- 1Proteomics & Mass Spectrometry Core Facility, Department for BioMedical Research (DBMR), University of Bern, Bern, Switzerland
- 2Department of Infectious Diseases and Pathobiology, Vetsuisse Faculty, Institute of Parasitology, University of Bern, Bern, Switzerland
Giardia lamblia is an important causative agent of persistent diarrhea in humans, domestic animals, and cattle. Basic research is usually performed with the strain WBC6 and includes genetic manipulations such as transfections. Here, we investigate how transfection with a plasmid causing stable expression of a foreign gene affects the whole proteome pattern. Using shotgun mass spectrometry, we compare the proteomes of untransfected trophozoites to trophozoites transfected with Escherichia coli glucuronidase A (GusA). Besides GusA, which is detected in the transfected trophozoites only, the proteomes of untransfected and transfected trophozoites differ by 132 differentially expressed proteins. In particular, transfection induces antigenic variation. Since transfection causing stable expression affects the proteome pattern, transfection experiments should take into account this effect. Due to a unique peptide panel, GusA is an example for a suitable internal standard for experiments involving transfected cells. Data are available via ProteomeXchange with identifier PXD022565.
Introduction
The diplomonadid Giardia lamblia (syn. G. duodenalis, G. intestinalis), is an early diverging, anaerobic eukaryote (Müller and Müller, 2016; Cernikova et al., 2018) causing persistent diarrhea, especially in regions with low hygienic standards (Hemphill et al., 2019). After stomach passage, ingested cysts transform into trophozoites colonizing the duodenum. Hosts in good physical condition face the strongest symptoms of giardiasis 1 week post infection, and recover within two to three weeks. Rarely, the infection becomes chronical causing severe damage of the intestinal epithelium, which may result in the development of irritable bowel syndrome (Allain et al., 2017; Litleskare et al., 2018). Giardiasis can be regarded as a zoonosis since it occurs in humans as well as in other mammals (Thompson, 2004).
The largest part of laboratory research is based on the strain WBC6, cloned from an isolate obtained from a patient (with the initials W.B.) suffering from chronical giardiasis (Nash et al., 1985; Campbell and Faubert, 1994). WBC6 is amenable to reverse genetics via transfection with double-stranded RNA (Furfine and Wang, 1990) or DNA (Singer et al., 1998; Sun et al., 1998), respectively. The genome of WBC6 (Morrison et al., 2007) and of other strains have been sequenced so far (see www.giardiadb.org). One unique feature of the Giardia genome is the presence of more than hundred open reading frames encoding cysteine-rich surface proteins, the so-called “variant-specific surface proteins” (VSPs), the “cysteine-rich proteins”, and the “high cysteine membrane proteins” (HCMPs). In a recently published chromosome-scale reference genome, 133 genes encoding for VSPs, as well as other highly repetitive genes such as 184 never-in-mitosis gene a (NIMA) related kinases and 305 ankyrin-repeat proteins (formerly annotated as proteins 21.1), are distributed over all five chromosomes (Xu et al., 2020). VSPs are considered as the predominant surface antigens of G. lamblia trophozoites (Adam et al., 2010). According to a generally admitted hypothesis, one single trophozoite expresses only one VSP at any one time (Nash et al., 2001). The switching from one VSP to another is called “antigenic variation” (Nash, 2002). Antigenic variation depends on epigenetic and post-transcriptional mechanisms, as evidenced by various studies (Kulakova et al., 2006; Prucca et al., 2008) and reviewed elsewhere (Prucca et al., 2011; Lagunas-Rangel and Bermudez-Cruz, 2018).
Shotgun mass spectrometry proteome studies have revealed that WBC6 trophozoite populations and trophozoite populations from other strains express an impressive number of VSPs at the same time (Emery et al., 2014; Emery, Lacey et al., 2015a; Emery et al., 2015b). Moreover, strain-dependent antigenic variation occurs upon drug pressure, concomitantly to large changes in expression patterns of other proteins (Emery et al., 2018; Müller et al., 2019).
As for many other model organisms, reverse genetics based on transfection with suitable plasmids or RNA viruses leading to transient or stable overexpression of genes of interest constitute an important research tool for Giardia (Davis-Hayman and Nash, 2002). In current protocols, transfectants are selected via a resistance marker encoded by the transfected plasmid, in particular neomycin phosphotransferase (Sun et al., 1998) and puromycin acetyltransferase (Singer et al., 1998; Jiménez-García et al., 2008). Since exposure to drugs and selection of resistance causes marked changes in gene expression patterns, it is logical to ask which side effects the transfection procedure has on gene expression in the transfected cells, independently of the transfected gene. In one of the first studies addressing this topic on the transcriptional level, neomycin and puromycin selection affected the expression patterns of various genes (Su et al., 2007).
Based on these findings, we contend that stable transfection of G. lamblia based on puromycin selection induces changes in proteome patterns to the same extent as differences amongst strains from different genotypes and differences between drug resistant and susceptible strains of the same background. Using shotgun mass spectrometry, we compare the proteomes of G. lamblia WBC6 trophozoites transfected with a plasmid containing Escherichia coli glucuronidase A (GusA) and Streptomyces alboniger puromycin_N-acetyltransferase (Pac) as a resistance marker (Singer et al., 1998) with untransfected trophozoites. The GusA transfection plasmid has been used by our group as a standard control in previous experiments (Nillius et al., 2011; Müller et al., 2013) involving transgenic Giardia. GusA-transfected trophozoites are not affected in growth (Müller et al., 2009) and have a metabolomics profile similar to untransfected trophozoites (Müller et al., 2020b). Here, we investigate the impact of transfection on the proteome pattern with a major focus on antigenic variation. Furthermore, we ask the question whether the transfected transgenes, i.e. the resistance marker Pac, GusA, or both can be used to estimate the expression level of the transgene in comparison to “housekeeping” proteins.
Materials and Methods
Chemicals
If not otherwise stated, all biochemical reagents were from Sigma (St Louis, MO, USA). Puromycin was obtained from Invivogen (Toulouse, France).
Axenic Culture, Harvest, and Storage of Giardia lamblia Trophozoites
Trophozoites from G. lamblia WBC6 were grown under anaerobic conditions in 10 ml culture tubes (Nunc, Roskilde, Denmark) on modified TYI-S-33 medium as previously described (Clark and Diamond, 2002). Subcultures were performed by inoculating 100 μl of cells from a confluent culture detached by incubation on ice for 15 min to a new tube containing 10 ml culture medium (Müller et al., 2006). Trophozoites were harvested by incubation on ice for 15 min followed by centrifugation (300×g, 10 min, 4°C).
Transfection of Giardia lamblia Trophozoites
Transfection with the plasmid pPAC-V-GusA (Müller et al., 2009) and selection of transgenic trophozoites were performed as previously described (Yee and Nash, 1995; Singer et al., 1998). Briefly, prior to transfection, 1 µg of pPAC-V-GusA (in 20 µl digest mix) were linearized by digestion with SwaI (New England Biolabs, Ipswitch, MA) according to the instructions by the manufacturer. Then, 107 trophozoites from a confluent culture were mixed with the digested plasmid DNA and incubated on ice for 5 min. Electroporation of trophozoites with linearized plasmid DNA was done in a 0.4 cm cuvette using an ECM 600 (BTX, San Diego, CA) at setting 350 V, 1000 µF, and 720 Ω. Electroporated trophozoites were immediately transferred to 10 ml medium in a plastic tube and incubated overnight at 37°C before puromycin was added to a final concentration of 100 µM. After 4 days, the medium was replaced by fresh medium containing 100 µM puromycin and drug-resistant cells visible after about 8 days post transfection were grown to confluence and then passaged once in the same medium. Before shotgun mass spectrometry analysis, the untransfected cultures were routinely passaged two times, the GusA-transfected cultures in absence of puromycin. Pellets were washed three times with ice-cold PBS, counted, and stored at −80°C for subsequent proteomic analysis or for enzymatic assays, respectively.
Proteomics
Cell pellets were lysed in 100 μL 8M urea/100 mM Tris/HCl pH8/cOmplete™ protease inhibitor cocktail (Roche Diagnostics, Rotkreuz, Switzerland) by incubation for 15 min at room temperature followed by 15 min in an ultrasonic water bath. Protein concentration was determined by BCA assay, followed by reduction and alkylation of proteins with 10 mM DTT for 30 min at 37°C and 50 mM iodoacetamide for 30 min at 37°C in the dark. Proteins were precipitated at −20°C by addition of 5 vol cold acetone and incubation at −20°C overnight. All liquid was carefully removed and the pellet dried in ambient air for 15 min before reconstitution of proteins to a concentration of 1mg/ml in 8 M urea, 50 mM Tris-HCl pH 8.0. An aliquot corresponding to 10 μg protein was digested by trypsin (1:50 trypsin/protein ratio) for 6 hours at 37°C after dilution of urea concentration to 1.6M with 20 mM Tris-HCl pH 8.0 and 2 mM CaCl2. The digests were acidified with TFA (1%) and analyzed by LC-MS/MS. Three repetitive injections of an aliquot corresponding to 500 ng protein digest were analyzed on an EASY-nLC 1000 coupled to a QExactive HF mass spectrometer (ThermoFisher, Reinach, Switzerland). Peptides were trapped on an Acclaim PepMap100 C18 pre-column (3μm, 100 Å, 300 μm x 5 mm, ThermoFisher, Reinach, Switzerland) and separated by backflush on a C18 column (3μm, 100 Å, 75μm x 15 cm, Nikkyo Technos, Tokyo, Japan) by applying a 60 min gradient of 5% acetonitrile to 40% in water, 0.1% formic acid, at a flow rate of 400 nl/min. Peptides of m/z 400–1,400 were detected with resolution of 60,000 applying an automatic gain control (AGC) target of 1E06 and a maximum ion injection time of 50 ms. A top fifteen data dependent method for precursor ion fragmentation with a stepped 27% normalized collision energy was applied with the following settings: precursor isolation width of 1.6 m/z, resolution 15,000, AGC of 1E05 with a minimum target of 1E03, maximum ion time of 110 ms, charge exclusion of unassigned and 1+ ions, peptide match on, and dynamic exclusion for 20 s, respectively. The mass spectrometry proteomics data have been deposited to the ProteomeXchange Consortium via the PRIDE (Perez-Riverol et al., 2019) partner repository with the dataset identifier PXD022565.
Statistics
The MS data for each strain consisted of three biological replicates, with three technical replicates each. All MS data were processed by MaxQuant (version 1.6.14.0) with matching between runs for the same strain activated, but not between different strains, in order to avoid over-interpretation of the data. Fragment spectra were interpreted against a recent Giardia protein sequence database in FASTA format (GiardiaDB-47_GintestinalisAssemblageAWB_AnnotatedProteins), supplemented by the two protein sequences of GusA and Pac from Swissprot database (accession numbers P05804 and P13249). The trypsin cleavage rule allowed amide bond cleavage after lysine and arginine but not if a proline follows and up to three missed cleavage sites, fixed carbamidomethylation modification of cysteine residues, variable oxidation of methionine and acetylation of protein N-termini. Precursor and fragment mass tolerances were set to 10 and 20 ppm, respectively. Peptide spectrum matches, peptide and protein group identifications were filtered to a 1% false discovery rate (FDR) based on reversed database sequence matches, and a minimum of two razor or unique peptides were required to accept a protein group identification.
Protein identifications considered as contaminations (e.g. trypsin) as well as proteins identified only by site were removed for statistical validation. The normalized label-free quantification (LFQ) protein group intensities as calculated by MaxQuant and a top3 approach were used for relative proteome quantifications. For top3, peptide intensities were median-normalized to the global median, then missing peptide imputation was done per sample by drawing values from a Gaussian distribution of width 0.3x sample standard deviation centered at the sample distribution mean minus 1.8x sample standard deviation if there were at least two peptide intensities in a group, otherwise a Maximum Likelihood Estimation (MLE) method was applied, followed by summation of the three most intense peptides per protein group to a surrogate of the real protein abundance, named iTop3. For LFQ values, we imputed with the same left-censored method as for peptides if there was a single missing protein LFQ value in the three biological replicates. Any remaining missing values were again imputed by the MLE method. In order to perform statistical tests, missing iTop3 or LFQ values were further imputed at the protein level using the MLE method, and the final imputed LFQ or iTop3 values were called iLFQ or iiTop3, respectively.
Differential expression tests were performed by applying the (Welch-) Student’s t-test (unequal variance). Log2-fold changes and adjusted p-values (FDR-controlled Benjamini and Hochberg correction) were reported. Furthermore, a check was performed by repeating the imputation cycle and significance testing 20 times. Protein groups with a persistent reporting of differential expression were considered as true differentially expressed between the two groups. A log2-fold change of at least one and a corrected p-value of ≦0.05 were required to be considered as significant. Statistical testing and imputation were made using a set of freely available R package tools running under R studio.
Results
Proteome Parameters of Giardia lamblia Trophozoites
Shotgun mass spectrometry of the proteomes of untransfected G. lamblia WBC6 trophozoites (WT) and of trophozoites transfected with a plasmid containing the S. alboniger puromycin-N-acetlytransferase (Pac) as a resistance marker and the E. coli glucuronidase A gene (Gus A) resulted in the identification of 20’091 unique peptides matching to 1’705 proteins. Moreover, 12 unique peptides matching to GusA were consistently detected in the transfected trophozoites, but not in the WT. The identified peptides are depicted in Figure 1. Unique peptides matching to Pac could not be detected.
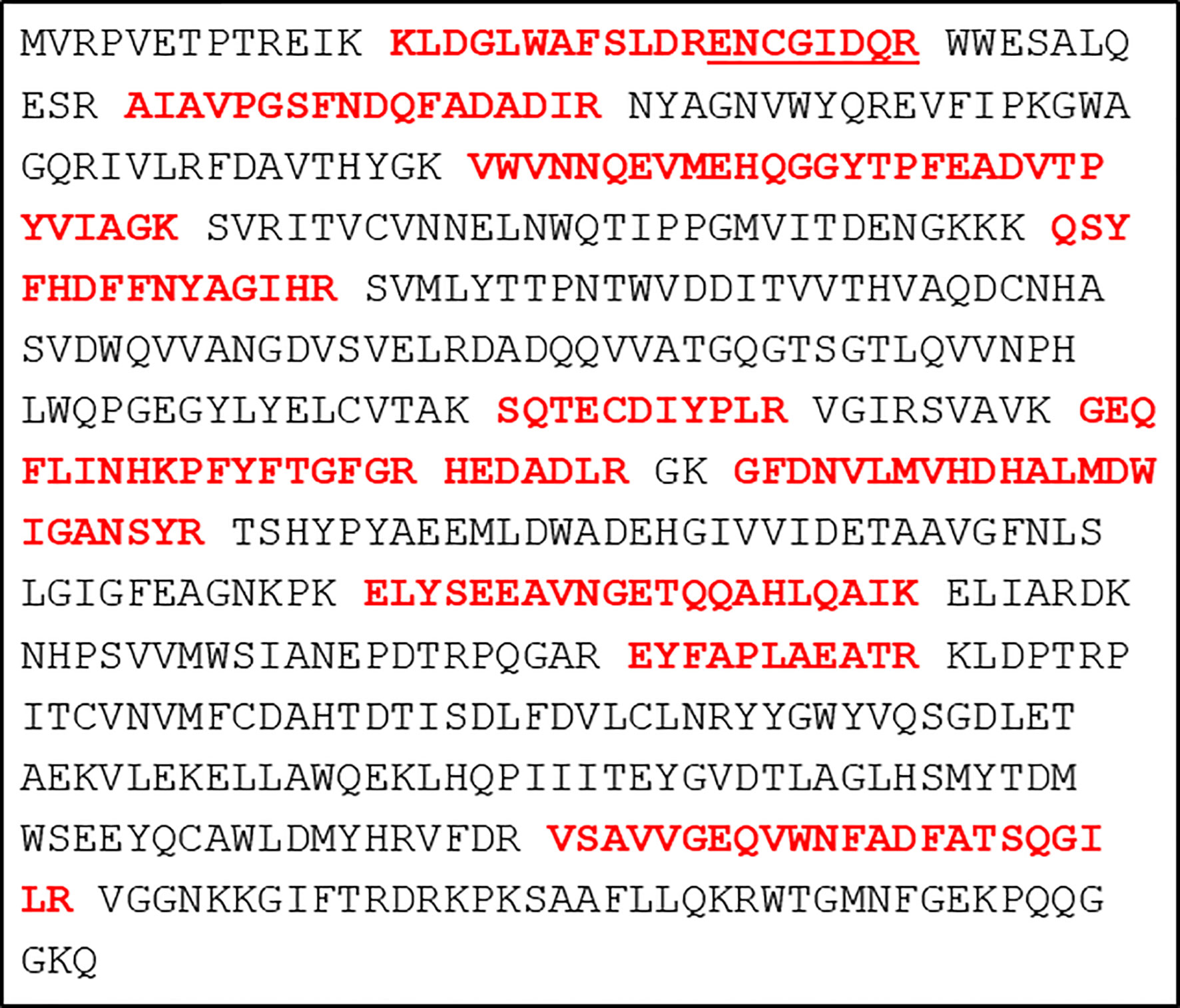
Figure 1 Primary sequence of the transgene E. coli glucuronidase A with the identified peptides highlighted in bold red letters. The underlined sequence is a separate peptide generated from the longer peptide sequence with a missed trypsin cleavage site at position twelve. Otherwise, trypsin cleavage sites are indicated by a space. Each line consists of 40 amino acids.
The expression levels of GusA in the transfected trophozoites were compared to four housekeeping proteins expressed at equal LFQ and iTop3 levels in both transfected and untransfected strains, namely pyruvate-ferredoxin oxidoreductase, arginine deiminase, thioredoxin reductase, and glutamate dehydrogenase. The levels of GusA were one to two magnitudes (in base 10) lower than the levels of these proteins (Table 1).
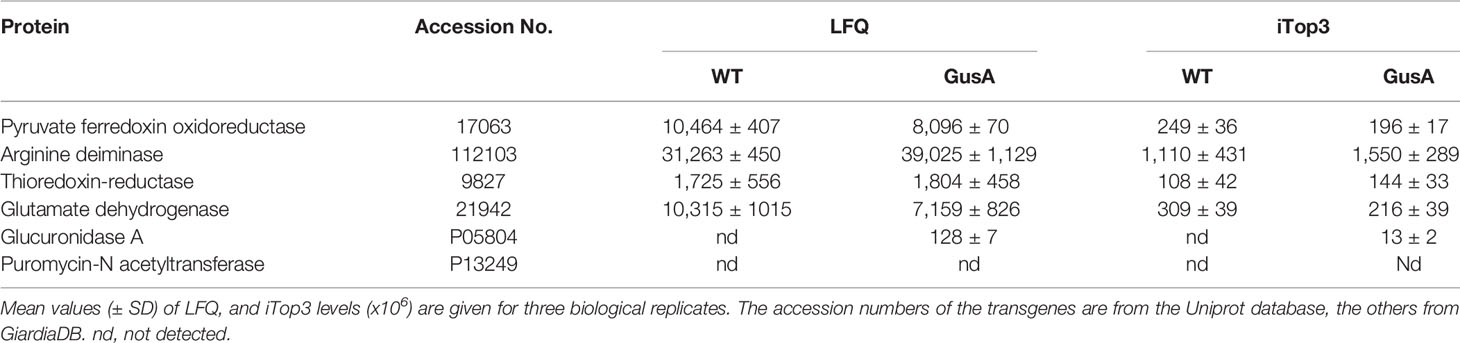
Table 1 Major housekeeping proteins and transgenes in untransfected Giardia lamblia WBC6 wildtype (WT) and Escherichia coli glucuronidase A (GusA)-transfected trophozoites.
Transfection Induces Significant Changes in Giardia lamblia Trophozoite Proteomes
Overall analysis of the data by principal component analysis (PCA) revealed that the proteomes from WT and GusA trophozoites were distinctly separated along the principal component 1. As expected, technical replicates clustered together, whereas biological replicates were separated by principal component 2 for both groups (Figures 2A, B). Differences in the proteome patterns of both strains were confirmed by Volcano plot analysis (Figure 2 C).
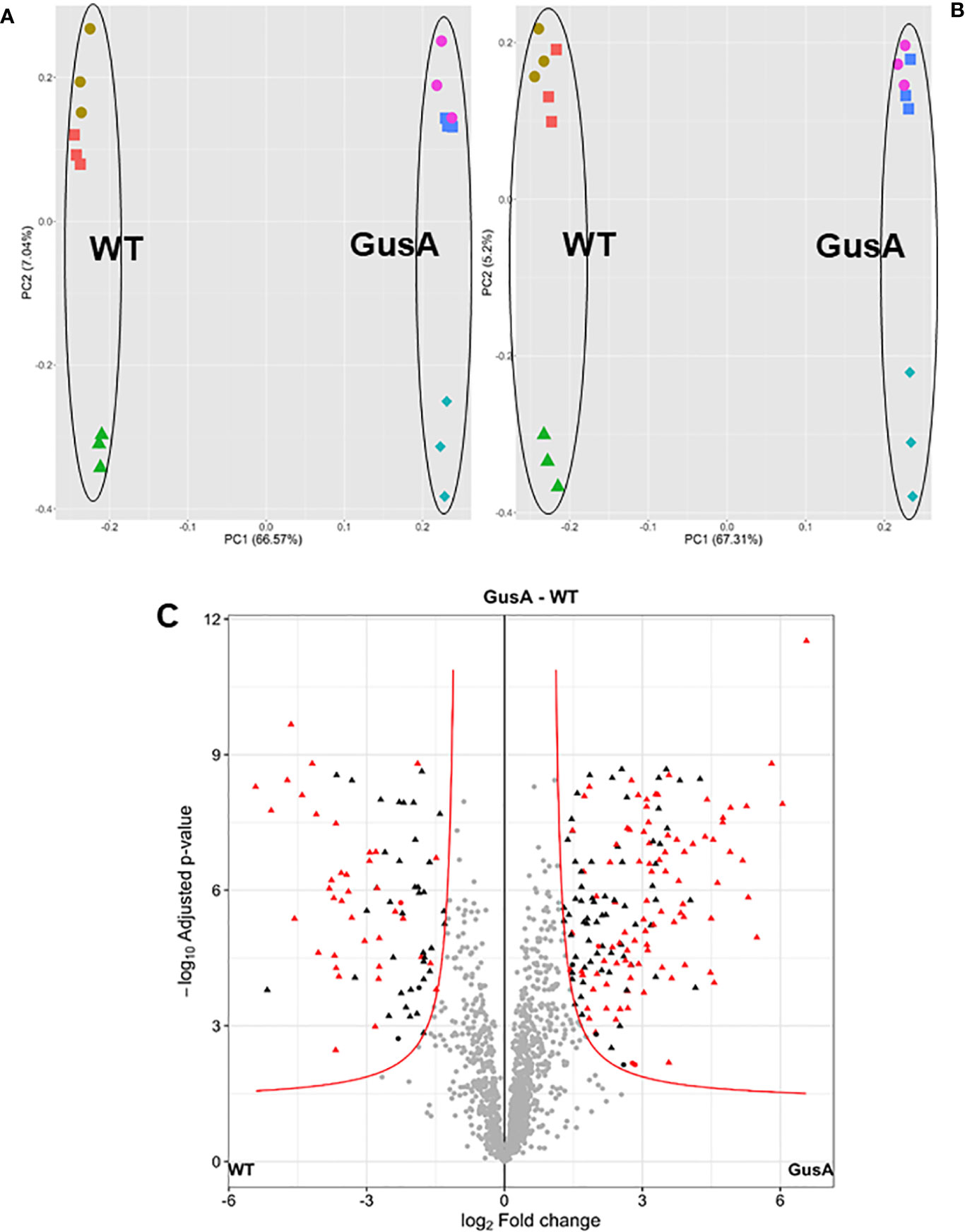
Figure 2 Principal component analysis plots (A, B) and volcano plot (C) of proteome data set from G. lamblia trophozoites transfected with a plasmid containing E. coli glucuronidase A (GusA) and of not transfected, control trophozoites (WT). The principal component analysis plots show for each strain, all technical and biological (red square, brown circle, green triangle for WT; pink circle, blue square, turquoise diamond for GusA) replicates based on iiTop3 data (A) or iLFQ data (B). The volcano plot (C) is based on iiTop3 data. Differential proteins identified by both iiTop3 and iLFQ algorithms are depicted in red.
Differentially Expressed Proteins
Further analysis of the dataset revealed that the proteomes of WT and GusA contained 132 differentially expressed proteins, namely 39 with higher levels in WT and 93 with higher levels in GusA trophozoites, when the evaluation was done by both iLFQ and iiTop3 algorithms as shown in Figure 3.
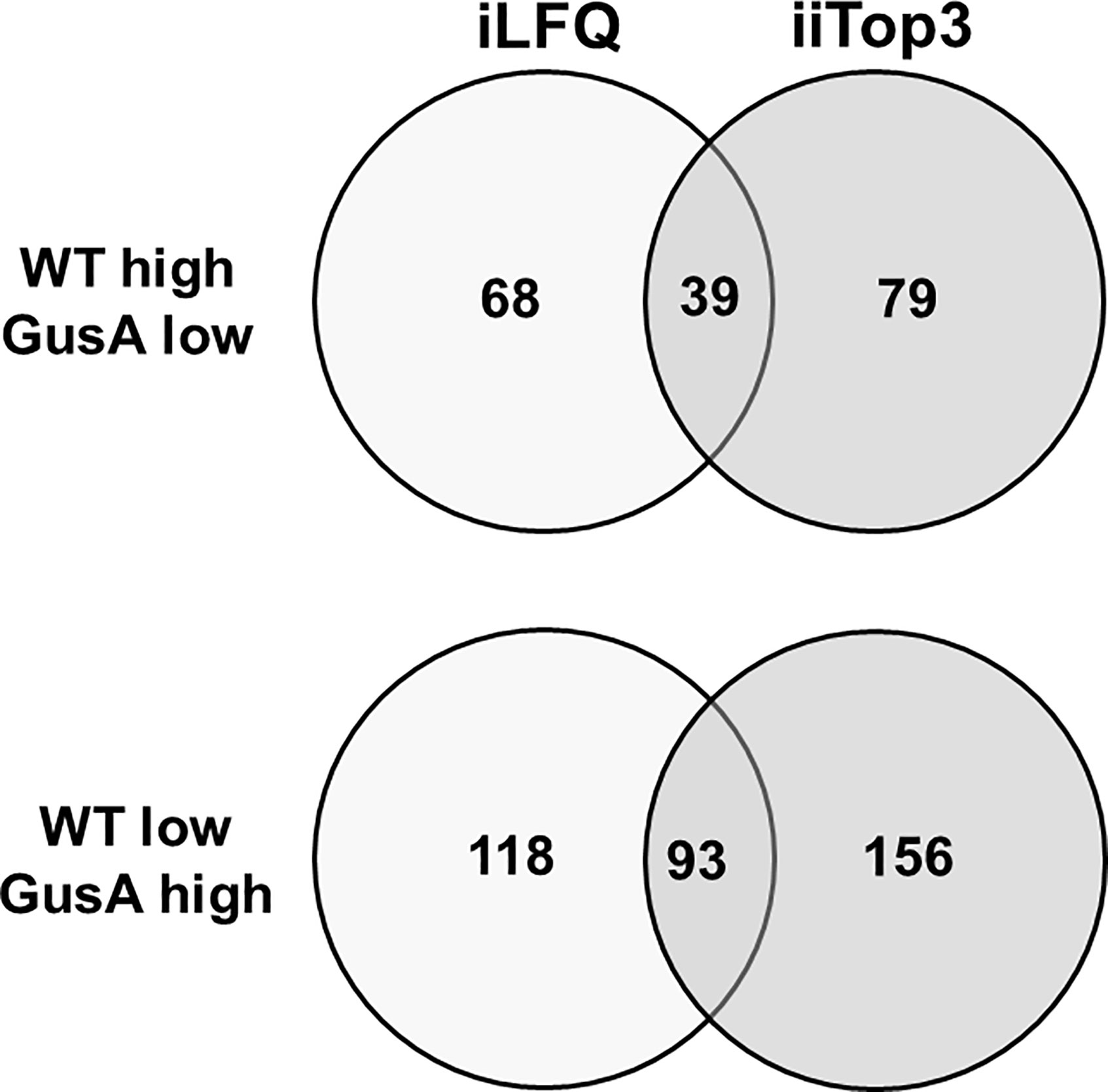
Figure 3 Venn diagram depicting the number of differentially expressed proteins in transfected and untransfected G. lamblia trophozoites. Untransfected trophozoites (WT) and trophozoites transfected with E. coli glucuronidase A were subjected to MS shotgun analysis as described in Materials and Methods. The differential proteins were determined via the iLFQ, the iiTop3 algorithm or via both.
Besides 70 differentially expressed (DE) hypothetical proteins, the two categories with the highest numbers of DE proteins, namely 17, comprised surface antigens and proteins involved in intermediary metabolism, followed by 14 proteins involved in gene expression, cell cycle and development and 14 proteins involved in cytoskeleton, flagella, adhesion and organelle transport (Table 2). The complete list of the accession numbers of the DE proteins is given as Supplementary Table S1.
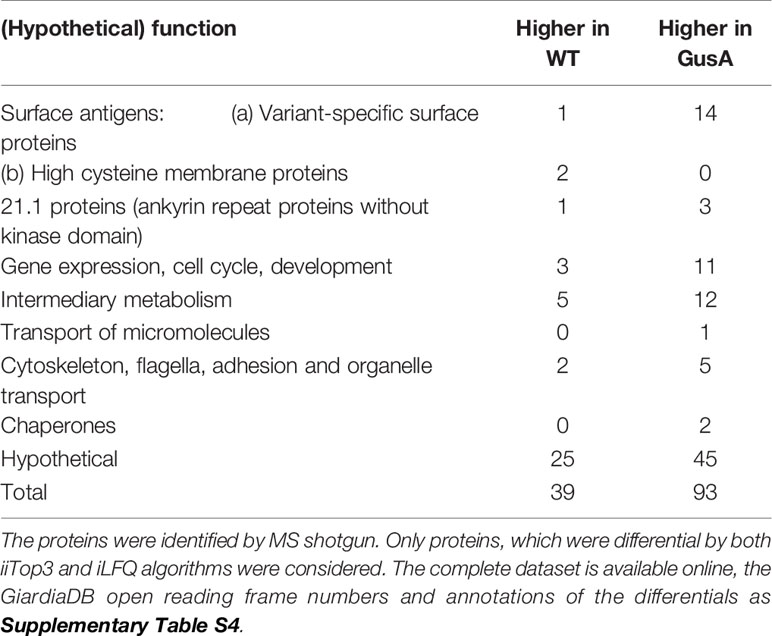
Table 2 Overview of differentially expressed proteins in untransfected G. lamblia wildtype (WT) and E. coli glucuronidase A-transfected trophozoites (GusA).
Antigenic Variation
As mentioned above, surface antigens constituted one of the two categories with the highest amount of DE proteins, namely 17. In particular, 14 variant-specific surface proteins (VSP) had significantly higher levels in GusA-transfected trophozoites and one VSP in wildtype trophozoites. Conversely, only two high cysteine membrane proteins (HCMP), namely 115066 and 25816, had significantly higher levels in WT than in GusA trophozoites. The VSP 89315 was the predominant differential surface antigen with significantly higher levels in wildtype trophozoites. VSP14586 was the predominant DE VSP in GusA trophozoites (Figure 4).
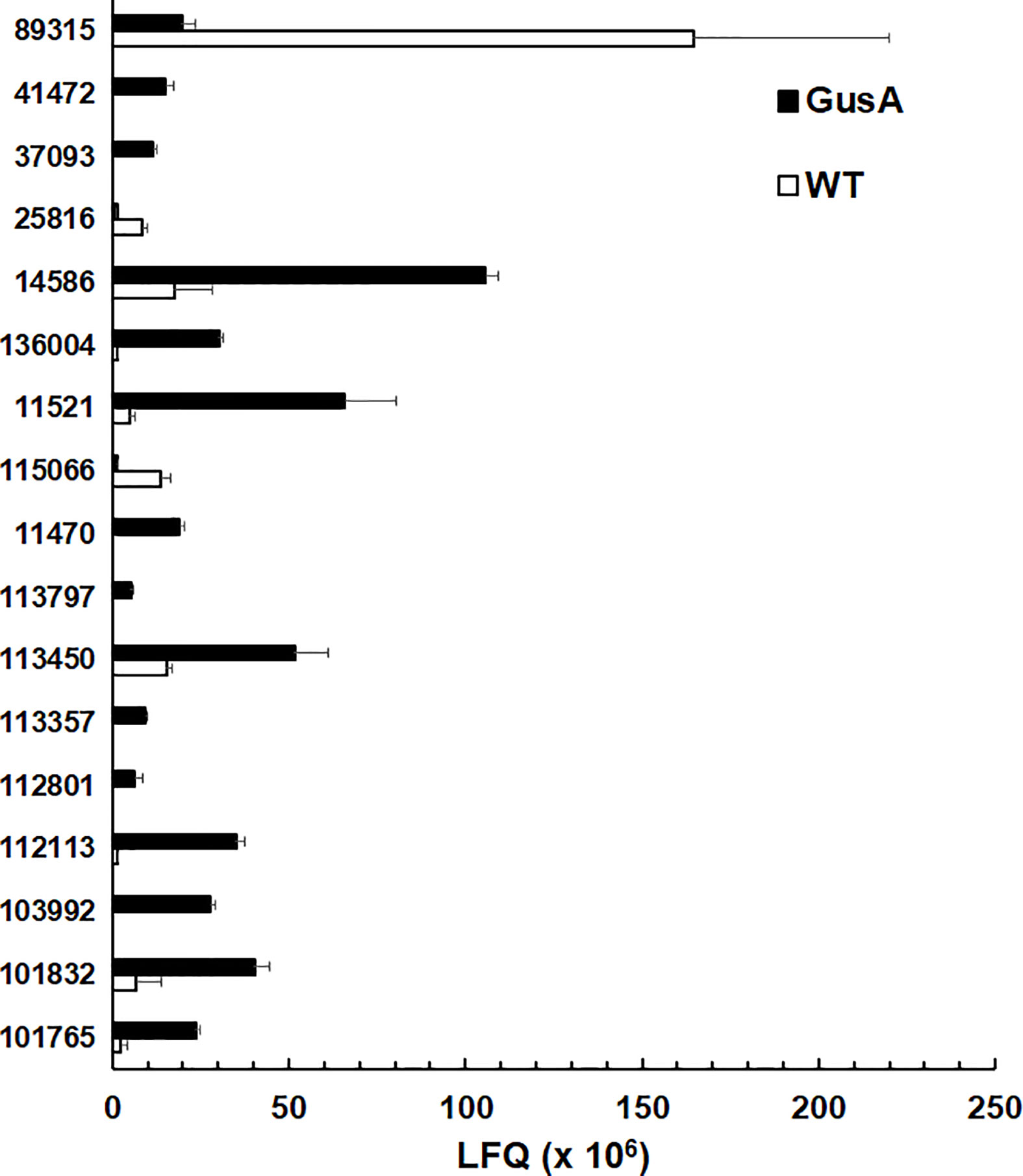
Figure 4 Quantitative assessments of differential surface antigens. Untransfected G. lamblia WBC6 trophozoites (WT; white bars) and E. coli glucuronidase A-transfected trophozoites (GusA; black bars) were subjected to MS shotgun analysis as described in Materials and Methods section. For all proteins, mean values ± one standard deviation for LFQ intensities (x106) in three biological replicates are shown. The proteins are termed by their respective accession numbers in the GiardiaDB. 25816 and 113416 are high cysteine membrane proteins.
The expression levels of the major surface antigens, namely the VSPs 188, 88, 8, and the HCMP with the ORF number 15317, however, were not significantly affected (Table 3).
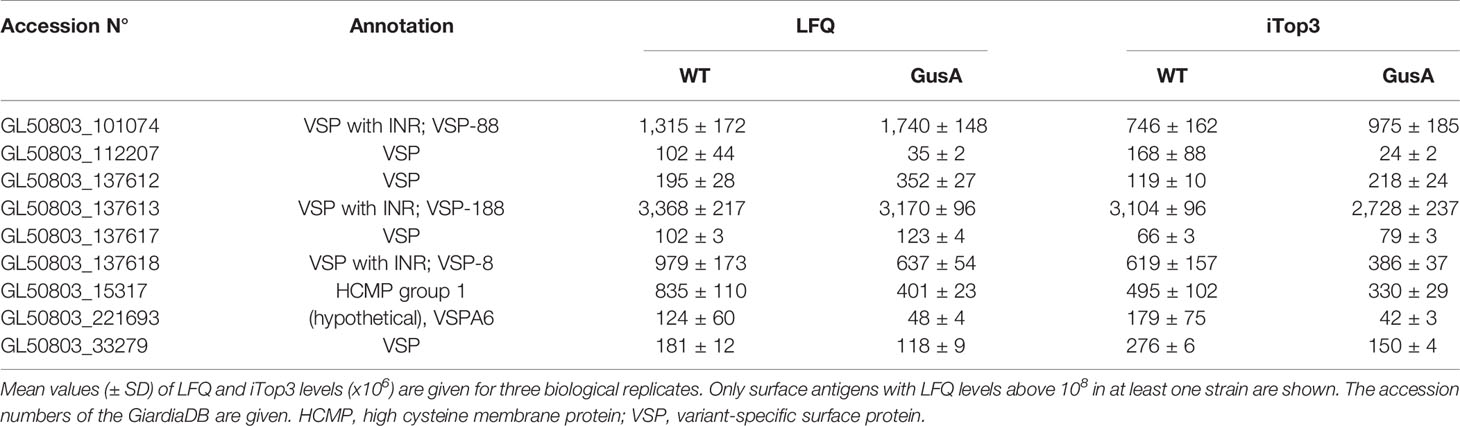
Table 3 Major surface antigens without significant differences in expression levels between untransfected G. lamblia WBC6 wildtype (WT) and E. coli glucuronidase A (GusA)-transfected trophozoites.
Discussion
The present dataset confirms our initial hypothesis that the transfection of G. lamblia by plasmids followed by an antibiotic-based selection of transfected trophozoites affects the whole genome expression pattern thereby confirming and extending previous findings (Su et al., 2007). Furthermore, it induces antigenic variation like other drug-based selections (Emery et al., 2018; Müller et al., 2019) or in strains from different genetic backgrounds (Emery et al., 2014; Emery et al., 2015a; Müller et al., 2020a). For instance, we have found 17 DE surface antigens between WT and Gus (one permutation) and 89 between WT and nitro drug resistant C4 cells grown either in the presence of metronidazole or nitazoxanide (three permutations), as shown in a previous study (Müller et al., 2019; in particular Supplementary Table S2 therein).
Since antigenic variation is caused by epigenetic (Lagunas-Rangel and Bermudez-Cruz, 2018) and post-transcriptional (Prucca et al., 2008) mechanisms, the degree of variation could be used as a tool to estimate the impact of a given treatment, in our case transfection, on these mechanisms. As generally admitted, trophozoites express only one VSP on their surface at the same time (Nash et al., 2001). Thus, the fact that the number of differentially expressed VSPs is higher in the transfected than in the untransfected trophozoite population is indicative for a higher degree of diversification most likely caused by the selection process. Moreover, the transfection and the subsequent selection procedure may influence metabolism, cell organization and motility, as indicated by a number of differentials in these categories. In fact, we observe only small metabolic differences between WT and GusA transfected strains, as published in a previous study (Müller et al., 2020b).
It is surprising that 12 unique peptides can be attributed to the GusA protein thereby allowing an easy identification, but no peptides to the puromycin resistance marker Pac protein, which has allowed to select the transfectants. The expression of both proteins is controlled by the same promoter, namely the strong promoter of the glutamate dehydrogenase (Singer et al., 1998). The lack of unique Pac peptides could be due to a high similarity to peptides derived from Giardia proteins—what is, however, very unlikely—or to a rapid post-transcriptional silencing.
Consequently, each experiment investigating the role of a given transgene should contain an irrelevant transfection (e.g. with GusA) as a control. Untransfected WT trophozoites do not reflect the impact of the transfection and selection procedures on gene expression. So far, it is unclear how other genetic manipulations such as RNA virus-mediated transfection (Janssen et al., 2015) or CRISPR/Cas9 (Lin et al., 2019; McInally et al., 2019) affect gene expression in G. lamblia. Proteome studies should be performed with trophozoites transfected by these methods and investigate the impact on epigenetic and post-transcriptional regulation of gene expression by estimating the degree of antigenic variation. Further, more detailed studies comparing either different selection methods, or different control genes, or both, would be useful for helping researchers to determine methods that induce minimal proteomic perturbation. Indeed, the ideal vector control will induce minimal proteomic changes relative to wild-type untransfected cells. By including a reference transgene such as Gus A, expressing a clearly detectable, unique peptide may help to establish an internal standard, e.g. for comparative studies in different genetic backgrounds.
Data Availability Statement
The mass spectrometry proteomics data have been deposited to the ProteomeXchange Consortium via the PRIDE (Perez-Riverol et al., 2019) partner repository with the dataset identifier PXD022565.
Author Contributions
MH and SB analyzed the trophozoite proteomes. NM and JM conceptualized the study, constructed and cultured the strains, and evaluated the results. JM wrote the manuscript. All authors contributed to the article and approved the submitted version.
Funding
This research was funded by the Swiss National Science Foundation, grant number 31003A_163230 and grant no. 206021-128736.2.
Conflict of Interest
The authors declare that the research was conducted in the absence of any commercial or financial relationships that could be construed as a potential conflict of interest.
Supplementary Material
The Supplementary Material for this article can be found online at: https://www.frontiersin.org/articles/10.3389/fcimb.2020.602756/full#supplementary-material
References
Adam R. D., Nigam A., Seshadri V., Martens C. A., Farneth G. A., Morrison H. G., et al. (2010). The Giardia lamblia vsp gene repertoire: characteristics, genomic organization, and evolution. BMC Genomics 11, 424. doi: 10.1186/1471-2164-11-424
Allain T., Amat C. B., Motta J. P., Manko A., Buret A. G. (2017). Interactions of Giardia sp. with the intestinal barrier: Epithelium, mucus, and microbiota. Tissue Barriers 5 (1), e1274354. doi: 10.1080/21688370.2016.1274354
Campbell J. D., Faubert G. M. (1994). Comparative studies on Giardia lamblia encystation in vitro and in vivo. J. Parasitol. 80 (1), 36–44. doi: 10.2307/3283342
Cernikova L., Faso C., Hehl A. B. (2018). Five facts about Giardia lamblia. PloS Pathog. 14 (9), e1007250. doi: 10.1371/journal.ppat.1007250
Clark C. G., Diamond L. S. (2002). Methods for cultivation of luminal parasitic protists of clinical importance. Clin. Microbiol. Rev. 15 (3), 329–341. doi: 10.1128/CMR.15.3.329-341.2002
Davis-Hayman S. R., Nash T. E. (2002). Genetic manipulation of Giardia lamblia. Mol. Biochem. Parasitol. 122 (1), 1–7. doi: 10.1016/S0166-6851(02)00063-4
Emery S. J., van Sluyter S., Haynes P. A. (2014). Proteomic analysis in Giardia duodenalis yields insights into strain virulence and antigenic variation. Proteomics 14 (21-22), 2523–2534. doi: 10.1002/pmic.201400144
Emery S. J., Lacey E., Haynes P. A. (2015a). Quantitative proteomic analysis of Giardia duodenalis assemblage A: A baseline for host, assemblage, and isolate variation. Proteomics 15 (13), 2281–2285. doi: 10.1002/pmic.201400434
Emery S. J., Pascovi D., Lacey E., Haynes P. A. (2015b). The generation gap: Proteome changes and strain variation during encystation in Giardia duodenalis. Mol. Biochem. Parasitol. 201 (1), 47–56. doi: 10.1016/j.molbiopara.2015.05.007
Emery S. J., Baker L., Ansell B. R. E., Mirzaei M., Haynes P. A., McConville M. J., et al. (2018). Differential protein expression and post-translational modifications in metronidazole-resistant Giardia duodenalis. Gigascience 7 (4), 1–22. doi: 10.1093/gigascience/giy024
Furfine E. S., Wang C. C. (1990). Transfection of the Giardia lamblia double-stranded RNA virus into Giardia lamblia by electroporation of a single-stranded RNA copy of the viral genome. Mol. Cell Biol. 10 (7), 3659–3662. doi: 10.1128/MCB.10.7.3659
Hemphill A., Müller N., Müller J. (2019). Comparative pathobiology of the intestinal protozoan parasites Giardia lamblia, Entamoeba histolytica, and Cryptosporidium parvum. Pathogens 8 (116), 1–21. doi: 10.3390/pathogens8030116
Janssen M. E., Takagi Y., Parent K. N., Cardone G., Nibert M. L., Baker T. S. (2015). Three-dimensional structure of a protozoal double-stranded RNA virus that infects the enteric pathogen Giardia lamblia. J. Virol. 89 (2), 1182–1194. doi: 10.1128/JVI.02745-14
Jiménez-García L. F., Zavala G., Chávez-Munguía B., Ramos-Godínez M., López-Velázquez G., Segura-Valdez M., et al. (2008). Identification of nucleoli in the early branching protist Giardia duodenalis. Int. J. Parasitol. 38 (11), 1297–1304. doi: 10.1016/j.ijpara.2008.04.012
Kulakova L., Singer S. M., Conrad J., Nash T. E. (2006). Epigenetic mechanisms are involved in the control of Giardia lamblia antigenic variation. Mol. Microbiol. 61 (6), 1533–1542. doi: 10.1111/j.1365-2958.2006.05345.x
Lagunas-Rangel F. A., Bermudez-Cruz R. M. (2018). Epigenetics in the early divergent eukaryotic Giardia duodenalis: An update. Biochimie 156, 123–128. doi: 10.1016/j.biochi.2018.10.008
Lin Z. Q., Gan S. W., Tung S. Y., Ho C. C., Su L. H., Sun C. H. (2019). Development of CRISPR/Cas9-mediated gene disruption systems in Giardia lamblia. PloS One 14 (3), e0213594. doi: 10.1371/journal.pone.0213594
Litleskare S., Rortveit G., Eide G. E., Hanevik K., Langeland N., Wensaas K. A. (2018). Prevalence of irritable bowel syndrome and chronic fatigue 10 years after Giardia infection. Clin. Gastroenterol. Hepatol. 16 (7), 1064–1072 e1064. doi: 10.1016/j.cgh.2018.01.022
McInally S. G., Hagen K. D., Nosala C., Williams J., Nguyen K., Booker J., et al. (2019). Robust and stable transcriptional repression in Giardia using CRISPRi. Mol. Biol. Cell 30 (1), 119–130. doi: 10.1091/mbc.E18-09-0605
Morrison H. G., McArthur A. G., Gillin F. D., Aley S. B., Adam R. D., Olsen G. J., et al. (2007). Genomic minimalism in the early diverging intestinal parasite Giardia lamblia. Science 317 (5846), 1921–1926. doi: 10.1126/science.1143837
Müller N., Müller J. (2016). Giardia. Molecular Parasitology. Eds. Walochnik J., Duchêne M. (Vienna: Springer-Verlag), 93–114. doi: 10.1007/978-3-7091-1416-2_4
Müller J., Rühle G., Müller N., Rossignol J. F., Hemphill A. (2006). In vitro effects of thiazolides on Giardia lamblia WB clone C6 cultured axenically and in coculture with Caco2 cells. Antimicrob. Agents Chemother. 50 (1), 162–170. doi: 10.1128/AAC.50.1.162-170.2006
Müller J., Nillius D., Hehl A., Hemphill A., Müller N. (2009b). Stable expression of Escherichia coli beta-glucuronidase A (GusA) in Giardia lamblia: application to high-throughput drug susceptibility testing. J. Antimicrob. Chemother. 64 (6), 1187–1191. doi: 10.1093/jac/dkp363
Müller J., Schildknecht P., Müller N. (2013). Metabolism of nitro drugs metronidazole and nitazoxanide in Giardia lamblia: characterization of a novel nitroreductase (GlNR2). J. Antimicrob. Chemother. 68 (8), 1781–1789. doi: 10.1093/jac/dkt106
Müller J., Braga S., Heller M., Müller N. (2019). Resistance formation to nitro drugs in Giardia lamblia: No common markers identified by comparative proteomics. Int. J. Parasitol.: Drugs Drug Resist. 9, 112–119. doi: 10.1017/S0031182018000975
Müller J., Braga S., Uldry A. C., Heller M., Müller N. (2020a). Comparative proteomics of three Giardia lamblia strains: investigation of antigenic variation in the post-genomic era. Parasitology 147, 1008–1018. doi: 10.1017/S0031182020000657
Müller J., Vermathen M., Leitsch D., Vermathen P., Müller N. (2020b). Metabolomic profiling of wildtype and transgenic Giardia lamblia strains by 1H HR-MAS NMR Spectroscopy. Metabolites 10 (2). doi: 10.3390/metabo10020053
Nash T. E., McCutchan T., Keister D., Dame J. B., Conrad J. D., Gillin F. D. (1985). Restriction-endonuclease analysis of DNA from 15 Giardia isolates obtained from humans and animals. J. Infect. Dis. 152 (1), 64–73. doi: 10.1093/infdis/152.1.64
Nash T. E., Luján H. T., Mowatt M. R., Conrad J. T. (2001). Variant-specific surface protein switching in Giardia lamblia. Infect. Immun. 69 (3), 1922–1923. doi: 10.1128/IAI.69.3.1922-1923.2001
Nash T. E. (2002). Surface antigenic variation in Giardia lamblia. Mol. Microbiol. 45 (3), 585–590. doi: 10.1046/j.1365-2958.2002.03029.x
Nillius D., Müller J., Müller N. (2011). Nitroreductase (GlNR1) increases susceptibility of Giardia lamblia and Escherichia coli to nitro drugs. J. Antimicrob. Chemother. 66 (5), 1029–1035. doi: 10.1093/jac/dkr029
Perez-Riverol Y., Csordas A., Bai J., Bernal-Llinares M., Hewapathirana S., Kundu D. J., et al. (2019). The PRIDE database and related tools and resources in 2019: improving support for quantification data. Nucleic Acids Res. 47 (D1), D442–D450. doi: 10.1093/nar/gky1106
Prucca C. G., Slavin I., Quiroga R., Elías E. V., Rivero F. D., Saura A., et al. (2008). Antigenic variation in Giardia lamblia is regulated by RNA interference. Nature 456 (7223), 750–754. doi: 10.1038/nature07585
Prucca C. G., Rivero F. D., Luján H. D. (2011). Regulation of antigenic variation in Giardia lamblia. Annu. Rev. Microbiol. 65, 611–630. doi: 10.1146/annurev-micro-090110-102940
Singer S. M., Yee J., Nash T. E. (1998). Episomal and integrated maintenance of foreign DNA in Giardia lamblia. Mol. Biochem. Parasitol. 92 (1), 59–69. doi: 10.1016/S0166-6851(97)00225-9
Su L. H., Lee G. A., Huang Y. C., Chen Y. H., Sun C. H. (2007). Neomycin and puromycin affect gene expression in Giardia lamblia stable transfection. Mol. Biochem. Parasitol. 156 (2), 124–135. doi: 10.1016/j.molbiopara.2007.07.015
Sun C. H., Chou C. F., Tai J. H. (1998). Stable DNA transfection of the primitive protozoan pathogen Giardia lamblia. Mol. Biochem. Parasitol. 92 (1), 123–132. doi: 10.1016/S0166-6851(97)00239-9
Thompson R. C. (2004). The zoonotic significance and molecular epidemiology of Giardia and giardiasis. Vet. Parasitol. 126 (1-2), 15–35. doi: 10.1016/j.vetpar.2004.09.008
Xu F., Jex A., Svard S. G. (2020). A chromosome-scale reference genome for Giardia intestinalis WB. Sci. Data 7 (1), 38. doi: 10.1038/s41597-020-0377-y
Keywords: model organisms, reverse genetics, untargeted proteomics, experimental proteomics, controls and standards
Citation: Heller M, Braga S, Müller N and Müller J (2020) Transfection With Plasmid Causing Stable Expression of a Foreign Gene Affects General Proteome Pattern in Giardia lamblia Trophozoites. Front. Cell. Infect. Microbiol. 10:602756. doi: 10.3389/fcimb.2020.602756
Received: 04 September 2020; Accepted: 19 November 2020;
Published: 18 December 2020.
Edited by:
Luiz Gustavo Gardinassi, Universidade Federal de Goiás (IPTSP-UFG), BrazilReviewed by:
Rosa Maria Bermudez-Cruz, Instituto Politécnico Nacional de México (CINVESTAV), MexicoBrendan Robert E. Ansell, The University of Melbourne, Australia
Copyright © 2020 Heller, Braga, Müller and Müller. This is an open-access article distributed under the terms of the Creative Commons Attribution License (CC BY). The use, distribution or reproduction in other forums is permitted, provided the original author(s) and the copyright owner(s) are credited and that the original publication in this journal is cited, in accordance with accepted academic practice. No use, distribution or reproduction is permitted which does not comply with these terms.
*Correspondence: Joachim Müller, am9hY2hpbS5tdWVsbGVyQHZldHN1aXNzZS51bmliZS5jaA==; Norbert Müller, bm9yYmVydC5tdWVsbGVyQHZldHN1aXNzZS51bmliZS5jaA==