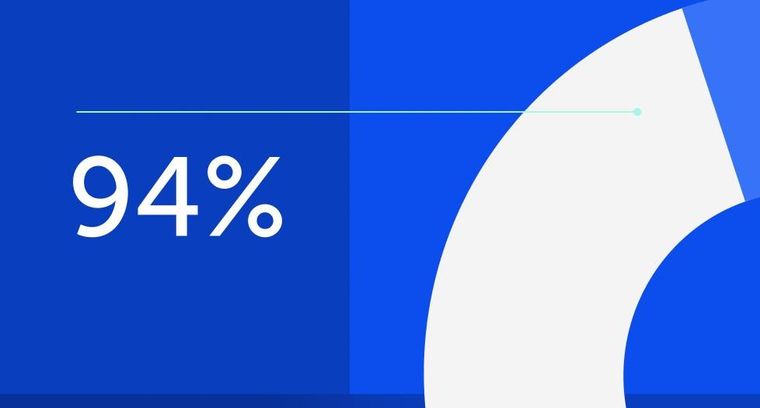
94% of researchers rate our articles as excellent or good
Learn more about the work of our research integrity team to safeguard the quality of each article we publish.
Find out more
ORIGINAL RESEARCH article
Front. Cell. Infect. Microbiol., 28 October 2020
Sec. Fungal Pathogenesis
Volume 10 - 2020 | https://doi.org/10.3389/fcimb.2020.598823
This article is part of the Research TopicFungal Respiratory Infections in Cystic FibrosisView all 13 articles
Scedosporium and Lomentospora species are filamentous fungi that cause a wide range of infections in humans. They are usually found in the lungs of cystic fibrosis (CF) patients and are the second most frequent fungal genus after Aspergillus species. Several studies have been recently performed in order to understand how fungi and bacteria interact in CF lungs, since both can be isolated simultaneously from patients. In this context, many bacterial molecules were shown to inhibit fungal growth, but little is known about how fungi could interfere in bacterial development in CF lungs. Scedosporium and Lomentospora species present peptidorhamnomannans (PRMs) in their cell wall that play crucial roles in fungal adhesion and interaction with host epithelial cells and the immune system. The present study aimed to analyze whether PRMs extracted from Lomentospora prolificans, Scedosporium apiospermum, Scedosporium boydii, and Scedosporium aurantiacum block bacterial growth and biofilm formation in vitro. PRM from L. prolificans and S. boydii displayed the best bactericidal effect against methicillin resistant Staphylococcus aureus (MRSA), Burkholderia cepacia, and Escherichia coli, but not Pseudomonas aeruginosa, all of which are the most frequently found bacteria in CF lungs. In addition, biofilm formation was inhibited in all bacteria tested using PRMs at minimal inhibitory concentration (MIC). These results suggest that PRMs from the Scedosporium and Lomentospora surface seem to play an important role in Scedosporium colonization in CF patients, helping to clarify how these pathogens interact to each other in CF lungs.
Cystic Fibrosis (CF) is an autosomal recessive disease originated from a mutation in the gene encoding the cystic fibrosis transmembrane conductance regulator (CFTR), a cAMP-regulated epithelial chloride channel (Cohen and Prince, 2012). It results in deficient mucociliary functions, leading to the presence of thicker mucus especially in bronchia and pancreas and, consequently, patients suffer of digestive and respiratory problems (Delhaes et al., 2012). The occurrence of CF is estimated to be one in 3,000 or 4,000 births, and one in 25–30 Caucasians carries the mutation in CFTR gene. In the USA about 1,000 people are diagnosed every year, with CF (Sanders and Fink, 2016).
Sticky bronchial mucus is the most challenging problem in CF patients, because it facilitates the occurrence of airway infections and neutrophilic inflammation that are mostly responsible for morbidity and death in these patients (Stoltz et al., 2015). For these reasons, chronic pulmonary infections are frequent and extensively studied. Several pathogens including bacteria and fungi are commonly associated with CF lung colonization. Bacterial pathogens are the most common cause of CF lung infections, in which Staphylococcus aureus and Haemophilus influenza are frequent in children and Burkholderia cepacia complex and Pseudomonas aeruginosa are found especially in adults (Ciofu et al., 2013; Vandeplassche et al., 2019). However, fungal colonization is usually found in the lungs of CF patients, although its dynamics are less clear (Middleton et al., 2013). In this context, the most frequent fungi are Aspergillus, Penicillium, Scedosporium, and Candida species (Ziesing et al., 2016; Engel et al., 2019).
For these reasons, polymicrobial colonization is a well-known situation found in CF lungs and the interaction between bacteria and fungi is a field of extensive studies in order to better understand the complexity and dynamics of polymicrobial relationship in this context (Delhaes et al., 2012). A variety of studies demonstrate that bacteria produce molecules known to inhibit fungal growth. Phenazine and other secreted molecules, for instance, are produced by P. aeruginosa and inhibit Aspergillus fumigatus, Candida albicans, and Scedosporium species (Gibson et al., 2009; Mowat et al., 2010; Kaur et al., 2015; Chen et al., 2018; Briard et al., 2019). On the other hand, little is known about how fungi can affect bacterial growth in CF conditions. C. albicans inhibits P. aeruginosa growth by producing farnesol, a quorum-sensing molecule which plays a role in fungal morphogenesis (Semighini et al., 2006).
In the context of fungal molecules that could influence bacterial growth, the present work studied peptidorhamnomannans (PRMs), glycoconjugates commonly exposed on Scedosporium and Lomentospora cell wall that play important roles in cell adhesion and interaction with host immune system, leading to the release of inflammatory cytokines by phagocytic cells (Lopes et al., 2011). PRMs have been already described and characterized in Scedosporium boydii, Scedosporium apiospermum, and Lomentospora prolificans (Pinto et al., 2001; Lopes et al., 2010; Xisto et al., 2015), as well as in Scedosporium aurantiacum (de Meirelles et al., 2020). The PRMs of these four species possess similar epitopes as well as distinct species-specific oligosaccharide chains. For these reasons, in the present work PRMs extracted and purified from these four species were tested for their effect on bacterial species relevant to CF, such as P. aeruginosa, B. cepacia, methicillin-resistant S. aureus, and Escherichia coli.
B. cepacia (American Type Culture Collection ATCC 25416), E. coli (ATCC 11229), Methicillin Resistant S. aureus—MRSA (ATCC 9393) and P. aeruginosa (ATCC 27853) were studied in this work. Strains were maintained in Luria-Bertani (LB) broth medium (peptone 10 g/l, yeast extract 5 g/l and NaCl 5 g/l) under refrigeration at 4°C. For recent overnight cultures, an aliquot of each strain was spread on LB agar and incubated for 24 h at 37°C, and bacterial suspensions were prepared from scrapings.
L. prolificans (FMR3569 strain), S. apiospermum (RK107-0417 strain) and S. aurantiacum (IHEM21147 strain) were supplied by Dr. J. Guarro, Unitat de Microbiologia, Facultat de Medicina e Institut d`Estudis Avançats, Réus, Spain. S. boydii (HLPB strain) was supplied by Dr. Bodo Wanke, Hospital Evandro Chagas, Instituto Oswaldo Cruz, Rio de Janeiro, Brazil. Strains were maintained at room temperature on Sabouraud (SAB; 2% glucose, 1% peptone, 0.5% yeast extract) agar slants as stock culture. Mycelia were obtained by growing cells in SAB liquid culture medium for seven days at room temperature with shaking.
The crude glycoprotein was extracted from L. prolificans, S. apiospermum, S. boydii, and S. aurantiacum with 0.05M phosphate buffer, pH 7.2, at 100°C for 2 h. After filtration, the solution was dialyzed, evaporated to a small volume, and lyophilized. Peptidorhamnomannans were purified by hexadecyltrimethylammonium bromide (Cetavlon, Merck, Darmstadt, Germany) fractionation, according to Barreto-Bergter et al. (2008).
Minimum inhibitory concentration (MIC) of the PRM isolated from L. prolificans, S. apiospermum, S. boydii, and S. aurantiacum was determined through broth microdilution method in LB broth against bacteria, according to Vieira et al. (2018). MIC was defined as the lowest concentration capable of inhibiting 50% of bacterial growth (MIC%). Tests were performed on 96-well microplates. PRM at final concentrations ranging from 500 μg/ml to 1.95 μg/ml in LB broth medium were added to each well (50 µl/well). From recent overnight cultures, 50 µl of bacterial suspensions with turbidity equivalent to 0.5 tube of the McFarland scale (1.5 x 108 CFU/ml) were added in each well and incubated at 37°C for 24 h. Streptomycin/penicillin was used as reference compounds (8–0.015 µg/ml) and cultures in LB broth without antibiotics were used as control. After determining MIC values, MBC (minimum bactericidal concentration) was determined by subculturing an aliquot of 10 µl from each well that showed complete growth inhibition in LB agar medium without addition of PRM and evaluation of bacterial growth (Vieira et al., 2018). After 24 h, MBC values were defined as the lowest concentration of PRM able to kill bacteria.
For biofilm formation, 50 µl of B. cepacia (ATCC 25416) and MRSA (ATCC9393) bacterial solutions (prepared as described in MIC section) were added in a 96-well plate and mixed with 1/4, 1/2 and 1 MIC of each PRM. After 24 h of incubation at 37°C, the formed biofilm was gently washed with PBS to remove planktonic cells, air-dried for 10 min and stained for 10 min with 0.5% crystal violet (total biofilm biomass) or 1% safranin (biofilm matrix). The staining solutions were discarded and biofilms were gently rinsed twice with sterile distilled water. Crystal violet impregnated in the biofilm was dissolved in 200 µl of ethanol (95%, v/v), and the colored solution was read at an absorbance of 595 nm using a spectrophotometer (Spectra MAX 340 Tunable; Molecular Devices Ltd., San Jose, CA, USA). Safranin was dissolved in water (100 µl), and the absorbance read at 492 nm.
The B. cepacia and MRSA biofilms were prepared as described in the previous section, and after incubation for 24 h at 37°C, the supernatant was removed and 1/2, 1 and 2 MICs of each PRM were added to the formed biofilm. After 24 h at 37°C, the biofilm was gently washed with PBS and crystal violet and safranin staining were performed as in the previous section.
In order to evaluate the effect of fungal PRMs on oxidative stress and membrane integrity and potential, B. cepacia cells were grown in LB broth in the presence of ¼ MIC of all four PRMs for 24 h at 37°C. Furthermore, fluorescence staining was performed using DCFDA (2’,7’-dichlorodihydrofluorescein diacetate), Nile Red and JC-1 (5,5’,6,6’-tetrachloro-1,1’,3,3’tetraethylbenzimidazolylcarbocyanine iodide) (both from Sigma-Aldrich, MO, USA), according to Ullrich et al. (2003); Pereira et al. (2007) and Van Acker et al. (2016), with modifications.
Staining with DCFDA was performed at a final concentration of 10 μM in PBS for 45 min at room temperature in the dark. After washing with PBS, fluorescence (λ excitation = 485 nm, λ emission = 535 nm) was measured using a SpectraMax plate reader (Van Acker et al., 2016).
Nile Red staining at a final concentration of 8 μg/ml was done for 45 min at room temperature in the dark. After washing with PBS, fluorescence (λ excitation = 550 nm, λ emission = 635 nm) was measured as above (Pereira et al., 2007).
JC-1 stain was used at 2.5 μg/ml in PBS 0.01 M, pH 7.2, for 45 min at 37°C in the dark. After washing with PBS, fluorescence (λ excitation = 515 nm, λ emission = 529 nm (green) and 590 nm (red) was measured as above (Ullrich et al., 2003). The membrane potential was determined by the red/green fluorescence intensity ratio.
All statistical analyses were performed using GraphPad Prism 5.0 software (GraphPad, San Diego, CA, USA). A variance two-way ANOVA was performed using Tukey’s and Bonferroni’s comparisons tests to evaluate biofilm formation and inhibition of preformed biofilm.
MIC and MBC values were determined for PRMs isolated from L. prolificans, S. apiospermum, S. boydii, and S. aurantiacum against B. cepacia, E. coli, MRSA, and P. aeruginosa (Table 1). L. prolificans PRM showed considerable inhibitory activity against B. cepacia and MRSA, with MIC of 31.3 and 15.6 µg/ml, respectively. However, for E. coli MIC was 500 µg/ml. S. apiospermum PRM present MIC of 500 µg/ml for B. cepacia and MRSA, and >500 µg/ml for E. coli. S. boydii PRM displayed MIC of 125 µg/ml for B. cepacia, 125 µg/ml for MRSA and >500 µg/ml for E. coli. S. aurantiacum PRM showed MIC at 125 µg/ml for B. cepacia and MRSA, and >500 µg/ml for E. coli. All PRMs tested displayed MIC at >500 µg/ml for P. aeruginosa.
Table 1 Minimal inhibitory concentration (MIC) and minimal bactericidal concentration (MBC) of fungal PRMs isolated from L. prolificans, S. apiospermum, S. boydii and S. aurantiacum tested against B. cepacia, E. coli, MRSA and P. aeruginosa.
Regarding MBC (Table 1), L. prolificans PRM was found to be bactericidal at 31.3 µg/ml for B. cepacia and MRSA, at 500 µg/ml for E. coli, but was not able to kill P. aeruginosa. S. boydii PRM displayed MBC at 500 µg/ml for B. cepacia and 250 µg/ml for MRSA, and was not able to kill either E. coli or P. aeruginosa. S. apiospermum and S. aurantiacum PRMs did not show bactericidal activity for any bacterium tested. Supplementary Material shows bacteria incubated with different concentrations of all PRMs. After 24h at 37°C, aliquots were spotted on LB agar in the absence of any PRM, in order to determine MBCs shown in Table 1.
These results indicate that L. prolificans PRM was most active against bacteria compared to the other PRMs, whereas S. apiospermum PRM was the less potent molecule to inhibit bacterial proliferation. In addition, B. cepacia and MRSA were the most susceptible species when incubated with all four PRMs.
Since B. cepacia and MRSA were the species most susceptible to fungal PRMs, we chose these two species to evaluate whether fungal PRMs could also affect bacterial biofilm formation. In this context, the total formed biomass and the extracellular matrix were evaluated. When B. cepacia was analyzed, L. prolificans and S. apiospermum PRMs could inhibit biomass formation at MIC and ½ MIC (Figure 1A). However, PRM isolated from S. boydii and S. aurantiacum showed a significant inhibition of B. cepacia biomass formation at MIC, ½ MIC and also ¼ MIC (Figure 1A). Biofilm matrix was reduced by all PRMs at 1 MIC, ½ MIC and ¼ MIC (Figure 1B).
Figure 1 Biofilm formation of B cepacia (A, B) and MRSA (C, D) in the presence of PRMs isolated from L. prolificans, S. apiospermum, S. boydii and S. aurantiacum. (A, C) represents the evaluation of biofilm growth. (B, D) show extracellular matrix. Ctl, control without addition of PRM. MIC, ¼ MIC and ½ MIC were based on the values shown in Table 1. The values represent the mean ± S.D. of three independent experiments performed in triplicate. Asterisks denote values statistically different from control. *p < 0.05; **p < 0.01; ***p < 0.001. ns, no significant.
Regarding biofilm formation by MRSA, similar results were observed for biomass (Figure 1C). However, extracellular matrix was reduced at MIC and ½ MIC by L. prolificans and S. apiospermum PRMs, and at all three concentrations used for S. boydii and S. aurantiacum PRMs (Figure 1D).
These data suggest that besides growth inhibition observed in MIC and MBC experiments, biofilm formation is also decreased when bacteria are grown in the presence of fungal PRM.
Due to the inhibitory potential of fungal PRMs on bacterial growth and biofilm formation, we decided to check whether these four PRMs could also be active against preformed biofilms. L. prolificans PRM reduced preformed biomass and extracellular matrix of B. cepacia at MIC and ½ MIC, whereas PRMs isolated from S. apiospermum, S. boydii and S. aurantiacum showed similar results at all concentrations tested (Figures 2A, B). When preformed MRSA biofilm was evaluated, all four PRMs decreased biomass matrix at all concentrations used (Figures 2C, D), except for L. prolificans PRM at ¼ MIC, which was not able to significantly reduce MRSA matrix (Figure 2D).
Figure 2 Effect of PRMs isolated from L. prolificans, S. apiospermum, S. boydii and S. aurantiacum on preformed biofilm of B cepacia (A, B) and MRSA (C, D). (A, C) represent the evaluation of biofilm formation. (B, D) show extracellular matrix. Ctl, control without addition of PRM. MIC, ¼ MIC and ½ MIC were based on the values shown in Table 1. The values represent the mean ± S.D. of three independent experiments performed in triplicate. Asterisks denote values statistically different from control. *p < 0.05; **p < 0.01; ***p < 0.001. ns, no significant.
These results suggest that fungal PRMs affect not only bacterial growth and the process of biofilm formation, but they affect also preformed bacterial biofilms.
In order to understand the effects of PRMs on bacteria, oxidative stress was evaluated in bacterial cells, as well as the integrity and the potential of the membrane. To perform these analyses, B. cepacia was chosen as a representative model due to its relevance in CF patients with worse prognosis.
The production of reactive oxygen species (ROS), which provoke oxidative stress, was measured using DCFDA staining. DCFDA is oxidized by ROS inside the cells to form fluorescent 2’, 7’-dichlorofluorescein (DCF). In this context, L. prolificans PRM was the only molecule able to increase ROS production, indicating induction of oxidative stress in B. cepacia (Figure 3A). The other PRMs did not influence ROS production when compared to the control.
Figure 3 Effect of PRMs isolated from L. prolificans, S. apiospermum, S. boydii and S. aurantiacum on oxidative stress (A), membrane integrity (B) and membrane potential (C) of B cepacia. Oxidative stress, membrane integrity and potential were measured by DCFDA, Nile Red and JC-1 staining, respectively. Bact-, unstained control. Bact+, stained cells in the absence of PRM. L. prol, L prolificans. S. apio, S. apiospermum. S. boy, S. boydii. S. aur, S. aurantiacum. ¼ MIC was based on the values shown in Table 1. The values represent the mean ± S.D. of three independent experiments performed in triplicate. Asterisks denote values statistically different from control. *p < 0.05; **p < 0.01; ****p < 0.001.
Disruption of membrane integrity is a mechanism by which some compounds cause cell killing. For this reason, we used Nile Red staining that binds to polyhydroxyalkanoates (PHAs), common prokaryotic storage compounds of carbon and energy present in intracellular lipid droplets (Jendrossek, 2009). S. boydii PRM decreased Nile Red fluorescent intensity, suggesting that it affects lipid integrity in B. cepacia, whereas the other PRMs did not alter bacterial lipids when compared to the control (Figure 3B).
Reduction of energy generation as consequence of cell killing could be measured by evaluating the membrane potential using JC-1 staining. All fungal PRMs tested caused a decrease in membrane potential compared to the control (untreated cells), indicating that PRMs induce a lower membrane polarization in B. cepacia (Figure 3C).
Cystic fibrosis patients exhibit a reduced clearance of mucus from the lungs which leads to chronic infections caused by bacteria and fungus, being the primary cause of mortality (Lipuma, 2010; Cohen and Prince, 2012). The study of polymicrobial infections in this environment is not only important for understanding the pathogenesis but also for providing insights for the development of new antimicrobial drugs, since the relationships between these microorganisms in the context of CF are frequently antagonistic and therefore may reveal new approaches for pathogen inhibition (Peleg et al., 2010).
Bacteria play a major part in lungs of CF patients. They are usually the first microorganisms to colonize this site and are the cause of a common exacerbated inflammatory response observed in the respiratory tract. Guss et al. (2011) identified eight bacterial phyla, including more than 60 genera, in lungs from CF patient, therefore evidencing the diversity of this microbial community. However, as previously mentioned, there are some more prevalent species colonizing the pulmonary tract in CF, such as P. aeruginosa, S. aureus, and B. cepacia. The presence of B. cepacia is considered a threat to CF patients, due to its high patient-to-patient transmissibility and antibiotic resistance, as well as a poorer prognosis of the disease outcome (Kenna et al., 2017). A recent study showed that E. coli was recovered from the sputum of up to 25% of patients with CF, mainly from those with poor nutritional status and lung function, although this did not predict clinical decline (Edwards et al., 2020). In our study, P. aeruginosa, S. aureus, B. cepacia, and E. coli were used as representative species for CF to evaluate the bacterial inhibitory effect of fungal PRMs isolated from L. prolificans, S. boydii, S. apiospermum, and S. aurantiacum.
Bacteria are the main cause of CF lung infections, but fungal species are also able to chronically colonize the respiratory tract of these patients. It is known that fungi from the genus Scedosporium are the third most prevalent in CF patients after Aspergillus and Penicillium. S. apiospermum is the most frequent (28.6%), followed by S. boydii (19.3%), S. aurantiacum (10.0%), and L. prolificans (3.6%) (Engel et al., 2019). Other studies described Scedosporium species as the second most prevalent filamentous fungi in CF patients (Pihet et al., 2009; Sudfeld et al., 2010).
Considering the polymicrobial pattern of CF lung infections, it is relevant to study the bacteria-fungi interactions and the molecules produced by fungi that could be involved in these interactions. In this context, the present work used PRMs isolated from L. prolificans (Barreto-Bergter et al., 2008), S. boydii (Pinto et al., 2005), S. apiospermum (Barreto-Bergter et al., 2011), and S. aurantiacum (de Meirelles et al., 2020). These glycoconjugates are exposed on fungal surfaces, since they are recognized by antibodies anti-PRM (Lopes et al., 2010; Xisto et al., 2015). Cell culture supernatants of Scedosporium and Lomentospora species for the presence of PRM were not examined in this manuscript. In vitro studies show that variable amounts of galactomannan (GM), a component of the cell wall of several Aspergillus species as well of a diverse range of fungi, are released during early logarithmic growth (Latgé et al., 1994). In vivo it is known that galactomannans can be detected in the bronchoalveolar lavage of patients with invasive aspergillosis, released from A. fumigatus cell wall during infections (Sarfati et al., 1995; He et al., 2012; Teering et al., 2014). Further studies, not only focused on the characterization of extracellular PRM from Scedosporium and Lomentospora species but also on carbohydrate-containing molecules isolated from cell wall or secreted into the cell culture supernatant of these species cultivated in synthetic cystic fibrosis sputum medium (SCFM) (Han et al., 2017), that mimics the environment in the CF lungs species will improve the knowledge of this complex bacteria-fungus interaction in CF.
In addition, PRMs of S. boydii and S. apiospermum are involved in the adhesion to an epithelial cell line and in intracellular survival within macrophages, respectively (Pinto et al., 2004; Lopes et al., 2010). PRMs isolated from S. boydii and L. prolificans also play a role in host immune stimulation, increasing the release of pro-inflammatory cytokines such as TNF-α (Figueiredo et al., 2010; Xisto et al., 2015).
Taken together, all data presented in our study demonstrated that PRMs possess antibacterial and anti-biofilm effects. Regarding the inhibition of bacterial growth, PRM from L. prolificans was the most effective, followed by those from S. aurantiacum, S. boydii, and S. apiospermum, against B. cepacia and MRSA, which were the most susceptible bacteria. P. aeruginosa and E. coli seemed to be resistant to all PRM. Considering the anti-biofilm effect, PRM from S. boydii and S. aurantiacum showed to be the most effective, because ¼ MIC was necessary to observe B. cepacia and MRSA biofilm reduction.
Although S. apiospermum is described as the most common Scedosporium species identified in lungs of CF patients, MIC and MBC results obtained in this work showed that its PRM was the less potent molecule against bacteria, whereas L. prolificans PRM, which is the less frequent fungi of Scedosporium/Lomentospora group isolated from CF patients, was the most active against bacteria. In addition, all PRMs presented high MICs against P. aeruginosa and E. coli. Rhamnose-containing molecules, such as the rhamnolipid biosurfactant isolated from a sponge associated marine fungus Aspergillus spp has been described with antimicrobial activity against C. albicans and some Gram-negative bacteria (Kiran et al., 2009).
Variation in the antibacterial potential among PRMs could be due to differences in their oligosaccharide chains. PRMs isolated from the Scedosporium and Lomentospora species used in this work share similar epitopes, such as the α-Rhap-(1→3) α-Manp-(1→2)- α-Manp (Lopes et al., 2011; de Meirelles et al., 2020). On the other hand, structural differences are observed among these PRMs, which could result in changes in the antimicrobial activity. L. prolificans PRM, for instance, contains a pentasaccharide lacking the β-Galp side-chain (Barreto-Bergter et al., 2008) and a high proportion of 2-O-substituted Rhap units, which are absent in S. boydii and S. apiospermum PRMs (Pinto et al., 2005; Barreto-Bergter et al., 2008; Barreto-Bergter et al., 2011). Nevertheless, more studies are needed in order to clarify the reason why L. prolificans PRM present a higher activity against the bacteria tested when compared to the other PRMs.
Due to the relevance of biofilms in infection, the influence of PRM on bacterial biofilms was also evaluated. All four PRMs inhibit biofilm formation and are active against preformed biofilms of B. cepacia and MRSA at MIC values. Extracellular matrix was also reduced by PRMs, indicating that bacterial biofilms were weakened in the presence of PRM. Several glycoconjugates and polysaccharides have been already described as potent inhibitors of bacterial biofilms. C-fucosylpeptide and galactosylated peptide dendrimers have been shown to inhibit biofilm formation and to disperse preformed biofilm by interfering with P. aeruginosa LecA and B, a lectin molecule responsible for bacterial adherence on host tissues (Kadam et al., 2011; Reymond et al., 2013). Human and plant oligosaccharides, such as galactooligosaccharides, have already been known to block bacterial adhesion to surfaces, decreasing biofilm formation of E. coli, Burkholderia pseudomallei and other bacteria (Thomas and Brooks, 2004; Shoaf et al., 2006; Lane et al., 2010; Quintero et al., 2011; Rendueles et al., 2013). Regarding fungal molecules, chitosan, a deacetylated derivative of chitin found on the fungal cell wall is recognized as a potent antibacterial agent and inhibits growth and biofilm formation by different bacteria, such as S. aureus and E. coli (Asli et al., 2017). For other fungal glycoconjugates, such as the rhamnolipid biosurfactant of Aspergillus spp., antimicrobial activity against Streptococcus spp., Micrococcus luteus, and Enterococcus faecalis has also been reported (Kiran et al., 2009).
In order to evaluate some possible mechanisms involved in bacterial death, we evaluated oxidative stress, membrane integrity and membrane potential in B. cepacia treated with PRMs using DCFDA, Nile Red and JC-1 staining, respectively. L. prolificans PRM increased ROS production when compared to control. PRMs isolated from S. boydii, S. apiospermum and S. aurantiacum did not cause any effect, suggesting that structural differences among PRMs might be related to ROS production by B. cepacia. On the other hand, a decrease of the membrane potential was observed in B. cepacia in the presence of all PRMs used. Membrane integrity was only affected when bacterial cells were treated with S. boydii PRM. Membrane alteration detected by Nile Red is well known in bacteria treated with penicillin, which leads to disorganized cell surface (Pereira et al., 2007). However, few information about ROS induction, membrane disorganization and changes in membrane potential caused by glycoconjugates and polysaccharides is described in the literature.
The knowledge of how fungi can influence bacterial growth in the polymicrobial colonization of lungs from CF patients is still scarce. Several studies have been performed in order to understand how bacteria, especially P. aeruginosa, inhibit competitors in CF airways (Gibson et al., 2009; Mowat et al., 2010; Delhaes et al., 2012; Kaur et al., 2015; Chen et al., 2018; Briard et al., 2019). However, studies on the mechanisms by which fungal molecules inhibit bacteria are rare. Therefore, the present work aimed to evaluate the effect of the main glycoconjugate found on the Scedosporium and Lomentospora cell wall on bacterial growth. PRMs from Scedosporium and Lomentospora species were able to kill bacteria associated to lungs of CF patients and interfere with both, bacterial biofilm formation and preformed biofilms. The mechanisms involved in B. cepacia death are different among the PRMs tested in this work, and can be associated with structural differences in these PRMs. Since CF prognosis is hard for all patients and infections of the respiratory tract are the main cause of mortality in this population, studies that contribute to the understanding of the dynamics of bacteria-fungi interactions in the context of CF are crucial for developing better approaches to increase the patients’ quality of life.
The raw data supporting the conclusions of this article will be made available by the authors, without undue reservation.
EO, MX, RRP, and VR conceived, designed, and performed the experiments. EO, MX, RRP, VR, and EBB analyzed the experiments. MX, RRP, and EBB drafted the manuscript. All authors contributed to the article and approved the submitted version.
This study was financed in part by the Coordenação de Aperfeiçoamento de Pessoal de Nível Superior—Brasil (CAPES)—Finance Code 001; Conselho Nacional de Desenvolvimento Científico e Tecnológico (CNPq) and Fundação de Amparo à Pesquisa do estado do Rio de Janeiro (Faperj).
The authors declare that the research was conducted in the absence of any commercial or financial relationships that could be construed as a potential conflict of interest.
The authors thank Walter Oelemann for English revision and the Universidade Federal do Rio de Janeiro (UFRJ) for the structure to develop the work.
The Supplementary Material for this article can be found online at: https://www.frontiersin.org/articles/10.3389/fcimb.2020.598823/full#supplementary-material
Supplementary Material 1 | Growth of B. cepacia, E. coli, MRSA and P. aeruginosa in the presence of different concentrations (0.98 – 500 μg/ml) of PRM isolated from L. prolificans, S. apiospermum, S. boydii and S. aurantiacum.
Asli A., Brouillette E., Ster C., Ghinet M. G., Brzezinski R., Lacasse P., et al. (2017). Antibiofilm and antibacterial effects of specific chitosan molecules on Staphylococcus aureus isolates associated with bovine mastitis. PloS One 12 (5), e0176988. doi: 10.1371/journal.pone.0176988
Barreto-Bergter E., Sassaki G. L., Wagner R., Souza L. M., Souza M. V., Pinto M. R., et al. (2008). The opportunistic fungal pathogen Scedosporium prolificans: carbohydrate epitopes of its glycoproteins. Int. J. Biol. Macromol. 42 (2), 93–102. doi: 10.1016/j.ijbiomac.2007.09.015
Barreto-Bergter E., Sassaki G. L., Souza L. M., Rollin R. R., Wagner R., Bittencourt V. C. B., et al. (2011). Carbohydrate epitopes in glycoprotein from the opportunistic fungal pathogen Scedosporium apiospermum. Carbohydr. Polym. 85 (2), 349–355. doi: 10.1016/j.carbpol.2011.02.033
Briard B., Mislin G. L. A., Latge J. P., Beauvais A. (2019). Interactions between Aspergillus fumigatus and Pulmonary Bacteria: Current State of the Field, New Data, and Future Perspective. J. Fungi (Basel) 5 (2), 48. doi: 10.3390/jof5020048
Chen S. C., Patel S., Meyer W., Chapman B., Yu H., Byth K., et al. (2018). Pseudomonas aeruginosa Inhibits the Growth of Scedosporium and Lomentospora In Vitro. Mycopathologia 183 (1), 251–261. doi: 10.1007/s11046-017-0140-x
Ciofu O., Hansen C. R., Hoiby N. (2013). Respiratory bacterial infections in cystic fibrosis. Curr. Opin. Pulm. Med. 19 (3), 251–258. doi: 10.1097/MCP.0b013e32835f1afc
Cohen T. S., Prince A. (2012). Cystic fibrosis: a mucosal immunodeficiency syndrome. Nat. Med. 18 (4), 509–519. doi: 10.1038/nm.2715
de Meirelles J. V., Xisto M., Rollin-Pinheiro R., Serrato R. V., Haido R. M. T., Barreto-Bergter E. (2020). Peptidorhamanomannan: A surface fungal glycoconjugate from Scedosporium aurantiacum and Scedosporium minutisporum and its recognition by macrophages. Med. Mycol. 0 (0), 12. doi: 10.1093/mmy/myaa065
Delhaes L., Monchy S., Frealle E., Hubans C., Salleron J., Leroy S., et al. (2012). The airway microbiota in cystic fibrosis: a complex fungal and bacterial community–implications for therapeutic management. PloS One 7 (4), e36313. doi: 10.1371/journal.pone.0036313
Edwards B. D., Somayaji R., Greysson-Wong J., Izydorczyk C., Waddell B., Storey D. G., et al. (2020). Clinical Outcomes Associated With Escherichia coli Infections in Adults With Cystic Fibrosis: A Cohort Study. Open Forum Infect. Dis. 7 (1), ofz476. doi: 10.1093/ofid/ofz476
Engel T. G. P., Slabbers L., de Jong C., Melchers W. J. G., Hagen F., Verweij P. E., et al. (2019). Prevalence and diversity of filamentous fungi in the airways of cystic fibrosis patients - A Dutch, multicentre study. J. Cyst. Fibros. 18 (2), 221–226. doi: 10.1016/j.jcf.2018.11.012
Figueiredo R. T., Fernandez P. L., Dutra F. F., Gonzalez Y., Lopes L. C., Bittencourt V. C., et al. (2010). TLR4 recognizes Pseudallescheria boydii conidia and purified rhamnomannans. J. Biol. Chem. 285 (52), 40714–40723. doi: 10.1074/jbc.M110.181255
Gibson J., Sood A., Hogan D. A. (2009). Pseudomonas aeruginosa-Candida albicans interactions: localization and fungal toxicity of a phenazine derivative. Appl. Environ. Microbiol. 75 (2), 504–513. doi: 10.1128/AEM.01037-08
Guss A. M., Roeselers G., Newton I. L., Young C. R., Klepac-Ceraj V., Lory S., et al. (2011). Phylogenetic and metabolic diversity of bacteria associated with cystic fibrosis. ISME J. 5 (1), 20–29. doi: 10.1038/ismej.2010.88
Han Z., Kautto L., Nevalainen H. (2017). Secretion of Proteases by an Opportunistic Fungal Pathogen Scedosporium aurantiacum. PloS One 12 (1), e0169403. doi: 10.1371/journal.pone.0169403
He H., Ding L., Sun B., Li F., Zhan Q. (2012). Role of galactomannan determinations in bronchoalveolar lavage fluid samples from critically ill patients with chronic obstructive pulmonary disease for the diagnosis of invasive pulmonary aspergillosis: a prospective study. Crit Care. 16 (4), R138.
Jendrossek D. (2009). Polyhydroxyalkanoate granules are complex subcellular organelles (carbonosomes). J. Bacteriol. 191 (10), 3195–3202. doi: 10.1128/JB.01723-08
Kadam R. U., Bergmann M., Hurley M., Garg D., Cacciarini M., Swiderska M. A., et al. (2011). A glycopeptide dendrimer inhibitor of the galactose-specific lectin LecA and of Pseudomonas aeruginosa biofilms. Angew. Chem. Int. Ed. Engl. 50 (45), 10631–10635. doi: 10.1002/anie.201104342
Kaur J., Pethani B. P., Kumar S., Kim M., Sunna A., Kautto L., et al. (2015). Pseudomonas aeruginosa inhibits the growth of Scedosporium aurantiacum, an opportunistic fungal pathogen isolated from the lungs of cystic fibrosis patients. Front. Microbiol. 6, 866. doi: 10.3389/fmicb.2015.00866
Kenna D. T. D., Lilley D., Coward A., Martin K., Perry C., Pike R., et al. (2017). Prevalence of Burkholderia species, including members of Burkholderia cepacia complex, among UK cystic and non-cystic fibrosis patients. J. Med. Microbiol. 66 (4), 490–501. doi: 10.1099/jmm.0.000458
Kiran G. S., Hema T. A., Gandhimathi R., Selvin J., Thomas T. A., Rajeetha Ravji T., et al. (2009). Optimization and production of a biosurfactant from the sponge-associated marine fungus Aspergillus ustus MSF3. Colloids Surf. B Biointerfaces 73 (2), 250–256. doi: 10.1016/j.colsurfb.2009.05.025
Lane J. A., Mehra R. K., Carrington S. D., Hickey R. M. (2010). The food glycome: a source of protection against pathogen colonization in the gastrointestinal tract. Int. J. Food Microbiol. 142 (1–2), 1–13. doi: 10.1016/j.ijfoodmicro.2010.05.027
Latgé J. P., Kobayashi H., Debeaupuis J. P., Diaquin M., Sarfati J., Wieruszeski J. M., et al. (1994). Chemical and immunological characterization of the extracellular galactomannan of Aspergillus fumigatus. Infect. Immun. 62 (12), 5424–5433. doi: 10.1128/iai.62.12.5424-5433.1994
Lipuma J. J. (2010). The changing microbial epidemiology in cystic fibrosis. Clin. Microbiol. Rev. 23 (2), 299–323. doi: 10.1128/CMR.00068-09
Lopes L. C., Rollin-Pinheiro R., Guimarães A. J., Bittencourt V. C., Martinez L. R., Koba W., et al. (2010). Monoclonal antibodies against peptidorhamnomannans of Scedosporium apiospermum enhance the pathogenicity of the fungus. PloS Negl. Trop. Dis. 4 (10), e853. doi: 10.1371/journal.pntd.0000853
Lopes L. C., da Silva M. I., Bittencourt V. C., Figueiredo R. T., Rollin-Pinheiro R., Sassaki G. L., et al. (2011). Glycoconjugates and polysaccharides from the Scedosporium/Pseudallescheria boydii complex: structural characterisation, involvement in cell differentiation, cell recognition and virulence. Mycoses 54 Suppl 3, 28–36. doi: 10.1111/j.1439-0507.2011.02105.x
Middleton P. G., Chen S. C., Meyer W. (2013). Fungal infections and treatment in cystic fibrosis. Curr. Opin. Pulm. Med. 19 (6), 670–675. doi: 10.1097/MCP.0b013e328365ab74
Mowat E., Rajendran R., Williams C., McCulloch E., Jones B., Lang S., et al. (2010). Pseudomonas aeruginosa and their small diffusible extracellular molecules inhibit Aspergillus fumigatus biofilm formation. FEMS Microbiol. Lett. 313 (2), 96–102. doi: 10.1111/j.1574-6968.2010.02130.x
Peleg A. Y., Hogan D. A., Mylonakis E. (2010). Medically important bacterial-fungal interactions. Nat. Rev. Microbiol. 8 (5), 340–349. doi: 10.1038/nrmicro2313
Pereira S. F., Henriques A. O., Pinho M. G., de Lencastre H., Tomasz A. (2007). Role of PBP1 in cell division of Staphylococcus aureus. J. Bacteriol. 189 (9), 3525–3531. doi: 10.1128/JB.00044-07
Pihet M., Carrere J., Cimon B., Chabasse D., Delhaes L., Symoens F., et al. (2009). Occurrence and relevance of filamentous fungi in respiratory secretions of patients with cystic fibrosis–a review. Med. Mycol. 47 (4), 387–397. doi: 10.1080/13693780802609604
Pinto M. R., Mulloy B., Haido R. M., Travassos L. R., Barreto Bergter E. (2001). A peptidorhamnomannan from the mycelium of Pseudallescheria boydii is a potential diagnostic antigen of this emerging human pathogen. Microbiology 147 (Pt 6), 1499–1506. doi: 10.1099/00221287-147-6-1499
Pinto M. R., de Sá A. C., Limongi C. L., Rozental S., Santos A. L., Barreto-Bergter E. (2004). Involvement of peptidorhamnomannan in the interaction of Pseudallescheria boydii and HEp2 cells. Microbes Infect. 6 (14), 1259–1267. doi: 10.1016/j.micinf.2004.07.006
Pinto M. R., Gorin P. A., Wait R., Mulloy B., Barreto-Bergter E. (2005). Structures of the O-linked oligosaccharides of a complex glycoconjugate from Pseudallescheria boydii. Glycobiology 15 (10), 895–904. doi: 10.1093/glycob/cwi084
Quintero M., Maldonado M., Perez-Munoz M., Jimenez R., Fangman T., Rupnow J., et al. (2011). Adherence inhibition of Cronobacter sakazakii to intestinal epithelial cells by prebiotic oligosaccharides. Curr. Microbiol. 62 (5), 1448–1454. doi: 10.1007/s00284-011-9882-8
Rendueles O., Kaplan J. B., Ghigo J. M. (2013). Antibiofilm polysaccharides. Environ. Microbiol. 15 (2), 334–346. doi: 10.1111/j.1462-2920.2012.02810.x
Reymond J. L., Bergmann M., Darbre T. (2013). Glycopeptide dendrimers as Pseudomonas aeruginosa biofilm inhibitors. Chem. Soc. Rev. 42 (11), 4814–4822. doi: 10.1039/c3cs35504g
Sanders D. B., Fink A. K. (2016). Background and Epidemiology. Pediatr. Clin. North Am. 63 (4), 567–584. doi: 10.1016/j.pcl.2016.04.001
Sarfati J., Boucias D. G., Latgé J. P. (1995). Antigens of Aspergillus fumigatus produced in vivo. J. Med. Vet. Mycol. 33 (1), 9–14.
Semighini C. P., Hornby J. M., Dumitru R., Nickerson K. W., Harris S. D. (2006). Farnesol-induced apoptosis in Aspergillus nidulans reveals a possible mechanism for antagonistic interactions between fungi. Mol. Microbiol. 59 (3), 753–764. doi: 10.1111/j.1365-2958.2005.04976.x
Shoaf K., Mulvey G. L., Armstrong G. D., Hutkins R. W. (2006). Prebiotic galactooligosaccharides reduce adherence of enteropathogenic Escherichia coli to tissue culture cells. Infect. Immun. 74 (12), 6920–6928. doi: 10.1128/IAI.01030-06
Stoltz D. A., Meyerholz D. K., Welsh M. J. (2015). Origins of cystic fibrosis lung disease. N. Engl. J. Med. 372 (16), 1574–1575. doi: 10.1056/NEJMc1502191
Sudfeld C. R., Dasenbrook E. C., Merz W. G., Carroll K. C., Boyle M. P. (2010). Prevalence and risk factors for recovery of filamentous fungi in individuals with cystic fibrosis. J. Cyst. Fibros. 9 (2), 110–116. doi: 10.1016/j.jcf.2009.11.010
Teering S., Verreth A., Peeters A., Van Regenmortel N., De Laet I., Schoonheydt K., et al (2014). Prognostic value of serum galactomannan in mixed ICU patients: a retrospective observational study. Anaesthesiol. Intensive Ther. 46 (3), 145-54
Thomas R., Brooks T. (2004). Common oligosaccharide moieties inhibit the adherence of typical and atypical respiratory pathogens. J. Med. Microbiol. 53 (Pt 9), 833–840. doi: 10.1099/jmm.0.45643-0
Ullrich S., Berchtold S., Boehmer C., Fillon S., Jendrossek V., Palmada M., et al. (2003). Pseudomonas aeruginosa activates Cl- channels in host epithelial cells. Pflugers Arch. 447 (1), 23–28. doi: 10.1007/s00424-003-1136-6
Van Acker H., Gielis J., Acke M., Cools F., Cos P., Coenye T. (2016). The Role of Reactive Oxygen Species in Antibiotic-Induced Cell Death in Burkholderia cepacia Complex Bacteria. PloS One 11 (7), e0159837. doi: 10.1371/journal.pone.0159837
Vandeplassche E., Tavernier S., Coenye T., Crabbe A. (2019). Influence of the lung microbiome on antibiotic susceptibility of cystic fibrosis pathogens. Eur. Respir. Rev. 28 (152), 190041. doi: 10.1183/16000617.0041-2019
Vieira E. R., Xisto M., Pele M. A., Alviano D. S., Alviano C. S., Barreto-Bergter E., et al. (2018). Monohexosylceramides from Rhizopus Species Isolated from Brazilian Caatinga: Chemical Characterization and Evaluation of Their Anti-Biofilm and Antibacterial Activities. Molecules 23 (6), 1331. doi: 10.3390/molecules23061331
Xisto M. I., Bittencourt V. C., Liporagi-Lopes L. C., Haido R. M., Mendonça M. S., Sassaki G., et al. (2015). O-glycosylation in cell wall proteins in Scedosporium prolificans is critical for phagocytosis and inflammatory cytokines production by macrophages. PloS One 10 (4), e0123189. doi: 10.1371/journal.pone.0123189
Keywords: cystic fibrosis, peptidorhamnomannan, Scedosporium, bacteria, interaction
Citation: de Oliveira EB, Xisto MIDdS, Rollin-Pinheiro R, Rochetti VP and Barreto-Bergter E (2020) Peptidorhamnomannans From Scedosporium and Lomentospora Species Display Microbicidal Activity Against Bacteria Commonly Present in Cystic Fibrosis Patients. Front. Cell. Infect. Microbiol. 10:598823. doi: 10.3389/fcimb.2020.598823
Received: 25 August 2020; Accepted: 07 October 2020;
Published: 28 October 2020.
Edited by:
Jean-Philippe Bouchara, Université d’Angers, FranceReviewed by:
Aitor Rementeria, University of the Basque Country, SpainCopyright © 2020 de Oliveira, Xisto, Rollin-Pinheiro, Rochetti and Barreto-Bergter. This is an open-access article distributed under the terms of the Creative Commons Attribution License (CC BY). The use, distribution or reproduction in other forums is permitted, provided the original author(s) and the copyright owner(s) are credited and that the original publication in this journal is cited, in accordance with accepted academic practice. No use, distribution or reproduction is permitted which does not comply with these terms.
*Correspondence: Eliana Barreto-Bergter, eliana.bergter@micro.ufrj.br
†These authors have contributed equally to this work
Disclaimer: All claims expressed in this article are solely those of the authors and do not necessarily represent those of their affiliated organizations, or those of the publisher, the editors and the reviewers. Any product that may be evaluated in this article or claim that may be made by its manufacturer is not guaranteed or endorsed by the publisher.
Research integrity at Frontiers
Learn more about the work of our research integrity team to safeguard the quality of each article we publish.