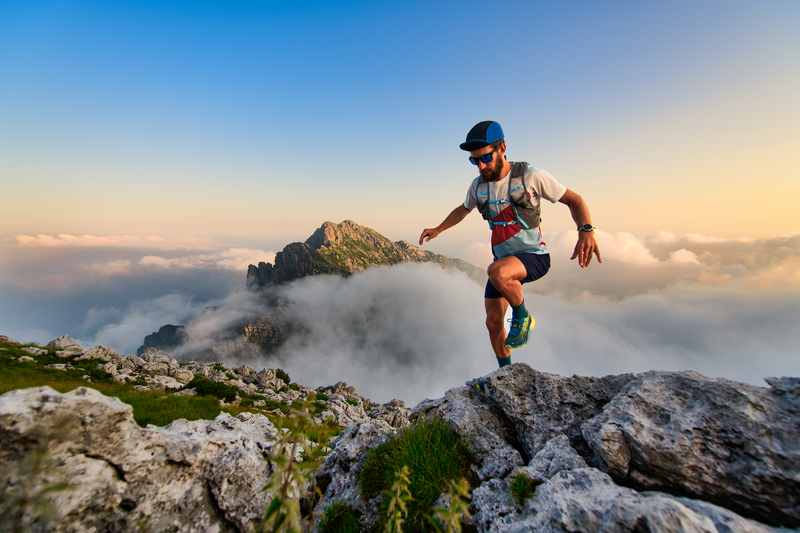
95% of researchers rate our articles as excellent or good
Learn more about the work of our research integrity team to safeguard the quality of each article we publish.
Find out more
ORIGINAL RESEARCH article
Front. Cell. Infect. Microbiol. , 25 March 2021
Sec. Parasite and Host
Volume 11 - 2021 | https://doi.org/10.3389/fcimb.2021.591868
This article is part of the Research Topic Advances in the Molecular Biology of Trypanosomatid Pathogens View all 14 articles
The intracellular protozoan parasites of the Leishmania genus are responsible for Leishmaniases, vector borne diseases with a wide range of clinical manifestations. Leishmania (L.) donovani causes visceral leishmaniasis (kala azar), the most severe of these diseases. Along their biological cycle, Leishmania parasites undergo distinct developmental transitions including metacyclogenesis and differentiation of metacyclic promastigotes (MPs) to amastigotes. Metacyclogenesis inside the phlebotomine sandfly host’s midgut converts the procyclic dividing promastigotes to non-dividing infective MPs eventually injected into the skin of mammalian hosts and phagocytosed by macrophages where the MPs are converted inside modified phagolysosomes to the intracellular amastigotes. These developmental transitions involve dramatic changes in cell size and shape and reformatting of the flagellum requiring thus membrane and cytoskeleton remodeling in which phosphoinositide (PI) signaling and metabolism must play central roles. This study reports on the LDBPK_220120.1 gene, the L. donovani ortholog of LmjF.22.0250 from L. major that encodes a phosphatase from the “Atypical Lipid Phosphatases” (ALPs) enzyme family. We confirmed the expression of the LDBPK_220120.1 gene product in both L. donovani promastigotes and axenic amastigotes and showed that it behaves in vitro as a Dual Specificity P-Tyr and monophosphorylated [PI(3)P and PI(4)P] PI phosphatase and therefore named it LdTyrPIP_22 (Leishmaniad onovani Tyrosine PI Phosphatase, gene locus at chromosome 22). By immunofluorescence confocal microscopy we localized the LdTyrPIP_22 in several intracellular sites in the cell body of L. donovani promastigotes and amastigotes and in the flagellum. A temperature and pH shift from 25°C to 37°C and from pH 7 to 5.5, induced a pronounced recruitment of LdTyrPIP_22 epitopes to the flagellar pocket and a redistribution around the nucleus. These results suggest possible role(s) for this P-Tyr/PI phosphatase in the regulation of processes initiated or upregulated by this temperature/pH shift that contribute to the developmental transition from MPs to amastigotes inside the mammalian host macrophages.
Leishmania parasites, a class of trypanosomatid protozoans of the kinetoplastidae family, parasitize both invertebrate (sandflies of the genus Phlebotomus or Lutzomyia) and vertebrate hosts. When transmitted to the mammal host by the sandfly bite upon blood-feeding, Leishmania parasites are responsible for leishmaniases, a wide spectrum of diseases, which are major public health threats in endemic areas (WHO/PAHO, 2020). The most severe and potentially fatal form of these diseases transmitted by Leishmania (L.) donovani is visceral leishmaniasis (kala-azar) (Burza et al., 2018; WHO/PAHO, 2020). Up to 1 million new cases of all forms of leishmaniasis occur annually in about 100 endemic countries and over 20,000 deaths are attributed annually to them (WHO/PAHO, 2020). The few anti-leishmania drugs available to date present serious limitations. They are associated with severe side effects/toxicity or teratogenicity, high cost or emergence/spread of drug-resistant parasites in the field (Ghorbani and Farhoudi, 2018). Therefore, there is an urgent need to develop new specific and safe anti-parasitic compounds. In such framework, the identification of molecular determinants along the complex life cycle of these parasites should enlarge the target repertoire for designing anti-parasitic strategies.
To achieve its complex life cycle in the hematophagous insect host and in the mammalian host, Leishmania spp. undergo a series of differentiation processes that give rise to distinct biological stages allowing tight adjustments in each of their hosts (e.g., the insect’s luminal digestive tract and the interior of mammalian cells) that are significantly different in temperature, pH, nutrient availability and immune status (Bates, 1994; Mauel, 1996; Sunter and Gull, 2017). The main Leishmania developmental forms are: 1) the flagellated promastigotes, living in the sandfly host vector’s digestive tract (Bates, 1994; Dostalova and Volf, 2012) and eventually delivered into the mammalian host skin and 2) the ovioid amastigotes with a short mostly internal flagellum (Glaser et al., 1990; Gupta et al., 2001; Wheeler et al., 2015), propagating within macrophages of the vertebrate hosts inside a modified parasitophorous phagolysosome [(Desjardins and Descoteaux, 1997), reviewed by (Mauel, 1996; Handman and Bullen, 2002; Young and Kima, 2019)]. In the digestive tract of the insect, the dividing promastigotes go through several stages of differentiation the last step of which is called metacyclogenesis (Bates, 1994; Rogers et al., 2002; Bates, 2007). This developmental phase leads to the emergence of infectious metacyclics, short and slender flagellated forms never seen in division. They are highly motile and unattached to the insect’s digestive track and have a flagellum at least twice the length of their cell body (Bates, 2007; Sunter and Gull, 2017). The metacyclic promastigotes are believed to be the only forms injected into the skin when an infected fly takes a blood meal. Metacyclogenesis can be mimicked in vitro by cultivating promastigotes under chemically defined conditions allowing the generation of intermediate differentiation forms as well as fully differentiated metacyclic Leishmania promastigotes (Bates et al., 1992; Zakai et al., 1998; Nayak et al., 2018). Conditions have also been defined to reproduce the developmental transition from metacyclic promastigotes (MPs) to amastigotes by shifting stationary phase promastigotes’ cultures to 37°C and pH 5.5, mimicking thereby the conditions inside the parasitophorous phagolysosomes of mammalian macrophages (Doyle et al., 1991; Debrabant et al., 2004; Zilberstein, 2020)
The developmental transitions of metacyclogenesis and MP to amastigote enable Leishmania parasites to cope with the distinct microenvironments of their hosts by adjusting gene expression (Iantorno et al., 2017; Coutinho-Abreu et al., 2020) and consequently metabolism. These adaptations are reflected by the broad alterations in the absolute and relative RNA and protein levels and activity observed over metacyclogenesis and MP to amastigote transiton [(De Pablos et al., 2016; Inbar et al., 2017) recently reviewed by (Karamysheva et al. (2020)]. In this context, changes in the phosphorylation state of many proteins carried out by specific kinases and phosphatases have already been highlighted (Nascimento et al., 2003; McNicoll et al., 2006; Nascimento et al., 2006; Zhou et al., 2006; Depledge et al., 2009; Morales et al., 2010; Tsigankov et al., 2013; Soulat and Bogdan, 2017) pointing to the importance of P-Tyr phosphatases (PTPs) in the differentiation process of Leishmania parasites although few proteins (0.4%) were found to be phosphorylated on tyrosine during the L. donovani promastigotes’ differentiation to amastigotes in vitro (Tsigankov et al., 2013).
Besides the human homologs of PTPs expressed in Leishmania (Nascimento et al., 2006; Soulat and Bogdan, 2017), atypical Dual Specificity Phosphatases (aDSPs) with P-Tyr phosphatase activity have been detected in a phosphatome analysis of Leishmania genome sequences (Brenchley et al., 2007). These phosphatases seem to have no human homologs or to be very divergent from human homologs (Brenchley et al., 2007; Soulat and Bogdan, 2017). Moreover, another Bioinformatics study identified four Leishmania aDSPs as members of an “Atypical Lipid Phosphatase” family (ALPs) (Beresford et al., 2010), a group of enzymes found only in bacteria and lower eukaryotes. All ALPs share the characteristic structural P-loop feature (HCXXGKDR) of the catalytic site of PTPs, a signature also found in MptpB, a triple specificity (i.e., P-Tyr, P-Ser/P-Thr, and PI) phosphatase from M. tuberculosis (Beresford et al., 2007) and the LipA, a P-Tyr, and PI phosphatase from Listeria monocytogens (Kastner et al., 2011). Of note, both MptpB and LipA are secreted by the bacteria into their respective host cells where they subvert PI signaling, hence contributing to bacteria virulence (Beresford et al., 2007; Kastner et al., 2011). One of the Leishmania members of the ALP family encoded by the LmjF.22.0250 gene was shown in the same study to dephosphorylate P-Tyr peptides and monophosphorylated PIs. Interestingly, the LmjF.22.0250 gene transcript was later reported to be highly enriched during metacyclogenesis in the natural sandfly vector (Inbar et al., 2017). In the same line, L. mexicana parasites in which was ablated the LmxM.22.0250 gene, orthologous to the LmjF.22.0250, showed severely impaired survival in primary mouse macrophages in an in vitro infection system (Kraeva et al., 2019), results supporting a strong contribution of this ALP to the parasite’s fitness. Overall, the unique sequence- and biochemical- related features of ALPs, in addition to their acting as fitness determinants, make them attractive candidate targets for the development of specific antimicrobial inhibitors/drugs.
Herein, we report data from the biochemical characterization of the LdTyrPIP_22 protein (Leishmania donovani Tyrosine PI Phosphatase with gene locus at chromosome 22), encoded by the LdBPK_220120 gene, the L. donovani ortholog of the LmjF.22.0250 and LmxM.22.0250 genes mentioned above. We found that the LdTyrPIP_22 sequence is highly conserved in all sequenced Leishmania spp. from the Leishmania and Sauroleishmania subgenera and detected the expression of the LdBPK_220120 encoded protein in different morphological forms of cultured L. donovani promastigotes and axenic amastigotes. LdTyrPIP_22, when expressed in bacteria, dephosphorylates P-Tyr peptides and monophosphorylated PIs similarly to its L. major ALP ortholog. It is specifically distributed in distinct subcellular compatments in the promastigote and amastigote cells and is recruited to the flagellar pocket upon a temperature shift from 25°C to 37°C, one of the main parameters that drive the developmental transition of the Leishmania MP to amastigote.
All chemicals used, unless otherwise stated, were of analytical grade and purchased from Sigma-Aldrich or Applichem. Specifically, Digitonin (D141-100MG), Triton-X 100 (X100-100ML) and Ponceau S (P3504-50G) were from Sigma-Aldrich. Nourseothricin (NTC-AB-102L0) was from Jena Biosciences. Restriction enzymes were purchased from Roche (New England Biolabs) and/or KAPA Biosystems. Taq DNA polymerase (R001A) and T4 ligase (2011B) were from TaKaRa. All primers used in the PCR reactions (synthesized by VBC Biotech) are listed in Table S1. DNase I (2270A) was from TaKaRa and RNAse (10109134001) from Sigma Aldrich (Merck). One kb DNA ladder (N3232L) was from New England Biolabs (NEB), protein molecular mass standards (17-0446-01) were purchased from Amersham Biosciences and Nippon Genetics (Broad range:10–180 kDa, MWP03) while protein quantification reagent Bradford (B6916) and proteolytic inhibitors (P8465) were from Sigma-Aldrich. Fetal Bovine Serum (FBS) was from Thermo Fisher Scientific (10270106, Gibco) or Biosera (FB-1001/500). Bacto-tryptone (211705), Bacto Yeast extract (212750), and Bacto-agar (14050) were from BD Biosciences. The mouse monoclonal 6xHistidine epitope tag antibody (Ab) was from Acris Antibodies (SM1693PS), the a-tubulin (T5168) mouse monoclonal (mAb) was from Sigma, the rabbit polyclonal (pAb) a-Leish actin was kind gift from Dr Amogh Sahasrabuddhe (Division of Molecular and Structural Biology Central Drug Research Institute Lucknow, India). The GAPDH pAb was a kind gift of Frédéric Bringaud (U. of Bordeaux/CNRS, France). The a-mRFP pAb was prepared by us as described by Papadaki et al. (2015). The a-A2 Ab was from Abcam (ab150344). The a-EF1a Ab clone CBP-KK1 (05-235) was purchased from Merck. All Fluorochrome-conjugated secondary Abs [Alexa Fluor® 546 (A-11030 or A-11035) and Alexa Fluor® 488 (A32723 or A32731)] were from Thermo Fisher Scientific. Goat anti-rabbit HRP (41460) and goat anti-mouse HRP (31230) were from Pierce while the a-mouse and a-rabbit Abs conjugated with CF488A were from Biotinum (20010 and 20012, respectively). Hoechst 33342 (H3570) and FM 4-64 FX fixable membrane stain (F34653) were purchased from Thermo Fisher Scientific.
The murine monocytic cell line J774 was cultured in high glucose RPMI (1640) (Biosera LM1638) containing 10% (v/v) hiFBS [heat-inactivated (56°C, 30 min) fetal bovine serum], 1 U/ml penicillin and 0.1 mg/ml streptomycin. Cell counting was performed with a Neubauer hemocytometer.
L. donovani (strain LG13, MHOM/ET/0000/HUSSEN) promastigotes were cultured in RPMI 1640 containing 10% (v/v) hiFBS 1 U/ml penicillin, 0.1 mg/ml streptomycin (Gibco) and 10 mM Hepes (Gibco), at 25°C as previously described (Papadaki and Boleti, 2019). Cell counting was performed with a Malassez hemocytometer as described (Papadaki and Boleti, 2019). L. donovani axenic amastigotes were obtained according to modified published protocol (Barak et al., 2005; Zilberstein, 2020). Briefly, promastigotes at the stationary phase of growth, obtained after 8 days incubation at 25°C, pH 7, were harvested and resuspended in prewarmed (37°C) Medium 199 (pH of 5.5) containing 25% v/v FBS. Axenic amastigotes were obtained after 120 h incubation under these conditions (37°C, pH 5.5). Parasites incubated in the conditions inducing transformation of promastigotes to amastigotes (37°C, pH 5.5) were also analyzed after 24 h.
The gene encoding the LdTyrPIP_22 [1-258 amino acids (aa), GenBank®, accession No MF461274] was amplified by PCR from genomic L. donovani DNA (strain LG13) and inserted into the BglII site of the pLexsy-sat-mrfp1 plasmid (Papadaki et al., 2015) to create the pLexsy-ldtyrpip_22-mrfp1 plasmid. Secondly, the ldtyrpip_22 gene was cloned into the BglII/XhoI sites of the pTriEx1.1 vector (Invitrogen), in frame with the C-terminal His tag to produce the pTriEx1.1-ldtyrpip_22 and pTriEx1.1-N15-ldtyrpip_22 plasmids. N15 corresponds to the 15 additional aa (Met-Ala-Ile-Ser-Arg-Glu-Leu-Val-Asp-Pro-Asn-Ser-Gln-Ile-Ser) added to the N-terminus of LdTyrPIP_22 by expression from the second construct. All plasmid constructs were propagated in the Escherichia coli (E. coli) Top10F’strain for small (mini) and large scale (midi) plasmid DNA preparations. Two positive clones were selected and sequenced in each case (VBC-Biotech). The results showed 100% nucleotide sequence identity at the DNA level between the two clones. For recombinant protein production we used clones of E. coli BL21 (DE3) strain harboring the pTriEx1.1 based plasmids.
The transgenic promastigotes were generated based on minor modifications of a protocol previously described (Papadaki et al., 2015). Briefly, for episomal expression of LdTyrPIP_22-mRFP1, Leishmania promastigotes (2x107 cells/ml) at the stationary phase of growth were transfected by electroporation with supercoiled circular pLexsy-sat-ldtyrpip_22-mrfp1 plasmid. For electroporation, Leishmania promastigotes from a 10 ml culture were washed once and incubated (10 min, on ice) in 10 ml ice cold electroporation buffer (21 mM HEPES, pH 7.5, 0.7 mM Na2HPO4, 137 mM NaCl, 6 mM glucose, 5 mM KCl). Subsequently, they were centrifuged (1,000 g, 10 min), resuspended in 1 ml ice cold electroporation buffer and 400 ul of this suspension (2 x108 cells/ml) were added in a 1.5 ml Eppendorf tube containing 20-50 μg plasmid DNA dissolved in 50 μl dH2O. After mixing, the promastigotes’/DNA suspension was transferred to chilled electroporation cuvette (Gene Pulser/Micro Pulser Electroporation cuvette 0.2 cm, 165-2086, BioRad) and electroporated (50 μF, 0.45 kV, pulse time ~ 4-5 msec). The electroporated promastigotes (8x107 cells), after a 10 min incubation on ice, were transferred in 10 mL RPMI [20% (v/v) hiFBS] and incubated for 16 h at 25°C before the addition of the antibiotic Nourcethricin. A pool of positive transgenic promastigotes was selected by the addition (once a week) of gradually increasing concentration (20–100 μg/ml) of Nourcethricin.
The recombinant proteins used in the biochemical characterization of LdTyrPIP_22 were produced in E. coli as follows: A 4 L culture of BL21 (DE3) cells carrying the plasmid pTriEx1.1-rN15-ldtyrpip_22-His was induced overnight (~16 h) with 0.5 mM isopropyl β-D-thiogalactoside (IPTG) at 20°C and the cells were harvested by centrifugation (4,000 g, 15 min) 20 h later. Subsequently, the cell pellet was resuspended in lysis buffer (50 mM Tris, 300 mM NaCl, 30 mM imidazole, pH 8) with proteolytic inhibitors (Sigma, P 2714) and lysed by a freeze-thaw process repeated three times followed by 6–8 sonications (30–60 s, 100 W) each followed by a 30 s pause step of incubation in ice. The fusion rN15-LdTyrPIP_22-His protein was purified by nickel-affinity chromatography. The soluble fraction of the bacterial lysate was passed through a GE Healthcare (His TrapTM HP) His-binding column (ÄKTA-FPLC) with Ni2+ charged resin. The bound proteins, after been washed with lysis buffer, were eluted with 50 mM Tris, 300 mM NaCl (pH 8) and gradient concentration of imidazole up to 400 mM. As most of the proteins were eluted at fraction 1 (200 mM imidazole), this was used for a second purification step through a Talon column (Clontech Laboratories/A TaKaRa Bio company) packed with Co2+ charged resin. Finally, a total amount of ~1.6 mg of highly purified protein was obtained.
The fusion protein rN15-LdTyrPIP_22-His purified by Metal-Affinity Chromatography (Qiagen Ni–NTA Superflow resin) was injected into New Zealand white rabbits and BALB/c mice to raise polyclonal anti-sera, according to published protocols (Papadaki et al., 2015). All experimental procedures were approved by the Institutional Animal Bioethics Committee following the EU Directive 2010/63 and the National Law 2013/56. Purified rabbit anti-LdTyrPIP_22 pAb was obtained by low pH elution from immunoblots of purified rN15- LdTyrPIP_22-His, as previously described (Harlow and Lane, 1988).
Initially, the rN15-LdTyrPIP_22-His phosphatase activity was assayed at 25°C and 37°C in a reaction buffer (100 mM Tris, 150 mM NaCl, 4mM DTT) containing 10 mM of the generic phosphatase substrate p-nitrophenyl phosphate (pNPP) and pH ranging from 4 to 8. The reaction (200 μl buffer with substrate) was initiated by the addition of enzyme and quenched after 60 min by addition of two volumes 0.5 N NaOH. The absorbance of the reaction product (p-nitrophenolate = pNP) in the supernatant was measured at λ=405 nm.
To study the kinetic parameters of rN15-LdTyrPIP_22-His, phosphatase assays were carried out three times in triplicates, in a total volume of 60 μl, containing reaction buffer (pH 6), enzyme (2 μM), and pNPP (0.5–100 mM) at 25°C. The absorbance (λ=405 nm) was measured in Infinite M1000PRO plate reader (TECAN). Kinetic constants were determined by fitting the data to the Michaelis-Menten equation using GraphPad Prism Software 5.01 (GraphPad, San Diego, CA).
To determine the substrate specificity of rN15-LdTyrPIP_22-His a number of reactions were carried out using as substrates P-Tyr (1 mM) or P-Ser/P-Thr (60 μM) peptides in reaction buffer (100 mM Tris, 150 mM NaCl, 4 mM DTT, pH 7) at 25°C (Table S2). The peptides were synthesized and purified according to standard procedures. Additionally, mono, bis, or trisphosphorylated PIs (100 μM) (Avanti polar lipids or Echelon) (Table S2) were tested as plausible substrates of the recombinant LdTyrPIP_22 using the EnzChek® (Thermo Scientific) protocol (pH 7, 25°C) following the manufactures’ instructions. The absorbance reading at 360 nm was performed with the X Infinite M1000PRO plate reader (TECAN).
The effect of inhibitors was evaluated in phosphatase assays where rN15-LdTyrPIP_22-His solutions (2 μM) were pre-incubated (25 min, RT) with a) sodium orthovanadate (Na3VO4) concentrations ranging from 1–20 mM, b) 100 mM sodium fluoride (NaF) or c) with reaction buffer only (100 mM Tris, 150 mM NaCl, 4 mM DTT, pH 7). The phosphatase activities in the solutions were subsequently assayed using as substrate the pNPP (10 mM) and reading the absorbance at λ=405 nm (max absorbance of the chromogenic product pNP). Estimation of the product concentration was according to a standard curve generated with pNP samples of known concentration.
Promastigotes were resuspended in lysis buffer [20 mM Tris-HCl (pH 6.8), 0.1% SDS] (108 parasites in 100 µl lysis buffer), boiled for 5 min, cooled on ice and treated with DNase I (5–10 U/sample, 15 min, on ice) to remove DNA. Protein was estimated by the Bradford assay method. For further analysis by SDS-PAGE, 6X Laemmli sample buffer was added and the samples were either boiled for 5 min or incubated at 37°C for 30 min in the presence of proteolytic inhibitors.
Digitonin permeabilization of L. donovani-rLdTyrPIP_22-mRFP1 stationary phase L. donovani promastigotes is based on protocols previously described (Foucher et al., 2006; Doukas et al., 2019) with slight modifications. Briefly, L. donovani (wt or transgenic) promastigotes (~2 x109) were harvested by centrifugation (1,000 g, 7 min, 4°C), washed twice in resuspension buffer (145 mM NaCl, 11 mM KCl, 75 mM Tris-HCl, pH 7.4) resuspended in 0.5 ml of the same buffer and supplemented with protease inhibitors. A stock of 100 mM digitonin solution in dH2O was prepared. From this 100 mM digitonin solution were subsequently prepared by serial dilution solutions of 20 mM, 2 mM, 400 μM and 40 μM. Membrane permeabilization and protein fractionation were achieved by adding 0.5 ml digitonin solution prewarmed at 37°C of progressively increased detergent concentrations (stepwise, four steps) to achieve digitonin concentrations of 20 μM, 200 μM, 1 mM, or 10 mM. In each step a soluble fraction and a corresponding pellet were recovered. The final pellet recovered after treatment with 10 mM digitonin (F5; enriched in plasma membrane, nuclei and cytoskeletal proteins), was further solubilized with 0.5 ml 1% (v/v) TritonX-100 (1 h, 4°C) and the soluble fraction (F5 S) was recovered from the insoluble fraction (F5 P) by centrifugation (20,000 g, 20 min, 4°C). The soluble fractions (F1-F4 and F5 S) were subjected to acetone precipitation by the addition of an acetone (prechilled at -20°C) volume equal to four sample volumes followed by 1h incubation at -20°C. The protein pellets recovered and the F5 P pellet were solubilized in Laemmli buffer, samples were boiled (5 min, 95°C) and further analyzed by SDS-PAGE and Western blot.
Proteins were separated by SDS-PAGE (12% gel) and transferred to Hybond-C nitrocellulose (Amersham) membrane using a wet blotting apparatus (BioRad). After protein transfer, Hybond-C membranes were stained with Ponceau S solution [0.5% (w/v) Ponceau S dissolved in 1% (v/v) acetic acid]. Nonspecific sites for Ab binding on the nitrocellulose membrane were blocked by incubation (1 h, RT) with Blocking buffer [Tris-buffered saline (TBS; 50 mM Tris-Cl, pH 7.5, 150 mM NaCl), 0.05% (v/v) Tween 20, 5% w/v BSA]. Incubation with the primary Abs was performed overnight (~16 h) at 4°C. The a-LdTyrPIP_22 mouse or rabbit pAbs were used at 1:1200 (serum) or ~1 μg/ml (affinity purified) respectively diluted in [Tris-buffered saline, 0.1% (v/v) Tween 20 (TBST)]. Other Abs were used as indicated in figure legends. After three washes in TBST, the blots were incubated (1 h, RT) with HRP-labeled a-mouse or a-rabbit Abs used at 1:5,000 dilution. Following three washes in TBST and one final wash in TBS Ab reactivity was revealed either by the ECL plus system (Amersham) or by the chromogenic DAB method. In the former case, membranes were exposed to Kodak photographic films further developed with Kodak reagents. Reprobing with the a-mRFP1 pAb (0.5 μg/ml) or a-EF1A (1:10,000) where required, was performed after stripping the membrane by incubation (30 min, RT) with a low pH buffer (25 mM glycine-HCl, 1% (w/v) SDS, pH 2) followed by washing and reblocking with the Blocking buffer. Apparent molecular weights of proteins detected with the specific Abs were assigned by using low (14–98 kDa, Amersham) or broad range molecular weight markers (10–180 kDa, Nippon).
L. donovani promastigotes were fixed with 2% (w/v) paraformaldehyde (PFA) (20 min, RT) in PBS, allowed to adhere to poly-L-lysine coated coverslips and treated with 50 mM NH4Cl in PBS (10 min, RT) followed by PBS wash. Fixed cells were incubated (1 h, RT) first with primary Abs in blocking buffer (PBS, 1% (w/v) BSA) and after extensive washing, the appropriate secondary Abs conjugated to Alexa Fluor® 546 and Alexa Fluor® 488 were added at a final concentration of 2 μg/ml in blocking buffer (1 h, RT). The secondary Abs were removed with extensive washing and the parasite DNA was stained (10 min, RT) either with 10 μg/ml propidium iodide solution in PBS containing 100 μg/ml RNase or with Hoechst 33342 (Mol. Probes H3570) at final concentration of 10 μg/ml. Coverslips were mounted with Mowiol 4-88 (10% (w/v) Mowiol-Calbiochem, 25% (v/v) glycerol, 100 mM Tris-HCl, pH 8.5) on microscope slides, sealed with nail polish, and stored at 4°C.
For the infection of macrophages, stationary phase L. donovani promastigotes, obtained after 8 days in culture without subculturing, were added to semi confluent cultures of J774 mouse macrophage cells grown on coverslips in multiwell dishes, at 20:1 parasite/macrophage ratio and the macrophages/parasites co culture were incubated at 37°C for 4 h. At the end of this period, the parasites were removed, the macrophages were washed once with prewarmed (37°C) fresh medium and were either processed for immunofluorescence confocal microscopy analysis or they were further incubated in fresh medium (RPMI, 10% v/v FBS, 1% w/v penicillin/streptomycin, 37°C) according to each experiment’s specification. For immunofluorescence analysis, infected J744 macrophages were fixed (20 min, RT) with PFA [4% (w/v) in PBS]. The non-reacted PFA was neutralized with 50 mM NH4Cl in PBS (10 min, RT) and the cells were stained with primary and secondary Abs or phalloidin-Alexa-546®. Coverslips were mounted as described in the previous paragraph.
Microscopic analysis of the Leishmania cells or the infected J744 macrophages was performed with the Leica TCS SP or SP8 confocal microscopes using the 63X apochromat lens. Image acquisition included collecting z stacks of 0.3 or 1 μm step size for parasites or infected macrophages respectively.
The extent of co localization between the LdActin and the LdTyrPIP_22 (green and red FL respectively) was measured using the 3D “Coloc” module of Imaris v9.2.1, which utilizes the algorithms introduced by Costes et al. for the automatic selection of thresholds of the image channels (Costes et al., 2004). We have used a third channel as a masking area for the entire analysis (created with the channel Arithmetic function “sqrt (ch1*ch2)”, to exclude the background pixels of the dataset from the co localization analysis. The mask channel is used in conjunction with the automatic threshold function. This way Imaris “3D Coloc” can generate a new channel (the co localization channel), which only contains voxels representing the co localization between the red and green channels. Co localization was quantitated separately in the promastigotes’ cell bodies and the flagella. Threshold 5 was used for analysis in the cell body and 2.9 for the entire promastigote and the flagellum.
L. donovani promastigotes in a stationary phase culture (50 ml) were enumerated with a Malassez hemocytometer or by measuring turbidity at OD600 (Papadaki and Boleti, 2019) and were then harvested by centrifugation (1,000 g, 10 min, RT). The Leishmania cell pellet was resuspended for washing in the same volume of RPMI and the cells were collected by centrifugation (1,000 g, 10 min, RT). Subsequently the promastigotes’ pellet was resuspended in RPMI/10 mM Hepes (1/5th of the starter’s culture volume) without FBS and the promastigotes were incubated at 25°C for 9 h or at 37°C for 6 h. At the end of the incubation period, the cells were separated from the culture supernatant by centrifugation, washed with PBS and stored (-20°C) until use. The culture supernatant was centrifuged (21,000 g, 20 min, 4°C) and filtered through a 0.2 μm filter to remove cell debris. Finally, the proteins in the culture supernatant were precipitated by adding 4 volumes of prechilled acetone (-20°C), vortexing and incubating overnight at -20°C. The precipitated proteins were collected by centrifugation at 14,000 g and recovered by decanting the supernatant. Acetone was allowed to evaporate from the uncapped tube at room temperature for 30 min. Appropriate volume of buffer for the downstream process was added and the sample was vortexed thoroughly to dissolve the protein pellet. Alternatively, the culture supernatant was concentrated through 10 kDa cutoff centrifugal filters (Merck) and the buffer was changed to 20 mM Tris-HCl, 150 mM NaCl, pH 9. The same buffer was used to equilibrate a Superdex 200 10/300 column which was subsequently used for analysis of the supernatant proteins according to molecular size. After fractionation, the samples were concentrated with the use of filters (10 kDa MW cutoff, Thermo Fischer) and stored at -20°C until analyzed by SDS-PAGE and WB.
The algorithms used for identifying sequence similarities prediction (Blast) and multiple sequence alignment/editing were: 1) TriTrypDB BLAST 1 2) ClustalW22; 3) BioEdit Sequence Alignment Editor, version 7.0.9.0 (Ibis Biosciences). Signal P 3.03) and NetPhos3.1b; 4) ExPaSy ProScale Hphob./Kyte & Doolittle4; 5) NetPhos3.1b tool5 and GPS-Lipid6 were algorithms used to predict sites for post-translational modifications, signal sequence for secretion. The sequence used in this study to design primers for PCR amplifications of the ldtyrpip_22 gene from L. donovani is the ortholog LINF_220007400 from L. infantum [(clone JPCM5 (MCAN/ES/98/LLM-877)] (TritrypDB) (Table S1). To perform the Blast search for ldtyrpip_22 orthologs in Leishmania spp. genome, we used the ldtyrpip_22 sequence registered in GenBank as ‘Leishmania donovani tyrosine phosphoinositide phosphatase, LdTyrPIP_22’ (accession No MF461274). The rooted phylogenetic tree analysis was based on the UPGMA method. Data for the Enzyme kinetics were analyzed using the GraphPad Prism Software 5.01 Michaelis-Menten model algorithm7. Graphs of enzyme activities were generated with the use of GraphPad. Standard deviations and t-test for statistical significance were calculated using the Excel’s Formula and Data tools. The Icy computational algorithm7,8 and the Adobe Photoshop CS6 Portable were used for image evaluation and processing, while for the co localization analysis, we used the 3D “Coloc” module of Imaris v9.2.1. The Fiji algorithm was used for quantification of the band intensities in the Western Blot digital images.
Our interest on PI phosphatases in Leishmania spp. focused on a study identifying the Leishmania major LmjF.22.0250 gene that encodes for a dual specificity PI and P-Tyr phosphatase (Beresford et al., 2010). This protein (LM1) contains the putative P-loop motif (HCXXGKDR[TA]G) of PTPs detected in the Mptpb triple specificity PI phosphatase from M. tuberculosis and in the LipA dual specificity P-Tyr and PI phosphatase from L. monocytogenes (Beresford et al., 2010). Moreover, a later study showing an increase in the levels of the LmjF.22.0250 gene transcripts during metacyclogenesis in the natural sandfly vector (Inbar et al., 2017) prompted us to better characterize the structural and functional features of this phosphatase in the related L. donovani species, which causes in humans the potentially fatal disease of visceral leishmaniasis (Burza et al., 2018). We therefore cloned the LmjF.22.0250 ortholog gene from L. donovani by a PCR approach using genomic DNA isolated from the L. donovani LG13 strain (MHOM/ET/0000/HUSSEN) (Materials and Methods; Gene Bank accession No MF461274). This was found to be 99.9% identical to the LDBPK_220120.1 gene (777 bp) from the sequenced L. donovani Nepalese strain BPK282A1 registered in the TriTrypDB database with one base difference leading to substitution of Ala171 by a Thr171 (Figure 1A). The LDBPK_220120.1 gene, from now on designated in our study as ldtyrpip_22 (L eishmania d onovani Tyrosine PI Phosphatase on chromosome 22), is located at positions 91159 to 91935 of chromosome 22. A Blast search using the full length ldtyrpip_22gene product from the L. donovani, strain LG13, identified orthologs in all Leishmania spp. genome sequences available in the TriTrypDB with exception the L. braziliensis strain M2904, MHOM/BR/75M2904 from the Viania subgenus. In this case a pseudogene (LbrM.22.2.000240) was retrieved by our search. Multiple sequence alignment indicated that the LdTyrPIP_22 protein sequence (length 258 aa, calculated MW: 29081.69 Da, PI: 8.30) is highly conserved (81.34–99.22% identity) (Figure 1, Table S2) in several species of the Leishmania and Sauroleismania subgenera of the Leishmania genus according to a recent classification of Leishmania spp. (Akhoundi et al., 2016; Akhoundi et al., 2017). The rooted phylogenetic tree (UPGMA) from a multiple sequence alignment of the LdTyrPIP_22 orthologs from several Leishmania spp. (Figure 1B) highlighted the sequence similarities depicted in the representation of the ClustalW2 sequence analysis shown in Figure 1A and Table S2. Interestingly, no homologs were detected by this BLAST analysis in the closely related species of Trypanosoma.
Figure 1 Sequence comparison of the LdTyrPIP_22 orthologs in Leishmania spp. (A) ClustalW multiple sequence alignment of the ldtyrpip_22 gene product orthologs in Leishmania spp. The L. donovani (strain LG13, MHOM/ET/0000/HUSSEN) LdTyrPIP_22 aa sequence (GenBank® accession No MF461274) was generated in this work. The aa sequences for the L. donovani LdBPK_220120.1gene product from the Nepalese strain BPK282A1 and from another eleven Leishmania spp. (Table S2) with genomes sequenced were obtained from the TriTrypDB database and were analyzed/edited using the software BioEdit Sequence Alignment Editor. The aa of the PTP P-loop motif are framed by a red box; The aa predicted as S-Palmitoylation site are framed by the black box. (B) Rooted phylogenetic tree (UPGMA) from a multiple sequence alignment of the LdTyrPIP_22 orthologs from Leishmania spp.
With respect to its bacteria homologs (Beresford et al., 2010) from M. tuberculosis (MptpB, GenBank: CCC62750.1), L. monocytogenes [lmo1800, NP_465325; lmo1935, NP_465459)], Yersinia pestis KIM10+ (WP_002228183), and Bacillus anthracis strain Ames (NP_845680), the LdTyrPIP_22 shows in its entire sequence length 19.4%, 29.6%, 36.1%, 27.4% and 32.4% aa sequence identity, respectively. In the P-loop active site-containing region the sequence identity is 73-91% with the respective protein sequences of the aforementioned bacterial homologs (Figure S1).
Finally, by applying several tools of bioinformatics, we searched for predicted structural motifs and post-translational modifications in the LdTyrPIP_22 aa sequence. As the bacteria homologs of this putative phosphatase are secreted, we first analyzed by the SignalP 3.0 software for the presence of a eukaryotic secretion signal sequence motif in the N-terminus of LdTyrPIP_22. No such sequence was detected. However the absence of a secretion signal does not exclude the possibility that LdTyrPIP_22 is secreted since a significant number of Leishmania proteins with no signal sequence motif are secreted via exosomes (Silverman et al., 2008; Dong et al., 2019) or ectosomes [recently reviewed by (de Souza and Barrias, 2020)]. Subsequently, we searched for putative phosphorylation sites using the NetPhos3.1b bioinformatics tool. Interestingly, the extreme C-terminal Ser residue of a series of Ser and Thr residues (TSSSS) was predicted to be phosphorylated with high score (0.970). Three more residues in the N-terminal half of the molecule, Ser74, Ser116, and Tyr127, were also predicted to be phosphorylated (scores 0.991, 0.973, and 0.945, respectively). This information, suggests that the LdTyrPIP_22 may be post-translationally regulated by phosphorylation. Moreover, the LdTyrPIP_22 sequence was checked for other possible post translational modifications. In this search, a putative palmitoylation site at Cys182 (S-Palmitoylation: Cluster C: FMLTNRCCVPPSCE) in the LdTyrPIP_22 sequence was revealed by the GPS-Lipid algorithm with moderately high score (1.495 with cutoff 1.396).
From the primary structure of LdTyrPIP_22 was predicted that this protein must act as a PTP since it contains the characteristic P-loop motif sequence of the PTP catalytic center also described to be present in the sequence of the ALP enzyme family members (Beresford et al., 2010). To confirm the predicted PTP catalytic properties of LdTyrPIP_22 we analyzed the enzyme kinetics and the substrate specificity of a LdTyrPIP_22 recombinant form expressed in bacteria. For this we constructed two plasmids encoding the full length protein (residues 1–777) with a polyHis tag at the C-terminus. In the first plasmid the initiation of translation of the insert begun at the ATG codon of the ldtyrpip_22 sequence, whereas in the second plasmid translation was inititiated at the pTriEx1.1 plasmid’s ATG codon located 45 bp upstream the site where the ldtyrpip_22 sequence was inserted. The second construction encodes for a polypeptide with an additional 15-mer at the N-terminus (pTriEx1.1-N15-ldtyrpip_22-His; Figure 2A). The recombinant protein produced from the first plasmid was mostly insoluble (Figure S2A). In contrast, the rN15-LdTyrPIP_22-His polypeptide produced from the second plasmid (Figure 2A), was mostly soluble (Figure S2B). The rN15-LdTyrPIP_22-His (281 aa, calculated MW 32062 Da), was subsequently purified by affinity chromatography on Ni2+ and/or Co2+ beads (Figure 2B, inset) and its enzymatic activity was assessed by monitoring the kinetics of dephosphorylation of the generic phosphatase substrate pNPP (Table 1, Figure 2B).
Figure 2 Study of the catalytic properties and substrate specificity of the LdTyrPIP_22. (A) Schematic representation of the rLdTyrPIP_22 protein expressed in bacteria for this study (281 aa, with calculated Molecular mass: 32,062). (B) Michaelis-Menten saturation curve for the enzymatic reaction of the rN15-LdTyrPIP_22-His (2 μM) using the pNPP (0.5–100 mM) as substrate. The reaction was performed at 25°C, pH 6. Inset: Coomassie stained SDS-PAGE of eluted fractions (E1-E9) from the purification of rN15-LdTyrPIP_22-His from bacteria lysates (Materials and Methods) were analyzed on a 4%–12% gradient SDS-PAGE gel. Arrow on the right side indicates the expected position for migration of the rN15-LdTyrPIP_22-His polypeptide; Time course of dephosphorylation of PI species (100 μM) (C), P-Tyr peptides (1 mM) (D), and P-Ser/P-Thr peptides (60 μM) (E). Peptide aa sequences are shown in Table S2.
As expected, the rN15-LdPIP22-His specific activity [74.8 ± 1.01 nmol pNP/min/mg (n=4)] estimated at 37°C and pH 6, was in the same range as the specific activity estimated for the L. major ortholog phosphatase (45.47 ± 1.52 nmol pNP/min/mg) reported in the original publication describing the ALP family in bacteria and lower eukaryotes (Beresford et al., 2010). Finally, the catalytic activity of rN15-LdTyrPIP_22-His towards pNPP was shown to be optimal at pH 6 and 7 when measured at 25°C, while at 37°C the activity was found to be optimal at pH 6 (Figures 3A and S3C). Phosphatase activity was also detected at more acidic conditions (Figures 3A and S3C).
Figure 3 Effect of pH and inhibitors in the rN15-LdTyrPIP_22-His phosphatase activity. The rN15-LdTyrPIP_22-His phosphatase activity was assayed at 25°C (Materials and Methods) with pNPP (10 mM) as substrate, (A) in pH ranging from 4-8; (B) in the presence of the specific PTP inhibitor, Na3VO4 (1–20 mM) or (C) in the presence of the specific P-Ser/P-Thr phosphatase inhibitor, NaF (100 mM) at pH 6.
Apart from the pNPP substrate, we also compared the ability of rN15-LdTyrPIP_22 to dephosphorylate a panel of PIs (Figure 2C) and peptides containing in their sequence P-Tyr or P-Ser/Thr (Figures 2D, E; Table S3). Our results indicated that rN15-LdTyrPIP_22-His has a double substrate specificity. It dephosphorylated both monophosphorylated PIs [PI(3)P and PI(4)P] (Figure 2C) and P-Tyr containing peptides (Figure 2D), while it did not dephosphorylate any of the small number of P-Ser/P-Thr containing peptides tested (Figure 2E and Table S2). As control enzymes were used the bacterially expressed human phosphatase of regenerating liver-3 (PRL3) (Goldstein, 2001, Mc Parland et al., 2011) (Figure S3A) and the human protein-tyrosine phosphatase PTP1B (Wassef et al., 1985) (Figure S3B). The substrate selectivity of rN15-LdTyrPIP_22 for P-Tyr was further confirmed by carrying out inhibition assays with the P-Tyr phosphatase inhibitor Na3VO4 and the P-Ser/P-Thr phosphatase inhibitor NaF using pNPP as substrate (10 mM) (Figure 3). Na3VO4 inhibited almost quantitatively the dephosphorylation of pNPP even at 1/10th of the substrate concentration (Figure 3B), whereas NaF showed no inhibitory effect even at a concentration 10 fold higher than the substrate’s concentration (Figure 3C).
All the above described data confirmed that the recombinant bacterially expressed LdTyrPIP_22 behaves as a dual specificity P-Tyr and PI phosphatase as expected from its sequence similarity to phosphatases from the ALP family (Beresford et al., 2010).
To confirm the expression of the LDBPK_220120.1 encoded LdTyrPIP_22 protein in L. donovani cells and assess its abundance at different developmental stages of the parasite we used axenic L. donovani promastigote cultures growing at 25°C and pH 7 at the logarithmic (enriched in dividing cells) and stationary (enriched in non-dividing cells/metacyclis) phases of growth. We also used stationary phase promastigotes cultured for another 24 h at 37°C and pH 5.5, conditions mimicking the temperature and pH of the parasitophorous phagolysosome’s environment in mammalian macrophages (Mc Parland et al., 2006). For this, total lysates from these parasites were analyzed by Western Blot using a-LdTyrPIP_22 specific polyclonal antibodies (pAbs) generated for this project (Materials and Methods and Figure S4A). The rabbit pAb detected in all three cases [i.e., logarithmic and stationary phase promastigotes growing at 25°C and pH 7 and promastigotes subjected to heat (37°C) and low pH (5.5) treatment] a protein species with apparent MW ~ 30 kDa (Figure 4Aa). Given that the calculated MW of LdTyrPIP_22 is 29.1 kDa we consider that this ~30 kDa protein species corresponds to the nascent LdTyrPIP_22 form. Occasionally we also detected a pair of bands migrating with lower mobility below the 35 kDa protein size marker (Figure S4B). Quantification of the signal intensity of the ~ 30 kDa band detected by the specific a-LdTyrPIP_22 rabbit pAb showed significantly higher levels in the logarithmically growing cells than in the stationary phase ones (Figure 4Ad).
Figure 4 Biochemical detection of the endogenous LdTyrPIP_22 forms in wt L. donovani (LG13) and in L. donovani-ldtyrpip_22-mrfp1 transgenic promastigotes. Subcellular distribution of LdTyrPIP_22 by detergent treatment. (A) Total lysates (40 μg protein/well) from logarithmic (log) and stationary (stat) phase cultures of promastigotes (~5X106 cells), as well as from parasites after treatment of stationary phase promastigotes for 24 h at 37°C and pH 5.5, were analyzed by SDS-PAGE (12% w/v) and immunoblotted with the purified rabbit a-LdTyrPIP_22 pAb (2 μg/ml) (a). The membrane was then rebloted after stripping with the a-EF1a mAb (1:10,000). LdEF1a was used as loading indicator (b). The region of the membrane with tubulin stained with Ponceau-S is shown (c) as a 2nd loading indicator. (d) Quantification of the intensity of the LdTyrPIP_22 band signal for each condition was performed using the Fiji algorithm. The intensity of the LdTyrPIP_22 band signal for each condition was normalized to the intensity of the corresponding LdEF1a or LdTubulin band signals and expressed as % of the normalized LdTyrPIP_22 signal at the logarithmic phase in each experiment. The mean values from three independent experiments with standard deviations were plotted. ** (P ≤ 0.01), * (P ≤ 0.05) and ns (P > 0.05). (B) Protein fractions (F1-F5ins) from stationary phase L. donovani-ldtyrpip_22-mrfp1 transgenic promastigotes, produced by permeabilization of total cell pellet with digitonin and Triton X-100 (Materials and Methods) were analyzed by SDS-PAGE (12% w/v) and immunoblotted with the mouse a-LdTyrPIP_22 serum (1:1,200) (a) and subsequently, after stripping, with the purified a-mRFP1 (0.5 μg/ml) pAb (b). Ponceau-S of respective membrane regions (c), is shown as loading indicator. Black arrowhead indicate the protein bands corresponding rabbit to rLdTyrPIP_22-mRFP1 and grey arrowhead to band assigned to the endogenous LdTyrPIP_22. Molecular weights are indicated in kDa.
To examine whether the LdTyrPIP_22 protein could preferentially associate with certain subcellular compartments, we prepared subcellular fractions from transgenic L. donovani promastigotes expressing a chimeric LdTyrPIP_22-mRFP1 which would serve as an internal positive control for the specificity of the a-LdTyrPIP_22 pAbs. The biochemical subcellular protein fractionation performed was based on a protocol using a stepwise permeabilization of promastigote membranes by incubating with gradually increasing concentrations of the natural detergent digitonin (Foucher et al., 2006) followed by an additional membrane solubilization step in which the final pellet [Fraction 5 (F5)] was treated with 1% (v/v) Triton X-100 [(Doukas et al., 2019), Materials and Methods]. This procedure allowed preparation of subcellular fractions enriched in soluble cytosolic proteins (Fractions F1 and F2), proteins associated with intracellular organelles (Fraction F3 and F4), endoplasmic reticulum (ER) (Fraction F4), pelicular membrane (Fraction F5s), and an insoluble fraction (F5in, final insoluble pellet) enriched in nuclear/cytoskeleton proteins. We further analyzed these fractions by WB using the mouse a-LdTyrPIP_22 pAb that we generated (Figure S4A).
A band detected with apparent MW ~ 55 kDa (Figure 4Ba) probably corresponds to the recombinant LdTyrPIP_22-mRFP1 polypeptide (calculated MW 54.5 kDa) since it was also detected by the a-mRFP1 specific pAb (Figure 4Bb). Three more protein species were detected at the 30-35 kDa range with the a-LdTyrPIP_22 pAb (Figure 4Bc), not recognized by the a-mRFP1 pAb (Figure 4Bb). The lower size band of ~30 KDa most probably corresponds to the endogenous LdTyrPIP_22 form identified in the wt L. donovani total lysates by the rabbit a-LdTyrPIP_22 pAb (Figure 4Aa). The doublet just below 35 kDa could be assigned to proteolytic fragments of the LdTyrPIP_22-mRFP1 not recognized by the a-mRFP1 pAb under the specific conditions of the experiment. Interestingly, the recombinant full length LdTyrPIP_22-mRFP1 was detected in all subcellular fractions analyzed [F1-F5ins]. The three protein species of lower size were detected in all fractions except the F5s (Figure 4Bc) a fraction shown before (Doukas et al., 2019) to be enriched in pelicular membrane proteins. The presence of LdTyrPIP_22-mRFP1 in the F5s fraction could represent a translocation artifact due to overexpression, as previously observed for other chimeric proteins tagged with fluorescent proteins (Moore and Murphy, 2009). Finally, a small fraction of the ~ 30 kDa species, assigned to the endogenous LdTyrPIP_22 and of the full length LdTyrPIP_22-mRFP1 were faintly detected in the F5ins fraction which is enriched in nuclear/cytoskeletal proteins (Foucher et al., 2006). Monomeric free mRFP1 (MW ~27 kDa), probably produced by proteolysis of the full length chimera LdTyrPIP_22-mRFP1, was only detected in fraction F5s (Figure 4Bc). In previously published work we have shown that free mRFP19 produced in transgenic L. tarentolae-mRFP1 cells was mostly recovered in fractions F1 and F2 whereas ER-associated or pelicular membrane proteins were recovered in fractions F4 and F5s (Papadaki et al., 2015; Doukas et al., 2019). It is worth emphasizing that both a-LdTyrPIP_22 Abs (mouse and rabbit) detected the 30 kDa protein species (Figures 4Aa, Ba), a result that strengthens the assignment of this band to the endogenous LdTyrPIP_22 protein.
Since there is evidence for secretion of the bacteria ALP homologs of LdTyrPIP_22 (i.e., MptpB and LipA) (Koul et al., 2000; Kastner et al., 2011), we also investigated whether the LdTyrPIP_22 could be secreted by wt L. donovani. For this, we analyzed by WB the proteins in the extracellular medium of axenic L. donovani promastigotes growing at 25°C (temperature of the invertebrate host) at the stationary phase of growth or at 37°C (temperature of the mammalian host) following the two approaches described in the Materials and Methods. Despite repeated attempts we were not able to detect the LdTyrPIP_22 amongst the proteins collected from the extracellular medium of the L. donovani LG13 strain incubated without serum for 9 h at 25°C or for 6 h at 37°C (data not shown). These assays did not include however the challenging analysis of proteins that would be released by the amastigotes inside the infected macrophages.
Thus, the LdTyrPIP_22 protein, encoded by the LDBPK_220120.1 gene, is expressed in L. donovani promastigotes at the logarithmic and stationary phases of growth, as well as in promastigotes subjected to heat (37°C) and pH (5.5) stress for 24 h. Interestingly, the LdTyrPIP_22 was detected in several different subcellular fractions enriched in cytoplasmic, membrane or cytoskeleton associated proteins.
To study further the LdTyrPIP_22 subcellular distribution in Leismania cells we performed immunofluorescence labeling and confocal microscopy analysis using our homemade mouse and rabbit a-LdTyrPIP_22 pAbs (Figure S4A, B) in combination with Abs recognizing other Leishmania proteins of known subcellular localization, including LdActin (Sahasrabuddhe et al., 2004), LdTubulin, and GAPDH as a glycosome marker (Herman et al., 2008).
We first analyzed the LdTyrPIP_22 localization in axenic dividing and non-dividing promastigotes grown at 25°C using logarithmic or stationary phase promastigote cultures. Having at our disposal a-LdTyrPIP_22 Abs from two species, allowed us: 1) to ensure that the epitopes highlighted by each Ab belong to the endogenous LdTyrPIP_22 (Figure S4B) and 2) to perform co localization studies with other Leishmania proteins using specific primary Abs either from rabbit or mouse origin. For imaging of the parasites besides the TCS SP Leica confocal microscope we used the SP5 and SP8 Leica more advanced confocal models which allowed us to benefit from maximum optical resolution of the lenses and higher digital resolution in the image acquisition than the older TCS SP confocal microscope.
The localization pattern of LdTyrPIP_22 varied in parasites at different cell cycle dependent morphological stages (Figure 5 and Figure S5). Depending on the cell cycle stage, axenic Leismania promastigotes obtain diverse morphologies most of which, with exception the procyclic and the metacyclic forms, coexist both in the exponential and stationary phases of growth (Wheeler et al., 2011). These morphologies, following nomenclature used for description of the flagellated promastigote forms observed in the midgut of sandflies (Sunter and Gull, 2017), could be assigned as: 1) dividing procyclic-like with short cell body (6.5–11.5 μm) and flagellum shorter than the cell body; 2) leptomonad-like with cell body between 6.5–11.5 μm and longer flagellum; 3) nectomonad-like with cell body longer than 12 μm and long flagellum and 4) the metacyclic-like with short (8 μm) and slender cell body and long flagellum (>2X longer than the cell body).
Figure 5 Localization of LdTyrPIP_22 in different cell cycle dependent morphological forms of cultured L. donovani promastigotes growing at 25°C, pH 7; co staining for LdActin. L. donovani promastigotes from logarithmic or stationary phase cultures were fixed (4% w/v PFA) and permeabilized with 0.1% (v/v) TritonX-100. They were then co stained by IF with the a-LdTyrPIP_22 mouse pAb (1:250) and rabbit a-LeishActin pAb (1:1,000) followed by secondary Abs conjugated to Alexa Fluor®546 and Alexa Fluor® 488, respectively. Nuclear and kinetoplast DNA was stained with Hoechst 33342 (1:5,000). Representative images of different cell cycle dependent morphological forms of promastigotes were acquired by z-scanning performed at 0.3 μm step size using the TCS SP8 Leica confocal microscope. A single z section with representative staining from each case is shown. Single FL images are shown in black and white (BW) for better contrast while images of the merged FL signals are shown in color. The molecule highlighted in the BW images with single color FL is indicated at the top in the same color as the respective FL signal. Arrowheads point to the flagellar pocket region/flagellum base. Small arrows point to the kinetoplast (k) and nucleus (N). Magnifications (1.5-2X) of framed areas are shown as insets. Scale bar size: 10 μm.
Interestingly, in dividing and non-dividing morphological forms of promastigotes in culture, LdTyrPIP_22 was localized at the periphery of the cell body (Figure 5, Figures S5Ac, d, S6 and S9) in areas rich in LdActin (Figures 5A–D insets) and microtubules (Figures S6) or stained with the FM4-64 dye (Figure S7) (Vince et al., 2008). Occasionally, LdTyrPIP_22 epitopes were also detected in internal tubular/filament-like structures (Figure 5D, bottom inset, Figures S5Ab, S7b) which could be part of the tubular MTV endosome (Halliday et al., 2019; Wang et al., 2020) and at the posterior region of the cell body (Figure 5A, Figure S7b), area where internalized/endocytosed cargo accumulates in late endosomes (Ghedin et al., 2001; Fernandes et al., 2020). Lower intensity punctate staining was also observed along the entire flagellum in most parasites (Figures 5B and S5Aa, c, d and S5Bb). In this analysis, LdActin was detected both at the promastigote’s cell body and along the flagellum with the labelling of the a-LdActin Ab more evident at the pepriphery of the cell body including the area where the flagellum buds. The pellicular membrane-associated actin has been described to be closely localized with the subpellicular microtubules (sMTs) (Sahasrabuddhe et al., 2004). Consistently, co staining with the LdTyrPIP_22 rabbit pAb and a mouse Ab for Leishmania tubulin, highlighting the subpelicular microtubules, confirmed the localization of LdTyrPIP_22 at the periphery of the promastigote’s cell body (Figure S6) either subpelicularly or at the pelicular membrane.
Given the limitation of the optical resolution of confocal microscopes we cannot ascertain protein co-localization or distinguish between the subpelicular actin or microtubules and the pelicular membrane. However, quantification of the % of red pixels in the cell body (corresponding to LdTyrPIP_22 epitopes), overlapping with the green pixels (corresponding to LdActin epitopes), resulted in an estimation of 59 ± 14% (n=49) of LdTyrPIP_22 signal superimposed with the LdActin signal in all the morphological forms of promastigotes from a promastigotes’ culture. Higher levels of pixel co-localization were observed in procyclic-like/dividing and metacyclic-like parasites (60 ± 12, 63 ± 10 and 65 ± 11% respectively) while for the leptomonad- and nectomonad-like forms the overlapping pixels ranged from 50 ± 11-52 ± 14%. Therefore, from this pixel intensity analysis, a molecular proximity of LdTyrPIP_22 with LdActin is inferred with the possibility of a direct or indirect interaction.
Additional sites where LdTyrPIP_22 was detected in axenic promastigote, regardless the a-LdTyrPIP_22 pAb used were: 1) next to or surrounding the kinetoplast (the mass of concatenated mitochondrial DNA) (Figure S5, arrowheads), 2) the base or the sides of the flagellar pocket (invagination of the cell membrane forming a vase-like structure at the base of the flagellum) and 3) the proximal end of the flagellum (Figures 5 and S5, short arrowheads). Often the entire flagellar pocket was decorated by the a-LdTyrPIP_22 Ab staining (Figures S5Ac and S5Ba,b insets). In newly divided (Figure S5Ac) and dividing promastigote, identified by the two nuclei (Figure S5Ad), we also observed staining of vesicular-like structures. Co staining with the a-GAPDH antibody, GAPDH was used as a glycosome marker, showed that the LdTyrPIP_22 vesicular staining was excluded from the structures highlighted by this Ab (Figure S8). The punctate staining observed around large compartments (S5A a inset, c) could mark acidocalcisomes or megasomes (Rodrigues et al., 2014).
Overall, LdTyrPIP_22 epitopes were detected in multiple sites in the L. donovani axenic promastigote with the most prominent ones being 1) the periphery of the cell body, 2) adjacent to the kinetoplast, 3) intracellular small vesicles, 4) the proximal end of the flagelum, and 5) the flagellar pocket area.
In the in vivo situation, the differentiation process converting the Leishmania promastigote to amastigote takes place within the mammalian host macrophages when the infective metacyclic promastigote is phagocytozed and enclosed in the parasitophorous phagolysosome (Sunter and Gull, 2017). The metacyclic promastigote (MP) to the amastigote transition involves dramatic changes in cell shape and results in a minimized cell surface to volume ratio, hence reducing the area over which the cell is exposed to the harsh environment of the parasitophorous vacuole reformatting of the flagellum and also occurs. These changes require substantial membrane and cytoskeleton remodeling in which, from what is known for higher eukaryotes (Schink et al., 2016; Senju et al., 2017), PI metabolism and signaling play central roles. Therefore, we decided to monitor by microscopy the localization of the LdTyrPIP_22 over time under conditions resembling those experienced by the promastigote when enclosed in the parasitophorous phagolysome.
To this end, a stationary phase promastigote culture, 8 days post subculturing, was subjected to a temperature shift at 37°C while the pH was adjusted to 5.5. The conversion of the promastigotes’ population to amastigotes in most protocols begins 4 h after the temperature and pH shift. In the first 24 h of incubation of the axenic parasites’ culture at 37°C and pH 5.5, a mixed population of flagellated promastigotes and amastigote-like parasites with short flagella coexist. (Doyle et al., 1991; Debrabant et al., 2004; Zilberstein, 2020). Imaging of promastigotes from this population using immunofluorescence and confocal microscopy showed a systematic and drastic recruitment of the LdTyrPIP_22 towards the base of the flagellum and the flagellar pocket (Figures 6 and 7). The other two prominent localizations observed were: 1) a perinuclear punctate staining (Figures 6A, 7A–C) and 2) a vesicular staining throughout the cell body (Figure 6B). Of note, in this axenic promastigote culture we also observed LdActin in the nucleus (Figures 6A, 7A, B) as it has already been reported (Sahasrabuddhe et al., 2004).
Figure 6 Localization of LdTyrPIP_22 epitopes in axenic L. donovani promastigotes after 24 h exposure at 37°C and pH 5.5 or during a 4 h in vitro infection of J744 macrophages. (A, B) Confocal microscopy images of L. donovani stationary phase promastigotes incubated for 24 h at 37°C and pH 5.5. Images were acquired with a Leica SP8 confocal microscope by z-scanning with 0.3 μm step size. Images in (A) were acquired at 1X digital magnification while in B at 4X. (A) Double IF staining of fixed cells permeabilized with 0.1% (v/v) Τriton X-100 was performed with the a-LdTyrPIP_22 mouse pAb (1:100) and a-LdActin rabbit pAb (1:1,000) followed by secondary Abs conjugated to Alexa Fluor ® 488 or Alexa Fluor ® 546. Nuclear and kinetoplast DNA was stained with Hoechst 33342 (1:5,000). Images (max projections) of several promastigotes are presented. In the DNA stain the kinetoplast (small arrow, k) is more intensely stained than the nucleus. (B) An image of a promastigote (single z section of a z-series) acquired with a 4X magnification factor. IF staining for LdTyrPIP_22 and DNA staining were performed as in (A). The nuclear (N) and kinetoplast (k) DNA staining is presented here in red pseudo color for better contrast in merged image. (C) A J774 murine macrophage 4 h post-infection in vitro with L. donovani promastigotes imaged by confocal microscopy. Stationary phase promastigotes were added to adhered macrophages at a 20:1 ratio. IF staining of fixed cells permeabilized with 0.1% (v/v) Τriton X-100 was performed with the a-LdTyrPIP_22 mouse pAb (1:100) followed by secondary Ab conjugated to Alexa Fluor ® 488. Macrophage polymerized actin was stained with phalloidine conjugated to Alexa Fluor ® 546. Images were acquired with a Leica TCS SP5 confocal microscope by z-scanning performed at 1 μm step size. A single optical section from the top of the cell is presented. A promastigote, still extracellular in most part but in the process of being phagocytozed is shown in the insets (1.5X magnification). Single FL and phase contrast images are presented in BW while images of the three merged FL signals are shown in color. The molecule highlighted in the BW images with single color FL is indicated at the top in the same color as the respective FL signal. In (A), (B), and (C) arrowheads point to the flagellar pocket/base of flagellum. Small arrows in (A) and (B) point to the kinetoplast (k) and nucleus (N) while large arrows in (C) point to polymerized actin surrounding part of the phagosytozed parasites’ flagellum. Scale bar size in (A–C): 10 μm.
Figure 7 Localization of LdTyrPIP_22 epitopes in tationary phase L. donovani parasites after 24 h exposure at 37°C and pH 5.5. Co staining with LdActin and LdGABDH. Confocal microscopy images of L. donovani promastigote- and amastigote–like forms. Double IF staining of fixed cells permeabilized with 0.1% (v/v) TritonX-100 was performed with the anti-LdTyrPIP_22 mouse pAb (1:100) and the anti-LdActin rabbit pAb (1:1,000) in (A, B) or the anti-GAPDH rabbit pAb (1:100) in (C) followed by secondary Abs conjugated to Alexa Fluor ® 488 or Alexa Fluor ® 546. Nuclear and kinetoplast DNA was stained with Hoechst 33342 (1:5,000). In (A, C), single z-sections of axenic promastigote-like forms. Inset in (A): an amastigote-like parasite from the same field at a 2X magnification. In (B), the maximum intensity projection of an amastigote-like parasite (i.e., smaller than promastigotes, ovoid, with retracted/short flagellum). Inset in (B): a single z-section at the same magnification as the maximum intensity projection. Single FL and phase contrast images are presented in BW while images of the three FL signals merged are shown in color. The molecule highlighted in the BW images with single color FL is indicated at the top in the same color of the respective FL signal. Images were acquired with a Leica TCS SP8 confocal microscope by z-scanning performed at 0.3 μm step size. Large arrowheads point to the flagellar pocket/base of flagellum. Small arrowhead in inset (B), shows the small flagellum. Small arrows in (A–C) point to the kinetoplast (k) and nucleus (N). Scale bar size in A-C: 10 μm. Inset (A) scale bar size: 5 μm.
To refine the conditions that induce accumulation of the LdTyrPIP_22 at the promastigote’s flagellar pocket, we tested separately the effect of temperature and pH. We found that as early as 30 min after transferring the promastigote to 37°C we could detect the distinct accumulation of LdTyrPIP_22 at the promastigote’s flagellar pocket (Figure S7, arrowhed). A similar LdTyrPIP_22 distribution was observed in extracellular promastigotes in an in vitro infection assay of J744 murine macrophages exposed only to 37°C but not to low pH shift 4 h post-infection (Figure 6C, insets).
In this case the promastigotes were promastigotes grown at 25°C and pH 5.5 for 24 h did not show this characteristic recruitment of LdTyrPIP_22 at the flagellar pocket (data not shown). Moreover, in amastigote-like forms, observed in the axenic culture grown at 37°C and pH 5.5 for 24 h up to 144 h, LdTyrPIP_22 epitopes were detected at the flagellar pocket near the kinetoplast (Figures 7A inset, 7B and S9B), in vesicles (Figure S9A) or at the tip of the small flagellum barely emerging from the flagellar pocket (Figures 7B and S9B).
Concluding, at 37°C and pH 5.5, conditions resembling the ones encountered by Leishmania parasites in the mammalian hosts where the developmental transition of the MPs to amastigotes is initiated, LdTyrPIP_22 is strongly recruited to the flagellar pocket and redistributes perinuclearly.
This study provides the first experimental evidence documenting the expression of the L. donovani LDBPK_220120.1 gene product in the L. donovani parasites, a Leishmania spp. highly pathogenic to humans. The ortholog gene LmjF_22_0250 from L. major, shown to be upregulated in metacyclic L. major promastigote (Dillon et al., 2015; Inbar et al., 2017), was earlier reported to encode a protein which when expressed as recombinant in bacteria exhibited PTP and PI phosphatase activities (Beresford et al., 2010).
We cloned the LDBPK_220120.1 gene from a Sudanese L. donovani (LG13) strain and showed that its protein product, when expressed heterologously in bacteria, dephosphorylated P-Tyr peptides and monophosphorylated PIs [PI(3)P and PI(4)P], similarly to its L. major ortholog LM1 (Beresford et al., 2010), properties that classify both as atypical Dual Specificity Lipid-like phosphatases (Brenchley et al., 2007). The L. donovani aDSP, that we named LdTyrPIP_22, together with its L. major ortholog, represent to our knowledge the first PI phosphatases characterized in Leishmania spp. to date. With specific antibodies that we generated we analyzed the subcellular localization of LdTyrPIP_22 using detergent based subcellular fractionation and immunofluorescence microscopy. An array of intracellular localizations, comprising membrane and cytoskeleton associated elements, were observed by both approaches, suggesting that LdTyrPIP_22 has multiple cellular partners/substrates participating thereby in multiple cellular functions which are consistent with its identity as a dual specificity P-Tyr/PI phosphatase.
Interestingly, the recombinant LdTyrPIP_22 used in this study has a narrower substrate preference than its bacterial homologs MptpB and LipA from the ALP family (Beresford et al., 2010). MptpB from Mycobacterium tuberculosis has triple specificity, dephosphorylating P-Ser, P-Thr, and P-Tyr peptides as well as mono- and di-phosphorylated PIs (i.e., PI(3)P, P(4)P, PI(5)P, and PI(4,5)P2), (Beresford et al., 2010). LipA, from Listeria monocytogenes although it has similar substrate preference to the PIs with MptpB, it dephosphorylates only P-Tyr peptides (Beresford et al., 2010). The P-loop sequence in the catalytic site of the three proteins is almost identical with only one aa difference at position 2 (i.e., Phe161 in MptpB instead of Thr148 in rLdTyrPIP_22 and Thr188 in LipA). Point mutation studies in the MptpB (Beresford et al., 2007) showed that the nature of this aa plays an important role in the substrate specificity for PIs as well as in the levels of enzymatic activity. However, other determinants in the 3D structure of the three proteins must play critical role in the substrate preference. The bacterially expressed ortholog of LdTyrPIP_22 from L. major (i.e., LM1), additionally to the PI(3)P and PI(4)P, was shown to also dephosphorylate PI(5)P (Beresford et al., 2010). The predicted primary structures of the two proteins differ in 7 aa (Figure 1). Whether these differences play a critical role on the two enzymes’ substrate preferences remains to be shown.
Phosphorylation and dephosphorylation are well-documented post translational modifications regulating the developmental transitions of Leishmania spp. along their biological cycle (Chow et al., 2011; Tsigankov et al., 2013; Tsigankov et al., 2014; Dillon et al., 2015). Especially, phosphorylation on tyrosine residues, although comprising a small fraction of all protein phosphorylation events, plays a pivotal role in signaling, cell-cycle control, and differentiation (Cool and Blum, 1993; Nascimento et al., 2006). Indeed, inhibition of tyrosine phosphorylation in Leishmania promotes partial differentiation from promastigote to amastigote forms (Nascimento et al., 2003). Therefore tyrosine kinases and P-Tyr phosphatases can be viewed as master regulators of the Leishmania developmental cycle. The LdTyrPIP_22 phosphatase represents a putative player in these regulations with its P-Tyr phosphatase activity. The finding that the LDBPK_220120.1 ortholog gene transcription in L. major is significantly upregulated in both cultured and sandfly metacyclic promastigotes (Dillon et al., 2015; Inbar et al., 2017) suggests a need for this enzyme’s activity over the developmental transition of Leishmania from the forms adapted in the blood feeding insects’ midgut/foregut to the ones living in the mammal dermis and skin-distant organs. Moreover, the substantial upregulation (5.92 fold vs 2.63 fold) of this L. major transcript in the sandfly living metacyclics (Inbar et al., 2017) versus the cultured metacyclis (Dillon et al., 2015), points to its importance in the survival of the parasite under the stress and nutrient scarcity conditions in the anterior midgut of the fly where metacyclogenesis occurs. Moreover, the MP to the amastigote transition involves dramatic changes in cell size and shape and a reformatting of the flagellum. These changes require substantial membrane and cytoskeleton remodeling and dynamics in which, from what is known for higher eukaryotes (Schink et al., 2016; Senju et al., 2017), PI signaling and metabolism play central roles. LdTyrPIP_22 with its PI dephosphorylating activity may contribute to events resulting in these developmental transitions of Leishmania promastigotes.
In this study, the microscopy imaging of promastigotes growing at 25°C revealed LdTyrPIP_22 epitopes at the flagellar pocket region (Figures S5A small arrows, B and Ba,b insets), adjacent to the kinetoplast (Figure S5A arrowheads) where the Golgi apparatus is located (Halliday et al., 2019) and occasionally in vesicular (Figure S5A), and tubular structures (Figures S5b and S7b) resembling MTV endosomes (Wang et al., 2020). All these localizations, considering that 1) the flagellar pocket region in protozoans of the Leishmania genus is highly specialized for uptake of macromolecular nutrients and secretion via the conventional exocytic route (Landfear and Ignatushchenko, 2001) and 2) the LdTyrPIP_22 dephosphorylates selectively PI(3)P and PI(4)P, are compatible with a role for this phosphatase in endocytosis/exocytosis event(s) and/or membrane trafficking in Leishmania promastigotes. Diverse functions of PI(3)P and PI(4)P PIs in the regulation of membrane traffic and cell signaling pathways are based, at least in part, on their spatiotemporally controlled formation and turnover at defined subcellular sites (Marat and Haucke, 2016). In metazoan cells, PI 3-phosphates such as PI(3)P and PI(3,5)P2 are found predominantly in membranes of early and late endosomes or lysosomes with key importance for their function (Balla, 2013). Endosomal recycling to the cell surface requires PI(3)P hydrolysis and the concomitant generation of PI(4)P is required for exocytosis to occur (Wallroth and Haucke, 2018). PI(4)P is mainly concentrated in the exocytic pathway, in particular in the Golgi complex, the trans-Golgi network (TGN), and the plasma membrane (Balla, 2013; Hanes et al., 2017; Wallroth and Haucke, 2018). However, the Leishmania cell is largely an unmapped territory with respect to its PIs’ localization, metabolism and signaling pathways (Zhang and Beverley, 2010; Velasquez et al., 2015). In Trypanosoma brucei, one of the closest relatives of Leishmania spp. in the Trypanosomatidae family, it is known that PIs and their related kinases and phosphatases function as a regulatory system in addition to their role in the synthesis of membrane or glycoconjugate structures (Cestari, 2020). This is evidenced by the numerous cellular processes affected when genes encoding PI-related proteins or proteins generating PI metabolites are mutated or knocked out (reviewed in (Cestari, 2020)). Interestingly, in Trypanosoma brucei, PIs have diversified in function with respect to their role in metazoans to control specialized processes (Cestari and Stuart, 2018; Cestari et al., 2019). If we extrapolate from the localizations of PI(3)P and PI(4)P in metazoans and what is known for Trypanosoma brucei, LdTyrPIP_22 may be involved in endocytosis/exocytosis event(s) and/or membrane trafficking in Leishmania promastigotes.
The distinct, although not prominent, detection of LdTyrPIP_22 epitopes along the flagellum could support the hypothesis that this enzyme may also be involved in the regulation of intraflagellar transport or the flagellum’s remodeling during MP to amastigote differentiation. This could be another explanation for the LdTyrPIP_22 epitopes detected to sites adjacent to the kinetoplast, known to be connected via a tripartite attachment complex (TAC) to the flagellum basal body (Gluenz et al., 2010). Recent studies on mammalian epithelial cells have shown that PI(4)P homeostasis, coordinated by a pair of PI kinase and phosphatase at the centrosome/ciliary base, is vital for ciliogenesis (Xu et al., 2016). It is possible that a similar mechanism also exists in Leishmania cells.
Finally, the observed localization of LdTyrPIP_22 in regions enriched in LdActin, mainly at the periphery of the cell body of promastigotes growing at 25°C (Figure 5), suggests a possible role of this phosphatase in the regulation of actin cytoskeleton properties, as it is the case for a number of other phosphatases in lower (Delorme et al., 2003; Schuler and Matuschewski, 2006; Fernandez-Pol et al., 2013) and higher eukaryotes (Larsen et al., 2003; Nakashima and Lazo, 2010). From the quantitative analysis on the microscopy images of promastigotes co stained with the a-LdTyrPIP_22 and the a-LdActin specific Abs, a molecular proximity of these two proteins was inferred with the possibility of a direct or indirect interaction. This could drive a working hypothesis on a dynamic interplay between the LdTyrPIP_22 phosphatase and actin via its PI or its P-Tyr dephosphorylating activities. Interestingly, actin controls the motile behavior of the Leishmania promastigote and is involved in intracellular vesicular trafficking and flagellar-pocket organization in Trypanosomatids (Sahasrabuddhe et al., 2004; Katta et al., 2010). Both functions are compatible with the LdTyrPIP_22 localizations in the L. donovani promastigotes.
One of the most novel findings of this study was the pronounced recruitment of LdTyrPIP_22 in the flagellar pocket region when stationary phase promastigotes were subjected to temperature 37°C and pH 5.5 for 24 h (Figures 6, 7). This accumulation did not seem to be due to higher expression levels of LdTyrPIP_22 since quantification by Western Blot of the LdTyrPIP_22 protein levels in axenic L. donovani promastigotes treated as above did not show a significant difference with the levels observed in stationary phase promastigotes growing at 25°C and pH 7 (Figures 4Ba and d). Environmental signals like elevated temperature and acidity have already been shown to be crucial in triggering the developmental transition from the motile MP to the immotile amastigote inside the mammalian host macrophage (Zilberstein and Shapira, 1994; Sereno and Lemesre, 1997; Barak et al., 2005). This differentiation marked by a dramatic change in the parasite’s cell shape from the elongated slender cell body to a small ovoid shape one and from a long motile flagellum with a 9 + 2 axoneme arrangement to a short non motile flagellum with a 9 + 0 axoneme arrangement (Gluenz et al., 2010). Additionally, a large restructuring of the flagellar pocket and neck region takes place. This associates with changes in the localization of the flagellum attachment zone proteins. As a consequence, the flagellar pocket neck closes to fit tightly around the remaining short flagellum end (Sunter and Gull, 2017) the tip of which is considered to play a sensory role in the interaction with the host (Kelly et al., 2020). Moreover, the growth rate and the metabolism of the amastigotes slow down considerably (McConville et al., 2007) by the expression of stage specific survival factors (Leifso et al., 2007; Biyani and Madhubala, 2012). If the ldtyrpip_22 gene transcription is upregulated in metacyclogenesis as for its ortholog LmjF.22.0250 in L. major (Inbar et al., 2017), it may be involved in a process preparing the parasite for adaptation to the mammalian host microenvironment, part of which is the transformation to the amastigote form. Interestingly, in this study, LdTyrPIP_22 was recruited to the flagellar pocket soon after the stationary axenic promastigote culture was shifted from 25°C to 37°C (Figure S8). This recruitment was more dramatic in axenic amastigote-like L. donovani forms (Figures 7A inset, 7B, and S9B) identified in L. donovani culture subjected to temperature and low pH stress conditions for 24–144 h, pointing to a role of this phosphatase in the MP to amastigote transition. In that sense, LdTyrPIP_22 could be involved in the regulation of endo/exocytosis processes, upregulated when parasites are subjected to temperature stress (Hassani et al., 2011) but, also in mechanisms regulating flagellum disassembly with flagellar pocket maintenance through remodeling. The latter is a process that follows the demands of minimizing the amastigote cell’s total surface area as an adaptation in the phagolysosome environment (Wheeler et al., 2016). Moreover, the presence of LdTyrPIP_22 epitopes in the short flagellum tip of amastigote-like parasites (Figures 7B and S9B small arrowheads) suggests a possible role in the communication of the parasite with the parasitophorous phagosome membrane (Gluenz et al., 2010). All the above stated hypotheses merit further extensive investigation in Leishmania cells genetically engineered to overexpress LdTyrPIP_22 or dominant negative mutants of LdTyrPIP_22 or in KO parasites with the ldtyrpip_22 gene deleted.
The punctate perinucear redistribution of LdTyrPIP_22, consistently observed at different levels, resembles nuclear pores’ staining pattern. In metazoans, a large number of phosphatases have been shown to localize at the Nuclear envelope and this membrane is attracting attention as a cell’s compartment for the control and transduction of several signaling pathways (Sales Gil et al., 2018). Moreover, PIs are localized inside the nucleus and are shown to play important roles in chromatin remodeling, gene transcription, and RNA processing (Castano et al., 2019). PI kinases, phosphatases and phospholipases have also been shown to localize in the nucleus (Barlow et al., 2010). Moreover, given that LdTyrPIP_22 is a P-Tyr phosphatase as well, its nuclear localization may imply a regulatory role through P-Tyr dephophorylation in nuclear processes, as shown for certain mammalian PTPs. Multiple evidence exists for a turnover of phosphotyrosine at the nuclear envelope of intact metazoan cells (Otto et al., 2001).
An unexpected result of this study was the detection of higher amount of LdTyrPIP_22 protein in total lysates of logarithmically growing promastigotes as compared to promastigotes in stationary phase. The latter constitute the promastigotes’ population enriched in metacyclic forms in which the gene transcript of the L. major ortholog of ldtyrpip_22 (i.e., the LmjF.22.0250 gene) was observed to be upregulated 2.6 fold (Dillon et al., 2015) in axenic parasites. However, the metacyclic forms in the stationary phase promastigotes of the L. donovani complex Leishmania spp. constitute a small pool (below 6%–7%) of the total population (Santarem et al., 2014). Even if this small pool of L. donovani metacyclics expressed higher levels of LdTyrPIP_22 this would not affect significantly the levels of this protein in the total population of stationary phase promastigotes, mostly consisting of leptomonad- and nectomonad-like forms (Sunter and Gull, 2017). It remains however an interesting finding that the amount of LdTyrPIP_22 protein detected in the promastigotes’ population enriched in dividing L. donovani is ~ 2 fold higher as compared to the non-dividing population, a result that merits further investigation.
Before concluding, it worths mentioning a recent interesting study which showed that L. mexicana knock out parasites in the LmxM.22.0250 gene (i.e., the ldtyrpip_22 L. mexicana ortholog) presented severely attenuated virulence in the infection of primary mouse macrophages in vitro (Kraeva et al., 2019). This finding highlights further the importance of our study given that the LdTyrPIP_22 homologs from the pathogenic bacteria M. tuberculosis and L. monocytogenes, MptpB and LipA, are known virulence factors (Beresford et al., 2009; Kastner et al., 2011). If LdTyrPIP_22 is shown to play a role in L. donovani virulence it will gain a value as a candidate drug target, especially because it does not have a homolog in higher eukaryotes (Brenchley et al., 2007; Beresford et al., 2010). Other enzymes of PI metabolism in kinetoplastids have already gained attention as candidate antiparasitic drugs (Cestari, 2020).
To sum up, this work describing the enzymatic substrate specificity and subcellular localization of the LdTyrPIP_22 P-Tyr/PI phosphatase, the first such aDSP to be described in L. donovani, forms the basis and will serve as a compass for more detailed studies toward the exploration of this phosphatase’s functional role(s). The results of this study point to an involvement of LdTyrPIP_22 in the regulation of the endocytic/exocytic pathways and in the MP to amastigote differentiation, key processes for the parasite’s fitness to complete its life cycle. The Blast analysis we performed showing that LdTyrPIP_22 is highly conserved amongst several Leishmania spp. also highlights the importance of this phosphatase in the parasite’s life cycle. Further follow up studies using L. donovani strains with genetic modifications in the ldtyrpip_22 gene will enable exploration of the LdTyrPIP_22 putative role(s) in PI signaling in Leishmania cells. Although PIs constitute 10% of the total phospholipid content of Leishmania promastigotes (Zhang and Beverley, 2010), PI metabolism and signaling in these protozoans remain understudied and PI regulatory molecules identified by bioinformatics analyses (Velasquez et al., 2015) (Brenchley et al., 2007) remain poorly characterized. To conclude, this study emphasizes the importance of Leishmania phosphatases classified as aDSPs Lipid-like in the Trypanosomatids’ phosphatome (Brenchley et al., 2007) and as Atypical Lipid phosphatases (Beresford et al., 2010) which, given that they are highly divergent from human homologs or have no human homologs at all, may prove to be valuable candidate drug targets for human leishmaniasis treatment, a goal of strong urgency.
The datasets presented in this study can be found in online repositories. The names of the repository/repositories and accession number(s) can be found in the article/Supplementary Material.
The animal study was reviewed and approved by the Hellenic Pasteur Institute's Institutional Animal Bioethics Committee following the EU Directive 2010/63 and the National Law 2013/56.
Conceived and designed the experiments: HB, AP, PR, OT, MK, and IT. Performed the experiments: AP, AK, OT, PK, and AD. Analyzed the data: AP, AK, PR, PK, MK, and HB. Contributed reagents/materials/analysis tools: HB, MK, and IT. Wrote the paper: AP and HB. Edited the paper: MK, IT, OT, and PK. Revised the paper: HB and OT. All authors contributed to the article and approved the submitted version.
This work was a Bilateral Research & Technology Collaboration Greece-France 2013 grant (no 1811) funded by the Greek General Secretariat for Research and Technology (http://www.gsrt.gr/central.aspx?sId=119I428I1089I323I488743) (HB and IT); IKYDA, the bilateral research promotion program, concluded between the DAAD with Greece and the Greek State Scholarship Foundation (IKY) (2014-2015) (HB and MK); KRIPIS I & II (2013-2019) Development Grants for Research Institutions, funded by the Greek General Secretariat for Research and Technology (HB), the Hellenic Foundation for Research and Innovation (HFRI) under the HFRI PhD Fellowship grant (Fellowship Number: 606) and the “BIOIMAGING-GR: A Greek Research Infrastructure for Visualizing and Monitoring Fundamental Biological Processes (MIS 5002755)”, funded by the Operational Program “Competitiveness, Entrepreneurship and Innovation” (NSRF 2014-2020), co-financed by Greece and the European Union (European Regional Development Fund). The funders had no role in study design, data collection, and analysis, decision to publish, or preparation of the manuscript.
The authors declare that the research was conducted in the absence of any commercial or financial relationships that could be construed as a potential conflict of interest.
Microscopy work was performed in the Light Microscopy unit of the Hellenic Pasteur Institute (HPI-LMU). The technical help of Dr Evangelia Xingi and Mrs Virginie Gonzalez is highly acknowledged. Special thanks to Dr Marios Zouridakis for his assistance with the FPLC experiments. The polyclonal Abs were generated in the Department of Animal Models for Biomedical Research of the Hellenic Pasteur Institute. We thank Dr Amogh Sahasrabuddhe (Division of Molecular and Structural Biology Central Drug Research Institute Lucknow, India) for the a-LdActin rabbit pAb and Dr Frédéric Bringaud (U. of Bordeaux/CNRS, France) for the a-GAPDH pAb. Special thanks to Dr Genevieve Milon for fruitful discussions and critical editing suggestions for this manuscript.
The Supplementary Material for this article can be found online at: https://www.frontiersin.org/articles/10.3389/fcimb.2021.591868/full#supplementary-material
Supplementary Figure 1 | Bioinformatics analysis of the LdTyrPIP_22 active site including the P-loop region HCXXGKDR. (A) ClustalW multiple sequence alignment of the L. donovani including the P-loop (strain LG13) sequence with homologs from Mycobacterium tuberculosis (MptpB, GenBank: CCC62750.1), Listeria monocytogenes [lmo1800, NP_465325; lmo1935, NP_465459)], Yersinia pestis KIM10+ (WP_002228183), and Bacillus anthracis strain Ames (NP_845680). The aa sequences were obtained from the Genbank database and were analyzed/edited using the software BioEdit Sequence Alignment Editor. P-loop signature amino acids are framed by a blue box; (B) Rooted phylogenetic tree (UPGMA) from a multiple sequence alignment of the LdTyrPIP_22 orthologs from pathogenic bacteria.
Supplementary Figure 2 | Assessment of solubility of the LdTyrPIP_22-His and 15N-LdTyrPIP_22-His recombinant proteins in E. coli BL21 lysates. (A)The solubility of the rLdTyrPIP_22-His produced in bacteria was tested after 16 h induction with 0.1 mM IPTG at 20 oC, 25 oC and 37 oC. At the end of the induction, bacteria were lysed by sonication, soluble and insoluble fractions were separated by centrifugation (14 000×g, 30 min, 4 °C) and the insoluble fraction was resuspended in the same volume of lysis buffer. Equal volumes from total bacteria lysates (lane 1), soluble faction (lane 2) and insoluble fraction (lane 3) were analyzed by SDS-PAGE (12% w/v) followed by WB using the a-LdTyrPIP_22 mouse serum (1:1200). The positions of the MW markers (kDa) are indicated on the left and right of the panels. Two protein species were revealed to be induced, recovered mostly in the insoluble fraction at all 3 temperatures; (B) The solubility of 15N-LdTyrPIP_22 produced by bacteria was tested after 16 h induction at 25 °C without IPTG. Equal volumes from total bacteria lysates (lane 1) soluble faction (lane 2) and insoluble fraction (lane 3) were analyzed by SDS-PAGE (12% w/v) followed by WB using the a-His mAb (1.0 μg/mL). The arrow indicates the protein band corresponding to 15N-LdTyrPIP_22 in the part of the blot within the 30-45 kDa range; (C) Testing the effect of the length of induction to the solubility of 15N-LdTyrPIP_22 in bacteria lysates. WB of the soluble and pellet fractions from BL21-pTriEx-N15-ldtyrpip_22after incubation at 25 °C for 16 h and addition of IPTG (0.5 mM) for the indicated hours. Proteins were analyzed on 12% w/v SDS PAGE and transferred to nitrocellulose which was subsequently incubated with the a-His mAb (1.0 μg/mL). S: soluble supernatant, P: insoluble pellet. Arrow indicates the protein band corresponding to 15N-LdTyrPIP_22.
Supplementary Figure 3 | (A, B), Time course of dephosphorylation of four different PIs by the bacterially expressed recombinant human PRL-3 phosphatase (A), or two P-Tyr peptides (1 mM) by the bacterially expressed recombinant human PTP1B (B). The aa sequences for the pTyr2 and pTyr4 peptides are shown in Table S3. The assays were performed as described in Figure 2. (C), Effect of pH in the rN15-LdTyrPIP_22-8His phosphatase activity at 37°C. The rN15-LdTyrPIP_22-8His phosphatase activity was assayed in 1 ml reaction volume with pNPP as substrate at 37°C (pH 4-8) and at 25°C (pH 6) in parallel as described in Materials and Methods. The absorbance of the reaction product (p-nitrophenolate = pNP) in the supernatant was measured at λ=405 nm. Error bars represent standard deviations calculated from results acquired from four independent experiments performed in duplicates with three different batches of purified recombinant protein.
Supplementary Figure 4 | Testing of a-LdTyrPIP_22 Abs’ reactivity (A) Western Blot analysis of the a-LdTyrPIP_22 pAbs reactivity (rabbit and mouse polyclonals) in detecting the rN15-LdTyrPIP_22-His protein produced in E. coli BL21 cells carrying the pTriEx1.1-rN15-ldtyrpip_22plasmid. Bacteria were grown at 25oC for 16 h and induced with 0.5 mM IPTG (4 h, 25oC) to express the rN15-LdTyrPIP_22-His protein. Subsequently, the rN15-LdTyrPIP_22-His protein was affinity purified on Ni2+ NTA agarose beads from the bacteria lysates (Materials and Methods). Bacteria lysates (lane 1, left panel) or Ni2+ NTA beads with rN15-LdTyrPIP_22-His bound on them (lane 2, left panel) were analyzed by 12% SDS-PAGE and transferred on nitrocellulose membranes. Membrane strips with bacteria lysates were probed with the affinity purified rabbit pAb [0.2 & 0,1 μg/ml from the 5th and 1st bleed, (i & ii respectively, middle panel)]. Additionally strips with rN15-LdTyrPIP_22-His (material shown in lane 2 of left panel) were probed with the mouse pAb [dilution 1:100 (iii) & 1:1000 (iv) right panel]. HRP conjugated a-rabbit and a-mouse pAbs were used as secondary Abs. In all the panels the Ab’s reactivity was revealed by the chromogenic DAB method. Arrow heads point to the rN15-LdTyrPIP_22-His band. (B) The a-LdTyrPIP_22 pAbs (rabbit and mouse) were used to detect LdTyrPIP_22 epitopes in axenic L. donovani static phase promastigotes by IF and confocal microscopy. IF staining of fixed cells permeabilized with 0.1% (v/v) Triton X-100 was performed with the a-LdTyrPIP_22 mouse pAb (1:250) and the a-LdTyrPIP_22 rabbit pAb (1:70) used together. As secondary Abs were used a-mouse Ab conjugated to Alexa Fluor® 633 and an a-rabbit Alexa Fluor 546 respectively. Nuclear and kinetoplast DNA was stained with DAPI. Images were acquired with a TCS SP confocal microscopy by z-scanning performed at 0.3 μm step size. Single optical sections from representative images are shown. Single FL and phase contrast images are presented in Black and White (BW) while merged FL images are shown in color. The 1st Ab used in each case or the dye used for staining are shown at the top in color corresponding to the respective FL signal. Arrow heads point to the flagellar pocket/beginning of flagellum while arrows point to kinetoplast (k) and Nucleus (N). Scale bar size: 8 μm.
Supplementary Figure 5 | Localization of LdTyrPIP_22 epitopes in different cell cycle morphological forms of axenic L. donovani promastigotes growing at 25oC, pH 7. Confocal microscopy images of L. donovani promastigotes. IF staining of fixed cells permeabilized with 0.1% (v/v) Triton X-100 was performed with the a-LdTyrPIP_22 mouse (1:250) (A) or rabbit (1:70) (B) pAbs followed by the corresponding secondary Abs conjugated to Alexa Fluor® 488. Nuclear and kinetoplast DNA was stained with PI. Images were acquired by z-scanning with the TCS-SP confocal microscope at 0.5 μm step size. Single z sections are shown; Details in B depict 2X magnifications of the framed areas. Phase contrast and FL images are presented in BW. Merged images of FL or Phase and FL are shown in color. The molecule highlighted in each image or the dye used for staining is indicated at the top in the same color of the respective FL signal. Arrow heads point to the kinetoplast while short arrows point to the flagellar pocket. Long arrows point to the kinetoplast (k) and Nucleus (N). Scale bar size: 8 μm
Supplementary Figure 6 | Localization of LdTyrPIP_22 epitopes in promastigotes growing at 25oC, pH 7; co staining for LdTubulin. Confocal microscopy images of axenic L. donovani promastigotes from logarithmic (A) and stationary phase (B, C) cultures growing at 25oC and pH 7. IF staining of fixed cells permeabilized with 0.1% (v/v) Triton X-100 was performed with the a-LdTyrPIP_22 rabbit pAb (2 μg/ml) and a-LdTubulin mouse mAb (1:2000) followed by an a-rabbit Alexa Fluor 546 and an a-mouse Ab conjugated to Alexa Fluor® 488 respectively. Nuclear and kinetoplast DNA was stained with Hoechst 33342 (1:5000). Images were acquired with the Leica TCS SP8 confocal microscope by z-scanning performed at 0.3 μm step size. A single optical section of a procyclic-like promastigote with two flagella is presented in (A) while in (B) and (C) are shown max projections of 7 optical sections from a leptomonad-like (B) and a nectomonad-like (C) promastigotes. Insets in (A): the framed area with the FL signal intensified so that the two flagella are distinguished. Single FL images are shown in BW and merged in color. The molecule highlighted in each image or the dye used for staining is indicated at the top in the color of the respective FL signal. Arrowheads point to the beginning of flagellum/flagelar pocket region. Arrows point to kinetoplast (k) and Nucleus (N). Scale bar size: 10 μm.
Supplementary Figure 7 | Localization of LdTyrPIP_22 in stationary phase L. donovani promastigotes stained with the FM4-64FX marker of the endocytic pathway. Confocal microscopy images of axenic L. donovani promastigotes grown for 192 h at 25oC, (pH 7) and stained alive with the FM4-64FX red fluorescent dye (20 μg/ml, Molecular Probes F34653) for 1 min at 4oC (A) or 5 min at 25oC (B). After removing the dye, promastigotes were washed with ice-cold PBS and fixed with PFA 2% w/v in PBS for 10 min on ice. IF staining of fixed cells permeabilized with 0.1% (v/v) Triton X-100 was performed with the a-LdTyrPIP_22 mouse pAb (1:200) followed by an a-mouse Ab conjugated to Alexa Fluor® 488. The molecule highlighted in each image or the dye used for staining is indicated at the top in the same color as the respective FL signal. Nuclear and kinetoplast DNA was stained with Hoechst 33342 (1:5000). Images were acquired with the Leica TCS SP8 confocal microscope by z-scanning performed at 0.3 μm step size. Single z-sections are shown. Arrowheads point to the beginning of flagellum/flagelar pocket region. Arrows point to kinetoplast (k) and Nucleus (N). Scale bar size: 10 μm.
Supplementary Figure 8 | Localization of LdTyrPIP_22 in axenic stationary phase L. donovani promastigotes after a quick thermal shift at 37oC. Confocal microscopy images of L. donovani grown for 192 h at 25oC (pH 7) and after a 15 min incubation at 37oC (pH 7). IF staining of fixed cells permeabilized with 0.1% (v/v) TritonX-100 was performed with the a-LdTyrPIP_22 mouse pAb (1:200) and a-GAPDH rabbit pAb (1:200) followed by an a-mouse Ab conjugated to Alexa Fluor® 488 and an a-rabbit Alexa Fluor 633 respectively (here presented in red pseudocolor in the merged image). Nuclear and kinetoplast DNA was stained with Hoechst 33342 (1:5000). Single color FL images are presented in BW while merged FL or Phase-FL signals are shown in color. The molecule highlighted in each image or the dye used for staining is indicated at the top in the same color as the respective FL signal. Images were acquired with the Leica TCS SP8 confocal microscope by z-scanning performed at 0.3 μm step size. Single optical sections are shown. Arrowheads point to the beginning of flagellum/flagelar pocket region. Arrows point to kinetoplast (k) and Nucleus (N). Scale bar size: 10 μm.
Supplementary Figure 9 | Localization of LdTyrPIP_22 epitopes in axenic amastigote-like forms; co staining for LdTubulin. Confocal microscopy images of L. donovani grown for 5 days at 37oC and pH 5.5. IF staining of fixed cells permeabilized with 0.1% (v/v) TritonX-100 was performed as described in Figure S6. The molecule highlighted in each image or the dye used for staining is indicated at the top in the same color as the respective FL signal. Single FL and phase contrast images are presented in BW. Images were acquired with a Leica TCS SP5 confocal microscope by z-scanning performed at 0.3 μm step size. Single z-sections are shown. (A a, b): Two different z sections of the same parasite. Arrow heads point to the remaining short flagellum. (B) A dividing parasite with amastigote-like morphology. Arrow head points to the tip of the short flagellum. Scale bar size: 5 μm.
LdTyrPIP_22, Leishmania donovani Tyrosine and Phosphoinositide Phosphatase in chromosome 22; DSP, dual-specificity phosphatase; aDSP, atypical dual-specificity phosphatase; PTPs, Protein Tyrosine Phosphatases; P-Tyr; phosphotyrosine; P-Ser, phosphoserine; P-Thr, phosphotheronine; PI(s), phosphoinositide(s); ALPs, atypical lipid phosphatases; PIPs, PI phosphatases; wt, wild type; ORF, open reading frame; aa, amino acids; Ab, antibody; pAb, polyclonal antibody; mRFP1, monomeric red fluorescent protein; GFP, green fluorescent protein; PFA, paraformaldehyde; ATCC, American Type Culture Collection; MW, Molecular Weight; WB, western blot; h, hours; PI(3)P, phosphatidylinositol 3‐phosphate; PI(4)P, phosphatidylinositol 4‐phosphate; PI(3,5)P2, phosphatidylinositol 3,5‐bisphosphate; IF, immunofluorescence; FL, Fluorescence; BW, black and white; KO, knock out.
Akhoundi, M., Kuhls, K., Cannet, A., Votypka, J., Marty, P., Delaunay, P., et al. (2016). A Historical Overview of the Classification, Evolution, and Dispersion of Leishmania Parasites and Sandflies. PLoS Negl. Trop. Dis. 10 (3), e0004349. doi: 10.1371/journal.pntd.0004349
Akhoundi, M., Downing, T., Votypka, J., Kuhls, K., Lukes, J., Cannet, A., et al. (2017). Leishmania infections: Molecular targets and diagnosis. Mol. Aspects Med. 57, 1–29. doi: 10.1016/j.mam.2016.11.012
Balla, T. (2013). Phosphoinositides: tiny lipids with giant impact on cell regulation. Physiol. Rev. 93 (3), 1019–1137. doi: 10.1152/physrev.00028.2012
Barak, E., Amin-Spector, S., Gerliak, E., Goyard, S., Holland, N., Zilberstein, D. (2005). Differentiation of Leishmania donovani in host-free system: analysis of signal perception and response. Mol. Biochem. Parasitol. 141 (1), 99–108. doi: 10.1016/j.molbiopara.2005.02.004
Barlow, C. A., Laishram, R. S., Anderson, R. A. (2010). Nuclear phosphoinositides: a signaling enigma wrapped in a compartmental conundrum. Trends Cell Biol. 20 (1), 25–35. doi: 10.1016/j.tcb.2009.09.009
Bates, P. A., Robertson, C. D., Tetley, L., Coombs, G. H. (1992). Axenic cultivation and characterization of Leishmania mexicana amastigote-like forms. Parasitology 105 (Pt 2), 193–202. doi: 10.1017/s0031182000074102
Bates, P. A. (1994). The developmental biology of Leishmania promastigotes. Exp. Parasitol. 79 (2), 215–218. doi: 10.1006/expr.1994.1084
Bates, P. A. (2007). Transmission of Leishmania metacyclic promastigotes by phlebotomine sand flies. Int. J. Parasitol. 37 (10), 1097–1106. doi: 10.1016/j.ijpara.2007.04.003
Beresford, N., Patel, S., Armstrong, J., Szoor, B., Fordham-Skelton, A. P., Tabernero, L. (2007). MptpB, a virulence factor from Mycobacterium tuberculosis, exhibits triple-specificity phosphatase activity. Biochem. J. 406 (1), 13–18. doi: 10.1042/BJ20070670
Beresford, N. J., Mulhearn, D., Szczepankiewicz, B., Liu, G., Johnson, M. E., Fordham-Skelton, A., et al. (2009). Inhibition of MptpB phosphatase from Mycobacterium tuberculosis impairs mycobacterial survival in macrophages. J. Antimicrob. Chemother. 63 (5), 928–936. doi: 10.1093/jac/dkp031
Beresford, N., Saville, C., Bennett, H., Roberts, I., Tabernero, L. (2010). A new family of phosphoinositide phosphatases in microorganisms: identification and biochemical analysis. BMC Genomics 11 (1), 457. doi: 10.1186/1471-2164-11-457
Biyani, N., Madhubala, R. (2012). Quantitative proteomic profiling of the promastigotes and the intracellular amastigotes of Leishmania donovani isolates identifies novel proteins having a role in Leishmania differentiation and intracellular survival. Biochim. Biophys. Acta 1824 (12), 1342–1350. doi: 10.1016/j.bbapap.2012.07.010
Brenchley, R., Tariq, H., McElhinney, H., Szoor, B., Huxley-Jones, J., Stevens, R., et al. (2007). The TriTryp phosphatome: analysis of the protein phosphatase catalytic domains. BMC Genomics 8, 434. doi: 10.1186/1471-2164-8-434
Burza, S., Croft, S. L., Boelaert, M. (2018). Leishmaniasis. Lancet 392 (10151), 951–970. doi: 10.1016/S0140-6736(18)31204-2
Castano, E., Yildirim, S., Faberova, V., Krausova, A., Ulicna, L., Paprckova, D., et al. (2019). Nuclear Phosphoinositides-Versatile Regulators of Genome Functions. Cells 8 (7):649. doi: 10.3390/cells8070649
Cestari, I., Stuart, K. (2018). Inositol phosphate pathway controls transcription of telomeric expression sites in trypanosomes. Proc. Natl. Acad. Sci. U. S. A. 112 (21), E2803–E2812. doi: 10.1073/pnas.1501206112
Cestari, I., McLeland-Wieser, H., Stuart, K. (2019). Nuclear Phosphatidylinositol 5-Phosphatase Is Essential for Allelic Exclusion of Variant Surface Glycoprotein Genes in Trypanosomes. Mol. Cell Biol. 39 (3). e00395-18 doi: 10.1128/MCB.00395-18
Cestari, I. (2020). Phosphoinositide signaling and regulation in Trypanosoma brucei: Specialized functions in a protozoan pathogen. PLoS Pathog. 16 (1), 1–8. doi: 10.1371/journal.ppat.1008167
Chow, C., Cloutier, S., Dumas, C., Chou, M. N., Papadopoulou, B. (2011). Promastigote to amastigote differentiation of Leishmania is markedly delayed in the absence of PERK eIF2alpha kinase-dependent eIF2alpha phosphorylation. Cell Microbiol. 13 (7), 1059–1077. doi: 10.1111/j.1462-5822.2011.01602.x
Cool, D. E., Blum, J. J. (1993). Protein tyrosine phosphatase activity in Leishmania donovani. Mol. Cell Biochem. 127-128, 143–149. doi: 10.1007/BF01076765
Costes, S. V., Daelemans, D., Cho, E. H., Dobbin, Z., Pavlakis, G., Lockett, S. (2004). Automatic and quantitative measurement of protein-protein colocalization in live cells. Biophys. J. 86 (6), 3993–4003. doi: 10.1529/biophysj.103.038422
Coutinho-Abreu, I. V., Serafim, T. D., Meneses, C., Kamhawi, S., Oliveira, F., Valenzuela, J. G. (2020). Distinct gene expression patterns in vector-residing Leishmania infantum identify parasite stage-enriched markers. PloS Negl. Trop. Dis. 14 (3), e0008014. doi: 10.1371/journal.pntd.0008014
De Pablos, L. M., Ferreira, T. R., Walrad, P. B. (2016). Developmental differentiation in Leishmania lifecycle progression: post-transcriptional control conducts the orchestra. Curr. Opin. Microbiol. 34, 82–89. doi: 10.1016/j.mib.2016.08.004
de Souza, W., Barrias, E. S. (2020). Membrane-bound extracellular vesicles secreted by parasitic protozoa: cellular structures involved in the communication between cells. Parasitol Res. 119 (7), 2005–2023. doi: 10.1007/s00436-020-06691-7
Debrabant, A., Joshi, M. B., Pimenta, P. F., Dwyer, D. M. (2004). Generation of Leishmania donovani axenic amastigotes: their growth and biological characteristics. Int. J. Parasitol. 34 (2), 205–217. doi: 10.1016/j.ijpara.2003.10.011
Delorme, V., Cayla, X., Faure, G., Garcia, A., Tardieux, I. (2003). Actin dynamics is controlled by a casein kinase II and phosphatase 2C interplay on Toxoplasma gondii Toxofilin. Mol. Biol. Cell 14 (5), 1900–1912. doi: 10.1091/mbc.e02-08-0462
Depledge, D. P., Evans, K. J., Ivens, A. C., Aziz, N., Maroof, A., Kaye, P. M., et al. (2009). Comparative expression profiling of Leishmania: modulation in gene expression between species and in different host genetic backgrounds. PloS Negl. Trop. Dis. 3 (7), e476. doi: 10.1371/journal.pntd.0000476
Desjardins, M., Descoteaux, A. (1997). Inhibition of phagolysosomal biogenesis by the Leishmania lipophosphoglycan. J. Exp. Med. 185 (12), 2061–2068. doi: 10.1084/jem.185.12.2061
Dillon, L. A., Okrah, K., Hughitt, V. K., Suresh, R., Li, Y., Fernandes, M. C., et al. (2015). Transcriptomic profiling of gene expression and RNA processing during Leishmania major differentiation. Nucleic Acids Res. 43 (14), 6799–6813. doi: 10.1093/nar/gkv656
Dong, G., Filho, A. L., Olivier, M. (2019). Modulation of Host-Pathogen Communication by Extracellular Vesicles (EVs) of the Protozoan Parasite Leishmania. Front. Cell Infect. Microbiol. 9, 100. doi: 10.3389/fcimb.2019.00100
Dostalova, A., Volf, P. (2012). Leishmania development in sand flies: parasite-vector interactions overview. Parasit. Vectors 5, 276. doi: 10.1186/1756-3305-5-276
Doukas, A., Karena, E., Botou, M., Papakostas, K., Papadaki, A., Tziouvara, O., et al. (2019). Heterologous expression of the mammalian sodium-nucleobase transporter rSNBT1 in Leishmania tarentolae. Biochim. Biophys. Acta Biomembr. 1861 (9), 1546–1557. doi: 10.1016/j.bbamem.2019.07.001
Doyle, P. S., Engel, J. C., Pimenta, P. F., da Silva, P. P., Dwyer, D. M. (1991). Leishmania donovani: long-term culture of axenic amastigotes at 37 degrees C. Exp. Parasitol. 73 (3), 326–334. doi: 10.1016/0014-4894(91)90104-5
Fernandes, A. C. S., Soares, D. C., Neves, R. F. C., Koeller, C. M., Heise, N., Adade, C. M., et al. (2020). Endocytosis and Exocytosis in Leishmania amazonensis Are Modulated by Bromoenol Lactone. Front. Cell Infect. Microbiol. 10, 39. doi: 10.3389/fcimb.2020.00039
Fernandez-Pol, S., Slouka, Z., Bhattacharjee, S., Fedotova, Y., Freed, S., An, X., et al. (2013). A bacterial phosphatase-like enzyme of the malaria parasite Plasmodium falciparum possesses tyrosine phosphatase activity and is implicated in the regulation of band 3 dynamics during parasite invasion. Eukaryot. Cell 12 (9), 1179–1191. doi: 10.1128/EC.00027-13
Foucher, A. L., Papadopoulou, B., Ouellette, M. (2006). Prefractionation by digitonin extraction increases representation of the cytosolic and intracellular proteome of Leishmania infantum. J. Proteome Res. 5 (7), 1741–1750. doi: 10.1021/pr060081j
Ghedin, E., Debrabant, A., Engel, J. C., Dwyer, D. M. (2001). Secretory and endocytic pathways converge in a dynamic endosomal system in a primitive protozoan. Traffic 2 (3), 175–188. doi: 10.1034/j.1600-0854.2001.020304.x
Ghorbani, M., Farhoudi, R. (2018). Leishmaniasis in humans: drug or vaccine therapy? Drug Des. Devel. Ther. 12, 25–40. doi: 10.2147/DDDT.S146521
Glaser, T. A., Wells, S. J., Spithill, T. W., Pettitt, J. M., Humphris, D. C., Mukkada, A. J. (1990). Leishmania major and L. donovani: a method for rapid purification of amastigotes. Exp. Parasitol. 71 (3), 343–345. doi: 10.1016/0014-4894(90)90039-f
Gluenz, E., Ginger, M. L., McKean, P. G. (2010). Flagellum assembly and function during the Leishmania life cycle. Curr. Opin. Microbiol. 13 (4), 473–479. doi: 10.1016/j.mib.2010.05.008
Goldstein, B. J. (2001). Protein-tyrosine phosphatase 1B (PTP1B): a novel therapeutic target for type 2 diabetes mellitus, obesity and related states of insulin resistance. Curr. Drug Targets Immune Endocr. Metabol. Disord. 1 (3), 265–275. doi: 10.2174/1568008013341163
Gupta, N., Goyal, N., Rastogi, A. K. (2001). In vitro cultivation and characterization of axenic amastigotes of Leishmania. Trends Parasitol. 17 (3), 150–153. doi: 10.1016/S1471-4922(00)01811-0
Halliday, C., Billington, K., Wang, Z., Madden, R., Dean, S., Sunter, J. D., et al. (2019). Cellular landmarks of Trypanosoma brucei and Leishmania mexicana. Mol. Biochem. Parasitol. 230, 24–36. doi: 10.1016/j.molbiopara.2018.12.003
Handman, E., Bullen, D. V. (2002). Interaction of Leishmania with the host macrophage. Trends Parasitol. 18 (8), 332–334. doi: 10.1016/S1471-4922(02)02352-8
Hanes, C. M., D’Amico, A. E., Ueyama, T., Wong, A. C., Zhang, X., Hynes, W. F., et al. (2017). Golgi-Associated Protein Kinase C-epsilon Is Delivered to Phagocytic Cups: Role of Phosphatidylinositol 4-Phosphate. J. Immunol. 199 (1), 271–277. doi: 10.4049/jimmunol.1700243
Hassani, K., Antoniak, E., Jardim, A., Olivier, M. (2011). Temperature-induced protein secretion by Leishmania mexicana modulates macrophage signalling and function. PLoS One 6 (5), e18724. doi: 10.1371/journal.pone.0018724
Herman, M., Perez-Morga, D., Schtickzelle, N., Michels, P. A. (2008). Turnover of glycosomes during life-cycle differentiation of Trypanosoma brucei. Autophagy 4 (3), 294–308. doi: 10.4161/auto.5443
Iantorno, S. A., Durrant, C., Khan, A., Sanders, M. J., Beverley, S. M., Warren, W. C., et al. (2017). Gene Expression in Leishmania Is Regulated Predominantly by Gene Dosage. mBio 8 (5). doi: 10.1128/mBio.01393-17
Inbar, E., Hughitt, V. K., Dillon, L. A., Ghosh, K., El-Sayed, N. M., Sacks, D. L. (2017). The Transcriptome of Leishmania major Developmental Stages in Their Natural Sand Fly Vector. MBio 8 (2), 00029-17. doi: 10.1128/mBio.00029-17
Karamysheva, Z. N., Gutierrez Guarnizo, S. A., Karamyshev, A. L. (2020). Regulation of Translation in the Protozoan Parasite Leishmania. Int. J. Mol. Sci. 21 (8), 2981. doi: 10.3390/ijms21082981
Kastner, R., Dussurget, O., Archambaud, C., Kernbauer, E., Soulat, D., Cossart, P., et al. (2011). LipA, a tyrosine and lipid phosphatase involved in the virulence of Listeria monocytogenes. Infect. Immun. 79 (6), 2489–2498. doi: 10.1128/IAI.05073-11
Katta, S. S., Tammana, T. V., Sahasrabuddhe, A. A., Bajpai, V. K., Gupta, C. M. (2010). Trafficking activity of myosin XXI is required in assembly of Leishmania flagellum. J. Cell Sci. 123 (Pt 12), 2035–2044. doi: 10.1242/jcs.064725
Kelly, F. D., Sanchez, M. A., Landfear, S. M. (2020). Touching the Surface: Diverse Roles for the Flagellar Membrane in Kinetoplastid Parasites. Microbiol. Mol. Biol. Rev. 84 (2), e00079-19. doi: 10.1128/MMBR.00079-19
Koul, A., Choidas, A., Treder, M., Tyagi, A. K., Drlica, K., Singh, Y., et al. (2000). Cloning and characterization of secretory tyrosine phosphatases of Mycobacterium tuberculosis. J. Bacteriol. 182 (19), 5425–5432. doi: 10.1128/JB.182.19.5425-5432.2000
Kraeva, N., Lestinova, T., Ishemgulova, A., Majerova, K., Butenko, A., Vaselek, S., et al. (2019). LmxM.22.0250-Encoded Dual Specificity Protein/Lipid Phosphatase Impairs Leishmania mexicana Virulence In Vitro. Pathogens 8 (4), 241–255. doi: 10.3390/pathogens8040241
Landfear, S. M., Ignatushchenko, M. (2001). The flagellum and flagellar pocket of trypanosomatids. Mol. Biochem. Parasitol. 115 (1), 1–17. doi: 10.1016/S0166-6851(01)00262-6
Larsen, M., Tremblay, M. L., Yamada, K. M. (2003). Phosphatases in cell-matrix adhesion and migration. Nat. Rev. Mol. Cell Biol. 4 (9), 700–711. doi: 10.1038/nrm1199
Leifso, K., Cohen-Freue, G., Dogra, N., Murray, A., McMaster, W. R. (2007). Genomic and proteomic expression analysis of Leishmania promastigote and amastigote life stages: the Leishmania genome is constitutively expressed. Mol. Biochem. Parasitol. 152 (1), 35–46. doi: 10.1016/j.molbiopara.2006.11.009
Marat, A. L., Haucke, V. (2016). Phosphatidylinositol 3-phosphates-at the interface between cell signalling and membrane traffic. EMBO J. 35 (6), 561–579. doi: 10.15252/embj.201593564
Mauel, J. (1996). Intracellular survival of protozoan parasites with special reference to Leishmania spp., Toxoplasma gondii and Trypanosoma cruzi. Adv. Parasitol. 38, 1–51. doi: 10.1016/S0065-308X(08)60032-9
McConville, M. J., de Souza, D., Saunders, E., Likic, V. A., Naderer, T. (2007). Living in a phagolysosome; metabolism of Leishmania amastigotes. Trends Parasitol. 23 (8), 368–375. doi: 10.1016/j.pt.2007.06.009
McNicoll, F., Drummelsmith, J., Muller, M., Madore, E., Boilard, N., Ouellette, M., et al. (2006). A combined proteomic and transcriptomic approach to the study of stage differentiation in Leishmania infantum. Proteomics 6 (12), 3567–3581. doi: 10.1002/pmic.200500853
McParland, V., Varsano, G., Li, X., Thornton, J., Baby, J., Aravind, A., et al. (2006). The metastasis-promoting phosphatase PRL-3 shows activity toward phosphoinositide. Biochemistry 50 (35), 7579–7590. doi: 10.1021/bi201095
Moore, I., Murphy, A. (2009). Validating the location of fluorescent protein fusions in the endomembrane system. Plant Cell 21 (6), 1632–1636. doi: 10.1105/tpc.109.068668
Morales, M. A., Pescher, P., Spath, G. F. (2010). Leishmania major MPK7 protein kinase activity inhibits intracellular growth of the pathogenic amastigote stage. Eukaryot. Cell 9 (1), 22–30. doi: 10.1128/EC.00196-09
McParland, V., Varsano, G., Li, X., Thornton, J., Baby, J., Aravind, A., et al. (2011). The metastasis-promoting phosphatase PRL-3 shows activity toward phosphoinositides. Biochemistry 50 (35), 7579–7590. doi: 10.1021/bi201095z
Nakashima, M., Lazo, J. S. (2010). Phosphatase of regenerating liver-1 promotes cell migration and invasion and regulates filamentous actin dynamics. J. Pharmacol. Exp. Ther. 334 (2), 627–633. doi: 10.1124/jpet.110.167809
Nascimento, M., Abourjeily, N., Ghosh, A., Zhang, W. W., Matlashewski, G. (2003). Heterologous expression of a mammalian protein tyrosine phosphatase gene in Leishmania: effect on differentiation. Mol. Microbiol. 50 (5), 1517–1526. doi: 10.1046/j.1365-2958.2003.03811.x
Nascimento, M., Zhang, W. W., Ghosh, A., Houston, D. R., Berghuis, A. M., Olivier, M., et al. (2006). Identification and characterization of a protein-tyrosine phosphatase in Leishmania: Involvement in virulence. J. Biol. Chem. 281 (47), 36257–36268. doi: 10.1074/jbc.M606256200
Nayak, A., Akpunarlieva, S., Barrett, M., Burchmore, R. (2018). A defined medium for Leishmania culture allows definition of essential amino acids. Exp. Parasitol. 185, 39–52. doi: 10.1016/j.exppara.2018.01.009
Otto, H., Dreger, M., Bengtsson, L., Hucho, F. (2001). Identification of tyrosine-phosphorylated proteins associated with the nuclear envelope. Eur. J. Biochem. 268 (2), 420–428. doi: 10.1046/j.1432-1033.2001.01901.x
Papadaki, A., Boleti, H. (2019). Measurement of Acid Ecto-phosphatase Activity in Live Leishmania donovani Parasites. Bio-protocol. 9 (19), e3384 doi: 10.21769/BioProtoc.3384
Papadaki, A., Politou, A. S., Smirlis, D., Kotini, M. P., Kourou, K., Papamarcaki, T., et al. (2015). The Leishmania donovani histidine acid ecto-phosphatase LdMAcP: insight into its structure and function. Biochem. J. 467, 473–486. doi: 10.1042/BJ20141371
Rodrigues, J. C., Godinho, J. L., de Souza, W. (2014). Biology of human pathogenic trypanosomatids: epidemiology, lifecycle and ultrastructure. Subcell Biochem. 74, 1–42. doi: 10.1007/978-94-007-7305-9_1
Rogers, M. E., Chance, M. L., Bates, P. A. (2002). The role of promastigote secretory gel in the origin and transmission of the infective stage of Leishmania mexicana by the sandfly Lutzomyia longipalpis. Parasitology 124 (Pt 5), 495–507. doi: 10.1017/S0031182002001439
Sahasrabuddhe, A. A., Bajpai, V. K., Gupta, C. M. (2004). A novel form of actin in Leishmania: molecular characterisation, subcellular localisation and association with subpellicular microtubules. Mol. Biochem. Parasitol. 134 (1), 105–114. doi: 10.1016/j.molbiopara.2003.11.008
Sales Gil, R., de Castro, I. J., Berihun, J., Vagnarelli, P. (2018). Protein phosphatases at the nuclear envelope. Biochem. Soc. Trans. 46 (1), 173–182. doi: 10.1042/BST20170139
Santarem, N., Cunha, J., Silvestre, R., Silva, C., Moreira, D., Ouellette, M., et al. (2014). The impact of distinct culture media in Leishmania infantum biology and infectivity. Parasitology 141 (2), 192–205. doi: 10.1017/S0031182013001388
Schink, K. O., Tan, K. W., Stenmark, H. (2016). Phosphoinositides in Control of Membrane Dynamics. Annu. Rev. Cell Dev. Biol. 32, 143–171. doi: 10.1146/annurev-cellbio-111315-125349
Schuler, H., Matuschewski, K. (2006). Regulation of apicomplexan microfilament dynamics by a minimal set of actin-binding proteins. Traffic 7 (11), 1433–1439. doi: 10.1111/j.1600-0854.2006.00484.x
Senju, Y., Kalimeri, M., Koskela, E. V., Somerharju, P., Zhao, H., Vattulainen, I., et al. (2017). Mechanistic principles underlying regulation of the actin cytoskeleton by phosphoinositides. Proc. Natl. Acad. Sci. U. S. A. 114 (43), E8977–E8986. doi: 10.1073/pnas.1705032114
Sereno, D., Lemesre, J. L. (1997). Axenically cultured amastigote forms as an in vitro model for investigation of antileishmanial agents. Antimicrob. Agents Chemother. 41 (5), 972–976. doi: 10.1128/AAC.41.5.972
Silverman, J. M., Chan, S. K., Robinson, D. P., Dwyer, D. M., Nandan, D., Foster, L. J., et al. (2008). Proteomic analysis of the secretome of Leishmania donovani. Genome Biol. 9 (2), R35. doi: 10.1186/gb-2008-9-2-r35
Soulat, D., Bogdan, C. (2017). Function of Macrophage and Parasite Phosphatases in Leishmaniasis. Front. Immunol. 8, 1838. doi: 10.3389/fimmu.2017.01838
Sunter, J., Gull, K. (2017). Shape, form, function and Leishmania pathogenicity: from textbook descriptions to biological understanding. Open Biol. 7 (9), 170165–170178. doi: 10.1098/rsob.170165
Tsigankov, P., Gherardini, P. F., Helmer-Citterich, M., Spath, G. F., Zilberstein, D. (2013). Phosphoproteomic analysis of differentiating Leishmania parasites reveals a unique stage-specific phosphorylation motif. J. Proteome Res. 12 (7), 3405–3412. doi: 10.1021/pr4002492
Tsigankov, P., Gherardini, P. F., Helmer-Citterich, M., Spath, G. F., Myler, P. J., Zilberstein, D. (2014). Regulation dynamics of Leishmania differentiation: deconvoluting signals and identifying phosphorylation trends. Mol. Cell Proteomics 13 (7), 1787–1799. doi: 10.1074/mcp.M114.037705
Velasquez, V., Ochoa, R., Muskus, C. (2015). [Detection of molecular targets on the phosphatidylinositol signaling pathway of Leishmania spp. through bioinformatics tools and mathematical modeling]. Biomedica 35 (2), 235–246. doi: 10.1590/S0120-41572015000200012
Vince, J. E., Tull, D. L., Spurck, T., Derby, M. C., McFadden, G. I., Gleeson, P. A., et al. (2008). Leishmania adaptor protein-1 subunits are required for normal lysosome traffic, flagellum biogenesis, lipid homeostasis, and adaptation to temperatures encountered in the mammalian host. Eukaryot. Cell 7 (8), 1256–1267. doi: 10.1128/EC.00090-08
Wallroth, A., Haucke, V. (2018). Phosphoinositide conversion in endocytosis and the endolysosomal system. J. Biol. Chem. 293 (5), 1526–1535. doi: 10.1074/jbc.R117.000629
Wang, Z., Wheeler, R. J., Sunter, J. D. (2020). Lysosome assembly and disassembly changes endocytosis rate through the Leishmania cell cycle. Microbiologyopen 9 (2), e969. doi: 10.1002/mbo3.969
Wassef, M. K., Fioretti, T. B., Dwyer, D. M. (1985). Lipid analyses of isolated surface membranes of Leishmania donovani promastigotes. Lipids 20 (2), 108–115. doi: 10.1007/BF02534216
Wheeler, R. J., Gluenz, E., Gull, K. (2011). The cell cycle of Leishmania: morphogenetic events and their implications for parasite biology. Mol. Microbiol. 79 (3), 647–662. doi: 10.1111/j.1365-2958.2010.07479.x
Wheeler, R. J., Gluenz, E., Gull, K. (2015). Basal body multipotency and axonemal remodelling are two pathways to a 9+0 flagellum. Nat. Commun. 6, 8964. doi: 10.1038/ncomms9964
Wheeler, R. J., Sunter, J. D., Gull, K. (2016). Flagellar pocket restructuring through the Leishmania life cycle involves a discrete flagellum attachment zone. J. Cell Sci. 129 (4), 854–867. doi: 10.1242/jcs.183152
Xu, Q., Zhang, Y., Wei, Q., Huang, Y., Hu, J., Ling, K. (2016). Phosphatidylinositol phosphate kinase PIPKIgamma and phosphatase INPP5E coordinate initiation of ciliogenesis. Nat. Commun. 7, 10777. doi: 10.1038/ncomms10777
Young, J., Kima, P. E. (2019). The Leishmania Parasitophorous Vacuole Membrane at the Parasite-Host Interface. Yale J. Biol. Med. 92 (3), 511–521.
Zakai, H. A., Chance, M. L., Bates, P. A. (1998). In vitro stimulation of metacyclogenesis in Leishmania braziliensis, L. donovani, L. major and L. mexicana. Parasitology 116 ( Pt 4), 305–309. doi: 10.1017/S0031182097002382
Zhang, K., Beverley, S. M. (2010). Phospholipid and sphingolipid metabolism in Leishmania. Mol. Biochem. Parasitol. 170 (2), 55–64. doi: 10.1016/j.molbiopara.2009.12.004
Zhou, Y., Bhattacharjee, H., Mukhopadhyay, R. (2006). Bifunctional role of the leishmanial antimonate reductase LmACR2 as a protein tyrosine phosphatase. Mol. Biochem. Parasitol. 148 (2), 161–168. doi: 10.1016/j.molbiopara.2006.03.009
Zilberstein, D., Shapira, M. (1994). The role of pH and temperature in the development of Leishmania parasites. Annu. Rev. Microbiol. 48, 449–470. doi: 10.1146/annurev.mi.48.100194.002313
Keywords: Leishmania developmental transitions, atypical lipid phosphatase, phosphoinositide signaling and metabolism, P-Tyr/PI phosphatase, flagellar pocket, endocytosis/exocytosis
Citation: Papadaki A, Tziouvara O, Kotopouli A, Koumarianou P, Doukas A, Rios P, Tardieux I, Köhn M and Boleti H (2021) The Leishmania donovani LDBPK_220120.1 Gene Encodes for an Atypical Dual Specificity Lipid-Like Phosphatase Expressed in Promastigotes and Amastigotes; Substrate Specificity, Intracellular Localizations, and Putative Role(s). Front. Cell. Infect. Microbiol. 11:591868. doi: 10.3389/fcimb.2021.591868
Received: 05 August 2020; Accepted: 25 January 2021;
Published: 25 March 2021.
Edited by:
Juan David Ramírez, Rosario University, ColombiaReviewed by:
Jose M. Requena, Autonomous University of Madrid, SpainCopyright © 2021 Papadaki, Tziouvara, Kotopouli, Koumarianou, Doukas, Rios, Tardieux, Köhn and Boleti. This is an open-access article distributed under the terms of the Creative Commons Attribution License (CC BY). The use, distribution or reproduction in other forums is permitted, provided the original author(s) and the copyright owner(s) are credited and that the original publication in this journal is cited, in accordance with accepted academic practice. No use, distribution or reproduction is permitted which does not comply with these terms.
*Correspondence: Haralabia Boleti, aGJvbGV0aUBwYXN0ZXVyLmdy
†These authors have contributed equally to this work
Disclaimer: All claims expressed in this article are solely those of the authors and do not necessarily represent those of their affiliated organizations, or those of the publisher, the editors and the reviewers. Any product that may be evaluated in this article or claim that may be made by its manufacturer is not guaranteed or endorsed by the publisher.
Research integrity at Frontiers
Learn more about the work of our research integrity team to safeguard the quality of each article we publish.