- 1Division of Cell Biology and Immunology, CSIR-Institute of Microbial Technology, Chandigarh, India
- 2Immunology Laboratory, Center for Biomedical Engineering, Indian Institute of Technology, Ropar, India
For a long time, tuberculosis (TB) has been inflicting mankind with the highest morbidity and mortality. Although the current treatment is extremely potent, a few bacilli can still hide inside the host mesenchymal stem cells (MSC). The functional capabilities of MSCs are known to be modulated by TLRs, NOD-2, and RIG-1 signaling. Therefore, we hypothesize that modulating the MSC activity through TLR-4 and NOD-2 can be an attractive immunotherapeutic strategy to eliminate the Mtb hiding inside these cells. In our current study, we observed that MSC stimulated through TLR-4 and NOD-2 (N2.T4) i) activated MSC and augmented the secretion of pro-inflammatory cytokines; ii) co-localized Mtb in the lysosomes; iii) induced autophagy; iv) enhanced NF-κB activity via p38 MAPK signaling pathway; and v) significantly reduced the intracellular survival of Mtb in the MSC. Overall, the results suggest that the triggering through N2.T4 can be a future method of immunotherapy to eliminate the Mtb concealed inside the MSC.
Introduction
Tuberculosis (TB) is the cause of 2 million deaths each year and an estimated 1.8 billion people with latent disease worldwide (Mwaba et al., 2020). It is one of the top 10 diseases in terms of high morbidity and mortality worldwide (Herbert et al., 2014). Currently, drug-resistant TB is a major threat to mankind and quite common in TB endemic countries viz., India and China. Even though the available drugs remain the mainstay for the treatment of TB, certain limitations, such as their narrow therapeutic index and the associated toxicities, dilute their effectiveness (Forget and Menzies, 2006; Trauner et al., 2014). Due to its long duration, many patients fail to abide by the current regimen and quit before the completion of the course. This leads to the development of the very lethal drug-resistant TB (Munro et al., 2007). Innate and adaptive immune responses are responsible for protecting against invading pathogens. Early events include the engulfment of Mtb by the alveolar macrophages and dendritic cells, followed by their bactericidal mechanisms, such as the generation of reactive nitrogen intermediates (RNI) and reactive oxygen intermediates (ROI) (Sia and Rengarajan, 2019). Cytokines (IFN-γ, TNF-α, IL-6, IL-12, IL-17, and IL-23) and chemokines (CCL2, CCL3, CCL5, CXCL8, and CXCL10) help in restricting the Mtb burden and recruiting other immune cells to the site (Peters and Ernst, 2003; Saunders and Britton, 2007). Mtb has successfully evolved specialized immune evasion strategies that permit it to establish, multiply, and extend its infection within the host. Macrophages are the primary cells for Mtb infection. The bug acquires various strategies to persist in a dormant state in the hostile environment of the macrophage by inhibiting phagosome-lysosome (PL) fusion and de-acidification of lysosomes; thus, it averts its degradation and killing (Russell, 2013). Another mechanism of circumvention is the neutralization of reactive oxygen radicals by secretion of powerful anti-oxidants (Kumar et al., 2011). Most importantly, the lipid-rich cell wall of Mtb shields it from various defensive mechanisms. Thus, it becomes difficult to eliminate the latent form of Mtb with the current regimen and demands an urgent need for novel remedies for treating TB (Gomez and McKinney, 2004).
Recently, many studies have illustrated that bone marrow mesenchymal stem cells (MSCs) may provide a niche for shielding latent Mtb. MSCs are multipotent cells with a prospect to differentiate into adipocytes, osteocytes, chondrocytes, and neuronal cells. In the murine model of TB, rapid dissemination of Mtb was noticed, after aerosol exposure from the primary infection sites to bone marrow; where it infected MSCs (Garhyan et al., 2015). The murine model of TB dormancy demonstrates long-term intracellular viability and maintenance of Mtb in the MSCs (Das et al., 2013). It has been demonstrated that MSCs have a high number of ATP-binding cassette (ABC) transporter efflux pumps, which expel anti-TB drugs from host cells. Interestingly, viable Mtb is seen in the MSCs of patients, who had undergone successful anti-TB chemotherapy (Beamer et al., 2014). Thus, it can be inferred from these findings that Mtb can successfully hide in the MSCs until it gets ambient conditions to reactivate itself.
Innate immunity is the first line of defense, which subsequently imparts a significant impact on adaptive immune responses (Hoebe et al., 2004). Toll-like receptors (TLRs), NOD-like receptors (NOD-2), and RIG-like receptors (RIG-1) serve as a frontline defense system to defend against pathogens. These potentiate the ability of innate cells to recognize and subsequently respond to microbial infections (Kawai and Akira, 2011). Our group has already shown the importance of various innate signaling molecules against Mtb (Khan et al., 2016b; Khan et al., 2016c; Pahari et al., 2016; Pahari et al., 2020). MSCs substantially express an array of innate receptors like TLRs, NOD-2, or RIG-1 (Kim et al., 2010; Lei et al., 2011; Yang et al., 2013). TLRs are well-defined molecules that play an important role in the differentiation and self-renewal of MSC (Hwa Cho et al., 2006; Pevsner-Fischer et al., 2007). Recently, the switch of pro-inflammatory from anti-inflammatory polarization was also accredited to the activation of MSCs by delivering signals through TLRs (Waterman et al., 2010). This shows that the MSCs can be stimulated by signaling through TLRs, NLRs, and RIGs.
Based on the above-mentioned findings, the current study was designed to exploit the immunomodulatory potential of TLR-4 and NOD-2 in eliminating Mtb concealed inside the MSCs. Interestingly, signaling MSCs through NOD-2 and TLR-4 exhibited augmented secretion of pro-inflammatory cytokines, improved co-localization of Mtb in lysosomes, and significantly cleared the intracellularly masked mycobacterium. Mechanistically, stimulation of MSCs through NOD-2 and TLR-4 activated NF-κB activity via the p38 MAPK pathway and induced autophagy. In future, this novel strategy of host-directed therapy may open new avenues to eradicate Mtb hidden within the MSC.
Materials and Methods
Animals
Female BALB/c mice (6–8 weeks) were obtained from the Animal Facility, CSIR-Institute of Microbial Technology, Chandigarh, India. All the animal experiments were performed as approved by the ‘Institutional Animal Ethics Committee’ (IAEC) and accomplished according to the National Regulatory Guidelines issued by the ‘Committee for the Purpose of Control and Supervision of Experiments on Animals’ (No. 55/1999/CPCSEA), Ministry of Environment and Forest, Government of India.
Strains of Mycobacterium
The Mycobacterium tuberculosis (Mtb) strains (H37Rv, H37Ra) were obtained from Dr. V. M. Katoch (National JALMA Institute for Leprosy and Other Mycobacterial Diseases, Agra, India). Mycobacterium strains were grown and cultivated in Middlebrook 7H9 broth supplemented with glycerol (0.2%), tween-80 (0.05%), dextrose, albumin, and catalase. Bacterial viability was enumerated through colony-forming units (CFUs) by plating them on Middlebrook 7H11 medium, supplemented with dextrose, albumin, oleic acid, and catalase after 21d of plating.
Antibodies and Reagents
The standard reagents and chemicals were obtained from Sigma (St. Louis, MO). Recombinant cytokines and antibodies of IL-6, IL-12, TNF-α, and IL-10 were purchased from BD Biosciences (San Diego, CA). Fluorochrome-labelled antibodies (CD29 FITC, CD34 eFluor 660, CD44 PerCP-Cyanine5.5, CD45 APC, and Sca-1 PE) were purchased from eBiosciences (San Diego, CA) unless otherwise mentioned. Fetal bovine serum (FBS) was from GIBCO. LPS and N-glycolyl MDP used as ligands for TLR-4 and NOD-2 in the experiments were procured from InvivoGen (San Diego, CA). Oil Red-O stain was bought from Himedia (Mumbai, India). Alizarin Red S stain was acquired from Sigma (St. Louis, MO).
Isolation of Bone-Marrow-Derived Mesenchymal Stem Cells From Mice
Mouse MSCs were isolated according to a protocol reported previously (Huang et al., 2015). Briefly, the tibia and femur bones were taken from the hind limb of BALB/c and kept in sterile phosphate buffer saline (PBS) (1X), after the removal of all residual soft tissues. Then, with the help of a 23G needle and syringe, bone cavities were flushed with Dulbecco’s modified Eagle’s medium (DMEM) having 10% heat-inactivated FBS, 1X penicillin-streptomycin, and 2 mM L-glutamine. Bone cavities were flushed repeatedly to obtain enough marrow cells. The cells were then cultured in cell culture dishes (100mm) for 5 days in 5% CO2/37°C. Later, the cells were washed twice with PBS (1X) and digested with trypsin (0.25%) containing ethylenediaminetetraacetic acid (EDTA) (0.02%) at RT for 2 min followed by trypsin neutralization with DMEM + FBS (10%). The cells were then centrifuged at 2000 rpm for 3 min and replated at a split ratio of 1:3 in fresh complete media. The experiments were performed when the MSCs showed a homogeneous pattern after several set of passages. The MSCs were harvested, washed, and cultured for the experiments.
The activation of MSC was done by signaling through NOD-2 and TLR-4. The MSCs (2x105 cells/ml) were stimulated with a combination of N-glycolyl MDP (10 µg/ml) and ultra-purified LPS (5 ng/ml), the ligands of NOD-2 and TLR-4, respectively. The control cultures consist of unstimulated MSCs or cultured with either the ligand of NOD-2 or TLR-4. The cells were cultured in DMEM + FBS (10%) for 48h at 5% CO2/37°C. The culture supernatants (SNs) were harvested after 48h for the estimation of cytokines by ELISA and cells for the isolation of total RNA at 6h to perform RT-PCR.
Estimation of Cytokine Secretion by ELISA
The cultures were set as mentioned above, and the cytokines (IL-12, IL-6, TNF-α, and IL-10) were estimated in the culture SNs of the MSCs by ELISA methods, according to the manufacturer’s instructions. Briefly, ELISA plates were coated with antibodies to mouse IL-12 (2μg/ml), IL-6 (2μg/ml), TNF-alpha (2µg/ml) or IL-10 (4μg/ml) in phosphate buffer (0.01 M Na2HPO4, pH 9.2, and pH 6, respectively) at 4°C for overnight. Blocking was performed with 1% BSA at RT for 2h. Later, SNs (50μl/well) were added in the wells, or their respective recombinant cytokines as standards, and kept at 4°C overnight. Then, the respective biotinylated anti-mouse IL-12 (2μg/ml), IL-6 (2μg/ml), TNF-alpha (2µg/ml) or IL-10 (2μg/ml) antibodies were added into plates and incubated for 2h at RT. Afterward, avidin-HRP (1:10,000) was added and incubated at 37°C for 45 min. After each incubation, regular steps of washing were carried out. Subsequently, the color was developed using H2O2-OPD substrate-chromogen, and the reaction was stopped by the addition of 7% H2SO4 in the plates. The plates were then read at 492 nm in an ELISA reader. Serial dilutions of recombinant cytokines (rIL-6, rIL-12 and rIL-10) were used to plot standard curves for the estimation of cytokines in SNs. Results of ELISA were expressed in pg/ml.
RT-qPCR for the Quantification of IL-12, IL-6, TNF-α, IL-10, iNOS, and TGF-β
Isolation of total RNA was performed using TRIzol reagent from MSCs stimulated with N2.T4 (MSCN2.T4) for 6h, according to the manufacturer’s instruction (Invitrogen, Carlsbad, CA). Briefly, quantification of RNA was done using the NanoDrop spectrophotometer. The purity of all isolated RNA was in the range of 1.90 to 2.00 upon measured at A260/A280 (BioTek, Winooski, VT). The cDNA was synthesized using the Maxima first-strand cDNA synthesis kit for RT-qPCR (Thermo Fischer Scientific, K1642). Amplification-grade DNase1 (Sigma Aldrich, AMPD1-1KT) was used for removing DNA contamination from RNA samples. RNA samples (1μg) were treated with DNase1 (1U) in the reaction buffer for 15 min. DNase activity was stopped with the addition of a stop solution followed by incubating samples at 70°C for 10 min. Analysis was performed by the comparative Ct method, whereas normalization of the Ct values was done against a housekeeping control β-actin. Relative gene expression was determined using the comparative Ct method as 2(-ΔΔCt), where ΔCt = Ct (gene of interest) - Ct (normalizer = β-actin) and the ΔΔCt = ΔCt (sample) - ΔCt (calibrator). RT-qPCR, along with the analysis of data, was carried out using the ABI 7500 Fast Real-time PCR system (Applied Biosystems, Chromas, Singapore). Results were presented as a relative expression (fold change). The primer sequences for RT-qPCR are mentioned below.
TNF-α
Fwd 5’-CCTGTAGCCCACGTCGTAG -3’
Rev 5’-GGGAGTAGACAAGGTACAACCC -3’
TGF-β
Fwd 5’- TGACGTCACTGGAGTTGTACGG-3’
Rev 5’-GGTTCATGTCATGGATGGTGC-3’
β-actin
Fwd 5’-AGAGGGAAATCGTGCGTGAC-3’
Rev 5’-CAATAGTGATGACCTGGCCGT-3’
IL-6
Fwd 5’-GAGGATACCACTCCCAACAGACC-3’
Rev 5’-AAGTGCATCATCATCGTTGTTCATACA-3’
IL-12
Fwd 5’-GGAAGCACGGCAGCAGCAGAATA-3’
Rev 5’-AACTTGAGGGAGAAGTAGGAATGG-3’
iNOS
Fwd 5’-AACGGAGAACGTTGGATTTG-3’
Rev 5’-CAGCACAAGGGGTTTTCTT-3’
The Characterization of Phenotypic Markers of MSCs
For the immunophenotype assay, flow cytometry analysis was carried out. MSCs (2X105 cells) were washed and harvested from the plates after they attained the confluency. Cells were then incubated with Fc receptor blocking antibody (anti-CD16/32) for 20 min at 4°C. Subsequently, cells were stained with fluorochrome-conjugated Abs specific for CD34, CD44 (osteopontin and hyaluronate marker), Sca-1 (stem cell antigen-1), CD29 (Integrin β-1), and CD45 (pan-leukocyte marker) Abs at 4°C for 30 min. Washing was done at each step. Later, cells were fixed using paraformaldehyde (1X) and acquired on the FACS ARIA flowcytometer. The data were analyzed using BD DIVA software (BD Biosciences, San Jose, CA).
Evaluation of the Differentiation of MSCs
For adipogenesis differentiation assay, MSCs (2 X 105/well) were seeded in a 6-well plate in DMEM+10% FBS complete media. The next day, a fresh medium was poured along with a pre-warmed complete adipogenesis differentiation medium (StemPro® Adipogenesis Differentiation Kit; A10070-01) and kept at 37°C in a 5% CO2 incubator. Cultures were fed every 3–4 days with adipogenesis differentiation media. After 21 days, cells were washed twice with PBS (1X) followed by fixation with 10% formalin. Cells were then stained with Oil red O (Himedia: TC256) stain. The pictures were taken under a phase-contrast microscope (10X) (Olympus IX71, Tokyo, Japan).
For osteogenesis differentiation assay, MSCs (2 X 105/well) were seeded in a 6-well plate in DMEM+10% FBS complete media. After 24h, fresh media (DMEM+10% FBS) was added along with a pre-warmed osteogenesis differentiation medium (StemPro® Osteogenesis Differentiation Kit; A10072-01). Cultures were replenished every 3–4 days with osteogenesis differentiation media. After 3 weeks, fixation of cells was done using formalin (10%) and stained for calcium deposition with Alizarin Red S (Sigma Aldrich, St. Louis, MO).
In Vitro Infection of MSCs With Mtb and Determination of CFUs
Mtb (H37Rv) was grown till the mid-log phase and stored in a glycerol stock at -80°C. Later, the bacterium was thawed, and MSCs (2 X 105/well) were infected with Mtb at multiplicity of infection (MOI) 1:5 for 4h. The extracellular bacteria were eliminated by treatment with gentamicin (10 μg/ml) for 1h and then washed with PBS (1X). Mtb-infected MSCs were then stimulated with ligands of NOD-2 and TLR-4 (N2.T4) for 48h and plates were kept in a CO2 (5%) incubator at 37°C. Later, the SNs were collected for the cytokine ELISA and cells were lysed with saponin (0.1%). 100X serial dilutions of cell lysate were plated on 7H11 agar plates. Plates were kept in an incubator at 37°C. Bacterial colonies were enumerated for CFUs after 3 weeks.
Tracking of Mtb Into Autolysosomes by LysoTracker Red Staining
MSC (2 X 105 cells/well) were infected with GFP-Mtb (H37Ra) for 4h at MOI of 1:5. The extensive washing was done with PBS (1X) to get rid of extracellular Mtb followed by gentamicin (10 µg/ml) treatment for 1h. The cells were stimulated with N2.T4 for 12 h. The cells were stained with 200 nM of LysoTracker Red (prepared in media) for 20 min at 37°C/5% CO2. The cells were then washed twice with PBS (1X) followed by fixing with PFA (4%). After fixation, the nucleus was stained with DAPI (1 µg/ml) for 10 min, followed by washing three times with PBS (1X). The coverslips were mounted onto the slide with help of a mounting reagent and observed under a confocal microscope (Nikon A1R, Nikon, Yokohama, Japan), using the lasers 488 nm (GFP-MtbH37Rv), 561 nm (LysoTracker Red), and 405 nm (DAPI) with the same power set for controls. In total, 10 random fields were imaged, and the percentage of Mtb containing autophagosomes colocalized with lysosomes was counted.
Evaluation of Signaling in MSCN2.T4 by Western Blotting
MSC (2 X 106 cells/well) were stimulated with N2.T4 (MSCN2.T4) for 24h. Cells were then harvested, washed, and lysed in a lysis buffer (RIPA buffer, protease and phosphatase inhibitor cocktail). Proteins in the lysate were then estimated and equal concentrations of lysates were subjected to SDS-PAGE electrophoresis. After transfer to the nitrocellulose membrane, followed by blocking with BSA (2%), the membranes were then immunoblotted with Abs specific for LC3-I/LC3II, beclin-1, phospho-p38/p38, and NF-κB-p65. Actin was used as a loading control. The blots were developed using a chemiluminescence kit (Amersham Pharmacia Biotech, Buckinghamshire, UK). Blots were then scanned with ImageQuant LAS 4000 (GE Healthcare, Pittsburgh, PA). The image analysis was performed with ImageJ software.
Statistical Analysis
All data were analyzed using student “t-test” and one-way analysis of variance (ANOVA) with post-Tukey-Kramer multiple comparisons test by Graph Pad Prism 6 software (GraphPad Software, La Jolla, CA). Data were expressed as mean ± SD. The *p<0.05 was considered significant.
Results
Characterization of MSCs Isolated From the Bone Marrow
Bone-marrow-derived MSCs were isolated and cultured, as described elsewhere (Huang et al., 2015). The cellular morphology of MSCs was spindle shaped (fibroblast like), as observed under a microscope (Figures 1A, B). Further, isolated MSCs were stained with rhodamine phalloidin (selectively binds to F-actin) and DAPI to study their morphological features. MSCs have the potential to differentiate into various lineages (Robert et al., 2020). Subsequently, the MSC were checked for their adipogenic and osteogenic differentiation characteristics. We observed red-colored intracellular lipid vacuoles after Oil Red O staining (Figure 1C). Further, Alizarin Red S stained the calcium nodules deposition, which confirmed the osteoblasts formation in MSC (Figure 1D). It has been reported that the MSCs can be phenotypically characterized by the expression of several positive and negative markers on their surface (Soleimani and Nadri, 2009). Flow cytometric analysis confirmed the expression of Sca-1, CD44, and CD29 markers and the absence of CD45 molecules (Supplementary Figure 1). Thus, the cells isolated from bone marrow were phenotypically and functionally characterized as MSCs and all the subsequent experiments were performed using these cells.
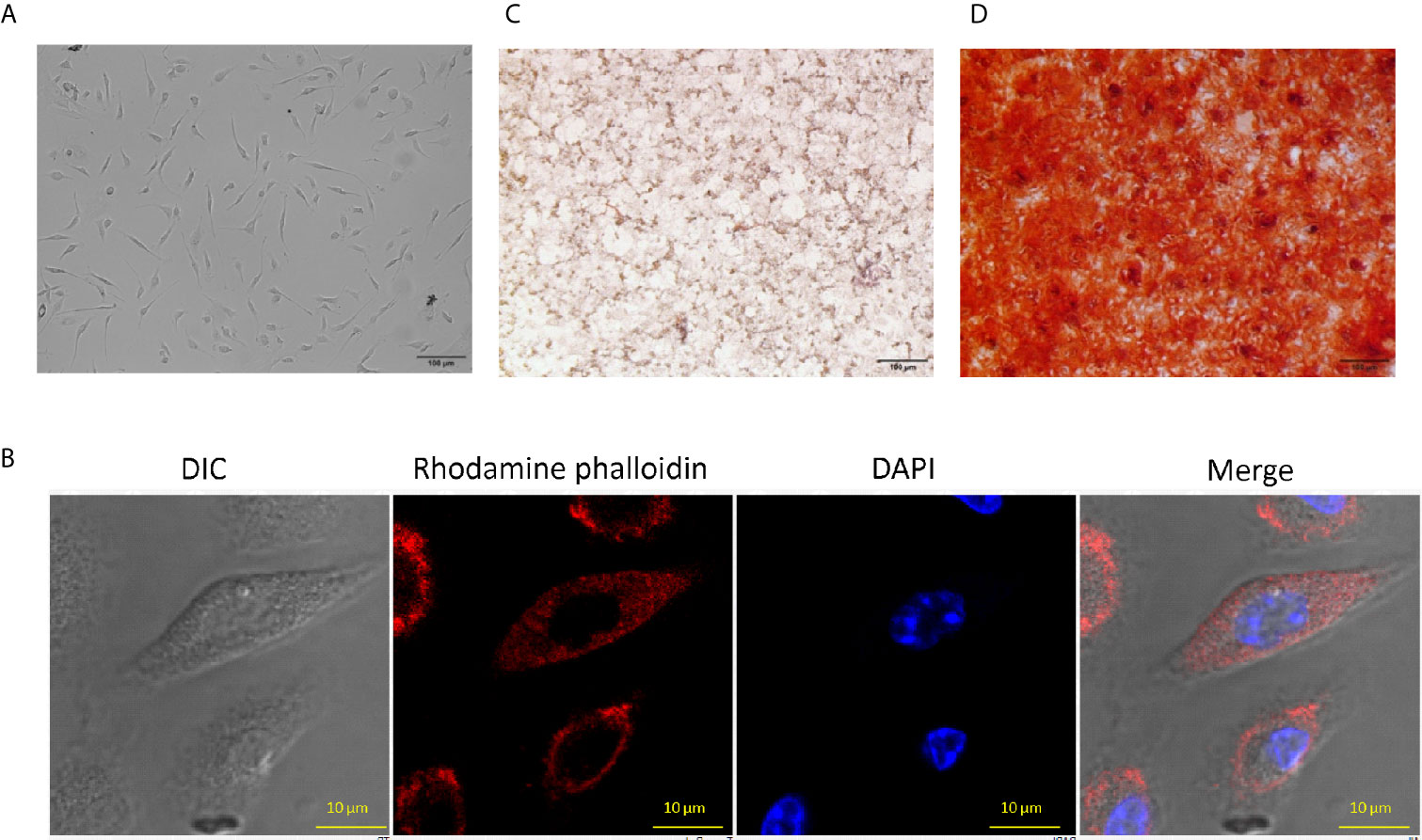
Figure 1 Characteristics of mouse bone marrow-derived mesenchymal stem cells (MSC). (A) MSCs were isolated from mouse bone marrow and expanded to several passages that showed fibroblast-like morphology and arranged in the swirl. The photographs were taken using an Olympus IX71phase contrast microscope (10X). (B) MSCs were stained with rhodamine phalloidin (red) and DAPI (blue) and observed under a confocal microscope (60X). (C) Intercellular lipid vacuoles were stained with Oil Red O to determine the adipogenic differentiation potential of MSC. The pictures were taken using an Olympus phase contrast microscope (10X). (D) The MSC were stained with the Alizarin Red S to assess the osteogenic differentiation by the formation of calcium nodules. The pictures were clicked using a phase contrast microscope (10X). The photographs shown are the representative of three experiments.
Signaling Delivered Through NOD-2 and TLR-4 Stimulates MSCs
MSC expresses innate receptors, such as TLRs, NOD-2, and RIG-1 (Kim et al., 2010; Lei et al., 2011; Yang et al., 2013). Hence, we thought whether ligation of NOD-2 and TLR-4 can stimulate MSCs. Unfortunately, we could not observe any statistical change in the activation of MSCs, as depicted by the release of IL-6 and IL-12 (Figure 2A). Intriguingly, when we activated MSCs by combinatorial signaling through NOD-2 and TLR-4 (MSCN2.T4), a substantial (p<0.001) increase in the release of IL-6 and IL-12 was noted, as compared to the control MSCs stimulated with the ligand of either NOD-2 or TLR-4 (Figures 2A, B). Therefore, the combination of ligands of NOD-2 and TLR-4 (N2.T4) were used in all the subsequent experiments to stimulate MSCs (MSCN2.T4). It has been reported that TNF-α, IL-12, IL-6, and iNOS play a crucial role in curbing the intracellular growth of pathogens like Mtb, leishmania, salmonella, HIV, etc. (Black et al., 1990; Jankovic et al., 2002; Chakravortty and Hensel, 2003). Further, this observation was corroborated through gene expression in MSCs by RT-qPCR. Besides IL-12 (p<0.001) and IL-6 (p<0.001), iNOS (p<0.001) along with TNF-α (p<0.001) also showed elevation in their expressions upon stimulation with N2.T4 (Figures 2C–F). Contrary to this, there was a substantial (p<0.001) reduction in the anti-inflammatory cytokine TGF-β (Figure 2G). Hence, we observed a shift from anti-inflammatory to pro-inflammatory phenotype after triggering MSCs through N2.T4.
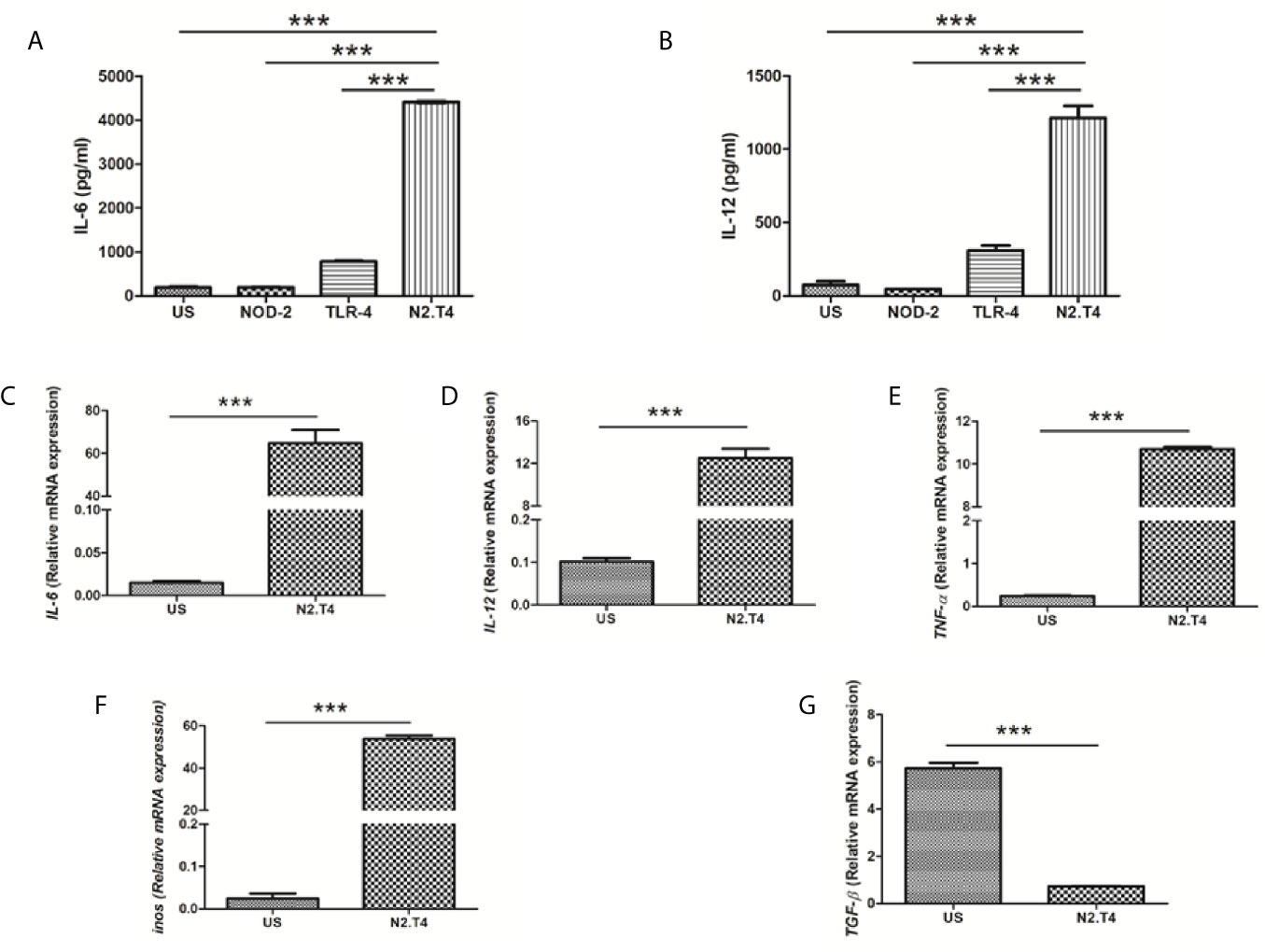
Figure 2 Cumulative signaling through NOD-2 and TLR-4 stimulates MSC to release IL-6 and IL-12. (A, B) MSCs were cultured with the ligands of NOD-2 and TLR-2 (N2.T4). The controls were set using unstimulated MSCs (US) or stimulated with either the ligand of NOD-2 (NOD-2L) or TLR-4 (TLR-4L). The culture SNs were collected after 48h and estimated for the production of (A) IL-6 and (B) IL-12 cytokines by ELISA. (C–G) The MSCs were stimulated as indicated above (A, B) and the mRNA expression of Il6 (C), Il12 (D), Tnfa (E), Inos (F) and Tgfb (G) was performed by RT-PCR. Graphs depict the ‘mRNA expression relative to unstimulated (US) control’. Data expressed as mean ± SD are representative of two independent experiments. Statistical analysis was done using one way ANOVA for ELISA and unpaired t-test for RT-PCR. ***p < 0.001.
Signaling Mtb-Infected MSCs Through N2.T4 Augments the Release of Pro-Inflammatory Cytokines and Constrains the Intracellular Growth of the Mtb
Recent studies have shown that Mtb can successfully infect and hide inside the MSCs (Das et al., 2013). Hence, we were curious to monitor the influence of N2.T4 signaling of MSCs (MSCN2.T4) on the intracellular survival of the bacterium. Interestingly, MSCs infected with Mtb (H37Rv) upon N2.T4 stimulation significantly (p<0.01) restricted the bacterial burden compared to unstimulated (US) MSCs (Figure 3A). Furthermore, remarkable elevation was noticed in the secretion of pro-inflammatory cytokines TNF-α (p<0.01) and IL-6 (p<0.001) by MSCN2.T4 (Figures 3B, C). The non-significant decrease was observed in the level of anti-inflammatory cytokine IL-10 (Figure 3D). These results suggest that combinatorial stimulation of MSCs through NOD-2 and TLR-4 can successfully restrict the intracellular growth of Mtb masked inside these cells.
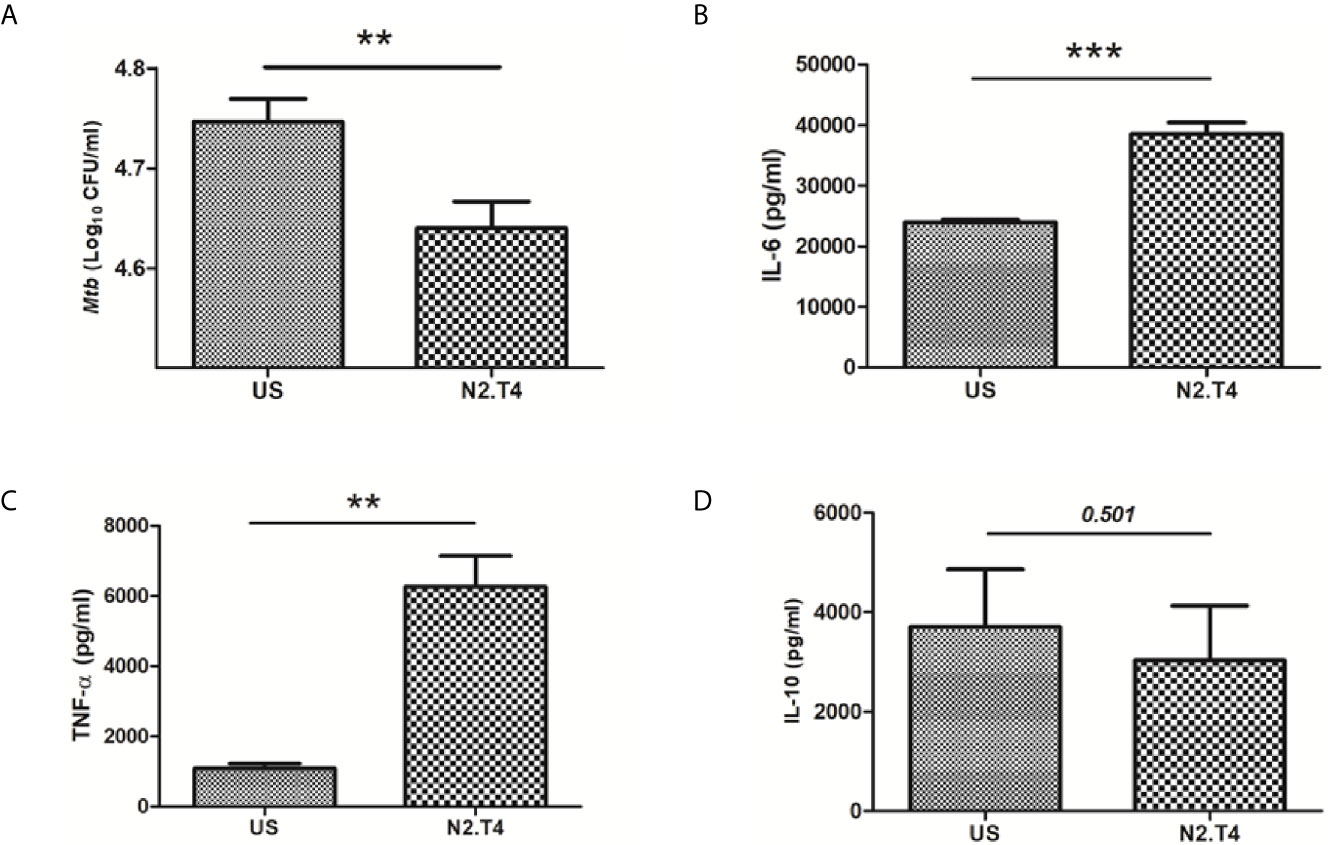
Figure 3 Signaling MSCs through N2.T4 restricted the intracellular growth of Mtb and elicited the secretion of pro-inflammatory cytokines. (A) MSCs were infected with Mtb (GFP-H37Ra) for 4h and then stimulated with N2.T4 for 48h. Later, cells were lysed and CFUs were enumerated on 21d by CFU assay. The Bar diagram represents the mean ± SEM and is indicative of three independent experiments. Statistical analysis was done using one-way ANOVA. The culture SNs were collected after 48h for determining the yield of cytokines (B) IL-6, (C) TNF-α and (D) IL-10 by ELISA. Statistical analysis was done using an unpaired t-test. The inset represents p value. **p < 0.01 and ***p < 0.001.
Signaling of MSCN2.T4 Augments the Co-Localization of Mtb in Lysosomes
One of the potent mechanisms responsible for the killing of various intracellular pathogens is the lysosomal degradation pathway (Jo, 2010). Scavenger receptors like MARCO and SR-B1 present on MSCs play an important role in internalizing Mtb (Khan et al., 2017). Phagosome lysosome fusion is decisive for the eradication of the intracellularly hidden Mtb (Khan et al., 2017). However, Mtb has a unique tendency to evade the immune system by inhibiting the phagosome lysosome fusion; thereby can successfully survive in the hostile environment of macrophages and MSC (Jamwal et al., 2016). Consequently, we next studied the signaling of MSCs through N2.T4 and its influence on the intracellular trafficking of Mtb. MSCs were infected with Mtb overexpressing GFP. Further, MSCs were stained with LysoTracker Red dye to monitor the acidification of the lysosome. Later, the signaling was delivered in MSCs through N2.T4. We observed that Mtb inhibited the phagosome lysosome fusion of the infected MSC (Figure 4A). It was interesting to note that MSCN2.T4 could efficiently overcome the Mtb induced inhibition of phagosome lysosome fusion, as demonstrated by a significant (p<0.01) increase in the co-localization of Mtb and LysoTracker Red dye (Figures 4A, B). These results signify that the mechanism responsible for the killing of Mtb by MSCN2.T4 may be operating through the enhanced fusion of phagolysosomes (Figures 3A and 4A, B). Hence, N2.T4 may have an important immunotherapeutic role in eliminating Mtb concealed in the MSCs.
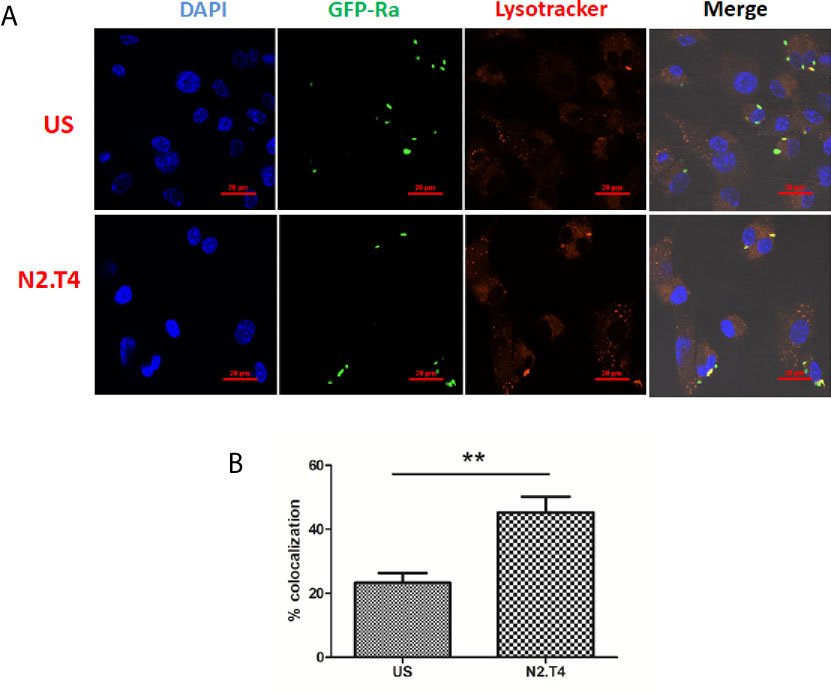
Figure 4 Triggering MSCs through N2.T4 induced trafficking of Mtb to autolysosomes. (A) MSCs (2 X 105 cells/well)were infected with GFP-Mtb (H37Ra) for 4h followed by signaling through N2.T4 for 12h. Later, the lysosomes were stained with LysoTracker Red for 20 min. The cells were fixed with PFA (4%) and slides were prepared for confocal microscopy. The panel shows the representative image of Mtb (green channel, 488 nm) colocalization with LysoTracker Red (red channel, 561 nm) (60X). (B) The data from (A) are also illustrated as a graph depicting the percentage of colocalization of Mtb with the lysosome. The data shown as mean ± SEM are representative of three independent experiments. Statistical significance was determined using the unpaired t-test. **p < 0.01.
Signaling Through N2.T4 Induces Autophagy in MSC
The intrinsic autophagy mechanism is known to inhibit the growth of Mtb inside MSCs (Khan et al., 2017). The transition of LC3-I to LC3-II is evidence of autophagy. Consequently, we next checked the induction of autophagy in MSCsN2.T4. Interestingly, we observed conversion of LC3-I to LC3-II (Figures 5A, B). These results suggest a novel role of signaling of MSC through N2.T4 in inducing autophagy. Beclin-1 is a well-known initiator and master regulator of autophagy. Thus, the levels of beclin-1 in MSCN2.T4 were monitored. We observed an increased level of beclin-1 in MSCN2.T4 (Figures 5C, D). The modulation of the expression of markers LC3 and beclin-1, suggests the role of autophagy as a possible mechanism operating in curtailing the intracellular growth of Mtb in MSCN2.T4.
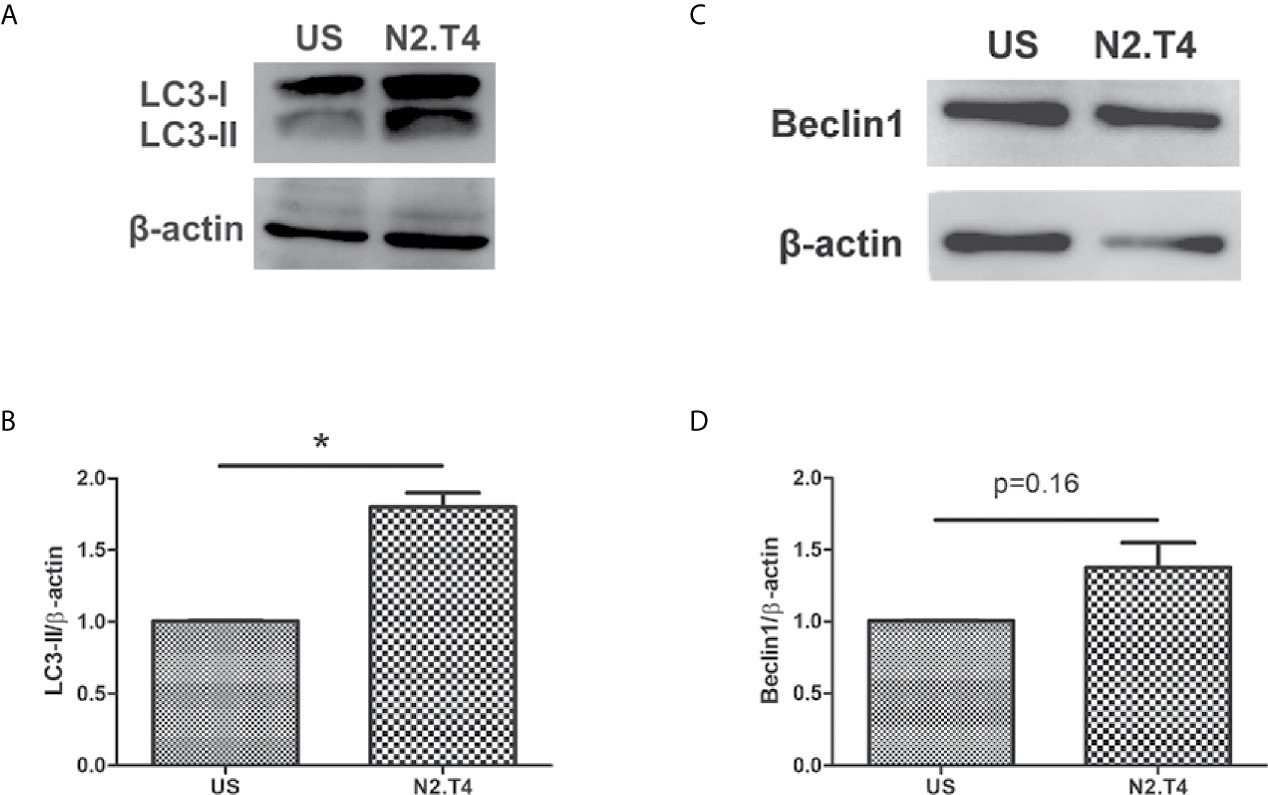
Figure 5 N2.T4 signaling induced autophagy in MSC. MSC (2 X 106 cells/well) were stimulated with either N2.T4 or left untreated for 24h. The cells were lysed with a RIPA buffer containing a protease inhibitor cocktail. The samples were subjected to SDS-PAGE and Western blotting. The blots were probed with antibodies against autophagy markers LC3 and beclin-1 (A, C). The densitometric analysis of LC3 and beclin-1, normalized with β-actin is represented in graphs (B, D), respectively. The data shown are representative of three independent experiments. The inset represents p value. *p < 0.05.
N2.T4 Signaling of MSC Induces NF-κB Activity via p38 MAPK Pathway
The TLR signaling is known to initiate MAPK pathways through MyD88 molecules (Kim et al., 2010). Further, NF-κB is activated after degradation of IκB and translocates into the nucleus to affect the target genes, denoting the activation status of the cell. We noticed a substantial induction of p38 in MSCN2.T4, as compared to unstimulated cells (Figures 6A, B). Furthermore, we noticed increased levels of NF-κB-p65 (Figures 6C, D). These results suggest the importance of combinatorial signaling of NOD-2 and TLR-4 in enhancing the activation and functionality of the MSCN2.T4 to kill covert Mtb.
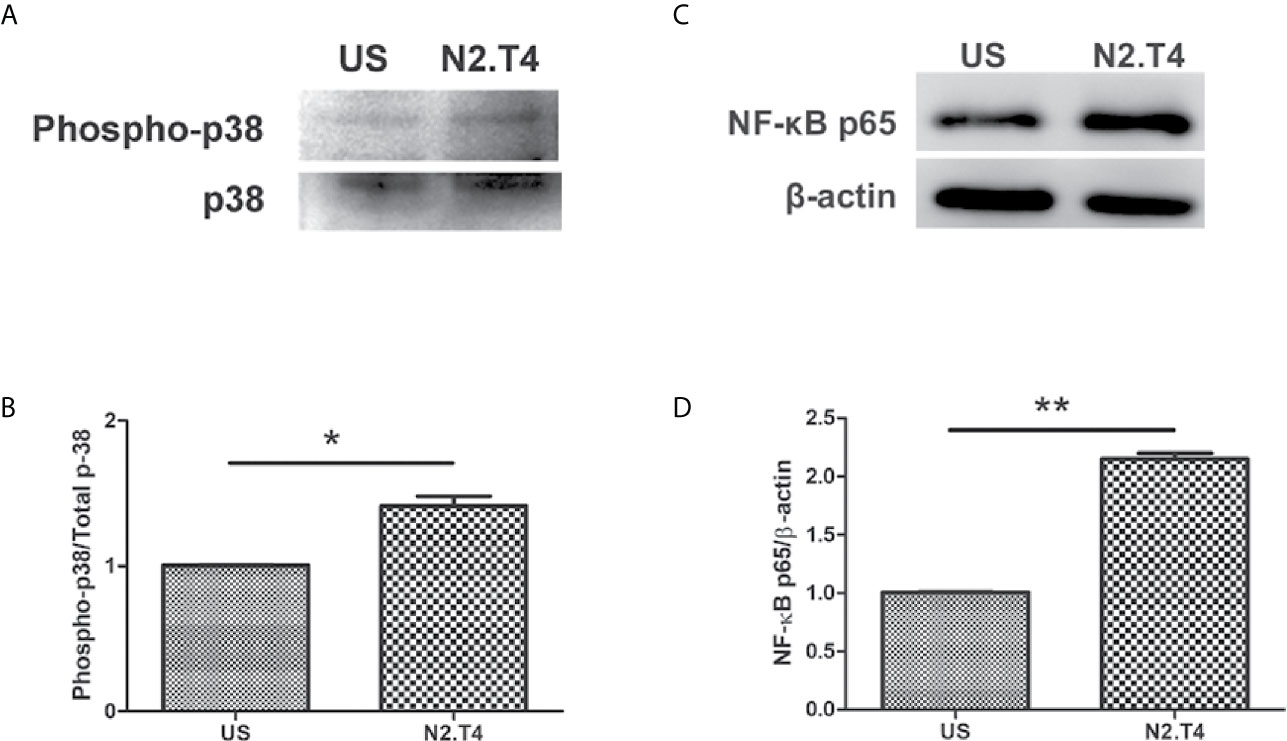
Figure 6 Stimulation of MSCs through N2.T4 induced NF-κB activity via the p38 MAPK pathway. MSCs were stimulated with the ligands of N2.T4 for 24 hours and control cells were left unstimulated (US). The cells were lysed with a RIPA buffer containing protease inhibitor cocktail. Total cell lysates were subjected to SDS-PAGE and Western blotting. The blots were probed with antibodies against (A) phosphor-p38/p38 and (C) NF-κB-p65 with densitometric analysis normalized with β-actin, represented in graphs (B, D), respectively. The data shown are representative of two independent experiments. *p < 0.05, **p < 0.01.
Discussion
Mycobacterium tuberculosis (Mtb) is one of the most astute pathogens that the human race has ever encountered. This is evident by the fact that it understands the mechanism of i) living in a dormant state in a hostile environment of the host; ii) impairing the functioning of BCG vaccine; iii) developing resistance against the drugs designed to kill it; iv) developing coalition with HIV; and v) attacking malnourished individuals. Further, the problem has been compounded with a recent discovery of enduring residency of Mtb in the multipotent cells i.e. MSC (Das et al., 2013; Beamer et al., 2014). Consequently, Mtb continues to make its elimination a daunting task for the scientific community.
Only 5–15% of people infected with Mtb develop TB. This indicates 85–95% of infected individuals develop a remarkably strong immunity to remain protected throughout their lives. This indicates that host immunity plays a primary role in protecting against TB. Therefore, boosting host immunity can play a cardinal role in protecting against Mtb. Recently, host-directed therapy (HDT) has gained considerable momentum following the observation that it not only controls the infection and devastating inflammatory responses inflicted by Mtb to the host but also the emergence of drug-resistant strains of the bacterium (Zumla et al., 2016; Young et al., 2020). Exploration and exploitation of the molecules of innate immunity may be an estimable idea for bolstering host immunity since innate immunity plays an imperative role against Mtb (Fremond et al., 2004). The NOD-2 is an important receptor of innate immunity because its role has been reported in effectively modulating the cell’s immunity (Divangahi et al., 2008; Jo, 2008). Likewise, TLR-4 plays a crucial function in boosting immunity against many pathogens (Kleinnijenhuis et al., 2011; Mortaz et al., 2015). Recently, we have demonstrated a combinatorial role of NOD-2 and TLR-4 in substantially augmenting the functionality of dendritic cells in priming T cells and killing Mtb (Khan et al., 2016a). Mesenchymal stem cells express various innate receptors on their surface, including NOD-2 and TLR-4. These receptors assist in the recognition and delivering signals on encounters with the pathogens (Kim et al., 2010; Lei et al., 2011; Yang et al., 2013). Signaling through innate receptors can polarize MSCs from anti-inflammatory to pro-inflammatory phenotype (Waterman et al., 2010).
Based on the above-mentioned studies, we thought to examine the influence of signaling through NOD-2 and TLR-4 in modulating the activity of MSCs against Mtb. MSCs were infected with Mtb, and signaling was delivered using the ligands of NOD-2 and TLR-4. Following major findings emerged out of this study. MSCN2.T4 exhibited i) activation phenotype, as illustrated by the enhanced release of IL-6, IL-12, TNF-alpha, iNOS and decrease in TGF-β; ii) increased co-localization of Mtb in lysosomes; iii) induction of NF-κB activity via the p38 MAPK pathway; iv) augmented autophagy; and v) a decline in the survival of Mtb inside the MSCs.
NODs and TLRs are innately expressed on MSCs (Delarosa et al., 2012). NOD-2 and TLR-4 coordinate with each other in imparting protection against pathogens like Mtb (Khan et al., 2016c). Likewise, our study demonstrated that combinatorial signaling of TLR-4 and NOD-2 can activate MSCs and thereby can restrict the intracellular growth of Mtb. N-glycolyl MDP was selected as a NOD-2 agonist because it is 10–100 fold more effective, as compared to N-acetylated MDP (Coulombe et al., 2009). Ultrapure LPS was used for triggering TLR-4, which has remarkable adjuvant properties. Further, the Food and Drug Administration (FDA) has approved the immunotherapeutic use of the ligand of TLR-4 (Bohannon et al., 2013; Needham et al., 2013).
It was intriguing to note that the signaling of MSCs through NOD-2 and TLR-4 (MSCN2.T4) exhibited anti-Mtb immunity, as evidenced by a significant increase in the pro-inflammatory molecules IL-12, IL-6, TNF-α, and iNOS and reduction in anti-inflammatory cytokine TGF-β. This was further reflected by a substantial decline in the survival of Mtb in the MSCs. Mesenchymal stem cells express TLR-4 as a type I transmembrane glycoprotein (Takeda and Akira, 2005). The activation of TLR-4 requires adaptors and co-receptors (MD2, LMP, and CD14) for dimerization that facilitates MyD88/TRIF-dependent activation of the transcription factors (Zhu et al., 2006; Najar et al., 2017). It has been shown that the effect of LPS is compromised in MSCs derived from MyD88−/− mice (Chu et al., 2019). Moreover, LPS has been shown to convert MSCs from anti-inflammatory to pro-inflammatory phenotype (Waterman et al., 2010). Further, TLR-4 activation enhanced the proliferation of MSCs (Pevsner-Fischer et al., 2007). Thus, indicating the potential role of TLR-4 in the signaling of MSCs.
In general, TLR stimulation activates MyD88-dependent and independent signaling pathways. MyD88 recruitment to TLRs triggers numerous signaling pathways via IRAKs, which subsequently initiate MAPK pathways. Therefore, to decipher the mechanism operating in restricting the survival of Mtb in MSC, we checked the level of the p38 MAPK signaling pathway. We observed elevated expression of p38 molecule in MSCN2.T4. It is already reported that the p38 phosphorylation activates NF-κB followed by subsequent translocation to the nucleus (Olson et al., 2007; Karunakaran and Ravindranath, 2009). Increased levels of NF-κB p65 in MSCN2.T4 were observed, as compared to unstimulated cells. Furthermore, it was observed that the decline in the survival of Mtb was through autophagy, as revealed by the modulation in the expression LC3 and beclin-1. Autophagy is an inherent quality of many stem cell types and is considered to be vital for their pluripotency, differentiation, and self-renewal (Phadwal et al., 2013). Autophagy has been described to exhibit a dual role in Mtb protection. Firstly, it targets the antigen for lysosomal degradation, and, secondly, it prevents the inflammatory reaction; thus protecting from tissue necrosis along with the associated pathology (Castillo et al., 2012).
Conclusion
Overall, our results suggest that host-directed therapy through N2.T4 may be a good option for priming MSC to kill non-replicating quiescent intracellular Mtb. Further, the results suggest that this strategy may have enough potential in inhibiting the intracellular growth of drug-resistant Mtb. Finally, in future, this novel immunotherapeutic strategy may sufficiently contribute in successfully treating TB patients.
Data Availability Statement
The original contributions presented in the study are included in the article/Supplementary Material. Further inquiries can be directed to the corresponding author.
Ethics Statement
The animal study was reviewed and approved by Institutional Animal Ethics Committees (IAEC) of IMTECH, Chandigarh.
Author Contributions
The concept, experiment designing, and data analysis were done by JA and MAq. The experiments were conducted by MAq, SS, SP, MA, and SM. The manuscript was written by JA, MAq, and SS. All authors contributed to the article and approved the submitted version.
Funding
The work was carried out under funding support from the Council of Scientific and Industrial Research (CSIR), India. MAq received a fellowship from the Department of Science and Technology (DST), SS from the Indian Council of Medical Research (ICMR), MA from University Grant Commission, and SM and SP were the recipients of fellowships from CSIR.
Conflict of Interest
The authors declare that the research was conducted in the absence of any commercial or financial relationships that could be construed as a potential conflict of interest.
Supplementary Material
The Supplementary Material for this article can be found online at: https://www.frontiersin.org/articles/10.3389/fcimb.2021.669168/full#supplementary-material
Supplementary Figure 1 | The MSC were stained with the fluorochrome-labeled respective Abs and analyzed by flowcytometry for the expression of phenotypic markers viz CD44, CD29, Sca-1, and CD45. The black and red histograms represent unstained and stained MSCs, respectively.
References
Beamer, G., Major, S., Das, B., Campos-Neto, A. (2014). Bone Marrow Mesenchymal Stem Cells Provide an Antibiotic-Protective Niche for Persistent Viable Mycobacterium Tuberculosis That Survive Antibiotic Treatment. Am. J. Pathol. 184 (12), 3170–3175. doi: 10.1016/j.ajpath.2014.08.024
Black, C. M., Bermudez, L. E., Young, L. S., Remington, J. S. (1990). Co-Infection of Macrophages Modulates Interferon Gamma and Tumor Necrosis Factor-Induced Activation Against Intracellular Pathogens. J. Exp. Med. 172 (3), 977–980. doi: 10.1084/jem.172.3.977
Bohannon, J. K., Hernandez, A., Enkhbaatar, P., Adams, W. L., Sherwood, E. R. (2013). The Immunobiology of Toll-Like Receptor 4 Agonists: From Endotoxin Tolerance to Immunoadjuvants. Shock 40 (6), 451–462. doi: 10.1097/SHK.0000000000000042
Castillo, E. F., Dekonenko, A., Arko-Mensah, J., Mandell, M. A., Dupont, N., Jiang, S., et al. (2012). Autophagy Protects Against Active Tuberculosis by Suppressing Bacterial Burden and Inflammation. Proc. Natl. Acad. Sci. U. S. A. 109 (46), E3168–E3176. doi: 10.1073/pnas.1210500109
Chakravortty, D., Hensel, M. (2003). Inducible Nitric Oxide Synthase and Control of Intracellular Bacterial Pathogens. Microbes Infect. 5 (7), 621–627. doi: 10.1016/s1286-4579(03)00096-0
Chu, X., Xu, B., Gao, H., Li, B. Y., Liu, Y., Reiter, J. L., et al. (2019). Lipopolysaccharides Improve Mesenchymal Stem Cell-Mediated Cardioprotection by MyD88 and Stat3 Signaling in a Mouse Model of Cardiac Ischemia/Reperfusion Injury. Stem Cells Dev. 28 (9), 620–631. doi: 10.1089/scd.2018.0213
Coulombe, F., Divangahi, M., Veyrier, F., de Leseleuc, L., Gleason, J. L., Yang, Y., et al. (2009). Increased NOD2-Mediated Recognition of N-Glycolyl Muramyl Dipeptide. J. Exp. Med. 206 (8), 1709–1716. doi: 10.1084/jem.20081779
Das, B., Kashino, S. S., Pulu, I., Kalita, D., Swami, V., Yeger, H., et al. (2013). CD271(+) Bone Marrow Mesenchymal Stem Cells may Provide a Niche for Dormant Mycobacterium Tuberculosis. Sci. Trans. Med. 5 (170), 170ra113. doi: 10.1126/scitranslmed.3004912
Delarosa, O., Dalemans, W., Lombardo, E. (2012). Toll-Like Receptors as Modulators of Mesenchymal Stem Cells. Front. Immunol. 3, 182. doi: 10.3389/fimmu.2012.00182
Divangahi, M., Mostowy, S., Coulombe, F., Kozak, R., Guillot, L., Veyrier, F., et al. (2008). NOD2-Deficient Mice Have Impaired Resistance to Mycobacterium Tuberculosis Infection Through Defective Innate and Adaptive Immunity. J. Immunol. 181 (10), 7157–7165. doi: 10.4049/jimmunol.181.10.7157
Forget, E. J., Menzies, D. (2006). Adverse Reactions to First-Line Antituberculosis Drugs. Expert Opin. Drug Saf. 5 (2), 231–249. doi: 10.1517/14740338.5.2.231
Fremond, C. M., Yeremeev, V., Nicolle, D. M., Jacobs, M., Quesniaux, V. F., Ryffel, B. (2004). Fatal Mycobacterium Tuberculosis Infection Despite Adaptive Immune Response in the Absence of Myd88. J. Clin. Invest. 114 (12), 1790–1799. doi: 10.1172/JCI21027
Garhyan, J., Bhuyan, S., Pulu, I., Kalita, D., Das, B., Bhatnagar, R. (2015). Preclinical and Clinical Evidence of Mycobacterium Tuberculosis Persistence in the Hypoxic Niche of Bone Marrow Mesenchymal Stem Cells After Therapy. Am. J. Pathol. 185 (7), 1924–1934. doi: 10.1016/j.ajpath.2015.03.028
Gomez, J. E., McKinney, J. D. (2004). M. Tuberculosis Persistence, Latency, and Drug Tolerance. Tuberculosis 84 (1–2), 29–44. doi: 10.1016/j.tube.2003.08.003
Herbert, N., George, A., Baroness Masham of, I., Sharma, V., Oliver, M., Oxley, A., et al. (2014). World TB Day 2014: Finding the Missing 3 Million. Lancet 383 (9922), 1016–1018. doi: 10.1016/S0140-6736(14)60422-0
Hoebe, K., Janssen, E., Beutler, B. (2004). The Interface Between Innate and Adaptive Immunity. Nat. Immunol. 5 (10), 971–974. doi: 10.1038/ni1004-971
Huang, S., Xu, L., Sun, Y., Wu, T., Wang, K., Li, G. (2015). An improved protocol for isolation and culture of mesenchymal stem cells from mouse bone marrow. J. Orthop. Translat. 3 (1), 26–33. doi: 10.1016/j.jot.2014.07.005
Hwa Cho, H., Bae, Y. C., Jung, J. S. (2006). Role of Toll-Like Receptors on Human Adipose-Derived Stromal Cells. Stem Cells 24 (12), 2744–2752. doi: 10.1634/stemcells.2006-0189
Jamwal, S. V., Mehrotra, P., Singh, A., Siddiqui, Z., Basu, A., Rao, K. V. (2016). Mycobacterial Escape From Macrophage Phagosomes to the Cytoplasm Represents an Alternate Adaptation Mechanism. Sci. Rep. 6, 23089. doi: 10.1038/srep23089
Jankovic, D., Kullberg, M. C., Hieny, S., Caspar, P., Collazo, C. M., Sher, A. (2002). In the Absence of IL-12, CD4(+) T Cell Responses to Intracellular Pathogens Fail to Default to a Th2 Pattern and are Host Protective in an IL-10(-/-) Setting. Immunity 16 (3), 429–439. doi: 10.1016/s1074-7613(02)00278-9
Jo, E. K. (2008). Mycobacterial Interaction With Innate Receptors: TLRs, C-Type Lectins, and NLRs. Curr. Opin. Infect. Dis. 21 (3), 279–286. doi: 10.1097/QCO.0b013e3282f88b5d
Jo, E. K. (2010). Innate Immunity to Mycobacteria: Vitamin D and Autophagy. Cell Microbiol. 12 (8), 1026–1035. doi: 10.1111/j.1462-5822.2010.01491.x
Karunakaran, S., Ravindranath, V. (2009). Activation of P38 MAPK in the Substantia Nigra Leads to Nuclear Translocation of NF-kappaB in MPTP-Treated Mice: Implication in Parkinson’s Disease. J. Neurochem 109 (6), 1791–1799. doi: 10.1111/j.1471-4159.2009.06112.x
Kawai, T., Akira, S. (2011). Toll-Like Receptors and Their Crosstalk With Other Innate Receptors in Infection and Immunity. Immunity 34 (5), 637–650. doi: 10.1016/j.immuni.2011.05.006
Khan, N., Aqdas, M., Vidyarthi, A., Negi, S., Pahari, S., Agnihotri, T., et al. (2016a). Triggering Through NOD-2 Differentiates Bone Marrow Precursors to Dendritic Cells With Potent Bactericidal Activity. Sci. Rep. 6, 27263. doi: 10.1038/srep27263
Khan, A., Mann, L., Papanna, R., Lyu, M. A., Singh, C. R., Olson, S., et al. (2017). Mesenchymal Stem Cells Internalize Mycobacterium Tuberculosis Through Scavenger Receptors and Restrict Bacterial Growth Through Autophagy. Sci. Rep. 7 (1), 15010. doi: 10.1038/s41598-017-15290-z
Khan, N., Pahari, S., Vidyarthi, A., Aqdas, M., Agrewala, J. N. (2016b). NOD-2 and TLR-4 Signaling Reinforces the Efficacy of Dendritic Cells and Reduces the Dose of TB Drugs Against Mycobacterium Tuberculosis. J. Innate Immun. 8 (3), 228–242. doi: 10.1159/000439591
Khan, N., Vidyarthi, A., Pahari, S., Negi, S., Aqdas, M., Nadeem, S., et al. (2016c). Signaling Through NOD-2 and TLR-4 Bolsters the T Cell Priming Capability of Dendritic Cells by Inducing Autophagy. Sci. Rep. 6, 19084. doi: 10.1038/srep19084
Kim, H. S., Shin, T. H., Yang, S. R., Seo, M. S., Kim, D. J., Kang, S. K., et al. (2010). Implication of NOD1 and NOD2 for the Differentiation of Multipotent Mesenchymal Stem Cells Derived From Human Umbilical Cord Blood. PloS One 5 (10), e15369. doi: 10.1371/journal.pone.0015369
Kleinnijenhuis, J., Oosting, M., Joosten, L. A., Netea, M. G., Van Crevel, R. (2011). Innate Immune Recognition of Mycobacterium Tuberculosis. Clin. Dev. Immunol. 2011, 405310. doi: 10.1155/2011/405310
Kumar, A., Farhana, A., Guidry, L., Saini, V., Hondalus, M., Steyn, A. J. (2011). Redox Homeostasis in Mycobacteria: The Key to Tuberculosis Control? Expert Rev. Mol. Med. 13, e39. doi: 10.1017/S1462399411002079
Lei, J., Wang, Z., Hui, D., Yu, W., Zhou, D., Xia, W., et al. (2011). Ligation of TLR2 and TLR4 on Murine Bone Marrow-Derived Mesenchymal Stem Cells Triggers Differential Effects on Their Immunosuppressive Activity. Cell. Immunol. 271 (1), 147–156. doi: 10.1016/j.cellimm.2011.06.014
Mortaz, E., Adcock, I. M., Tabarsi, P., Masjedi, M. R., Mansouri, D., Velayati, A. A., et al. (2015). Interaction of Pattern Recognition Receptors With Mycobacterium Tuberculosis. J. Clin. Immunol. 35 (1), 1–10. doi: 10.1007/s10875-014-0103-7
Munro, S. A., Lewin, S. A., Smith, H. J., Engel, M. E., Fretheim, A., Volmink, J. (2007). Patient Adherence to Tuberculosis Treatment: A Systematic Review of Qualitative Research. PloS Med. 4 (7), e238. doi: 10.1371/journal.pmed.0040238
Mwaba, P., Chakaya, J. M., Petersen, E., Wejse, C., Zumla, A., Kapata, N. (2020). Advancing New Diagnostic Tests for Latent Tuberculosis Infection Due to Multidrug-Resistant Strains of Mycobacterium Tuberculosis - End of the Road? Int. J. Infect. Dis. 92S, S69–S71. doi: 10.1016/j.ijid.2020.02.011
Najar, M., Krayem, M., Meuleman, N., Bron, D., Lagneaux, L. (2017). Mesenchymal Stromal Cells and Toll-Like Receptor Priming: A Critical Review. Immune Netw. 17 (2), 89–102. doi: 10.4110/in.2017.17.2.89
Needham, B. D., Carroll, S. M., Giles, D. K., Georgiou, G., Whiteley, M., Trent, M. S. (2013). Modulating the Innate Immune Response by Combinatorial Engineering of Endotoxin. Proc. Natl. Acad. Sci. U. S. A. 110 (4), 1464–1469. doi: 10.1073/pnas.1218080110
Olson, C. M., Hedrick, M. N., Izadi, H., Bates, T. C., Olivera, E. R., Anguita, J. (2007). P38 Mitogen-Activated Protein Kinase Controls NF-kappaB Transcriptional Activation and Tumor Necrosis Factor Alpha Production Through RelA Phosphorylation Mediated by Mitogen- and Stress-Activated Protein Kinase 1 in Response to Borrelia Burgdorferi Antigens. Infect Immun. 75 (1), 270–277. doi: 10.1128/IAI.01412-06
Pahari, S., Khan, N., Aqdas, M., Negi, S., Kaur, J., Agrewala, J. N. (2016). Infergen Stimulated Macrophages Restrict Mycobacterium Tuberculosis Growth by Autophagy and Release of Nitric Oxide. Sci. Rep. 6, 39492. doi: 10.1038/srep39492
Pahari, S., Negi, S., Aqdas, M., Arnett, E., Schlesinger, L. S., Agrewala, J. N. (2020). Induction of Autophagy Through CLEC4E in Combination With TLR4: An Innovative Strategy to Restrict the Survival of Mycobacterium Tuberculosis. Autophagy 16 (6), 1021–1043. doi: 10.1080/15548627.2019.1658436
Peters, W., Ernst, J. D. (2003). Mechanisms of Cell Recruitment in the Immune Response to Mycobacterium Tuberculosis. Microbes Infect. 5 (2), 151–158. doi: 10.1016/s1286-4579(02)00082-5
Pevsner-Fischer, M., Morad, V., Cohen-Sfady, M., Rousso-Noori, L., Zanin-Zhorov, A., Cohen, S., et al. (2007). Toll-Like Receptors and Their Ligands Control Mesenchymal Stem Cell Functions. Blood 109 (4), 1422–1432. doi: 10.1182/blood-2006-06-028704
Phadwal, K., Watson, A. S., Simon, A. K. (2013). Tightrope Act: Autophagy in Stem Cell Renewal, Differentiation, Proliferation, and Aging. Cell. Mol. Life Sci: CMLS 70 (1), 89–103. doi: 10.1007/s00018-012-1032-3
Robert, A. W., Marcon, B. H., Dallagiovanna, B., Shigunov, P. (2020). Adipogenesis, Osteogenesis, and Chondrogenesis of Human Mesenchymal Stem/Stromal Cells: A Comparative Transcriptome Approach. Front. Cell Dev. Biol. 8, 561. doi: 10.3389/fcell.2020.00561
Russell, D. G. (2013). The Evolutionary Pressures That Have Molded Mycobacterium Tuberculosis Into an Infectious Adjuvant. Curr. Opin. Microbiol. 16 (1), 78–84. doi: 10.1016/j.mib.2012.11.007
Saunders, B. M., Britton, W. J. (2007). Life and Death in the Granuloma: Immunopathology of Tuberculosis. Immunol. Cell Biol. 85 (2), 103–111. doi: 10.1038/sj.icb.7100027
Sia, J. K., Rengarajan, J. (2019). Immunology of Mycobacterium Tuberculosis Infections. Microbiol. Spectr. 7 (4), 10.1128/microbiolspec.GPP3-0022-2018. doi: 10.1128/microbiolspec.GPP3-0022-2018
Soleimani, M., Nadri, S. (2009). A Protocol for Isolation and Culture of Mesenchymal Stem Cells From Mouse Bone Marrow. Nat. Protoc. 4 (1), 102–106. doi: 10.1038/nprot.2008.221
Takeda, K., Akira, S. (2005). Toll-Like Receptors in Innate Immunity. Int. Immunol. 17 (1), 1–14. doi: 10.1093/intimm/dxh186
Trauner, A., Borrell, S., Reither, K., Gagneux, S. (2014). Evolution of Drug Resistance in Tuberculosis: Recent Progress and Implications for Diagnosis and Therapy. Drugs 74 (10), 1063–1072. doi: 10.1007/s40265-014-0248-y
Waterman, R. S., Tomchuck, S. L., Henkle, S. L., Betancourt, A. M. (2010). A New Mesenchymal Stem Cell (MSC) Paradigm: Polarization Into a Pro-Inflammatory MSC1 or an Immunosuppressive MSC2 Phenotype. PloS One 5 (4), e10088. doi: 10.1371/journal.pone.0010088
Yang, K., Wang, J., Xiang, A. P., Zhan, X., Wang, Y., Wu, M., et al. (2013). Functional RIG-I-Like Receptors Control the Survival of Mesenchymal Stem Cells. Cell Death Dis. 4, e967. doi: 10.1038/cddis.2013.504
Young, C., Walzl, G., Du Plessis, N. (2020). Therapeutic Host-Directed Strategies to Improve Outcome in Tuberculosis. Mucosal Immunol. 13 (2), 190–204. doi: 10.1038/s41385-019-0226-5
Zhu, X., Zhao, H., Graveline, A. R., Buys, E. S., Schmidt, U., Bloch, K. D., et al. (2006). MyD88 and NOS2 Are Essential for Toll-Like Receptor 4-Mediated Survival Effect in Cardiomyocytes. Am. J. Physiol. Heart Circ. Physiol. 291 (4), H1900–H1909. doi: 10.1152/ajpheart.00112.2006
Keywords: tuberculosis, mesenchymal stem cell, NOD-2, TLR-4, autophagy
Citation: Aqdas M, Singh S, Amir M, Maurya SK, Pahari S and Agrewala JN (2021) Cumulative Signaling Through NOD-2 and TLR-4 Eliminates the Mycobacterium Tuberculosis Concealed Inside the Mesenchymal Stem Cells. Front. Cell. Infect. Microbiol. 11:669168. doi: 10.3389/fcimb.2021.669168
Received: 18 February 2021; Accepted: 21 June 2021;
Published: 07 July 2021.
Edited by:
Natarajaseenivasan Kalimuthusamy, Bharathidasan University, IndiaReviewed by:
Oleh Andrukhov, University Dental Clinic Vienna, AustriaShinsmon Jose, University of Cincinnati, United States
Copyright © 2021 Aqdas, Singh, Amir, Maurya, Pahari and Agrewala. This is an open-access article distributed under the terms of the Creative Commons Attribution License (CC BY). The use, distribution or reproduction in other forums is permitted, provided the original author(s) and the copyright owner(s) are credited and that the original publication in this journal is cited, in accordance with accepted academic practice. No use, distribution or reproduction is permitted which does not comply with these terms.
*Correspondence: Javed Naim Agrewala, amFncmV3YWxhQGlpdHJwci5hYy5pbg==
†These authors share first authorship