Y., Min, B.-H., Yang, S. S., Khademhosseini, A., Kaplan, D. L. (2012). Chip-Based Comparison of the Osteogenesis of Human Bone Marrow- and Adipose Tissue-Derived Mesenchymal Stem Cells Under Mechanical Stimulation. PloS One 7, e46689. doi: 10.1371/journal.pone.0046689
Patras, K. A., Coady, A., Babu, P., Shing, S. R., Ha, A. D., Rooholfada, E., et al. (2020). Host Cathelicidin Exacerbates Group B Streptococcus Urinary Tract Infection. mSphere 5, e00932–e00919. doi: 10.1128/mSphere.00932-19
Peterson, N. C., Mahalingaiah, P. K., Fullerton, A., Piazza, M. D. (2020). Application of Microphysiological Systems in Biopharmaceutical Research and Development. Lab. Chip 20, 697–708. doi: 10.1039/C9LC00962K
Petzoldt, J. L., Leigh, I. M., Duffy, P. G., Masters, J. R. W. (1994). Culture and Characterisation of Human Urothelium In Vivo and In Vitro. Urol. Res. 22, 67–74. doi: 10.1007/BF00310994
Petzoldt, J. L., Leigh, I. M., Duffy, P. G., Sexton, C., Masters, J. R. W. (1995). Immortalisation of Human Urothelial Cells. Urol. Res. 23, 377–380. doi: 10.1007/BF00698738
Pingyu, Z., Binglei, J., Qilong, J., Tao, W, Wei, T. (2019). Cyclic Stretch Promotes Proliferation and Contraction of Human Bladder Smooth Muscle Cells by Cajal-Mediated C-Kit Expression in Interstitial Cells. Med. Sci. Monitor Int. Med. J. Exp. Clin. Res. 25, 4784–4792. doi: 10.12659/MSM.917549
Prattley, S., Geraghty, R., Moore, M., Somani, B. K. (2020). Role of Vaccines for Recurrent Urinary Tract Infections: A Systematic Review. Eur. Urol. Focus 6, 593–604. doi: 10.1016/j.euf.2019.11.002
Ragnarsdóttir, B., Fischer, H., Godaly, G., Grönberg-Hernandez, J., Gustafsson, M., Karpman, D., et al. (2008). TLR- and CXCR1-dependent Innate Immunity: Insights Into the Genetics of Urinary Tract Infections. Eur. J. Clin. Invest. 38, 12–20. doi: 10.1111/j.1365-2362.2008.02004.x
Reid, G., Charbonneau-Smith, R. N., Lam, D., Kang, Y. S., Lacerte, M., Hayes, K. C. (1992). Bacterial Biofilm Formation in the Urinary Bladder of Spinal Cord Injured Patients. Spinal Cord 30, 711–717. doi: 10.1038/sc.1992.138
Reitzer, L., Zimmern, P. (2019). Rapid Growth and Metabolism of Uropathogenic Escherichia Coli in Relation to Urine Composition. Clin. Microbiol. Rev. 33, e00101–e00119. doi: 10.1128/CMR.00101-19
Reznikoff, C. A., Johnson, M. D., Norback, D. H., Bryan, G. T. (1983). Growth and Characterization of Normal Human Urothelium In Vitro. In Vitro 19, 326–343. doi: 10.1007/BF02619511
RHEINWALD, JAMES G., O’CONNELL, THERESE M. (1985). Intermediate Filament Proteins as Distinguishing Markers of Cell Type and Differentiated State in Cultured Human Urinary Tract Epithelia. Ann. New Y Acad. Sci. 455, 259–267. doi: 10.1111/j.1749-6632.1985.tb50416.x
Robino, L., Scavone, P., Araujo, L., Algorta, G., Zunino, P., Pírez, MC., et al. (2014). Intracellular Bacteria in the Pathogenesis of Escherichia Coli Urinary Tract Infection in Children. Clin. Infect. Dis. 59, e158–ee64. doi: 10.1093/cid/ciu634
Robino, L., Scavone, P., Araujo, L., Algorta, G., Zunino, P., Vignoli, R. (2013). Detection of Intracellular Bacterial Communities in a Child With Escherichia Coli Recurrent Urinary Tract Infections. Pathog. Dis. 68, 78–81. doi: 10.1111/2049-632X.12047
Rosen, D. A., Hooton, T. M., Stamm, W. E., Humphrey, P. A., Hultgren, S. J. (2007). Detection of Intracellular Bacterial Communities in Human Urinary Tract Infection. PloS Med. 4, e329. doi: 10.1371/journal.pmed.0040329
Rosen, D. A., Pinkner, J. S., Jones, J. M., Walker, J. N., Clegg, S., Hultgren, S. J. (2008). Utilization of an Intracellular Bacterial Community Pathway in Klebsiella Pneumoniae Urinary Tract Infection and the Effects of FimK on Type 1 Pilus Expression. Infection Immun. 76, 3337. doi: 10.1128/IAI.00090-08
Rosen, D. A., Pinkner, J. S., Walker, J. N., Elam, J. S., Jones, J. M., Hultgren, S. J. (2008). Molecular Variations in Klebsiella Pneumoniae and Escherichia Coli FimH Affect Function and Pathogenesis in the Urinary Tract. Infection Immun. 76, 3346. doi: 10.1128/IAI.00340-08
Rossi, M. R., Masters, J. R., Park, S., Todd, J. H., Garrett, S. H., Sens, M. A., et al. (2001). The Immortalized UROtsa Cell Line as a Potential Cell Culture Model of Human Urothelium. Environ. Health Perspect. 109, 801–808. doi: 10.1289/ehp.01109801
Rowe, T. A., Juthani-Mehta, M. (2013). Urinary Tract Infection in Older Adults. Aging Health 9, 519–528. doi: 10.2217/ahe.13.38
Rubenwolf, P. C., Georgopoulos, N. T., Clements, L. A., Feather, S., Holland, P., Thomas, D. F.M., et al. (2009). Expression and Localisation of Aquaporin Water Channels in Human Urothelium In Situ and In Vitro. Eur. Urol. 56, 1013–1024. doi: 10.1016/j.eururo.2008.08.013
Rubenwolf, P. C., Georgopoulos, N. T., Kirkwood, L. A., Baker, S. C., Southgate, J. (2012). Aquaporin Expression Contributes to Human Transurothelial Permeability in Vitro and Is Modulated by Nacl. PloS One 7, e45339. doi: 10.1371/journal.pone.0045339
Rudick, C. N., Taylor, A. K., Yaggie, R. E., Schaeffer, A. J., Klumpp, D. J. (2014). Asymptomatic Bacteriuria Escherichia Coli Are Live Biotherapeutics for UTI. PloS One 9, e109321. doi: 10.1371/journal.pone.0109321
Saini, H., Chhibber, S., Harjai, K. (2015). Azithromycin and Ciprofloxacin: A Possible Synergistic Combination Against Pseudomonas Aeruginosa Biofilm-Associated Urinary Tract Infections. Int. J. Antimicrobial Agents 45, 359–367. doi: 10.1016/j.ijantimicag.2014.11.008
Scriven, S. D., Booth, C., Thomas, D. F. M., Trejdosiewicz, L. K., Southgate, J. (1997). ‘Reconstitution of Human Urothelium From Monolayer Cultures. J. Urol. 158, 1147–1152. doi: 10.1016/S0022-5347(01)64407-0
Selekman, R. E., Shapiro, D. J., Boscardin, J., Williams, G., Craig, J. C., Brandström, P., et al. (2018). Uropathogen Resistance and Antibiotic Prophylaxis: A Meta-Analysis. Pediatrics 142, e20180119. doi: 10.1542/peds.2018-0119
Shah, P., Fritz, J. V., Glaab, E., Desai, M. S., Greenhalgh, K., Frachet, A., et al. (2016). A Microfluidics-Based In Vitro Model of the Gastrointestinal Human–Microbe Interface. Nat. Commun. 7, 11535. doi: 10.1038/ncomms11535
Shankar, N., Lockatell, C. V., Baghdayan, A. S., Drachenberg, C., Gilmore, M. S., Johnson, D. E. (2001). Role of Enterococcus Faecalis Surface Protein Esp in the Pathogenesis of Ascending Urinary Tract Infection. Infection Immun. 69, 4366–4372. doi: 10.1128/IAI.69.7.4366-4372.2001
Sharma, K., Dhar, N., Thacker, V. V., Simonet, T. M., Signorino-Gelo, F, Knott, G., et al. (2021). Dynamic Persistence of Intracellular Bacterial Communities of Uropathogenic Escherichia Coli in a Human Bladder-Chip Model of Urinary Tract Infections. bioRxiv, 2021.01.03.424836.
Shi, M., Majumdar, D., Gao, Y., Brewer, B. M., Goodwin, C. R., McLean, J. A., et al. (2013). Glia Co-Culture With Neurons in Microfluidic Platforms Promotes the Formation and Stabilization of Synaptic Contacts’. Lab. Chip 13, 3008–3021. doi: 10.1039/c3lc50249j
Shin, W., Hinojosa, C. D., Ingber, D. E., Kim, H. J. (2019). Human Intestinal Morphogenesis Controlled by Transepithelial Morphogen Gradient and Flow-Dependent Physical Cues in a Microengineered Gut-on-a-Chip. iScience 15, 391–406. doi: 10.1016/j.isci.2019.04.037
Shin, K., Lee, J., Guo, N., Kim, J., Lim, A., Qu, L., et al. (2011). Hedgehog/Wnt Feedback Supports Regenerative Proliferation of Epithelial Stem Cells in Bladder. Nature 472, 110–114. doi: 10.1038/nature09851
Shin, M., Matsuda, K., Ishii, O., Terai, H., Kaazempur-Mofrad, M., Borenstein, J., et al. (2004). Endothelialized Networks With a Vascular Geometry in Microfabricated Poly(Dimethyl Siloxane). Biomed. Microdevices 6, 269–278. doi: 10.1023/B:BMMD.0000048559.29932.27
Shirure, V. S., George, S. C. (2017). Design Considerations to Minimize the Impact of Drug Absorption in Polymer-Based Organ-on-a-Chip Platforms. Lab. Chip 17, 681–690. doi: 10.1039/C6LC01401A
Si, L., Bai, H., Rodas, M., Cao, W., Oh, C. Y., Jiang, A., et al. (2020). Human Organ Chip-Enabled Pipeline to Rapidly Repurpose Therapeutics During Viral Pandemics. bioRxiv, 2020.04.13.039917.
Sidar, B., Jenkins, B. R., Huang, S., Spence, J. R., Walk, S. T., Wilking, J. N. (2019). Long-Term Flow Through Human Intestinal Organoids With the Gut Organoid Flow Chip (Goflowchip). Lab. Chip 19, 3552–3562. doi: 10.1039/C9LC00653B
Sillanpää, J., Nallapareddy, S. R., Singh, K. V., Prakash, V. P., Fothergill, T., Ton-That, H., et al. (2010). Characterization of the Ebpfm Pilus-Encoding Operon of Enterococcus Faecium and its Role in Biofilm Formation and Virulence in a Murine Model of Urinary Tract Infection. Virulence 1, 236–246. doi: 10.4161/viru.1.4.11966
Simon, M. M., Greenaway, S., White, J. K., Fuchs, H., Gailus-Durner, V., Wells, S., et al. (2013). A Comparative Phenotypic and Genomic Analysis of C57BL/6J and C57BL/6N Mouse Strains. Genome Biol. 14, R82. doi: 10.1186/gb-2013-14-7-r82
Sin, A., Chin, K. C., Jamil, M. F., Kostov, Y., Rao, G., Shuler, M. L. (2004). The Design and Fabrication of Three-Chamber Microscale Cell Culture Analog Devices With Integrated Dissolved Oxygen Sensors. Biotechnol. Prog. 20, 338–345. doi: 10.1021/bp034077d
Sivaraman, A., Leach, J. K., Townsend, S., Iida, T., Hogan, B. J., Stolz, D. B., et al. (2005). A Microscale in Vitro Physiological Model of the Liver: Predictive Screens for Drug Metabolism and Enzyme Induction’. Curr. Drug Metab. 6, 569–591. doi: 10.2174/138920005774832632
Smith, Y. C., Grande, K. K., Rasmussen, S. B., Brien, A. D. (2006). Novel Three-Dimensional Organoid Model for Evaluation of the Interaction of Uropathogenic Escherichia Coli With Terminally Differentiated Human Urothelial Cells. Infection Immun. 74, 750. doi: 10.1128/IAI.74.1.750-757.2006
Smith, N. J., Varley, C. L., Eardley, I., Feather, S., Trejdosiewicz, L. K., Southgate, J. (2011). Toll-Like Receptor Responses of Normal Human Urothelial Cells to Bacterial Flagellin and Lipopolysaccharide. J. Urol. 186, 1084–1092. doi: 10.1016/j.juro.2011.04.112
Snouber, L. C., Letourneur, F., Chafey, P., Broussard, C., Monge, M., Legallais, C., et al. (2012). Analysis of Transcriptomic and Proteomic Profiles Demonstrates Improved Madin–Darby Canine Kidney Cell Function in a Renal Microfluidic Biochip. Biotechnol. Prog. 28, 474–484. doi: 10.1002/btpr.743
Snyder, J. A., Haugen, B. J., Buckles, E. L., Lockatell, C. V., Johnson, D. E., Donnenberg, M. S., et al. (2004). Transcriptome of Uropathogenic Escherichia Coli During Urinary Tract Infection. Infection Immun. 72, 6373. doi: 10.1128/IAI.72.11.6373-6381.2004
Song, J., Abraham, S. N. (2008). TLR-Mediated Immune Responses in the Urinary Tract. Curr. Opin. Microbiol. 11, 66–73. doi: 10.1016/j.mib.2007.12.001
Southgate, J., Hutton, K. A. R., Thomas, D. F. M., Trejdosiewicz, L. K. (1994). Normal Human Urothelial Cells In Vitro. Proliferation Induction Stratification 71, 583–594.
Southgate, J., Varley, C. L., Garthwaite, M. A.E., Hinley, J., Marsh, F., Stahlschmidt, J., et al. (2007). Differentiation Potential of Urothelium From Patients With Benign Bladder Dysfunction. BJU Int. 99, 1506–1516. doi: 10.1111/j.1464-410X.2007.06795.x
Spaulding, C. N., Klein, R. D., Ruer, S., Kau, A. L., Schreiber, H. L., Cusumano, Z. T., et al. (2017). Selective Depletion of Uropathogenic E. Coli From the Gut by a FimH Antagonist. Nature 546, 528–532. doi: 10.1038/nature22972
Stahlhut, S. G., Struve, C., Krogfelt, K. A., Reisner, A. (2012). Biofilm Formation of Klebsiella Pneumoniae on Urethral Catheters Requires Either Type 1 or Type 3 Fimbriae. FEMS Immunol. Med. Microbiol. 65, 350–359. doi: 10.1111/j.1574-695X.2012.00965.x
Stamm, W. E., Norrby, S. R. (2001). Urinary Tract Infections: Disease Panorama and Challenges. J. Infect. Dis. 183, S1–S4. doi: 10.1086/318850
Stapleton, A. E., Au-Yeung, M., Hooton, T. M., Fredricks, D. N., Roberts, P. L., Czaja, C. A., et al. (2011). Randomized, Placebo-Controlled Phase 2 Trial of a Lactobacillus Crispatus Probiotic Given Intravaginally for Prevention of Recurrent Urinary Tract Infection. Clin. Infect. Dis. 52, 1212–1217. doi: 10.1093/cid/cir183
Sugasi, S., Lesbros, Y., Bisson, I., Zhang, Y. Y., Kucera, P., Frey, P. (2000). In VITRO Engineering OF Human STRATIFIED Urothelium: ANALYSIS of ITS Morphology AND Function. J. Urol. 164, 951–957. doi: 10.1016/S0022-5347(05)67224-2
SUN, Y. A. N., CHAI, TOBY C. (2004). Up-Regulation of P2X3 Receptor During Stretch of Bladder Urothelial Cells From Patients With Interstitial Cystitis. J. Urol. 171, 448–452. doi: 10.1097/01.ju.0000099660.46774.3c
Sun, Y. A. N., Keay, S., De Deyne, P. G., Chai, T. C. (2001). Augmented STRETCH Activated ADENOSINE Triphosphate RELEASE From BLADDER Uroepithelial CELLS in PATIENTS With INTERSTITIAL Cystitis. J. Urol. 166, 1951–1956. doi: 10.1016/S0022-5347(05)65726-6
Sunuwar, L., Yin, J., Kasendra, M., Karalis, K., Kaper, J., Fleckenstein, J., et al. (2020). Mechanical Stimuli Affect Escherichia Coli Heat-Stable Enterotoxin-Cyclic GMP Signaling in a Human Enteroid Intestine-Chip Model. Infection Immun. 88, e00866–e00819. doi: 10.1128/IAI.00866-19
Sutherland, G. R., Bain, A. D. (1972). Culture of Cells From the Urine of Newborn Children. Nature 239, 231–231. doi: 10.1038/239231a0
Suzuki, K., Koyanagi-Aoi, M., Uehara, K., Hinata, N., Fujisawa, M., Aoi, T. (2019). Directed Differentiation of Human Induced Pluripotent Stem Cells Into Mature Stratified Bladder Urothelium. Sci. Rep. 9, 10506. doi: 10.1038/s41598-019-46848-8
Svanborg Edén, C., Briles, D., Hagberg, L., McGhee, J., Michalec, S. (1984). Genetic Factors in Host Resistance to Urinary Tract Infection. Infection 12, 118–122. doi: 10.1007/BF01641692
Svanborg Edén, C., Jodal, U., Hanson, L. A., Lindberg, U., Sohl Åkerlund, A. (1976). Variable ADHERENCE to NORMAL Human URINARY-TRACT Epithelial CELLS of ESCHERICHIA Coli STRAINS Associated WITH Various FORMS of URINARY-TRACT Infection. Lancet 308, 490–492. doi: 10.1016/S0140-6736(76)90788-1
Szabados, F., Kleine, B., Anders, A., Kaase, M., Sakınç, T., Schmitz, I., et al. (2008). Staphylococcus Saprophyticus ATCC 15305 is Internalized Into Human Urinary Bladder Carcinoma Cell Line 5637. FEMS Microbiol. Lett. 285, 163–169. doi: 10.1111/j.1574-6968.2008.01218.x
Szabados, F., Mohner, A., Kleine, B., Gatermann, S. G. (2013). Staphylococcus Saprophyticus Surface-Associated Protein (Ssp) is Associated With Lifespan Reduction in Caenorhabditis Elegans. Virulence 4, 604–611. doi: 10.4161/viru.25875
Tabel, Y., Berdeli, A., Mir, S. (2007). Association of TLR2 Gene Arg753Gln Polymorphism With Urinary Tract Infection in Children. Int. J. Immunogen 34, 399–405. doi: 10.1111/j.1744-313X.2007.00709.x
Takeuchi, O., Akira, S. (2010). Pattern Recognition Receptors and Inflammation. Cell 140, 805–820. doi: 10.1016/j.cell.2010.01.022
Takeuchi, O., Hoshino, K., Kawai, T., Sanjo, H., Takada, H., Ogawa, T., et al. (1999). Differential Roles of TLR2 and TLR4 in Recognition of Gram-Negative and Gram-Positive Bacterial Cell Wall Components. Immunity 11, 443–451. doi: 10.1016/S1074-7613(00)80119-3
Tanaka, I., Nagase, K., Tanase, K., Aoki, Y., Akino, H., Yokoyama, O. (2011). Modulation of Stretch Evoked Adenosine Triphosphate Release From Bladder Epithelium by Prostaglandin E2. J. Urol. 185, 341–346. doi: 10.1016/j.juro.2010.09.042
Tan, C. K., Carey, A. J., Cui, X., Webb, R. I., Ipe, D., Crowley, M., et al. (2012). Genome-Wide Mapping of Cystitis Due to Streptococcus Agalactiae and Escherichia Coli in Mice Identifies a Unique Bladder Transcriptome That Signifies Pathogen-Specific Antimicrobial Defense Against Urinary Tract Infection. Infection Immun. 80, 3145–3160. doi: 10.1128/IAI.00023-12
Tan, K., Coppeta, J., Azizgolshani, H., Isenberg, B. C., Keegan, P. M., Cain, B. P., et al. (2020). Correction: A High-Throughput Microfluidic Microphysiological System (PREDICT-96) to Recapitulate Hepatocyte Function in Dynamic, Re-Circulating Flow Conditions. Lab. Chip 20, 3653–3653. doi: 10.1039/D0LC90069A
Tang, H., Abouleila, Y., Si, L., Ortega-Prieto, A. M., Mummery, C. L., Ingber, D. E., et al. (2020). Human Organs-on-Chips for Virology. Trends Microbiol. 28, 934–946. doi: 10.1016/j.tim.2020.06.005
Tash, J. A., David, S. G., Vaughan, E. D., Herzlinger, D. A. (2001). Fibroblast GROWTH Factor-7 REGULATES Stratification OF the BLADDER Urothelium. J. Urol. 166, 2536–2541. doi: 10.1016/S0022-5347(05)65630-3
Tavana, H., Zamankhan, P., Christensen, P. J., Grotberg, J. B., Takayama, S. (2011). Epithelium Damage and Protection During Reopening of Occluded Airways in a Physiologic Microfluidic Pulmonary Airway Model. Biomed. Microdevices 13, 731–742. doi: 10.1007/s10544-011-9543-5
Terry, J., Neethirajan, S. (2014). A Novel Microfluidic Wound Model for Testing Antimicrobial Agents Against Staphylococcus Pseudintermedius Biofilms’. J. Nanobiotechnol. 12, 1. doi: 10.1186/1477-3155-12-1
Thacker, V. V., Dhar, N., Sharma, K., Barrile, R., Karalis, K., McKinney, J. D. (2020). A Lung-on-Chip Model of Early Mycobacterium Tuberculosis Infection Reveals an Essential Role for Alveolar Epithelial Cells in Controlling Bacterial Growth. eLife 9, e59961. doi: 10.7554/eLife.59961
Thänert, R., Reske, K. A., Hink, T., Wallace, M. A., Wang, B., Schwartz, D. J., et al. (2019). Comparative Genomics of Antibiotic-Resistant Uropathogens Implicates Three Routes for Recurrence of Urinary Tract Infections. mBio 10, e01977–e01919. doi: 10.1128/mBio.01977-19
Threadgill, D. W., Dlugosz, A. A., Hansen, L. A., Tennenbaum, T., Lichti, U., Yee, D., et al. (1995). Targeted Disruption of Mouse EGF Receptor: Effect of Genetic Background on Mutant Phenotype. Science 269, 230. doi: 10.1126/science.7618084
Thumbikat, P., Berry, R. E., Zhou, G., Billips, B. K., Yaggie, R. E., Zaichuk, T., et al. (2009). Bacteria-Induced Uroplakin Signaling Mediates Bladder Response to Infection. PloS Pathog. 5, e1000415. doi: 10.1371/journal.ppat.1000415
Thumbikat, P., Waltenbaugh, C., Schaeffer, A. J., Klumpp, D. J. (2006). Antigen-Specific Responses Accelerate Bacterial Clearance in the Bladder. J. Immunol. 176, 3080. doi: 10.4049/jimmunol.176.5.3080
Timmermans, S., Montagu, M. V., Libert, C. (2017). Complete Overview of Protein-Inactivating Sequence Variations in 36 Sequenced Mouse Inbred Strains. Proc. Natl. Acad. Sci. 114, 9158. doi: 10.1073/pnas.1706168114
Toh, Y.-C., Lim, T. C., Tai, D., Xiao, G., van Noort, D., Yu, H. (2009). A Microfluidic 3D Hepatocyte Chip for Drug Toxicity Testing. Lab. Chip 9, 2026–2035. doi: 10.1039/b900912d
Torisawa, Y.-s., Spina, C. S., Mammoto, T., Mammoto, A., Weaver, J. C., Tat, T., et al. (2014). Bone Marrow–on–a–Chip Replicates Hematopoietic Niche Physiology In Vitro. Nat. Methods 11, 663–669. doi: 10.1038/nmeth.2938
Tötemeyer, S., Foster, N., Kaiser, P., Maskell, D. J., Bryant, C. E. (2003). Toll-Like Receptor Expression in C3H/HeN and C3H/HeJ Mice During Salmonella Enterica Serovar Typhimurium Infection. Infection Immun. 71, 6653–6657. doi: 10.1128/IAI.71.11.6653-6657.2003
Tovaglieri, A., Sontheimer-Phelps, A., Geirnaert, A., Prantil-Baun, R., Camacho, D. M., Chou, D. B. (2019). Species-Specific Enhancement of Enterohemorrhagic E. Coli Pathogenesis Mediated by Microbiome Metabolites. Microbiome 7, 43. doi: 10.1186/s40168-019-0650-5
Truschel, S. T., Wang, E., Ruiz, W. G., Leung, S.-M., Rojas, R., Lavelle, J., et al. (2002). Stretch-Regulated Exocytosis/Endocytosis in Bladder Umbrella Cells. Mol. Biol. Cell 13, 830–846. doi: 10.1091/mbc.01-09-0435
Tsantoulas, C., Farmer, C., Machado, P., Baba, K., McMahon, S. B., Raouf, R. (2013). Probing Functional Properties of Nociceptive Axons Using a Microfluidic Culture System. PloS One 8, e80722. doi: 10.1371/journal.pone.0080722
Uchio-Yamada, K., Monobe, Y., Akagi, K.-I., Yamamoto, Y., Ogura, A., Manabe, N. (2016). Tensin2-deficient Mice on FVB/N Background Develop Severe Glomerular Disease. J. Veterinary Med. Sci. 78, 811–818. doi: 10.1292/jvms.15-0442
Ulett, G. C., Webb, R. I., Ulett, K. B., Cui, X., Benjamin, W. H., Crowley, M., Schembri, M. A. (2010). Group B Streptococcus (GBS) Urinary Tract Infection Involves Binding of GBS to Bladder Uroepithelium and Potent but GBS-Specific Induction of Interleukin 1α. J. Infec. Dis. 201 (6), 866–870. doi: 10.1086/650696
Vance, R. E., Jamieson, A. M., Cado, D., Raulet, D. H. (2002). Implications of CD94 Deficiency and Monoallelic NKG2A Expression for Natural Killer Cell Development and Repertoire Formation. Proc. Natl. Acad. Sci. 99, 868. doi: 10.1073/pnas.022500599
van de Wijdeven, R., Ramstad, O. H., Bauer, U. S., Halaas, Øyvind, Sandvig, A., Sandvig, I. (2018). Structuring a Multi-Nodal Neural Network In Vitro Within a Novel Design Microfluidic Chip. Biomed. Microdevices 20, 9. doi: 10.1007/s10544-017-0254-4
Varley, C., Hill, G., Pellegrin, S., Shaw, N. J., Selby, P. J., Trejdosiewicz, L. K., et al. (2005). Autocrine Regulation of Human Urothelial Cell Proliferation and Migration During Regenerative Responses In Vitro. Exp. Cell Res. 306, 216–229. doi: 10.1016/j.yexcr.2005.02.004
Vasl, J., Oblak, A., Gioannini, T. L., Weiss, J. P., Jerala, R. (2009). Novel Roles of Lysines 122, 125, and 58 in Functional Differences Between Human and Murine MD-2. J. Immunol. (Baltimore Md. 1950) 183, 5138–5145. doi: 10.4049/jimmunol.0901544
Vasyutin, I., Zerihun, L., Ivan, C., Atala, A. (2019). Bladder Organoids and Spheroids: Potential Tools for Normal and Diseased Tissue Modelling. Anticancer Res. 39, 1105. doi: 10.21873/anticanres.13219
Vaure, C, Liu, Y. (2014). A Comparative Review of Toll-Like Receptor 4 Expression and Functionality in Different Animal Species. Front. Immunol. 5, 316–316. doi: 10.3389/fimmu.2014.00316
Vedula, E. M., Alonso, J. L., Amin Arnaout, M., Charest, J. L. (2017). A Microfluidic Renal Proximal Tubule With Active Reabsorptive Function. PloS One 12, e0184330. doi: 10.1371/journal.pone.0184330
Vidal, S. M., Pinner, E., Lepage, P., Gauthier, S., Gros, P. (1996). Natural Resistance to Intracellular Infections: Nramp1 Encodes a Membrane Phosphoglycoprotein Absent in Macrophages From Susceptible (Nramp1 D169) Mouse Strains. J. Immunol. 157, 3559.
Villenave, R., Wales, S. Q., Hamkins-Indik, T., Papafragkou, E., Weaver, J. C., Ferrante, T. C., et al. (2017). Human Gut-On-A-Chip Supports Polarized Infection of Coxsackie B1 Virus In Vitro. PloS One 12, e0169412. doi: 10.1371/journal.pone.0169412
Višnjar, T., Kreft, M. E. (2013). Air–Liquid and Liquid–Liquid Interfaces Influence the Formation of the Urothelial Permeability Barrier In Vitro. In Vitro Cell. Dev. Biol. Anim. 49, 196–204. doi: 10.1007/s11626-013-9585-5
Vormann, M. K., Vriend, J., Lanz, H. L., Gijzen, L., van den Heuvel, A., Hutter, S., et al. (2021). Implementation of a Human Renal Proximal Tubule on a Chip for Nephrotoxicity and Drug Interaction Studies. J. Pharm. Sci 110 (4), 1601–1614. doi: 10.1016/j.xphs.2021.01.028.
Wang, C., Bauckman, K. A., Ross, A. S.B., Symington, J. W., Ligon, M. M., Scholtes, G., et al. (2019). A non-Canonical Autophagy-Dependent Role of the ATG16L1(T300A) Variant in Urothelial Vesicular Trafficking and Uropathogenic Escherichia Coli Persistence. Autophagy 15, 527–542. doi: 10.1080/15548627.2018.1535290
Wang, S., Gao, D., Chen, Y. (2017). The Potential of Organoids in Urological Cancer Research. Nat. Rev. Urol. 14, 401–414. doi: 10.1038/nrurol.2017.65
Wang, E. C.Y., Lee, J.-M., Ruiz, W. G., Balestreire, E. M., von Bodungen, M., Barrick, S., et al. (2005). ATP and Purinergic Receptor–Dependent Membrane Traffic in Bladder Umbrella Cells’. J. Clin. Invest. 115, 2412–2422. doi: 10.1172/JCI24086
Wang, E., Truschel, S., Apodaca, G. (2003). Analysis of Hydrostatic Pressure-Induced Changes in Umbrella Cell Surface Area. Methods 30, 207–217. doi: 10.1016/S1046-2023(03)00027-6
Wan, Q., Xiong, G., Liu, G., Shupe, T. D., Wei, G., Zhang, D., et al. (2018). Urothelium With Barrier Function Differentiated From Human Urine-Derived Stem Cells for Potential Use in Urinary Tract Reconstruction. Stem Cell Res. Ther. 9, 304. doi: 10.1186/s13287-018-1035-6
Weaver, W. M., Milisavljevic, V., Miller, J. F., Carlo, D. D. (2012). Fluid Flow Induces Biofilm Formation in Staphylococcus Epidermidis Polysaccharide Intracellular Adhesin-Positive Clinical Isolates. Appl. Environ. Microbiol. 78, 5890–5896. doi: 10.1128/AEM.01139-12
Wen, H., Lei, Y., Eun, S.-Y., Ting, J. P.Y. (2010). Plexin-A4-semaphorin 3A Signaling is Required for Toll-like Receptor- and Sepsis-Induced Cytokine Storm. J. Exp. Med. 207, 2943–2957. doi: 10.1084/jem.20101138
Wezel, F., Pearson, J., Southgate, J. (2013). Plasticity of In Vitro-Generated Urothelial Cells for Functional Tissue Formation. Tissue Eng. Part A 20, 1358–1368. doi: 10.1089/ten.tea.2013.0394
Winder, M., Tobin, G., Zupančič, Daša, Romih, R. (2014). Signalling Molecules in the Urothelium. BioMed. Res. Int. 2014, 297295. doi: 10.1155/2014/297295
Wolfe, G., Gasper, E., Stoke, J., Kretchman, J., Anderson, D., Czuba, N., et al. (2015). “11.9 Pressures in the Body,” in College Physics for AP® Courses (Houston, Texas: OpenStax).
Wood, T. K., Knabel, S. J., Kwan, B. W. (2013). Bacterial Persister Cell Formation and Dormancy. Appl. Environ. Microbiol. 79, 7116. doi: 10.1128/AEM.02636-13
Wright, E., Neethirajan, S., Weng, X. (2015). Microfluidic Wound Model for Studying the Behaviors of Pseudomonas Aeruginosa in Polymicrobial Biofilms. Biotechnol. Bioengineer. 112, 2351–2359. doi: 10.1002/bit.25651
Wu, X.-R., Kong, X.-P., Pellicer, A., Kreibich, G., Sun, T.-T. (2009). Uroplakins in Urothelial Biology, Function, and Disease. Kidney Int. 75, 1153–1165. doi: 10.1038/ki.2009.73
Wu, X. R., Sun, T. T., Medina, J. J. (1996). In Vitro Binding of Type 1-Fimbriated Escherichia Coli to Uroplakins Ia and Ib: Relation to Urinary Tract Infections. Proc. Natl. Acad. Sci. 93, 9630. doi: 10.1073/pnas.93.18.9630
Xiao, R.-R., Zeng, W.-J., Li, Y.-T., Zou, W., Wang, L., Pei, X.-F., et al. (2013). Simultaneous Generation of Gradients With Gradually Changed Slope in a Microfluidic Device for Quantifying Axon Response. Analytical Chem. 85, 7842–7850. doi: 10.1021/ac4022055
Xie, C., Sharma, R., Wang, H., Zhou, X. J., Mohan, C. (2004). Strain Distribution Pattern of Susceptibility to Immune-Mediated Nephritis. J. Immunol. 172, 5047. doi: 10.4049/jimmunol.172.8.5047
Yang, P. J., Pham, J., Choo, J., Hu, D. L. (2014). Duration of Urination Does Not Change With Body Size. Proc. Natl. Acad. Sci. 111, 11932. doi: 10.1073/pnas.1402289111
Yelin, I., Snitser, O., Novich, G., Katz, R., Tal, O., Parizade, M., et al. (2019). Personal Clinical History Predicts Antibiotic Resistance of Urinary Tract Infections. Nat. Med. 25, 1143–1152. doi: 10.1038/s41591-019-0503-6
Yu, W. (2015). Polarized ATP Distribution in Urothelial Mucosal and Serosal Space is Differentially Regulated by Stretch and Ectonucleotidases. Am. J. Physiol. Renal Physiol. 309, F864–FF72. doi: 10.1152/ajprenal.00175.2015
Yue, B. (2014). Biology of the Extracellular Matrix: An Overview. J. Glaucoma 23, S20–S23. doi: 10.1097/IJG.0000000000000108
Yu, W., Khandelwal, P., Apodaca, G. (2009). Distinct Apical and Basolateral Membrane Requirements for Stretch-Induced Membrane Traffic At the Apical Surface of Bladder Umbrella Cells. Mol. Biol. Cell 20, 282–295. doi: 10.1091/mbc.e08-04-0439
Yu, H.-S., Kim, J.-J., Kim, H.-W., Lewis, M. P., Wall, I. (2016). Impact of Mechanical Stretch on the Cell Behaviors of Bone and Surrounding Tissues. J. Tissue Eng. 7, 2041731415618342. doi: 10.1177/2041731415618342
Yu, Z., Liao, J., Chen, Y., Zou, C., Zhang, H., Cheng, J., et al. (2019). Single-Cell Transcriptomic Map of the Human and Mouse Bladders. J. Am. Soc. Nephrol. 30, 2159. doi: 10.1681/ASN.2019040335
Zalewska-Piątek, B., Olszewski, M., Lipniacki, T., Błoński, S., Wieczór, M., Bruździak, P., et al. (2020). A Shear Stress Micromodel of Urinary Tract Infection by the Escherichia Coli Producing Dr Adhesin. PloS Pathog. 16, e1008247. doi: 10.1371/journal.ppat.1008247
Zhang, Y. S., Aleman, J., Shin, S. R., Kilic, T., Kim, D., Shaegh, S. A. M., et al. (2017). Multisensor-Integrated Organs-on-Chips Platform for Automated and Continual in Situ Monitoring of Organoid Behaviors. Proc. Natl. Acad. Sci. 114, E2293. doi: 10.1073/pnas.1612906114
Zhang, Y., Atala, A. (2013). “Urothelial Cell Culture: Stratified Urothelial Sheet and Three-Dimensional Growth of Urothelial Structure,” in Epithelial Cell Culture Protocols: Second Edition. Eds. Randell, H., Leslie Fulcher, M. (Totowa, NJ: Humana Press).
Zhang, B., Korolj, A., Lun Lai, B. F., Radisic, M. (2018). Advances in Organ-on-a-Chip Engineering. Nat. Rev. Mater. 3, 257–278. doi: 10.1038/s41578-018-0034-7
Zhang, W., Lee, W. Y., Siegel, D. S., Tolias, P., Zilberberg, J. (2014). Patient-Specific 3d Microfluidic Tissue Model for Multiple Myeloma. Tissue Eng. Part C: Methods 20, 663–670. doi: 10.1089/ten.tec.2013.0490
Zhang, M., Wang, P., Luo, R., Wang, Y., Li, Z., Guo, Y., et al. (2021). Biomimetic Human Disease Model of SARS-CoV-2-Induced Lung Injury and Immune Responses on Organ Chip System. Advanced Sci. 8, 2002928. doi: 10.1002/advs.202002928
Zheng, J., Zhai, K., Chen, Y., Zhang, X., Miao, L., Wei, B., et al. (2016). Nitric Oxide Mediates Stretch-Induced Ca2+oscillation in Smooth Muscle. J. Cell Sci. 129, 2430. doi: 10.1242/jcs.180638
Zhou, J., Luan, G.-D., Ren, L.-M., Wu, Z.-G., Wang, X., Zhao, Y. (2010). Pharmacologic Characteristics of Bladder Micturition Function in Anesthetized Mice. Comp. Med. 60, 436–442.
Zhou, G., Mo, W.-J., Sebbel, P., Min, G., Neubert, T. A., Glockshuber, R., et al. (2001). Uroplakin Ia is the Urothelial Receptor for Uropathogenic Escherichia Coli: Evidence From In Vitro FimH Binding. J. Cell Sci. 114, 4095. doi: 10.1242/jcs.114.22.4095
Zhu, W., Gilmour, M. I. (2009). Comparison of Allergic Lung Disease in Three Mouse Strains After Systemic or Mucosal Sensitization With Ovalbumin Antigen. Immunogenetics 61, 199–207. doi: 10.1007/s00251-008-0353-8
Zhu, Y., Warrick, J. W., Haubert, K., Beebe, D. J., Yin, J. (2009). Infection on a Chip: A Microscale Platform for Simple and Sensitive Cell-Based Virus Assays. Biomed. Microdevices 11, 565–570. doi: 10.1007/s10544-008-9263-7
Zimmermann, W.-H. (2013). Biomechanical Regulation of In Vitro Cardiogenesis for Tissue-Engineered Heart Repair. Stem Cell Res. Ther. 4, 137. doi: 10.1186/scrt348
Keywords: urinary tract infection (UTI), microphysiological systems, in vitro infection model systems, organ-on-chip, organoid, urothelium, uropathogenic E. coli (UPEC), mouse models
Citation: Murray BO, Flores C, Williams C, Flusberg DA, Marr EE, Kwiatkowska KM, Charest JL, Isenberg BC and Rohn JL (2021) Recurrent Urinary Tract Infection: A Mystery in Search of Better Model Systems. Front. Cell. Infect. Microbiol. 11:691210. doi: 10.3389/fcimb.2021.691210
Received: 05 April 2021; Accepted: 04 May 2021;
Published: 26 May 2021.
Edited by:
Marco Metzger, Fraunhofer Society (FHG), GermanyReviewed by:
Sarah Maddocks, Cardiff Metropolitan University, United KingdomRaja Veerapandian, University of Texas at San Antonio, United States
Copyright © 2021 Murray, Flores, Williams, Flusberg, Marr, Kwiatkowska, Charest, Isenberg and Rohn. This is an open-access article distributed under the terms of the Creative Commons Attribution License (CC BY). The use, distribution or reproduction in other forums is permitted, provided the original author(s) and the copyright owner(s) are credited and that the original publication in this journal is cited, in accordance with accepted academic practice. No use, distribution or reproduction is permitted which does not comply with these terms.
*Correspondence: Jennifer L. Rohn, ai5yb2huQHVjbC5hYy51aw==
†These authors have contributed equally to this work and share first authorship