- Department of Environmental and Occupational Health, Graduate School of Public Health, University of Pittsburgh, Pittsburgh, PA, United States
Bacterial infections contribute to accelerated progression and severity of chronic obstructive pulmonary disease (COPD). Apples have been associated with reduced symptoms of COPD and disease development due to their polyphenolic content. We examined if phloretin, an apple polyphenol, could inhibit bacterial growth and inflammation induced by the main pathogens associated with COPD. Phloretin displayed bacteriostatic and anti-biofilm activity against nontypeable Haemophilus influenzae (NTHi), Moraxella catarrhalis, Streptococcus pneumoniae, and to a lesser extent, Pseudomonas aeruginosa. In vitro, phloretin inhibited NTHi adherence to NCI-H292 cells, a respiratory epithelial cell line. Phloretin also exhibited anti-inflammatory activity in COPD pathogen-induced RAW 264.7 macrophages and human bronchial epithelial cells derived from normal and COPD diseased lungs. In mice, NTHi bacterial load and chemokine (C-X-C motif) ligand 1 (CXCL1), a neutrophil chemoattractant, was attenuated by a diet supplemented with phloretin. Our data suggests that phloretin is a promising antimicrobial and anti-inflammatory nutraceutical for reducing bacterial-induced injury in COPD.
Introduction
Exposure to oxidants formed during infection and environmental exposures, such as tobacco smoke, and the resulting inflammation are major factors in chronic respiratory disease development and progression (Birru and Di, 2012; Barnes, 2020). Diet is a potential factor that could influence respiratory health in response. Past cross-sectional epidemiological studies have found associations between diets rich in fruits and vegetables and protection of the airways against oxidant-mediated damage that leads to COPD (Smit et al., 1999; Tabak et al., 1999; Butland et al., 2000; Tabak et al., 2001). For example, an inverse relationship was observed between baseline consumption of total and solid (i.e. apples and pears) fruit and incidence of chronic lung disease over 25 years in a cohort of 793 men (Miedema et al., 1993). Similarly, daily apple intake has been associated with reduced incidence of cough and phlegm production in a population with chronic respiratory symptoms (Butler et al., 2004). A more recent study by Kaluza et al. found that high total fruit and vegetable consumption (≥5.3 servings/day) as compared with low consumption (<2 servings/day) was associated with a reduced risk of COPD by 40% in current smokers and by 34% in ex-smokers (Kaluza et al., 2017). In longitudinal studies that investigated the association of vitamin C intake with indicators of COPD, no clear association has been observed (Miedema et al., 1993; Butland et al., 2000; Walda et al., 2002). Thus, apple and other fruit polyphenols with antioxidant properties were noted as possible contributors to the beneficial effects found in these studies.
Apple polyphenols include quercetin glycoside, chlorogenic acid, epicatechin, and phloretin. A member of the chalcone family of 1,3-diaryl-2-propen-1-ones, phloretin could be of particular interest as an intervention in COPD due to its broad range of therapeutic targets (Behzad et al., 2017). Phloretin can inhibit the growth and biofilm formation of various bacteria by disrupting energy metabolism (Barreca et al., 2014), nuclear conjugated additions to essential bacterial proteins (Nowakowska, 2007), and suppressing fimbria production (Lee et al., 2011). Phloretin also can inhibit inflammatory mediator secretion induced by bacterial lipopolysaccharide (LPS) and cigarette smoke exposure by reducing activation of the mitogen-activated protein kinase (MAPK) pathway and p65, a component of nuclear transcription factor kappa-B (Huang et al., 2013; Huang et al., 2016; Wang et al., 2018).
In this study, we investigated the potential role of phloretin to protect against the primary respiratory pathogens isolated from persons with COPD. The COPD lung is more prone to airway infections due to impaired mucociliary clearance and lung defense (Sethi, 2010). Pathogenic bacteria accelerate disease progression, mucus overproduction, and lung function decline in COPD and increase frequency of acute exacerbations of COPD (AECOPD), which are sudden worsening of disease symptoms (Patel et al., 2002; Wilkinson et al., 2003; Sethi and Murphy, 2008). AECOPD are associated with irreversible declines in lung function. The primary pathogens isolated from persons experiencing AECOPD are nontypeable Haemophilus influenzae (NTHi), Moraxella catarrhalis (M. catarrhalis), Streptococcus pneumoniae (S. pneumoniae), and Pseudomonas aeruginosa (P. aeruginosa) (Sethi et al., 2002; Sethi and Murphy, 2008).
The antibacterial effects of phloretin have primarily been assessed in cell free systems. Limited in vitro and in vivo work has been performed examining the role of phloretin against pathogen-induced inflammation and bacterial growth. Previously, Lee et al. found that phloretin could reduce Escherichia coli O157:H7 adhesion to and TNF-α-induced monocyte adhesion to HT-29 human colonic epithelial cells (Lee et al., 2011). In addition, in a rat model of colitis induced by trinitrobenzene sulfonic acid (TNBS), phloretin significantly ameliorated colon inflammation and body weight loss (Lee et al., 2011). Similarly, phloretin inhibited Listeria monocytogenes bacterial burden and inflammatory lesions in the livers and spleens of mice and improved their survival (Wang et al., 2017). We examined the effects of phloretin on COPD associated bacterial growth and induced inflammation in cell coculture models and in mouse lungs. Furthermore, to more closely model the human exposure, we administered phloretin orally through supplemented chow. We demonstrate that dietary administration of phloretin is a promising therapeutic to inhibit injury induced by the predominant pathogens associated with COPD.
Materials and Methods
Materials
A complete list of the reagents, cells, and animals and their commercial sources is provided (Supplemental Table 1). Briefly, a clinical isolate of nontypeable Haemophilus influenzae (NTHi) was provided by Dr. Hong Wei Chu (National Jewish Health). Moraxella catarrhalis (M. catarrhalis), Streptococcus pneumoniae (S. pneumoniae), and Pseudomonas aeruginosa (P. aeruginosa) strain PAO1 were obtained from ATCC. LPS from P. aeruginosa was obtained from Sigma-Aldrich. Chocolate agar and sheep blood agar plates, brain heart infusion broth, and Todd-Hewitt broth (THB) were obtained from BD Biosciences. Tryptic soy broth (TSB), tryptic soy agar, and hemin were obtained from MP Biomedicals. β-Nicotinamide adenine dinucleotide hydrate, gentamicin, and DMEM for the biofilm assay were obtained from Sigma-Aldrich. Phloretin was obtained from Cayman Chemicals. Crystal violet stain was obtained from Fisher Scientific. RAW 264.7 and NCI-H292 cells were obtained from ATCC. Normal and COPD human bronchial epithelial (HBE) cells were obtained from MatTek.
Bacterial Strains and Exposure Conditions
NTHi and M. catarrhalis bacterial strains were grown overnight on chocolate agar plates and S. pneumoniae was grown on sheep blood agar plates (37°C, 15h, 5% CO2). PAO1 was grown in TSB (37°C, 15h, with shaking). A portion was subcultured in fresh TSB (37°C, 2h, with shaking), centrifuged (4000 RCF, 5 min), and washed in PBS. Bacterial concentrations were determined by optical density (OD600) and were confirmed by serial dilutions on the appropriate agar plates. A detailed table of bacterial growth conditions for each assay is provided (Supplemental Table 2).
Phloretin Antibacterial Activity Assay
The antibacterial activity of phloretin was examined in a cell-free system by incubating multiple doses of phloretin with bacteria in 96-well plates. Vehicle, phloretin (0.1-1 mM, diluted in PBS), or gentamicin (100 µg/mL) was incubated with control (appropriate bacterial diluent), NTHi (106 colony forming units (CFU), in brain heart infusion broth supplemented with hemin and β-nicotinamide adenine dinucleotide hydrate (sBHI)), M. catarrhalis (105 CFU, in THB), S. pneumoniae (5x105 CFU, in THB supplemented with yeast extract), or PAO1 (105 CFU, in 20% TSB) at a 1:1 v/v. Microtitre plate lids were coated with 0.5% Triton X-100 in ethanol to prevent condensation. Plates were incubated (37°C, 18 h) and the OD570 was determined hourly using a plate reader (BioTek). Prior to a reading, the plate was shaken for 10 min by the plate reader. Following the assays, the bacteria mixtures were serially diluted and plated on their corresponding agar plates to manually count the CFU. Two to three independent trials were conducted with each condition performed in triplicate for each assay (n=6-9 total wells per condition).
Crystal Violet Biofilm Assay
Quantification of crystal violet stained biofilm was determined using a microtiter plate assay, as modified by O’Toole (O’Toole, 2011). Vehicle (0.1% DMSO), 100 µM phloretin, or 100 µg/mL gentamicin in PBS was added to control (PBS), NTHi (106 CFU in sBHI), M. catarrhalis (106 CFU in THB), S. pneumoniae (105 CFU in THB), or PAO1 (106 CFU in DMEM) at a 1:1 v/v in 96-well plates. Plates were incubated under the same conditions (37°C, 16 h, 5% CO2) except for PAO1, which was incubated without CO2. Cells were stained with 0.5% crystal violet (22°C, 20 min with shaking). Plates were dried (22°C, 16 h) and bound dye was released from the cells with methanol and quantified by measuring the OD570. Two independent trials were conducted with each condition performed in triplicate for each assay (n=6 total per condition).
Cell Culture
NCI-H292 cells (a human pulmonary epithelial cell line) or RAW 264.7 cells (a mouse macrophage cell line) were grown in 75 cm2 tissue culture flasks in RPMI-1640 (ATCC) or DMEM (Thermo Fisher Scientific), respectively, supplemented with 10% FBS and penicillin (100 U/mL) and streptomycin (100 µg/mL). HBE cells derived from normal and COPD donors were seeded onto collagen-coated T75 flasks and grown in bronchial epithelial growth medium (Lifeline Cell Technology). For experiments, cells were seeded into 12-well plates (NCI-H292 cells: 5,000 cells/cm2; HBE cells: 5,000 cells/cm2), or 96-well plates (RAW 264.7 cells: 312,500/cm2). Upon confluency, medium was replaced with supplement-free medium prior to experiments (18h). All cultures were maintained at 37°C in 5% CO2/95% air.
Antibacterial Co-Culture Assay
The antibacterial activity of phloretin against NTHi was measured in the presence of NCI-H292 cells using an assay adapted from previous studies (Morey et al., 2011; Euba et al., 2017). Cells were pretreated for 1 h with 0.1 mM phloretin and exposed to NTHi (3x104 CFU/mL, 500 µL) diluted in 20% sBHI in RPMI. After 4 h, the cells were washed twice with PBS and lysed with 0.1% w/v saponin (Alfa Aesar); this was considered the adherent and intracellular collection. Another collection of treated cells were washed twice with PBS and treated with 200 µg/mL gentamicin (1 h) prior to lysing; this was considered the intracellular collection. Two independent trials were conducted with biological replicates performed in triplicate for each assay (n=6 total). Each replicate was serially diluted and plated on chocolate agar and incubated overnight (37°C, 15 h, 5% CO2). The CFU was enumerated to compare the number of bacteria in each collection.
ELISA
The anti-inflammatory activity of phloretin against the COPD pathogens in RAW 264.7 cells was measured. Cells were pretreated 1 h with vehicle (0.1% DMSO) or 0.1 mM phloretin and exposed to NTHi (2x105 CFU/mL, 50 µL), M. catarrhalis (2x105 CFU/mL, 50 µL), S. pneumoniae (2x105 CFU/mL, 50 µL), or PAO1 (2x104 CFU/mL, 50 µL) for 2h. Tumor necrosis factor (TNF) was measured in the supernatant of cells by ELISA (BioLegend). Two independent trials were conducted with biological replicates performed in triplicate (n=6 total) and 2 technical replicates for the ELISA.
Real-Time Quantitative PCR
Normal and COPD HBE cells were pretreated with 0.1 mM phloretin (1 h) and exposed to 500 µL 1.5x107 CFU/mL of NTHi. RNA was extracted from HBE cells after 2 h with TRI Reagent (Sigma-Aldrich) and reverse transcribed into first-strand cDNA (Bio-Rad). RT-qPCR was performed using TaqMan gene expression master mix (Life Technologies) and primers with a thermal cycler (Applied Biosystems) under the following conditions: 50°C (2 min), 95°C (10 min), and 40 cycles of 95°C (15 sec) and 60°C (1 min). Interleukin (IL) 6, IL8, C-C motif chemokine ligand 20 (CCL20), and ribosomal protein (RPL32) transcripts were analyzed. Relative gene expression was calculated using the 2-ΔΔCT method normalized to RPL32. Two independent trials were conducted with biological replicates performed in triplicate (n=6 total), each with 3 technical replicates for the RT-qPCR assays.
Cytotoxicity Assessment
RAW 264.7 cells and HBE cells were pretreated with vehicle (0.1% DMSO) or 0.1 mM phloretin (1 h) and exposed to medium control or bacteria. Lactate dehydrogenase activity was measured using CytoTox-ONE reagent (Promega). Two independent trials were conducted with biological replicates performed in triplicate (n=6 total), with no technical replicates.
Animal Experiments
Animal studies were conducted with approval of the Institutional Animal Use and Care Committee of the University of Pittsburgh. FVB/NJ mice (Jackson Laboratories, female and male, 8 wk) were housed under pathogen-free conditions and maintained on an AIN-93G diet (Envigo) for 3 days prior to the start of the experiment. Mice were then fed an AIN-93G standard diet without or with the addition of 0.157% phloretin ad libitum for 1 week. Diet was administered within 2 weeks upon receipt from the manufacturer to maintain freshness and efficacy. Single colonies of NTHi were incubated in sBHI (37°C, 1.5 h, with shaking). The culture was centrifuged (4000 RCF), washed twice in PBS, and adjusted to an OD600 of 2. Mice were inoculated intratracheally with PBS or 105 CFU of NTHi. The dose was confirmed by plating serial dilutions on chocolate agar plates. After 24 h, mice were anesthetized with sodium pentobarbital. The dorsal aorta was severed and the diaphragm punctured. Bronchoalveolar lavage (BAL) fluid was collected by cannulating the trachea and washing the lungs with 1 mL of PBS, reinserted twice.
To analyze CFU content, lungs were harvested and homogenized in 500 µL PBS. Serial dilutions of the homogenate and BAL fluid were plated on chocolate agar and CFU was counted. Results presented are total CFU in the lung homogenate. BAL was also analyzed for chemokine (C-X-C motif) ligand 1 (CXCL1 aka GRO-1) protein by a Luminex-based assay (Research And Diagnostic Systems).
Statistical Analysis
All cell-free and in vitro data is expressed as mean ± SEM of 2-3 independent trials, with every condition performed in triplicate within each trial. In vivo data is expressed as mean ± SEM of 2 independent trials, with 5-6 mice tested within each trial. Dot plots represent results from the combined trials, with each biological replicate presented as a single dot. Data was analyzed using GraphPad software and significant difference (P<0.05) between groups was assessed by t-test or analysis of variance (ANOVA) with multiple comparisons test (Dunnett’s, Tukey’s).
Results
Phloretin Inhibits Bacterial Growth and Biofilm Formation
A microtiter plate-based antibacterial assay was used to examine the antibacterial capability of phloretin against the COPD pathogens. Bacteria were incubated with vehicle, 0.1-1 mM phloretin, or 100 µg/mL gentamicin (antibiotic control). Phloretin inhibited NTHi, M. catarrhalis, S. pneumoniae, and PAO1 growth (Figure 1A). Inhibition was significant at ≥ 0.1 mM for NTHi, M. catarrhalis, and S. pneumoniae, and ≥ 1 mM for PAO1. Microtitre results were confirmed by manual CFU counts of the wells following the completion of the assays (18 h) and were comparable to the growth curves, where phloretin had antibacterial activity against all of the bacterial strains in a dose-dependent manner, except for S. pneumoniae (Supplemental Table 3). This may be due to a loss of viability of the bacteria. When the CFU was manually enumerated at the beginning of the stationary phase (11 h), phloretin (0.3-1 mM) displayed antibacterial activity against S. pneumoniae (Figure 1A). In another abiotic assay, biofilm biomass was measured by crystal violet staining (OD570). Phloretin (0.1 mM) inhibited biofilm formation of NTHi, M. catarrhalis, and S. pneumoniae but not PAO1 (Figure 1B).
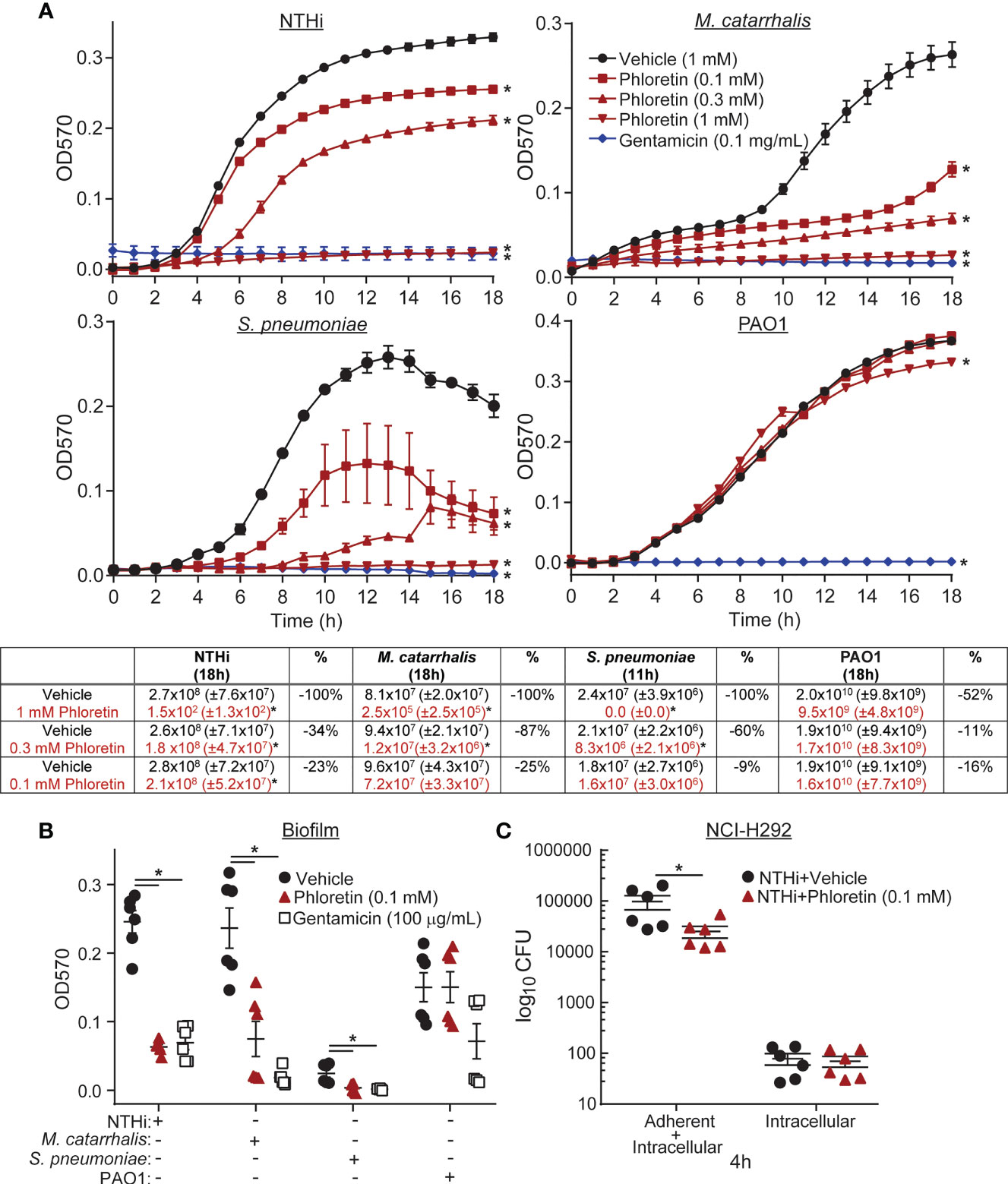
Figure 1 Phloretin inhibits COPD pathogen growth and biofilm formation in abiotic assays and in coculture with lung epithelial cells. (A) Phloretin delays COPD pathogen growth in a dose dependent manner. (Graphs) 106 CFU nontypeable Haemophilus influenzae (NTHi), 105 CFU Moraxella catarrhalis (M. catarrhalis), 5x105 CFU Streptococcus pneumoniae (S. pneumoniae), and 105 CFU Pseudomonas aeruginosa strain PAO1 were grown with vehicle, phloretin (0.1-1 mM), or gentamicin (100 µg/mL) at a 1:1 v/v over 18h. Bacterial growth was assessed by measuring the OD570 hourly. *Significantly different from vehicle+pathogen treated cells (P<0.05) assessed by two-way ANOVA followed by Dunnett’s multiple comparisons test. (Table) Microtitre results were confirmed by plating the treated bacteria from the assays on corresponding agar plates and manually counting the CFU. The percent change (%) in the antibacterial effect of phloretin relative to vehicle is presented. Results are the means ± SEM of 2-3 independent trials with 3 replicates per condition tested within each trial (n=6-9). *Significantly different from vehicle+pathogen treated cells (P<0.05) assessed by paired t-test. (B) Phloretin inhibits COPD pathogen biofilm formation on abiotic plastic surfaces. 106 CFU NTHi, 106 CFU M. catarrhalis, 105 CFU S. pneumoniae, and 106 CFU PAO1 were grown with vehicle, phloretin (0.1 mM), or gentamicin (100 µg/mL) at a 1:1 v/v. Biofilm biomass was quantified by crystal violet staining (OD570). Results are the means ± SEM of 2-3 independent trials with 3 replicates per condition tested within each trial (n=6-9). *Significantly different from vehicle+pathogen treated cells (P<0.05) as determined by one-way ANOVA followed by Dunnett's multiple comparisons test. (C) Phloretin reduces NTHi adhesion to NCI-H292 cells. NCI-H292 cells were pretreated with vehicle or 0.1 mM phloretin and exposed to 500 µL of 3x104 CFU/mL NTHi (4 h, 37°C, 5% CO2). Cells were lysed to collect adherent and intracellular bacteria ("adherent+intracellular") or treated with gentamicin and then lysed to collect intracellular bacteria ("intracellular" collection). Bacteria were enumerated in each collection by plating serial dilutions on chocolate agar plates and counting the CFU. Results are the means ± SEM of 2 independent trials with 3 biological replicates per condition tested within each trial (n=6). *Significantly different from vehicle+NTHi treated cells (P<0.05) as assessed by paired t-test.
The antibacterial effect of phloretin was examined in co-culture with NCI-H292 cells, a human pulmonary epithelial cell line. Over time, phloretin inhibited NTHi adhesion to the cells over 8 h. After 4 h exposure, phloretin reduced adherent bacteria but did not have an impact on bacterial invasion at this time (Figure 1C). Phloretin was previously found to non-cytotoxic to NCI-H292 cells at this dose, as measured by lactate dehydrogenase release and ATP levels (Birru et al., 2020).
Phloretin Reduces Inflammatory Cell Recruitment Induced by COPD Associated Pathogens
LPS is a component of the cell wall of gram-negative bacteria that is recognized and bound by Toll-like receptors (TLRs) on cell surfaces, initiating an innate immune response (Lee and Kim, 2007; Knobloch et al., 2011). NTHi contains short-chain LPS, or lipooligosaccharide (LOS), with structural variant oligosaccharide components attached to its core region (Choi et al., 2014) and can bind to TLR4 in lung epithelial cells (Birru et al., 2020) and macrophages (Lorenz et al., 2005). S. pneumoniae is a gram-positive bacterium that does not express LPS. However, TLR2 and TLR4 recognize lipoteichoic acid, present on its cell wall, and pneumolysin, which it secretes (Sánchez-Tarjuelo et al., 2020). Upon TLR activation, macrophages secrete cytokines to stimulate activation and migration of neutrophils and other immune cells to the site of injury (Wu et al., 2009). To evaluate the anti-inflammatory effects of phloretin, we exposed RAW 264.7 macrophages to the COPD associated pathogens and measured TNF secretion as a marker of inflammation. TNF protein released from the cells into the supernatant was measured at 2 h, prior to the phloretin-induced inhibition of bacterial growth (Figure 1). Each pathogen increased TNF secretion relative to vehicle control (Figure 2A). Phloretin pretreatment inhibited pathogen-induced TNF secretion in RAW 264.7 cells, indicating its anti-inflammatory role. In pathogen exposed cells at 24 h, vehicle treated cells had a significant increase in TNF secretion while levels in phloretin treated cells remained at baseline and were comparable to the vehicle plus control treatment group (data not shown). This may be a result of both the anti-inflammatory and antimicrobial activity of phloretin. At this concentration, phloretin did not induce cytotoxicity in RAW 264.7 cells (Supplemental Figure 2A). Furthermore, the bacteria did not impact cell viability (Supplemental Figure 2A).
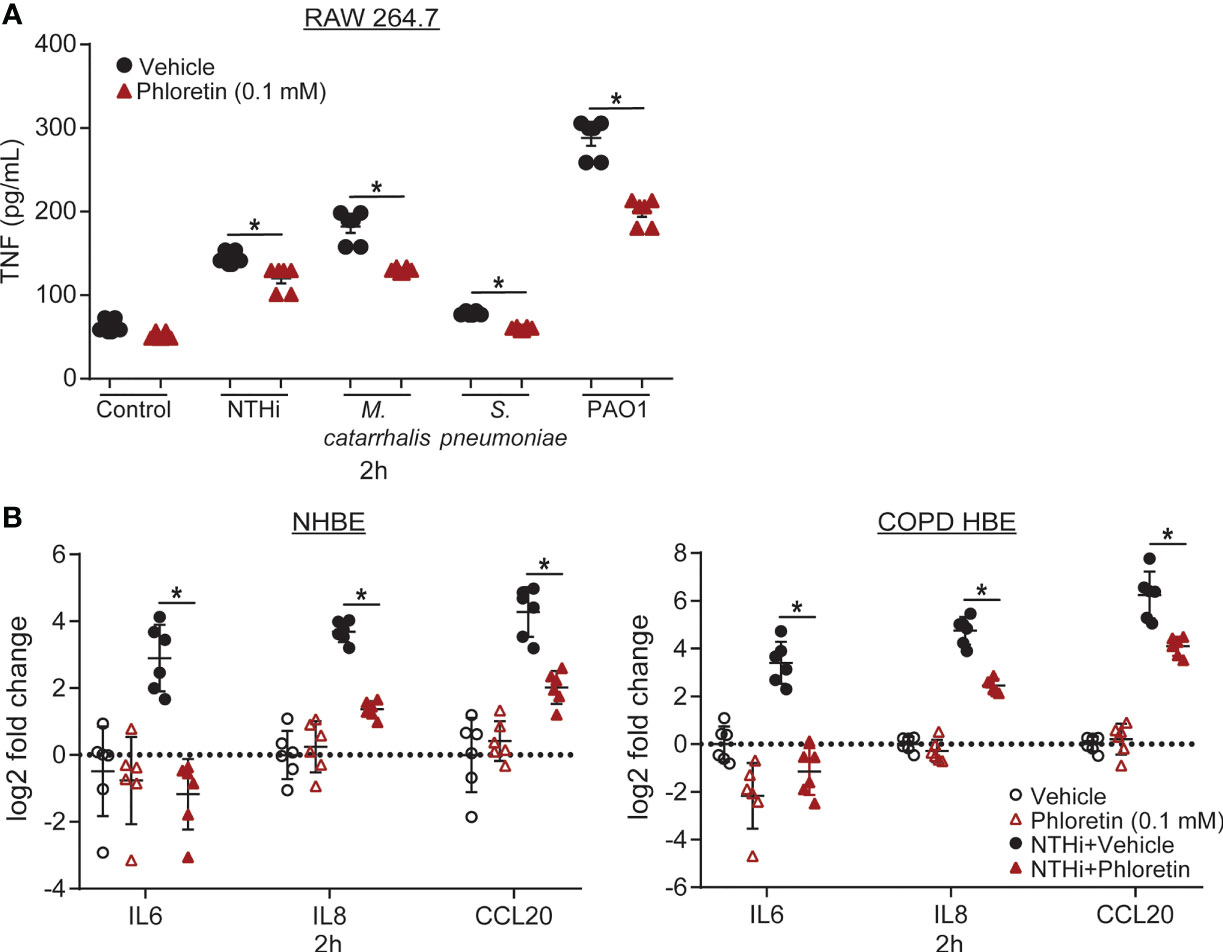
Figure 2 Phloretin exhibits anti-inflammatory activity in COPD pathogen-induced RAW 264.7 cells and nontypeable Haemophilus influenzae (NTHi)-induced human bronchial epithelial (HBE) cells. (A) COPD pathogens significantly increase TNF secretion from RAW 264.7 cells, which phloretin inhibits. RAW 264.7 cells were pretreated with 0.1 mM phloretin (1 h) and exposed to medium control, NTHi (2x105 CFU/mL, 50 µL), Moraxella catarrhalis (M. catarrhalis) (2x105 CFU/mL, 50 µL), Streptococcus pneumoniae (S. pneumoniae) (2x105 CFU/mL, 50 µL), or PAO1 (2x104 CFU/mL, 50 µL) for 2h. TNF was measured in the supernatant by ELISA. Results are the mean ± SEM of 2 independent trials with 3 biological replicates per condition tested within each trial (n=6). P values were assessed by one-way ANOVA followed by Tukey’s multiple comparisons test to compare vehicle to vehicle+pathogen treated cells and to compare vehicle to phloretin treated cells for each condition. *Significantly different from vehicle treated cells (P<0.05). (B) Phloretin inhibits NTHi-induced inflammatory cytokine production in normal and COPD HBE cells. Normal and COPD HBE cells were pretreated with 0.1 mM phloretin (1 h) and exposed to 500 µL 1.5x107 CFU/mL of NTHi (2 h). Interleukin (IL6), IL8, C-C motif chemokine ligand 20 (CCL20), and ribosomal protein L32 (RPL32) transcripts were measured by RT-qPCR. Values are log2 fold change of IL6, IL8, and CCL20 mRNA normalized to RPL32 relative to vehicle control treated cells. Results are the means ± SEM of 2 independent trials with 3 biological replicates per condition tested within each trial (n=6). P values were assessed by one-way ANOVA followed by Tukey’s multiple comparisons test to compare vehicle to vehicle+NTHi treated cells and to compare vehicle to phloretin treated cells for each transcript. *Significantly different from vehicle treated cells (P<0.05).
Phloretin Maintains Anti-Inflammatory Activity in a COPD Model of Infection
To confirm these results in a human model of infection, we used HBE cells derived from normal and COPD subjects. Airway epithelial cells release cytokines in response to pathogens to induce chemotaxis of inflammatory mediators to the site of injury. In HBE cells, we measured IL6, an inflammatory mediator, IL8, a neutrophil chemoattractant, and CCL20, a lymphocyte chemoattractant, which have all been found to be induced by respiratory epithelial cells following NTHi exposure (Clemans et al., 2000; Amatngalim et al., 2017). NTHi increased IL6, IL8, and CCL20 transcript levels in HBE cells. IL6 and CCL20 transcript levels were significantly higher in COPD HBE cells compared to NHBE cells. Phloretin suppressed these biomarkers in both NHBE and COPD HBE cells (Figure 2B). At this concentration, phloretin did not induce cytotoxicity in HBE cells (Supplemental Figure 2B). NTHi had a small but significant effect on cell viability in NHBE cells but not COPD HBE cells (Supplemental Figure 2B).
A Diet Supplemented With Phloretin Inhibits NTHi-Induced Bacterial Growth and Inflammation in Mouse Lung
To examine its antibacterial effects in vivo, mice were supplied a diet supplemented with phloretin (0.157%, 1 wk) and exposed intratracheally to NTHi (24 h). Serial dilutions of the bronchoalveolar lavage fluid and lung tissue homogenate were plated to quantify the bacterial load by enumerating the CFU. NTHi CFU was reduced in the BAL fluid and lung tissue of mice exposed to phloretin (Figure 3A). Previously, we showed that NTHi exposure induced neutrophil infiltration into the lungs, which was inhibited by phloretin treatment (Birru et al., 2020). To strengthen these results, we measured CXCL1, a neutrophil chemoattractant, in BAL fluid from NTHi-treated mice. CXCL1 increased after NTHi exposure and was reduced with a phloretin diet (Figure 3B). The reduction in neutrophils and CXCL1 in mouse lung was in agreement with the inhibition of IL8 in HBE cells. Together these findings indicate that phloretin displays both bacteriostatic and anti-inflammatory activity against COPD associated pathogens in vitro and in vivo.
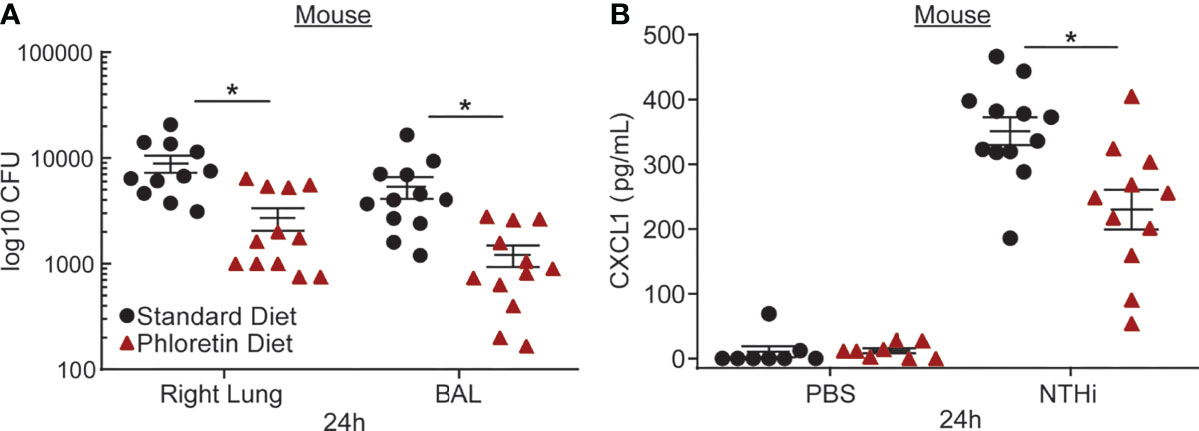
Figure 3 Phloretin reduces nontypeable Haemophilus influenzae (NTHi) burden and inflammation in mouse lungs. (A) Phloretin inhibits NTHi growth in mouse lungs. FVB/NJ mice (8 wks) were supplied with food pellets with or without phloretin supplementation ad libitum (0.157%, 1 wk) and exposed to NTHi (105 CFU, 24 h) intratracheally. Bacterial counts were measured in lung homogenate and BAL at 24 h. Results are the means ± SEM of 2 independent trials of 5-6 mice per condition tested within each trial (n=11-12). *Significantly different from standard diet exposed mice (P<0.05) as determined by unpaired t-test. (B) Phloretin inhibits C-X-C motif chemokine ligand 1 (CXCL1) protein, a neutrophil chemoattractant, induced by NTHi in BAL fluid. CXCL1 was measured in BAL fluid with a Luminex assay. Results are means ± SEM (n=8-12). P values were assessed by one-way ANOVA followed by Tukey’s multiple comparisons test to compare standard diet+PBS to standard diet+NTHi treated mice and to compare standard diet to phloretin diet treated mice. *Significantly different from standard diet treated mice (P<0.05).
Discussion
Pathophysiological changes in COPD include excessive mucus production and impaired mucociliary clearance. These changes contribute to a shift in the respiratory microbiome, increasing the colonization and growth of pathogenic microbiota (Sze et al., 2014). Dietary polyphenols have been proposed to improve COPD symptoms due to their broad antioxidant capacity, and have been shown to improve lung function (measured by forced expiratory volume in one second) and arterial oxygen tension (measured by PaO2) (Domej et al., 2014). In this study, we also demonstrate that phloretin, an apple polyphenol, may provide antibacterial and anti-inflammatory activity in the context of bacterial infections most common in COPD. In practice, consumption of phloretin as a supplement would be a more reasonable to achieve the doses used in our experiments rather than increased apple intake. For example, the mouse dose used in our experiments is equivalent to approximately 200 apples per day (Birru et al., 2020).
In a cell-free system, we found that phloretin demonstrated bacteriostatic and anti-biofilm activity against the COPD pathogens NTHi, M. catarrhalis, S. pneumoniae, and to a lesser extent, PAO1. Gentamicin similarly imparted less antibacterial activity against PAO1 relative to the other bacteria. Compared to other bacteria, PAO1 is particularly resistant to antibiotics due to multidrug efflux transporters, low outer membrane permeability, and production of antibiotic deactivating enzymes (Poole, 2001; Pang et al., 2019). Therefore, antimicrobial agents, such as phloretin and gentamicin, may be less effective against this bacteria.
Using NCI-H292 as a coculture model of infection, we found that phloretin modulated NTHi adhesion. We also demonstrated that NTHi clearance is enhanced in mice supplied a diet supplemented with phloretin, as measured in lung tissue homogenate and BAL fluid. Currently, the primary pharmacological intervention for treating bacterial-induced exacerbations is antibiotic therapy, which may reduce an individual’s frequency of exacerbations (Ramos et al., 2014; Huckle et al., 2018) but overall, may increase the risk of resistant bacterial growth in patients (Huckle et al., 2018). Polyphenols reduce bacterial antibiotic resistance mechanisms, such as drug efflux, and potentiate antibiotic efficacy (Dey et al., 2015; Suriyanarayanan and Sarojini Santhosh, 2015), including compounds in the chalcone structural family in which phloretin belongs (Ferraz et al., 2020; Hellewell and Bhakta, 2020). These results provide the basis for further studies to examine the ability of phloretin to improve bacterial-induced symptoms in COPD or to augment antibiotic therapy and potentiate its efficacy.
Pathogens induce respiratory neutrophil-driven inflammation in persons with COPD, contributing to airway epithelial cell damage and AECOPD (Sethi and Murphy, 2001). We demonstrated that phloretin reduced the pathogen-induced increase in chemokine transcripts in NHBE and COPD HBE cells. These chemokines can amplify inflammation by recruiting neutrophils and lymphocytes to the lung, activating the adaptive immune system to respond to the injury. In mouse lung, NTHi stimulated inflammation, particularly neutrophil influx as measured by CXCL1, which was suppressed by a phloretin diet. Previously, phloretin was found to inhibit IL8/CXCL1 in vitro in LPS/IFNγ exposed human monocytic cells (Jung et al., 2009) and TNF-exposed human keratinocyte cells (Huang et al., 2015). However, the antibacterial effect of the phloretin diet may have reduced the initial inflammatory response to NTHi, therefore also contributing to the CXCL1 inhibition. Airway neutrophilia is a common feature among all subtypes of COPD and contributes to key pathological features of the disease, including airway obstruction, lung function decline, and emphysema (Birru and Di, 2012; Jasper et al., 2019). Therefore the effectiveness of phloretin in reducing bacterial-induced neutrophil mediated inflammation is promising for a therapeutic intervention.
Pathogenic bacteria in COPD is associated with disease morbidity, lung function decline, and AECOPD (Sethi and Murphy, 2001). Our studies demonstrate the antibacterial and anti-inflammatory effects of phloretin in acute models of infection using COPD associated bacteria. Taken together, our results provide a basis for the potential usage of a phloretin isolate as a therapeutic agent against pathological features induced by bacterial infections in COPD. Future studies are warranted to determine the impact of phloretin in in vitro and pre-clinical mouse models of COPD.
Data Availability Statement
The data that support the findings of this study are available from the corresponding author upon reasonable request.
Ethics Statement
The animal study was reviewed and approved by Institutional Animal Use and Care Committee of the University of Pittsburgh.
Author Contributions
All authors contributed to the manuscript and approved the submitted version.
Conflict of Interest
The authors declare that the research was conducted in the absence of any commercial or financial relationships that could be construed as a potential conflict of interest.
Publisher’s Note
All claims expressed in this article are solely those of the authors and do not necessarily represent those of their affiliated organizations, or those of the publisher, the editors and the reviewers. Any product that may be evaluated in this article, or claim that may be made by its manufacturer, is not guaranteed or endorsed by the publisher.
Supplementary Material
The Supplementary Material for this article can be found online at: https://www.frontiersin.org/articles/10.3389/fcimb.2021.652944/full#supplementary-material
Abbreviations
AECOPD, acute exacerbations of chronic obstructive pulmonary disease; CXCL1, chemokine (C-X-C motif) ligand 1; CFU, colony forming unit; COPD, chronic obstructive pulmonary disease; HBE, human bronchial epithelial; IL, interleukin; LPS, lipopolysaccharide; M. catarrhalis, Moraxella catarrhalis; NTHi, nontypeable Haemophilus influenzae; P. aeruginosa, Pseudomonas aeruginosa; sBHI, brain heart infusion broth supplemented with hemin and β-nicotinamide adenine dinucleotide hydrate; S. pneumoniae, Streptococcus pneumoniae; THB, Todd-Hewitt broth; TLR, Toll-like receptor; TNF, tumor necrosis factor; TSB, tryptic soy broth.
References
Amatngalim, G. D., Schrumpf, J. A., Henic, A., Dronkers, E., Verhoosel, R. M., Ordonez, S. R., et al. (2017). Antibacterial Defense of Human Airway Epithelial Cells From Chronic Obstructive Pulmonary Disease Patients Induced by Acute Exposure to Nontypeable Haemophilus Influenzae: Modulation by Cigarette Smoke. J. Innate Immun. 9, 359–374. doi: 10.1159/000455193
Barnes, P. J. (2020). Oxidative Stress-Based Therapeutics in COPD. Redox Biol. 33, 101544. doi: 10.1016/j.redox.2020.101544
Barreca, D., Bellocco, E., Lagana, G., Ginestra, G., Bisignano, C. (2014). Biochemical and Antimicrobial Activity of Phloretin and its Glycosilated Derivatives Present in Apple and Kumquat. Food Chem. 160, 292–297. doi: 10.1016/j.foodchem.2014.03.118
Behzad, S., Sureda, A., Barreca, D., Nabavi, S. F., Rastrelli, L., Nabavi, S. M. (2017). Health Effects of Phloretin: From Chemistry to Medicine. Phytochem. Rev. 16, 527–533. doi: 10.1007/s11101-017-9500-x
Birru, R. L., Bein, K., Wells, H., Bondarchuk, N., Barchowsky, A., Di, Y. P., et al. (2020). Phloretin, an Apple Polyphenol, Inhibits Pathogen-Induced Mucin Overproduction. Mol. Nutr. Food Res. 65, e2000658. doi: 10.1002/mnfr.202000658
Birru, R. L., Di, Y. P. (2012). Pathogenic Mechanism of Second Hand Smoke Induced Inflammation and COPD. Front. Physiol. 3, 348. doi: 10.3389/fphys.2012.00348
Butland, B. K., Fehily, A. M., Elwood, P. C. (2000). Diet, Lung Function, and Lung Function Decline in a Cohort of 2512 Middle Aged Men. Thorax 55, 102–108. doi: 10.1136/thorax.55.2.102
Butler, L. M., Koh, W. P., Lee, H. P., Yu, M. C., London, S. J. (2004). Dietary Fiber and Reduced Cough With Phlegm: A Cohort Study in Singapore. Am. J. Respir. Crit. Care Med. 170, 279–287. doi: 10.1164/rccm.200306-789OC
Choi, J., Cox, A. D., Li, J., Mccready, W., Ulanova, M. (2014). Activation of Innate Immune Responses by Haemophilus Influenzae Lipooligosaccharide. Clin. Vaccine Immunol. 21, 769–776. doi: 10.1128/CVI.00063-14
Clemans, D. L., Bauer, R. J., Hanson, J. A., Hobbs, M. V., St Geme, J. W., 3rd, Marrs, C. F., et al. (2000). Induction of Proinflammatory Cytokines From Human Respiratory Epithelial Cells After Stimulation by Nontypeable Haemophilus Influenzae. Infect. Immun. 68, 4430–4440. doi: 10.1128/IAI.68.8.4430-4440.2000
Dey, D., Ray, R., Hazra, B. (2015). Antimicrobial Activity of Pomegranate Fruit Constituents Against Drug-Resistant Mycobacterium Tuberculosis and β-Lactamase Producing Klebsiella Pneumoniae. Pharm. Biol. 53, 1474–1480. doi: 10.3109/13880209.2014.986687
Domej, W., Oettl, K., Renner, W. (2014). Oxidative Stress and Free Radicals in COPD–implications and Relevance for Treatment. Int. J. Chron Obstruct Pulmon Dis. 9, 1207–1224. doi: 10.2147/COPD.S51226
Euba, B., López-López, N., Rodríguez-Arce, I., Fernández-Calvet, A., Barberán, M., Caturla, N., et al. (2017). Resveratrol Therapeutics Combines Both Antimicrobial and Immunomodulatory Properties Against Respiratory Infection by Nontypeable Haemophilus Influenzae. Sci. Rep. 7, 12860. doi: 10.1038/s41598-017-13034-7
Ferraz, C., Tintino, S. R., Teixeira, A. M. R., Bandeira, P. N., Santos, H. S., Cruz, B. G., et al. (2020). Potentiation of Antibiotic Activity by Chalcone (E)-1-(4’-Aminophenyl)-3-(Furan-2-Yl)-Prop-2-En-1-One Against Gram-Positive and Gram-Negative MDR Strains. Microb. Pathog. 148, 104453. doi: 10.1016/j.micpath.2020.104453
Hellewell, L., Bhakta, S. (2020). Chalcones, Stilbenes and Ketones Have Anti-Infective Properties via Inhibition of Bacterial Drug-Efflux and Consequential Synergism With Antimicrobial Agents. Access Microbiol. 2, acmi000105. doi: 10.1099/acmi.0.000105
Huang, W. C., Chang, W. T., Wu, S. J., Xu, P. Y., Ting, N. C., Liou, C. J. (2013). Phloretin and Phlorizin Promote Lipolysis and Inhibit Inflammation in Mouse 3T3-L1 Cells and in Macrophage-Adipocyte Co-Cultures. Mol. Nutr. Food Res. 57, 1803–1813. doi: 10.1002/mnfr.201300001
Huang, W. C., Dai, Y. W., Peng, H. L., Kang, C. W., Kuo, C. Y., Liou, C. J. (2015). Phloretin Ameliorates Chemokines and ICAM-1 Expression via Blocking of the NF-κb Pathway in the TNF-α-Induced HaCaT Human Keratinocytes. Int. Immunopharmacol 27, 32–37. doi: 10.1016/j.intimp.2015.04.024
Huang, W. C., Lai, C. L., Liang, Y. T., Hung, H. C., Liu, H. C., Liou, C. J. (2016). Phloretin Attenuates LPS-Induced Acute Lung Injury in Mice via Modulation of the NF-KappaB and MAPK Pathways. Int. Immunopharmacol 40, 98–105. doi: 10.1016/j.intimp.2016.08.035
Huckle, A. W., Fairclough, L. C., Todd, I. (2018). Prophylactic Antibiotic Use in COPD and the Potential Anti-Inflammatory Activities of Antibiotics. Respir. Care 63, 609–619. doi: 10.4187/respcare.05943
Jasper, A. E., Mciver, W. J., Sapey, E., Walton, G. M. (2019). Understanding the Role of Neutrophils in Chronic Inflammatory Airway Disease. F1000Res 8, 557. doi: 10.12688/f1000research.18411.1
Jung, M., Triebel, S., Anke, T., Richling, E., Erkel, G. (2009). Influence of Apple Polyphenols on Inflammatory Gene Expression. Mol. Nutr. Food Res. 53, 1263–1280. doi: 10.1002/mnfr.200800575
Kaluza, J., Larsson, S. C., Orsini, N., Linden, A., Wolk, A. (2017). Fruit and Vegetable Consumption and Risk of COPD: A Prospective Cohort Study of Men. Thorax 72, 500–509. doi: 10.1136/thoraxjnl-2015-207851
Knobloch, J., Schild, K., Jungck, D., Urban, K., Müller, K., Schweda, E. K., et al. (2011). The T-Helper Cell Type 1 Immune Response to Gram-Negative Bacterial Infections is Impaired in COPD. Am. J. Respir. Crit. Care Med. 183, 204–214. doi: 10.1164/rccm.201002-0199OC
Lee, M. S., Kim, Y. J. (2007). Signaling Pathways Downstream of Pattern-Recognition Receptors and Their Cross Talk. Annu. Rev. Biochem. 76, 447–480. doi: 10.1146/annurev.biochem.76.060605.122847
Lee, J. H., Regmi, S. C., Kim, J. A., Cho, M. H., Yun, H., Lee, C. S., et al. (2011). Apple Flavonoid Phloretin Inhibits Escherichia Coli O157:H7 Biofilm Formation and Ameliorates Colon Inflammation in Rats. Infect. Immun. 79, 4819–4827. doi: 10.1128/IAI.05580-11
Lorenz, E., Chemotti, D. C., Jiang, A. L., Mcdougal, L. D. (2005). Differential Involvement of Toll-Like Receptors 2 and 4 in the Host Response to Acute Respiratory Infections With Wild-Type and Mutant Haemophilus Influenzae Strains. Infect. Immun. 73, 2075–2082. doi: 10.1128/IAI.73.4.2075-2082.2005
Miedema, I., Feskens, E. J., Heederik, D., Kromhout, D. (1993). Dietary Determinants of Long-Term Incidence of Chronic Nonspecific Lung Diseases. The Zutphen Study. Am. J. Epidemiol. 138, 37–45. doi: 10.1093/oxfordjournals.aje.a116775
Morey, P., Cano, V., Martí-Lliteras, P., López-Gómez, A., Regueiro, V., Saus, C., et al. (2011). Evidence for a non-Replicative Intracellular Stage of Nontypable Haemophilus Influenzae in Epithelial Cells. Microbiol. (Reading) 157, 234–250. doi: 10.1099/mic.0.040451-0
Nowakowska, Z. (2007). A Review of Anti-Infective and Anti-Inflammatory Chalcones. Eur. J. Med. Chem. 42, 125–137. doi: 10.1016/j.ejmech.2006.09.019
O’toole, G. A. (2011). Microtiter Dish Biofilm Formation Assay. J. Vis. Exp. 30 (47), 2437. doi: 10.3791/2437
Pang, Z., Raudonis, R., Glick, B. R., Lin, T. J., Cheng, Z. (2019). Antibiotic Resistance in Pseudomonas Aeruginosa: Mechanisms and Alternative Therapeutic Strategies. Biotechnol. Adv. 37, 177–192. doi: 10.1016/j.biotechadv.2018.11.013
Patel, I. S., Seemungal, T. A., Wilks, M., Lloyd-Owen, S. J., Donaldson, G. C., Wedzicha, J. A. (2002). Relationship Between Bacterial Colonisation and the Frequency, Character, and Severity of COPD Exacerbations. Thorax 57, 759–764. doi: 10.1136/thorax.57.9.759
Poole, K. (2001). Multidrug Efflux Pumps and Antimicrobial Resistance in Pseudomonas Aeruginosa and Related Organisms. J. Mol. Microbiol. Biotechnol. 3, 255–264.
Ramos, F. L., Krahnke, J. S., Kim, V. (2014). Clinical Issues of Mucus Accumulation in COPD. Int. J. Chron Obstruct Pulmon Dis. 9, 139–150. doi: 10.2147/COPD.S38938
Sánchez-Tarjuelo, R., Cortegano, I., Manosalva, J., Rodríguez, M., Ruíz, C., Alía, M., et al. (2020). The TLR4-MyD88 Signaling Axis Regulates Lung Monocyte Differentiation Pathways in Response to Streptococcus Pneumoniae. Front. Immunol. 11, 2120. doi: 10.3389/fimmu.2020.02120
Sethi, S. (2010). Infection as a Comorbidity of COPD. Eur. Respir. J. 35, 1209–1215. doi: 10.1183/09031936.00081409
Sethi, S., Evans, N., Grant, B. J., Murphy, T. F. (2002). New Strains of Bacteria and Exacerbations of Chronic Obstructive Pulmonary Disease. N Engl. J. Med. 347, 465–471. doi: 10.1056/NEJMoa012561
Sethi, S., Murphy, T. F. (2001). Bacterial Infection in Chronic Obstructive Pulmonary Disease in 2000: A State-of-the-Art Review. Clin. Microbiol. Rev. 14, 336–363. doi: 10.1128/CMR.14.2.336-363.2001
Sethi, S., Murphy, T. F. (2008). Infection in the Pathogenesis and Course of Chronic Obstructive Pulmonary Disease. N. Engl. J. Med. 359, 2355–2365. doi: 10.1056/NEJMra0800353
Smit, H. A., Grievink, L., Tabak, C. (1999). Dietary Influences on Chronic Obstructive Lung Disease and Asthma: A Review of the Epidemiological Evidence. Proc. Nutr. Soc. 58, 309–319. doi: 10.1017/S0029665199000427
Suriyanarayanan, B., Sarojini Santhosh, R. (2015). Docking Analysis Insights Quercetin can be a non-Antibiotic Adjuvant by Inhibiting Mmr Drug Efflux Pump in Mycobacterium Sp. And its Homologue EmrE in Escherichia Coli. J. Biomol Struct. Dyn 33, 1819–1834. doi: 10.1080/07391102.2014.974211
Sze, M. A., Hogg, J. C., Sin, D. D. (2014). Bacterial Microbiome of Lungs in COPD. Int. J. Chron Obstruct Pulmon Dis. 9, 229–238. doi: 10.2147/COPD.S38932
Tabak, C., Arts, I. C., Smit, H. A., Heederik, D., Kromhout, D. (2001). Chronic Obstructive Pulmonary Disease and Intake of Catechins, Flavonols, and Flavones: The MORGEN Study. Am. J. Respir. Crit. Care Med. 164, 61–64. doi: 10.1164/ajrccm.164.1.2010025
Tabak, C., Smit, H. A., Räsänen, L., Fidanza, F., Menotti, A., Nissinen, A., et al. (1999). Dietary Factors and Pulmonary Function: A Cross Sectional Study in Middle Aged Men From Three European Countries. Thorax 54, 1021–1026. doi: 10.1136/thx.54.11.1021
Walda, I. C., Tabak, C., Smit, H. A., Räsänen, L., Fidanza, F., Menotti, A., et al. (2002). Diet and 20-Year Chronic Obstructive Pulmonary Disease Mortality in Middle-Aged Men From Three European Countries. Eur. J. Clin. Nutr. 56, 638–643. doi: 10.1038/sj.ejcn.1601370
Wang, J., Liu, B., Teng, Z., Zhou, X., Wang, X., Zhang, B., et al. (2017). Phloretin Attenuates Listeria Monocytogenes Virulence Both In Vitro and In Vivo by Simultaneously Targeting Listeriolysin O and Sortase A. Front. Cell Infect. Microbiol. 7, 9. doi: 10.3389/fcimb.2017.00009
Wang, H., Yang, T., Wang, T., Hao, N., Shen, Y., Wu, Y., et al. (2018). Phloretin Attenuates Mucus Hypersecretion and Airway Inflammation Induced by Cigarette Smoke. Int. Immunopharmacol 55, 112–119. doi: 10.1016/j.intimp.2017.12.009
Wilkinson, T. M., Patel, I. S., Wilks, M., Donaldson, G. C., Wedzicha, J. A. (2003). Airway Bacterial Load and FEV1 Decline in Patients With Chronic Obstructive Pulmonary Disease. Am. J. Respir. Crit. Care Med. 167, 1090–1095. doi: 10.1164/rccm.200210-1179OC
Wu, T. T., Chen, T. L., Chen, R. M. (2009). Lipopolysaccharide Triggers Macrophage Activation of Inflammatory Cytokine Expression, Chemotaxis, Phagocytosis, and Oxidative Ability via a Toll-Like Receptor 4-Dependent Pathway: Validated by RNA Interference. Toxicol. Lett. 191, 195–202. doi: 10.1016/j.toxlet.2009.08.025
Keywords: bacteria, phloretin, antibacterial, anti-inflammatory, neutrophil, COPD
Citation: Birru RL, Bein K, Bondarchuk N, Wells H, Lin Q, Di YP and Leikauf GD (2021) Antimicrobial and Anti-Inflammatory Activity of Apple Polyphenol Phloretin on Respiratory Pathogens Associated With Chronic Obstructive Pulmonary Disease. Front. Cell. Infect. Microbiol. 11:652944. doi: 10.3389/fcimb.2021.652944
Received: 13 January 2021; Accepted: 11 October 2021;
Published: 22 November 2021.
Edited by:
Annette C. Vergunst, U1047, Bacterial Virulence and Chronic Infections (INSERM), FranceReviewed by:
Cristina Cigana, Division of Immunology, Transplantation and Infectious Diseases, San Raffaele Scientific Institute (IRCCS), ItalyBegoña Euba, Institute of Agrobiotechnology, Spanish National Research Council (CSIC), Spain
Fabienne Gally, National Jewish Health, United States
Copyright © 2021 Birru, Bein, Bondarchuk, Wells, Lin, Di and Leikauf. This is an open-access article distributed under the terms of the Creative Commons Attribution License (CC BY). The use, distribution or reproduction in other forums is permitted, provided the original author(s) and the copyright owner(s) are credited and that the original publication in this journal is cited, in accordance with accepted academic practice. No use, distribution or reproduction is permitted which does not comply with these terms.
*Correspondence: George D. Leikauf, Z2xlaWthdWZAcGl0dC5lZHU=