- 1Center of Infectious Diseases, Parasitology, Heidelberg University Hospital, Heidelberg, Germany
- 2Department of Biochemistry and Molecular Biology and Research Institute Hospital 12 de Octubre (Imas12), Universidad Complutense de Madrid, Madrid, Spain
After a century of constant failure to produce an in vitro culture of the most widespread human malaria parasite Plasmodium vivax, recent advances have highlighted the difficulties to provide this parasite with a healthy host cell to invade, develop, and multiply under in vitro conditions. The actual level of understanding of the heterogeneous populations of cells—framed under the name ‘reticulocytes’—and, importantly, their adequate in vitro progression from very immature reticulocytes to normocytes (mature erythrocytes) is far from complete. The volatility of its individual stability may suggest the reticulocyte as a delusory cell, particularly to be used for stable culture purposes. Yet, the recent relevance gained by a specific subset of highly immature reticulocytes has brought some hope. Very immature reticulocytes are characterized by a peculiar membrane harboring a plethora of molecules potentially involved in P. vivax invasion and by an intracellular complexity dynamically changing upon its quick maturation into normocytes. We analyze the potentialities offered by this youngest reticulocyte subsets as an ideal in vitro host cell for P. vivax.
Introduction
Plasmodium vivax (P. vivax) is the reigning malaria-causing parasite outside the African continent (Gallup and Sachs, 2001). The strong morbidity burden carried by populations living in areas endemic for this understudied parasite rebounds in the chronic impoverishment and underdevelopment of these communities (WHO, 2016). For a long time, P. vivax has been considered as the causing agent of the historically—yet inaccurately—termed “benign malaria”. This inappropriate stigma has disregarded P. vivax at the end of the row in terms of malaria research priorities (Mueller et al., 2009). Happily, in the last decade, there have been remarkable efforts to promote P. vivax research for its capacity to remain dormant in the liver in the form of hyponozites for long periods of time and then relapse (Krotoski, 1985). Insights into intriguing biological features of P. vivax, such as the real contribution of the hematopoietic niches in bone marrow and spleen (with extramedullary erythropoiesis potentially occurring under adverse conditions, including malaria) as homes for a significant proportion of parasite biomass, are also on the increase (Silva-Filho et al., 2020). Advancment in this field is very much warranted due to the potential of the reticulocyte’s intracellular environment to trigger the sexual commitment of P. vivax (Obaldia et al., 2018) and subsequent importance for transmission and eradication efforts. For this and its very early sexual commitment in transmissible-stage gametocytes (Adapa et al., 2019), it seems plausible that P. vivax may become the last human Plasmodium parasite standing before the goal of malaria eradication is achieved (Tanner et al., 2015).
A much-needed tool is still missing to boost research into P. vivax’s intrinsic biological and pathophysiological singularities to the level that we have today for Plasmodium falciparum (P. falciparum), for which an in vitro culture system for blood stages has existed for more than 40 years (Trager and Jensen, 1976). This availability has allowed us to genetically unravel P. falciparum’s genes functionalities through already in place forward and reverse genetic approaches (Kirchner et al., 2016; Zhang et al., 2018), proteomics (Bautista et al., 2014), or immunomics (Doolan, 2011), which can ultimately lead to more rational development of new antimalarial drugs and promotion of vaccines candidates (Bourgard et al., 2018).
Several breakthroughs in the cultivation of P. knowlesi (Moon et al., 2013; Grüring et al., 2014) and P. cynomolgi (Chua et al., 2019) have been achieved in the last decade. As for P. vivax, we are still missing a reliable method for its in vitro culture; the major impediment has been our inability to efficiently handle it under in vitro culture conditions its sole target cell for asexual blood-stage replication: the reticulocyte (Thomson-Luque et al., 2019). Improved methods for reticulocyte enrichment from different sources have been provided (Vettore et al., 1980; Brun et al., 1990; Kumar et al., 2015; Shaw-Saliba et al., 2016), as well as the production of reticulocytes through better optimized hematopoietic stem cell (HSC) cultures (Giarratana et al., 2005, Noulin et al., 2013; Scully et al., 2019) and immortalized lines (Satchwell et al., 2019; Heshusius et al., 2019; Trakarnsanga et al., 2020). The lack of a more efficient enucleation (Menon and Ghaffari, 2021) can be overcome by genetic complementation (Scully et al., 2019). Humanized mouse models, such as the human liver-chimeric FRG KO huHep to recapitulate the liver, and blood-stage cycles of P. vivax (Mikolajczak et al., 2015; Schäfer et al., 2020) are readily available, although at a high cost and low efficiency in terms of blood-stage breakthrough; the liver stage in vitro systems (Roth et al., 2018) are currently being optimized to unravel the mechanism of hypnozoite production, though again at a high cost. Furthermore, non-human primate monkey models, such as Aotus, Saimiri, and Rhesus, are also a possibility to study this parasite in vivo (Shaw-Saliba et al., 2016; Pasini and Kocken, 2021), although rising ethics concerns makes this model only available to certain facilities. Thus, all expectations are put on the development of affordable in vitro cultures, and, for this, a substantial leap in healthily handling in vitro reticulocytes to offer P. vivax the right host cell capable of providing specific receptors and an intracellular niche for the parasite to mature and replicate, is still needed. This is the way forward.
The Reticulocyte: not a Specific Cell Type but a Continuum in Maturation Difficult to Reproduce In Vitro
The persisting and adverse scenario of the lack of an in vitro culture system for P. vivax is indicative of the lack of understanding of the reticulocyte biology in vitro. Far from a homogeneous cluster of erythroid cells, reticulocytes are a population in constant phenotypical change. The most immature reticulocytes formed in the bone marrow’s erythroblastic islands continuously develop, both internally in its cytoplasm as well as in its external surface membrane, to finally become, while in circulation, fully mature red blood cells (RBC) called normocytes (Figure 1). Different approaches aimed at characterizing these dynamic reticulocytes have (imperfectly) attempted to establish a classification to by focusing on different features of reticulocytes. The earliest, the Heilmeyer staging I-V focused on their microscopical appearance after New Methylene Blue (NMB) staining (Heilmeyer and Westha üser, 1932). Later, they were classified as R1 vs R2 reticulocytes groups based on the shape and movement of reticulocytes in live cell cytology studies (Mel et al., 1977). More recently, the amount of transferrin receptor 1 (TfR1 or CD71) expression in the membrane of reticulocytes (Brun et al., 1990; Kono et al., 2009) has become the current trending classification. Remarkably, all three phenotypic viewpoints must be acknowledged and taken into consideration when attempting to unravel keys to efficiently sustain healthy reticulocyte populations in an in vitro culture system.
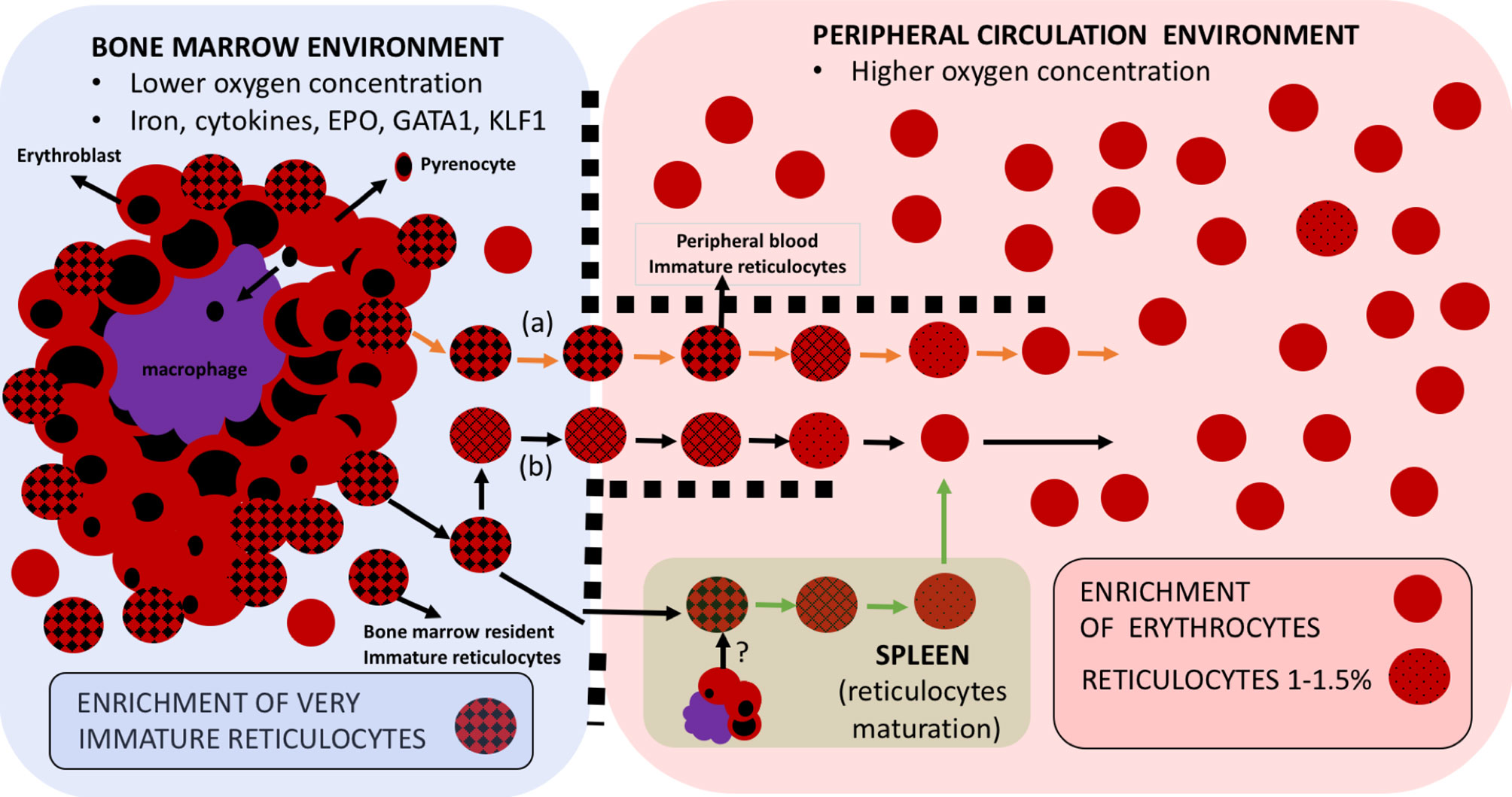
Figure 1 Erythroid cell maturation occurs in different compartments. Reticulocytes emerge in the hypoxic bone marrow compartment upon their predecessor cell in the erythroid lineage, the orthochromatic erythroblasts, expelling its nucleus, in the form of a pyrenocyte, which will be thereafter engulfed by the key scaffold cell of the erythroblastic islands, the central macrophage. These macrophages also play an important role in secreting cytokines that will contribute to the maturation of the whole erythroid lineage from erythroid committed stem cells to CFU/BFU and all the way to reticulocytes. Some recently-enucleated reticulocytes may leave the bone marrow to start maturation in peripheral blood (A), But mostly they start maturing within the bone marrow compartment (B) to ultimately progress to fully mature RBCs in the peripheral blood compartment with higher oxygen concentration. Immature reticulocytes, sometimes referred to as CD71high reticulocytes are thus preferentially enriched in the bone marrow but can also be found in peripheral circulation. The spleen represents a hematopoietic organ with potential for erythropoiesis under stress circumstances and where reticulocyte maturation is postulated to happen.
Maintaining, under in vitro conditions, the correct fitness of these heterogeneous cells at all steps throughout their developmental continuum is thus paramount to advance in establishing a P. vivax in vitro culture; but this turns out to not be an easy task. Not enough importance has been given to the fact that the widely accepted 2–3 days necessary for a correct in vivo maturation of reticulocytes (Seip, 1953) takes place in between two compartments with the divergent environment. For instance, from the low oxygen concentration niche at the bone marrow, reticulocytes depart to a highly oxygenated milieu into the peripheral blood where they finally reach and transform into normocytes (Tugba et al., 2010). This maturation when pursued in vitro is very much inhibited (Malleret et al., 2013). Size and morphological discrepancies are also observed between ex vivo (Malleret et al., 2013) and in vitro maturation (Griffiths et al., 2012). Further exploration into adjusting the in vitro development of reticulocytes under hypoxic culture conditions, at least partially during the first steps of maturation, is desirable to satisfactorily provide reticulocytes with an ideal environment from the very first moments of its maturation to finally obtain the healthiest cell that could support efficient parasite invasion and correct development.
CD71high Reticulocytes: a Promising Reticulocyte Subtype to Identify Missing Receptors/Co-Receptors
Our limited knowledge of reticulocyte molecular mediators of invasion by P. vivax contrasts with the vast repertoire reported for P. falciparum invasion (Beeson et al., 2016). The clear role of the Duffy antigen receptor for chemokines (DARC/CD234) in red blood cells (RBCs) as a receptor for the DBL domain in Region II of P. vivax (DBPII) is clearly established (Adams et al., 1992). However, DARC is present in both reticulocytes and normocytes and its protein expression does not change during reticulocyte maturation. The use of monoclonal antibodies to specific epitopes within DARC has pointed at an increased DARC-DBP binding site accessibility in reticulocytes and, importantly, in very immature reticulocytes (Ovchynnikova et al., 2017). Yet, the strict tropism of P. vivax for reticulocytes (Kitchen, 1939; Mons, 1990) cannot be totally explained by this well-described molecular interaction. Several receptors/co-receptors-ligand interactions have been envisioned to unravel P. vivax invasion pathway/s (Ménard et al., 2013; Ntumngia et al., 2016). Recently, CD71 present in younger reticulocytes (CD71+ reticulocytes) has been promoted as the receptor for the P. vivax ligand reticulocyte-binding protein 2b (RBP2b), shedding stronger insight into the strict reticulocyte attraction by P. vivax (Gruszczyk et al., 2018a and Gruszczyk et al., 2018b). The suggested dependency on CD71 for invasion has furthermore re-fueled the idea that a great proportion of P. vivax biomass resides in hematopoietic organs, such as the bone marrow (Baird, 2013) (and potentially the spleen, contributing to the final steps of reticulocyte maturation) (Rhodes et al., 2016; Toda et al., 2020). These are environments full of the younger CD71+ reticulocytes and, particularly, the homes of a subset of reticulocytes whose surfaces are extremely enriched in CD71: the CD71high reticulocytes. These most immature reticulocytes are nowadays considered as the key reticulocyte subset to unlock the way for obtaining an in vitro culture system for P. vivax.
Although an attractive proposal, there is currently no definitive in vivo evidence on a clear tropism of P. vivax to invade the youngest reticulocytes when infecting humans. Since the first report on the presence of P. vivax in bone marrow aspirates in humans (Marchiafava and Bignami, 1984), the following findings of this parasite in hematopoietic organs till present have been merely incidental (Lacerda et al., 2008; Baro et al., 2017). Histological analysis in a nonhuman primate model has just reflected a moderate enrichment of asexual stages in the parenchyma (Obaldia et al., 2018). These data cannot firmly support that the subset of reticulocytes being invaded in vivo are only bone marrow-residing CD71high. Yet, in an in vitro experimental setting, an immature CD71high reticulocyte subpopulation as preferentially chosen for invasion by P. vivax (Malleret et al., 2015). This has likewise been demonstrated for the reticulocyte-prone rodent malaria parasite Plasmodium yoelii 17X NL (Martín-Jaular et al., 2013). This finding has promoted investigating molecules present in the membrane of CD71high that may act as yet-unreported receptors involved in P. vivax invasion.
Phenotypical characterization of reticulocytes in cord blood samples has tightly measured the abundance of many different RBC surface markers among different subsets showing that reticulocyte maturation is concomitant with decreasing CD71 expression (Malleret et al., 2013; Wilson et al., 2016; Chu et al., 2018). This has been later expanded to peripheral blood and bone marrow samples to study a broader set of markers (Thomson-Luque et al., 2018), and corroborating that, although at low levels compared to bone marrow, reticulocytes with very high CD71 loads can be released very rapidly to peripheral blood (even during the first 30 minutes after detaching from erythroblastic islands when assuming a linear age distribution) (Ovchynnikova et al., 2017) (Figure 1). Therefore, if P. vivax invasion is specifically restricted to reticulocytes with the highest CD71 expression, replication could also occur in peripheral circulation. Other surface molecules such as CD49d (α4β1integrin), which is drastically lost at the very early hours of reticulocyte maturation, CD44 (Indian blood group), and CD98 are enriched in the most immature CD71high reticulocytes (Griffiths et al., 2012). Consequently, wide cell-surface screenings are particularly relevant if some of these molecules are ever promoted as a potential receptor to explore.
Young Reticulocytes: the Keystone for P. vivax Infection of the Duffy Negative African Population?
P. vivax infections have long been considered to be inexistent in the African continent (Miller et al., 1976). The clear contrast in the geographic distribution among P. vivax and the deadliest species in Africa P. falciparum has been historically based on the imperative of P. vivax to invade human populations positive for DARC, which presence is very limited in Africa (Howes et al., 2011). Yet, initial reports have put on the table the potential ability of P. vivax to cause disease all the way from East (Bray, 1958; Ryan et al., 2006; Ménard et al., 2010; Woldearegai et al., 2013; Lover, 2014) to Central (Culleton et al., 2009; Wurtz et al., 2011) and West Africa (Fru-Cho et al., 2014; Niangaly et al., 2017; and extensively reviewed in Popovici et al., 2020). The question of how P. vivax merozoites invade reticulocytes from African populations not carrying DARC (Gunalan et al., 2018) can be answered through in vitro dissections of invasion ligand/host receptor interactions.
Studies of various culture conditions in red cells have revealed switching mechanisms in mature RBCs invasion by P. falciparum (Dolan et al., 1990). Recently, by blocking reticulocytes’ DARC and TfR1 receptors in short-term cultures of P. vivax isolates, a significant variation in receptor usage was observed, suggesting that P. vivax may use alternative invasion pathways (Kanjee et al., 2020). Immature reticulocytes clearly represent the right cell to search for these alternative pathways’ mediators.
Moreover, the leaky expression of DARC on Duffy negative (Duffy-) RBCs has been previously suggested (Gunalan et al., 2020) as a potential explanation for P. vivax invasion into Duffy-reticulocytes. Some DARC- individuals may not be fully Duffy-null, as residual RNA transcription may still happen marginally (Popovici et al., 2020). This phenomenon has recently been described in bone marrow-derived DARC- RBC progenitors (Dechavanne et al., 2018). The possibility that some very immature DARC- reticulocytes in the bone marrow, but also in peripheral blood (Thomson-Luque et al., 2018), harbor marginal expression of DARC tempts us to speculate that they may be the explanation behind the possibility of transmission between Duffy- individuals infected with P. vivax. This is further supported if the molecules characteristic of reticulocyte immaturity that they carry have indeed a role as alternative receptors/co-receptors. However, aside from receptor molecules, other players constitutive of the immature reticulocyte membrane may need to be looked into and taken care of under in vitro conditions.
A Constant Remodeling of the Reticulocyte Membrane in Vitro is Needed
A healthy reticulocyte membrane is not just needed as a cytoskeletal platform [composed of networking molecules spectrin, actin, tubulin, ankyrin, adducin, tropomyosin, and tropomodulin, linking the major structural elements protein 4.1 and band 3 (Liu et al., 2010)] to anchor receptor molecules. Such a structured membrane is also requested to support the biophysical requirements for invasion, establishing the correct tension for the DBP-DARC tight junction and, potentially, of unidentified receptor/ligand interactions, to efficiently interact (Kariuki et al., 2020). DARC expression dependency on the junctional complex with protein 4.1 supports this fact, as protein 4.1 deficiency reduces the expression of DARC (Salomao et al., 2008; Azouzi et al., 2015). The gradual remodeling of the reticulocyte membrane’s nanostructure (Li et al., 2018), involving the loss of up to one-third of its surface area (Griffiths et al., 2012), has been widely studied with the use of a variety of approaches, such as SEM, TEM, micropipette aspiration, and atomic force microscopy (Malleret et al., 2013; Malleret et al., 2015). Yet again, very little is known of the correct cytoskeleton maturation of reticulocytes in vitro, with membrane dismembering being common under standard P. vivax culture settings (Thomson-Luque et al., 2017). A decrease in osmotic stability has been shown to be a major cause for the loss of structural integrity of reticulocytes undergoing in vitro maturation in HSC cultures (Clark et al., 2021). This suggests that immature reticulocytes are more osmotically stable, pointing to an advantage for P. vivax to develop when invading these cell subtypes (Figure 2). Yet, a relevant uncertainty remains as to whether reticulocyte’s membrane maturation is modified as a consequence of the P. vivax infection itself.
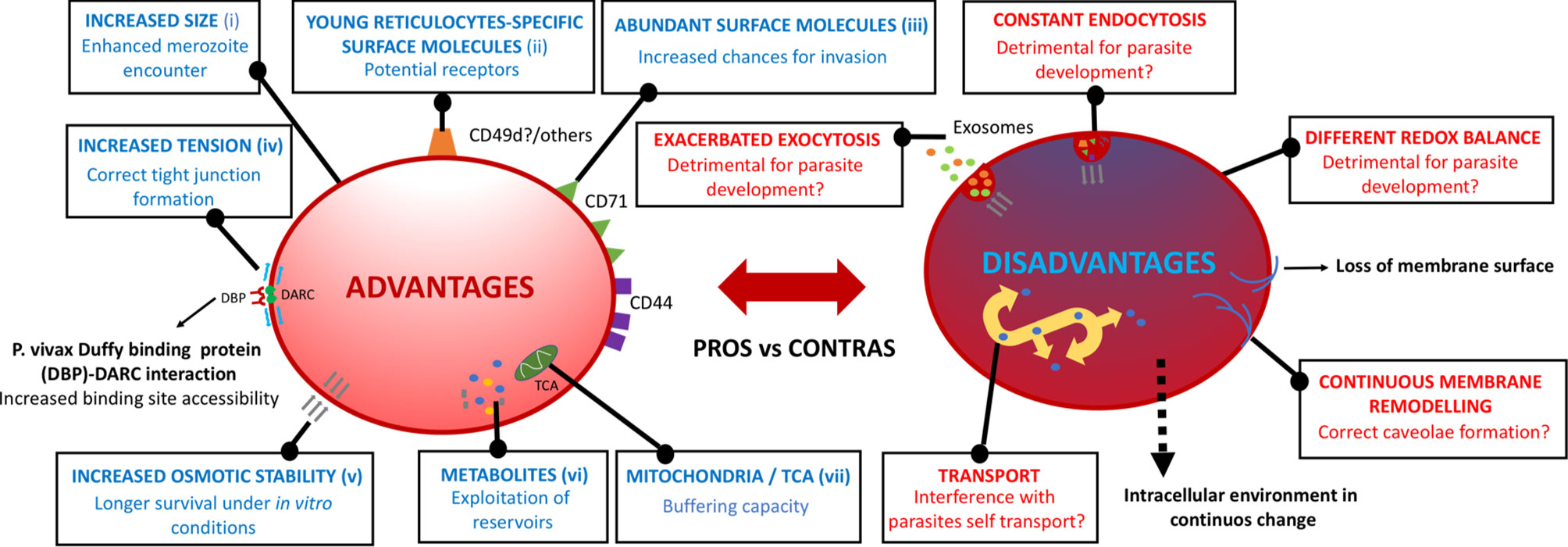
Figure 2 Potential advantages and disadvantages of immature reticulocytes. Surface and intracellular phenotypic features at the very initial steps of very immature reticulocyte maturation that may confer/impede the P. vivax parasite’s subsequent physiological progression throughout their intra-reticulocytic developmental cycle. (i) Griffiths et al., 2012, (ii) Thomson-Luque et al., 2018, (iii) Malleret et al., 2013, (iv) Kariuki et al., 2020, (v) Clark et al., 2021, (vi) Srivastava et al., 2015, (vii) Starkov, 2008.
A reported acceleration in the maturation of cord blood-derived reticulocytes triggered by P. vivax right after merozoite invasion in vitro has described a fast decline of CD71 from the surface of P. vivax-infected CD71+ reticulocytes (Malleret et al., 2015). This is accompanied by rapid loss of the inner reticulae (a conglomerate of polyribosomes, RNA, endothelium reticulum, and sometimes mitochondria) 3 hours post-invasion. These events are found to go hand to hand with a concomitant shift in membrane nanostructure components, from clathrin pit-enriched towards caveolae-enriched infected reticulocyte membrane, as the parasite matures in an in vitro environment. In the context of these findings we need to consider the following: (i) the accelerated disappearance of CD71 is not homogeneous, and some P. vivax late stages are inside some CD71+ reticulocytes; and (ii) the discrepancy with findings of P. vivax late stages inside NMB+ reticulocytes (Lim et al., 2016) as well as inside CD71+ reticulocytes (Clark et al., 2021) in patients’ field isolates. In the Aotus lemurinus lemurinus monkey malaria model (Shaw-Saliba et al., 2016), co-staining of in vivo samples containing P. vivax infected reticulocytes with both Giemsa and supravital New Methylene blue revealed that in Aotus monkeys in vivo P. vivax Sal-1 of different developmental stage can be found inside reticulocytes of different maturity (from Heilmeyer I to IV). In addition, the second generation of these parasites in vitro cultured for 20 hours right after bleeding an infected monkey were also found inside Heilmeyer II to III reticulocytes [a subtype described as part of a CD71+ subpopulation of reticulocytes sorted by flow cytometry (Malleret et al., 2013)]. Yet, different P. vivax strains may vary largely in their reticulocyte preference (Lim et al., 2016) and infection variability regarding DARC polymorphisms (Fyb and Fya) should not be looked aside. Whether the fast remodeling is indeed triggered by the parasite or it is just an in vitro effect warrants further exploration; and furthermore, to answer as to what extent we need to experimentally pursue these fast dynamic changes in order to achieve the fittest in vitro culture possible for P. vivax.
The Inner Intracellular Environment of Immature Reticulocytes and its Remodeling Pace
Fast events occurring in the membrane of reticulocytes upon maturation are highly intertwined with the massive inner remodeling. Understanding the intra-host cell environment and its corresponding changes upon the different maturity states of reticulocytes, and especially in the initial steps of CD71high maturation, is crucial as these singular cells may provide metabolic reservoirs for P. vivax to take advantage upon developmental advancement (Srivastava et al., 2015) (Figure 2). In cord blood, for example, differing levels of amino acids, nucleotides, and sugars, among others, have been found in the different age-related subsets of reticulocytes, with decreasing concentrations as the reticulocyte matures (Darghouth et al., 2011; Malleret et al., 2013). Active metabolic pathways have been shown to remain in reticulocytes, whilst they tend to disappear in normocytes (Srivastava et al., 2017). Thus, the correct metabolite content and redox balance need to be mimicked in an in vitro setting as well, especially at the first steps of maturation, as slight differences may alter a wide range of fast processes occurring both in the membrane as well as in the cytoplasm.
Deleterious metabolic conditions in cultured reticulocytes may have a consequence in the clathrin pits-originated endocytosis mechanism for the sorting of disposable membrane proteins, such as CD71. Consequently, not leading to the ideal formation of multi-vesicular bodies due to the malfunction of the endosomal sorting complex required for transport, or even their correct fusion to the membrane, can have an effect on P. vivax invasion and development (Rieu et al., 2000). An altered redox regulation may also affect the ubiquitin-proteasome degradation pathway required for degrading cytosolic actin and tubulin (Liu et al., 2010). A functional tricarboxylic acid cycle (TCA cycle) is present in reticulocytes, consistent with the presence of residual mitochondria in the most immature subsets but lost thereafter (Srivastava et al., 2017) through a process of mitophagy (Lee et al., 2012). Whether the presence of mitochondria in the younger subset of reticulocytes is beneficial or detrimental for P. vivax to progress in the asexual cycle is also unknown. In favor of immature reticulocytes, mitochondrial by-products may be scavenged by the parasite for its own benefit, while the loss of mitochondrial observed in older reticulocytes could lead to the lack of enough buffering capacity against reactive oxygen species excess (Starkov, 2008) during parasite development, and therefore triggering host cell and parasite damage.
Discussion: the Need for a More in-Depth Understanding of Immature Reticulocyte Fitness In Vitro
The advantage of using the youngest of the reticulocytes for facilitating P. vivax in vitro invasion seems sound, as its densely populated surface carries molecules potentially functioning as receptors. Due to the longer time to progress to normocytes, young reticulocytes can contribute to ameliorate the technical challenge of parasitemia dilution at every sub-culturing cycle resulting from the addition of new reticulocytes to the system characteristic of P. vivax cultures. The youngest reticulocytes can also provide the parasite with an extra supply of metabolites and a specific environment that is progressively lost as the reticulocytes mature to a low synthesizing cell. Some uncertainty may arise regarding the extent to which the hemoglobin provided by very young reticulocytes is enough for P. vivax as a source of amino acids. Yet, this would not seem a problem as P. vivax development inside even more immature nucleated erythroid cells, such as polychromatic erythroblasts, has been proven (Panichakul et al., 2007).
There have been a plethora of studies aiming at determining optimal culture media components to be used for P. vivax in vitro cultures to sustain not only parasite development but correct host cell survival (Roobsoong et al., 2015; Rangel et al., 2018; Thomson-Luque et al., 2019; Clark et al., 2021). A specific culture media recipe may as well need to be rationally designed and tested to specifically keep physiological reticulocyte remodeling (extensively reviewed in Thomson-Luque et al., 2019). Promoting this subpopulation of reticulocytes for its use for in vitro culture will require investing in an expensive and still inefficient large-scale isolation and storage, which is not available in every laboratory. More experimentation is clearly deserved on sustaining a parallel and healthy reticulocyte maturation of both its surface as well as internal components under in vitro conditions. Are both of these cytoplasmic and membrane maturations needed for P. vivax to develop inside? Is there a certain rate for a fine-tuned progression of P. vivax inside this delusory host cell? These are the key questions to be addressed; in order to gain more confidence in immature reticulocytes for achieving P. vivax culture in vitro, we must first discard that some of the described observations on the parasite’s biology are not just an artifact of non-viable reticulocytes in a non-optimized in vitro environment.
Author Contributions
RT-L and JB wrote the manuscript. RT-L is funded by the European Union’s Horizon 2020 Research and Innovation Programme under Marie Skłodowska-Curie grant agreement DLV-839998. All authors contributed to the article and approved the submitted version.
Funding
RT-L is funded by the European Union’s Horizon 2020 Research and Innovation Programme under Marie Skłodowska-Curie grant agreement DLV-839998. Part of this work has been funded by grant BIO2016-77430-R from the Ministerio de Economía y Competitividad (Spain).
Conflict of Interest
The authors declare that the research was conducted in the absence of any commercial or financial relationships that could be construed as a potential conflict of interest.
References
Adams, J. H., Sim, B. K., Dolan, S. A., Fang, X., Kaslow, D. C., Miller, L. H. (1992). A Family of Erythrocyte Binding Proteins of Malaria Parasites. Proc. Natl. Acad. Sci. U.S.A. 89, 7085–7089. doi: 10.1073/pnas.89.15.7085
Adapa, S. R., Taylor, R. A., Wang, C., Thomson-Luque, R., Johnson, L. R., Jiang, R. H. Y. (2019). Plasmodium Vivax Readiness to Transmit: Implication for Malaria Eradication. BMC Syst. Biol. 13 (1), 5. doi: 10.1186/s12918-018-0669-4
Azouzi, S., Collec, E., Mohandas, N., An, X., Colin, Y., Le Van Kim, C. (2015). The Human Kell Blood Group Binds the Erythroid 4.1R Protein: New Insights Into the 4.1R-dependent Red Cell Membrane Complex. Br. J. Haematol 171 (5), 862–871. doi: 10.1111/bjh.13778
Baird, J. (2013). Evidence and Implications of Mortality Associated With Acute Plasmodium Vivax Malaria. Clin. Microbiol. Rev. 26 (1), 36–57. doi: 10.1128/cmr.00074-12
Baro, B., Deroost, K., Raiol, T., Brito, M., Almeida, A. C., de Menezes-Neto, A., et al. (2017). Plasmodium Vivax Gametocytes in the Bone Marrow of an Acute Malaria Patient and Changes in the Erythroid miRNA Profile. PloS Negl. Trop. Dis. 11, e0005365. doi: 10.1371/journal.pntd.0005365
Bautista, J. M., Marín-García, P., Diez, A., Azcárate, I. G., Puyet, A. (2014). Malaria Proteomics: Insights Into the Parasite-Host Interactions in the Pathogenic Space. J. Proteomics 97, 107–125. doi: 10.1016/j.jprot.2013.10.011
Beeson, J. G., Drew, D. R., Boyle, M. J., Feng, G., Fowkes, F. J. I., Richards, J. S. (2016). Merozoite Surface Proteins in Red Blood Cell Invasion, Immunity and Vaccines Against Malaria. FEMS Microbiol. Rev. 40, 343–372. doi: 10.1093/femsre/fuw001
Bourgard, C., Albrecht, L., Kayano, A. C. A. V., Sunnerhagen, P., Costa, F. T. M. (2018). Plasmodium Vivax Biology: Insights Provided by Genomics, Transcriptomics and Proteomics. Front. Cell Infect. Microbiol. 8, 34. doi: 10.3389/fcimb.2018.00034
Bray, R. S. (1958). The Susceptibility of Liberians to the Madagascar Strain of Plasmodium Vivax. J. Parasitol 44 (4), 371–373. doi: 10.2307/3274317
Brun, A., Gaudernack, G., Sandberg, S. (1990). A New Method for Isolation of Reticulocytes: Positive Selection of Human Reticulocytes by Immunomagnetic Separation. Blood 76 (11), 2397–2403. doi: 10.1182/blood.V76.11.2397.2397
Chua, A. C. Y., Ong, J. J. Y., Malleret, B., Suwanarusk, R., Kosaisavee, V., Zeeman, A. M., et al. (2019). Robust Continuous In Vitro Culture of the Plasmodium Cynomolgi Erythrocytic Stages. Nat. Commun. 10 (1), 3635. doi: 10.1038/s41467-019-11332-4
Chu, T. T. T., Sinha, A., Malleret, B., Suwanarusk, R., Park, J. E., Naidu, R., et al. (2018). Quantitative Mass Spectrometry of Human Reticulocytes Reveal Proteome-Wide Modifications During Maturation. Br. J. Haematol. 180 (1), 118–133. doi: 10.1111/bjh.14976
Clark, M. A., Kanjee, U., Rangel, G. W., Chery, L., Mascarenhas, A., Gomes, E., et al. (2021). Plasmodium Vivax Infection Compromises Reticulocyte Stability. Nat. Commun. 12 (1), 1629. doi: 10.1038/s41467-021-21886-x
Culleton, R., Ndounga, M., Zeyrek, F. Y., Coban, C., Casimiro, P. N., Takeo, S., et al. (2009). Evidence for the Transmission of Plasmodium Vivax in the Republic of the Congo, West Central Africa. J. Infect. Dis. 200 (9), 1465–1469. doi: 10.1086/644510
Darghouth, D., Koehl, B., Madalinski, G., Heilier, J. F., Bovee, P., Xu, Y., et al. (2011). Pathophysiology of Sickle Cell Disease is Mirrored by the Red Blood Cell Metabolome. Blood 117, e57–e66. doi: 10.1182/blood-2010-07-299636
Dechavanne, C., Dechavanne, S., Metral, S., Roeper, B., Krishnan, S., Fong, R., et al. (2018). Duffy Antigen Expression in Erythroid Bone Marrow Precursor Cells of Genotypically Duffy Negative Individuals. BioRxiv. doi: 10.1101/508481
Dolan, S. A., Miller, L. H., Wellems, T. E. (1990). Evidence for a Switching Mechanism in the Invasion of Erythrocytes by Plasmodium Falciparum. J. Clin. Invest 86 (2), 618–624. doi: 10.1172/JCI114753
Doolan, D. L. (2011). Plasmodium Immunomics. Int. J. Parasitol. 41 (1), 3–20. doi: 10.1016/j.ijpara.2010.08.002
Fru-Cho, J., Bumah, V., Safeukui, I., Nkuo-Akenji, T., Titanji, V., Haldar, K. (2014). Molecular Typing Reveals Substantial Plasmodium Vivax Infection in Asymptomatic Adults in a Rural Area of Cameroon. Malaria J. 13 (1), 170. doi: 10.1186/1475-2875-13-170
Gallup, J., Sachs, J. (2001). The Economic Burden of Malaria. Am. J. Trop. Med. Hyg 64 (1_Suppl), 85–96. doi: 10.4269/ajtmh.2001.64.85
Giarratana, M., Kobari, L., Lapillonne, H., et al. (2005). Ex Vivo Generation of Fully Mature Human Red Blood Cells From Hematopoietic Stem Cells. Nat. Biotechnol. 23, 69–74. doi: 10.1038/nbt1047
Griffiths, R. E., Kupzig, S., Cogan, N., Mankelow, T. J., Betin, V. M., Trakarnsanga, K., et al. (2012). Maturing Reticulocytes Internalize Plasma Membrane in Glycophorin A-containing Vesicles That Fuse With Autophagosomes Before Exocytosis. Blood 119 (26), 6296–6306. doi: 10.1182/blood-2011-09-376475
Grüring, C., Moon, R. W., Lim, C., Holder, A. A., Blackman, M. J., Duraisingh, M. T. (2014). Human Red Blood Cell-Adapted Plasmodium Knowlesi Parasites: A New Model System for Malaria Research. Cell Microbiol. 16 (5), 612–620. doi: 10.1111/cmi.12275
Gruszczyk, J., Huang, R. K., Chan, L.-J., Menant, S., Hong, C., Murphy, J. M., et al. (2018b). Cryo-EM Structure of an Essential Plasmodium Vivax Invasion Complex. Nature 559, 135–139. doi: 10.1038/s41586-018-0249-1
Gruszczyk, J., Kanjee, U., Chan, L.-J., Menant, S., Malleret, B., Lim, N. T. Y., et al. (2018a). Transferrin Receptor 1 is a Reticulocyte-Specific Receptor for Plasmodium Vivax. Science 359, 48–55. doi: 10.1126/science.aan1078
Gunalan, K., Niangaly, A., Thera, M. A., Doumbo, O. K., Miller, L. H. (2018). Plasmodium Vivax Infections of Duffy Negative Erythrocytes: Historically Undetected or a Recent Adaptation? Trends Parasitol. 34, 420–429. doi: 10.1016/j.pt.2018.02.006
Gunalan, K., Rowley, E. H., Miller, L. H. (2020). A Way Forward for Culturing Plasmodium Vivax. Trends Parasitol 36 (6), 512–519. doi: 10.1016/j.pt.2020.04.002
Heilmeyer, L., Westha üser, R. (1932). Reifungsstadien an UbÂerlebenden Reticu- Lozyten In Vitro Und Ihre Bedeutung FR Die Schaetzung Der Täglichen Haemoglobin-Produktion In Vivo. Ztschr Klin Med. 121, 361–379.
Heshusius, S., Heideveld, E., Burger, P., Thiel-Valkhof, M., Sellink, E., Varga, E., et al. (2019). Large-Scale In Vitro Production of Red Blood Cells From Human Peripheral Blood Mononuclear Cells. Blood Adv. 3 (21), 3337–3350. doi: 10.1182/bloodadvances.2019000689
Howes, R., Patil, A., Piel, F., Nyangiri, O. A., Kabaria, C. W., Gething, P. W., et al. (2011). The Global Distribution of the Duffy Blood Group. Nat. Commun. 2, 266. doi: 10.1038/ncomms1265
Kanjee, U., Grüring, C., Babar, P., Meyers, A., Dash, R., Pereira, L., et al. (2020). Plasmodium Vivax Strains Use Alternative Pathways for Invasion. J. Infect. Dis. 17, jiaa592. doi: 10.1093/infdis/jiaa592
Kariuki, S. N., Marin-Menendez, A., Introini, V., Ravenhill, B. J., Lin, Y. C., Macharia, A., et al. (2020). Red Blood Cell Tension Protects Against Severe Malaria in the Dantu Blood Group [Published Online Ahead of PrinSep 16]. Nature 585 (7826), 579–583. doi: 10.1038/s41586-020-2726-6. 10.1038/s41586-020-2726-6.
Kirchner, S., Power, B. J., Waters, A. P. (2016). Recent Advances in Malaria Genomics. and Epigenomics. Genome Med. 8, 92. doi: 10.1186/s13073-016-0343-7
Kitchen, S. (1939). The Infection of Mature and Immature Erythrocytes By Plasmodium Falciparum And Plasmodium Malariae. Am. J. Trop. Med. Hyg 1, 47–62. doi: 10.4269/ajtmh.1939.s1-19.47
Kono, M., Kondo, T., Takagi, Y., Wada, A., Fujimoto, K. (2009). Morphological definition of CD71 positive reticulocytes by various staining techniques and electron microscopy compared to reticulocytes detected by an automated hematology analyzer. Clin. Chim. Acta. 404 (2), 105–110. doi: 10.1016/j.cca.2009.03.017
Krotoski, W. A. (1985). Discovery of the Hypnozoite and a New Theory of Malarial Relapse. Trans. R Soc. Trop. Med. Hyg 79, 1–11. doi: 10.1016/0035-9203(85)90221-4
Kumar, A. A., Lim, C., Moreno, Y., Mace, C. R., Syed, A., Van Tyne, D., et al. (2015). Enrichment of Reticulocytes From Whole Blood Using Aqueous Multiphase Systems of Polymers. Am. J. Hematol. 90, 31–36. doi: 10.1002/ajh.23860
Lacerda, M. V., Hipólito, J. R., Passos, L. N. (2008). Chronic Plasmodium Vivax Infection in a Patient With Splenomegaly and Severe Thrombocytopenia. Rev. Soc Bras. Med. Trop. 41, 522–523. doi: 10.1590/S0037-86822008000500021
Lee, J., Giordano, S., Zhang, J. (2012). Autophagy, Mitochondria and Oxidative Stress: Crosstalk and Redox Signalling. Biochem. J. 441, 523–540. doi: 10.1042/BJ20111451
Lim, C., Pereira, L., Saliba, K. S., Mascarenhas, A., Maki, J. N., Chery, L., et al. (2016). Reticulocyte Preference and Stage Development of Plasmodium Vivax Isolates. J Infect. Dis. 214 (7), 1081–1084. doi: 10.1093/infdis/jiw303
Liu, J., Guo, X., Mohandas, N., Chasis, J. A., An, X. (2010). Membrane Remodeling During Reticulocyte Maturation. Blood 115 (10), 2021–2027. doi: 10.1182/blood-2009-08-241182
Li, H., Yang, J., Chu, T. T., Naidu, R., Lu, L., Chandramohanadas, R., et al. (2018). Cytoskeleton Remodeling Induces Membrane Stiffness and Stability Changes of Maturing Reticulocytes. Biophys. J. 114 (8), 2014–2023. doi: 10.1016/j.bpj.2018.03.004
Lover, A. A. (2014). Note on the Origin of the Madagascar Strain of Plasmodium Vivax. Am. J. Trop. Med. Hyg 91 (6), 1283–. doi: 10.4269/ajtmh.14-0507
Malleret, B., Li, A., Zhang, R., Tan, K. S. W., Suwanarusk, R., Claser, C., et al. (2015). Plasmodium Vivax: Restricted Tropism and Rapid Remodeling of CD71-positive Reticulocytes. Blood 125, 1314–1324. doi: 10.1182/blood-2014-08-596015
Malleret, B., Xu, F., Mohandas, N., Suwanarusk, R., Chu, C., Leite, J. A., et al. (2013). Significant Biochemical, Biophysical and Metabolic Diversity in Circulating Human Cord Blood Reticulocytes. PloS One 8, e76062. doi: 10.1371/journal.pone.0076062
Martín-Jaular, L., Elizalde-Torrent, A., Thomson-Luque, R., Ferrer, M., Segovia, C., Herreros-Aviles, E., et al. (2013). Reticulocyte-Prone Malaria Parasites Predominantly Invade CD71hi Immature Cells: Implications for the Development of an In Vitro Culture for Plasmodium Vivax. Malar J. 12, 434. doi: 10.1186/1475-2875-12-434
Mel, H. C., Prenant, M., Mohandas, N. (1977). Reticulocyte Motility and Form: Studies on Maturation and Classification. Blood 49, 1001–1009. doi: 10.1182/blood.V49.6.1001.1001
Ménard, D., Barnadas, C., Bouchier, C., Henry-Halldin, C., Gray, L. R., Ratsimbasoa, A., et al. (2010). Plasmodium Vivax Clinical Malaria is Commonly Observed in Duffy-negative Malagasy People. Proc. Natl. Acad. Sci. 107 (13), 5967–5971. doi: 10.1073/pnas.0912496107
Ménard, D., Chan, E. R., Benedet, C., Ratsimbasoa, A., Kim, S., Chim, P., et al. (2013). Whole Genome Sequencing of Field Isolates Reveals a Common Duplication of the Duffy Binding Protein Gene in Malagasy Plasmodium Vivax Strains. PloS Negl. Trop. Dis. 7, e2489. doi: 10.1371/journal.pntd.0002489
Menon, V., Ghaffari, S. (2021). Erythroid Enucleation: A Gateway Into a “Bloody” World. Exp. Hematol. 95, 13–22. doi: 10.1016/j.exphem.2021.01.001
Mikolajczak, S. A., Vaughan, A. M., Kangwanrangsan, N., Roobsoong, W., Fishbaugher, M., Yimamnuaychok, N., et al. (2015). Plasmodium Vivax Liver Stage Development and Hypnozoite Persistence in Human Liver-Chimeric Mice. Cell Host Microbe 17 (4), 526–535. doi: 10.1016/j.chom.2015.02.011
Miller, L. H., Mason, S. J., Clyde, D. F., McGinniss, M. H. (1976). The Resistance Factor to Plasmodium Vivax in Blacks. The Duffy-Blood-Group Genotype, Fyfy. N Engl. J. Med. 295 (6), 302–304. doi: 10.1056/NEJM197608052950602
Mons, B. (1990). Preferential Invasion of Malarial Merozoites Into Young Red Blood Cells. Blood Cells 16, 299–312.
Moon, R. W., Hall, J., Rangkuti, F., Ho, Y. S., Almond, N., Mitchell, G. H., et al. (2013). Adaptation of the Genetically Tractable Malaria Pathogen Plasmodium Knowlesi to Continuous Culture in Human Erythrocytes. Proc. Natl. Acad. Sci. U.S.A. 110, 531–536. doi: 10.1073/pnas.1216457110
Mueller, I., Galinski, M. R., Baird, J. K., Carlton, J. M., Kochar, D. K., Alonso, P. L., et al. (2009). Key Gaps in the Knowledge of Plasmodium Vivax, a Neglected Human Malaria Parasite. Lancet Infect. Dis. 9, 555–566. doi: 10.1016/S1473-3099(09)70177-X
Niangaly, A., Karthigayan, G., Amed, O., Coulibaly, D., Sá, J. M., Adams, M., et al. (2017). Plasmodium Vivax Infections Over 3 Years in Duffy Blood Group Negative Malians in Bandiagara, Mali. Am. J. Trop. Med. Hyg 97 (3), 744–752. doi: 10.4269/ajtmh.17-0254
Noulin, F., Borlon, C., Van Den Abbeele, J., D’Alessandro, U., Erhart, A. (2013). 1912–2012: A Century of Research on Plasmodium Vivax In Vitro Culture. Trends Parasitol 29, 286–294. doi: 10.1016/j.pt.2013.03.012
Ntumngia, F. B., Thomson-Luque, R., Torres, L. D. M., Gunalan, K., Carvalho, L. H., Adams, J. H. (2016). A Novel Erythrocyte Binding Protein of Plasmodium Vivax Suggests an Alternate Invasion Pathway Into Duffy-positive Reticulocytes. mBio 7, e01261. doi: 10.1128/mBio.01261-16
Obaldia, N., Meibalan, E., Sa, J. M., Ma, S., Clark, M. A., Mejia, P., et al. (2018). Bone Marrow is a Major Parasite Reservoir in Plasmodium Vivax Infection. mBio 9, e00625–e00618. doi: 10.1128/mBio.00625-18
Ovchynnikova, E., Aglialoro, F., Bentlage, A. E. H., Vidarsson, G., Salinas, N. D., von Lindern, M., et al. (2017). DARC extracellular domain remodeling in maturating reticulocytes explains Plasmodium vivax tropism. Blood 130 (12), 1441–1444. doi: 10.1182/blood-2017-03-774364
Panichakul, T., Sattabongkot, J., Chotivanich, K., Sirichaisinthop, J., Cui, L., Udomsangpetch, R. (2007). Production of Erythropoietic Cells In Vitro for Continuous Culture of Plasmodium Vivax. Int. J. Parasitol. 37 (14), 1551–1557. doi: 10.1016/j.ijpara.2007.05.009
Pasini, E. M., Kocken, C. H. M. (2021). Parasite-Host Interaction and Pathophysiology Studies of the Human Relapsing Malarias Plasmodium vivax and Plasmodium ovale Infections in Non-Human Primates. Front. Cell Infect. Microbiol. 10, 614122. doi: 10.3389/fcimb.2020.614122
Popovici, J., Roesch, C., Rougeron, V. (2020). The Enigmatic Mechanisms by Which Plasmodium Vivax Infects Duffy-negative Individuals. PLoS Pathog. 16 (2), e1008258. doi: 10.1371/journal.ppat.1008258
Rangel, G. W., Clark, M. A., Kanjee, U., Lim, C., Shaw-Saliba, K., Menezes, M. J., et al. (2018). Enhanced Ex Vivo Plasmodium Vivax Intraerythrocytic Enrichment and Maturation for Rapid and Sensitive Parasite Growth Assays. Antimicrob. Agents Chemother. 62, e02519. doi: 10.1128/AAC.02519-17
Rhodes, M. M., Koury, S. T., Kopsombut, P., Alford, C. E., Price, J. O., Koury, M. J. (2016). Stress Reticulocytes Lose Transferrin Receptors by an Extrinsic Process Involving Spleen and Macrophages. Am. J. Hematol. 91, 875–882. doi: 10.1002/ajh.24421
Rieu, S., Géminard, C., Rabesandratana, H., Sainte-Marie, J., Vidal, M. (2000). Exosomes released during reticulocyte maturation bind to fibronectin via integrin α4β1. Eur. J. Biochem. 267, 583–590. doi: 10.1046/j.1432-1327.2000.01036.x
Roobsoong, W., Tharinjaroen, C. S., Rachaphaew, N., Chobson, P., Schofield, L., Cui, L., et al. (2015). Improvement of Culture Conditions for Long-Term In Vitro Culture of Plasmodium Vivax. Malar J. 14, 297. doi: 10.1186/s12936-015-0815-z
Roth, A., Maher, S. P., Conway, A. J., Ubalee, R., Chaumeau, V., Andolina, C., et al. (2018). Author Correction: A comprehensive model for assessment of liver stage therapies targeting Plasmodium vivax and Plasmodium falciparum. Nat. Commun. 9 (1), 2317. doi: 10.1038/s41467-018-04817-1
Ryan, J. R., Stoute, J. A., Amon, J., Dunton, R. F., Mtalib, R., Koros, J., et al. (2006). Evidence for Transmission of Plasmodium Vivax Among a Duffy Antigen Negative Population in Western Kenya. Am. J. Trop. Med. Hyg 75 (4), 575–581. doi: 10.4269/ajtmh.2006.75.575
Salomao, M., Zhang, X., Yang, Y., Lee, S., Hartwig, J. H., Chasis, J. A., et al. (2008). Protein 4.1R-dependent multiprotein complex: new insights into the structural organization of the red blood cell membrane. Proc. Natl. Acad. Sci. U.S.A. 105 (23), 8026–8031.
Satchwell, T. J., Wright, K. E., Haydn-Smith, K. L., Sánchez-Román Terán, F., Moura, P. L., Hawksworth, J., et al. (2019). Genetic manipulation of cell line derived reticulocytes enables dissection of host malaria invasion requirements. Nat. Commun. 10 (1), 3806. doi: 10.1038/s41467-019-11790-w
Schäfer, C., Roobsoong, W., Kangwanrangsan, N., Bardelli, M., Rawlinson, T. A., Dambrauskas, N., et al. (2020). A Humanized Mouse Model for Plasmodium vivax to Test Interventions that Block Liver Stage to Blood Stage Transition and Blood Stage Infection. iScience 23 (8), 101381. doi: 10.1016/j.isci.2020.101381
Scully, E. J., Shabani, E., Rangel, G. W., Grüring, C., Kanjee, U., Clark, M. A., et al. (2019). Generation of an Immortalized Erythroid Progenitor Cell Line From Peripheral Blood: A Model System for the Functional Analysis of Plasmodium Spp. invasion. Am. J. Hematol. 94 (9), 963–974. doi: 10.1002/ajh.25543
Seip, M. (1953). “Chapter V : The reticulocyte maturation in the peripheral blood,” in Acta Medica Scandinavica 146, vol. 119. , 6296–6306.
Shaw-Saliba, K., Thomson-Luque, R., Obaldía N, I. I. I., Nuñez, M., Dutary, S., Lim, C., et al. (2016). Insights Into an Optimization of Plasmodium Vivax Sal-1 In Vitro Culture: The Aotus Primate Model. PloS Negl. Trop. Dis. 10, e0004870. doi: 10.1371/journal.pntd.0004870
Silva-Filho, J. L., Lacerda, M. V. G., Recker, M., Wassmer, S. C., Marti, M., Costa, F. T. M. (2020). Plasmodium vivax in Hematopoietic Niches: Hidden and Dangerous. Trends Parasitol 36 (5), 447–458. doi: 10.1016/j.pt.2020.03.002
Srivastava, A., Creek, D. J., Evans, K. J., De Souza, D., Schofield, L., Müller, S., et al. (2015). Host Reticulocytes Provide Metabolic Reservoirs That can be Exploited by Malaria Parasites. PLoS Pathog. 11 (6), e1004882. doi: 10.1371/journal.ppat.1004882
Srivastava, A., Evans, K. J., Sexton, A. E., Schofield, L., Creek, D. J. (2017). Metabolomics-Based Elucidation of Active Metabolic Pathways in Erythrocytes and HSC-Derived Reticulocytes. J Proteome Res. 16 (4), 1492–1505. doi: 10.1021/acs.jproteome.6b00902
Starkov, A. A. (2008). The role of mitochondria in reactive oxygen species metabolism and signaling. Ann. N Y Acad. Sci. 1147, 37–52. doi: 10.1196/annals.1427.015
Tanner, M., Greenwood, B., Whitty, C. J. M., Ansah, E. K., Price, R. N., Dondorp, A. M., et al. (2015). Malaria Eradication and Elimination: Views on How to Translate a Vision Into Reality. BMC Med. 13, 167. doi: 10.1186/s12916-015-0384-6
Thomson-Luque, R., Adams, J. H., Kocken, C. H. M., Pasini, E. M. (2019). From Marginal to Essential: The Golden Thread Between Nutrient Sensing, Medium Composition and Plasmodium Vivax Maturation in In Vitro Culture. Malar J. 18 (1), 344. doi: 10.1186/s12936-019-2949-x
Thomson-Luque, R., Shaw Saliba, K., Kocken, C. H. M., Pasini, E. M. (2017). A Continuous, Long-Term Plasmodium Vivax In Vitro Blood-Stage Culture: What are We Missing? Trends Parasitol. 33 (12), 921–924. doi: 10.1016/j.pt.2017.07.001
Thomson-Luque, R., Wang, C., Ntumngia, F. B., Xu, S., Szekeres, K., Conway, A., et al. (2018). In-Depth Phenotypic Characterization of Reticulocyte Maturation Using Mass Cytometry. Blood Cells Mol. Dis. 72, 22–33. doi: 10.1016/j.bcmd.2018.06.004
Toda, H., Diaz-Varela, M., Segui-Barber, J., Roobsoong, W., Baro, B., Garcia-Silva, S., et al. (2020). Plasma-derived extracellular vesicles from Plasmodium vivax patients signal spleen fibroblasts via NF-kB facilitating parasite cytoadherence. Nat. Commun. 11, 1 2761. doi: 10.1038/s41467-020-16337-y
Trager, W., Jensen, J. (1976). Human Malaria Parasites in Continuous Culture. Science 193, 673–675. doi: 10.1126/science.781840
Trakarnsanga, K., Tipgomut, C., Metheetrairut, C., Wattanapanitch, M., Khuhapinant, A., Poldee, S., et al. (2020). Generation of an Immortalised Erythroid Cell Line From Haematopoietic Stem Cells of a Haemoglobin E/β-Thalassemia Patient. Sci. Rep. Oct 810 (1), 16798. doi: 10.1038/s41598-020-73991-4
Tugba, S., Kocabas, F., Zheng, J., DeBerardinis, R. J., Mahmoud, A. I., Olson, E. N., et al. (2010). The Distinct Metabolic Profile of Hematopoietic Stem Cells Reflects Their Location in a Hypoxic Niche. Cell Stem Cell 7 (3), 380–390. doi: 10.1016/j.stem.2010.07.011
Vettore, L., De Matteis, M. C., Zampini, P. (1980). A New Density Gradient System for the Separation of Human Red Blood Cells. Am. J. Hematol. 8 (3), 291–297. doi: 10.1002/ajh.2830080307
WHO (2016). The global technical strategy for malaria 2016–2030 (Geneva: World Health Organization).
Wilson, M. C., Trakarnsanga, K., Heesom, K. J., Cogan, N., Green, C., Toye, A. M., et al. (2016). Comparison of the proteome of adult and cord erythroid cells, and changes in the proteome following reticulocyte maturation. Mol. Cell Proteomics 15 (6), 1938–1946. doi: 10.1074/mcp.M115.057315
Woldearegai, T. G., Kremsner, P. G., Kun, J. F., Mordmüller, B. (2013). Plasmodium Vivax Malaria in Duffy-negative Individuals From Ethiopia. Trans. R. Soc. Trop. Med. Hyg 107 (5), 328–331. doi: 10.1093/trstmh/trt016
Wurtz, N., Mint Lekweiry, K., Bogreau, H., Pradines, B., Rogier, C., Ould Mohamed Salem Boukhary, A., et al. (2011). Vivax Malaria in Mauritania Includes Infection of a Duffy-negative Individual. Malar J. 10 (1), 336. doi: 10.1186/1475-2875-10-336
Keywords: malaria, reticulocytes, host cell, Plasmodium vivax, fitness
Citation: Thomson-Luque R and Bautista JM (2021) Home Sweet Home: Plasmodium vivax-Infected Reticulocytes—The Younger the Better? Front. Cell. Infect. Microbiol. 11:675156. doi: 10.3389/fcimb.2021.675156
Received: 02 March 2021; Accepted: 16 April 2021;
Published: 13 May 2021.
Edited by:
Robert William Moon, London School of Hygiene and Tropical Medicine, United KingdomReviewed by:
Benoit Malleret, National University of Singapore, SingaporeWanlapa Roobsoong, Mahidol University, Thailand
Copyright © 2021 Thomson-Luque and Bautista. This is an open-access article distributed under the terms of the Creative Commons Attribution License (CC BY). The use, distribution or reproduction in other forums is permitted, provided the original author(s) and the copyright owner(s) are credited and that the original publication in this journal is cited, in accordance with accepted academic practice. No use, distribution or reproduction is permitted which does not comply with these terms.
*Correspondence: Richard Thomson-Luque, cmljaGFyZHRob21zb25sdXF1ZUBnbWFpbC5jb20=