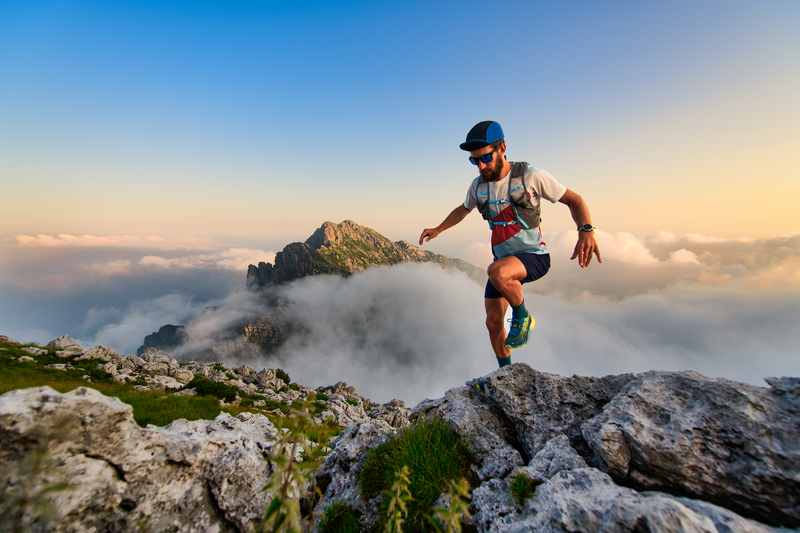
95% of researchers rate our articles as excellent or good
Learn more about the work of our research integrity team to safeguard the quality of each article we publish.
Find out more
REVIEW article
Front. Cell. Infect. Microbiol. , 27 April 2021
Sec. Biofilms
Volume 11 - 2021 | https://doi.org/10.3389/fcimb.2021.660048
Biofilm is a syntrophic association of sessile groups of microbial cells that adhere to biotic and abiotic surfaces with the help of pili and extracellular polymeric substances (EPS). EPSs also prevent penetration of antimicrobials/antibiotics into the sessile groups of cells. Hence, methods and agents to avoid or remove biofilms are urgently needed. Enzymes play important roles in the removal of biofilm in natural environments and may be promising agents for this purpose. As the major component of the EPS is polysaccharide, amylase has inhibited EPS by preventing the adherence of the microbial cells, thus making amylase a suitable antimicrobial agent. On the other hand, salivary amylase binds to amylase-binding protein of plaque-forming Streptococci and initiates the formation of biofilm. This review investigates the contradictory actions and microbe-associated genes of amylases, with emphasis on their structural and functional characteristics.
Biofilm is a consortium of sessile microbial species formed on the surfaces of various natural habitats. Eighty per cent of infections may be caused by biofilm-associated pathogens (Donlan and Costerton, 2002; Lahiri et al., 2021b), and about 90% of the mass of biofilm is composed of extracellular polymeric substance (EPS) (Costerton, 1999). The biofilm stores carbohydrates, proteins, and nucleic acids, which provide nutrients to the developing sessile communities and stabilize indwelling cells. This action also mediates attachment of the sessile cells to the biotic or abiotic surfaces and acts as a scaffold for the enzymes and cells and the attachment of antibiotics (Stewart and Costerton, 2001; Flemming and Wingender, 2010; Mann and Wozniak, 2012).The EPS associated with the biofilm consist of various types of cationic and anionic molecules, such as glycoproteins, glycolipids, and proteins, that can bound with antimicrobial agents, thus providing shelter for microbial species (Nadell et al., 2015). The EPS acts as a coating to protect bacterial cells from antibiotics, thereby enhancing tolerance of the bacteria to the drug. Most of the biofilm matrix consists of extracellular polysaccharides that crosslink with eDNA, thereby stabilizing the structure of the biofilm. The development of resistance to drugs is mainly due to the presence of e-DNA since it can be easily absorbed onto the bacteria, thereby promoting DNA communication (Madsen et al., 2012).
The structural components of the EPS play important roles in the development of the biofilm. DNABII is a structural protein that helps stabilize the biofilm (Devaraj et al., 2015), which is followed by activation of the quorum sensing (QS) pathway, thereby facilitating the development of biofilm (Rasamiravaka et al., 2015). The sessile microcolonies masked within the matrix are concerning, as, compared with planktonic form (Lam et al., 2015), they decrease the permeability of bactericides to enhance drug tolerance. The development of resistance can be due to the rapid exchange of DNA, thereby rendering the antibiotic ineffective by decreasing its antibacterial property (Król et al., 2013; Jennings et al., 2015). The development of resistance reduces the effectiveness of traditional treatments regarding the biofilm, which is a serious concern among health practitioners. The development of EPS results in changes that lead to physiological drift and the development of special environments of oxygen gradient and local acidity (Chang et al., 2015).
The EPS also prevents the penetration of drug molecules to the sessile cells, thus resulting in the development of antimicrobial resistance (Figure 1). Bacterial resistance against various antimicrobial agents, including antibiotics, is an emerging health care crisis (Jana et al., 2017) and has significantly affected the global economy. Most chronic bacterial infections are linked to the development of biofilms, and in-dwelling bacterial colonies are inherently resistance to host immune responses (Patel et al., 2014).
Hypoxia imparts tolerance in the biofilm towards antimicrobials; biofilms exposed to antimicrobials in anaerobic environments were more resistant than were those exposed in aerobic environments (Borriello et al., 2004). Accumulation of colistin-resistant subpopulations within the biofilm determines the toxic region within the biofilm, which in turn indicates the decreased growth of the biofilm and enhanced tolerance towards antimicrobials. Hypoxia reduces the potential of the outer membrane of the bacterial cell, resulting in the development of antibiotic resistance against aminoglycosides. Table 1 lists the genes associated with the development of bacterial resistance.
Resistance to antimicrobial drugs is mediated by EPS, which renders conventional drugs ineffective. This effect has led to the drift from conventional methods of treatment to the use of other agents, such as plant secondary metabolites, antimicrobial peptides, and enzymes, as therapeutic measures (Schachtele et al., 1975; Juntarachot et al., 2020). Enzymes have been effective anti-biofilm agents, and they are environmentally friendly and easily biodegradable (Xavier et al., 2005). Enzymes can inhibit biofilms when the bacterial exopolysaccharides serve as substrate (Brisou, 1995; Sutherland, 1995). Application of suitable enzymes for degrading the structural components of the biofilm matrix will weaken it so that it can be more easily removed by mechanical processes. Since the sugar backbone of the biofilm matrix is composed mainly of carbohydrate residues, carbohydrate-based enzymes, such as amylase, might be used to hydrolyze and thereby denature the biofilm matrix (Lembre et al., 2012).
On the other hand, oral biofilm contains amylase binding proteins, which may indicate that that amylases play a role in establishing the biofilm (Rogers et al., 2001). For example, α-amylase in human saliva binds to α-amylase-binding proteins (ABPs) that are present on bacterial surfaces. Glucose and maltose released from processed starches by salivary amylase are metabolized by oral bacteria to form the biofilm of dental plaque. This process induces oral colonization by streptococci, which leads to the formation of oral biofilm by the plaque-forming bacteria (Nikitkova et al., 2013) The extracellular protein network of AbpA-amylase-Gtf may influence the ecology of oral biofilms, likely during initial phases of colonization. Thus, AbpA-amylase-Gtf may help in co-aggregation and colonization within the oral cavity. The functional significance of amylase binding proteins in oral colonization by Streptococci is important for understanding how salivary components influence oral biofilm formation by these important dental-plaque species. Therefore, the question is raised of whether amylase assists in the formation of dental biofilm or, paradoxically, it can be used as a biofilm inhibitor (Haase et al., 2017; Wu et al., 2020). Amylase seems to be useful for removing biofilm by disintegrating the carbohydrate moiety of the biofilm matrices, but at the same time it can also induce biofilm formation. The present review explores the evidence on the role of amylase in biofilm formation at the molecular level and the mechanisms that may use for eradicating the biofilm.
A biofilm is an assemblage of microbial cells that is irreversibly associated with a surface and is enclosed in a matrix mostly made of polysaccharide material (Donlan and Costerton, 2002). The lower layers of a biofilm contain microbes that are bound together in a polysaccharide matrix with other organic components such as eDNA, proteins, and inorganic materials. The upper layer is a loose amorphous layer extending into the surrounding medium. The fluid layer bordering the biofilm has stationary and dynamic sublayers (Chandki et al., 2011). The biofilm matrix is comprised of microbial consortia with indwelling water channels, assorted cells and extracellular polymers that are composed of glycoproteins, polysaccharides, and proteins (Christensen, 1989; Christensen and Characklis, 1990). The primary colonizers form a biofilm by auto-aggregation (attraction between same species) and co-aggregation (attraction between different species). The attached bacteria multiply and secrete an extracellular matrix, which results in a mature mixed-population biofilm (Chandki et al., 2011).
Genetic adaptation is an important mechanism of survival, which results from genetic mutations and recombination, regulation of expression of the existing genetic material, and acquisition of genetic material. The genomic plasticity or metabolic flexibility of expression within bacterial cells helps them survive rapidly changing environmental conditions and to live in diverse environmental niches (Brooks et al., 2011). Bacterial cells can colonize various parts of the human body by modifying their regulatory and metabolic activities (Yang et al., 2016). Various pathogenic bacteria possess the ability to move from the external environment to the human body by changing the nutrient uptake mechanism and the ability to resist primary and secondary immune defenses (Pickard et al., 2017). Bacterial cells can also alter their gene expression and convert from the planktonic form to the sessile form by enclosing themselves within extracellular polymeric substances (EPS) (Berlanga and Guerrero, 2016). Recent studies in the field of biofilm have focused predominantly on molecular genetics that regulate the formation of biofilms by conversion of planktonic cells to sessile forms (Davey and O’toole, 2000).
Biofilms can resist various types of antimicrobial agents (Lewis, 2001). Although more research is needed to understand the molecular mechanism behind the formation of biofilm, it is known that numerous genes change the metabolomics of bacterial cells, leading to their conversion from planktonic to sessile forms. Biofilms formed on the surface of medical devices include Gram-positive as well as Gram-negative cells. The most common Gram-positive bacteria are Enterococcus faecalis, Streptococcus pyogenes, Staphylococcus mutans, Staphylococcus epidermidis, Bacillus subtilis, and Staphylococcus aureus, whereas Gram-negative bacterial cells are Klebsiella pneumoniae, Escherichia coli, Proteus mirabilis, and Pseudomonas aeruginosa (Kwakman et al., 2006). Apart from bacteria, various groups of filamentous fungi and yeasts can form biofilms on abiotic surfaces, but these biofilms differ from those formed by bacterial cells: In yeast, the attachment is mediated by special types of proteins known as adhesion proteins (Willaert, 2018), which are usually located outside the cell wall and are subjected to epigenetic switching that results in the development of stochastic expression pattern (Verstrepen and Klis, 2006). Biofilm-derived L. pneumophila replicate more in murine macrophages than in planktonic bacteria. The biofilm is the most important determinant of survival and proliferation of bacteria in warm, humid environments.
To remove biofilms from medical devices, coatings made of acylase and α-amylase are used (Ivanova et al., 2015). The enzymes amylase, cellulase, protease, DNase, alginate, and lyase are reported to support removal of biofilms from medical devices (Stiefel et al., 2016). Therefore, enzymes can be considered natural agents for degradation of biofilm.
The composition of the EPS matrix greatly varies structurally and temporally, based on the type of microorganism, availability of substrate, local mechanical shear force, and the environment of the host. The EPS matrix helps in cell-cell adhesion, adhesion to the surface, and aggregation (Flemming and Wingender, 2010), whereas the 3D scaffold helps protect the sessile communities from antimicrobial therapies and provides mechanical stability. EPSs also can reorient the chemical and nutrient gradient and delineate the pathogenic environment; thus, they are important for determining virulence (Hobley et al., 2015; Flemming et al., 2016). This feature makes EPS an important target for therapeutics that act by disaggregating bacterial cells wall to slow the growth of pathogenic bacteria (Gunn et al., 2016). The EPS can be targeted by inhibiting its production or preventing its binding or adhesion to surfaces, thus interfering with biofilm development (Figure 2).
The enzymes that aid in removing existing biofilms can be categorized into six major groups: transferases, oxidoreductases, hydrolases, lyases, and ligases or synthetases (Table 2). The enzyme-associated antifouling activity involves the lysis of cells by degrading cell-membrane components and destabilizing its anchoring to the solid surface. Saccharolytic enzymes produced by certain bacterial cells disintegrate the biofilm, resulting in release of the cells (Gupta et al., 2016). The enzymes prevent the production of adhesives and the formation of EPS, thus preventing the formation of biofilm (Oulahal et al., 2007). Starch is a predominant chemical component in the formation of biofilms (Klein et al., 2009); thus, enzymatic degradation of the polysaccharide results in the removal of the biofilm.
Amylase is an important group of enzymes, which are classified into α, β, γ subtypes, isoamylase, glucoamylase, and others. α and β-amylase have the potential to catalyze the hydrolysis of chitosan (Rokhati et al., 2013) and reduce its molecular weight, which makes it more soluble (Pati et al., 2020a) and thus may lead to diversified applications (Pati et al., 2020b). Since the discovery of the first amylase by Anselme Payen, in 1833 (Krikorian, 1970), many more have been found within living systems that have specific substrates (Guzmàn-Maldonado et al., 1995; Gupta et al., 2003). Amylases can be found in both plant and microbial sources. Based on the mode of action, amylases can be classified into exo-amylases and endo-amylases. Exo-amylases hydrolyze substrates from the non-reducing ends, resulting in shorter end products (Gupta et al., 2003), whereas endo-amylases act on internal glycosidic linkages in a random manner within starch molecules, resulting in oligosaccharides of various lengths (Stütz and Wrodnigg, 2011). Multiple amylases present in L. pneumophila are essential for hydrolyzing polysaccharides into glucose and in helping intracellular proliferation. Amylase also helps trigger pro-inflammatory responses, which further helps prevent bacterial replication (Douglas et al., 1990, Murray et al., 1992, Souza et al., 2020).
α-1,4-glucan-4-glucanohydrolase, EC. 3.2.1.1, which predominantly acts on starch (polysaccharide) as the major substrate, consists of two glucose polymers – amylose and amylopectin. α-amylase helps in the hydrolysis of α-1,4 and α-1,6-glycosidic linkages, which results in the formation of small glucose (monosaccharides) and maltose (disaccharide). α-amylase is essentially a metalloenzyme, which requires metals such Ca2+, for maintaining the stability of the enzyme molecule (Saboury, 2002). Sequence alignment studies have found that α-amylases possess four conserved regions that are also present within the β strands (Møller et al., 2004). The α-amylases are present widely within plants, microorganisms, and higher animals (Kandra, 2003). The end products obtained by the action of this amylase are oligosaccharides of various length of limit dextrin and configurations (Van Der Maarel et al., 2002). The end products also consist of the of branched malto- oligosaccharides possessing 6-8 glucose units that have -1,6 and -1,4, linkages, maltose, and maltotriose (Whitcomb and Lowe, 2007). These amylase enzymes can bind with substrates via catalytic groups that catalyze breakage of the glycosidic bond (Iulek et al., 2000).
β-amylase (E.C.3.2.1.2, α-1,4-D-maltoglucan hydrolase) can hydrolyze starch to β-maltose and β-limit dextrin (Chia et al., 2004). Most of the commercial amylases are obtained from plant sources, but microbial sources are preferred because of lower cost of production, greater stability, easy genetic manipulation, and easier extraction (Ray and Nanda, 1996). Also, fungi have become a source of β-amylases (Ray, 2004).
Glucoamylase (EC 3.2.1.3) successively cleaves each glycosidic starch bond from the non-reducing end to form glucose. α-glucosidase (EC 3.2.1.20) resembles glucoamylase when the α-1,4-linkages are hydrolyzed from the non-reducing ends of alpha-glucans. However, the two enzymes adopt numerous pathways of distinct anomeric arrangements to release glucose. Glucoamylase inverts the α-d-glucose release mechanism, while alpha-glucosidase follows the retention process to generate α-d-glucose (Kumar and Satyanarayana, 2009). Most glucoamylases are multidomain enzymes that consist of a catalytic domain linked by an O-glycosylated linker region to a starch-binding domain (Sauer et al., 2000). A glucoamylase [gamA] gene encodes a eukaryotic-like glucoamylase that is responsible for the degradation of glycogen and starch in bacteria such as Legionella pneumophila.
Salivary amylase is a glucose-polymer enzyme, which cleaves large starch molecules into dextrin and subsequently into smaller malto-oligosaccharides containing α-D-(1,4) linkages, iso-malto-oligosaccharides containing α-D-(1,6) linkages, the trisaccharide maltotriose, and the disaccharide maltose (Jacobsen et al., 1972). Salivary and pancreatic amylases hydrolyze starch (Bonnefond et al., 2017). Human pancreatic amylase cannot cleave the 1,6-linkages nor the terminal glucose residues (Whitcomb and Lowe, 2007). Human amylase is a calcium-containing enzyme comprised of 512 amino acids with a single chain of oligosaccharide having a molecular weight of 57.6 kDa (Whitcomb and Lowe, 2007). The protein is comprised of three domains, namely, A, B and C, of which A is the largest and is mainly barrel shaped with eight superstructures. The B domain is located between A and C and is linked with A via disulphide bonds. The C domain has a sheet-like structure that remains attached to the A domain via a simple polypeptide chain, which appears as an independent domain having no known function. The active site of the amylase is between the carboxyl end of the A and B domains that have the calcium ion and help stabilize the three-dimensional structure (Muralikrishna and Nirmala, 2005) (Figure 3).
Figure 3 (A) Human salivary alpha amylase, (B) human pancreatic amylase (C) alpha amylase, and (D) beta amylase.
The oral cavity contains biofilms of microbial species such as C. albicans, C. glabrata, E. faecalis, S. mutans, V. dispar and F. nucleatum (Berger et al., 2018). Though saliva is rich in amylase, plaque formation has been reported to occur in the presence of the enzyme. Natural selection has dictated the mechanisms working in vivo (not often mimicked in vitro). Amylase has potent antibiofilm activity (Kalpana et al., 2012). However, in vitro studies have shown that α-amylase is a potential antibiofilm agent against biofilm-forming bacterial species such as S. aureus and P. aeruginosa (Lahiri et al., 2021a). Although α-amylase did not have much effect on the biofilm formed by S. epidermidis, it reduced biofilm formation, and it completely inhibited biofilm formation by S. aureus (Bradford, 2011). A 79% reduction in the biofilm was observed in S. aureus when challenged with enzyme for 5 minutes. Increase in the concentration of amylase from 10 mg/mL to 100 mg/mL decreased the biofilm formation from 72% to 90% and inhibited EPS by 82% (Bradford, 2011). Six strains of MRSA had a dose-dependent response to α-amylase of about 92%–97% reduction in biofilm biomass, which is evidence that α-amylase is a potent inhibitor of biofilm formation (Watters et al., 2016).
In a study by Kalpana et al. (2012), the α-amylase obtained from Bacillus subtilis had antibiofilm activity against S. aureus (MRSA), P. aeruginosa, and V. cholerae (Kalpana et al., 2012). The crude enzyme also was effective against S. aureus and P. aeruginosa and degraded the EPS, with efficacy of 51.8% to 73.1%; the purified enzyme reduced biofilm formation by 43.8% to 61.7%. Stronger antibiofilm effect was found in the work of Watters et al. (2016), where human plasma (10%) was supplemented in a culture of S. aureus biofilm; α-amylase was effective against both methicillin-sensitive and methicillin-resistant organisms. The work of Molobela et al. (2010) showed the successful use of α-amylase from Bacillus amyloliquefaciens and glucoamylase from Aspergillus niger on the Gram-negative biofilm-forming bacteria Pseudomonas fluorescence. EPS was reduced by 42.5% in the presence of the enzyme in a challenge of 90 minutes. Microscopic studies were performed to assess the reduction of biofilm and the ability of the enzyme to degrade the EPS and disperse the cells, which resulted in reduction of the biofilm (Molobela et al., 2010). Amylase (glucoamylase and amyloglucosidase units) was successfully used as a hydrolytic enzyme in controlling coaggregation in dental plaque, though it did not significantly alter bacterial viability within the plaque microcosm. (Ledder et al., 2009). Enzymatic inhibition of polysaccharides has been investigated, and α-amylase was found the most efficient enzyme (Divakaran et al., 2011).
The α-amylase produced from A. oryzae inhibits the biofilm formed by S. aureus. β-amylase is an exo-acting carbohydrolase that hydrolyzes the α-1,4-glucosidic linkages of starch only from the non-reducing end of the polysaccharide. The α-amylase can act anywhere on the substrate, thus it tends to act faster than does β-amylase (Toda et al., 1993). The enzyme prevents the surface adherence and helps in the dispersal of the cells, for instance the biofilm formed by Aggregatibacter actinomycetemcomitans when treated with dispersin B and poly-β-1,6-N-acetyl-D-glucoseamine hydrolyzing enzymes (Kaplan et al., 2003a; (Kaplan et al., 2003b; Izano et al., 2008). It has often been observed that a single enzyme is not sufficient to reduce biofilm formation. Therefore, researchers often test combined treatments of biofilms with various enzymes. The combination of levan hydrolase, amylase, and dextrin hydrolase has helped remove the biofilm on inanimate objects (Hatanaka and Sugiura, 1993), and beta-glucanase, protease, and alpha amylase in combination were effective in removing industrial slime (Wiatr, 1990).
The activity of β-amylases in inhibiting the biofilm is less than that of α-amylases. The reason for this difference is that β-amylases can be an exo-acting carbohydrase, which can hydrolyze 1,4-glucosidic linkages of the starch from the non-reducing end. This action opposes the activity of the α-amylases, which can act faster at any position on the substrate (Toda et al., 1993). Although amylases have acclaimed biofilm degrading activity, few reports are available on the biofilm-inducing activity of amylase.
α-amylase is the most abundant enzyme produced primarily from the serous cells of the parotid, submaxillary, sublingual, and minor glands. The reported concentration of amylase in the saliva ranges from 0.04-0.4 mg/ml and comprises about 5% of the total salivary proteins (Jacobsen et al., 1972). The concentration of α-amylase increases with the intake of food (Rohleder et al., 2006). Inui et al. (2019) found that the stimulatory protein responsible for the development of biofilm by Streptococcus anginosus and Streptococcus gordonii was enhanced in the presence of saliva (Inui et al., 2019). Salivary-α-amylase belongs to the family of α-1,4-glucan-4-glucanhydrolase, which catalyzes the α-1,4-glycosidic bonds of glycogen, starch, and other polysaccharides (Scannapieco et al., 1989; Scannapieco et al., 1992). Digestion of starch involves enzymatic degradation, beginning in the oral cavity with the formation of maltose and maltodextrin. The result is an abundance of carbohydrate for nutrition of the oral bacteria. Apart from having hydrolyzing activity, α-amylase can be adsorbed onto the tooth enamel (Al-Hashimi and Levine, 1989; Dufour et al., 2014), where it is a substrate for bacteria (Brown et al., 1999). The prominence of α-amylase in the saliva and the dental pellicle (Jensen et al., 1992; Yao et al., 2001) is a potent precursor for the development of dental biofilm. α-amylase can convert the long chains of malto-oligosaccharides to maltose as their end product, and glycosylated α-amylase is a potent converter of maltotriose into maltose and glucose (Koyama et al., 2000). The amylase-binding site is present in the glycosylated and the non-glycosylated forms of the enzyme (Scannapieco et al., 1989). Salivary α-amylase exists as monomeric (Ragunath et al., 2008) and dimeric (Fisher et al., 2006) forms that possess calcium and chloride ions, which enhance its enzymatic activity. The ability of α-amylase to bind with the bacteria is a calcium-independent mechanism that is independent of hydrolysis. The active site of the enzyme enables the binding of the saccharide hydrolysate of the starch. The enzyme also has several oligosaccharide binding sites, which enhance affinity of α-amylase to the starch granules (Ragunath et al., 2008). The secondary oligosaccharide binding sites are important sites for the bacteria (Ragunath et al., 2008; Spöring et al., 2018). Mutations at the aromatic rings on the secondary oligosaccharide residues decrease the affinity of Streptococcus gordonii for the α-amylases (Ragunath et al., 2008). The α-amylase retains its enzymatic activity even though being attached to the bacterial cells; thus, the site for enzymatic activity needs to be distinct from the bacterial binding site (Scannapieco et al., 1989) (Table 3).
The bacterial surface adhesins, under the influence of salivary agglutinins, help form biofilm (Ahn et al., 2008; Khan et al., 2011). Salivary amylase plays a vital role in the formation of the S. mutans biofilm (Ahn et al., 2008; Klein et al., 2010; Khan et al., 2011). Some conditions result in the expression of virulence factors within S. mutans and favor the development of the oral biofilm: the presence of other organisms and their interaction with S. mutans (Wen et al., 2010), ability of the organisms to survive in aerobic conditions (Ahn et al., 2008), and the availability of oxygen, which is responsible for bringing about variations in the composition of bacterial cell surfaces by the production of autolysins, and activation of the signal transduction system of VicRK. The AtlA autolysin is controlled by the SMu629 gene expression of oxidoreductases. Anaerobic conditions inhibit the expression of genes that control the overproduction of AtlA autolysins, thus inhibiting the formation of the biofilm (Figure 4). Pilus biogenesis gene PilC can be bound to salivary α-amylase by its multiple salivary components (Figure 5).
Figure 5 The contribution/role of Streptococcus mutans in the process of the formation of biofilms (Zhu et al., 2018).
Amylase-binding Streptococci (ABS) express different proteins ranging from 20-87 kDa which bind salivary a-amylase in vitro (Gwynn and Douglas, 1994; Brown et al., 1999; Haase et al., 2017). The heterogeneity of these proteins varies from species to species with some expressing more than one ABP. It has been observed that lower molecular weight ABPs range from 20-36 kDa, whereas higher molecular weight ABPs range from 82-87 kDa. BLAST searches terminal sequences obtained from several ABPs identified AbpA, AbpB, AbpC and several unique ABPs in the NCBI database (Haase et al., 2017). The most predominantly studied ABPs are AbpA (20 kDa) and AbpB (82 kDa) from S. gordonii (Chaudhuri et al., 2008). Amylase-binding protein C (AbpC) obtained from S. mitis which is about 36 kDa was cloned and sequenced.
Although it is able to bind salivary a-amylase, sequence analysis showed no homology to AbpA or AbpB (Vorrasi et al., 2010). Further in silico analysis suggested homology with choline-binding proteins (Haase et al., 2017). Alignment and phylogenetic analysis found that ABPs cluster into at least six phylogenetic groups with no evidence that one group evolved from another (Haase et al., 2017).
AbpA is the most studied ABP. Obtained from S. gordonii, it is about 20kDa and is an externally located and cell wall-associated target protein that is expressed maximally at the mid-log phase of bacterial growth (Brown et al., 1999). Abp A is an essential receptor for binding of α-amylase; inactivation of it eliminates the α-amylase binding capacity of the bacterium (Rogers et al., 2001). Abp A is located on the surface of the cells, as revealed by immunogold electron microscopy (Scannapieco et al., 1992). Cells in the logarithmic phase can bind α-amylase better than can those in the stationary phase; this feature predicts that the receptor is mainly present in the nascent cell wall and is shed into the supernatant as the cell matures. The electron microscopic studies also indicated that binding of the α-amylase does not change the morphology of the bacterial cells or perturb the cell surface (Scannapieco et al., 1992). Studies of the biofilm-forming genes of S. gordonii by Tn 916 mutagenesis revealed that abpA is the potential biofilm-forming gene (Loo et al., 2000; Costa et al., 2020). Other research revealed that the absence of AbpA in S. gordonii impaired biofilm formation on saliva coated flow cells (Rogers et al., 2001). S. gordonii and other ABS are important in the formation of oral biofilm by metabolism of the dietary starch and delivery of nutrients to the non-ABS species in the biofilm. Thus, this type of interaction makes ABS a competitor to the pathogenic species of bacterial cells. Studies performed with the abpA mutant strain, with the help of pathogen-free Osborne-Mendel rats, yielded results contradictory to those of the in vitro studies. The abpA mutant strains reportedly colonize on the tooth surface better than do the wild type, especially when the rats are provided a starch diet (Tanzer et al., 2003). The expression of the glucosyltransferase G, which is one of the potent enzymes promoting the formation of biofilm, was found greater in the abpA mutant strain (Tanzer et al., 2003). Chaudhuri et al. (2007) have reported that glucosyltransferase G forms a complex with AbpA and salivary amylase to form biofilm by S. gordonii. The complex enhances the enzymatic activity of glucosyltransferase G and salivary amylase (Chaudhuri et al., 2007) and the activity of amylase in S. mutans.
AbpB, with a molecular weight of 82 kDa protein, is co-precipitated with α-amylase, AbpA, and glucosyltransferase G. Although AbpB has the ability to bind α-amylase, as confirmed by Western blot studies (Chaudhuri et al., 2008), it does not have homology with AbpA or AbpC. AbpB, however, has homology with bacterial peptidases (Chaudhuri et al., 2008). Abp B shows predominance in hydrolytic activity for Ala-Pro, Gly-Prp and Arg-Pro peptides, which suggests that it restricts enzymatic activities to protein-containing proline-containing residues (Chaudhuri et al., 2008). AbpB has been found to play an important role in the colonization of bacterial cells within the oral cavity, which helps in nutrient acquisition by various pathways. It also helps the bacterial cells present in the oral cavity and dental plaques obtain nutrients from the salivary-proline rich proteins (Chaudhuri et al., 2008).
AbpC is a 36 kDa protein consisting of 292 amino acid residues, with a hydrophobic signal peptide comprising the first 31 N-terminal amino acid residues, as obtained from the supernatant of S. mitis (Vorrasi et al., 2010). AbpA protein does not share homology with AbpA and AbpB but has similarities at the level of amino acids. AbpC is associated with the bacterial cell wall, and it also is a potent receptor of α-amylase.
Biofilm, being the consortia of microbial species and mostly responsible in the development of chronic human diseases, is an important target for therapeutics, as most antimicrobial agents cannot penetrate the EPS matrix of the biofilm (Campoccia et al., 2006). EPS degrading agents – more precisely, natural agents – are being prioritized to manage biofilms. Amylases, which hydrolyze the polysaccharide backbone of EPS, may be useful in the management of biofilms. A combination of enzymes may be used to reduce the accumulation of biofilm on various biotic and abiotic surfaces (Stiefel et al., 2016); the combination would contain amylase, especially α-amylase, β amylase, and amyloglucosidase. High levels of α-amylase, as present in saliva, may enhance or control biofilm formation on dental surfaces with the help of proteins such as AbpA-binding protein. Streptococcus mutans, (which does not bind amylase) builds a potentially more cariogenic biofilm when sucrose is combined with starch because starch hydrolysates may be acceptors during glucan synthesis, altering the branching and the tridimensional structure. Starch by itself is not a “molecular backbone” for those biofilms, but it could enhance their pathogenicity (Klein et al., 2009). Amylase, in combination with other enzymes, may have antibiofilm efficacy against pathogens such as E. coli, S. aureus, and methicillin-resistant Staphylococcus aureus. Hence, the paradoxical actions of amylase raise questions about the exact role of amylase on biofilm in vivo.
All authors contributed to the article and approved the submitted version.
This work was supported by Fundamental Research Grant Scheme (304/PPSK/615059) awarded by Ministry of Higher Education, Malaysia and Odisha Higher Education Program for Excellence and Equity (OHEPEE), Govt of Odisha.
The authors declare that the research was conducted in the absence of any commercial or financial relationships that could be construed as a potential conflict of interest.
Ahn, S. J., Ahn, S. J., Wen, Z. T., Brady, J., Burne, R. A. (2008). Characteristics of Biofilm Formation by Streptococcus Mutans in the Presence of Saliva. Infect. Immun. 76, 4259–4268. doi: 10.1128/IAI.00422-08
Al-Hashimi, I., Levine, M. J. (1989). Characterization of In Vivo Salivary-Derived Enamel Pellicle. Arch. Oral. Biol. 34, 289–295. doi: 10.1016/0003-9969(89)90070-8
Ansari, J. M., Abraham, N. M., Massaro, J., Murphy, K., Smith-Carpenter, J., Fikrig, E. (2017). Anti-Biofilm Activity of a Self-Aggregating Peptide Against Streptococcus Mutans. Front. Microbiol. 8, 488. doi: 10.3389/fmicb.2017.00488
Berger, D., Rakhamimova, A., Pollack, A., Loewy, Z. (2018). Oral Biofilms: Development, Control, and Analysis. High Throughput 7. 24. doi: 10.3390/ht7030024
Berlanga, M., Guerrero, R. (2016). Living Together in Biofilms: The Microbial Cell Factory and its Biotechnological Implications. Microb. Cell Fact. 15, 165. doi: 10.1186/s12934-016-0569-5
Bonnefond, A., Yengo, L., Dechaume, A., Canouil, M., Castelain, M., Roger, E., et al. (2017). Relationship Between Salivary/Pancreatic Amylase and Body Mass Index: A Systems Biology Approach. BMC Med. 15, 37. doi: 10.1186/s12916-017-0784-x
Borriello, G., Werner, E., Roe, F., Kim, A. M., Ehrlich, G. D., Stewart, P. S. (2004). Oxygen Limitation Contributes to Antibiotic Tolerance of Pseudomonas Aeruginosa in Biofilms. Antimicrob. Agents Chemother. 48, 2659–2664. doi: 10.1128/AAC.48.7.2659-2664.2004
Bradford, C. (2011). The Use of Commercially Available Alpha-Amylase Compounds to Inhibit and Remove Staphylococcus Aureus Biofilms. Open Microbiol. J. 5, 21–31. doi: 10.2174/1874285801105010021
Brisou, J. F. (1995). Biofilms, Methods for Enzymatic Release of Microorganisms (New York, USA: CRC Press Inc).
Brooks, A. N., Turkarslan, S., Beer, K. D., Lo, F. Y., Baliga, N. S. (2011). Adaptation of Cells to New Environments. Wiley Interdiscip. Rev. Syst. Biol. Med. 3, 544–561. doi: 10.1002/wsbm.136
Brown, A. E., Rogers, J. D., Haase, E. M., Zelasko, P. M., Scannapieco, F. A. (1999). Prevalence of the Amylase-Binding Protein A Gene (abpA) in Oral Streptococci. J. Clin. Microbiol. 37, 4081–4085. doi: 10.1128/jcm.37.12.4081-4085.1999
Campoccia, D., Montanaro, L., Arciola, C. R. (2006). The Significance of Infection Related to Orthopedic Devices and Issues of Antibiotic Resistance. Biomaterials 27, 2331–2339. doi: 10.1016/j.biomaterials.2005.11.044
Chandki, R., Banthia, P., Banthia, R. (2011). Biofilms: A Microbial Home. J. Indian Soc. Periodontol. 15, 111–114. doi: 10.4103/0972-124X.84377
Chang, Y.-W., Fragkopoulos, A. A., Marquez, S. M., Kim, H. D., Angelini, T. E., Fernández-Nieves, A. (2015). Biofilm Formation in Geometries With Different Surface Curvature and Oxygen Availability. New J. Phys. 17:33017. doi: 10.1088/1367-2630/17/3/033017
Chaudhuri, B., Paju, S., Haase, E. M., Vickerman, M. M., Tanzer, J. M., Scannapieco, F. A. (2008). Amylase-Binding Protein B of Streptococcus Gordonii is an Extracellular Dipeptidyl-Peptidase. Infect. Immun. 76, 4530–4537. doi: 10.1128/IAI.00186-08
Chaudhuri, B., Rojek, J., Vickerman, M. M., Tanzer, J. M., Scannapieco, F. A. (2007). Interaction of Salivary Alpha-Amylase and Amylase-Binding-Protein A (AbpA) of Streptococcus Gordonii With Glucosyltransferase of S. Gordonii and Streptococcus Mutans. BMC Microbiol. 7:60. doi: 10.1186/1471-2180-7-60
Chia, T., Thorneycroft, D., Chapple, A., Messerli, G., Chen, J., Zeeman, S. C., et al. (2004). A Cytosolic Glucosyltransferase is Required for Conversion of Starch to Sucrose in Arabidopsis Leaves At Night. Plant J. 37, 853–863. doi: 10.1111/j.1365-313X.2003.02012.x
Christensen, B. E. (1989). The Role of Extracellular Polysaccharides in Biofilms. J. Biotechnol. 10, 181–202. doi: 10.1016/0168-1656(89)90064-3
Christensen, B., Characklis, W. (1990). “Physical and Chemical Properties of Biofilms,” in Biofilm. Eds. Characklis, W., Marshall, K. (New York: Wiley), 93–130.
Costa, R. C., Souza, J. G. S., Bertolini, M., Retamal-Valdes, B., Feres, M., Barão, V. A. R. (2020). Extracellular Biofilm Matrix Leads to Microbial Dysbiosis and Reduces Biofilm Susceptibility to Antimicrobials on Titanium Biomaterial: An In Vitro and in Situ Study. Clin. Oral. Implants Res. 31, 1173–1186. doi: 10.1111/clr.13663
Costerton, J. W. (1999). Introduction to Biofilm. Int. J. Antimicrob. Agents 11, 217–221. doi: 10.1016/S0924-8579(99)00018-7
Davey, M. E., O’toole, G. A. (2000). Microbial Biofilms: From Ecology to Molecular Genetics. Microbiol. Mol. Biol. Rev. 64, 847–867. doi: 10.1128/mmbr.64.4.847-867.2000
Devaraj, A., Justice, S. S., Bakaletz, L. O., Goodman, S. D. (2015). DNABII Proteins Play a Central Role in UPEC Biofilm Structure. Mol. Microbiol. 96, 1119–1135. doi: 10.1111/mmi.12994
Divakaran, D., Chandran, A., Pratap Chandran, R. (2011). Comparative Study on Production of a-Amylase From Bacillus Licheniformis Strains. Braz. J. Microbiol. 42, 1397–1404. doi: 10.1590/S1517-838220110004000022
Donlan, R. M., Costerton, J. W. (2002). Biofilms: Survival Mechanisms of Clinically Relevant Microorganisms. Clin. Microbiol. Rev. 15, 167–193. doi: 10.1128/CMR.15.2.167-193.2002
Douglas, C. W., Pease, A. A., Whiley, R. A. (1990). Amylase-Binding as a Discriminator Among Oral Streptococci. FEMS Microbiol. Lett. 54, 193–197. doi: 10.1016/0378-1097(90)90281-t
Dufour, Y. S., Fu, X., Hernandez-Nunez, L., Emonet, T. (2014). Limits of Feedback Control in Bacterial Chemotaxis. PloS Comput. Biol. 10, e1003694. doi: 10.1371/journal.pcbi.1003694
Fisher, S. Z., Govindasamy, L., Tu, C., Agbandje-McKenna, M., Silverman, D. N., Rajaniemi, H. J., et al. (2006). Structure of Human Salivary α-Amylase Crystallized in a C-centered Monoclinic Space Group. Acta Crystallogr. Sect. F Struct. Biol. Cryst. Commun. 62, 88–93. doi: 10.1107/S1744309105042491
Flemming, H. C., Wingender, J. (2010). The Biofilm Matrix. Nat. Rev. Microbiol. 8, 623–633. doi: 10.1038/nrmicro2415
Flemming, H.-C., Wingender, J., Szewzyk, U., Steinberg, P., Rice, S. A., Kjelleberg, S. (2016). Biofilms: An Emergent Form of Bacterial Life. Nat. Rev. Microbiol. 14, 563–575. doi: 10.1038/nrmicro.2016.94
Gunn, J. S., Bakaletz, L. O., Wozniak, D. J. (2016). What’s on the Outside Matters: The Role of the Extracellular Polymeric Substance of Gram-Negative Biofilms in Evading Host Immunity and as a Target for Therapeutic Intervention. J. Biol. Chem. 291, 12538–12546. doi: 10.1074/jbc.R115.707547
Gupta, R., Gigras, P., Mohapatra, H., Goswami, V. K., Chauhan, B. (2003). Microbial α-Amylases: A Biotechnological Perspective. Process Biochem. 38, 1599–1616. doi: 10.1016/S0032-9592(03)00053-0
Gupta, P., Sarkar, S., Das, B., Bhattacharjee, S., Tribedi, P. (2016). Biofilm, Pathogenesis and Prevention–a Journey to Break the Wall: A Review. Arch. Microbiol. 198, 1–15. doi: 10.1007/s00203-015-1148-6
Guzmàn-Maldonado, H., Paredes-Lòpez, O., Biliaderis, C. G. (1995). Amylolytic Enzymes and Products Derived From Starch: A Review. Crit. Rev. Food Sci. Nutr. 35, 373–403. doi: 10.1080/10408399509527706
Gwynn, J. P., Douglas, C. W. (1994). Comparison of Amylase-Binding Proteins in Oral Streptococci. FEMS Microbiol. Lett. 124, 373–379. doi: 10.1111/j.1574-6968.1994.tb07311.x
Haase, E. M., Kou, Y., Sabharwal, A., Liao, Y.-C., Lan, T., Lindqvist, C., et al. (2017). Comparative Genomics and Evolution of the Amylase-Binding Proteins of Oral Streptococci. BMC Microbiol. 17, 94. doi: 10.1186/s12866-017-1005-7
Hobley, L., Harkins, C., MacPhee, C. E., Stanley-Wall, N. R. (2015). Giving Structure to the Biofilm Matrix: An Overview of Individual Strategies and Emerging Common Themes. FEMS Microbiol. Rev. 39, 649–669. doi: 10.1093/femsre/fuv015
Inui, T., Palmer, R. J., Shah, N., Li, W., Cisar, J. O., Wu, C. D. (2019). Effect of Mechanically Stimulated Saliva on Initial Human Dental Biofilm Formation. Sci. Rep. 9, 11805. doi: 10.1038/s41598-019-48211-3
Iulek, J., Franco, O. L., Silva, M., Slivinski, C. T., Bloch, C., Rigden, D. J., et al. (2000). Purification, Biochemical Characterisation and Partial Primary Structure of a New α-Amylase Inhibitor From Secale Cereale (Rye). Int. J. Biochem. Cell Biol. 32, 1195–1204. doi: 10.1016/S1357-2725(00)00053-4
Ivanova, K., Fernandes, M. M., Francesko, A., Mendoza, E., Guezguez, J., Burnet, M., et al. (2015). Quorum-Quenching and Matrix-Degrading Enzymes in Multilayer Coatings Synergistically Prevent Bacterial Biofilm Formation on Urinary Catheters. ACS Appl. Mater. Interfaces 7, 27066–27077. doi: 10.1021/acsami.5b09489
Izano, E. A., Sadovskaya, I., Wang, H., Vinogradov, E., Ragunath, C., Ramasubbu, N., et al. (2008). Poly-N-Acetylglucosamine Mediates Biofilm Formation and Detergent Resistance in Aggregatibacter Actinomycetemcomitans. Microb. Pathog. 44, 52–60. doi: 10.1016/j.micpath.2007.08.004
Jacobsen, N., Melvaer, K. L., Hensten-Pettersen, A. (1972). Some Properties of Salivary Amylase: A Survey of the Literature and Some Observations. J. Dent. Res. 51, 381–388. doi: 10.1177/00220345720510022501
Jana, B., Cain, A. K., Doerrler, W. T., Boinett, C. J., Fookes, M. C., Parkhill, J., et al. (2017). The Secondary Resistome of Multidrug-Resistant Klebsiella Pneumoniae. Sci. Rep. 7, 42483. doi: 10.1038/srep42483
Jennings, L. K., Storek, K. M., Ledvina, H. E., Coulon, C., Marmont, L. S., Sadovskaya, I., et al. (2015). Pel is a Cationic Exopolysaccharide That Cross-Links Extracellular DNA in the Pseudomonas Aeruginosa Biofilm Matrix. Proc. Natl. Acad. Sci. 112, 11353–11358. doi: 10.1073/pnas.1503058112
Jensen, J. L., Lamkin, M. S., Oppenheim, F. G. (1992). Adsorption of Human Salivary Proteins to Hydroxyapatite: A Comparison Between Whole Saliva and Glandular Salivary Secretions. J. Dent. Res. 71, 1569–1576. doi: 10.1177/00220345920710090501
Juntarachot, N., Sirilun, S., Kantachote, D., Sittiprapaporn, P., Tongpong, P., Peerajan, S., et al. (2020). Anti-Streptococcus Mutans and Anti-Biofilm Activities of Dextranase and its Encapsulation in Alginate Beads for Application in Toothpaste. PeerJ 8, e10165–e10165. doi: 10.7717/peerj.10165
Kalpana, B., Aarthy, S., Karutha, S. (2012). Antibiofilm Activity of α -Amylase From Bacillus Subtilis S8-18 Against Biofilm Forming Human Bacterial Pathogens. Appl. Biochem. Biotechnol. 167, 1778–794. doi: 10.1007/s12010-011-9526-2
Kandra, L. (2003). α-Amylases of Medical and Industrial Importance. J. Mol. Struct. THEOCHEM 666, 487–498. doi: 10.1016/j.theochem.2003.08.073
Kaplan, J. B., Meyenhofer, M. F., Fine, D. H. (2003a). Biofilm Growth and Detachment of Actinobacillus Actinomycetemcomitans. J. Bacteriol. 185, 1399–404. doi: 10.1128/JB.185.4.1399-1404.2003
Kaplan, J. B., Ragunath, C., Ramasubbu, N., Fine, D. H. (2003b). Detachment of Actinobacillus Actinomycetemcomitans Biofilm Cells by an Endogenous β-Hexosaminidase Activity. J. Bacteriol. 185, 4693–4698. doi: 10.1128/JB.185.16.4693-4698.2003
Khan, A. U., Islam, B., Khan, S. N., Akram, M. (2011). A Proteomic Approach for Exploring Biofilm in Streptococcus Mutans. Bioinformation 5, 440–445. doi: 10.6026/97320630005440
Klein, M. I., DeBaz, L., Agidi, S., Lee, H., Xie, G., Lin, A. H.-M., et al. (2010). Dynamics of Streptococcus Mutans Transcriptome in Response to Starch and Sucrose During Biofilm Development. PloS One 5, e13478. doi: 10.1371/journal.pone.0013478
Klein, M. I., Duarte, S., Xiao, J., Mitra, S., Foster, T. H., Koo, H. (2009). Structural and Molecular Basis of the Role of Starch and Sucrose in Streptococcus Mutans Biofilm Development. Appl. Environ. Microbiol. 75, 837–841. doi: 10.1128/AEM.01299-08
Kohanski, M. A., Dwyer, D. J., Collins, J. J. (2010). How Antibiotics Kill Bacteria: From Targets to Networks. Nat. Rev. Microbiol. 8, 423–435. doi: 10.1038/nrmicro2333
Koyama, I., Komine, S., Yakushijin, M., Hokari, S., Komoda, T. (2000). Glycosylated Salivary -Amylases are Capable of Maltotriose Hydrolysis and Glucose Formation. Comp. Biochem. Physiol. B Biochem. Mol. Biol. 126, 553–560. doi: 10.1016/S0305-0491(00)00225-X
Krikorian, A. D. (1970). The Chemistry of Life. Eight Lectures on the History of Biochemistry. Joseph Needham. Q. Rev. Biol. 314–338. doi: 10.1086/406664
Król, J. E., Wojtowicz, A. J., Rogers, L. M., Heuer, H., Smalla, K., Krone, S. M., et al. (2013). Invasion of E. Coli Biofilms by Antibiotic Resistance Plasmids. Plasmid 70, 110–119. doi: 10.1016/j.plasmid.2013.03.003
Kumar, P., Satyanarayana, T. (2009). Microbial Glucoamylases: Characteristics and Applications. Crit. Rev. Biotechnol. 29, 225–255. doi: 10.1080/07388550903136076
Kwakman, P. H. S., Te Velde, A. A., Vandenbroucke-Grauls, C. M. J. E., Van Deventer, S. J. H., Zaat, S. A. J. (2006). Treatment and Prevention of Staphylococcus Epidermidis Experimental Biomaterial-Associated Infection by Bactericidal Peptide 2. Antimicrob. Agents Chemother. 50, 3977–3983. doi: 10.1128/AAC.00575-06
Lahiri, D., Nag, M., Sarkar, T., Dutta, B., Ray, R. R. (2021a). Antibiofilm Activity of α-Amylase From Bacillus Subtilis and Prediction of the Optimized Conditions for Biofilm Removal by Response Surface Methodology (RSM) and Artificial Neural Network (ANN). Appl. Biochem. Biotechnol. 193, 1–20. doi: 10.1007/s12010-021-03509-9
Lahiri, D., Nag, M., Sheikh, H. I., Sarkar, T., Edinur, H., Siddhartha, P., et al. (2021b). Microbiologically Synthesized Nanoparticles and Their Role in Silencing the Biofilm Signaling Cascade. Front. Microbiol. 12, 636588. doi: 10.3389/fmicb.2021.636588
Lam, K., Schleimer, R., Kern, R. C. (2015). The Etiology and Pathogenesis of Chronic Rhinosinusitis: A Review of Current Hypotheses. Curr. Allergy Asthma Rep. 15, 41. doi: 10.1007/s11882-015-0540-2
Lechner, S., Lewis, K., Bertram, R. (2012). Staphylococcus Aureus Persisters Tolerant to Bactericidal Antibiotics. J. Mol. Microbiol. Biotechnol. 22, 235–244. doi: 10.1159/000342449
Ledder, R. G., Madhwani, T., Sreenivasan, P. K., De Vizio, W., McBain, A. J. (2009). An In Vitro Evaluation of Hydrolytic Enzymes as Dental Plaque Control Agents. J. Med. Microbiol. 58, 482–491. doi: 10.1099/jmm.0.006601-0
Lembre, P., Lorentz, C., Di, P. (2012). “Exopolysaccharides of the Biofilm Matrix: A Complex Biophysical World,” in The Complex World of Polysaccharides. (London, UK: IntechOpen). Available at: https://www.intechopen.com/books/the-complex-world-of-polysaccharides/exopolysaccharides-of-the-biofilm-matrix-a-complex-biophysical-world.
Lequette, Y., Boels, G., Clarisse, M., Faille, C. (2010). Using Enzymes to Remove Biofilms of Bacterial Isolates Sampled in the Food-Industry. Biofouling 26, 421–431. doi: 10.1080/08927011003699535
Lewis, K. (2001). Riddle of Biofilm Resistance. Antimicrob. Agents Chemother. 45, 999–1007. doi: 10.1128/AAC.45.4.999-1007.2001
Liang, X., Liu, B., Zhu, F., Scannapieco, F. A., Haase, E. M., Matthews, S., et al. (2016). A Distinct Sortase SrtB Anchors and Processes a Streptococcal Adhesin AbpA With a Novel Structural Property. Sci. Rep. 6:30966. doi: 10.1038/srep30966
Loo, C. Y., Corliss, D. A., Ganeshkumar, N. (2000). Streptococcus Gordonii Biofilm Formation: Identification of Genes That Code for Biofilm Phenotypes. J. Bacteriol. 182, 1374–1382. doi: 10.1128/JB.182.5.1374-1382.2000
Møller, K., Sharif, M. Z., Olsson, L. (2004). Production of Fungal α-Amylase by Saccharomyces Kluyveri in Glucose-Limited Cultivations. J. Biotechnol. 111, 311–318. doi: 10.1016/j.jbiotec.2004.04.013
Madsen, J. S., Burmølle, M., Hansen, L. H., Sørensen, S. J. (2012). The Interconnection Between Biofilm Formation and Horizontal Gene Transfer. FEMS Immunol. Med. Microbiol. 65, 183–195. doi: 10.1111/j.1574-695X.2012.00960.x
Mann, E. E., Wozniak, D. J. (2012). Pseudomonas Biofilm Matrix Composition and Niche Biology. FEMS Microbiol. Rev. 36, 893–916. doi: 10.1111/j.1574-6976.2011.00322.x
Molobela, P., Cloete, T., Beukes, M. (2010). Protease and Amylase Enzymes for Biofilm Removal and Degradation of Extracellular Polymeric Substances (EPS) Produced by Pseudomonas Fluorescens Bacteria. Afr. J. Microbiol. Res. 4, 1515–1524.
Muralikrishna, G., Nirmala, M. (2005). Cereal α-Amylases—an Overview. Carbohydr. Polym. 60, 163–173. doi: 10.1016/j.carbpol.2004.12.002
Murray, P. A., Prakobphol, A., Lee, T., Hoover, C. I., Fisher, S. J. (1992). Adherence of Oral Streptococci to Salivary Glycoproteins. Infect. Immun. 60, 31–38. doi: 10.1128/IAI.60.1.31-38.1992
Nadell, C. D., Drescher, K., Wingreen, N. S., Bassler, B. L. (2015). Extracellular Matrix Structure Governs Invasion Resistance in Bacterial Biofilms. ISME J. 9, 1700–1709. doi: 10.1038/ismej.2014.246
Nikitkova, A. E., Haase, E. M., Scannapieco, F. A. (2013). Taking the Starch Out of Oral Biofilm Formation: Molecular Basis and Functional Significance of Salivary α-Amylase Binding to Oral Streptococci. Appl. Environ. Microbiol. 79, 416–423. doi: 10.1128/AEM.02581-12
Okahashi, N., Nakata, M., Terao, Y., Isoda, R., Sakurai, A., Sumitomo, T., et al. (2011). Pili of Oral Streptococcus Sanguinis Bind to Salivary Amylase and Promote the Biofilm Formation. Microb. Pathog. 50, 148–154. doi: 10.1016/j.micpath.2011.01.005
Oulahal, N., Martial-Gros, A., Bonneau, M., Blum, L. J. (2007). Removal of Meat Biofilms From Surfaces by Ultrasounds Combined With Enzymes and/or a Chelating Agent. Innov. Food Sci. Emerg. Technol. 8, 192–196. doi: 10.1016/j.ifset.2006.10.001
Patel, I., Patel, V., Thakkar, A., Kothari, V. (2014). Microbial Biofilms: Microbes in Social Mode. Int. J. Agric. Food Res. 3, 34–49. doi: 10.24102/ijafr.v3i2.499
Pati, S., Chatterji, A., Dash, B. P., Nelson, B. R., Sarkar, T., Shahimi, S., et al. (2020a). Structural Characterization and Antioxidant Potential of Chitosan by γ-Irradiation From the Carapace of Horseshoe Crab. Polymers (Basel). 12, 2361. doi: 10.3390/polym12102361
Pati, S., Jena, P., Shahimi, S., Nelson, B. R., Acharya, D., Dash, B. P., et al. (2020b). Characterization Dataset for Pre- and Post-Irradiated Shrimp Waste Chitosan. Data Brief. 32, 106081. doi: 10.1016/j.dib.2020.106081
Pickard, J. M., Zeng, M. Y., Caruso, R., Núñez, G. (2017). Gut Microbiota: Role in Pathogen Colonization, Immune Responses, and Inflammatory Disease. Immunol. Rev. 279, 70–89. doi: 10.1111/imr.12567
Ragunath, C., Manuel, S., Venkataraman, V., Sait, H., Kasinathan, C., Ramasubbu, N. (2008). Probing the Role of Aromatic Residues At the Secondary Saccharide-Binding Sites of Human Salivary -Amylase in Substrate Hydrolysis and Bacterial Binding. J. Mol. Biol. 384, 1232–1248. doi: 10.1016/j.jmb.2008.09.089
Rasamiravaka, T., Labtani, Q., Duez, P., El Jaziri, M. (2015). The Formation of Biofilms by Pseudomonas Aeruginosa: A Review of the Natural and Synthetic Compounds Interfering With Control Mechanisms. BioMed. Res. Int. 2015, 759348. doi: 10.1155/2015/759348
Ray, R. R. (2004). β-Amylases From Various Fungal Strains: A Review. Acta Microbiol. Immunol. Hung. 51, 85–95. doi: 10.1556/AMicr.51.2004.1-2.6
Ray, C. A., Gfell, L. E., Buller, T. L., Gregory, R. L. (1999). Interactions of Streptococcus Mutans Fimbria-Associated Surface Proteins With Salivary Components. Clin. Diagn. Lab. Immunol. 6, 400–404. doi: 10.1128/cdli.6.3.400-404.1999
Ray, R. R., Nanda, G. (1996). Microbial Beta-Amylases: Biosynthesis, Characteristics, and Industrial Applications. Crit. Rev. Microbiol. 22, 181–199. doi: 10.3109/10408419609106459
Ren, W., Cai, R., Yan, W., Lyu, M., Fang, Y., Wang, S. (2018). Purification and Characterization of a Biofilm-Degradable Dextranase From a Marine Bacterium. Mar. Drugs 16, 51. doi: 10.3390/md16020051
Rogers, J. D., Palmer, J., Kolenbrander, P. E., Scannapieco, F. A. (2001). Role of Streptococcus Gordonii Amylase-Binding Protein A in Adhesion to Hydroxyapatite, Starch Metabolism, and Biofilm Formation. Infect. Immun. 69, 7046–7056. doi: 10.1128/IAI.69.11.7046-7056.2001
Rohleder, N., Wolf, J. M., Maldonado, E. F., Kirschbaum, C. (2006). The Psychosocial Stress-Induced Increase in Salivary Alpha-Amylase is Independent of Saliva Flow Rate. Psychophysiology 43, 645–52.x. doi: 10.1111/j.1469-8986.2006.00457.x
Rokhati, N., Widjajanti, P., Pramudono, B., Susanto, H. (2013). Performance Comparison of α- and β-Amylases on Chitosan Hydrolysis. ISRN Chem. Eng. 2013, 186159. doi: 10.1155/2013/186159
Saboury, A. A. (2002). Stability, Activity and Binding Properties Study of α-Amylase Upon Interaction With Ca2+ and Co2+. Biol. Sect. Cell. Mol. Biol. 11, 221–228.
Sauer, J., Sigurskjold, B. W., Christensen, U., Frandsen, T. P., Mirgorodskaya, E., Harrison, M., et al. (2000). Glucoamylase: Structure/Function Relationships, and Protein Engineering. Biochim. Biophys. Acta 1543, 275–293. doi: 10.1016/s0167-4838(00)00232-6
Scannapieco, F. A., Bergey, E. J., Reddy, M. S., Levine, M. J. (1989). Characterization of Salivary Alpha-Amylase Binding to Streptococcus Sanguis. Infect. Immun. 57, 2853–2863. doi: 10.1128/IAI.57.9.2853-2863.1989
Scannapieco, F. A., Haraszthy, G. G., Cho, M. I., Levine, M. J. (1992). Characterization of an Amylase-Binding Component of Streptococcus Gordonii G9B. Infect. Immun. 60, 4726–4733. doi: 10.1128/IAI.60.11.4726-4733.1992
Schachtele, C. F., Staat, R. H., Harlander, S. K. (1975). Dextranases From Oral Bacteria: Inhibition of Water-Insoluble Glucan Production and Adherence to Smooth Surfaces by Streptococcus Mutans. Infect. Immun. 12, 309–317. doi: 10.1128/IAI.12.2.309-317.1975
Souza, J. G. S., Bertolini, M., Thompson, A., Mansfield, J. M., Grassmann, A. A., Maas, K., et al. (2020). Role of Glucosyltransferase R in Biofilm Interactions Between Streptococcus Oralis and Candida Albicans. ISME J. 14, 1207–1222. doi: 10.1038/s41396-020-0608-4
Spoering, A. L., Lewis, K. (2001). Biofilms and Planktonic Cells of Pseudomonas Aeruginosa Have Similar Resistance to Killing by Antimicrobials. J. Bacteriol. 183, 6746–6751. doi: 10.1128/JB.183.23.6746-6751.2001
Spöring, I., Martinez, V. A., Hotz, C., Schwarz-Linek, J., Grady, K. L., Nava-Sedeño, J. M., et al. (2018). Hook Length of the Bacterial Flagellum is Optimized for Maximal Stability of the Flagellar Bundle. PloS Biol. 16, e2006989. doi: 10.1371/journal.pbio.2006989
Stewart, P. S., Costerton, J. W. (2001). Antibiotic Resistance of Bacteria in Biofilms. Lancet 358, 135–138. doi: 10.1016/S0140-6736(01)05321-1
Stiefel, P., Mauerhofer, S., Schneider, J., Maniura-Weber, K., Rosenberg, U., Ren, Q. (2016). Enzymes Enhance Biofilm Removal Efficiency of Cleaners. Antimicrob. Agents Chemother. 60, 3647–3652. doi: 10.1128/AAC.00400-16
Stütz, A. E., Wrodnigg, T. M. (2011). Imino Sugars and Glycosyl Hydrolases: Historical Context, Current Aspects, Emerging Trends. Adv. Carbohydr. Chem. Biochem. 60, 187–298. doi: 10.1016/B978-0-12-385518-3.00004-3
Sutherland, I. W. (1995). Polysaccharide Lyases. FEMS Microbiol. Rev. 16, 323–347. doi: 10.1016/0168-6445(95)00020-D
Tang, X., Flint, S. H., Bennett, R. J., Brooks, J. D. (2010). The Efficacy of Different Cleaners and Sanitisers in Cleaning Biofilms on UF Membranes Used in the Dairy Industry. J. Membr. Sci. 352, 71–75. doi: 10.1016/j.memsci.2010.01.063
Tanzer, J. M., Grant, L., Thompson, A., Li, L., Rogers, J. D., Haase, E. M., et al. (2003). Amylase-Binding Proteins A (AbpA) and B (AbpB) Differentially Affect Colonization of Rats’ Teeth by Streptococcus Gordonii. Microbiology 149, 2653–2660. doi: 10.1099/mic.0.26022-0
Thomas, V. C., Hiromasa, Y., Harms, N., Thurlow, L., Tomich, J., Hancock, L. E. (2009). A Fratricidal Mechanism is Responsible for eDNA Release and Contributes to Biofilm Development of Enterococcus Faecalis. Mol. Microbiol. 72, 1022–1036. doi: 10.1111/j.1365-2958.2009.06703.x
Toda, H., Nitta, Y., Asanami, S., Kim, J. P., Sakiyama, F. (1993). Sweet Potato β-Amylase: Primary Structure and Identification of the Active-Site Glutamyl Residue. Eur. J. Biochem. 216, 25–38. doi: 10.1111/j.1432-1033.1993.tb18112.x
Van Acker, H., Coenye, T. (2016). The Role of Efflux and Physiological Adaptation in Biofilm Tolerance and Resistance. J. Biol. Chem. 291, 12565–12572. doi: 10.1074/jbc.R115.707257
Van Der Maarel, M. J. E. C., Van Der Veen, B., Uitdehaag, J. C. M., Leemhuis, H., Dijkhuizen, L. (2002). Properties and Applications of Starch-Converting Enzymes of the α-Amylase Family. J. Biotechnol. 94, 137–155. doi: 10.1016/S0168-1656(01)00407-2
Verstrepen, K. J., Klis, F. M. (2006). Flocculation, Adhesion and Biofilm Formation in Yeasts. Mol. Microbiol. 60, 5–15. doi: 10.1111/j.1365-2958.2006.05072.x
Vorrasi, J., Chaudhuri, B., Haase, E. M., Scannapieco, F. A. (2010). Identification and Characterization of Amylase-Binding Protein C From Streptococcus Mitis NS51. Mol. Oral. Microbiol. 25, 150–156. doi: 10.1111/j.2041-1014.2009.00554.x
Walker, S. L., Fourgialakis, M., Cerezo, B., Livens, S. (2007). Removal of Microbial Biofilms From Dispense Equipment: The Effect of Enzymatic Pre-Digestion and Detergent Treatment. J. Inst. Brew. 113, 61–66. doi: 10.1002/j.2050-0416.2007.tb00257.x
Watters, C. M., Burton, T., Kirui, D. K., Millenbaugh, N. J. (2016). Enzymatic Degradation of In Vitro Staphylococcus Aureus Biofilms Supplemented With Human Plasma. Infect. Drug Resist. 9, 71–78. doi: 10.2147/IDR.S103101
Webb, J. S., Thompson, L. S., James, S., Charlton, T., Tolker-Nielsen, T., Koch, B., et al. (2003). Cell Death in Pseudomonas Aeruginosa Biofilm Development. J. Bacteriol. 185, 4585–4592. doi: 10.1128/jb.185.15.4585-4592.2003
Wen, Z. T., Yates, D., Ahn, S. J., Burne, R. A. (2010). Biofilm Formation and Virulence Expression by Streptococcus Mutans are Altered When Grown in Dual-Species Model. BMC Microbiol. 10:111. doi: 10.1186/1471-2180-10-111
Whitcomb, D. C., Lowe, M. E. (2007). Human Pancreatic Digestive Enzymes. Dig. Dis. Sci. 52, 1–17. doi: 10.1007/s10620-006-9589-z
Whiteley, M., Bangera, M. G., Bumgarner, R. E., Parsek, M. R., Teitzel, G. M., Lory, S., et al. (2001). Gene Expression in Pseudomonas Aeruginosa Biofilms. Nature. 413, 860–864. doi: 10.1038/35101627
Wiatr, C. L. (1990). Application of Cellulase to Control Industrial Slime. U.S. Patent, U.S.A. 4,936,994.
Willaert, R. G. (2018). Adhesins of Yeasts: Protein Structure and Interactions. J. Fungi 4, 119. doi: 10.3390/jof4040119
Wu, J., Yang, Q., Jiang, X., Fan, Y., Zhang, Y., Huang, R. (2020). Oxyresveratrol Promotes Biofilm Formation, Cell Attachment and Aggregation of Streptococcus Gordonii in the Presence of Sucrose. FEMS Microbiol. Lett. 367, 1–24. doi: 10.1093/femsle/fnaa090
Xavier, J. B., Picioreanu, C., Abdul Rani, S., van Loosdrecht, M. C. M., Stewart, P. S. (2005). Biofilm-Control Strategies Based on Enzymic Disruption of the Extracellular Polymeric Substance Matrix - A Modelling Study. Microbiology 151, 3817–3832. doi: 10.1099/mic.0.28165-0
Yang, D. C., Blair, K. M., Salama, N. R. (2016). Staying in Shape: The Impact of Cell Shape on Bacterial Survival in Diverse Environments. Microbiol. Mol. Biol. Rev. 80, 187–203. doi: 10.1128/MMBR.00031-15
Yao, Y., Grogan, J., Zehnder, M., Lendenmann, U., Nam, B., Wu, Z., et al. (2001). Compositional Analysis of Human Acquired Enamel Pellicle by Mass Spectrometry. Arch. Oral. Biol. 46, 293–303. doi: 10.1016/S0003-9969(00)00134-5
Zanaroli, G., Negroni, A., Calisti, C., Ruzzi, M., Fava, F. (2011). Selection of Commercial Hydrolytic Enzymes With Potential Antifouling Activity in Marine Environments. Enzyme Microb. Technol. 49, 574–579. doi: 10.1016/j.enzmictec.2011.05.008
Zheng, Z., Stewart, P. S. (2004). Growth Limitation of Staphylococcus Epidermidis in Biofilms Contributes to Rifampin Tolerance. Biofilms 1, 31–35. doi: 10.1017/S1479050503001042
Keywords: biofilm, sessile, antibiofilm, amylase, antimicrobial
Citation: Lahiri D, Nag M, Banerjee R, Mukherjee D, Garai S, Sarkar T, Dey A, Sheikh HI, Pathak SK, Edinur HA, Pati S and Ray RR (2021) Amylases: Biofilm Inducer or Biofilm Inhibitor? Front. Cell. Infect. Microbiol. 11:660048. doi: 10.3389/fcimb.2021.660048
Received: 28 January 2021; Accepted: 12 April 2021;
Published: 27 April 2021.
Edited by:
Yann Dufour, Michigan State University, United StatesReviewed by:
Elaine M. Haase, University at Buffalo, United StatesCopyright © 2021 Lahiri, Nag, Banerjee, Mukherjee, Garai, Sarkar, Dey, Sheikh, Pathak, Edinur, Pati and Ray. This is an open-access article distributed under the terms of the Creative Commons Attribution License (CC BY). The use, distribution or reproduction in other forums is permitted, provided the original author(s) and the copyright owner(s) are credited and that the original publication in this journal is cited, in accordance with accepted academic practice. No use, distribution or reproduction is permitted which does not comply with these terms.
*Correspondence: Rina Rani Ray, cmF5cHVtaWNyb0BnbWFpbC5jb20=; Siddhartha Pati, cGF0aXNpZGRoYXJ0aGFAZ21haWwuY29t; Hisham Atan Edinur, ZWRpbnVyQHVzbS5teQ==
†These authors have contributed equally to this work
Disclaimer: All claims expressed in this article are solely those of the authors and do not necessarily represent those of their affiliated organizations, or those of the publisher, the editors and the reviewers. Any product that may be evaluated in this article or claim that may be made by its manufacturer is not guaranteed or endorsed by the publisher.
Research integrity at Frontiers
Learn more about the work of our research integrity team to safeguard the quality of each article we publish.