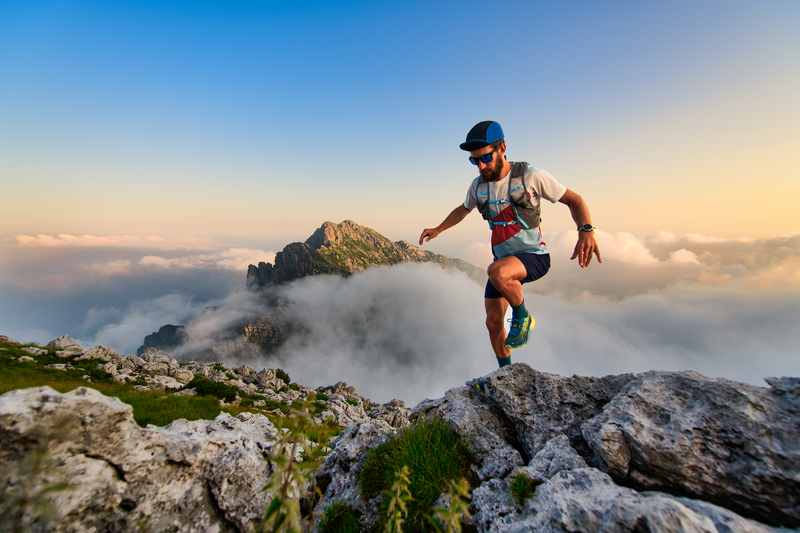
95% of researchers rate our articles as excellent or good
Learn more about the work of our research integrity team to safeguard the quality of each article we publish.
Find out more
ORIGINAL RESEARCH article
Front. Cell. Infect. Microbiol. , 17 December 2020
Sec. Parasite and Host
Volume 10 - 2020 | https://doi.org/10.3389/fcimb.2020.591046
This article is part of the Research Topic Malaria Targeting Toolkit: Host-Parasite Interaction View all 11 articles
Chimeric rodent malaria parasites with the endogenous circumsporozoite protein (csp) gene replaced with csp from the human parasites Plasmodium falciparum (Pf) and P. vivax (Pv) are used in preclinical evaluation of CSP vaccines. Chimeric rodent parasites expressing PfCSP have also been assessed as whole sporozoite (WSP) vaccines. Comparable chimeric P. falciparum parasites expressing CSP of P. vivax could be used both for clinical evaluation of vaccines targeting PvCSP in controlled human P. falciparum infections and in WSP vaccines targeting P. vivax and P. falciparum. We generated chimeric P. falciparum parasites expressing both PfCSP and PvCSP. These Pf-PvCSP parasites produced sporozoite comparable to wild type P. falciparum parasites and expressed PfCSP and PvCSP on the sporozoite surface. Pf-PvCSP sporozoites infected human hepatocytes and induced antibodies to the repeats of both PfCSP and PvCSP after immunization of mice. These results support the use of Pf-PvCSP sporozoites in studies optimizing vaccines targeting PvCSP.
Plasmodium falciparum (Pf) and P. vivax (Pv) are two major human malaria parasites that threaten public health, mainly in tropical areas. Malaria parasites are transmitted by Anopheles mosquitoes where they develop into infectious sporozoites that reside in the salivary glands. After a bite of an infected mosquito, sporozoites enter the blood stream of the mammalian host and migrate to the liver where they invade hepatocytes. Inside hepatocytes, parasites replicate to form thousands of daughter merozoites, which are released into the blood and invade erythrocytes. The pre-erythrocytic stages of Plasmodium parasites, i.e. sporozoites and liver stages, are attractive targets of leading malaria vaccine candidates, including RTS,S, the most advanced subunit vaccine against P. falciparum tested in Phase III clinical trials (Kester et al., 2009; Cohen et al., 2010; Agnandji et al., 2012; Olotu et al., 2016). RTS,S is based on the circumsporozoite protein (CSP), a major surface protein expressed by Plasmodium sporozoites and early liver stages which plays a critical role in sporozoite formation, invasion of mosquito salivary glands and invasion of host hepatocytes (Ménard, 2000; Sinnis and Coppi, 2007; Coppi et al., 2011). This protein contains a highly conserved central repeat region flanked by N- and C -terminal regions. B-cell responses to PfCSP target predominantly the protein’s central NANP repeat region, generating antibodies capable of protecting animal models from Plasmodium infection and NANP antibodies induced by RTS,S immunization are associated with clinical protection (White et al., 2013; Foquet et al., 2014; Sack et al., 2014; Triller et al., 2017). CSP from P. vivax also contains an immunogenic central repeat region with two major PvCSP alleles, VK210 and VK247 considered as important targets for a vaccine (Yadava and Waters, 2017). So far pre-erythrocytic subunit vaccines targeting CSP, including RTS,S, have shown low to modest protective efficacy in clinical trials and in field studies (Agnandji et al., 2015; Hoffman et al., 2015; Clemens and Moorthy, 2016; Long and Zavala, 2016; Olotu et al., 2016; Healer et al., 2017; Mahmoudi and Keshavarz, 2017) emphasizing the need to improve CSP-based vaccination approaches which requires efficient preclinical and clinical evaluation of different vaccines. Clinical evaluation of P. falciparum vaccines is greatly aided by the ability to vaccinate individuals and then examine vaccine efficacy in controlled human malaria infections (CHMI) (Sauerwein et al., 2011; Spring et al., 2014; Bijker et al., 2016; Stanisic et al., 2018). Although CHMI for P. vivax has been developed (Arévalo-Herrera et al., 2016; Bennett et al., 2016; Payne et al., 2017; Hall et al., 2019), the lack of a continuous in vitro culture system for P. vivax blood stages limits the availability of P. vivax sporozoites for clinical human infections. In addition, since P. vivax forms dormant hypnozoites in the liver, which can causing infection relapses, safe and effective means for clearance of hypnozoites are essential for P. vivax CHMI studies (Payne et al., 2017).
In multiple preclinical studies, the evaluation of the efficacy of CSP-based vaccines and anti-CSP antibodies has been performed using chimeric rodent malaria parasites expressing CSP from either P. falciparum or P. vivax (Espinosa et al., 2013; Gimenez et al., 2017; Salman et al., 2017; Vijayan et al., 2017; de Camargo et al., 2018; Marques et al., 2020). The availability of comparable chimeric P. falciparum parasites expressing PvCSP would permit the analysis of protective efficacy of PvCSP-based vaccines in clinical studies using P. falciparum-based CHMI, bypassing the need for P. vivax sporozoite production and hypnozoite elimination. However, in contrast to efficient PvCSP expression and sporozoite production in chimeric rodent parasites, we found that chimeric P. falciparum lines with the PfCSP gene replaced by PvCSP, failed to produce salivary gland sporozoites, indicating that PvCSP cannot fully complement the role of PfCSP in P. falciparum sporozoite formation (Marin-Mogollon et al., 2018). To develop chimeric P. falciparum sporozoites expressing PvCSP, we therefore aimed at generating a chimeric P. falciparum line expressing both the endogenous PfCSP and an engineered PvCSP. It has been shown that introducing the P. falciparum csp gene as an additional copy into the genome of the rodent parasite P. berghei (Pb) results in development of viable and infective sporozoites, expressing both PbCSP and PfCSP in pre-erythrocytic stages (Mendes et al., 2018a; Mendes et al., 2018b; Reuling et al., 2020). We used CRISPR/Cas9 gene editing to introduce a chimeric Pvcsp gene, containing repeats of both the VK210 and VK247 alleles, into the Pfp47 locus of the P. falciparum NF54 genome. We found that these Pf-PvCSP chimeric P. falciparum parasites produced viable, motile sporozoites expressing both PfCSP and chimeric PvCSP at the sporozoite surface that are infectious to primary human hepatocytes. Immunization of mice with these sporozoites resulted in induction of antibodies against the repeats of both PfCSP and chimeric PvCSP. These results not only show promise for the use of Pf-PvCSP sporozoites in clinical CHMI studies for optimization of vaccines targeting PvCSP, but also suggest that such chimeric sporozoites can be used in whole, attenuated sporozoite vaccination approaches, capable of inducing cross-protective immune responses against both P. falciparum and P. vivax.
Wild type P. falciparum NF54 (WT NF54) parasites (Ponnudurai et al., 1981) were obtained from the Radboud University Medical Center (Nijmegen, The Netherlands). Parasites were cultured in RPMI-1640 culture medium supplemented with L-glutamine and 25mM HEPES (Gibco Life Technologies), 50 mg/L hypoxanthine (Sigma), 0.225% NaHCO3 and 10% human serum at a 5% hematocrit under 4% O2, 3% CO2 and 93% N2 gas-conditions at 75 rpm at 37°C in a semi-automated culture system (Infers HT Multitron and Watson Marlow 520U) as previously described (Mogollon et al., 2016). Fresh human serum and human red blood cells (RBC) were obtained from the Dutch National Blood Bank (Sanquin Amsterdam, the Netherlands; permission granted from donors for the use of blood products for malaria research and microbiology tested for safety). Production of genetically modified parasites and characterization of these parasites throughout their life cycle, including mosquito transmission, was performed under GMO permits IG 17-230_II-k and IG 17-135_III.
Female C57BL/6Jico mice (6–7 weeks; Charles River, NL) were used. All animal experiments were granted a licence by the Competent Authority after an advice on the ethical evaluation by the Animal Experiments Committee Leiden (AVD1160020171625). All experiments were performed in accordance with the Experiments on Animals Act (Wod, 2014), the applicable legislation in the Netherlands and in accordance with the European guidelines (EU directive no. 2010/63/EU) regarding the protection of animals used for scientific purposes. All experiments were performed in a licenced establishment for the use of experimental animals (LUMC). Mice were housed in individually ventilated cages furnished with autoclaved aspen woodchip, fun tunnel, wood chew block and nestlets at 21 ± 2°C under a 12:12 h light-dark cycle at a relative humidity of 55 ± 10%.
Mosquitoes from a colony of Anopheles stephensi (line Nijmegen SDA500) were used. Larval stages were reared in water trays at a temperature of 28 ± 1°C and a relative humidity of 80%. Adult females were transferred to incubators with a temperature of 28 ± 0.2°C and a relative humidity of 80%. For the transmission experiments, 3- to 5-day-old mosquitoes were used.
Chimeric P. falciparum parasites expressing both PfCSP and chimeric PvCSP (PvCSP-chi), were generated using the previously described pLf0019 construct (Mogollon et al., 2016)(Figure S1), containing the cas9 gene, together with the gRNA-donor DNA containing plasmid, pLf0109, targeting Pfp47 which contains the Pvcsp-chi expression cassette. The pLf0019 construct contains a blasticidin (bsd) drug-selectable marker cassette and the pLf0109 gRNA-donor DNA construct contains a hdhfr-yfcu drug-selectable marker cassette for selection with WR99210 and 5-fluorocytocine (5-FC)(Figure S1). To generate pLf0109, the plasmid pLf0047 (Marin-Mogollon et al., 2019), containing two homology regions and a gRNA expression cassette targeting Pfp47 (PF3D7_1346800), was modified by introducing a Pvcsp-chi expression cassette, which was commercially synthesized (Integrated DNA Technologies, Inc.; see Figure S2). This Pvcsp-chi expression cassette contains a codon-optimized Pvcsp open reading frame (ORF) containing the N- and C-terminal regions of the Pvcsp VK210 allele that flank a chimeric repeat sequence comprising repeats of both the VK210 allele (three times the repeat GDRADGQPA/GDRAAGQPA) and the VK247 allele (three times the repeat ANGAGNQPG/ANGAGDQPG). The ORF of Pvcsp-chi is flanked by EcoRV/EcoRI restriction sites and cloned into pLf0040 (cut EcoRV/EcoRI) (Marin-Mogollon et al., 2018) which contains 967bp of the promoter regions of Pfcsp (PF3D7_0304600) and 917bp of 3’UTR of Pfcsp resulting in intermediate plasmid T24 (Figure S1). This plasmid was digested with ApaI/SacII to obtain the complete Pvcsp-chi expression cassette containing the Pfcsp promoter, the Pvcsp-chi ORF with the chimeric VK210/VK247 repeats, and the Pfcsp 3’UTR. This expression cassette was inserted into vector pLf0047 (Figure S1) using the same restriction enzymes (ApaI/SacII) resulting in the final construct pLf0109. Plasmids for transfection were isolated from 250 ml cultures of Escherichia coli XL10-Gold Ultracompetent Cells (Stratagene, NL) by maxi-prep (PureYield™ Plasmid Maxiprep System (Promega, NL) to generate 25–50 µg of DNA used per transfection. Transfection of WT PfNF54 parasites was performed using the ‘spontaneous uptake method’ as previously described (Miyazaki et al., 2020). Briefly uninfected RBC (300 μl of packed RBCs) were transfected with the constructs pLf0019 and pLf0109 (a mixture of 25 μg of each circular plasmid in 200 μl cytomix) using the Gene Pulser Xcell electroporator (BioRad) with a single pulse (310 V, 950 μF). Subsequently, the transfected RBCs were washed with complete RPMI1640 medium and mixed with WT PfNF54 infected RBC (iRBC) with a parasitemia of 0.5% and a hematocrit of 5%. These cells were transferred into a 10 ml culture flask and the cultures were maintained under standard conditions in the semi-automated culture system (Mogollon et al., 2016). Selection of transfected parasites was performed by applying double-positive drug pressure from day 3 after transfection using the drugs WR99210 (2.6 nM) and BSD (5 µg/ml). The positive drug pressure was maintained until parasites were detectable in thin blood-smears (at day 15 after transfection). Subsequently, the parasites were maintained in drug-free medium for 2–4 days until the parasitemia reached over 10%, followed by applying negative selection by addition of 5-FC (1 µM) as described before (Mogollon et al., 2016) in order to eliminate parasites that retained the donor DNA construct as episomal plasmid and to enrich for the transfected parasites containing the donor DNA integrated into the genome. Negative drug pressure in the cultures was maintained until thin blood-smears were parasite-positive (after 7 days). After the negative selection, iRBC were harvested from cultures for genotyping by diagnostic PCR and Southern blot analysis. Subsequently, selected parasites were cloned by limiting dilution as previously described (Marin-Mogollon et al., 2018). Briefly, serial dilutions were performed with uninfected RBC in complete medium (1% hematocrit and 20% serum) and cultured in a total volume of 100 µl incubated in 96-well plates, resulting in 8 rows with the following numbers of iRBC per well: 100, 10, 5, 2.5, 1.25, 0.6, 0.3, 0.15. Plates were incubated in a Candle Jar at 37°C and culture medium was changed every other day. Every 5 days RBC were added resulting in an increase of the hematocrit from 1% to 5%. At days 10 to 11, samples were collected for thick smear analysis from the rows with the highest numbers (100 and 10) of iRBC/well. At day 21 thick smears were made from samples from all rows. Clones were selected from dilutions/row with less than 40% of the wells being parasite-positive. These clones were transferred in 10 ml culture flasks at 5% hematocrit under standard culture conditions in the semi-automated culture system for collection of parasites for further genotype and phenotype analyses (see next section).
Diagnostic PCR and Southern blot analysis of digested DNA were performed from material isolated from iRBC obtained from 10 ml cultures, pelleted by centrifugation (1500 g, 5 min). RBC were then lysed with 5 ml of cold (4°C) erythrocyte lysis buffer (10× stock solution 1.5 M NH4Cl, 0.1 M KHCO3, 0.01 M Na2EDTA; pH 7.4; (Janse et al., 2006)) and parasites were treated with proteinase-K before DNA isolation by standard phenol-chloroform methods. Correct integration of the donor construct was analyzed by long-range PCR (LR-PCR) (primers P1/P3) and standard PCR amplification of the Pvcsp-chi gene (primers P4/P5), the Pfp47 gene (primers P6/P7) and the fragment for 5’ integration (5’int; primers P1/P2). The PCR fragments were amplified using KOD Hot start polymerase following standard conditions with an annealing temperature of 52°C for 15 s, 55°C for 15 s, and 63°C for 25 s and an elongation step of 68°C for 4 min for LR-PCR and with an annealing temperature of 50°C for 10 s, 55°C for 10 s, and 60°C for 10 s and an elongation step of 68°C for 1 min for the other PCRs. Southern blot analysis was performed with genomic DNA digested with HpaI and EcoRI (4 h at 37°C) to confirm integration of the replacement of the Pfp47 gene by the Pvcsp-chi expression cassette. Digested DNA was hybridized with probes targeting the Pfp47 homology region 1 (HR1), amplified from WT PfNF54 genomic DNA by PCR using the primers P8/P9 and a second probe targeting ampicillin (Amp) gene, amplified from plasmid pLf0109 by PCR using the primers P10/P11.
The growth rate of asexual blood stages of the Pf-PvCSP was determined in 10 ml cultures maintained in the semi-automated culture system under standard culture conditions. Briefly, a culture with 0.1% parasitemia was established in complete culture medium at a hematocrit of 5%. Medium was changed twice daily and the culture was maintained for a period of 3 days without refreshing RBC. For determination of the course of parasitemia, triplicate samples of 100 µl were collected daily from all cultures and cells pelleted by centrifugation (9485 g; 30 s) and stained with Giemsa. P. falciparum gametocytes cultures were generated using standard culture conditions as previously described (Marin-Mogollon et al., 2018) with some modifications. Briefly, parasites from asexual stage cultures were diluted to a final parasitemia of 0.5%, and cultures were followed during 14 days without adding new RBC. At day 14, gametocyte development was analyzed by counting male/female gametocytes (stage V) in Giemsa stained blood smears. To assess exflagellation, 20 µl samples of the P. falciparum gametocyte cultures at day 14 were diluted 1:1 with Fetal Calf Serum (FCS) at room temperature and exflagellation centers were examined and quantified 10–20 min after activation in a Bürker cell counter.
For analysis of oocyst and sporozoite production, A. stephensi mosquitoes were fed when cultures contained mature stage V gametocytes and showed more than 80 exflagellating parasites per 105 erythrocytes. Mosquito feeding was performed using the standard membrane feeding assay (SMFA) (Ponnudurai et al., 1989). Oocysts were analyzed between day 10 and 12 and salivary gland sporozoites were counted at day 18 and 21 after feeding. Oocyst were counted in 10–30 midguts, dissected in a droplet of mercurochrome (1%) in distilled water on a glass slide. Midguts were covered with a coverslip and oocyst images were recorded from dissected midgut in PBS 1 X (100× magnification). For counting sporozoites, salivary glands from 30–60 mosquitoes were dissected and homogenized using a grinder in 100 µl of RPMI pH 7.2 and sporozoites were analyzed in a Bürker cell counter using phase-contrast microscopy.
To analyze CSP expression in fixed Pf-PvCSP and WT PfNF54 salivary gland sporozoites by indirect immunofluorescence assay (IFA), samples of purified salivary gland sporozoites (20 µl) were placed on a 8-well black cell-line diagnostic microscope slide (Thermo Scientific, NL), dried for 10 min, and fixed with 4% paraformaldehyde for 30 min at room temperature. After fixation the slides were washed three times with PBS and permeabilized with 20 µl of 0.5% triton in PBS and then blocked with 10% of FCS in PBS for 1h. Fixed cells were washed with PBS and incubated overnight at 4°C with the following monoclonal CSP antibodies: mouse anti-PfCSP (mAb 2A10, MRA-183; 1:200 dilution of 1 mg/ml stock solution); mouse anti-PvCSP VK210 (mAb 2F2; MRA-184; (Salman et al., 2017); 1:200 dilution of 109 µg/ml stock solution) and mouse anti-PvCSP VK247 (mAb 2E10.E9; MRA-185; (Salman et al., 2017); 1:200 dilution of 125 µg/ml stock solution. Subsequently, cells were rinsed three times with PBS and incubated with the secondary antibodies Alexa FLuor®488/594-conjugated chicken anti-mouse (Invitrogen Detection technologies, NL at 1:500 dilution). Finally, the cells were washed again three times with PBS and stained with the DNA-specific dye Hoechst-33342 at a final concentration of 10 µM. Fixed cells were covered with 1–2 drops of an anti-fading agent (Image-iT™ FX, Life technologies), and a coverslip placed on the cells and sealed with nail polish. Stained cells were analyzed for fluorescence using a Leica fluorescence MDR microscope (100× magnification). Pictures were recorded with a DC500 digital camera microscope using Leica LAS X software with the following exposure times: Alexa 488: 1.0 s; Alexa 594: 0.6 s; Hoechst 0.2 s; bright field 0.62 s (1× gain) or with a Leica SP8 upright confocal microscope.
To analyze CSP expression of live Pf-PvCSP and WT PfNF54 salivary gland sporozoites by indirect immunofluorescence assay (IFA), samples of 2 × 105 salivary gland sporozoites were aliquoted in 1.5 ml Eppendorf tubes and were incubated with the corresponding antibody (mouse anti-PfCSP, mouse anti-PvCSP VK210 or mouse anti-PvCSPVK247 (1:100 dilution) in 100 µl of 1% (v/v) FCS in PBS 1×, for 1 h on ice. Subsequently, sporozoites were rinsed with 1.5 ml of 1% (v/v) FCS in PBS and pelleted by centrifugation at 9300 g for 4 min at 4°C. The sporozoite pellet was then resuspended in 100 µl 1% FCS in PBS containing the secondary antibodies; anti–mouse IgG Alexa 488 (1:200) and incubated for 1 h on ice. Sporozoites were rinsed with 1.5 ml of 1% (v/v) FCS in PBS and pelleted by centrifugation at 9300 g for 4 min at 4°C, and the resulting pellet was resuspended in 40 µl of PBS. Of the cell suspension containing sporozoites 20 µl was placed per well of an 8-well black cell-line diagnostic microscope slide (Thermo Scientific), incubated 30 min at room temperature (RT), and fixed with 80 µl of 4% PFA in PBS 1× for 30 min at RT. Finally, the wells were washed twice with PBS and stained with 10 µM Hoechst 33342 in PBS for 15 min at RT. For microscopy analysis, the preparations were covered with 1–2 drops of an anti-fading agent (Image-iT™ FX, Life technologies), and a coverslip was placed onto the cells and sealed with nail polish. Stained cells were analyzed for fluorescence using a Leica fluorescence MDR microscope (100× magnification). Pictures were recorded with a DC500 digital camera microscope using Leica LAS X software with the following exposure times: Alexa 488: 0.7 s; Hoechst 0.136 s; bright field 0.62 s (1× gain).
Sporozoite motility was determined using a sporozoite gliding assay (Stewart and Vanderberg, 1988; van Schaijk et al., 2008). Glass slides were placed in a 24 wells plate (Thermofisher, DK) and coated with an anti-PfCSP monoclonal 3SP2 (courtesy of Dr. M. McCall, Radboudumc, The Netherlands; 5 μg/ml in PBS) using 200 µl per slide, and left overnight at RT. The next day freshly isolated WT PfNF54 and Pf-PvCSP sporozoites, collected in RPMI (Thermofisher, Gibco 42401-018) with 10% FCS (Capricornscientific, FBS-12A CP15-1439) at RT were added to anti-PfCSP coated slides that were washed with PBS. Per slide 30.000 sporozoites were added (in 200 µl RPMI with 10% FCS) and these glass slides were incubated for 2.5 h at 37°C under 5% CO2 conditions. Slides were then washed three times with PBS and fixed with 4% PFA (Thermo Scientific, NL) for 20 min at RT. Then, the slides were washed three times with PBS and blocked with 1% BSA (Roche diagnostic, DE) in PBS for 15 min. After BSA blocking, the slides were washed three times with PBS, and the primary antibody anti-Pf-3SP2 or anti-PvCSP-VK210 Mab (mAb 2F2) (10 µg/ml) was added and incubated for 45 min at RT. Slides were then washed three times with PBS, and incubated with a secondary antibody goat anti-mouse Alexa fluor 488 (Invitrogen Thermofisher, Oregon, A11001) Mab (diluted 500 times in PBS) for 45 min (RT, in the dark). Finally, slides were washed three times with PBS in the dark, air dried until the slides were almost dry and mounted with Coverslips (with Image iT FX signal Enhancer; Invitrogen Thermofisher, USA) and examined with a Leica DMR fluorescent microscope with standard GFP filter for the gliding trails at 63× magnification. The percentage of gliding was calculated in 8–10 fields, measuring the number of gliding trails for the WT PfNF54 sporozoites in comparison with the gliding trails for the Pf-PvCSP sporozoites. The mean and standard deviation were determined for the percentage of gliding trail sporozoites.
In vitro primary human hepatocyte maturation assay was performed as described in (Marin-Mogollon et al., 2019) with few adaptations. Cryopreserved primary human hepatocytes, obtained from Xenothech, were thawed according to the manufacturer and seeded at a density of 60,000 cells/well in a collagen-coated 96-well clear-bottomed black plate for 2 days. Medium was refreshed daily (hepatocyte medium: Williams’s E medium supplemented with 10% heat inactivated fetal bovine serum, 1% penicillin-streptomycin, 1% fungizone, 0.1 lU/ml insulin, 70 µM hydrocortisone). Per well, 7 x 104 freshly dissected Pf-PvCSP and WT PfNF54 sporozoites were added to the hepatocyte monolayer. After a quick spin (10 min at 1900 g), the plate was incubated at 37°C under 5% CO2. The medium was replaced with fresh hepatocyte culture medium 3 h post-invasion, and daily for 4 days thereafter. Then, hepatocytes were fixed with ice-cold methanol for at least 10 min on ice. A standard IFA was performed using an anti-PfHSP70 primary antibody (StressMarq) with an Alexa fluor 594 (Invitrogen) secondary antibody and DAPI for nuclear staining. High content imaging was performed, using the ImageXpress Pico system (Molecular Devices). Number of parasites, number of hepatocytes and size of parasites were determined using the CRX software (Molecular Devices) and data analyzed using the GraphPad software.
For generation of sporozoite-extracts for immunization, salivary gland sporozoites were isolated as described in the previous sections. Aliquots of 105 sporozoites in 50 µl PBS were made and stored at −80°C. After two rounds of freezing and thawing, 105 sporozoites in 200 µl PBS were used to immunize mice by intravenous injection. Groups of six C57BL/6 mice were immunized three times with sporozoites at a 7-day interval, and blood was collected in heparinized capillaries from all mice by orbital vein puncture 1 day prior to the first and third immunization and one week after the last immunization. Plasma was collected after centrifugation of the blood samples (1500 g for 8 min at 4°C) and stored at −20°C until further analysis.
For assessment of anti-CSP antibody titers (total IgG), enzyme-linked immunosorbent assays (ELISAs) were performed. High protein-binding capacity 96-well ELISA plates (Nunc MaxiSorp™ flat-bottom) were coated with synthetic peptides (Sigma) based on the repeat sequences of PfCSP and PvCSP. Specifically, plates were coated with peptides with the amino acid sequence (NANP)4NVDPC for PfCSP, the amino sequence GD RAD GQP AGD RAA GQP A for the PvCSP VK210 and the amino sequence ANGAGNQPG ANGAGDQPG for the PvCSP VK247. The plates were coated overnight at 4°C with a peptide concentration of 5 µg/ml in PBS with a volume of 50 µl per well. Plates were washed three times with 0.05% Tween20/BS (PBST) and blocked with 200 µl of PBST with 5% milk for 30 min at RT. Plates were then washed one additional time and plasma samples were diluted 1:50 in 1% milk PBST and a 3-point 1:3 dilution was carried out for each sample. After 3h incubation at RT, plates were washed three times with PBST and incubated for 1h at RT with the secondary antibody, horseradish peroxidase-labelled goat anti-mouse IgG (GE Healthcare UK) at a dilution of 1:2000. After six PBST washes, the reaction was developed by adding BD OptEIA™ TMB Substrate Reagent and incubating for 1 to 5 min at RT, before stopping the reaction by adding 50 µl Stop solution (2N H2SO4). Absorbance was immediately measured at 450 nm using a Tecan Infinite M200 plate reader. Data were analyzed in relation to a standard titration curve of at least 8 points starting a dilution of 1.5u/ml of the corresponding mouse monoclonal antibody: mouse anti-PfCSP (mAb 2A10, MRA-183); mouse anti-PvCSP VK210 (mAb 2F2; MRA-184) and mouse anti-PvCSP VK247 (mAb 2E10.E9; MRA-185). All monoclonal antibodies were produced from hybridoma cell lines obtained from BEI Resources, NIAID, and kindly provided by Elizabeth Nardin.
Data were analyzed using GraphPad Prism software version 8.1.1 (GraphPad Software, Inc). Significance difference analyses between WT PfNF54 and Pf-PvCSP lines were performed using the unpaired Student’s t-test. The value of p < 0.05 was considered statistically significant (* p < 0.01, ** p < 0.001, *** p < 0.0001).
We have previously shown that chimeric P. falciparum parasites with the Pfcsp gene replaced by the two major Pvcsp alleles, VK210 and VK247, do not produce salivary gland sporozoites and most oocysts degenerate before formation of sporozoites (Marin-Mogollon et al., 2018). It has been shown that viable and infectious rodent P. berghei sporozoites can be engineered to express CSP proteins from two different Plasmodium species. These chimeric sporozoites were generated by introducing a P. falciparum csp gene as an additional copy into the P. berghei genome (Mendes et al., 2018a; Mendes et al., 2018b; Reuling et al., 2020). Based on this observation we aimed at generating chimeric P. falciparum sporozoites expressing both PfCSP and an chimeric PvCSP protein (PvCSP-chi). Using CRISPR/Cas9 gene editing, a chimeric P. falciparum line (Pf-PvCSP) was created that contains the Pvcsp-chi gene under the control of 967 bp of the Pfcsp gene promoter region. This expression cassette was introduced into the neutral Pfp47 locus (PF3D7_1346800) of the genome of P. falciparum NF54 wild type (WT) parasites. The Pfp47 locus was selected as it is suitable for introducing transgenes without compromising parasite development in the blood and liver stages or in A. stephensi mosquitoes (Talman et al., 2010; Vaughan et al., 2012; Vos et al., 2015; Marin-Mogollon et al., 2019; Miyazaki et al., 2020). The WT PfNF54 parasites were transfected with two plasmids: a previously described construct containing the Cas9 expression cassette with the blasticidin (bsd) drug-selectable marker cassette (pLf0019) and a donor DNA construct (pLf0109) designed to target the Pfp47 locus. The donor construct contains the Pvcsp-chi expression cassette flanked by two homology regions of Pfp47, the Pfp47 sgRNA sequence and a hdhfr-yfcu drug-selectable marker cassette (see Figures 1A, S1, S2 and Materials and Methods section for details of the generation of the constructs). This Pvcsp-chi gene contains the N- and C-terminal regions of the Pvcsp VK210 allele that flank a chimeric repeat sequence comprising repeats of both the VK210 allele (three times the repeat GDRADGQPA/GDRAAGQPA) and the VK247 allele (three times the repeat ANGAGNQPG/ANGAGDQPG). Transfection of WT PfNF54 parasites was performed using the ‘spontaneous uptake method’. Briefly, uninfected RBC were first transfected with the constructs pLf0019 and pLf0109. Subsequently, the transfected RBCs were mixed with WT PfNF54 infected RBC (iRBC). Selection of transfected parasites containing both plasmids was performed by applying double-positive selection with the drugs Blasticidin and WR99210 until parasites were detectable by thin blood-smear analysis. Subsequently negative drug selection with 5-fluorocytosine was applied to enrich the parasites in which the Pvcsp-chi gene expression cassette was integrated into the genome by double cross-over homologous recombination. Next drug-selected parasites were cloned by limiting dilution. Diagnostic (long range) PCR and Southern analyses of three clones (Pf-PvCSP cl1, cl3, and cl4) confirmed correct double crossover integration of the Pvcsp-chi gene expression into the Pfp47 locus in the genome of all Pf-PvCSP clones (Figures 1B, C). Two Pf-PvCSP clones (cls 1, 3) were selected for further phenotype characterization.
Figure 1 Generation and genotyping of the chimeric Pf-PvCSP parasites. A schematic representation of the Cas9 (plasmid Leiden falciparum (pLf) 0019) and donor DNA plasmids (pLf0109) constructs used to introduce the Pvcsp chimeric (Pvcsp-chi) expression cassette into the PfNF54 p47 gene locus. The Pvcsp-chi gene contains a chimeric repeat region of VK210 and VK247 alleles and is under the control of the promoter of the Pfcsp gene. The p47 homology regions (HR1, HR2) used to introduce the donor DNA, location of primers (P), sizes of restriction fragments (HpaI and EcoRI; in red), and PCR amplicons (in black) are indicated. WT, wild type; bsd, blasticidin selectable marker (bsd); hdhfr::yfcu, in donor plasmid. (B) Diagnostic PCRs confirming the correct integration of the Pvcsp-chi expression cassette into the Pfp47 locus. Diagnostic PCR: part of Pvcsp-chi open reading frame (primers P4/P5); part of Pfp47 open reading frame (primers P6/P7); 5’-integration of the plasmid into the Pf-PvCSP-chi genome (5’-Int; primers P1/P2); LR-PCR (primers P1/P3). (C) Southern analysis of HpaI and EcoRI restricted DNA of WT, and chimeric Pf-PvCSP parasites confirms the specific integration of the Pvcsp genes into the Pfp47 gene locus. (D) Growth of asexual blood-stages of Pf-PvCSP cl3 and WT PfNF54. Parasitemia (mean and S.D of 3 independent cultures) is shown during a 3-day culture period (in the semi-automated culture system). Cultures were initiated with a parasitemia of 0.1%.
The in vitro growth of Pf-PvCSP (cl3) blood stages was comparable to that of the parental WT PfNF54 blood stage parasites (Figure 1D). Pf-PvCSP parasites produced numbers of mature gametocytes in standardized gametocyte cultures similar to those of the parental line (Table 1; Figure S3) and male gametocytes underwent exflagellation upon activation. Using SMFA, A. stephensi mosquitoes were infected with gametocytes of either WT PfNF54 or Pf-PvCSP parasites. The number of oocysts in mosquito midguts was determined at days 10 to 12 after infection and the number of sporozoites in salivary glands was analyzed at days 18 to 24 after infection. These analyses revealed that Pf-PvCSP parasites produced comparable number of oocysts and salivary gland sporozoites to those of the parental parasites (Table 1; Figures S3 and S4). Approximately 90% of Pf-PvCSP oocysts already contained sporozoites at days 10 to 12 which is in contrast to the two previously created chimeric P. falciparum lines with the Pfcsp gene replaced by the Pvcsp alleles vk210 and vk247. Most of the oocysts of these replacement lines degenerated before the formation of sporozoites (Marin-Mogollon et al., 2018). The presence of Pf-PvCSP sporozoites in salivary glands (Table 1) indicated that PfCSP retains its normal function in the formation of sporozoites, and that expression of PvCSP-chi in these sporozoites does not appear to interfere with sporozoite formation and invasion of salivary glands.
To analyze the expression of PvCSP-chi and PfCSP in salivary gland sporozoites of the Pf-PvCSP line, we first performed an immunofluorescence on fixed/permeabilized sporozoites with antibodies specific for the repeat sequences of either PvCSP VK210, PvCSP VK247 or PfCSP. All Pf-PvCSP sporozoites analyzed (n=2 exp.) reacted with all three antibodies whereas WT PfNF54 sporozoites reacted only with anti-PfCSP antibodies (Figure 2), indicating that all Pf-PvCSP sporozoites expressed both the PfCSP and PvCSP-chi proteins.
Figure 2 Immunofluorescence analyses of PvCSP and PfCSP expression in fixed Pf-PvCSP salivary gland sporozoites. Fixed permeabilized sporozoites of Pf-PvCSP (clone 1 and clone 3) and WT PfNF54 parasites stained with mouse anti-PvCSPVK210, anti-PvCSP VK247 (green; alexa 488) and anti-PfCSP antibodies (red; alexa 594). Nuclei stained with the Hoechst-33342. Control negative, corresponds to the incubation without the primary antibody (2 experiments). Scale bar is 5 µm.
We next investigated whether PvCSP-chi protein is expressed at the surface of sporozoites, by performing IFA of live salivary gland sporozoites. Similarly to the results of IFA using fixed/permeabilized sporozoites, live Pf-PvCSP sporozoites reacted with anti-PvCSP VK210, anti-PvCSP VK247 and anti-PfCSP antibodies whereas WT PfNF54 sporozoites reacted only to anti-PfCSP antibodies (Figure 3A). This result demonstrates that the PvCSP-chi protein is expressed at the surface of Pf-PvCSP sporozoites.
Figure 3 Immunofluorescence analyses of PvCSP and PfCSP expression of live Pf-PvCSP salivary gland sporozoites. (A) Live sporozoites of Pf-PvCSP and WT PfNF54 parasites labelled with mouse anti-PvCSPVK210, anti-PvCSP VK247 and anti-PfCSP antibodies (green; alexa 488). Nuclei stained with the Hoechst-33342. Control negative, corresponds to the incubation without the primary antibody (2 experiments). Scale bar is 5 µm. (B) Typical sporozoite gliding trails of Pf-PvCSP and WT PfNF54 sporozoites stained with mouse anti-PvCSPVK210 and anti-PfCSP antibodies (left) and percentage of gliding trials (right) using primary antibody anti-Pf-3SP2 and anti-PvCSPVK210 (1 experiment). Scale bar is 15 µm. ns, not significant, as determined by One-way ANOVA test (GraphPad).
As CSP is shed during parasite gliding motility, we next assessed if PvCSP is also shed similarly to PfCSP by Pf-PvCSP sporozoites. CSP-trails in a sporozoite gliding assay also reacted with both anti-PfCSP and anti PvCSP VK210 antibodies (Figure 3B), confirming surface expression of PvCSP-chi and revealing shedding of PvCSP-chi during gliding. Comparable gliding trails were observed in WT PfNF54 and Pf-PvCSP sporozoites using anti-PfCSP antibody (Figure 3B), with a percentage of 85% gliding trails in WT PfNF54 sporozoites (with a range of 28–65 gliding trails per field, Stdev. 2.6) and 87.0% gliding trails in Pf-PvCSP sporozoites (with a range of 23–43 gliding trails per field, Stdev 7,6.).
We next assessed the in vitro infectivity of Pf-PvCSP sporozoites to primary human hepatocytes. At day 4 post infection of primary human hepatocytes with either WT or Pf-PvCSP sporozoites, the cells were fixed, and liver stages were stained with an anti-PfHSP70 antibody. Pf-PvCSP sporozoites showed comparable efficiency of hepatocyte infection to WT PfNF54 sporozoites (Figure 4). Pf-PvCSP and WT PfNF54 also displayed similar liver stage sizes at day 4 post infection (Figure 4). These results demonstrate that expression of PvCSP-chi at the surface of Pf-PvCSP sporozoites did not reduce parasite in vitro invasion and development in primary human hepatocytes.
Figure 4 In vitro infection of primary human hepatocytes by Pf-PvCSP sporozoites. (A) Representative images of liver stage development in primary human hepatocytes 4 days after infection with Pf-PvCSP and WT PfNF54 sporozoites. Liver stages were stained with anti-PfHSP70 antibodies and nuclei were stained with DAPI (1 experiment). Scale bar is 10 µm. (B) Percentage of infected hepatocytes (left) and size of liver stages (right) at day 4 after infection of hepatocytes by Pf-PvCSP and WT NF54 sporozoites. The size was measured by determining the area of the parasite cytoplasm using the red-positive (anti-HSP70) area (µm2). n.s., not significant, as determined by unpaired t-test (GraphPad).
We then analyzed the capacity of the Pf-PvCSP sporozoites to elicit the production of antibodies against the repeat sequences of PfCSP and PvCSP-chi by immunizing mice with killed Pf-PvCSP and WT PfNF54 sporozoites. Groups of six C57BL/6 mice were intravenously injected with 105 freeze-thaw killed sporozoites, three times with a 7-day interval, and blood was collected from all mice 1 day prior to the first and third immunization and 1 week after the last immunization (Figure 5A). Plasma of these mice was tested for antigen-specific antibody production by ELISA using plates that were coated with the following repeat sequences; (NANP)4NVDPC for PfCSP; GD RAD GQP AGD RAA GQP A for PvCSP VK210; and ANGAGNQPG ANGAGDQPG for PvCSP VK247 strain.
Figure 5 Antibody production against repeat sequences of PfCSP and PvCSP-chi in mice after immunization with Pf-PvCSP and WT PfNF54 sporozoites. (A) Schematic representation of the immunization protocol. Groups of six C57BL/6 mice were immunized three times with killed sporozoites with a 7-day interval and blood was collected from all mice by orbital vein puncture 1 day before the first and third immunization and one week after the last immunization (one experiment). (B) Total IgG titers against PfCSP and PvCSP repeat sequences in plasma prior, during and after immunization. Enzyme-linked immunosorbent assays were performed with plasma samples collected from mice as shown in (A) to assess total IgG titers specific for the repeat sequences of PfCSP ((NANP)4NVDPC) and two variants of PvCSP(GD RAD GQP AGD RAA GQP A for PvCSP VK210; ANGAGNQPG ANGAGDQPG for the PvCSP VK247. Mean and standard error of the mean and *p < 0.05; **p < 0.01; ***p < 0.001, as determined by unpaired t-test analysis.
Plasma samples of mice immunized with either WT PfNF54 or Pf-PvCSP sporozoites reacted strongly to the PfCSP repeat sequences after the second and third immunization with no significant differences in total IgG between the two groups of mice (Figure 5B), indicating comparable immunogenicity of the PfCSP repeat sequences in WT PfNF54 or Pf-PvCSP sporozoites. In contrast, only mice immunized with Pf-PvCSP sporozoites showed significantly increased antibody titers specific for the repeat sequences of PvCSP VK210 and PvCSP VK247 whereas no significant increase was observed in WT PfNF54-immunized mice (Figure 5B). Although the total IgG against the PvCSP repeats was lower than that against the PfCSP repeats, these results show that exposure to Pf-PvCSP sporozoites can induce the production of antibodies against PfCSP, as well as against both PvCSP repeat variants.
In this study we describe a chimeric P. falciparum line, Pf-PvCSP, which expresses CSP from both P. falciparum and P. vivax. We demonstrate that chimeric parasites produce sporozoites in similar numbers to those of the parental WT PfNF54 line and that both CSPs expressed in the chimeric parasites are present at the sporozoite surface and shed during gliding. Our results show that Pf-PvCSP sporozoites are infectious to primary human hepatocytes. These observations support the notion that the presence of the chimeric PvCSP protein at the surface of P. falciparum sporozoites does not affect sporozoite viability and infectivity. The formation of infective sporozoites expressing CSP of two different Plasmodium species has been previously demonstrated in studies using P. berghei expressing PfCSP (Mendes et al., 2018b). However, the viability and infectivity of these chimeric Pb-PfCSP sporozoites is expected, since PfCSP can fully complement the multiple functions of PbCSP as shown in replacement P. berghei lines with the endogenous PbCSP replaced by PfCSP (Triller et al., 2017; Flores-Garcia et al., 2019). This is distinct from PvCSP, since previous attempts to functionally complement PfCSP, by replacing the Pfcsp gene with two Pvcsp alleles, were unsuccessful (Marin-Mogollon et al., 2018). It is known that CSP fulfills multiple functions, both in the process of sporozoite formation and in later processes of sporozoite invasion of salivary glands, migration through the human skin and invasion of hepatocytes (Sinnis and Coppi, 2007; Vaughan et al., 2008; Ejigiri and Sinnis, 2009; Coppi et al., 2011; Vaughan and Kappe, 2017).
Although the previously generated gene-replacement Pf-PvCSP parasites were able to produce normal numbers of oocysts, no salivary gland sporozoite were formed and most oocyst degenerated before sporozoite formation, a phenotype that is comparable to P. falciparum mutants lacking the Pfcsp gene (Marin-Mogollon et al., 2018). Collectively, these observations indicate that PvCSP is unable to fully complement the role of PfCSP in the process of sporozoite formation inside oocysts but that the simultaneous expression of PvCSP with PfCSP in sporozoites of chimeric parasites does not hamper the formation of infectious sporozoites. The lack of full complementation of PfCSP by PvCSP in the process of sporozoite formation may result from the inability of PvCSP to interact with other P. falciparum proteins that are involved in (the initial steps of) sporozoite formation.
Our studies of immunization of mice with the chimeric Pf-PvCSP sporozoites shows that antibodies are induced against the repeat sequences of both PfCSP and the PvCSP-chi protein, including antibody responses against both PvCSP alleles, VK210 and VK247. Interestingly, although IgG responses against PfCSP were significantly higher than against PvCSP, anti- PfCSP titers were comparable for mice immunized with either WT PfNF54 sporozoites or Pf-PvCSP sporozoites. These observations indicate that the presence of PvCSP does not affect the immunogenicity of PfCSP with respect to IgG responses against the NANP/NVDP repeats. The lower IgG responses against the two PvCSP repeats compared to the PfCSP NANP/NVDP repeats can be caused by different factors. The PvCSP-chi protein contains only 3 copies of the VK247 and VK210 repeats, while the P. falciparum NF54 CSP protein contains 38 NANP and 4 NVDP repeats (Bowman et al., 1999; Oyen et al., 2018). In addition, although we provide evidence of the presence of PvCSP at the surface of the Pf-PvCSP sporozoites and in gliding trails, the total amount of PfCSP and PvCSP in the Pf-PvCSP sporozoites is unknown and may differ for the two proteins. The lower IgG responses might also result from a lower intrinsic immunogenicity of the PvCSP repeats compared to the P. falciparum NANP/NVDP repeats. The repeat regions of CSP of the P. vivax strains VK210 and VK247 consist of 10–11 copies of the repeats GDRA(A/D)GQPA or ANGA (G/D)(N/D)QPG, respectively (de Souza-Neiras et al., 2007)] and are not cross reactive (Hall et al., 2019). Of note, our study employed freeze-thaw killed sporozoites to immunize mice; it is therefore possible that antibody responses against PfCSP and PvCSP-chi proteins might be different when immunization is performed with live sporozoites.
Despite the differences in the repeat regions of P. falciparum and P. vivax CSP, both these proteins have been shown to be immunogenic and antibody levels against these repeats appear to be associated with protective immunity in animal malaria models and in humans (Porter et al., 2013; Foquet et al., 2014; Schwenk et al., 2014; Yadava et al., 2014; White et al., 2015; Gimenez et al., 2017; Atcheson et al., 2018; de Camargo et al., 2018; Dobaño et al., 2019). However, due to differences in methodologies for inducing and analyzing antibody production after immunization with full-length CSP proteins or the respective repeat sequences, the relative immunogenicity of the PfCSP and PvCSP repeats in both animal models and in humans is largely unknown. To our knowledge, no studies exist that have compared the immunogenicity of different CSP repeat sequences of the two human Plasmodium species using similar immunization approaches and delivery systems. In comparative P. vivax and P. falciparum CHMI studies, it has been found that the CSP of both species induced cross-reactive antibodies (Hall et al., 2019). However, it is unknown whether the observed cross-reactivity resulted from the repeat sequences or from other epitopes in the N- or C-terminal region of the CSP molecules. In future studies it will be relevant to analyze infections in the FRG huHep mouse model (repopulated with human RBCs) to show that Pf-PvCSP can form infectious liver merozoites. This would enable the use of this model for testing the efficacy of anti-PvCSP antibodies.
Combined, our observations regarding the expression and immunogenicity of the PvCSP on the surface of P. falciparum sporozoites hold promise for the use of the chimeric Pf-PvCSP sporozoites for clinical evaluation of vaccines targeting PvCSP in clinical CHMI studies. The use of such parasites would bypass the need for P. vivax sporozoite production and measures for hypnozoite removal (Payne et al., 2017). In addition, such chimeric sporozoites might also be used in whole, attenuated sporozoite vaccination approaches (Bijker et al., 2015; Goswami et al., 2020). Vaccination with chimeric, attenuated sporozoites might induce cross-protective immune responses against both P. falciparum and P. vivax.
This paper is dedicated to the memory of our friend and colleague, SMK, who initiated the studies described in this paper and recently passed away.
The raw data supporting the conclusions of this article will be made available by the authors, without undue reservation.
The animal study was reviewed and approved by Animal Experiments Committee Leiden (AVD1160020171625). All experiments were performed in accordance with the Experiments on Animals Act (Wod, 2014), the applicable legislation in the Netherlands and in accordance with the European guidelines (EU directive no. 2010/63/EU) regarding the protection of animals used for scientific purposes.
CM-M, BF-F, CJ, and SMK came up with the study concept and design. YM, CM-M, TI, AM, RL, AS, FG, SM, SC-M, JR, SK, HK, RS, AR-S, MP, CJ, and BF-F acquired the data. YM, CM-M, AM, MP, CJ, and BF-F conducted analysis and interpretation of the data. YM, CM-M, BF-F, and CJ wrote the draft of the manuscript. YM, CM-M, SM, AM, MP, RS, CJ, and BF-F critically revised the manuscript for important intellectual content. AM, KD, BW, and RS provided technical and/or material support. BF-F and CJ supervised the study. All authors reviewed the manuscript. All authors contributed to the article and approved the submitted version.
CM-M was, in part, supported by Colciencias Ph.D. fellowship (Call 568 from 2012 Resolution 01218 Bogotá, Colombia). TI was, in part, supported by Uehara Memorial Foundation grant. Work performed at IMM was supported by Fundação para a Ciência e Tecnologia (FCT-Portugal)’s grants PTDC/BBB-BMD/2695/2014 and PTDC-SAU-INF-29550-2017. AR-S is supported by the MRC-DPFS grant MR/N019008/1.
KD holds stock in TropIQ Health Sciences. RL, AS and KD were employed by TropIQ Health Sciences.
The remaining authors declare that the research was conducted in the absence of any commercial or financial relationships that could be construed as a potential conflict of interest.
We would like to acknowledge Els Baalbergen for the mosquito breeding.
The Supplementary Material for this article can be found online at: https://www.frontiersin.org/articles/10.3389/fcimb.2020.591046/full#supplementary-material
Supplementary Figure 1 | Plasmid maps of plasmids used in this study.
Supplementary Figure 2 | Plasmid map of pLf0109 and sequence of the commercially synthesized Pvcsp-chi gene. This gene that contains a codon-optimized Pvcsp open reading frame containing the N- and C-terminal regions of the Pvcsp VK210 allele that flank a chimeric repeat sequence comprising repeats of both the VK210 allele (three times the repeat GDRADGQPA/GDRAAGQPA) and the VK247 allele (three times the repeat ANGAGNQPG/ANGAGDQPG).
Supplementary Figure 3 | Percentage of male exflagellating gametocytes and number of oocyst and sporozoites of Pf-PvCSP cl3 and WT PfNF54 parasites. (A) Percentage of stage V exflagellating male gametocytes in day 14 gametocyte cultures (3 experiments). Each dot correspond to an independent experiment. (B) Number of oocyst per mosquito at day 10 after feeding (n=3). Each dot correspond to the number of oocysts per midgut. (C) Number of sporozoites per mosquito at day 18–24 after feeding (n=3). Each dot corresponds to the mean number of sporozoites per mosquito. Mean and standard error of the mean. n.s., not significant (unpaired t-test; Graphpad).
Supplementary Figure 4 | Sporozoite formation in oocysts of Pf-PvCSP and WT PfNF54 parasites. Representative images of oocyst during development (A) and sporozoite formation (B) in oocysts (day 10) from WT NF54 parasites and Pf-PvCSP parasites that express both PfCSP and PvCSP-chi. (A) Complete midgut and magnified area (×5). Scale bar: 200 µm (upper row) and 20 µm (lower row). (B) Sporozoite formation in an unstained oocyst. Scale bar: 20 µm.
Supplementary Table 1 | Primers used in this study.
Agnandji S. T., Lell B., Fernandes J. F., Abossolo B. P., Methogo B. G., Kabwende A. L., et al. (2012). A phase 3 trial of RTS,S/AS01 malaria vaccine in African infants. N. Engl. J. Med. 367, 2284–2295. doi: 10.1056/NEJMoa1208394
Agnandji S. T., Fernandes J. F., Bache E. B., Ramharter M. (2015). Clinical development of RTS,S/AS malaria vaccine: a systematic review of clinical Phase I-III trials. Future Microbiol. 10, 1553–1578. doi: 10.2217/fmb.15.90
Arévalo-Herrera M., Vásquez-Jiménez J. M., Lopez-Perez M., Vallejo A. F., Amado-Garavito A. B., Céspedes N., et al. (2016). Protective Efficacy of Plasmodium vivax Radiation-Attenuated Sporozoites in Colombian Volunteers: A Randomized Controlled Trial. PLoS Negl. Trop. Dis. 10, e0005070. doi: 10.1371/journal.pntd.0005070
Atcheson E., Bauza K., Salman A. M., Alves E., Blight J., Viveros-Sandoval M. E., et al. (2018). Tailoring a Plasmodium vivax Vaccine To Enhance Efficacy through a Combination of a CSP Virus-Like Particle and TRAP Viral Vectors. Infect. Immun. 86, 1–16. doi: 10.1128/IAI.00114-18
Bennett J. W., Yadava A., Tosh D., Sattabongkot J., Komisar J., Ware L. A., et al. (2016). Phase 1/2a Trial of Plasmodium vivax Malaria Vaccine Candidate VMP001/AS01B in Malaria-Naive Adults: Safety, Immunogenicity, and Efficacy. PLoS Negl. Trop. Dis. 10, e0004423. doi: 10.1371/journal.pntd.0004423
Bijker E. M., Borrmann S., Kappe S. H., Mordmuller B., Sack B. K., Khan S. M. (2015). Novel approaches to whole sporozoite vaccination against malaria. Vaccine 33, 7462–7468. doi: 10.1016/j.vaccine.2015.09.095
Bijker E. M., Sauerwein R. W., Bijker W. E. (2016). Controlled human malaria infection trials: How tandems of trust and control construct scientific knowledge. Soc. Stud. Sci. 46, 56–86. doi: 10.1177/0306312715619784
Bowman S., Lawson D., Basham D., Brown D., Chillingworth T., Churcher C. M., et al. (1999). The complete nucleotide sequence of chromosome 3 of Plasmodium falciparum. Nature 400, 532–538. doi: 10.1038/22964
Clemens J., Moorthy V. (2016). Implementation of RTS,S/AS01 Malaria Vaccine–The Need for Further Evidence. N. Engl. J. Med. 374, 2596–2597. doi: 10.1056/NEJMe1606007
Cohen J., Nussenzweig V., Nussenzweig R., Vekemans J., Leach A. (2010). From the circumsporozoite protein to the RTS, S/AS candidate vaccine. Hum. Vaccin. 6, 90–96. doi: 10.4161/hv.6.1.9677
Coppi A., Natarajan R., Pradel G., Bennett B. L., James E. R., Roggero M. A., et al. (2011). The malaria circumsporozoite protein has two functional domains, each with distinct roles as sporozoites journey from mosquito to mammalian host. J. Exp. Med. 208, 341–356. doi: 10.1084/jem.20101488
de Camargo T. M., de Freitas E. O., Gimenez A. M., Lima L. C., De Almeida Caramico K., Françoso K. S., et al. (2018). Prime-boost vaccination with recombinant protein and adenovirus-vector expressing Plasmodium vivax circumsporozoite protein (CSP) partially protects mice against Pb/Pv sporozoite challenge. Sci. Rep. 8, 1118. doi: 10.1038/s41598-017-19063-6
de Souza-Neiras W. C., De Melo L. M., Machado R. L. (2007). The genetic diversity of Plasmodium vivax–a review. Mem. Inst. Oswaldo Cruz 102, 245–254. doi: 10.1590/S0074-02762007000300002
Dobaño C., Sanz H., Sorgho H., Dosoo D., Mpina M., Ubillos I., et al. (2019). Concentration and avidity of antibodies to different circumsporozoite epitopes correlate with RTS,S/AS01E malaria vaccine efficacy. Nat. Commun. 10, 2174. doi: 10.1038/s41467-019-10195-z
Ejigiri I., Sinnis P. (2009). Plasmodium sporozoite-host interactions from the dermis to the hepatocyte. Curr. Opin. Microbiol. 12, 401–407. doi: 10.1016/j.mib.2009.06.006
Espinosa D. A., Yadava A., Angov E., Maurizio P. L., Ockenhouse C. F., Zavala F. (2013). Development of a chimeric Plasmodium berghei strain expressing the repeat region of the P. vivax circumsporozoite protein for in vivo evaluation of vaccine efficacy. Infect. Immun. 81, 2882–2887. doi: 10.1128/IAI.00461-13
Flores-Garcia Y., Herrera S. M., Jhun H., Pérez-Ramos D. W., King C. R., Locke E., et al. (2019). Optimization of an in vivo model to study immunity to Plasmodium falciparum pre-erythrocytic stages. Malar. J. 18, 426. doi: 10.1186/s12936-019-3055-9
Foquet L., Hermsen C. C., Van Gemert G. J., Van Braeckel E., Weening K. E., Sauerwein R., et al. (2014). Vaccine-induced monoclonal antibodies targeting circumsporozoite protein prevent Plasmodium falciparum infection. J. Clin. Invest. 124, 140–144. doi: 10.1172/JCI70349
Gimenez A. M., Lima L. C., Françoso K. S., Denapoli P. M. A., Panatieri R., Bargieri D. Y., et al. (2017). Vaccine Containing the Three Allelic Variants of the Plasmodium vivax Circumsporozoite Antigen Induces Protection in Mice after Challenge with a Transgenic Rodent Malaria Parasite. Front. Immunol. 8, 1275. doi: 10.3389/fimmu.2017.01275
Goswami D., Betz W., Locham N. K., Parthiban C., Brager C., Schäfer C., et al. (2020). A replication-competent late liver stage-attenuated human malaria parasite. JCI Insight 5 (13), 1–19 doi: 10.1172/jci.insight.135589
Hall C. E., Hagan L. M., Bergmann-Leitner E., Tosh D. M., Bennett J. W., Regules J. A., et al. (2019). Mosquito Bite-Induced Controlled Human Malaria Infection with Plasmodium vivax or P. falciparum Generates Immune Responses to Homologous and Heterologous Preerythrocytic and Erythrocytic Antigens. Infect. Immun. 87, 1–15. doi: 10.1128/IAI.00541-18
Healer J., Cowman A. F., Kaslow D. C., Birkett A. J. (2017). Vaccines to Accelerate Malaria Elimination and Eventual Eradication. Cold Spring Harb. Perspect. Med. 7, 1–17. doi: 10.1101/cshperspect.a025627
Hoffman S. L., Vekemans J., Richie T. L., Duffy P. E. (2015). The March Toward Malaria Vaccines. Am. J. Prev. Med. 49, S319–S333. doi: 10.1016/j.amepre.2015.09.011
Janse C. J., Franke-Fayard B., Mair G. R., Ramesar J., Thiel C., Engelmann S., et al. (2006). High efficiency transfection of Plasmodium berghei facilitates novel selection procedures. Mol. Biochem. Parasitol. 145, 60–70. doi: 10.1016/j.molbiopara.2005.09.007
Kester K. E., Cummings J. F., Ofori-Anyinam O., Ockenhouse C. F., Krzych U., Moris P., et al. (2009). Randomized, double-blind, phase 2a trial of falciparum malaria vaccines RTS,S/AS01B and RTS,S/AS02A in malaria-naive adults: safety, efficacy, and immunologic associates of protection. J. Infect. Dis. 200, 337–346. doi: 10.1086/600120
Long C. A., Zavala F. (2016). Malaria vaccines and human immune responses. Curr. Opin. Microbiol. 32, 96–102. doi: 10.1016/j.mib.2016.04.006
Mahmoudi S., Keshavarz H. (2017). Efficacy of phase 3 trial of RTS, S/AS01 malaria vaccine: The need for an alternative development plan. Hum. Vaccin. Immunother. 13, 2098–2101. doi: 10.1080/21645515.2017.1295906
Marin-Mogollon C., Van Pul F. J. A., Miyazaki S., Imai T., Ramesar J., Salman A. M., et al. (2018). Chimeric Plasmodium falciparum parasites expressing Plasmodium vivax circumsporozoite protein fail to produce salivary gland sporozoites. Malar. J. 17, 288. doi: 10.1186/s12936-018-2431-1
Marin-Mogollon C., Salman A. M., Koolen K. M. J., Bolscher J. M., Van Pul F. J. A., Miyazaki S., et al. (2019). A P. falciparum NF54 Reporter Line Expressing mCherry-Luciferase in Gametocytes, Sporozoites, and Liver-Stages. Front. Cell Infect. Microbiol. 9, 96. doi: 10.3389/fcimb.2019.00096
Marques R. F., Gimenez A. M., Aliprandini E., Novais J. T., Cury D. P., Watanabe I. S., et al. (2020). Protective Malaria Vaccine in Mice Based on the Plasmodium vivax Circumsporozoite Protein Fused with the Mumps Nucleocapsid Protein. Vaccines (Basel) 8, 1–19. doi: 10.3390/vaccines8020190
Ménard R. (2000). The journey of the malaria sporozoite through its hosts: two parasite proteins lead the way. Microbes Infect. 2, 633–642. doi: 10.1016/S1286-4579(00)00362-2
Mendes A. M., Machado M., Goncalves-Rosa N., Reuling I. J., Foquet L., Marques C., et al. (2018a). A Plasmodium berghei sporozoite-based vaccination platform against human malaria. NPJ Vaccines 3, 33. doi: 10.1038/s41541-018-0068-2
Mendes A. M., Reuling I. J., Andrade C. M., Otto T. D., Machado M., Teixeira F., et al. (2018b). Pre-clinical evaluation of a P. berghei-based whole-sporozoite malaria vaccine candidate. NPJ Vaccines 3, 54. doi: 10.1038/s41541-018-0091-3
Miyazaki S., Yang A. S. P., Geurten F. J. A., Marin-Mogollon C., Miyazaki Y., Imai T., et al. (2020). Generation of Novel Plasmodium falciparum NF135 and NF54 Lines Expressing Fluorescent Reporter Proteins Under the Control of Strong and Constitutive Promoters. Front. Cell Infect. Microbiol. 10, 270. doi: 10.3389/fcimb.2020.00270
Mogollon C. M., Van Pul F. J., Imai T., Ramesar J., Chevalley-Maurel S., De Roo G. M., et al. (2016). Rapid Generation of Marker-Free P. falciparum Fluorescent Reporter Lines Using Modified CRISPR/Cas9 Constructs and Selection Protocol. PLoS One 11, e0168362. doi: 10.1371/journal.pone.0168362
Olotu A., Fegan G., Wambua J., Nyangweso G., Leach A., Lievens M., et al. (2016). Seven-Year Efficacy of RTS,S/AS01 Malaria Vaccine among Young African Children. N. Engl. J. Med. 374, 2519–2529. doi: 10.1056/NEJMoa1515257
Oyen D., Torres J. L., Cottrell C. A., Richter King C., Wilson I. A., Ward A. B. (2018). Cryo-EM structure of P. falciparum circumsporozoite protein with a vaccine-elicited antibody is stabilized by somatically mutated inter-Fab contacts. Sci. Adv. 4, eaau8529. doi: 10.1126/sciadv.aau8529
Payne R. O., Griffin P. M., Mccarthy J. S., Draper S. J. (2017). Plasmodium vivax Controlled Human Malaria Infection - Progress and Prospects. Trends Parasitol. 33, 141–150. doi: 10.1016/j.pt.2016.11.001
Ponnudurai T., Leeuwenberg A. D., Meuwissen J. H. (1981). Chloroquine sensitivity of isolates of Plasmodium falciparum adapted to in vitro culture. Trop. Geogr. Med. 33, 50–54. doi: 10.1017/s0031182000062065
Ponnudurai T., Lensen A. H., Van Gemert G. J., Bensink M. P., Bolmer M., Meuwissen J. H. (1989). Infectivity of cultured Plasmodium falciparum gametocytes to mosquitoes. Parasitology 98 Pt 2, 165–173. doi: 10.1017/S0031182000062065
Porter M. D., Nicki J., Pool C. D., Debot M., Illam R. M., Brando C., et al. (2013). Transgenic parasites stably expressing full-length Plasmodium falciparum circumsporozoite protein as a model for vaccine down-selection in mice using sterile protection as an endpoint. Clin. Vaccine Immunol. 20, 803–810. doi: 10.1128/CVI.00066-13
Reuling I. J., Mendes A. M., De Jong G. M., Fabra-García A., Nunes-Cabaço H., Van Gemert G. J., et al. (2020). An open-label phase 1/2a trial of a genetically modified rodent malaria parasite for immunization against Plasmodium falciparum malaria. Sci. Transl. Med. 12, 1–13. doi: 10.1126/scitranslmed.aay2578
Sack B. K., Miller J. L., Vaughan A. M., Douglass A., Kaushansky A., Mikolajczak S., et al. (2014). Model for in vivo assessment of humoral protection against malaria sporozoite challenge by passive transfer of monoclonal antibodies and immune serum. Infect. Immun. 82, 808–817. doi: 10.1128/IAI.01249-13
Salman A. M., Montoya-Diaz E., West H., Lall A., Atcheson E., Lopez-Camacho C., et al. (2017). Rational development of a protective P. vivax vaccine evaluated with transgenic rodent parasite challenge models. Sci. Rep. 7, 46482. doi: 10.1038/srep46482
Sauerwein R. W., Roestenberg M., Moorthy V. S. (2011). Experimental human challenge infections can accelerate clinical malaria vaccine development. Nat. Rev. Immunol. 11, 57–64. doi: 10.1038/nri2902
Schwenk R., Debot M., Porter M., Nikki J., Rein L., Spaccapelo R., et al. (2014). IgG2 antibodies against a clinical grade Plasmodium falciparum CSP vaccine antigen associate with protection against transgenic sporozoite challenge in mice. PLoS One 9, e111020. doi: 10.1371/journal.pone.0111020
Sinnis P., Coppi A. (2007). A long and winding road: the Plasmodium sporozoite’s journey in the mammalian host. Parasitol. Int. 56, 171–178. doi: 10.1016/j.parint.2007.04.002
Spring M., Polhemus M., Ockenhouse C. (2014). Controlled human malaria infection. J. Infect. Dis. 209(Suppl 2), S40–S45. doi: 10.1093/infdis/jiu063
Stanisic D. I., Mccarthy J. S., Good M. F. (2018). Controlled Human Malaria Infection: Applications, Advances, and Challenges. Infect. Immun. 86, 1–17. doi: 10.1128/IAI.00479-17
Stewart M. J., Vanderberg J. P. (1988). Malaria sporozoites leave behind trails of circumsporozoite protein during gliding motility. J. Protozool. 35, 389–393. doi: 10.1111/j.1550-7408.1988.tb04115.x
Talman A. M., Blagborough A. M., Sinden R. E. (2010). A Plasmodium falciparum strain expressing GFP throughout the parasite’s life-cycle. PLoS One 5, e9156. doi: 10.1371/journal.pone.0009156
Triller G., Scally S. W., Costa G., Pissarev M., Kreschel C., Bosch A., et al. (2017). Natural Parasite Exposure Induces Protective Human Anti-Malarial Antibodies. Immunity 47, 1197–1209.e1110. doi: 10.1016/j.immuni.2017.11.007
van Schaijk B. C., Janse C. J., Van Gemert G. J., Van Dijk M. R., Gego A., Franetich J. F., et al. (2008). Gene disruption of Plasmodium falciparum p52 results in attenuation of malaria liver stage development in cultured primary human hepatocytes. PLoS One 3, e3549. doi: 10.1371/journal.pone.0003549
Vaughan A. M., Kappe S. H. I. (2017). Malaria Parasite Liver Infection and Exoerythrocytic Biology. Cold Spring Harb. Perspect. Med. 7, 1–21. doi: 10.1101/cshperspect.a025486
Vaughan A. M., Aly A. S., Kappe S. H. (2008). Malaria parasite pre-erythrocytic stage infection: gliding and hiding. Cell Host Microbe 4, 209–218. doi: 10.1016/j.chom.2008.08.010
Vaughan A. M., Mikolajczak S. A., Camargo N., Lakshmanan V., Kennedy M., Lindner S. E., et al. (2012). A transgenic Plasmodium falciparum NF54 strain that expresses GFP-luciferase throughout the parasite life cycle. Mol. Biochem. Parasitol. 186, 143–147. doi: 10.1016/j.molbiopara.2012.10.004
Vijayan A., Mejías-Pérez E., Espinosa D. A., Raman S. C., Sorzano C. O. S., Zavala F., et al. (2017). A Prime/Boost PfCS14K(M)/MVA-sPfCS(M) Vaccination Protocol Generates Robust CD8(+) T Cell and Antibody Responses to Plasmodium falciparum Circumsporozoite Protein and Protects Mice against Malaria. Clin. Vaccine Immunol. 24, 1–16. doi: 10.1128/CVI.00494-16
Vos M. W., Stone W. J., Koolen K. M., Van Gemert G. J., Van Schaijk B., Leroy D., et al. (2015). A semi-automated luminescence based standard membrane feeding assay identifies novel small molecules that inhibit transmission of malaria parasites by mosquitoes. Sci. Rep. 5, 18704. doi: 10.1038/srep18704
White M. T., Bejon P., Olotu A., Griffin J. T., Riley E. M., Kester K. E., et al. (2013). The relationship between RTS,S vaccine-induced antibodies, CD4+ T cell responses and protection against Plasmodium falciparum infection. PLoS One 8, e61395. doi: 10.1371/journal.pone.0061395
White M. T., Verity R., Griffin J. T., Asante K. P., Owusu-Agyei S., Greenwood B., et al. (2015). Immunogenicity of the RTS, S/AS01 malaria vaccine and implications for duration of vaccine efficacy: secondary analysis of data from a phase 3 randomised controlled trial. Lancet Infect. Dis. 15, 1450–1458. doi: 10.1016/S1473-3099(15)00239-X
Yadava A., Waters N. C. (2017). Rationale for Further Development of a Vaccine Based on the Circumsporozoite Protein of Plasmodium vivax. PLoS Negl. Trop. Dis. 11, e0005164. doi: 10.1371/journal.pntd.0005164
Yadava A., Hall C. E., Sullivan J. S., Nace D., Williams T., Collins W. E., et al. (2014). Protective efficacy of a Plasmodium vivax circumsporozoite protein-based vaccine in Aotus nancymaae is associated with antibodies to the repeat region. PLoS Negl. Trop. Dis. 8, e3268. doi: 10.1371/journal.pntd.0003268
Keywords: malaria, Plasmodium falciparum, Plasmodium vivax, circumsporozoite protein (CSP), chimeric parasites, vaccines
Citation: Miyazaki Y, Marin-Mogollon C, Imai T, Mendes AM, van der Laak R, Sturm A, Geurten FJA, Miyazaki S, Chevalley-Maurel S, Ramesar J, Kolli SK, Kroeze H, van Schuijlenburg R, Salman AM, Wilder BK, Reyes-Sandoval A, Dechering KJ, Prudêncio M, Janse CJ, Khan SM and Franke-Fayard B (2020) Generation of a Genetically Modified Chimeric Plasmodium falciparum Parasite Expressing Plasmodium vivax Circumsporozoite Protein for Malaria Vaccine Development. Front. Cell. Infect. Microbiol. 10:591046. doi: 10.3389/fcimb.2020.591046
Received: 03 August 2020; Accepted: 11 November 2020;
Published: 17 December 2020.
Edited by:
Nicolas Blanchard, INSERM U1043 Centre de Physiopathologie de Toulouse Purpan, FranceReviewed by:
Georgina Nuri Montagna, Consejo Nacional de Investigaciones Científicas y Técnicas (CONICET), ArgentinaCopyright © 2020 Miyazaki, Marin-Mogollon, Imai, Mendes, van der Laak, Sturm, Geurten, Miyazaki, Chevalley-Maurel, Ramesar, Kolli, Kroeze, van Schuijlenburg, Salman, Wilder, Reyes-Sandoval, Dechering, Prudêncio, Janse, Khan and Franke-Fayard. This is an open-access article distributed under the terms of the Creative Commons Attribution License (CC BY). The use, distribution or reproduction in other forums is permitted, provided the original author(s) and the copyright owner(s) are credited and that the original publication in this journal is cited, in accordance with accepted academic practice. No use, distribution or reproduction is permitted which does not comply with these terms.
*Correspondence: Blandine Franke-Fayard, YmZyYW5rZUBsdW1jLm5s
†Deceased
‡These authors have contributed equally to this work
Disclaimer: All claims expressed in this article are solely those of the authors and do not necessarily represent those of their affiliated organizations, or those of the publisher, the editors and the reviewers. Any product that may be evaluated in this article or claim that may be made by its manufacturer is not guaranteed or endorsed by the publisher.
Research integrity at Frontiers
Learn more about the work of our research integrity team to safeguard the quality of each article we publish.