- 1Evidence-Based Medicine Research Center, Jiangxi University of Chinese Medicine, Nanchang, China
- 2National Institute of Parasitic Diseases, Chinese Center for Disease Control and Prevention, and WHO Collaborating Center for Tropical Diseases, Shanghai, China
- 3Key Laboratory of Parasite and Vector Biology, Ministry of Public Health, Shanghai, China
- 4School of Global Health, Chinese Center for Tropical Diseases Research, Shanghai Jiao Tong University School of Medicine, Shanghai, China
MicroRNA (miRNA or miR)-based approaches to interrupt the transmission of mosquito-borne diseases have been explored since 2005. A review of these studies and areas in which to proceed is needed. In this review, significant progress is reviewed at the level of individual miRNAs, and miRNA diversification and relevant confounders are described in detail. Current miRNA studies in mosquitoes include four steps, namely, identifying miRNAs, validating miRNA-pathogen interactions, exploring action mechanisms, and performing preapplication investigations. Notably, regarding the Plasmodium parasite, mosquito miRNAs generally bind to mosquito immunity- or development-related mRNAs, indirectly regulating Plasmodium infection; However, regarding arboviruses, mosquito miRNAs can bind to the viral genome, directly modifying viral replication. Thus, during explorations of miRNA-based approaches, researchers need select an ideal miRNA for investigation based on the mosquito species, tissue, and mosquito-borne pathogen of interest. Additionally, strategies for miRNA-based approaches differ for arboviruses and protozoan parasites.
Background
Insecticide-based interventions [e.g., long-lasting insecticide-treated bed nets (LLINs) and indoor residual spraying (IRS)] are important components of integrated mosquito management programs designed to block the transmission of mosquito-borne diseases. The insecticides used in these interventions exert strong selection pressure on resistance and lead to the evolution and spread of mosquito resistance, representing a major concern in mosquito-borne disease control programs (Xu et al., 2014). The World Health Organization (WHO) claims that innovative vector control tools are urgently needed (World Health Organization, 2012). MicroRNAs (miRNAs) are single-stranded, conserved, and small endogenous noncoding RNAs that have important regulatory functions at the posttranscriptional level in diverse organisms (Bartel, 2004; Bartel, 2009). The regulation of miRNAs is indispensable for various processes, including apoptosis, development, differentiation, viral infection, and so on (Bartel, 2004; Bartel, 2009). More importantly, the functions of miRNAs can be explored and utilized. For instance, miR-15, miR-16, miR-34, and Let-7 have been patented and approved for cancer diagnosis or treatment (Mishra et al., 2016). Given their characteristics and functions, miRNAs represent one possibility for establishing a new tool, namely, miRNA-based approaches. Thus, numerous studies on mosquito miRNAs have been performed since 2005 (Wang et al., 2005) with the ultimate goal of utilizing miRNA-based approaches for disease control (Liu et al., 2017; Feng et al., 2018a). The development of a mosquito miRNA-based approach is presumed to always follow the research roadmap of identifying mosquito miRNAs, observing miRNA-pathogen interaction, exploring action mechanisms, performing preapplication investigations and conducting clinical or field trials. Advances at each step of the roadmap need to be outlined to provide important insight into potential applications.
The Mosquito miRNA Databases Analyzed
Publications focused on investigating mosquito global miRNA profiles were selected for extraction of information, including the authors, publication year, study materials, methods, miRNA names, canonical sequences, and so on. Then, this information was supplemented during the review of the other included papers. Approximately 1635 mature or predicted miRNAs were collected. Of the 1635 miRNAs, 853 (52.17%) were limited to identification and lacked any additional study information. The remaining 782 (47.83%) were further investigated; thus, they were tracked by their annotated names for study development.
Overall, the miRNAs of 24 mosquito species, including 2 species of Aedes (Li et al., 2009; Akbari et al., 2013; Gu et al., 2013; Campbell et al., 2014; Hu et al., 2015; Maharaj et al., 2015; Liu et al., 2015; Liu Y. X. et al., 2016; Batz et al., 2017; Su et al., 2017; Zhang et al., 2017), 2 species of Culex (Skalsky et al., 2010; Hong et al., 2014), and 20 species of Anopheles mosquitoes (Wang et al., 2005; Chatterjee and Chaudhuri, 2006; Mead and Tu, 2008; Dritsou et al., 2014; Liu et al., 2014; Allam et al., 2016; Liu et al., 2017; Carissimo et al., 2018; Feng et al., 2018a; Bruno et al., 2019), were studied. The study materials involved almost every possible type, including mosquito genome sequences obtained from websites; whole mosquitoes at all developmental stages; mosquitoes at different blood feeding states, ages, pathogen infection statuses, diapause statuses or insecticide resistance states; mosquito tissues except for legs; and even mosquito cell nuclei and cytoplasm (Tables 1–6).

Table 6 Alterations in miRNA abundance in response to infection with different pathogens in various mosquito samples.
Steps of the Research Roadmap
Progress in studying individual miRNAs with annotated names in the database was tracked (Tables 1–7 and S1), and an overview of study advances is provided in Figure 1. The exploration of miRNA-based approaches proceeded through the following four steps along the proposed research roadmap: identifying mosquito miRNAs (Tables 1–5); validating pathogen- miRNA interactions (Tables 6 and S1); exploring the mechanism of action, which refers mainly to target prediction and verification (Tables 7 and S1); and performing preapplication investigations (Liu P. et al., 2016). These steps involved the 20 items listed in Figure 1, for example, interactions between miRNAs and Plasmodium, dengue virus (DENV), Zika virus (ZIKA), Chikungunya virus (CHIKV), Wolbachia, West Nile virus (WNV), Palm Creek virus (PCV), Japanese Encephalitis virus (JEV), and o’nyong’nyong virus (ONNV).
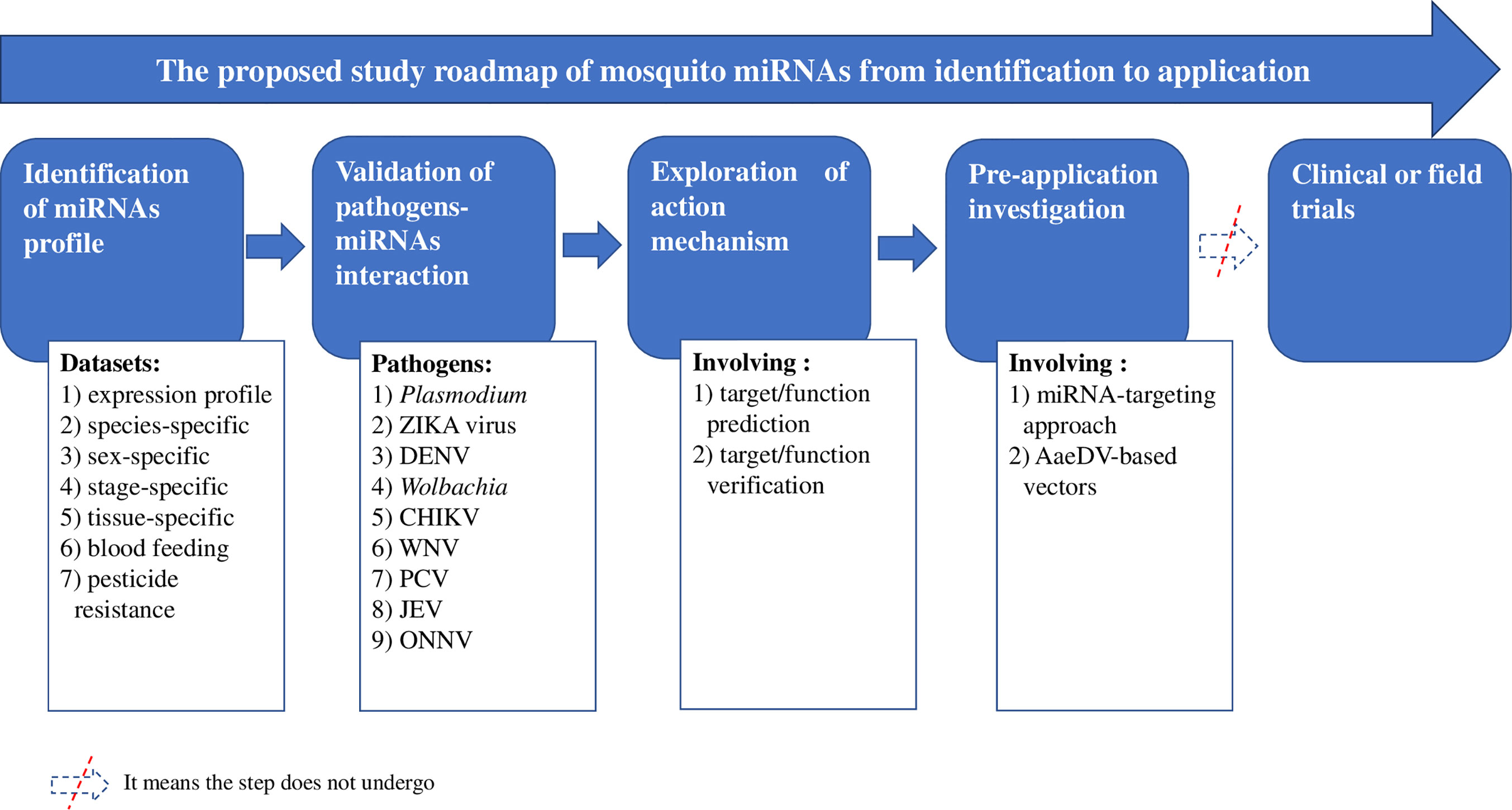
Figure 1 Overall advances in studies of mosquito miRNAs. ZIKA, Zika virus; DENV, Dengue virus; CHIKV, Chikungunya virus; WNV, West Nile virus; ONNV, o’nyong’nyong virus; JEV, Japanese encephalitis virus; PCV, Palm Creek virus; AaeDVs, Ae. aegypti densoviruses.
However, no clinical or field trial has been reported, indicating that the miRNA-based approach may have encountered a bottleneck of application in mosquito-borne disease prevention and control, although several attempts to establish application models have been conducted (Heiss et al., 2011; Tsetsarkin et al., 2015; Tsetsarkin et al., 2016a; Tsetsarkin et al., 2016b). The details of significant progress achieved at each step are reviewed below.
Advances at Each Step of the Research Roadmap
Identification of miRNA Profiles in Mosquitoes
The first step in the research roadmap is to understand mosquito miRNA profiles. Currently, studies of mosquito miRNA profiles focus mainly on mosquito miRNA identification (Mead and Tu, 2008; Skalsky et al., 2010; Gu et al., 2013; Jain et al., 2014; Castellano et al., 2015; Etebari et al., 2015; Hu et al., 2015; Allam et al., 2016; Su et al., 2017; Carissimo et al., 2018; Feng et al., 2018a) with the detection of spatial and temporal expression patterns (Jain et al., 2014; Yen et al., 2018), the functional arm between the 5’ and 3’ ends (Skalsky et al., 2010; Biryukova et al., 2014; Etebari et al., 2015), the production of miRNAs with varying lengths and sequences (i.e., isomiRs) (Skalsky et al., 2010; Biryukova et al., 2014; Castellano et al., 2015; Nouzova et al., 2018), and miRNA clusters (Biryukova et al., 2014). At this step in the roadmap, variations in the spatial and temporal expression of miRNAs have been observed by analyzing several factors. (i) Mosquito species: For example, miR-282-5p was found to be conserved in Ae. aegypti and An. gambiae but not in Cx. quinquefasciatus (Gu et al., 2013) (Table 2). Additionally, miR-1175-3p expression has been found to display opposite trends (upregulated or downregulated by blood feeding) in Aedes albopictus and Anopheles gambiae, and the corresponding molecular mechanisms may also be different in each species (Winter et al., 2007; Su et al., 2017) (Table 4). (ii) Sexes: For example, miR-989-5p was found to be restricted in female Anopheles coluzzii (Bruno et al., 2019) (Table 3). (iii) Developmental stages: Notably, miR-1-3p was found to be enriched in the pupa of female Anopheles stephensi (Jain et al., 2015) compared to the larvae and adult (Table 4). (iv) Blood feeding and insecticide resistance statuses: The expression of miR-999-3p was found to be downregulated in deltamethrin-resistant Culex pipiens (Hong et al., 2014) (Table 4). (v) Tissues: For example, miR-998-5p was found to be specifically expressed in the ovary of An. gambiae (Lampe and Levashina, 2018) (Table 5), and miR-8-3p was found to be particularly enriched in the salivary glands in An. coluzzii (Bruno et al., 2019) but in the fat body in Aedes aegypti (Bryant et al., 2010) (Table 5). Moreover, in addition to these specificities, miRNAs may even exhibit cellular cytoplasm- or nucleus- specificity (Mayoral et al., 2014) (Table 5).
More examples based on individual miRNAs are noted in Tables 2–5. Overall, the expression levels of miRNAs are regulated by complicated factors, including mosquito species, sexes, developmental stages, tissues or organs, aging, blood feeding, and so on (Tables 2–5). In addition to differences in expression levels, the preferred or functional arm also varies among these factors in terms of the change in 5p/3p ratio or even dominant arm shifts (Skalsky et al., 2010; Biryukova et al., 2014; Castellano et al., 2015). For example, the 5p/3p ratios of miR-956-3p and miR-219-5p are significantly reduced by blood feeding (Biryukova et al., 2014). Moreover, isomiR production based on acylation, uridylation, adenine and uracil extension/addition can be induced by blood feeding and insecticide resistance (Skalsky et al., 2010; Biryukova et al., 2014).
miRNA-Pathogen Interactions in the Mosquitoes
The second step in the research roadmap always begins with an observation of statistical correlations between miRNA regulation and pathogen infection in mosquitoes. The pathogens primarily include Plasmodium, DENV, CHIKV, Wolbachia, Zika virus, WNV, JEV, PCV and ONNV (Table 6). The miRNA abundance may vary upon pathogen infection in mosquitoes according to differences in the studied material, e.g., miR-10-5p is upregulated in CHIKV-infected Ae. aegypti (Dubey et al., 2017); conversely, it is downregulated in DENV-infected Ae. aegypti (Liu et al., 2015; Etebari et al., 2015) (Table 6). More importantly, a few miRNAs were found to exhibit similar regulation patterns in different independent studies, and the repeatability of these results makes them more reliable (Table 6), as described in the following examples below. The miR-10-5p, -125-5p, -143, -275-3p, -277-3p, -308-5p, and -927-5p have been consistently shown to be upregulated upon CHIKV infection (Table 6). Downregulation of miR-133-3p, -14-3p, -252-5p, -275-3p, miR-306-3p, -71-3p, -957-3p, -970-3p, -980-3p, or let-7-5p has been observed upon Plasmodium infection (Table 6). Upregulation of miR-1767, -34-5p, or -622 and downregulation of miR-1-3p, -275-3p, -317-3p, -4448, -8-3p, or bantam-5p have been detected upon DENV infection. Upregulation of miR-125-5p, -252-5p, -277-3p, -281-3p, -2940-5p, -2941-3p, -308-5p, or let-7-5p and downregulation of miR-210-3p, -2945-3p, or -989-3p have been observed upon Wolbachia infection. Notably, miR-2940-5p, -375-3p, -87-3p, -988-5p, and -999-3p are consistently regulated by CHIKV and DENV, which may provide insight into coregulation by these two pathogens and the subsequent codevelopment of miRNA-based approaches for transmission control. More specifically, miR-2940-5p is inversely regulated by DENV and Wolbachia, consistent with the results that Wolbachia uses miRNA-2940-5p to inhibit DENV infection in Ae. aegypti (Hussain et al., 2011; Zhang et al., 2013) (Table 6).
As in the first study step in the roadmap, in addition to differences in expression levels, changes in 5p/3p ratio, dominant arm shifts, and isomiR production can be modified by pathogen infection (Etebari et al., 2015).
JEV, PCV and ONNV pathogens do not appear in the summary presented in Table 6. Except for a study showing that miR-124 inhibits JEV replication in PK15 porcine kidney epithelial cells (Yang et al., 2016), no report that has indicated that miRNAs are statistically correlated with JEV infection in mosquito or mosquito cells. PCV and ONNV infection exert remarkably limited effects on the mosquito miRNA profile; therefore, miRNAs may not play an important role in the interaction of PCV with Ae. aegypti (Lee et al., 2017) or ONNV with Anopheles coluzzii (Carissimo et al., 2018). Thus, researchers are currently unable to select a miRNA as an ideal candidate to establish a miRNA-based approach for the control of three mosquito-borne diseases.
After statistical correlations between miRNA alterations and pathogen infection being observed, their causal relationship should be confirmed (Tables 7 and S1). Overexpression or suppression of a miRNA is the most widely used approach to study causality (Jones-Rhoades et al., 2006). Eighteen miRNAs have been validated to exert promotive or inhibitory effects on CHIKV (Maharaj et al., 2015; Dubey et al., 2019), DENV (Hussain et al., 2013; Zhang et al., 2013; Yan et al., 2014; Zhou et al., 2014; Su et al., 2017; Su et al., 2019; Avila-Bonilla et al., 2020), WNV (Slonchak et al., 2014), Plasmodium (Jain et al., 2014; Dennison et al., 2015; Lampe et al., 2019; Dong et al., 2020), Wolbachia (Hussain et al., 2011; Zhang et al., 2014), or JEV (Yang et al., 2016) infections via these types of experiments (Table 7). Notably, miR-2940-5p restricts the replication of both WNV and DENV in mosquitoes (Zhang et al., 2013; Slonchak et al., 2014), and miR-375-3p exerts the opposite effect on DENV-2 and CHIKV (Hussain et al., 2013; Maharaj et al., 2015). It is easy to find that the upregulating and downregulating miRNAs in response to pathogen infection co-exist in the mosquito (Table 7). No miRNA has been reported to induce multi-antipathogen effects on the two kinds of flaviviruses and Plasmodium protozoans. A more detailed description of the progress achieved by studies examining miRNA-pathogen interactions in mosquitoes is presented in Table S1.
Exploration of the Mechanism of Action
Research on the mechanism of action mostly focuses on the prediction and verification of miRNA targets or functions through genetic disruption methods. Bioinformatic analysis tools, such as TargetScan, PITA and RNAhybrid, are always used for target prediction. The verification methods usually include the transfection of miRNA-specific antagomirs into mosquito cells, miRNA mimic/inhibitor microinjection in mosquitoes, real-time quantitative polymerase chain reaction (qRT-PCR), or luciferase assays (Liu B. et al., 2016; Ma et al., 2017; Nouzova et al., 2018; Yen et al., 2019; Avila-Bonilla et al., 2020; Fu et al., 2020). The most recent method applied to elucidate the targets and biological functions of mosquito miRNAs is high-throughput sequencing of covalent ligation of endogenous Argonaute-bound RNAs isolated by crosslinking and immunoprecipitation (CLEAR-CLIP). In this assay, the miRNA and its target mRNA are joined in the purified RNA-induced silencing complex (RISC) complex to form one chimeric molecule. Analysis of the chimeric miRNA-target molecule among the RNA molecules associated with Argonaute (AGOs) proteins facilitates the systematic identification of miRNA-target interactions (Dong et al., 2020).
The miRNAs noted in bold in Table S1 have been confirmed to contribute to blood digestion (Bryant et al., 2010; Jain et al., 2014), egg development (Bryant et al., 2010; Puthiyakunnon et al., 2013; Jain et al., 2014; Lucas et al., 2015a; Zhang et al., 2017), ovary development (Ling et al., 2017), larval eclosion (Puthiyakunnon et al., 2013; Feng et al., 2018b), reproduction (Zhang et al., 2016; Fu et al., 2017), the stability and nuclear translocation of AGO1 (Hussain et al., 2013), lipid accumulation (Batz et al., 2017), metabolism (Ling et al., 2017), host-pathogen interactions (Yan et al., 2014; Lucas et al., 2015b; Dubey et al., 2019; Yen et al., 2019), and insecticide resistance (Hong et al., 2014) (Table S1).
In the canonical mechanism of action of miRNAs, mature miRNAs guide the RISC to the 3’ untranslated regions (UTRs) of target mRNAs via complementary base pair interactions, thus regulating the expression of target genes (Hammond et al., 2000; Lee et al., 2002; Lee et al., 2003). Most miRNA-target (mRNA) interactions are consistent with the canonical action mechanism; however, exceptions have been identified for miRNA-virus interactions (e.g., DENV and CHIKV) in terms of the target type or regulatory outcome. First, during arbovirus infection, mosquito miRNAs can directly bind to the 3’-UTR of the viral genome (not necessary an mRNA), regulating virus replication (Yan et al., 2014; Lucas et al., 2015b; Dubey et al., 2019; Yen et al., 2019), which differs from the canonical mechanism of action. However, the mechanisms underlying mosquito miRNA-Plasmodium interactions are always consistent with the canonical mechanism of action, namely, miRNAs generally bind to mosquito immunity- or development-related mRNAs, indirectly regulating pathogen infection (Jain et al., 2014; Dennison et al., 2015; Dong et al., 2020) (Table S1). However, the exact mechanism of translational or viral repression remains unclear (Winter et al., 2007). Second, miRNAs always negatively regulate their targets by inducing mRNA cleavage (Yekta et al., 2004) or degradation (Eichhorn et al., 2014), or by repressing translation (Fabian and Sonenberg, 2012); however, positive regulation by miRNAs is repeatedly observed in mosquitoes (Hussain et al., 2013; Zhou et al., 2014; Maharaj et al., 2015; Su et al., 2019). In addition to repressing gene expression, miRNAs can also induce the expression of genes with complementary promoter sequences, switching these genes from repressed to activated (Hussain et al., 2013; Zhou et al., 2014; Maharaj et al., 2015; Su et al., 2019).
More interestingly, the miRNA-target interaction may involve a complex network. A network was observed among clusters of miR-2-3p, miR-13-3p, miR-71-5p, CYP9J35 (a target of miR-2-3p and -13-3p), and CYP325BG3 (a target of miR-71-5p) in insecticide resistant Cx. pipiens (Hong et al., 2014).
Moreover, infection with one pathogen affects coinfection with another pathogen in mosquitoes, especially for Wolbachia or engineered mosquito densoviruses (MDVs), which can modify host miRNA profiles or use a specific host miRNA to manipulate pathogen invasion in mosquitoes (Osei-Amo et al., 2012; Maharaj et al., 2015; Liu P. et al., 2016).
Preapplication Investigation
Of the 1635 putative or mature miRNAs reported in mosquitoes, only a few have advanced to the step of preapplication investigations, and the names of these miRNAs are italicized in Table S1. The first attempt to establish an application is to exploit the vector specificity and stability of MDVs, which are restricted to mosquitoes. Anti-miRNA sponges targeting endogenous let-7-5p and miR-210-3p were introduced into MDVs in Ae. aegypti (noted as AaeDV-based vectors in Figure 1), and both sponges downregulated the expression levels of these miRNAs. According to the study, this recombinant vector is useful to purposefully inhibit or promote the expression of endogenous miRNAs and subsequently regulate pathogen infection in mosquitoes (Liu P. et al., 2016). This study is similar to a study research that used a plasmid construction technique to express artificial miRNAs that inhibit JEV (Wu et al., 2011) and DENV (Xie et al., 2013) in vitro or impede mosquito reproduction and embryonic development (Biedler et al., 2014).
Given the specificity of miRNA-virus interactions in which mosquito miRNAs can directly inhibit the virus via complementary base pair interactions, methods to introduce sequences complementary to mosquito miRNAs (noted as miRNA-targeting approaches in Figure 1) into arboviruses have been established (Heiss et al., 2011; Tsetsarkin et al., 2015; Tsetsarkin et al., 2016a; Tsetsarkin et al., 2016b). The introduction of a single copy of a miRNA target sequence into the DENV genome was shown to lead to the reduction of DENV 4 replication in vivo and in vitro (Heiss et al., 2011; Tsetsarkin et al., 2015), consistent with the results of a similar study with another pathogen, JEV (Yen et al., 2013). More interestingly, multiple insertions of heterologous target sequences of different miRNAs into the virus were shown to increase virus attenuation, whereas the insertion of two or three copies of homologous sequence (the same miRNAs) into the virus did not increase virus attenuation (Tsetsarkin et al., 2016a; Tsetsarkin et al., 2016b).
The two preapplication investigations indicate the possible application of miRNA-based approaches, e.g., 1) expressing a miRNA inhibitor in vector mosquitoes by establishing genetically modified mosquitoes, subsequently reducing the fitness between mosquitoes and pathogens and interrupting the transmission of mosquito-borne pathogens (Heiss et al., 2011; Tsetsarkin et al., 2015; Tsetsarkin et al., 2016a; Tsetsarkin et al., 2016b); and 2) inserting specific miRNA target sequences into the flavivirus genome, resulting in selective tissue-specific attenuation and nonhuman-range restriction of live attenuated vaccine viruses (Tsetsarkin et al., 2016a; Tsetsarkin et al., 2016b).
Concluding Remarks
Currently, miRNA-based approaches employ four steps that address 20 aspects as listed in Figure 1. These exploratory studies are limited because of the bottleneck at the preapplication investigation step (Figure 1) and require further advances towards field or clinical applications. Twenty-four mosquito species have been analyzed for miRNA-related studies. The study materials have ranged widely, from entire mosquitoes to the cytoplasm or nucleus of mosquito cells, from eggs to adult mosquitoes, or from sugar-fed to pathogen-infected mosquitoes (Tables 1–6).
The expression of miRNAs is regulated by complex factors, including mosquito species, sex, developmental stage, tissue or organ, age, blood feeding status, pathogen infection status and pathogen type (Tables 2–7). Thus, miRNA expression levels detected in entire mosquitoes may lead to biased results, and for one arbovirus, some miRNAs may promote infection in mosquitoes, while for another arbovirus, miRNAs may inhibit infection (Table 7). Thus, during the exploration of miRNA-based approaches for the interruption of mosquito-borne disease transmission, an irrational approach is to commonly define a miRNA as solely inhibiting or promoting pathogen infection in mosquitoes, when the actual effects of a miRNA depend on those complex factors. Most importantly, the results presented here indicate that the selection of a candidate miRNA according to unique conditions or objectives during miRNA-based approach development is crucial. The current statuses of individual miRNAs presented in Tables 1–7 and S1 provide guidance for selection.
As described above, the main variations in miRNAs attributed to the mosquito species or infecting pathogen include changes in the expression level, isomiR production, or 5p/3p ratio or even a shift in the dominant arm. In our opinion, these variations in miRNAs might collectively or individually affect the formulation of miRNA-target RISC complexes, and subsequently influence the fitness between the mosquito and pathogen (Winter et al., 2007). And for mosquito miRNA-arbovirus interactions, the targets of miRNAs can be RNA genomes of arboviruses, which are always mRNA obeying the canonical action mechanism. These viewpoints are presented in Figure 2.
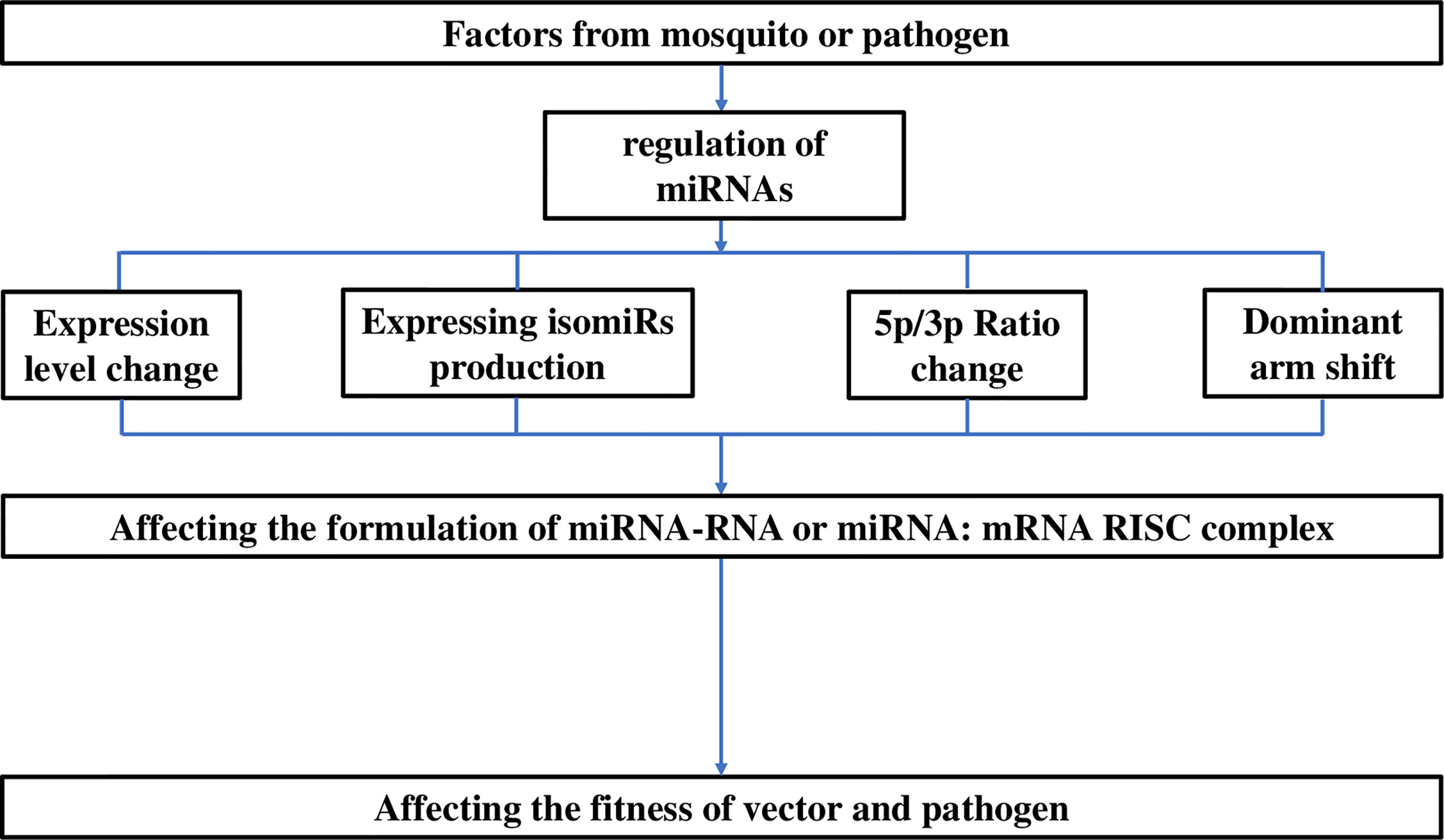
Figure 2 Responses of miRNAs to characteristics of mosquitoes or pathogens and subsequent miRNA-pathogen interactions in mosquitoes. The responses involve modifications in the expression level, isomiR production, or 5p/3p ratio or shift in dominant arm, and any of these alterations can affect the fitness between the vector and pathogen. During modification by miRNAs, the functional component is the formation of the miRNA-target RISC complex. For arboviruses, the complex can be composed of miRNAs and the virus genome (miR-RNA complex) in mosquitoes, directly regulating pathogen infection; however, for Plasmodium parasites, it is always composed of miRNAs and mosquito immunity-related mRNAs (miR-mRNA complex), indirectly regulating the infection.
Moreover, although the canonical action mechanism of miRNAs always results in repression, the mosquito miRNA-target interaction can lead to two possible forms of regulation, namely, repression or enhancement of pathogen infection in mosquitoes. Both upregulation and downregulation of miRNAs in response to pathogen infection widely coexist in the mosquito, subsequently promoting and inhibiting pathogen infection, respectively. In our opinion, these findings suggest that inhibitory and inducing miRNA expressions are essential to balance the miRNA-pathogen interaction, maintaining persistent infection and preventing considerable harm to the mosquito (Figure 3).
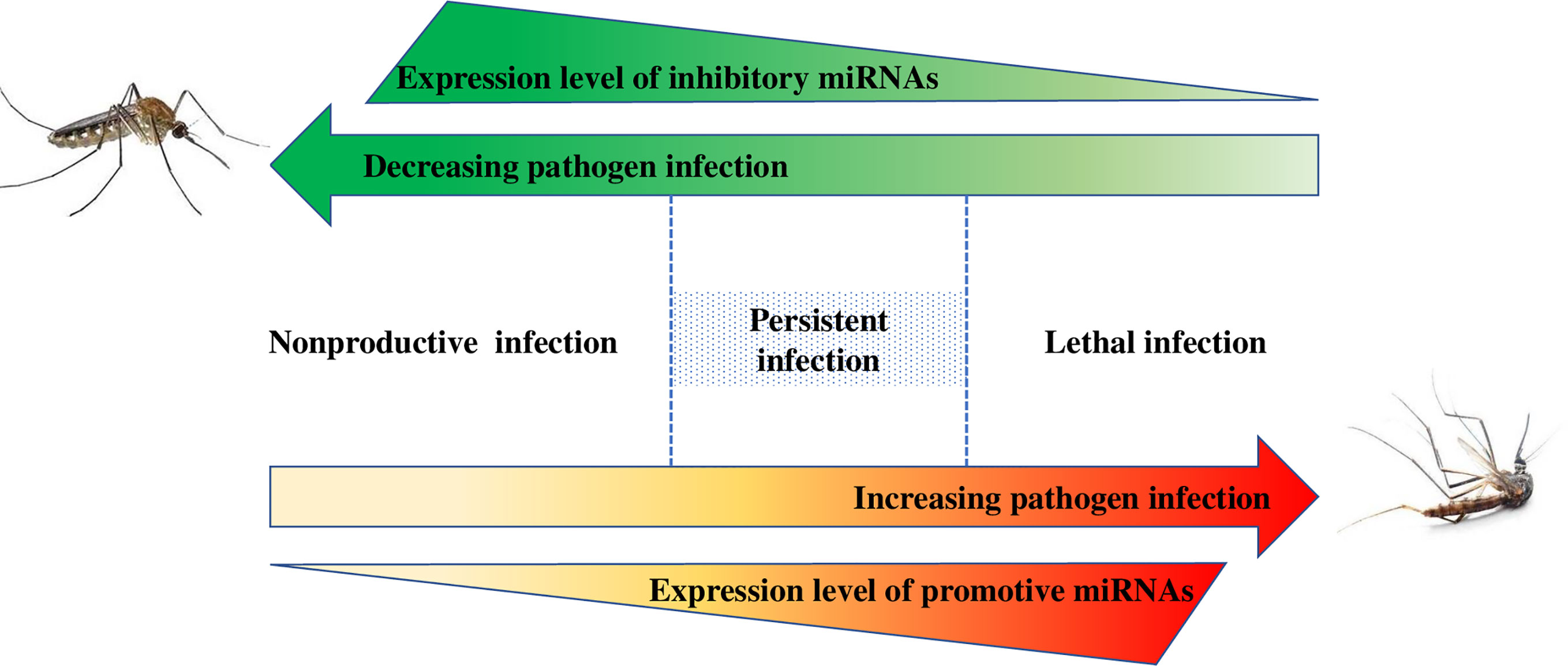
Figure 3 Causal relationship between mosquito miRNAs and pathogen infection. The manipulation by miRNAs is mediated via a network. The inhibitory miRNAs and promotive miRNAs always coexist in mosquitoes, inhibiting or promoting pathogen infection, respectively. These miRNAs can balance the miRNA-pathogen interaction, maintain persistent infection and preventing considerable harm to the mosquito.
Currently, the antiviral effects of mosquito miRNAs on pathogens in combination with genetic engineering and molecular biology techniques may allow the use of these miRNA-based approaches as new tools to interrupt the transmission of mosquito-borne diseases. In this review, the significant progress achieved at the level of individual miRNAs facilitates the selection of an abundant, specific and effective mosquito miRNA (see Tables 1–7 and S1) that can be referenced for further research with different and specific objectives to increase the pace of development of applications and overcome the bottleneck (Tsetsarkin et al., 2015). More importantly, mosquito miRNAs can directly bind to the arbovirus genome, modifying viral replication. However, regarding the Plasmodium parasite, mosquito miRNAs generally bind to mosquito immunity or development-related mRNAs, indirectly regulating Plasmodium infection. Hence, the strategies for miRNA-based approaches differ for arboviruses and protozoan parasites.
Author Contributions
Conceptualization and formal analysis: T-LX. Data curation: T-LX, Y-WS, and X-YF.
Supervision: BZ and X-NZ. Writing-original draft: T-LX. Writing-review & editing: BZ and X-NZ. All authors contributed to the article and approved the submitted version.
Funding
BZ received a grant from the National Science and Technology Major Program of China (No. 2018ZX10734-404). This project was financially supported by Ministry of Science and Technology of the People’s Republic of China. The funder had no role in study design, data collection and analysis, decision to publish, or preparation of the manuscript.
Conflict of Interest
The authors declare that the research was conducted in the absence of any commercial or financial relationships that could be construed as a potential conflict of interest.
Acknowledgments
The ideas presented here were developed during the completion of research projects funded by the Belt and Road Young Scientist Research Exchange Program (No. 17430741900).
Supplementary Material
The Supplementary Material for this article can be found online at: https://www.frontiersin.org/articles/10.3389/fcimb.2021.665444/full#supplementary-material
References
Akbari, O. S., Antoshechkin, I., Amrhein, H., Williams, B., Diloreto, R., Sandler, J., et al. (2013). The Developmental Transcriptome of the Mosquito Aedes Aegypti, an Invasive Species and Major Arbovirus Vector. G3 3, 1493–1509. doi: 10.1534/g3.113.006742
Allam, M., Spillings, B. L., Abdalla, H., Mapiye, D., Koekemoer, L. L., Christoffels, A. (2016). Identification and Characterization of microRNAs Expressed in the African Malaria Vector Anopheles Funestus Life Stages Using High Throughput Sequencing. Malaria J. 15, 542. doi: 10.1186/s12936-016-1591-0
Asad, S., Hussain, M., Hugo, L., Osei-Amo, S., Zhang, G., Watterson, D., et al. (2018). Suppression of the Pelo Protein by Wolbachia and Its Effect on Dengue Virus in Aedes Aegypti. PloS Negl. Trop. Dis. 12, e0006405. doi: 10.1371/journal.pntd.0006405
Avila-Bonilla, R. G., Yocupicio-Monroy, M., Marchat, L. A., De Nova-Ocampo, M. A., Del Angel, R. M., Salas-Benito, J. S. (2017). Analysis of the miRNA Profile in C6/36 Cells Persistently Infected With Dengue Virus Type 2. Virus Res. 232, 139–151. doi: 10.1016/j.virusres.2017.03.005
Avila-Bonilla, R. G., Yocupicio-Monroy, M., Marchat, L. A., Perez-Ishiwara, D. G., Cerecedo-Mercado, D. A., Del Angel, R. M., et al. (2020). miR-927 Has Pro-Viral Effects During Acute and Persistent Infection With Dengue Virus Type 2 in C6/36 Mosquito Cells. J. Gen. Virol. 101, 8. doi: 10.1099/jgv.0.001441
Bartel, D. P. (2004). MicroRNAs: Genomics, Biogenesis, Mechanism, and Function. Cell 116, 281–297. doi: 10.1016/S0092-8674(04)00045-5
Bartel, D. P. (2009). MicroRNAs: Target Recognition and Regulatory Functions. Cell 136, 215–233. doi: 10.1016/j.cell.2009.01.002
Batz, Z. A., Goff, A. C., Armbruster, P. A. (2017). MicroRNAs Are Differentially Abundant During Aedes Albopictus Diapause Maintenance But Not Diapause Induction. Insect Mol. Biol. 26, 721–733. doi: 10.1111/imb.12332
Behura, S. K., Haugen, M., Flannery, E., Sarro, J., Tessier, C. R., Severson, D. W., et al. (2011). Comparative Genomic Analysis of Drosophila Melanogaster and Vector Mosquito Developmental Genes. PloS One 6, e21504. doi: 10.1371/journal.pone.0021504
Biedler, J. K., Qi, Y., Pledger, D., Macias, V. M., James, A. A., Tu, Z. (2014). Maternal Germline-Specific Genes in the Asian Malaria Mosquito Anopheles Stephensi: Characterization and Application for Disease Control. G3 5, 157–166. doi: 10.1534/g3.114.015578
Biryukova, I., Ye, T., Levashina, E. (2014). Transcriptome-Wide Analysis of microRNA Expression in the Malaria Mosquito Anopheles Gambiae. BMC Genomics 15, 557. doi: 10.1186/1471-2164-15-557
Bruno, A., Alessio, C., Carmine, F., Francesco, S., Vladimir, B., Marco, D. L., et al. (2019). MicroRNAs From Saliva of Anopheline Mosquitoes Mimic Human Endogenous miRNAs and may Contribute to Vector-Hostpathogen Interactions. Sci. Rep. 9, 2955. doi: 10.1038/s41598-019-39880-1
Bryant, B., Macdonald, W., Raikhel, A. S. (2010). microRNA miR-275 Is Indispensable for Blood Digestion and Egg Development in the Mosquito Aedes Aegypti. Proc. Natl. Acad. Sci. U. S. A. 107, 22391–22398. doi: 10.1073/pnas.1016230107
Bryant, W. B., Mills, M. K., Olson, B. J., Michel, K. (2019). Small RNA-Seq Analysis Reveals Mirna Expression Dynamics Across Tissues in the Malaria Vector, Anopheles Gambiae. G3 9, 1507–1517. doi: 10.1534/g3.119.400104
Bryant, W. B., Ray, S., Mills, M. K. (2020). Global Analysis of Small non-Coding RNA Populations Across Tissues in the Malaria Vector, Anopheles Gambiae. Insects 11, 7. doi: 10.3390/insects11070406
Campbell, C. L., Harrison, T., Hess, A. M., Ebel, G. D. (2014). MicroRNA Levels Are Modulated in Aedes Aegypti After Exposure to Dengue-2. Insect Mol. Biol. 23, 132–139. doi: 10.1111/imb.12070
Carissimo, G., Pain, A., Belda, E., Vernick, K. D. (2018). Highly Focused Transcriptional Response of Anopheles Coluzzii to O’nyong Nyong Arbovirus During the Primary Midgut Infection. BMC Genomics 19, 526. doi: 10.1186/s12864-018-4918-0
Castellano, L., Rizzi, E., Krell, J., Di Cristina, M., Galizi, R., Mori, A., et al. (2015). The Germline of the Malaria Mosquito Produces Abundant miRNAs, endo-siRNAs, piRNAs and 29-Nt Small RNAs. BMC Genomics 16, 100. doi: 10.1186/s12864-015-1257-2
Chatterjee, R., Chaudhuri, K. (2006). An Approach for the Identification of microRNA With an Application to Anopheles Gambiae. Acta Biochim. Polonica 53, 303–309. doi: 10.18388/abp.2006_3343
Dennison, N. J., BenMarzouk-Hidalgo, O. J., Dimopoulos, G. (2015). MicroRNA-Regulation of Anopheles Gambiae Immunity to Plasmodium Falciparum Infection and Midgut Microbiota. Dev. Comp. Immunol. 49, 170–178. doi: 10.1016/j.dci.2014.10.016
Dong, S., Fu, X., Dong, Y., Simoes, M. L., Zhu, J., Dimopoulos, G. (2020). Broad Spectrum Immunomodulatory Effects of Anopheles Gambiae microRNAs and Their Use for Transgenic Suppression of Plasmodium. PloS Pathog. 16, e1008453. doi: 10.1371/journal.ppat.1008453
Dritsou, V., Deligianni, E., Dialynas, E., Allen, J., Poulakakis, N., Louis, C., et al. (2014). Non-Coding RNA Gene Families in the Genomes of Anopheline Mosquitoes. BMC Genomics 15, 1038. doi: 10.1186/1471-2164-15-1038
Dubey, S. K., Shrinet, J., Jain, J., Ali, S., Sunil, S. (2017). Aedes Aegypti microRNA miR-2b Regulates Ubiquitin-Related Modifier to Control Chikungunya Virus Replication. Sci. Rep. 7, 17666. doi: 10.1038/s41598-017-18043-0
Dubey, S. K., Shrinet, J., Sunil, S. (2019). Aedes Aegypti microRNA, miR-2944b-5p Interacts With 3’UTR of Chikungunya Virus and Cellular Target vps-13 to Regulate Viral Replication. PloS Negl. Trop. Dis. 13, e0007429. doi: 10.1371/journal.pntd.0007429
Eichhorn, S. W., Guo, H., McGeary, S. E., Rodriguez-Mias, R. A., Shin, C., Baek, D., et al. (2014). mRNA Destabilization Is the Dominant Effect of Mammalian microRNAs by the Time Substantial Repression Ensues. Mol. Cell 56, 104–115. doi: 10.1016/j.molcel.2014.08.028
Etebari, K., Osei-Amo, S., Blomberg, S. P., Asgari, S. (2015). Dengue Virus Infection Alters Post-Transcriptional Modification of microRNAs in the Mosquito Vector Aedes Aegypti. Sci. Rep. 5, 15968. doi: 10.1038/srep15968
Fabian, M. R., Sonenberg, N. (2012). The Mechanics of miRNA-Mediated Gene Silencing: A Look Under the Hood of Mirisc. Nat. Struct. Mol. Biol. 19, 586–593. doi: 10.1038/nsmb.2296
Feng, X., Wu, J., Zhou, S., Wang, J., Hu, W. (2018b). Characterization and Potential Role of microRNA in the Chinese Dominant Malaria Mosquito Anopheles Sinensis (Diptera: Culicidae) Throughout Four Different Life Stages. Cell Bioscience 8, 29. doi: 10.1186/s13578-018-0227-1
Feng, X., Zhou, X., Zhou, S., Wang, J., Hu, W. (2018a). Analysis of microRNA Profile of Anopheles Sinensis by Deep Sequencing and Bioinformatic Approaches. Parasites Vectors 11, 172. doi: 10.1186/s13071-018-2734-7
Fu, X., Dimopoulos, G., Zhu, J. (2017). Association of microRNAs With Argonaute Proteins in the Malaria Mosquito Anopheles Gambiae After Blood Ingestion. Sci. Rep. 7, 6493. doi: 10.1038/s41598-017-07013-1
Fu, X., Liu, P., Dimopoulos, G., Zhu, J. (2020). Dynamic miRNA-mRNA Interactions Coordinate Gene Expression in Adult Anopheles Gambiae. PloS Genet. 16, e1008765. doi: 10.1371/journal.pgen.1008765
Gu, J., Hu, W., Wu, J., Zheng, P., Chen, M., James, A. A., et al. (2013). miRNA Genes of an Invasive Vector Mosquito, Aedes Albopictus. PloS One 8, e67638. doi: 10.1371/journal.pone.0067638
Guo, Q., Huang, Y., Zou, F., Liu, B., Tian, M., Ye, W., et al. (2017). The Role of miR-2~13~71 Cluster in Resistance to Deltamethrin in Culex Pipiens Pallens. Insect Biochem. Mol. Biol. 84, 15–22. doi: 10.1016/j.ibmb.2017.03.006
Hammond, S. M., Bernstein, E., Beach, D., Hannon, G. J. (2000). An RNA-directed Nuclease Mediates Post-Transcriptional Gene Silencing in Drosophila Cells. Nature 404, 293–296. doi: 10.1038/35005107
Heiss, B. L., Maximova, O. A., Pletnev, A. G. (2011). Insertion of microRNA Targets Into the Flavivirus Genome Alters Its Highly Neurovirulent Phenotype. J. Virol. 85, 1464–1472. doi: 10.1128/JVI.02091-10
Hong, S., Guo, Q., Wang, W., Hu, S., Fang, F., Lv, Y., et al. (2014). Identification of Differentially Expressed microRNAs in Culex Pipiens and Their Potential Roles in Pyrethroid Resistance. Insect Biochem. Mol. Biol. 55, 39–50. doi: 10.1016/j.ibmb.2014.10.007
Hu, W., Criscione, F., Liang, S., Tu, Z. (2015). MicroRNAs of Two Medically Important Mosquito Species: Aedes Aegypti and Anopheles Stephensi. Insect Mol. Biol. 24, 240–252. doi: 10.1111/imb.12152
Hussain, M., Frentiu, F. D., Moreira, L. A., O’Neill, S. L., Asgari, S. (2011). Wolbachia Uses Host microRNAs to Manipulate Host Gene Expression and Facilitate Colonization of the Dengue Vector Aedes Aegypti. Proc. Natl. Acad. Sci. U. S. A. 108, 9250–9255. doi: 10.1073/pnas.1105469108
Hussain, M., Walker, T., O’Neill, S. L., Asgari, S. (2013). Blood Meal Induced microRNA Regulates Development and Immune Associated Genes in the Dengue Mosquito Vector, Aedes Aegypti. Insect Biochem. Mol. Biol. 43, 146–152. doi: 10.1016/j.ibmb.2012.11.005
Jain, S., Rana, V., Shrinet, J., Sharma, A., Tridibes, A., Sunil, S., et al. (2014). Blood Feeding and Plasmodium Infection Alters the miRNome of Anopheles Stephensi. PloS One 9, e98402. doi: 10.1371/journal.pone.0098402
Jain, S., Rana, V., Tridibes, A., Sunil, S., Bhatnagar, R. K. (2015). Dynamic Expression of miRNAs Across Immature and Adult Stages of the Malaria Mosquito Anopheles Stephensi. Parasites Vectors 8, 179. doi: 10.1186/s13071-015-0772-y
Jones-Rhoades, M. W., Bartel, D. P., Bartel, B. (2006). MicroRNAs and Their Regulatory Roles in Plants. Annu. Rev. Plant Biol. 57, 19–53. doi: 10.1146/annurev.arplant.57.032905.105218
Lampe, L., Jentzsch, M., Kierszniowska, S., Levashina, E. A. (2019). Metabolic Balancing by miR-276 Shapes the Mosquito Reproductive Cycle and Plasmodium Falciparum Development. Nat. Commun. 10, 5634. doi: 10.1038/s41467-019-13627-y
Lampe, L., Levashina, E. A. (2018). Microrna Tissue Atlas of the Malaria Mosquito Anopheles Gambiae. G3 8, 185–193. doi: 10.1534/g3.117.300170
Lee, Y., Ahn, C., Han, J., Choi, H., Kim, J., Yim, J., et al. (2003). The Nuclear RNase III Drosha Initiates microRNA Processing. Nature 425, 415–419. doi: 10.1038/nature01957
Lee, M., Etebari, K., Hall-Mendelin, S., van den Hurk, A. F., Hobson-Peters, J., Vatipally, S., et al. (2017). Understanding the Role of microRNAs in the Interaction of Aedes Aegypti Mosquitoes With an Insect-Specific Flavivirus. J. Gen. Virol. 98, 1892–1903. doi: 10.1099/jgv.0.000832
Lee, Y., Jeon, K., Lee, J. T., Kim, S., Kim, V. N. (2002). MicroRNA Maturation: Stepwise Processing and Subcellular Localization. EMBO J. 21, 4663–4670. doi: 10.1093/emboj/cdf476
Li, S., Mead, E. A., Liang, S., Tu, Z. (2009). Direct Sequencing and Expression Analysis of a Large Number of miRNAs in Aedes Aegypti and a Multi-Species Survey of Novel Mosquito Mirnas. BMC Genomics 10, 581. doi: 10.1186/1471-2164-10-581
Ling, L., Kokoza, V. A., Zhang, C., Aksoy, E., Raikhel, A. S. (2017). MicroRNA-277 Targets Insulin-Like Peptides 7 and 8 to Control Lipid Metabolism and Reproduction in Aedes Aegypti Mosquitoes. Proc. Natl. Acad. Sci. U. S. A. 114, E8017–E8024. doi: 10.1073/pnas.1710970114
Liu, W., Hao, Z., Huang, L., Chen, L., Wei, Q., Cai, L., et al. (2017). Comparative Expression Profile of microRNAs in Anopheles Anthropophagus Midgut After Blood-Feeding and Plasmodium Infection. Parasites Vectors 10, 86. doi: 10.1186/s13071-017-2027-6
Liu, W., Huang, H., Xing, C., Li, C., Tan, F., Liang, S. (2014). Identification and Characterization of the Expression Profile of microRNAs in Anopheles Anthropophagus. Parasites Vectors 7, 159. doi: 10.1186/1756-3305-7-159
Liu, P., Li, X., Gu, J., Dong, Y., Liu, Y., Santhosh, P., et al. (2016). Development of Non-Defective Recombinant Densovirus Vectors for microRNA Delivery in the Invasive Vector Mosquito, Aedes Albopictus. Sci. Rep. 6, 20979. doi: 10.1038/srep20979
Liu, Y. X., Li, F. X., Liu, Z. Z., Jia, Z. R., Zhou, Y. H., Zhang, H., et al. (2016). Integrated Analysis of miRNAs and Transcriptomes in Aedes Albopictus Midgut Reveals the Differential Expression Profiles of Immune-Related Genes During Dengue Virus Serotype-2 Infection. Insect Sci. 23, 377–385. doi: 10.1111/1744-7917.12339
Liu, B., Tian, M., Guo, Q., Ma, L., Zhou, D., Shen, B., et al. (2016). Mir-932 Regulates Pyrethroid Resistance in Culex Pipiens Pallens (Diptera: Culicidae). J. Med. Entomology 53, 1205–1210. doi: 10.1093/jme/tjw083
Liu, Y., Zhou, Y., Wu, J., Zheng, P., Li, Y., Zheng, X., et al. (2015). The Expression Profile of Aedes Albopictus miRNAs Is Altered by Dengue Virus Serotype-2 Infection. Cell Bioscience 5, 16. doi: 10.1186/s13578-015-0009-y
Lucas, K. J., Roy, S., Ha, J., Gervaise, A. L., Kokoza, V. A., Raikhel, A. S. (2015a). MicroRNA-8 Targets the Wingless Signaling Pathway in the Female Mosquito Fat Body to Regulate Reproductive Processes. Proc. Natl. Acad. Sci. U. S. A. 112, 1440–1445. doi: 10.1073/pnas.1424408112
Lucas, K. J., Zhao, B., Roy, S., Gervaise, A. L., Raikhel, A. S. (2015b). Mosquito-Specific microRNA-1890 Targets the Juvenile Hormone-Regulated Serine Protease JHA15 in the Female Mosquito Gut. RNA Biol. 12, 1383–1390. doi: 10.1080/15476286.2015.1101525
Maharaj, P. D., Widen, S. G., Huang, J., Wood, T. G., Thangamani, S. (2015). Discovery of Mosquito Saliva microRNAs During CHIKV Infection. PloS Negl. Trop. Dis. 9, e0003386. doi: 10.1371/journal.pntd.0003386
Ma, K., Li, X., Hu, H., Zhou, D., Sun, Y., Ma, L., et al. (2017). Pyrethroid-Resistance Is Modulated by miR-92a by Targeting CpCPR4 in Culex Pipiens Pallens. Comp. Biochem. Physiol. Part B Biochem. Mol. Biol. 203, 20–24. doi: 10.1016/j.cbpb.2016.09.002
ayoral, J. G., Etebari, K., Hussain, M., Khromykh, A. A., Asgari, S. (2014). Wolbachia Infection Modifies the Profile, Shuttling and Structure of microRNAs in a Mosquito Cell Line. PloS One 9, e96107. doi: 10.1371/journal.pone.0096107
Mead, E. A., Tu, Z. (2008). Cloning, Characterization, and Expression of microRNAs From the Asian Malaria Mosquito, Anopheles Stephensi. BMC Genomics 9, 244. doi: 10.1186/1471-2164-9-244
Meuti, M. E., Bautista-Jimenez, R., Reynolds, J. A. (2018). Evidence That microRNAs Are Part of the Molecular Toolkit Regulating Adult Reproductive Diapause in the Mosquito, Culex Pipiens. PloS One 13, e0203015. doi: 10.1371/journal.pone.0203015
Miesen, P., Ivens, A., Buck, A. H., van Rij, R. P. (2016). Small RNA Profiling in Dengue Virus 2-Infected Aedes Mosquito Cells Reveals Viral piRNAs and Novel Host Mirnas. PloS Negl. Trop. Dis. 10, e0004452. doi: 10.1371/journal.pntd.0004452
Mishra, S., Yadav, T., Rani, V. (2016). Exploring miRNA Based Approaches in Cancer Diagnostics and Therapeutics. Crit. Rev. Oncol. Hematol. 98, 12–23. doi: 10.1016/j.critrevonc.2015.10.003
Nouzova, M., Etebari, K., Noriega, F. G., Asgari, S. (2018). A Comparative Analysis of Corpora Allata-Corpora Cardiaca microRNA Repertoires Revealed Significant Changes During Mosquito Metamorphosis. Insect Biochem. Mol. Biol. 96, 10–18. doi: 10.1016/j.ibmb.2018.03.007
Osei-Amo, S., Hussain, M., O’Neill, S. L., Asgari, S. (2012). Wolbachia-Induced aae-miR-12 miRNA Negatively Regulates the Expression of MCT1 and MCM6 Genes in Wolbachia-infected Mosquito Cell Line. PloS One 7, e50049. doi: 10.1371/journal.pone.0050049
Puthiyakunnon, S., Yao, Y., Li, Y., Gu, J., Peng, H., Chen, X. (2013). Functional Characterization of Three MicroRNAs of the Asian Tiger Mosquito, Aedes Albopictus. Parasites Vectors 6, 230. doi: 10.1186/1756-3305-6-230
Qu, Z., Bendena, W. G., Nong, W., Siggens, K. W., Noriega, F. G., Kai, Z. P., et al. (2017). MicroRNAs Regulate the Sesquiterpenoid Hormonal Pathway in Drosophila and Other Arthropods. Proc. Biol. Sci. 284, 1869. doi: 10.1098/rspb.2017.1827
Saldana, M. A., Etebari, K., Hart, C. E., Widen, S. G., Wood, T. G., Thangamani, S., et al. (2017). Zika Virus Alters the microRNA Expression Profile and Elicits an RNAi Response in Aedes Aegypti Mosquitoes. PloS Negl. Trop. Dis. 11, e0005760. doi: 10.1371/journal.pntd.0005760
Shrinet, J., Jain, S., Jain, J., Bhatnagar, R. K., Sunil, S. (2014). Next Generation Sequencing Reveals Regulation of Distinct Aedes microRNAs During Chikungunya Virus Development. PloS Negl. Trop. Dis. 8, e2616. doi: 10.1371/journal.pntd.0002616
Skalsky, R. L., Vanlandingham, D. L., Scholle, F., Higgs, S., Cullen, B. R. (2010). Identification of microRNAs Expressed in Two Mosquito Vectors, Aedes Albopictus and Culex Quinquefasciatus. BMC Genomics 11, 119. doi: 10.1186/1471-2164-11-119
Slonchak, A., Hussain, M., Torres, S., Asgari, S., Khromykh, A. A. (2014). Expression of Mosquito Microrna Aae-miR-2940-5p Is Downregulated in Response to West Nile Virus Infection to Restrict Viral Replication. J. Virol. 88, 8457–8467. doi: 10.1128/JVI.00317-14
Slonchak, A., Shannon, R. P., Pali, G., Khromykh, A. A. (2015). Human MicroRNA Mir-532-5p Exhibits Antiviral Activity Against West Nile Virus Via Suppression of Host Genes SESTD1 and TAB3 Required for Virus Replication. J. Virol. 90, 2388–2402. doi: 10.1128/JVI.02608-15
Smith, J. L., Jeng, S., McWeeney, S. K., Hirsch, A. J. (2017). A MicroRNA Screen Identifies the Wnt Signaling Pathway as a Regulator of the Interferon Response During Flavivirus Infection. J. Virol. 91, 8. doi: 10.1128/JVI.02388-16
Su, J., Li, C., Zhang, Y., Yan, T., Zhu, X., Zhao, M., et al. (2017). Identification of microRNAs Expressed in the Midgut of Aedes Albopictus During Dengue Infection. Parasites Vectors 10, 63. doi: 10.1186/s13071-017-1966-2
Su, J., Wang, G., Li, C., Xing, D., Yan, T., Zhu, X., et al. (2019). Screening for Differentially Expressed miRNAs in Aedes Albopictus (Diptera: Culicidae) Exposed to DENV-2 and Their Effect on Replication of DENV-2 in C6/36 Cells. Parasites Vectors 12, 44. doi: 10.1186/s13071-018-3261-2
Tsetsarkin, K. A., Liu, G., Kenney, H., Bustos-Arriaga, J., Hanson, C. T., Whitehead, S. S., et al. (2015). Dual miRNA Targeting Restricts Host Range and Attenuates Neurovirulence of Flaviviruses. PloS Pathog. 11, e1004852. doi: 10.1371/journal.ppat.1004852
Tsetsarkin, K. A., Liu, G., Kenney, H., Hermance, M., Thangamani, S., Pletnev, A. G. (2016a). Concurrent micro-RNA Mediated Silencing of Tick-Borne Flavivirus Replication in Tick Vector and in the Brain of Vertebrate Host. Sci. Rep. 6, 33088. doi: 10.1038/srep33088
Tsetsarkin, K. A., Liu, G., Shen, K., Pletnev, A. G. (2016b). Kissing-Loop Interaction Between 5’ and 3’ Ends of Tick-Borne Langat Virus Genome ‘Bridges the Gap’ Between Mosquito- and Tick-Borne Flaviviruses in Mechanisms of Viral RNA Cyclization: Applications for Virus Attenuation and Vaccine Development. Nucleic Acids Res. 44, 3330–3350. doi: 10.1093/nar/gkw061
Wang, X., Zhang, J., Li, F., Gu, J., He, T., Zhang, X., et al. (2005). MicroRNA Identification Based on Sequence and Structure Alignment. Bioinformatics 21, 3610–3614. doi: 10.1093/bioinformatics/bti562
World Health Organization (2012). Global strategy for dengue prevention and control 2012-2020. WHO. Available at: https://apps.who.int/iris/handle/10665/75303.
Winter, F., Edaye, S., Huttenhofer, A., Brunel, C. (2007). Anopheles Gambiae miRNAs as Actors of Defence Reaction Against Plasmodium Invasion. Nucleic Acids Res. 35, 6953–6962. doi: 10.1093/nar/gkm686
Wu, Z., Xue, Y., Wang, B., Du, J., Jin, Q. (2011). Broad-Spectrum Antiviral Activity of RNA Interference Against Four Genotypes of Japanese Encephalitis Virus Based on Single microRNA Polycistrons. PloS One 6, e26304. doi: 10.1371/journal.pone.0026304
Xie, P. W., Xie, Y., Zhang, X. J., Huang, H., He, L. N., Wang, X. J., et al. (2013). Inhibition of Dengue Virus 2 Replication by Artificial Micrornas Targeting the Conserved Regions. Nucleic Acid Ther. 23, 244–252. doi: 10.1089/nat.2012.0405
Xing, S., Du, J., Gao, S., Tian, Z., Zheng, Y., Liu, G., et al. (2016). Analysis of the miRNA Expression Profile in an Aedes Albopictus Cell Line in Response to Bluetongue Virus Infection. Infection Genet. Evolution: J. Mol. Epidemiol. Evolutionary Genet. Infect. Dis. 39, 74–84. doi: 10.1016/j.meegid.2016.01.012
Xu, T., Zhong, D., Tang, L., Chang, X., Fu, F., Yan, G., et al. (2014). Anopheles Sinensis Mosquito Insecticide Resistance: Comparison of Three Mosquito Sample Collection and Preparation Methods and Mosquito Age in Resistance Measurements. Parasites Vectors 7, 54. doi: 10.1186/1756-3305-7-54
Yang, S., Pei, Y., Li, X., Zhao, S., Zhu, M., Zhao, A. (2016). miR-124 Attenuates Japanese Encephalitis Virus Replication by Targeting DNM2. Virol. J. 13, 105. doi: 10.1186/s12985-016-0562-y
Yan, H., Zhou, Y., Liu, Y., Deng, Y., Chen, X. (2014). miR-252 of the Asian Tiger Mosquito Aedes Albopictus Regulates Dengue Virus Replication by Suppressing the Expression of the Dengue Virus Envelope Protein. J. Med. Virol. 86, 1428–1436. doi: 10.1002/jmv.23815
Yekta, S., Shih, I. H., Bartel, D. P. (2004). MicroRNA-directed Cleavage of HOXB8 Mrna. Science 304, 594–596. doi: 10.1126/science.1097434
Yen, P. S., Chen, C. H., Sreenu, V., Kohl, A., Failloux, A. B. (2019). Assessing the Potential Interactions Between Cellular miRNA and Arboviral Genomic RNA in the Yellow Fever Mosquito, Aedes Aegypti. Viruses 11, 6. doi: 10.3390/v11060540
Yen, P. S., James, A., Li, J. C., Chen, C. H., Failloux, A. B. (2018). Synthetic miRNAs Induce Dual Arboviral-Resistance Phenotypes in the Vector Mosquito Aedes Aegypti. Commun. Biol. 1, 11. doi: 10.1038/s42003-017-0011-5
Yen, L. C., Lin, Y. L., Sung, H. H., Liao, J. T., Tsao, C. H., Su, C. M., et al. (2013). Neurovirulent Flavivirus Can be Attenuated in Mice by Incorporation of Neuron-Specific microRNA Recognition Elements Into Viral Genome. Vaccine 31, 5915–5922. doi: 10.1016/j.vaccine.2011.09.102
Zhang, X., Aksoy, E., Girke, T., Raikhel, A. S., Karginov, F. V. (2017). Transcriptome-Wide microRNA and Target Dynamics in the Fat Body During the Gonadotrophic Cycle of Aedes Aegypti. Proc. Natl. Acad. Sci. U. S. A. 114, E1895–E1903. doi: 10.1073/pnas.1701474114
Zhang, G., Hussain, M., Asgari, S. (2014). Regulation of Arginine Methyltransferase 3 by a Wolbachia-Induced microRNA in Aedes Aegypti and Its Effect on Wolbachia and Dengue Virus Replication. Insect Biochem. Mol. Biol. 53, 81–88. doi: 10.1016/j.ibmb.2014.08.003
Zhang, G., Hussain, M., O’Neill, S. L., Asgari, S. (2013). Wolbachia Uses a Host microRNA to Regulate Transcripts of a Methyltransferase, Contributing to Dengue Virus Inhibition in Aedes Aegypti. Proc. Natl. Acad. Sci. U. S. A. 110, 10276–10281. doi: 10.1073/pnas.1303603110
Zhang, Y., Zhao, B., Roy, S., Saha, T. T., Kokoza, V. A., Li, M., et al. (2016). microRNA-309 Targets the Homeobox Gene SIX4 and Controls Ovarian Development in the Mosquito Aedes Aegypti. Proc. Natl. Acad. Sci. U. S. A. 113, E4828–E4836. doi: 10.1073/pnas.1609792113
Keywords: miRNAs, mosquito, Plasmodium, interruption, miRNA-based approach, mosquito-borne diseases
Citation: Xu T-L, Sun Y-W, Feng X-Y, Zhou X-N and Zheng B (2021) Development of miRNA-Based Approaches to Explore the Interruption of Mosquito-Borne Disease Transmission. Front. Cell. Infect. Microbiol. 11:665444. doi: 10.3389/fcimb.2021.665444
Received: 08 February 2021; Accepted: 02 June 2021;
Published: 21 June 2021.
Edited by:
Lubin Jiang, Institut Pasteur of Shanghai (CAS), ChinaReviewed by:
Fabrizio Lombardo, Sapienza University of Rome, ItalyMaria Luisa Simões, Johns Hopkins University, United States
Copyright © 2021 Xu, Sun, Feng, Zhou and Zheng. This is an open-access article distributed under the terms of the Creative Commons Attribution License (CC BY). The use, distribution or reproduction in other forums is permitted, provided the original author(s) and the copyright owner(s) are credited and that the original publication in this journal is cited, in accordance with accepted academic practice. No use, distribution or reproduction is permitted which does not comply with these terms.
*Correspondence: Xiao-Nong Zhou, emhvdXhuMUBjaGluYWNkYy5jbg==; Bin Zheng, Y2RjaXBkemhlbmdiaW5AMTI2LmNvbQ==