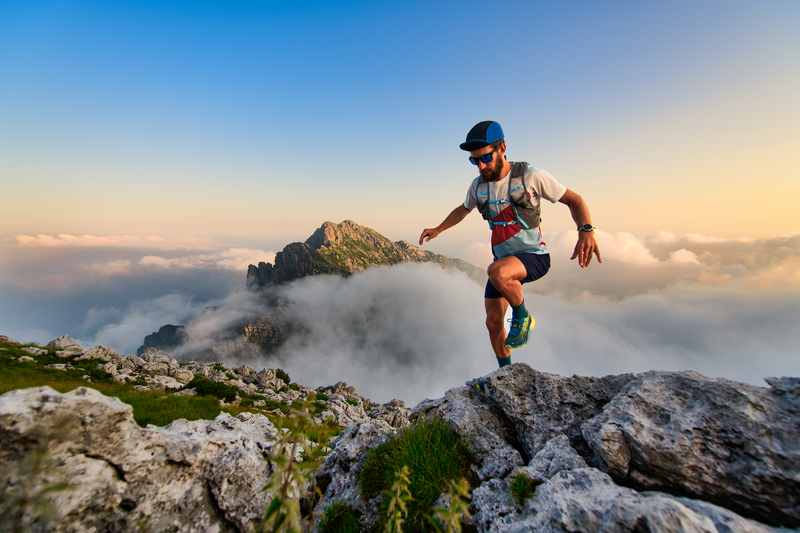
94% of researchers rate our articles as excellent or good
Learn more about the work of our research integrity team to safeguard the quality of each article we publish.
Find out more
REVIEW article
Front. Cell. Infect. Microbiol. , 23 June 2021
Sec. Bacteria and Host
Volume 11 - 2021 | https://doi.org/10.3389/fcimb.2021.690087
Aedes aegypti is inherently susceptible to arboviruses. The geographical expansion of this vector host species has led to the persistence of Dengue, Zika, and Chikungunya human infections. These viruses take advantage of the mosquito’s cell to create an environment conducive for their growth. Arboviral infection triggers transcriptomic and protein dysregulation in Ae. aegypti and in effect, host antiviral mechanisms are compromised. Currently, there are no existing vaccines able to protect human hosts from these infections and thus, vector control strategies such as Wolbachia mass release program is regarded as a viable option. Considerable evidence demonstrates how the presence of Wolbachia interferes with arboviruses by decreasing host cytoskeletal proteins and lipids essential for arboviral infection. Also, Wolbachia strengthens host immunity, cellular regeneration and causes the expression of microRNAs which could potentially be involved in virus inhibition. However, variation in the magnitude of Wolbachia’s pathogen blocking effect that is not due to the endosymbiont’s density has been recently reported. Furthermore, the cellular mechanisms involved in this phenotype differs depending on Wolbachia strain and host species. This prompts the need to explore the cellular interactions between Ae. aegypti-arboviruses-Wolbachia and how different Wolbachia strains overall affect the mosquito’s cell. Understanding what happens at the cellular and molecular level will provide evidence on the sustainability of Wolbachia vector control.
Aedes aegypti is a target of vector control owing to its complete susceptibility to Dengue (DENV), Zika (ZIKV), and Chikungunya (CHIKV) viruses. Substantively, these arboviruses are detectable from mosquito populations worldwide resulting in geographical spread of human infections (Espinal et al., 2019). To curb increasing prevalence of arboviral diseases, vector control has been at the forefront with Wolbachia drawing considerable attention this decade (Indriani et al., 2020). The use of Wolbachia to control arboviral transmission to humans stems from its ability to persistently establish itself in a diverse vector species (Shaikevich et al., 2019). Wolbachia pipentis naturally resides in approximately 65% of all insect species and in arthropods, this bacterium is known to be both parasitic and mutualistic (Rousset et al., 1992; Weeks and Breeuwer, 2001; Hilgenboecker et al., 2008; Riparbelli et al., 2012; Beckmann et al., 2017; LePage et al., 2017). Wolbachia manipulates host reproduction through male killing (Riparbelli et al., 2012), feminization (Rousset et al., 1992), parthenogenesis (Weeks and Breeuwer, 2001), and more commonly, cytoplasmic incompatibility (CI) (Beckmann et al., 2017; LePage et al., 2017). CI is induced by the deubiquitinating enzyme (DUB) encoded by a two-gene operon cidA-cidB, which causes embryonic mortality when infected males mate with either uninfected females or those with a different Wolbachia strain (Beckmann et al., 2017; LePage et al., 2017). Such principle became the basis for Wolbachia release programs aiming for mosquito population suppression or replacement (Indriani et al., 2020). Other than reproductive manipulation, multiple evidences prove that Wolbachia significantly confers protection to mosquitoes against viruses yet little information is known on the cellular mechanisms underlying its antiviral impact. This pathogen blocking effect coupled with efficient spread have contributed to its potential as vector control agent (White et al., 2017; Shaikevich et al., 2019).
Previous studies have reported that in general, Wolbachia elicits a pathogen blocking phenotype in arthropods either by priming the host’s immunity and/or fighting for scarce host cellular resources (Kambris et al., 2010; Caragata et al., 2016; Angleró-Rodríguez et al., 2017; Pan et al., 2018). For instance, Wolbachia transinfection in Ae. aegypti leads to the activation of antiviral mechanisms along with an elevated production of antimicrobial peptides (Angleró-Rodríguez et al., 2017; Pan et al., 2018). Similarly, Anopheles gambiae transiently infected with the endosymbiont displays an upregulated expression of malaria-related immune genes and reduction in Plasmodium infection, confirming Wolbachia’s direct influence on host immunity (Kambris et al., 2010). Other studies have also shown that host cellular resources mainly cholesterol is an essential determinant of Wolbachia’s viral inhibition in Drosophila (Caragata et al., 2013) whereas gene network analysis involving the same insect species and other RNA viruses reveal strong interactions between metabolism pathways related to host nutrient production and viral replication (Lindsey et al., 2021).
Existing review articles on Wolbachia’s pathogen blocking effect present a broad discussion encompassing various arthropod species (Caragata et al., 2016; Kamtchum-Tatuene et al., 2017; Lindsey et al., 2018; Pimentel et al., 2021). On the contrary, this review focuses on Ae. aegypti alone for the following reasons: Ae. aegypti is the main target of Wolbachia release programs therefore focusing on mechanisms specifically linked to this mosquito will provide significant information on the sustainability of vector control strategy. Also, pathogen blocking mechanisms are not conserved among host species which gives no guarantee that a particular cellular mechanism involved in pathogen blocking applies to all. Ae. aegypti is also unique in a sense that this mosquito has long been regarded as a novel Wolbachia host until natural infection has been recently reported (Coon et al., 2016; Carvajal et al., 2019). This characteristic together with the use of multiple Wolbachia strains for transinfection add more complexity to arboviruses-Wolbachia-Ae. aegypti interactions. Other review papers have also presented cellular mechanisms that covered competition for cholesterol and lipids, cellular stress, and immunity (Kamtchum-Tatuene et al., 2017; Lindsey et al., 2018). We now expand the coverage of these cellular mechanisms by adding direct viral inhibition via host cytoskeleton (Lu et al., 2020), antagonistic lipid modulation (Koh et al., 2020), and cellular regeneration (Ford et al., 2019) based on recent reports. We also incorporate new information on how Wolbachia’s density may not be a contributing factor to immunity contrary to previous findings. Furthermore, we present the corresponding host gene and protein changes linked to each cellular mechanism.
This review aims to explore Ae. aegypti’s cellular mechanisms which Wolbachia affects to block arboviruses. We begin by illustrating how these medically important viruses change the gene/protein expression patterns of the host cell and exploit corresponding cellular mechanisms to strengthen viral infection. Subsequently, we will discuss how Wolbachia directly or antagonistically interferes with Ae. aegypti-arbovirus interactions through a) inhibition of viral entry and replication, b) reduction of specific nutrients required in arboviral infection, c) increase in Reactive Oxygen Species (ROS) production and immunity, d) cellular regeneration to enhance midgut barrier, and e) regulation of genes with various cellular functions (Hussain et al., 2011; Hussain et al., 2011; Ford et al., 2019; Koh et al., 2020; Lu et al., 2020). Finally, we summarize the effects on Ae. aegypti when infected with either virus or Wolbachia or both.
Ae. aegypti’s intrinsic ability to act as a vector for disease is supported by its genome ingrained with genes that regulate cellular mechanisms for arboviral infection and defense. The latest annotated reference genome (AaegL5) of this mosquito portrays a wider coverage of gene families like chemosensory receptors, glutathione S-transferase, and C-type lectin with some unique quantitative trait loci (chromosome 2), all of which are paramount to viral susceptibility (Adelman and Myles, 2018; Matthews et al., 2018). DENV, ZIKV, and CHIKV, to some extent, impose differential transcriptomic changes and alter the function of translated proteins in Ae. aegypti (Mukherjee et al., 2019). Specifically, these arboviruses cause differential expression of transcripts under cytoskeletal, replication/transcription, immunity, ROS, and metabolism to control Ae. aegypti’s intracellular environment (Xi et al., 2008; Ramirez and Dimopoulos, 2010; Colpitts et al., 2011; Carvalho-Leandro et al., 2012; Sim et al., 2013; Angleró-Rodríguez et al., 2017; Mukherjee et al., 2019; Zhao et al., 2019) DENV, ZIKV, and CHIKV induce these changes in the mosquito in order to thrive without being pathogenic, allowing them to complete their life cycle (Mukherjee et al., 2019). In this section, we concentrate on the effects of arboviral infection on Ae. aegypti’s transcriptome, with only a brief discussion of proteomic and metabolomic expression patterns. These changes will be discussed together with the cellular mechanisms they affect to demonstrate host-virus interactions. Altogether, we explore the mechanisms on how arboviruses enforce viral infection and Ae. aegypti’s responses at the cellular level.
Arboviral infection in Ae. aegypti begins with the ingestion of a viremic blood meal followed by an Extrinsic Incubation Period (EIP). EIP is a period of viral incubation within the host where virus enters the midgut, passes through the hemolymph to reach other organs and culminates in the salivary glands for subsequent infection (Chan and Johansson, 2012). Throughout the EIP, arbovirus tries to take over the host cell by using the cytoskeleton and overcoming the midgut barrier.
Ae. aegypti’s cytoskeleton, composed of a network of microtubules and actin filaments, is required for an infecting arbovirus to successfully traverse viral entry, replication, assembly, and egress (Figure 1) (Foo and Chee, 2015). During infection, arboviruses cause differential expression of Ae. aegypti’s cytoskeletal transcripts and proteins which are cellular components essential for viral life cycle. Genes like dynein, vimentin, tubulin, actin, myosin, tropomyosin, and laminin are highly expressed in DENV-infected Ae. aegypti (Bonizzoni et al., 2012; Sim et al., 2012) whereas CHIKV infection has shown marked cytoskeletal protein expression (Cui et al., 2020) as opposed to the uninfected. These gene/protein modifications within the host may represent the way arboviruses’ take advantage of the mosquito’s cytoskeleton by rearranging it into tracks to actively transport endosomes containing viral particles (Walsh and Naghavi, 2019). To facilitate transport at a later phase, direct interaction between arboviral proteins and host cytoskeletal motor proteins (e.g., dynein and myosin) occur (Paingankar et al., 2010; Mairiang et al., 2013; Foo and Chee, 2015; Walsh and Naghavi, 2019). In the case of DENV infection in Ae. aegypti, link between non-structural (NS) protein 5 and myosin has been reported (Mairiang et al., 2013). Similarly, other cytoskeletal structures like actin and tubulin is said to interact with DENV to facilitate infection in vitro (Paingankar et al., 2010). Protein interaction network prediction in Ae. aegypti also suggests that tubulin is highly associated with DENV infection in the mosquito host with roles in transport and assembly (Guo et al., 2010). In CHIKV infection, impaired viral delivery from the cell surface to the cytoplasm occurred when microtubule network is disrupted (Hoornweg et al., 2020). While ZIKV’s effect on Ae. aegypti’s cytoskeleton has not been fully explored, differential expression of cytoskeletal-related transcripts signifies that ZIKV likely uses the cytoskeleton for its entry, replication, assembly, and egress as other arboviruses (Etebari et al., 2017).
Figure 1 Ae. aegypti's cellular mechanisms are affected by arboviral infection. Arboviral infections alter Ae. aegypti’s genes, proteins, and metabolites to control the host’s cellular mechanisms. First, arboviruses utilize host cytoskeletal proteins (e.g. dynein and myosin) to facilitate their intracellular transport. Second, the need for host cell nutrients allows the virus to alter host lipid/cholesterol and also triggers the formation of LD. LD can enhance host immunity via Toll/Imd, can aid viral replication through fatty acid synthase (FASN) recruitment by DENV NS3, and can be integrated into viral capsids. The activation of insulin receptor is also said to signal antiviral mechanisms i.e. ERK and JAK/STAT via upd2/3. Following activation of host immunity, antimicrobial peptides are produced. Third, arboviruses cause Ae. aegypti to produce elevated ROS. ROS can either directly harm invading arboviruses or activate the Toll immune mechanism. Arboviruses respond to the effects of ROS by upregulating antioxidant release which functions to lower down ROS in the cell. Fourth, miRNA-2b is initially processed inside the nucleus and brought out to the cytoplasm for further cleaving. A seed sequence produced from the RISC complex binds to URM, its target mRNA leading to the suppression of tRNA thiolation and inhibition of viral replication.
Following a blood meal, Ae. aegypti transcribes the glucosamine fructose-6 phosphate aminotransferase (AeGfAt-1) gene involved in the formation of a chitinous sac called peritrophic membrane (PM) that encloses the ingested blood (Figure 2). PM formation happens in the midgut within 6 to 12 h post-feeding and is a physiological response for digestion (Kato et al., 2002; Suwanmanee et al., 2009). However, intake of blood with DENV forms a PM earlier, showing clear visibility just an hour after meal and is otherwise thicker (Suwanmanee et al., 2009). It has been speculated that PM could function as part of the Midgut Infection Barrier (MIB) by preventing the virus from penetrating the midgut epithelium and reaching other mosquito organs. This is consistent with the reports that demonstrate how PM hinders systemic infection in other vector mosquitoes (Rodgers et al., 2017). Aside from the upregulation of AeGfAt-1, DENV-, ZIKV-, and CHIKV-infected Ae. aegypti markedly express proteolytic transcripts (e.g. serine proteases, metalloproteases, trypsin, serine type endopeptidases) concurrent with specific abundance in protein composition of serine type proteases (Sim et al., 2013; Etebari et al., 2017; Whiten et al., 2018; Cui et al., 2020). These proteases can break down other proteins that strengthen the midgut barrier and can therefore be utilized by arboviruses to assert systemic infection. Recent evidence demonstrates that in Ae. aegypti mosquitoes, a protease called plasmin enhances DENV infection by breaking glycocalyx, a layer covering midgut epithelia cells whereas inhibition of plasmin’s activity resulted in low infection (Figure 2) (Ramesh et al., 2019).
Figure 2 Arboviruses induce the formation of PM, cleaving of protective layer and Delta/Notch in Ae. aegypti’s midgut. An hour from the ingestion of virus-infected blood meal, Ae. aegypti forms a thick peritrophic membrane (PM) that encloses the virus separating it from the midgut epithelia. This PM prevents the virus from escaping the midgut and spreading the infection. Arboviruses may utilize proteolytic enzymes e.g. plasmin to break the glycocalyx, a protective layer at the surface of the midgut epithelia. Arboviruses may also directly damage the midgut tissue. Ae. aegypti can reverse this damage by activating the Delta/Notch resulting in the proliferation of Intestinal Stem Cells (ISC) for cellular regeneration.
Although arboviruses exploit different host cellular structures, viral replication and dissemination inside the host can be reversed by Ae. aegypti’s ability to regenerate the midgut epithelium (Figure 2). Taracena et al. describes how the exposure of the midgut epithelium to stress i.e. presence of arbovirus can result in the damage of the tissue. To counteract this, the host stimulates the proliferation of Intestinal Stem Cells (ISCs) responsible for cellular regeneration via a signaling pathway referred to as the Delta/Notch. Delta/Notch is able to increase DENV susceptibility of a refractory strain of Ae. aegypti when inhibited (Taracena et al., 2018). Hence, cellular regeneration via the Delta/Notch pathway is another important cellular response for arboviral blockade in Ae. aegypti.
Arboviruses take over Ae. aegypti’s metabolism by disrupting lipid homeostasis intracellularly as demonstrated in the mosquito’s altered transcriptome and metabolome. Ae. aegypti infected with either of these arboviruses demonstrates high abundance of differentially expressed genes/proteins for lipid biosynthesis (Figure 1) (Tchankouo-Nguetcheu et al., 2010; Raquin et al., 2017; Royle et al., 2017; Shrinet et al., 2017; Chotiwan et al., 2018; Fukutani et al., 2018). DENV infection in particular upregulates sterol regulatory element-binding protein (SREBP), calcium independent phospholipase A2 (iPLA2), ceramidase, lipase, Niemann pick-type C2 protein (NPC2), and Wnt pathway regulator genes significantly relative to uninfected control (Raquin et al., 2017). Moreover, elevated glycerophospholipids, glycerolipids, sphingolipids, and fatty acids are found not just in DENV-infected Ae. aegypti but also those infected with ZIKV and CHIKV. All in all, these represent a significant increase in lipids during infection (Tchankouo-Nguetcheu et al., 2010; Royle et al., 2017; Chotiwan et al., 2018). The exact cellular pathway that links these genes and metabolites to virus infection remain undiscovered. SREBP is the only gene whose function has been directly attributed to promote DENV infection whereas its knockdown decreases the viral load significantly (Raquin et al., 2017).
Further reports have associated high lipids with the viral replication and formation of lipid rich structures called lipid droplets (LD; Figure 1) (Heaton et al., 2010; Barletta et al., 2016). Initially, LD is regarded as a reservoir for cholesterol but has later been discovered to have a dynamic role. LD formation is accompanied by an increase in fatty acid synthase enzyme that catalyzes LD metabolism during DENV and CHIKV infection (Heaton et al., 2010; Tchankouo-Nguetcheu et al., 2010). Fatty acid synthase is said to be recruited by DENV NS3 at the site of replication in the absence of other NS proteins. Alongside its role in viral replication, LD is incorporated in the viral capsid during assembly. LD has also been linked to antiviral immunity in Ae. aegypti given that its accumulation strengthens the host’s immunity by activating Toll and Immune deficiency (Imd) cellular mechanisms (Barletta et al., 2016).
Interestingly, recent finding suggests that insulin and its receptor strengthen immunity against DENV and ZIKV infection in Ae. aegypti (Ahlers et al., 2019) by activating Janus kinase/signal transducers and activators of transcription (JAK/STAT). When mosquito cells are treated with insulin, the insulin receptor (InR) activates a cellular mechanism called the phosphatidylinositol 3’-kinase (PI3K) signaling pathway that send signals to the extracellular signal-regulated kinases (ERK). Once ERK is activated, it is said to prompt the release of molecules under the unpaired (upd2/3) family. Upd2/3 then engages the Domeless receptor (Dome), that sends signals to downstream proteins under the JAK/STAT leading to arboviral inhibition (Ahlers et al., 2019).
The involvement of the host’s immunity as a key player in regulating arboviral infection has been corroborated in several studies (Xi et al., 2008; Colpitts et al., 2011; Carvalho-Leandro et al., 2012; Sim et al., 2013; Angleró-Rodríguez et al., 2017; Caicedo et al., 2019; Mukherjee et al., 2019; Zhao et al., 2019). Arboviruses cause Ae. aegypti to actively respond to infection by switching immune-related genes on/off and utilizing the same genes to dictate the extent of viral susceptibility. This manifests at the transcriptomic level where high expression of leucine rich repeat (LRR)–containing proteins, Clip-domain serine proteases (CLIPBs), and Myeloid differentiation 2–related lipid recognition protein (MD-2) receptors are noted (Figure 1) (Xi et al., 2008; Angleró-Rodríguez et al., 2017; Caicedo et al., 2019; Zhao et al., 2019). LRR, CLIPBs, and MD-2 initiates Ae. aegypti’s immunity by functioning as Pattern Recognition Receptors (PRRs). PRRs recognize viral particles as Pathogen-Associated Molecular Patterns (PAMPs) at the surface of the host cell (Xi et al., 2008; Mukherjee et al., 2019). Consequently, the PRR-PAMPs binding causes Upd ligands to associate with Dome stimulating JAK tyrosine kinase Hopscotch (Hop) to phosphorylate. In turn, STAT proteins are activated and translocated to the nucleus where it is able to bind to the palindromic response element (PRE) to induce gene expression. The same signaling cascade applies to the Imd and Toll pathways only that different ligands and receptors are activated. Downstream of these pathways are proteins referred to as Relish (REL2, REL1) that binds to the PRE in the nucleus and triggers the expression anti-microbial peptides (AMPs) i.e. cecropin, defensin, lysozyme, and cathepsin (Hoffmann, 2003; Jang et al., 2006; Mukherjee et al., 2019). AMPs can directly kill microbes or further enhance the immunity. In both midgut and carcass tissue of DENV-, ZIKV-, and CHIKV-infected Ae. aegypti, AMP transcripts are highly evident (Xi et al., 2008; Sim et al., 2013; Angleró-Rodríguez et al., 2017). More so, silencing Cactus and Caspar that are inhibitory to Toll and Imd resulted in enhanced antimicrobial peptide gene expression (Xi et al., 2008). Indeed, these studies provide the basis for Ae. aegypti’s natural ability to resist viral infection. Contrary to this, viruses can increase host arboviral susceptibility by downregulating the same AMP genes as seen in transcriptomic and in vitro studies of DENV-, ZIKV-, and CHIKV-infected mosquitoes (Sim and Dimopoulos, 2010; Colpitts et al., 2011; Carvalho-Leandro et al., 2012). Some immune-related genes have also been directly linked to susceptibility. In a recent study by Caicedo et al., genes such as Gram-negative binding protein – GNBP (AAEL009176), NPC2 (AAEL015136), Keratinocyte lectin (AAEL009842) and Cathepsin-b (AAEL007585) when inhibited in a susceptible strain of Ae. aegypti led to a significant reduction in DENV dissemination (Caicedo et al., 2019). This proves the importance of these genes and their corresponding functions in DENV infection.
Arboviral infection results in production of ROS as part of the physiological response of Ae. aegypti to cellular stress (Figure 1). Excess ROS production act as secondary messengers that signals the innate immune response and can directly damage invading microbes (Tchankouo-Nguetcheu et al., 2010; Bottino-Rojas et al., 2018). To ensure infection, these arboviruses can neutralize the upregulated activity of ROS antiviral defense by modifying the gene expression profile of Ae. aegypti. Transcripts classified as antioxidants are markedly increased in arbovirus susceptible mosquito host. These antioxidants scavenge the ROS to decrease its harmful effects (Oliveira et al., 2017; Shrinet et al., 2018; Wang et al., 2019). During DENV infection, the antioxidant catalase functions to balance ROS and increase DENV concentration in mosquito’s midgut epithelia (Oliveira et al., 2017). Antioxidant hemopexin (HPX) exerts the same effect and enhances not just DENV infection but also ZIKV in Ae. aegytpi (Wang et al., 2019). Concomitantly, antioxidant transcripts like superoxide dismutase (CuSOD), thioredoxin peroxidase (TPX), and scavenger reporter (Scr) in Ae. aegypti infected with individual DENV/CHIKV as well as co-infection have demonstrated significant abundance (Shrinet et al., 2018). Downregulating the expression of antioxidants results in the reduction of DENV, ZIKV, and CHIKV titer (Oliveira et al., 2017; Wang et al., 2019). Similar to the manner by which virus directly exploits host immunity to their advantage, arboviruses must also ensure that oxidative stress represented by an increase in ROS is circumvented by adequate antioxidant release.
Arboviruses utilize Ae. aegypti’s micro-RNAs (miRNAs) for infection albeit the host can also use these for viral inhibition. miRNA is a type of non-coding RNA (ncRNA) that bind to DNA/RNA to either enhance or suppress the function of genes under various functional categories (Campbell et al., 2014). Initially, primary miRNAs (pri-miRNAs) undergo cleaving by Drosha and Pasha within the nucleus and exported to the cytoplasm as precursor miRNAs (pre-miRNAs) (Figure 1). Subsequently, pre-miRNAs go through additional modifications via Dicer to generate about ~22 nt base paired strands known as mature miRNA. This miRNA is loaded onto the RISC complex composed of Argonaute (Ago) proteins involved in the selection of one strand (aka guide strand). This guide strand constitutes two to six nucleotides referred to as the seed sequence that directly binds to the target mRNA for regulation. In general, arboviral infection give rise to a significant decline in Ae. aegypti global miRNA expression (Dubey et al., 2017; Saldaña et al., 2017; Dubey et al., 2019). Saldaña et al. reported 17 miRNAs that are significantly regulated in three time points during ZIKV infection. Maximum negative fold change has been observed in aae-miR-286a, aae-miR-2944b-3p, aae-miR-980-3p at 2 days post infection (dpi) and aae-miR-308-3p (7 dpi) whilst aae-miR-2940-3p and aae-miR-1-5p are enriched at 14 dpi. In addition, the abundance of some of the miRNAs particularly aae-miR-309-a and aae-miR-2941 are altered (Saldaña et al., 2017). A comparable observation has been reported in DENV2-infected Ae. aegypti (Campbell et al., 2014) but further studies investigating their target genes and effects are warranted. To illustrate the role of miRNA during virus infection, miRNAs and its potential targets in Ae. aegypti infected with CHIKV have been analyzed. miR-2b is one of the most significantly upregulated miRNA said to regulate ubiquitin-related modifier (URM), a cellular factor that promotes CHIKV replication (Dubey et al., 2017). This miRNA binds to the URM gene thereby exerting a suppressive effect on tRNA thiolation, a process required for gene translation. By inhibiting tRNA thiolation, CHIKV viral load is reduced (Dubey et al., 2017). Additionally, miR-2944b-5p has been said to enhance vps-13 expression which in turn stabilizes CHIKV in Ae. aegypti cells by maintaining mitochondrial stability (Dubey et al., 2019).
Mass release programs have been implemented to deploy Wolbachia in different communities that so far led to a reduction in dengue cases (O’Neill et al., 2019). Indeed, Wolbachia pipentis from other species when transinfected into Ae. aegypti do not only induce CI to suppress the mosquito population but also inhibits arboviruses (Lindsey et al., 2018). However, there is growing evidence that different Wolbachia strains carry varying blocking effects in Ae. aegypti with some strains failing to reduce viral replication and transmission despite being present at high density (Table 1) (Flores et al., 2020; Fraser et al., 2020). Under Wolbachia supergroup A, wAlbA is able to reduce ZIKV infection rates in orally infected Ae. aegypti but not in mosquitoes with intrathoracic ZIKV infection and oral/intrathoracic DENV infection (Chouin‐Carneiro et al., 2020). Meanwhile, Wolbachia strains wMelPop and wMelPop-CLA confers immune protection against DENV and CHIKV whereas the former strain together with wMel alters lipid/cholesterol content for DENV and ZIKV blocking (Moreira et al., 2009; Geoghegan et al., 2017; Asad et al., 2018; Fraser et al., 2020; Koh et al., 2020; Manokaran et al., 2020). Furthermore, wMel exhibits an anti-ZIKV effect that regulates the insulin receptor potentially suggesting another metabolic regulation (Haqshenas et al., 2019) as well as anti-DENV cellular regeneration (Ford et al., 2020). Meanwhile, Wolbachia strain wPip from the supergroup B has been tested on Ae. aegypti where it did not enhance the mosquito’s innate immunity against DENV (Fraser et al., 2020) while in other studies wAlbB directly intervenes with viral infection and increases ROS for immune activation (Pan et al., 2012; Lu et al., 2020). These studies may indicate that aside from Wolbachia strain, the variability in arboviral inhibition may be attributed to the mechanism/s and the extent to which these mechanisms are regulated by each strain inside the host.
The previous sections explained how arboviruses exploit Ae. aegypti cellular machinery by utilizing the mosquito’s cytoskeletal elements for viral infection and proteolytic enzymes to break the midgut barrier. In addition, arboviral infections take advantage of the host nutrients for their propagation. Counteracting ROS through antioxidant release and miRNA generation are also means for creating a conducive intracellular environment for these arboviruses to thrive. In the following sections, we present how Wolbachia adds another layer to Ae. aegypti-arbovirus interaction by interfering with the same molecular mechanisms and inducing cellular perturbations that are detrimental to the pathogen.
Studies have attributed Wolbachia’s pathogen blocking effect on its ability to decrease viral load. The mechanism by which this effect is accomplished, as well as the point in the virus’ life cycle at which such interference occurs, are previously unknown. The present study reveals that transinfected wAlbB strain in Ae. aegypti (Aag2) cell line infected with either DENV or ZIKV caused the downregulation of cytoskeletal membrane proteins, dystroglycan and beta-tubulin (Figure 3) (Lu et al., 2020). Concurrently, viral binding assays display a significant reduction in viral RNA copy number as early as 2 h post infection suggestive of an early viral interference in DENV and ZIKV binding as well as entry. Further validation done by silencing both cytoskeletal membrane proteins inhibited DENV binding to Aag2 cells (Lu et al., 2020). This is the first demonstration to confirm the direct involvement of Wolbachia in arbovirus binding and entry by taking advantage of the same host cytoskeletal proteins DENV and ZIKV utilize. Although there is a lack of data that demonstrates the same mechanism in CHIKV, this arbovirus necessitates an intact microtubule network consisting of alpha-tubulin (same family as beta-tubulin) for efficient viral entry and genome delivery to replication sites (Hoornweg et al., 2020).
Figure 3 Wolbachia interferes with Arboviral life cycle by interfering with host factors and strengthening host antiviral mechanisms. Wolbachia blocks DENV, ZIKV, and CHIKV by interfering with the same cellular mechanisms arboviruses control in Ae. aegypti. First, Wolbachia downregulates cytoskeletal structures that directly bind to arboval proteins for host cell infection. Next, Wolbachia competes for host’s lipid/cholesterol for its replication making this nutrient insufficient for the virus. Wolbachia can also deplete lipids namely sphingomyelins (SM), cardiolipins (CL), and acyl-carnitine that are essential for viruses. Wolbachia also strengthens the host’s immunity by activating Toll, IMD, and JAK/STAT via PGRP-LE. The endosymbiont further induces ROS release in the presence of an arbovirus by increasing oxidases in the cell. This inhibits viral replication and in turn, stimulates the Toll pathway. Wolbachia then counteracts the release of antioxidants to sustain its infection within the host. Lastly, Wolbachia upregulates receptor gene, AaNotch in Ae. aegypti. This receptor gene signals the Delta/Notch pathway. Consequently, the adhesion molecule Cadherin is increased strengthening the host’s reproductive ability and ensures maintenance of Wolbachia. Delta/Notch also promotes the regeneration of the midgut epithelia to prevent systemic viral infection.
In other host species that harbor Wolbachia infection, cytoskeletal proteins are vital for maintaining the endosymbiont’s density (Baldridge et al., 2014; Sheehan et al., 2016). Native Wolbachia in Drosophila expresses a type IV secretion system (T4SS) that releases an effector molecule called Wolbachia actin-localizing effector 1 protein (WalE1) (Sheehan et al., 2016). WalE1 directly binds to actin aiding in Wolbachia’s localization within the host (Sheehan et al., 2016). This is consistent with the report on significant reduction of the endosymbiont’s density coupled with inefficient maternal transmission when mutations in the actin gene are present (Newton et al., 2015). In like manner, Ae. albopictus expresses T4SS and a corresponding WalE1 homolog which may suggest contribution to the maintenance of Wolbachia infection in this species (Baldridge et al., 2014).
Based on the evidence, Wolbachia interacts with the host cytoskeleton in two ways. First, its ability to secrete effector molecules that bind to cytoskeletal structures ensures that Wolbachia resides in the host cell at an optimal density and guarantees transmission to other hosts. Second, Wolbachia regulates the expression of cytoskeletal proteins like dystroglycan and tubulin, required for arboviral infection (Lu et al., 2020). It is remarkable how arboviruses and Wolbachia have contrasting effects on the host cytoskeleton. While the former upregulates cytoskeletal structures for virus entry, replication, assemble, and egress, the latter downregulates the same structures to block arboviral binding/entry (Sim et al., 2012; Sim et al., 2013; Lu et al., 2020).
Wolbachia localizes within golgi-related vesicles in close proximity to the endoplasmic reticulum, the site of cell membrane biogenesis (Cho et al., 2011). Provided that membrane biogenesis relies on host lipids, this position allows Wolbachia to acquire nutrients for itself (Fattouh et al., 2019). Previous reports show that Ae. albopictus cells infected with wMel resulted in decreased sphingolipids, diacylglycerol, and phosphatidylcholine (Molloy et al., 2016). These host lipids constitute structures known as lipid rafts used by the bacteria to enter the cell and activate mechanisms required for bacterial invasion (Lafont and Goot, 2005). Likewise, Ae. aegypti cells infected with wMelPop or wMel experienced 15% to 25% reduction in total cholesterol signifying Wolbachia’s dependence on host cellular lipids (Caragata et al., 2014). The presence of either Wolbachia strain and DENV in Ae. aegypti perturbs cholesterol levels where an increase in stored cholesterol and localized accumulation of LD has been observed (Figure 3) (Geoghegan et al., 2017). This is represented by an upregulation of Niemann-Pick type C2 (NPC2), sterol carrier protein 2, calnexin 99 and a simultaneous downregulation of fatty acid synthase and LDL receptor proteins corresponding to an impaired intracellular cholesterol transport (Geoghegan et al., 2017). More so, treatment of Ae. aegypti infected with Wolbachia using a substance for stabilizing cholesterol resulted in the restoration of DENV replication (Geoghegan et al., 2017). Cholesterol supplementation that rescued viral replication has first been noted in other arthropod species such as Drosophila and Ae. albopictus (Caragata et al., 2013; Schultz et al., 2017). Several studies then pointed out that these metabolic changes induced by Wolbachia could be responsible for the pathogen-blocking mechanism in transinfected Ae. aegypti. Significant increase in the host’s cholesterol content takes place due to Wolbachia’s sequestration of cholesterol and lipid droplets, the same nutrients crucial for DENV, ZIKV, and CHIKV replication (Geoghegan et al., 2017; Cui et al., 2020; Leier et al., 2020). Wolbachia and arboviruses’ need for the same resources become the theoretical basis for competition between the two microbes with Wolbachia surpassing viral infection for its self-preservation (Caragata et al., 2013; Geoghegan et al., 2017; Schultz et al., 2017; Leier et al., 2020). Conversely, recent studies propose that instead of competition for lipids, antagonistic lipid modulation occurs (Koh et al., 2020; Manokaran et al., 2020). In the study by Koh et al., mono-infection of DENV results in lipid abundance whereas wMel causes mild depletion. However, co-infection of DENV and wMel in Ae. aegypti displays a lipid profile that resembles DENV induced perturbations signifying arbovirus control while certain lipids have been reported to be indirectly antagonistic (Koh et al., 2020). Take for example sphingomyelins (SM) and cardiolipins (CL) which were enriched in DENV3-infected Ae. aegypti but depleted in the presence of the same virus and the endosymbiont. CL knockdown also decreased DENV load regardless of wMel’s presence but replication of wMel is impaired only in the absence of DENV (Koh et al., 2020). This antagonistic interaction also applies in another study where another class of lipids called acyl-carnitine is elevated during DENV and ZIKV infection but significantly diminished in wMel-infected Aag2 cells (Manokaran et al., 2020). Reduction in acyl-carnitine enhanced wMel density yet addition of the said lipid in Aag2 containing wMel boosts DENV and ZIKV infection (Manokaran et al., 2020).
Wolbachia is similar with arboviruses in a sense that both are unable to synthesize lipids making them dependent on the host cell. While this competition means that one must utilize host nutrients at the expense of the other, recent findings show us that co-infection of these microbes within Ae. aegypti may also entail an antagonistic interaction. Perhaps the virus tries to establish an intracellular environment high in lipids for its replication which Wolbachia hampers.
Meanwhile, other factors that play a role in lipid homeostasis is insulin which promotes fat storage in cells. Latest study reveals that DENV and ZIKV suppression is mediated by the downregulation of insulin receptor in wMel-transinfected Ae. aegytpi (Haqshenas et al., 2019). In the previous section, insulin has been linked to the activation of host immune system. The underlying interaction between Wolbachia and arboviruses in the context of this mechanism warrants further investigation.
Existing view posits that transinfected Wolbachia infection in Ae. aegypti primes the mosquito’s immune system prior arboviral infection (Pan et al., 2018) yet the extent of immune activation differs among Wolbachia strains. Similar with the host’s response to virus, wAlbB-transinfected Ae. aegypti upregulates genes under the Toll (GNBP1, SPZ3B, MYD88) and Imd (PRGP-LE, REL2) pathways leading to antimicrobial peptide (e.g. cecropins, defensins) release during arboviral infection (Figure 3) (Xi et al., 2008; Angleró-Rodríguez et al., 2017; Pan et al., 2018; Zhao et al., 2019). Furthermore, innate immunity can be activated by ROS indicating cross-talk between immune and redox mechanisms (Pan et al., 2012). With DENV infection, wAlbB stimulates the production of NAPDH oxidases which are key producers of ROS. ROS then activates the Toll pathway to reduce DENV load. These oxidases, when silenced, deactivate the host’s immunity leading to an increased DENV titer (Pan et al., 2012). Simultaneously, Toll controls antioxidant expression that helps wAlbB maintain its infection in Ae. aegypti (Pan et al., 2012). It is interesting to note that ROS-dependent immune pathway activation does not occur in Wolbachia’s natural host, Ae. albopictus (Molloy and Sinkins, 2015). Adult mosquito lines carrying transinfected wAlb and wMel that varies in density show neither an upregulation in ROS-related genes nor innate immune activity (Molloy and Sinkins, 2015). In the same study, this effect has been observed in vitro using wMelPop, wMel, and wAlbB transinfection suggesting that ROS-dependent immune activation could be unique to Ae. aegypti (Molloy and Sinkins, 2015).
Meanwhile, wMelpop-CLA blocked DENV and CHIKV via JAK/STAT in Ae. aegypti (Moreira et al., 2009; Asad et al., 2018). wMelpop-CLA inhibits DENV through an upregulation of a Vago protein homolog (AeVago) that activates JAK/STAT (Asad et al., 2018). In CHIKV, this strain has been observed to cause an upregulation of AMPs but displayed unparalleled results in two independent experiments in terms of transcriptional modulation of immune-related genes (Moreira et al., 2009). All in all, multiple strains of Wolbachia demonstrate arboviral blocking that is partly mediated by the host’s immunity yet there remains an underlying issue on the magnitude of this effect. And whether this immunity merely depends on the strain or density is still debatable.
A recent study that compared multiple Wolbachia strains in a consistent Ae. aegypti line provided deeper understanding of viral blocking based on the endosymbiont’s strain and density (Fraser et al., 2020). Here, transinfected wPip at high density in Ae. aegypti, failed to block DENV replication and dissemination (Fraser et al., 2020). Notably, wPip together with DENV-inhibitory wAlbB and wMel only induce very small to no increase in expression of genes under the Toll pathway. Marked upregulation in immune-related genes has only been observed in wMelpop (Fraser et al., 2020). Contrary to other hypotheses, this study demonstrates that there is no association between Wolbachia strain/density and the extent of immune activation to block DENV. It is also interesting to highlight how wMel can effectively restrict DENV infection yet with only a small upregulation of Ae. aegypti’s immunity (Fraser et al., 2020). wMel is the strain that is predominantly used in mass release programs and is said to work against arboviruses by manipulating the host’s lipid/cholesterol (Geoghegan et al., 2017; Haqshenas et al., 2019; Koh et al., 2020; Manokaran et al., 2020). This then opens the possibility that specific Wolbachia strains induce their antiviral effect using different, not necessarily all of the host cellular mechanisms.
Wolbachia strains when traced back to their natural hosts may suggest potential pathogen blocking activity but it does not automatically lead to the same effect when transinfected in Ae. aegypti and in the case that it does, the immune pathways Wolbachia strains activate may not be conserved. Other than the strain or density per se, the regulation of innate immunity in Ae. aegypti seems more complex and influenced by intracellular responses that have yet to be elucidated.
New inferences on the molecular mechanisms of Wolbachia-mediated viral blocking in Ae. aegypti involves the Notch signaling pathway and cell-cell adhesion (Figure 3). Generally, Notch is a conserved mechanism that enhances the host’s fitness (Kopan and Ilagan, 2009; Chang et al., 2018). In fact, Ae. aegypti carries a Notch receptor gene (AaNotch) involve in maintaining micropyle pores and fecundity, all of which contribute to overall reproductive ability (Chang et al., 2018). Notably, this high fitness advantage in Ae. aegypti has been recently associated with stronger Wolbachia-mediated viral blocking (Ford et al., 2019; Ford et al., 2020). In the study by Ford et al., wMel-infected Ae. aegypti artificially selected for high wMel-mediated viral inhibition displays a gene profile in favor of Notch activation which significantly differs from mosquitos classified with low wMel-mediated DENV blocking. Notch activation coupled with high viral blocking has been linked to elevated cadherin expression (Ford et al., 2019; Ford et al., 2020). Although the mechanistic relationship between Notch and cadherin in Ae. aegypti has not been explicitly defined, a study in Drosophila shows how these two combine as a single complex to form cell-cell junctions implicated in Delta/Notch activation. Moreover, depletion of cadherin results in a downregulated activity of the said mechanism (Sasaki et al., 2007).
Consistently, studies on host-virus interactions also provided more context to this potential mechanism by proving that Notch has a regulatory role on midgut cell proliferation in Ae. aegypti during DENV infection. This proliferative response is referred to as endoreplication, a process that promotes tissue regeneration by producing cells with excess copies of genomic DNA (Serrato-Salas et al., 2018; Taracena et al., 2018). In a susceptible Ae. aegypti strain, delayed cellular regeneration has been observed upon DENV infection whereas induction of the midgut cell proliferation made this mosquito more resistant to the virus. Alternatively, Ae. aegypti refractory strain demonstrates higher susceptibility to DENV upon inhibition of Notch (Taracena et al., 2018). These findings therefore suggest that in Ae. aegypti the Notch specifically regulates Wolbachia’s viral blocking activity by increasing both host fitness and midgut cellular regeneration.
miRNA functions as a post-transcriptional regulator of gene expression and has a wide-ranged effect given its control over multiple genes. Some miRNAs that usually exist within the host are dysregulated while others become exclusively expressed in the presence of microbes. Successively, microbes can drive these miRNAs to alter the mosquito host’s responses as they persist inside the cell (Feng et al., 2018) (Table 2).
Studies have substantiated the impact of Wolbachia on Ae. aegypti’s miRNA profile (Hussain et al., 2011; Mayoral et al., 2014). wMelpop-CLA induces differential expression of miRNAs with exclusive induction of miR-2940 and miR-309a-2 in Wolbachia-positive mosquitoes (Hussain et al., 2011). Between the two, miR-2940 is well-known to target genes that regulate Wolbachia density (Hussain et al., 2011; Zhang et al., 2013; Zhang et al., 2014). In particular, this miRNA increases and stabilizes the expression of metalloprotease m41 ftsh gene and arginine methyl transferase 3 (AaArgM3) further enhancing wMelpop-CLA replication in both Ae. aegypti cells and mosquitoes. Inhibiting miR-2940 only led to a significant reduction of the target genes and endosymbiont (Hussain et al., 2011; Zhang et al., 2014). The specific role of this miR-2940 in arbovirus infection remains undiscovered although this miRNA is downregulated in mosquito cells to limit West Nile virus replication (Slonchak et al., 2014). Regardless, metalloprotease genes like m41 ftsh are upregulated in DENV and ZIKV-infected Ae. aegypti (Sim et al., 2012; Etebari et al., 2017) suggesting Wolbachia may be utilizing host miRNAs to control a specific host gene essential for its growth which arboviruses also need. Conversely, miR-2940 suppresses DNA methyltransferase (AaDnmt2) in wMelpop-CLA transinfected mosquitoes (Zhang et al., 2013). Some of the biological functions of AaDnmt2 are host defense, genome stability, and lifespan regulation. Presence of DENV in Ae. aegypti without the endosymbiont produces higher levels of this gene. In this case, Wolbachia creates a cellular environment unconducive or antagonistic to the virus (Zhang et al., 2013).
Meanwhile, miRNAs can also have an effect on host autophagy, viral replication, and cellular transport. As an example, wMelPop-CLA in Aag2 cells triggers marked expression of aae-miR-12 capable of suppressing two genes namely monocarboxylate transporter (MCT1) and DNA replication licensing factor (MCM6) (Osei-Amo et al., 2012). The exact role of these genes in Ae. Aegypti is unknown but existing studies in other insects reveal that MCT1 is a key player in autophagy (Velentzas et al., 2018) whereas in DENV and ZIKV infection, these viruses take over the host’s autophagy response to evade the host immune defenses (Choi et al., 2018). One possibility that requires further investigations is that Wolbachia produces a miRNA that can suppress the activity of MCT1 and therefore, autophagy.
Wolbachia infection also induces the expression of aae-miR-981 and in effect, downregulates importin β-4 in wMelPop-CLA infected Aag2. Reducing the activity of importin β-4 blocks AGO1 translocation to the nucleus (Hussain et al., 2013). There is no existing data that answers why hindering AGO1 translocation to the nucleus is advantageous for Wolbachia’s viral blocking. Nevertheless, importin β serves a different role in arboviral infection. Importin β binds to DENV and ZIKV non-structural proteins to assist in their nuclear migration for optimal replication as observed in Ae. Albopictus (Pryor et al., 2007; Ji and Luo, 2019). If this effect is the same for Ae. aegypti then the downregulation of importin β during Wolbachia infection may not only impair AGO1 translocation but also potentially block viral transcription.
Finally, Wolbachia-derived miRNAs that may contribute to viral blocking have also been reported. For instance, WsRNA-46 found in Wolbachia-infected Ae. aegypti promotes dynein expression, a cytoskeletal protein important in cellular transport, mRNA localization, and movement of lipid droplets (Mayoral et al., 2014). In both Wolbachia and arboviruses, dynein is crucial for maintaining density and infection (Baldridge et al., 2014; Mayoral et al., 2014). Given that arboviruses also use the same cytoskeletal structure for their benefit, this represents an overlapping need for the same host cellular factors.
In recent years, Wolbachia-infected Ae. aegypti are being released to control medically important arboviruses (O’Neill et al., 2019; Indriani et al., 2020). The impact of Wolbachia on arboviral inhibition has been validated by looking into disease prevalence after mass release programs have been implemented (Indriani et al., 2020) and by simulating its effect computationally (Zhang and Lui, 2020). However, elucidating the exact cellular mechanisms that block off these viruses inside the mosquito cell is still underway. The two well-established hypotheses suggest that the transinfection of Wolbachia into Ae. aegypti induces an immune reaction that fights off the invading viruses and that the endosymbiont wins the competition for scarce resources (Kambris et al., 2010; Pan et al., 2012; Caragata et al., 2016). Based on the facts presented in this review, there could be another potential explanation as to how Wolbachia blocks a pathogen. It is likely that Wolbachia directly interferes with arboviruses. For instance, Wolbachia has been discovered to reduce host proteins that help viruses enter the cell (Lu et al., 2020). Koh et al. also demonstrated this antagonistic effect when dual infection of Wolbachia and DENV in Ae. aegypti resulted in a decrease in specific types of lipids that are normally upregulated when only the virus is present (Koh et al., 2020). More so, the endosymbiont enhances ROS production (Pan et al., 2012) and cellular regeneration (Taracena et al., 2018) that can directly harm an invading pathogen as well as prevent systemic viral infection, respectively. More interestingly, these antagonistic effects are consistent with the contrasting patterns in gene expression when either microbe are present or both (Table 3).
Discovering the cellular mechanisms responsible for Wolbachia’s pathogen blocking effect remains challenging due to the complex relationship between three factors, namely the host, arboviruses, and Wolbachia. In terms of the host, Ae. aegypti colonies despite having the same strain can be highly divergent when reared in different laboratories and these laboratory-reared mosquitoes are less genetically variable compared to their field counterparts (Gloria-Soria et al., 2019). Susceptibility to viruses also vary depending on Ae. aegypti strain (Perrin et al., 2020) and possibly Wolbachia considering that some Ae. aegypti mosquitoes have been reported to carry natural infection. When it comes to arboviruses, DENV, ZIKV, and CHIKV may differ in the extent to which they can infect the host. Wolbachia pipentis also consists of several strains that may induce antiviral protection depending on the virus and the extent by which it alters host cellular mechanisms. Collectively, these make pathogen blocking mechanisms elusive.
Our understanding of Wolbachia’s pathogen blocking is mostly based on Drosophila melanogaster as a model organism highlighting the need to establish Ae. aegypti models. To determine if this vector control strategy is indeed sustainable, focusing on locations where mass release programs have been conducted could be a reliable indicator for effectiveness. Ae. aegypti mosquitoes can be collected from these sites and those Wolbachia-infected should be characterized using transcriptomic, proteomic, and metabolomic approaches. The first question that could be addressed is, what are the unique features found in field-collected, artificially Wolbachia-infected Ae. aegypti as opposed to the uninfected? This baseline information can then be used for succeeding in vitro approaches to answer the question, how are these unique genes, proteins, and metabolites affected when virus infection occurs in Wolbachia-infected cells? From this information, we can then focus on specific cellular mechanisms and validate how these are used/altered in virus-infected, Wolbachia-infected and Wolbachia+virus-infected Ae. aegypti. Most of the studies determined the role of a host cell mechanism by silencing genes under the said pathway. Alternatively, applying the CRISPR/Cas9 technology in vitro to ensure complete gene knockout may be a better approach. Taking into account natural infection, an in vitro model representing this (e.g. co-infection of isolated Wolbachia with stable Wolbachia-infected cell line prior the virus) must also be included in the experimental design. Finally, acknowledging the variable effects of multiple Wolbachia strains should prompt investigators to compare the cellular mechanisms each Wolbachia strain elicits for pathogen blocking and ask which mechanisms induce greater magnitude of viral inhibition. A targeted yet comprehensive view on the Wolbachia pathogen blocking mechanisms dissected using advanced approaches such as omics and gene editing will lead to efficient, sustainable and safe arbovirus control.
JR and KW conceptualized the review article. JR wrote and revised the manuscript with KW’s supervision. JR created the figures. YS and TC added concepts. KW, YS, TC, and MM reviewed and revised the initial drafts. All authors contributed to the article and approved the submitted version.
The publication of this article is supported by Japan Society for the Promotion of Science (JSPS) Core-to-Core Program B. Asia-Africa Science Platforms (JPJSCCB20210006) and JSPS Grant-in-Aid Fund for the Promotion of Joint International Research (Fostering Joint International Research (B)) (19KK0107).
The authors declare that the research was conducted in the absence of any commercial or financial relationships that could be construed as a potential conflict of interest.
Figures were created with BioRender.com. Preprint version of this manuscript has been submitted to https://www.preprints.org/ with DOI: 10.20944/preprints202104.0151.v1.
DENV, dengue virus; ZIKV, zika virus; CHIKV, chikungunya virus; LD, lipid droplet; Dpi, days post infection.
Adelman, Z. N., Myles, K. M. (2018). The C-Type Lectin Domain Gene Family in Aedes aegypti and Their Role in Arbovirus Infection. Viruses 10, 367. doi: 10.3390/v10070
Ahlers, L. R. H., Trammell, C. E., Carrell, G. F., Mackinnon, S., Torrevillas, B. K., Chow, C. Y., et al. (2019). Insulin Potentiates Jak/Stat Signaling to Broadly Inhibit Flavivirus Replication in Insect Vectors. Cell Rep. 29, 1946–1960.e5. doi: 10.1016/j.celrep.2019.10.029
Angleró-Rodríguez, Y. I., MacLeod, H. J., Kang, S., Carlson, J. S., Jupatanakul, N., Dimopoulos, G. (2017). Aedes Aegypti Molecular Responses to Zika Virus: Modulation of Infection by the Toll and Jak/Stat Immune Pathways and Virus Host Factors. Front. Microbiol. 8, 2050. doi: 10.3389/fmicb.2017.02050
Asad, S., Hussain, M., Hugo, L., Osei-Amo, S., Zhang, G., Watterson, D., et al. (2018). Suppression of the Pelo Protein by Wolbachia and Its Effect on Dengue Virus in Aedes Aegypti. PloS Neglected Trop. Dis. 12, e0006405. doi: 10.1371/journal.pntd.0006405
Baldridge, G. D., Baldridge, A. S., Witthuhn, B. A., Higgins, L., Markowski, T. W., Fallon, A. M. (2014). Proteomic Profiling of a Robust Wolbachia Infection in an Aedes Albopictus Mosquito Cell Line. Mol. Microbiol. 94, 537–556. doi: 10.1111/mmi.12768
Barletta, A. B. F., Alves, L. R., Nascimento Silva, M. C. L., Sim, S., Dimopoulos, G., Liechocki, S., et al. (2016). Emerging Role of Lipid Droplets in Aedes Aegypti Immune Response Against Bacteria and Dengue Virus. Sci. Rep. 6, 19928. doi: 10.1038/srep19928
Beckmann, J. F., Ronau, J. A., Hochstrasser, M. (2017). A Wolbachia Deubiquitylating Enzyme Induces Cytoplasmic Incompatibility. Nat. Microbiol. 2, 17007. doi: 10.1038/nmicrobiol.2017.7
Bonizzoni, M., Dunn, W. A., Campbell, C. L., Olson, K. E., Marinotti, O., James, A. A. (2012). Complex Modulation of the Aedes Aegypti Transcriptome in Response to Dengue Virus Infection. PloS One 7, e50512. doi: 10.1371/journal.pone.0050512
Bottino-Rojas, V., Talyuli, O. A. C., Carrara, L., Martins, A. J., James, A. A., Oliveira, P. L., et al. (2018). The Redox-Sensing Gene Nrf2 Affects Intestinal Homeostasis, Insecticide Resistance, and Zika Virus Susceptibility in the Mosquito Aedes Aegypti. J. Biol. Chem. 293, 9053–9063. doi: 10.1074/jbc.RA117.001589
Caicedo, P. A., Serrato, I. M., Sim, S., Dimopoulos, G., Coatsworth, H., Lowenberger, C., et al (2019). Immune Response-Related Genes Associated to Blocking Midgut Dengue Virus Infection in Aedes aegypti Strains That Differ in Susceptibility. Insect Sci. 26, 635–648. doi: 10.1111/1744-7917.12573
Campbell, C. L., Harrison, T., Hess, A. M., Ebel, G. D. (2014). MicroRNA Levels Are Modulated in Aedes Aegypti After Exposure to Dengue-2. Insect Mol. Biol. 23, 132–139. doi: 10.1111/imb.12070
Caragata, E. P., Dutra, H. L. C., Moreira, L. A. (2016). Exploiting Intimate Relationships: Controlling Mosquito-Transmitted Disease With Wolbachia. Trends Parasitol 32, 207–218. doi: 10.1016/j.pt.2015.10.011
Caragata, E. P., Rancès, E., Hedges, L. M., Gofton, A. W., Johnson, K. N., O’Neill, S. L., et al. (2013). Dietary Cholesterol Modulates Pathogen Blocking by Wolbachia. PloS Pathog. 9, e1003459. doi: 10.1371/journal.ppat.1003459
Caragata, E. P., Rancès, E., O’Neill, S. L., McGraw, E. A. (2014). Competition for Amino Acids Between Wolbachia and the Mosquito Host, Aedes Aegypti. Microb. Ecol. 67, 205–218. doi: 10.1007/s00248-013-0339-4
Carvajal, T. M., Hashimoto, K., Harnandika, R. K., Amalin, D. M., Watanabe, K. (2019). Detection of Wolbachia in Field-Collected Aedes Aegypti Mosquitoes in Metropolitan Manila, Philippines. Parasites Vectors 12, 361. doi: 10.1186/s13071-019-3629-y
Carvalho–Leandro, D., Ayres, C. F. J., Guedes, D.R.D., Suesdek, L., Melo-Santos, M. A. V., Oliveira, C. F. (2012). Immune Transcript Variations Among Aedes aegypti Populations with Distinct Susceptibility to Dengue Virus Serotype 2. Acta Trop. 124, 113–119. doi: 10.1016/j.actatropica.2012.07.006
Chang, C.-H., Liu, Y.-T., Weng, S.-C., Chen, I.-Y., Tsao, P.-N., Shiao, S.-H. (2018). The Non-Canonical Notch Signaling Is Essential for the Control of Fertility in Aedes Aegypti. PloS Negl. Trop. Dis. 12, e0006307. doi: 10.1371/journal.pntd.0006307
Chan, M., Johansson, M. A. (2012). The Incubation Periods of Dengue Viruses. PloS One 7, e50972. doi: 10.1371/journal.pone.0050972
Choi, Y., Bowman, J. W., Jung, J. U. (2018). Autophagy During Viral Infection — a Double-Edged Sword. Nat. Rev. Microbiol. 16, 341–354. doi: 10.1038/s41579-018-0003-6
Cho, K.-O., Kim, G.-W., Lee, O.-K. (2011). Wolbachia Bacteria Reside in Host Golgi-Related Vesicles Whose Position Is Regulated by Polarity Proteins. PloS One 6, e22703. doi: 10.1371/journal.pone.0022703
Chotiwan, N., Andre, B. G., Sanchez-Vargas, I., Islam, M. N., Grabowski, J. M., Hopf-Jannasch, A., et al. (2018). Dynamic Remodeling of Lipids Coincides With Dengue Virus Replication in the Midgut of Aedes Aegypti Mosquitoes. PloS Pathog. 14, e1006853. doi: 10.1371/journal.ppat.1006853
Chouin-Carneiro, T., Ant, T. H., Herd, C., Louis, F., Failloux, A. B., Sinkins, S. P. (2020). Wolbachia Strain wAlbA Blocks Zika Virus Transmission in Aedes Aegypti. Med. Vet. Entomol 34, 116–119. doi: 10.1111/mve.12384
Colpitts, T. M., Cox, J., Vanlandingham, D. L., Feitosa, F. M., Cheng, G., Kurscheid, S., et al (2011). Alterations in the Aedes aegypti Transcriptome During Infection with West Nile, Dengue and Yellow Fever Viruses. PLOS Pathogens 7, e1002189. doi: 10.1371/journal.ppat.1002189
Coon, K. L., Brown, M. R., Strand, M. R. (2016). Mosquitoes Host Communities of Bacteria That Are Essential for Development But Vary Greatly Between Local Habitats. Mol. Ecol. 25, 5806–5826. doi: 10.1111/mec.13877
Cui, Y., Liu, P., Mooney, B. P., Franz, A. W. E. (2020). Quantitative Proteomic Analysis of Chikungunya Virus-Infected Aedes Aegypti Reveals Proteome Modulations Indicative of Persistent Infection. J. Proteome Res. 19, 2443–2456. doi: 10.1021/acs.jproteome.0c00173
Dubey, S. K., Shrinet, J., Jain, J., Ali, S., Sunil, S. (2017). Aedes Aegypti microRNA miR-2b Regulates Ubiquitin-Related Modifier to Control Chikungunya Virus Replication. Sci. Rep. 7, 17666. doi: 10.1038/s41598-017-18043-0
Dubey, S. K., Shrinet, J., Sunil, S. (2019). Aedes Aegypti microRNA, miR-2944b-5p Interacts With 3’UTR of Chikungunya Virus and Cellular Target vps-13 to Regulate Viral Replication. PloS Neglected Trop. Dis. 13, e0007429. doi: 10.1371/journal.pntd.0007429
Espinal, M. A., Andrus, J. K., Jauregui, B., Waterman, S. H., Morens, D. M., Santos, J. I., et al. (2017). Emerging and Reemerging Aedes-Transmitted Arbovirus Infections in the Region of the Americas: Implications for Health Policy. Am J Public Health 109, 387–392. doi: 10.2105/AJPH.2018.304849
Etebari, K., Hegde, S., Saldaña, M. A., Widen, S. G., Wood, T. G., Asgari, S., et al. (2017). Global Transcriptome Analysis of Aedes Aegypti Mosquitoes in Response to Zika Virus Infection. mSphere 2. doi: 10.1128/mSphere.00456-17
Fattouh, N., Cazevieille, C., Landmann, F. (2019). Wolbachia Endosymbionts Subvert the Endoplasmic Reticulum to Acquire Host Membranes Without Triggering ER Stress. PloS Negl. Trop. Dis. 13, e0007218. doi: 10.1371/journal.pntd.0007218
Feng, X., Zhou, S., Wang, J., Hu, W. (2018). microRNA Profiles and Functions in Mosquitoes. PloS Negl. Trop. Dis. 12, e0006463. doi: 10.1371/journal.pntd.0006463
Flores, H. A., Bruyne, J. T. de, O’Donnell, T. B., Nhu, V. T., Giang, N. T., Trang, H. T. X., et al. (2020). Multiple Wolbachia Strains Provide Comparative Levels of Protection Against Dengue Virus Infection in Aedes Aegypti. PloS Pathog. 16, e1008433. doi: 10.1371/journal.ppat.1008433
Foo, K. Y., Chee, H.-Y. (2015). Interaction Between Flavivirus and Cytoskeleton During Virus Replication. BioMed. Res. Int. 2015, 427814. doi: 10.1155/2015/427814
Ford, S. A., Albert, I., Allen, S. L., Chenoweth, S. F., Jones, M., Koh, C., et al. (2020). Artificial Selection Finds New Hypotheses for the Mechanism of Wolbachia-Mediated Dengue Blocking in Mosquitoes. Front. Microbiol. 11, 1456. doi: 10.3389/fmicb.2020.01456
Ford, S. A., Allen, S. L., Ohm, J. R., Sigle, L. T., Sebastian, A., Albert, I., et al. (2019). Selection on Aedes Aegypti Alters Wolbachia -Mediated Dengue Virus Blocking and Fitness. Nat. Microbiol. 4, 1832–1839. doi: 10.1038/s41564-019-0533-3
Fraser, J. E., O’Donnell, T. B., Duyvestyn, J. M., O’Neill, S. L., Simmons, C. P., Flores, H. A. (2020). Novel Phenotype of Wolbachia Strain wPip in Aedes Aegypti Challenges Assumptions on Mechanisms of Wolbachia-mediated Dengue Virus Inhibition. PloS Pathog. 16, e1008410. doi: 10.1371/journal.ppat.1008410
Fukutani, K. F., Kasprzykowski, J. I., Paschoal, A. R., Gomes, M., de, S., Barral, A., et al. (2018). Meta-Analysis of Aedes Aegypti Expression Datasets: Comparing Virus Infection and Blood-Fed Transcriptomes to Identify Markers of Virus Presence. Front. Bioeng Biotechnol. 5, 84. doi: 10.3389/fbioe.2017.00084
Geoghegan, V., Stainton, K., Rainey, S. M., Ant, T. H., Dowle, A. A., Larson, T., et al. (2017). Perturbed Cholesterol and Vesicular Trafficking Associated With Dengue Blocking in Wolbachia -Infected Aedes Aegypti Cells. Nat. Commun. 8, 526. doi: 10.1038/s41467-017-00610-8
Gloria-Soria, A., Soghigian, J., Kellner, D., Powell, J. R. (2019). Genetic Diversity of Laboratory Strains and Implications for Research: The Case of Aedes Aegypti. PloS Neglected Trop. Dis. 13, e0007930. doi: 10.1371/journal.pntd.0007930
Guo, X., Xu, Y., Bian, G., Pike, A. D., Xie, Y., Xi, Z. (2010). Response of the Mosquito Protein Interaction Network to Dengue Infection. BMC Genomics 11, 380. doi: 10.1186/1471-2164-11-380
Haqshenas, G., Terradas, G., Paradkar, P. N., Duchemin, J.-B., McGraw, E. A., Doerig, C. (2019). A Role for the Insulin Receptor in the Suppression of Dengue Virus and Zika Virus in Wolbachia-Infected Mosquito Cells. Cell Rep. 26, 529–535.e3. doi: 10.1016/j.celrep.2018.12.068
Heaton, N. S., Perera, R., Berger, K. L., Khadka, S., Lacount, D. J., Kuhn, R. J., et al. (2010). Dengue Virus Nonstructural Protein 3 Redistributes Fatty Acid Synthase to Sites of Viral Replication and Increases Cellular Fatty Acid Synthesis. Proc. Natl. Acad. Sci. U .S. A. 107, 17345–17350. doi: 10.1073/pnas.1010811107
Hilgenboecker, K., Hammerstein, P., Schlattmann, P., Telschow, A., Werren, J. H. (2008). How Many Species Are Infected With Wolbachia? – A Statistical Analysis of Current Data. FEMS Microbiol. Lett. 281, 215–220. doi: 10.1111/j.1574-6968.2008.01110.x
Hoffmann, J. A. (2003). The Immune Response of Drosophila. Nature 426, 33–38. doi: 10.1038/nature02021
Hoornweg, T. E., Bouma, E. M., van de Pol, D. P. I., Rodenhuis-Zybert, I. A., Smit, J. M. (2020). Chikungunya Virus Requires an Intact Microtubule Network for Efficient Viral Genome Delivery. PloS Neglected Trop. Dis. 14, e0008469. doi: 10.1371/journal.pntd.0008469
Hussain, M., Frentiu, F. D., Moreira, L. A., O’Neill, S. L., Asgari, S. (2011). Wolbachia Uses Host microRNAs to Manipulate Host Gene Expression and Facilitate Colonization of the Dengue Vector Aedes Aegypti. PNAS 108, 9250–9255. doi: 10.1073/pnas.1105469108
Hussain, M., O’Neill, S. L., Asgari, S. (2013). Wolbachia Interferes With the Intracellular Distribution of Argonaute 1 in the Dengue Vector Aedes Aegypti by Manipulating the Host Micrornas. RNA Biol. 10, 1868–1875. doi: 10.4161/rna.27392
Indriani, C., Tantowijoyo, W., Rancès, E., Andari, B., Prabowo, E., Yusdi, D., et al. (2020). Reduced Dengue Incidence Following Deployments of Wolbachia-infected Aedes Aegypti in Yogyakarta, Indonesia: A Quasi-Experimental Trial Using Controlled Interrupted Time Series Analysis. Gates Open Res. 4. doi: 10.12688/gatesopenres.13122.1
Jang, I. H., Chosa, N., Kim, S. -H., Nam, H. -J., Lemaitre, B., Ochiai, M. E., et al. (2006). A Spätzle-Processing Enzyme Required for Toll Signaling Activation in Drosophila Innate Immunity. Dev. Cell 10, 45–55. doi: 10.1371/journal.ppat.1001143
Ji, W., Luo, G. (2019). Zika Virus NS5 Nuclear Accumulation Is Protective of Protein Degradation and Is Required for Viral RNA Replication. Virology 541, 124–135.. doi: 10.1016/j.virol.2019.10.010
Kambris, Z., Blagborough, A. M., Pinto, S. B., Blagrove, M. S. C., Godfray, H. C. J., Sinden, R. E., et al. (2010). Wolbachia Stimulates Immune Gene Expression and Inhibits Plasmodium Development in Anopheles Gambiae. PloS Pathog. 6, e1001143. doi: 10.1371/journal.ppat.1001143
Kamtchum-Tatuene, J., Makepeace, B. L., Benjamin, L., Baylis, M., Solomon, T. (2017). The Potential Role of Wolbachia in Controlling the Transmission of Emerging Human Arboviral Infections. Curr. Opin. Infect. Dis. 30, 108–116. doi: 10.1097/QCO.0000000000000342
Kato, N., Dasgupta, R., Smartt, C. T., Christensen, B. M. (2002). Glucosamine:Fructose-6-Phosphate Aminotransferase: Gene Characterization, Chitin Biosynthesis and Peritrophic Matrix Formation in Aedes aegypti. Insect Mol. Biol. 11, 207–216. doi: 10.1046/j.1365-2583.2002.00326.x
Koh, N., Islam, R., Ye, C. T., Chotiwan, B. M.. (2002). Glucosamine:Fructose-6-Phosphate Aminotransferase: Gene Characterization, Chitin Biosynthesis and Peritrophic Matrix Formation in Aedes aegypti. Insect Mol. Biol 11, 207–216. doi: 10.1046/j.1365-2583.2002.00326.x
Koh, C., Islam, M. N., Ye, Y. H., Chotiwan, N., Graham, B., Belisle, J. T., et al. (2020). Dengue Virus Dominates Lipid Metabolism Modulations in Wolbachia -Coinfected Aedes Aegypti. Commun. Biol. 3, 1–14. doi: 10.1038/s42003-020-01254-z
Kopan, R., Ilagan, M. X. G. (2009). The Canonical Notch Signaling Pathway: Unfolding the Activation Mechanism. Cell 137, 216–233. doi: 10.1016/j.cell.2009.03.045
Lafont, F., Goot, F. G. V. D. (2005). Bacterial Invasion Via Lipid Rafts. Cell. Microbiol. 7, 613–620. doi: 10.1111/j.1462-5822.2005.00515.x
Leier, H. C., Weinstein, J. B., Kyle, J. E., Lee, J.-Y., Bramer, L. M., Stratton, K. G., et al. (2020). A Global Lipid Map Defines a Network Essential for Zika Virus Replication. Nat. Commun. 11, 3652. doi: 10.1038/s41467-020-17433-9
LePage, D. P., Metcalf, J. A., Bordenstein, S. R., On, J., Perlmutter, J. I., Shropshire, J. D., et al. (2017). Prophage WO Genes Recapitulate and Enhance Wolbachia -Induced Cytoplasmic Incompatibility. Nature 543, 243–247. doi: 10.1038/nature21391
Lindsey, A. R. I., Bhattacharya, T., Hardy, R. W., Newton, I. L. G. (2021). Wolbachia and Virus Alter the Host Transcriptome at the Interface of Nucleotide Metabolism Pathways. mBio 12, e03472–20. doi: 10.1128/mBio.03472-20
Lindsey, A. R. I., Bhattacharya, T., Newton, I. L. G., Hardy, R. W. (2018). Conflict in the Intracellular Lives of Endosymbionts and Viruses: A Mechanistic Look at Wolbachia-Mediated Pathogen-Blocking. Viruses 10, 141. doi: 10.3390/v10040141
Lu, P., Sun, Q., Fu, P., Li, K., Liang, X., Xi, Z. (2020). Wolbachia Inhibits Binding of Dengue and Zika Viruses to Mosquito Cells. Front. Microbiol. 11, 1750. doi: 10.3389/fmicb.2020.01750
Mairiang, D., Zhang, H., Sodja, A., Murali, T., Suriyaphol, P., Malasit, P., et al. (2013). Identification of New Protein Interactions Between Dengue Fever Virus and Its Hosts, Human and Mosquito. PloS One 8, e53535. doi: 10.1371/journal.pone.0053535
Manokaran, G., Flores, H. A., Dickson, C. T., Narayana, V. K., Kanojia, K., Dayalan, S., et al. (2020). Modulation of Acyl-Carnitines, the Broad Mechanism Behind Wolbachia-Mediated Inhibition of Medically Important Flaviviruses in Aedes Aegypti. PNAS 117, 24475–24483. doi: 10.1073/pnas.1914814117
Matthews, B. J., Dudchenko, O., Kingan, S. B. , Koren, S., Antoshechkin, I., Crawford, J. E., et al (2018). Improved Reference Genome of Aedes aegypti Informs Arbovirus Vector Control. Nature 563, 501–507. doi: 10.1038/s41586-018-0692-z
Mayoral, J. G., Etebari, K., Hussain, M., Khromykh, A. A., Asgari, S. (2014). Wolbachia Infection Modifies the Profile, Shuttling and Structure of MicroRNAs in a Mosquito Cell Line. PloS One 9, e96107. doi: 10.1371/journal.pone.0096107
Molloy, J. C., Sinkins, S. P. (2015). Wolbachia Do Not Induce Reactive Oxygen Species-Dependent Immune Pathway Activation in Aedes Albopictus. Viruses 7, 4624–4639. doi: 10.3390/v7082836
Molloy, J. C., Sommer, U., Viant, M. R., Sinkins, S. P. (2016). Wolbachia Modulates Lipid Metabolism in Aedes Albopictus Mosquito Cells. Appl. Environ. Microbiol. 82, 3109–3120. doi: 10.1128/AEM.00275-16
Moreira, L. A., Iturbe-Ormaetxe, I., Jeffery, J. A., Lu, G., Pyke, A. T., Hedges, L. M., et al. (2009). A Wolbachia Symbiont in Aedes Aegypti Limits Infection With Dengue, Chikungunya, and Plasmodium. Cell 139, 1268–1278. doi: 10.1016/j.cell.2009.11.042
Mukherjee, D., Das, S., Begum, F., Mal, S., Ray, U. (2019). The Mosquito Immune System and the Life of Dengue Virus: What We Know and Do Not Know. Pathogens 8, 77. doi: 10.3390/pathogens8020077
Newton, I. L. G., Savytskyy, O., Sheehan, K. B. (2015). Wolbachia Utilize Host Actin for Efficient Maternal Transmission in Drosophila Melanogaster. PloS Pathog. 11, e1004798. doi: 10.1371/journal.ppat.1004798
Oliveira, J. H. M., Talyuli, O. A. C., Goncalves, R. L. S., Paiva-Silva, G. O., Sorgine, M. H. F., Alvarenga, P. H., et al. (2017). Catalase Protects Aedes Aegypti From Oxidative Stress and Increases Midgut Infection Prevalence of Dengue But Not Zika. PloS Negl. Trop. Dis. 11, e0005525. doi: 10.1371/journal.pntd.0005525
O’Neill, S. L., Ryan, P. A., Turley, A. P., Wilson, G., Retzki, K., Iturbe-Ormaetxe, I., et al. (2019). Scaled Deployment of Wolbachia to Protect the Community From Dengue and Other Aedes Transmitted Arboviruses. Gates Open Res. 2, 36. doi: 10.12688/gatesopenres.12844.3
Osei-Amo, S., Hussain, M., O’Neill, S. L., Asgari, S. (2012). Wolbachia-Induced aae-miR-12 miRNA Negatively Regulates the Expression of MCT1 and MCM6 Genes in Wolbachia-infected Mosquito Cell Line. PloS One 7, e50049. doi: 10.1371/journal.pone.0050049
Paingankar, M. S., Gokhale, M. D., Deobagkar, D. N. (2010). Dengue-2-virus-interacting Polypeptides Involved in Mosquito Cell Infection. Arch. Virol. 155, 1453–1461. doi: 10.1007/s00705-010-0728-7
Pan, X., Pike, A., Joshi, D., Bian, G., McFadden, M. J., Lu, P., et al. (2018). The Bacterium Wolbachia Exploits Host Innate Immunity to Establish a Symbiotic Relationship With the Dengue Vector Mosquito Aedes Aegypti. ISME J. 12, 277–288. doi: 10.1038/ismej.2017.174
Pan, X., Zhou, G., Wu, J., Bian, G., Lu, P., Raikhel, A. S., et al. (2012). Wolbachia Induces Reactive Oxygen Species (ROS)-Dependent Activation of the Toll Pathway to Control Dengue Virus in the Mosquito Aedes Aegypti. Proc. Natl. Acad. Sci. U. S. A. 109, E23–E31. doi: 10.1073/pnas.1116932108
Perrin, A., Gosselin-Grenet, A.-S., Rossignol, M., Ginibre, C., Scheid, B., Lagneau, C., et al. (2020). Variation in the Susceptibility of Urban Aedes Mosquitoes Infected With a Densovirus. Sci. Rep. 10, 18654. doi: 10.1038/s41598-020-75765-4
Pimentel, A. C., Cesar, C. S., Martins, M., Cogni, R. (2021). The Antiviral Effects of the Symbiont Bacteria Wolbachia in Insects. Front. Immunol. 11, 3690. doi: 10.3389/fimmu.2020.626329
Pryor, M. J., Rawlinson, S. M., Butcher, R. E., Barton, C. L., Waterhouse, T. A., Vasudevan, S. G., et al. (2007). Nuclear Localization of Dengue Virus Nonstructural Protein 5 Through Its Importin Alpha/Beta-Recognized Nuclear Localization Sequences is Integral to Viral Infection. Traffic 8, 795–807. doi: 10.1111/j.1600-0854.2007.00579.x
Ramesh, K., Walvekar, V. A., Wong, B., Sayed, A. M. M., Missé, D., Kini, R. M., et al. (2019). Increased Mosquito Midgut Infection by Dengue Virus Recruitment of Plasmin Is Blocked by an Endogenous Kazal-type Inhibitor. iScience 21, 564–576. doi: 10.1016/j.isci.2019.10.056
Ramirez, J. L., Dimopoulos, G. (2010). The Toll Immune Signaling Pathway Control Conserved Anti-Dengue Defenses Across Diverse Ae. aegypti Strains and Against Multiple Dengue Virus Serotypes. Dev. Comp. Immunol. 34, 625–629. doi: 10.1016/j.dci.2010.01.006
Raquin, V., Merkling, S. H., Gausson, V., Moltini-Conclois, I., Frangeul, L., Varet, H., et al. (2017). Individual Co-Variation Between Viral RNA Load and Gene Expression Reveals Novel Host Factors During Early Dengue Virus Infection of the Aedes Aegypti Midgut. PloS Neglected Trop. Dis. 11, e0006152. doi: 10.1371/journal.pntd.0006152
Riparbelli, M. G., Giordano, R., Ueyama, M., Callaini, G. (2012). Wolbachia-Mediated Male Killing Is Associated With Defective Chromatin Remodeling. PloS One 7, e30045. doi: 10.1371/journal.pone.0030045
Rodgers, F. H., Gendrin, M., Wyer, C. A. S., Christophides, G. K. (2017). Microbiota-Induced Peritrophic Matrix Regulates Midgut Homeostasis and Prevents Systemic Infection of Malaria Vector Mosquitoes. PLoS Pathog 13, e1006391. doi: 10.1371/journal.ppat.1006391
Rousset, F. H., Bouchon, M., Pintureau, C. A., Juchault, S., Solignac, G. K. (2017). Microbiota-Induced Peritrophic Matrix Regulates Midgut Homeostasis and Prevents Systemic Infection of Malaria Vector Mosquitoes. PLoS Pathog 13, e1006391. doi: 10.1371/journal.ppat.1006391
Rousset, F., Bouchon, D., Pintureau, B., Juchault, P., Solignac, M. (1992). Wolbachia Endosymbionts Responsible for Various Alterations of Sexuality in Arthropods. Proc. R. Soc. London Ser. B: Biol. Sci. 250, 91–98. doi: 10.1098/rspb.1992.0135
Royle, J., Donald, C. L., Merits, A., Kohl, A., Varjak, M. (2017). Differential Effects of Lipid Biosynthesis Inhibitors on Zika and Semliki Forest Viruses. Vet. J. 230, 62–64. doi: 10.1016/j.tvjl.2017.10.009
Saldaña, M. A., Etebari, K., Hart, C. E., Widen, S. G., Wood, T. G., Thangamani, S., et al. (2017). Zika Virus Alters the microRNA Expression Profile and Elicits an RNAi Response in Aedes Aegypti Mosquitoes. PloS Neglected Trop. Dis. 11, e0005760. doi: 10.1371/journal.pntd.0005760
Sasaki, N., Sasamura, T., Ishikawa, H. O., Kanai, M., Ueda, R., Saigo, K., et al. (2007). Polarized Exocytosis and Transcytosis of Notch During Its Apical Localization in Drosophila Epithelial Cells. Genes Cells 12, 89–103. doi: 10.1111/j.1365-2443.2007.01037.x
Schultz, M. J., Isern, S., Michael, S. F., Corley, R. B., Connor, J. H., Frydman, H. M. (2017). Variable Inhibition of Zika Virus Replication by Different Wolbachia Strains in Mosquito Cell Cultures. J. Virol. 91, e00339–17. doi: 10.1128/JVI.00339-17
Serrato–Salas, J., Hernández-Martínez, S., Martínez-Barnetche, J., Condé, R., Alvarado–Delgado, A., Zumaya–Estrada, F., et al (2019). De Novo DNA Synthesis in Aedes aegypti Midgut Cells as a Complementary Strategy to Limit Dengue Viral Replication. Front. Microbiol. 9, 801. doi: 10.3389/fmicb.2018.00801
Shaikevich, E., Bogacheva, A., Rakova, V., Ganushkina, L., Ilinsky, Y. (2019). Wolbachia Symbionts in Mosquitoes: Intra- and Intersupergroup Recombinations, Horizontal Transmission and Evolution. Mol. Phylogenet. Evol. 134, 24–34. doi: 10.1016/j.ympev.2019.01.020
Sheehan, K. B., Martin, M., Lesser, C. F., Isberg, R. R., Newton, I. L. G. (2016). Identification and Characterization of a Candidate Wolbachia Pipientis Type Iv Effector That Interacts With the Actin Cytoskeleton. mBio 7, e00622–16. doi: 10.1128/mBio.00622-16
Shrinet, J., Bhavesh, N. S., Sunil, S. (2018). Understanding Oxidative Stress in Aedes During Chikungunya and Dengue Virus Infections Using Integromics Analysis. Viruses 10, 314. doi: 10.3390/v10060314
Shrinet, J., Srivastava, P., Sunil, S. (2017). Transcriptome Analysis of Aedes Aegypti in Response to Mono-Infections and Co-Infections of Dengue Virus-2 and Chikungunya Virus. Biochem. Biophys. Res. Commun. 492, 617–623. doi: 10.1016/j.bbrc.2017.01.162
Sim, S., Dimopoulos, G. (2010). Dengue Virus Inhibits Immune Responses in Aedes aegypti Cells. PLOS ONE 5 5, e10678. doi: 10.1371/journal.pone.0010678
Sim, S., Jupatanakul, N., Ramirez, J. L., Kang, S., Romero-Vivas, C. M., Mohammed, H., et al. (2013). Transcriptomic Profiling of Diverse Aedes Aegypti Strains Reveals Increased Basal-Level Immune Activation in Dengue Virus-Refractory Populations and Identifies Novel Virus-Vector Molecular Interactions. PloS Negl. Trop. Dis. 7, e2295. doi: 10.1371/journal.pntd.0002295
Sim, S., Ramirez, J. L., Dimopoulos, G. (2012). Dengue Virus Infection of the Aedes Aegypti Salivary Gland and Chemosensory Apparatus Induces Genes That Modulate Infection and Blood-Feeding Behavior. PloS Pathog. 8, e1002631. doi: 10.1371/journal.ppat.1002631
Slonchak, A., Hussain, M., Torres, S., Asgari, S., Khromykh, A. A. (2014). Expression of Mosquito Microrna Aae-miR-2940-5p Is Downregulated in Response to West Nile Virus Infection To Restrict Viral Replication. J. Virol. 88, 8457–8467. doi: 10.1128/JVI.00317-14
Suwanmanee, S., Chaisri, U., Wasinpiyamongkol, L., Luplertlop, N. (2009). Peritrophic Membrane Structure of Aedes aegypti (Diptera: Culicidae) Mosquitoes After Infection With Dengue Virus Type 2 (D2-16681). Appl. Entomol. Zool. 44,257–265. doi: 10.1303/aez.2009.257
Taracena, M. L., Bottino-Rojas, V., Talyuli, O. A. C., Walter-Nuno, A. B., Oliveira, J. H. M., Angleró-Rodriguez, Y. I., et al. (2018). Regulation of Midgut Cell Proliferation Impacts Aedes Aegypti Susceptibility to Dengue Virus. PloS Negl. Trop. Dis. 12, e0006498. doi: 10.1371/journal.pntd.0006498
Tchankouo-Nguetcheu, S., Khun, H., Pincet, L., Roux, P., Bahut, M., Huerre, M., et al. (2010). Differential Protein Modulation in Midguts of Aedes Aegypti Infected With Chikungunya and Dengue 2 Viruses. PloS One 5, e13149. doi: 10.1371/journal.pone.0013149
Velentzas, P. D., Zhang, L., Das, G., Chang, T.-K., Nelson, C., Kobertz, W. R., et al. (2018). The Proton-Coupled Monocarboxylate Transporter Hermes Is Necessary for Autophagy During Cell Death. Dev. Cell 47, 281–293.e4. doi: 10.1016/j.devcel.2018.09.015
Walsh, D., Naghavi, M. H. (2019). Exploitation of Cytoskeletal Networks During Early Viral Infection. Trends Microbiol. 27, 39–50. doi: 10.1016/j.tim.2018.06.008
Wang, J.-M., Cheng, Y., Shi, Z.-K., Li, X.-F., Xing, L.-S., Jiang, H., et al. (2019). Aedes Aegypti HPX8C Modulates Immune Responses Against Viral Infection. PloS Negl. Trop. Dis. 13, e0007287. doi: 10.1371/journal.pntd.0007287
Weeks, A. R., Breeuwer, J. A. (2001). Wolbachia-Induced Parthenogenesis in a Genus of Phytophagous Mites. Proc. Biol. Sci. 268, 2245–2251. doi: 10.1098/rspb.2001.1797
White, P. M., Pietri, J. E., Debec, A., Russell, S., Patel, B., Sullivan, W. (2017). Mechanisms of Horizontal Cell-to-Cell Transfer of Wolbachia Spp. in Drosophila Melanogaster. Appl. Environ. Microbiol. 83, e03425–16. doi: 10.1128/AEM.03425-16
White, S. R., Pietri, W. K., Debec, R. F., Russell, Z. N. (2018). Characterization of the Adult Aedes aegypti Early Midgut Peritrophic Matrix Proteome Using LC-MS. PLOS ONE 13. 83, e0194734. doi: 10.1371/journal.pone.0194734
Xi, Z., Ramirez, J. L., Dimopoulos, G. (2008). The Aedes aegypti Toll Pathway Controls Dengue Virus Infection. PLOS Pathogens 4 4, e1000098. doi: 10.1371/journal.ppat.1000098
Zhang, G., Hussain, M., Asgari, S. (2014). Regulation of Arginine Methyltransferase 3 by a Wolbachia-Induced microRNA in Aedes Aegypti and Its Effect on Wolbachia and Dengue Virus Replication. Insect Biochem. Mol. Biol. 53, 81–88. doi: 10.1016/j.ibmb.2014.08.003
Zhang, G., Hussain, M., O’Neill, S. L., Asgari, S. (2013). Wolbachia Uses a Host microRNA to Regulate Transcripts of a Methyltransferase, Contributing to Dengue Virus Inhibition in Aedes Aegypti. Proc. Natl. Acad. Sci. U. S. A. 110, 10276–10281. doi: 10.1073/pnas.1303603110
Zhang, H., Lui, R. (2020). Releasing Wolbachia-Infected Aedes aegypti to Prevent the Spread of Dengue Virus: A Mathematical Study. Infect. Dis. Model. 5, 142–160. doi: 10.1016/j.idm.2019.12.004
Keywords: Aedes aegypti, dengue, zika, chikungunya, Wolbachia, vector control
Citation: Reyes JIL, Suzuki Y, Carvajal T, Muñoz MNM and Watanabe K (2021) Intracellular Interactions Between Arboviruses and Wolbachia in Aedes aegypti. Front. Cell. Infect. Microbiol. 11:690087. doi: 10.3389/fcimb.2021.690087
Received: 02 April 2021; Accepted: 31 May 2021;
Published: 23 June 2021.
Edited by:
Natarajaseenivasan Kalimuthusamy, Bharathidasan University, IndiaReviewed by:
Rhys Harold Parry, The University of Queensland, AustraliaCopyright © 2021 Reyes, Suzuki, Carvajal, Muñoz and Watanabe. This is an open-access article distributed under the terms of the Creative Commons Attribution License (CC BY). The use, distribution or reproduction in other forums is permitted, provided the original author(s) and the copyright owner(s) are credited and that the original publication in this journal is cited, in accordance with accepted academic practice. No use, distribution or reproduction is permitted which does not comply with these terms.
*Correspondence: Kozo Watanabe, watanabe.kozo.mj@ehime-u.ac.jp
Disclaimer: All claims expressed in this article are solely those of the authors and do not necessarily represent those of their affiliated organizations, or those of the publisher, the editors and the reviewers. Any product that may be evaluated in this article or claim that may be made by its manufacturer is not guaranteed or endorsed by the publisher.
Research integrity at Frontiers
Learn more about the work of our research integrity team to safeguard the quality of each article we publish.