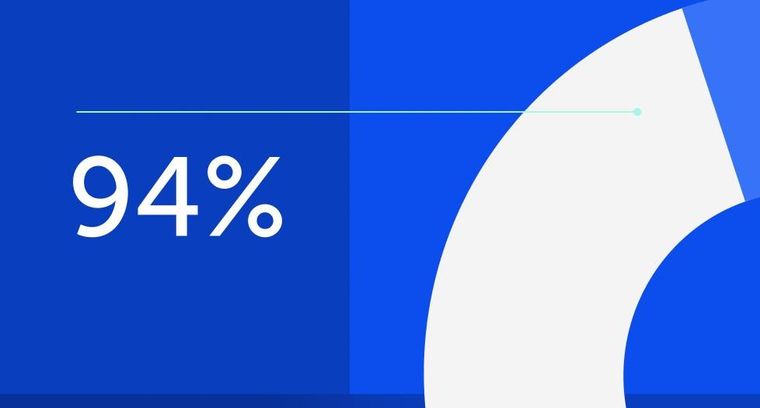
94% of researchers rate our articles as excellent or good
Learn more about the work of our research integrity team to safeguard the quality of each article we publish.
Find out more
BRIEF RESEARCH REPORT article
Front. Cell. Infect. Microbiol., 23 September 2020
Sec. Bacteria and Host
Volume 10 - 2020 | https://doi.org/10.3389/fcimb.2020.581812
Mycobacterium tuberculosis (Mtb) infection is one of the leading causes of death worldwide. The Modified Vaccinia Ankara (MVA) vaccine vector expressing the mycobacterial antigen 85A (MVA85A) was demonstrated to be safe, although it did not improve BCG efficacy, denoting the need to search for improved tuberculosis vaccines. In this work, we investigated the effect of IL-12 DNA -as an adjuvant- on an Ag85A DNA prime/MVA85A boost vaccination regimen. We evaluated the immune response profile elicited in mice and the protection conferred against intratracheal Mtb H37Rv challenge. We observed that the immunization scheme including DNA-A85A+DNA-IL-12/MVA85A induced a strong IFN-γ production to Ag85A in vitro, with a significant expansion of IFN-γ+CD4+ and IFN-γ+CD8+ anti-Ag85A lymphocytes. Furthermore, we also detected a significant increase in the proportion of specific CD8+CD107+ T cells against Ag85A. Additionally, inclusion of IL-12 DNA in the DNA-A85A/MVA85A vaccine scheme induced a marked augment in anti-Ag85A IgG levels. Interestingly, after 30 days of infection with Mtb H37Rv, DNA-A85A+DNA-IL-12/MVA85A vaccinated mice displayed a significant reduction in lung bacterial burden. Together, our findings suggest that IL-12 DNA might be useful as a molecular adjuvant in an Ag85A DNA/MVA prime-boost vaccine against Mtb infection.
Tuberculosis (TB) continues to be one of the main global health problems. In fact, despite the excellent progress of the Directly Observed Treatment (DOT) strategy, and affordable and effective anti-TB treatments, the World Health Organization estimated 1.45 million deaths due to Mycobacterium tuberculosis (Mtb) infection in 2018 (World Health Organization, 2019). TB has always been correlated with poverty, especially in low- and middle-income countries. However, the incidence of TB has increased even in industrialized countries, mainly in vulnerable groups. Furthermore, the strategies for TB control have been hampered by the Human Immunodeficiency Virus (HIV) co-infection and the emergence of drug-resistant Mtb strains. Therefore, developing a vaccine capable of preventing Mtb infection would contribute to controlling the TB epidemic.
The attenuated Mycobacterium bovis Bacillus Calmette-Guérin (BCG) has been the only vaccine available to be used in humans for almost 100 years. BCG is effective in protecting against pulmonary and extrapulmonary TB in children up to 10 years old (Abubakar et al., 2013), but protection against the pulmonary form of TB in adults remains highly controversial (Hatherill et al., 2020).
Currently, there are 15 vaccines in clinical trial phase I, II, or III (Home—ClinicalTrials.gov1). The promising vaccine Modified Vaccinia Ankara (MVA) vector expressing the mycobacterial antigen 85A (MVA85A) was particularly well-tolerated in HIV-1 infected adults (Ndiaye et al., 2015) and infants (Tameris et al., 2013). Unfortunately, the BCG/MVA85A immunization scheme failed to provide protection in phase IIb clinical trials including both neonates and adults (Tameris et al., 2013; Ndiaye et al., 2015). One possibility regarding the low efficacy of the MVA85A might be the restrictive replication capacity of the MVA (Gilbert, 2013). Several strategies have been explored in order to augment MVA immunogenicity. Some of those strategies include heterologous prime/boost protocols, the use of co-stimulatory molecules, the deletion of viral immunomodulatory genes still present in the poxvirus genome and the combined use of adjuvants (García-Arriaza and Esteban, 2014). Therefore, protocols that enhance the immune response induced by MVA85A might be useful to improve the protection of TB vaccines.
Interleukin-12 (IL-12) is a heterodimeric cytokine that plays an essential role in cellular and humoral immunity. Indeed, IL-12 constitutes an important link between innate and adaptive immunity (Gately et al., 1998; Trinchieri, 2003). IL-12 is a pro-inflammatory cytokine produced by antigen-presenting cells like dendritic cells (DCs) and macrophages. IL-12 regulates T-cell and natural killer (NK) cell responses. The importance of IL-12 during the host immune response against Mtb infection has been demonstrated both experimentally and by the increased susceptibility of humans deficient in the IL-12 pathway (Trinchieri, 2003). Moreover, the use of IL-12 as an adjuvant expressed from a plasmid vector in heterologous schemes has been successfully explored (Gherardi et al., 2000, 2001, 2003; Abaitua et al., 2006; Rodríguez et al., 2012; Kalams et al., 2013; Maeto et al., 2014; Elizaga et al., 2018). Additionally, the immune modulator role of IL-12 DNA plasmid when included in DNA vaccines against Leishmania major (Tapia et al., 2003) and Hepatitis B Virus (Chow et al., 1998; Du et al., 2003) infections, has been also evaluated (Hanlon et al., 2001). However, so far, IL-12 has been scarcely used as an adjuvant in the context of TB vaccines.
In the present work, we investigated the use of IL-12 as a molecular adjuvant included in a particular DNA prime/MVA boost vaccine schedule. Our findings indicate that IL-12 DNA might be used to increase the host immune response generated by MVA85A against Mtb infection.
The plasmid pCI-neo (Promega) carrying the coding sequence of Ag85A (pCI-Ag85A) was previously obtained at Dr. Calamante's laboratory at the IABIMO. The expression of Ag85A was confirmed by Western blot using total protein extracts from Baby hamster kidney (BHK-21) transfected cells and a polyclonal anti-Ag85A antibody (Supplementary Figure 1).
The DNA plasmid expressing murine IL-12 (carrying the p35 and p40 murine genes, pCI-IL12) (Gherardi et al., 2000; Tapia et al., 2003) was kindly provided by Dr. Mariano Esteban [Department of Molecular and Cellular Biology, National Center of Biotechnology (CNB), Superior Council of Scientific Investigations (CSIC), Madrid, Spain]. The correct expression of the cytokine from the plasmid pCI-IL12 was corroborated by quantifying IL-12 release in transfected cells' supernatants. Briefly, mouse 3T3 cells were transfected with 5 μg of DNA-IL-12, using Lipofectamine (Invitrogen) according to the manufacturer's instructions. Forty-eight hours post-transfection, 1.8 × 105 pg/ml of IL-12 were detected by ELISA (optEIA, BD) (Maeto, 2015).
Plasmids were purified from Escherichia coli DH5α with Endo free Maxi-Prep purification kits (NucleoBond Xtra Maxi Plus EF, Macherey-Nalgen) using pyrogen-free material.
MVA85A encoding the complete sequence of Ag85A was previously obtained at Dr. Calamante's laboratory at IABIMO. Viral stocks of MVA (expressing ovalbumin, an irrelevant protein that does not overlap with Ag85A) and recombinant MVA85A were propagated in chicken embryo fibroblasts, clarified and titrated as described elsewhere (Cotter et al., 2017). The expression of Ag85A was assessed by Western blot using total protein extracts of chicken embryo fibroblasts infected with MVA85A and a polyclonal anti-Ag85A antibody (Supplementary Figure 1).
In vitro stimulation of cells was performed with recombinant Ag85A (BEI Resources, NIAID, NIH: Ag85A, Recombinant Protein Reference Standard, NR-49427) resuspended in phosphate-buffered saline (PBS; 1.37 mM NaCl, 27 mM KCl, 2 mM KH2PO4, 80 mM Na2H2PO4 anhydrous in water).
Six- to eight-week-old, pathogen-free BALB/c female mice were purchased from the Central animal facility at the University of Buenos Aires, School of Sciences. Animals were housed in biosafety level 3 animal facilities at the Operative Unit of the Biological Containment Center from the National Administration of Laboratories and Health Institutes “Dr. Carlos G. Malbrán” (UOCCCB-ANLIS), according to approved proceedings (Approval code: DEP-20-00). Mice were kept on ventilated racks and fed a standard ad libitum diet. All experimental work was performed following International Guidelines (European Parliament European Council, 2010; National Research Council, 2011). All efforts were made to minimize suffering of the animals.
Mice (4–5/group) received three intramuscular (i.m.) administrations (separated by 2-week intervals) of plasmid DNA (100 μg each time). Two weeks after the last immunization, mice were intraperitoneally (i.p.) inoculated with 107 plaque-forming units (PFU) of MVA. DNA vaccine vectors were diluted in sterile PBS (final volume 50 μl). pCI-IL12 was administered in conjunction with pCI-Ag85A. Sera and splenocytes were collected 8 days after the last immunization. An empty pCI plasmid was used as a DNA control. A recombinant MVA expressing an irrelevant protein was employed as MVA control.
Mice were anesthetized, and blood samples were obtained by cardiac puncture. Then blood was allowed to clot (4 h at room temperature), centrifuged and sera were collected and stored at −80°C.
Splenocytes were isolated by routine methods. Briefly, the spleens were gently disrupted through a 40-μm cell-strainer (BD Biosciences). Subsequently, red blood cells were lysed, and splenocytes were washed with RPMI (RPMI-1640 medium, Gibco BRL) plus 5% (v/v) fetal bovine serum (FBS, Natocor). Splenocytes were then resuspended at 10 × 106 cells/mL in RPMIc [RPMI-1640 medium (Gibco BRL) supplemented with 2 mM L-glutamine (Gibco BRL), 100 U/mL penicillin (Gibco BRL), 0.1 mg/mL streptomycin (Gibco BRL), 10 mM HEPES (Gibco BRL), and 0.055 mM β-Mercaptoethanol (Gibco BRL), with 10% (v/v) FBS (Natocor)].
Splenocytes were cultured (106 cells/wells) in 96-well U bottom plates with RPMIc for 16 h, with or without Ag85A protein (5 μg/mL) plus anti-CD28 (1 ng/mL; BD Biosciences). In some experiments, anti-CD107a and anti-CD107b (CD107a/b-FITC; BD Biosciences) antibodies were added to Ag85A stimulated cells to measure the cytotoxic activity of CD8 T lymphocytes (Betts et al., 2003). Cells stimulated during 5 h with phorbol myristate acetate (PMA, 10 ng/mL, Sigma-Aldrich) plus ionomycin (250 ng/mL, Sigma-Aldrich) were used as positive controls. Monensin (2 μM; Sigma-Aldrich) was added for the last 5 h of culture. After washing, anti-CD4-APC and anti-CD8-PerCP (BioLegend) antibodies were added for 30 min. Then, cells were fixed using 2% paraformaldehyde (PFA) and permeabilizated with 0.5% (v/v) saponin (Sigma-Aldrich), 0.01% (w/v) sodium azide, and 10% (v/v) FBS (Natocor) in PBS. Finally, cells were stained using anti-IFN-γ-PE (Biolegend) for 30 min at 4°C in the dark. Cells were acquired on a FACSAria II flow cytometer (BD Biosciences). Isotype matched controls and fluorescence minus one (FMO) controls were included in each experiment. Data were analyzed using FlowJo v10 software (BD Biosciences). Gates strategies and FACS examples are shown in Figure 3.
Splenocytes were cultured at 106 cells/well (in triplicate wells) in 96-well U bottom plates with RPMIc for 72 h with or without recombinant Ag85A (5 μg/mL). Cells stimulated with Concanavalin A (ConA, 1 mg/mL) or PBS were used as positive and negative controls, respectively. Culture supernatants were stored at −80°C until assayed. IFN-γ analysis was performed using ELISA BD OptEIA set (BD Biosciences), according to the manufacturer's instructions.
The presence of serum anti-Ag85A Abs was assayed by ELISA. Ninety-six-well plates were coated with purified Ag85A at 2.5 μg/mL. Blockage was performed with 10% (v/v) FBS in PBS-0.05% Tween 20. Samples (duplicates) were diluted in blocking solution. Detection was performed using mouse anti-IgG conjugated to peroxidase enzyme (Jackson Immuno Research). TMB Peroxidase substrate (Sigma-Aldrich) was added and the reaction was stopped by adding 2 N H2SO4. Absorbance was measured at 450 nm.
Mycobacterium tuberculosis H37Rv strain (Mtb H37Rv) was grown in Middlebrook 7H9 broth (BD Biosciences) or in 7H10 agar (BD Biosciences), 0.2% (v/v) glycerol, and albumin-dextrose-catalase-oleic acid supplement (BD Biosciences). Cultures were harvested at exponential growing phase at 37°C and clumps were disaggregated by glass bead vortexing and passage through a 25 Gauge needle. Bacteria were centrifuged twice for 10 min at 3,300 × g and supernatants were diluted with physiological solution. Finally, OD at 600 nm was determined. Mtb H37Rv bacterial growth was performed in BSL3 security cabinets at the UOCCCB-ANLIS.
Groups of five female BALB/c mice were intratracheally inoculated with Mtb H37Rv (1 × 104 colony-forming unit (CFU)/mice) as previously described (Revelli et al., 2012). Briefly, animals were anesthetized with a mixture of ketamine and xylazine (100 and 10 mg/kg, respectively), placed in supine position over an acrylic backboard and the inoculum (20 μL) was injected through the trachea with a Hamilton syringe coupled to a blunt-ended probe. Mice were euthanized at 30 days post-infection by an intraperitoneal injection of a lethal dose of ketamine and xylazine, and their spleens and lungs were aseptically removed. The whole organs were homogenized in 2 mL of sterile RPMI. Then, triplicates of serial dilutions were plated in Middlebrook 7H10 agar for CFU counting.
The quantitative data were expressed as mean ± standard error of the mean (SEM). Analysis of variances (ANOVA) followed by Holm-Sidak's multiple comparison tests were used to analyze differences between groups. For categorical variables, the Fisher exact test was performed to compare proportions of infected spleens. GraphPad Prism 6.0 (GraphPad Software) was used for all statistical analysis. A p < 0.05 was considered as statistically significant.
In order to evaluate the potential adjuvant role of IL-12 (expressed from a DNA vector) to increase the efficacy of pCI-Ag85A and the heterologous pCI-Ag85A/MVA85A immunization scheme, we immunized 6- to 8-week-old BALB/c mice as shown in Figure 1. Briefly, each group of animals was inoculated by the i.m. route with three doses of DNA vectors separated by 2 weeks among them. In some groups, after 14 days of the last priming dose, mice were i.p. boosted with 107 PFU/animal of MVA85A. Mice from control groups were immunized with empty DNA plasmid (pCI) and a recombinant MVA expressing an irrelevant protein. Eight days after the last dose, we analyzed the humoral and cellular immune responses against Ag85A elicited by animals from the different vaccinated groups.
Figure 1. Experimental design. ROA, Route of Administration; i.m., intramuscular; i.p., intraperitoneally; pCI, pCI empty; pCI-IL12, pCI expressing IL-12; pCI-Ag85A, pCI expressing Ag85A; MVA, recombinant MVA expressing an irrelevant protein; MVA85A, recombinant MVA expressing Ag85A.
To characterize the host cellular immune response generated by vaccination during the acute phase, we isolated splenocytes 8 days after the last inoculation. Cells were then stimulated in vitro with Ag85A, PBS (negative control), or ConA (positive control) for 72 h. Considering that a type 1 immune response generated after vaccination is critical for protection against Mtb, we analyzed IFN-γ levels in culture supernatants (Figures 2A,B). Mice immunized with pCI-Ag85A expressed low levels of IFN-γ. However, a significant increase in the amount of this cytokine was detected when pCI-IL12 was co-administered to the animals. Furthermore, the highest levels of IFN-γ were observed in mice that were boosted with MVA85A (Figure 2A). As expected, splenocytes from pCI-Ag85A, pCI-Ag85A+pCI-IL12, pCI-Ag85A/MVA85A, and pCI-Ag85A+pCI-IL12/MVA85A mice produced significantly higher IFN-γ levels in response to Ag85A stimulation as compared to their controls. Interestingly, co-administration of pCI-IL12 and pCI-Ag85A significantly augmented the levels of IFN-γ, denoting the clear adjuvant effect of IL-12 (Figure 2B).
Figure 2. IL-12 DNA administration enhances the immune response of the host to Ag85A. (A,B) Splenocytes were stimulated in vitro with Ag85A (5 μg/mL), ConA or PBS for 72 h. IFN-γ production was then determined by ELISA. Each bar represents the mean of IFN-γ production (PBS subtracted) ± SEM. (C,D) Anti-Ag85A IgG levels in mice serum were determined by ELISA. The samples were diluted (C) 1/100 and (D) 1/10,000 in PBS-10% fetal bovine serum. Each bar represents the mean of OD 450nm ± SEM. Comparisons among groups were determined by one-way ANOVA followed by Holm-Sidak's multiple comparison test. A value of p < 0.05 was considered statistically significant. *p < 0.05, **p < 0.01, ***p < 0.001, and ****p < 0.0001. Results are representative of two independent experiments.
Although the relevance of antibodies in the protection against Mtb is not clear, the humoral response cannot be ruled out as a factor that contributes to Mtb containment. Protective antibodies might promote and increase intracellular bacterial death through phagocytosis mediated by FcR, and improve the immune response via the rapid antigen adsorption and processing. Therefore, we evaluated the levels of anti-Ag85A IgG in serum. As shown in Figure 2C, we observed a slight increment of anti-Ag85A IgG levels when pCI-IL12 was co-administered with pCI-Ag85A. Nevertheless, MVA85A boost induced a significant increase in anti-Ag85A IgG in serum of vaccinated mice. Importantly, when we evaluated the effect of IL-12 on the MVA boosted strategy, mice that received pCI-IL12 as an adjuvant displayed the highest levels of anti-Ag85A IgG (Figure 2D).
Next, we investigated whether the use of IL-12 as an adjuvant stimulated the production of IFN-γ by different populations of T lymphocytes. To do this, 8 days after the last antigenic dose, mice were sacrificed, and recovered splenocytes were stimulated in vitro with Ag85A. We observed that pCI-Ag85A/MVA85A immunization induced a marked augment in the percentage of CD4+ and CD8+ IFN-γ+ T lymphocytes (Figures 3B,C). Even more, when pCl-IL12 was co-administrated with pCI-Ag85A, a significantly higher increase in the percentage of CD4+IFN-γ+ and CD8+ IFN-γ+ T cells was detected as compared to the pCI-Ag85A animal group (Figures 3B,C).
Figure 3. The co-administration of IL-12 DNA induced a strong cellular immune response against the Ag85A in mice spleen. Splenocytes from immunized mice were stimulated in vitro with PBS or recombinant Ag85A for 16 h. Then, IFN-γ production and CD107a/b surface expression were determined by flow cytometry. (A) Gate strategy. CD4+ or CD8+ IFN-γ+ T cells and CD8+CD107a/b+ T lymphocytes were identified by flow cytometry first gating on lymphocytes by light scatter; then, gating on CD4+ and CD8+ T cells; and finally, gating according to IFN-γ+ and CD107a/b+ expression using the AND boolean gate tool from FlowJo v10 software. Each bar represents the mean (PBS subtracted) of the (B) %CD4+ IFN-γ+ cells ± SEM, (C) %CD8+IFN-γ+ cells ± SEM, (D) %CD8+CD107a/b+ cells ± SEM, (E) %CD8+CD107a/b+IFN-γ+ cells ± SEM. Comparisons among groups were determined by one-way ANOVA followed by Holm-Sidak's multiple comparison test. A value of p < 0.05 was considered statistically significant. n.s., non-significant difference, *p < 0.05, **p < 0.01, ***p < 0.001, and ****p < 0.0001. Results are representative of two independent experiments. Representative dot plots are shown for (F) % CD4+IFN-γ+ T cells, (G) % CD8+IFN-γ+ T cells, and (H) % CD8+CD107a/b+ T lymphocytes (large quadrants). Controls (small quadrants) are shown.
Besides, in order to evaluate the cytotoxic potential of specific CD8+ T cells expanded by the immunization, we incubated splenocytes from the different immunized groups with Ag85A plus anti-CD107 monoclonal antibody. As shown in Figure 3D, pCI-Ag85A/MVA85A immunization induced the expression of CD107a/b on CD8+ T lymphocytes. Importantly, this response resulted significantly improved when IL-12 was included in the immunization scheme (Figure 3D). Even more, the adjuvant effect of IL-12 was also observed when the percentage of CD8+IFN-γ+CD107a/b+ cells induced in immunized mice was analyzed (Figure 3E). In conclusion, these results denote that additional benefits of including IL-12 adjuvant in vaccine formulations may be the expansion and activation of CD8+ cytotoxic T lymphocytes.
Considering our findings demonstrating that pCI-IL12 potentiates the anti-Ag85 systemic immune response induced by pCI-85A/MVA85A vaccination, we next decided to evaluate its efficacy against Mtb containment. For this, 4 weeks after the last immunization (Figure 1), mice were intratracheally infected with pathogenic Mtb H37Rv strain. After 30 days, the number of CFU per lung and the presence of bacteria in spleen were determined. As shown in Figure 4, pCI-Ag85A/MVA85A vaccination slightly diminished the total CFU per lung as compared to control animals. In contrast, by using pCI-IL12 during vaccination, a significant decrease in the number of CFU per lung was observed as compared to animals that did not receive the adjuvant (Figure 4A). In line with these results, 75% of pCI-Ag85A/MVA85A vaccinated mice displayed Mtb in their spleens, whereas only 25% of pCI-Ag85A+pCI-IL12/MVA85A vaccinated animals presented bacteria in their spleens (Figure 4B). These results suggest an association between the increase in the cellular immune response and the protection improvement in mice that received IL-12.
Figure 4. pCI-IL12 improves control of Mtb H37Rv in mice. Immunizations were performed as described above. Four weeks after the last immunization, mice were challenged with Mtb H37Rv via intratracheal route (n = 4–5/group). Mice were sacrificed 4 weeks after challenge. (A) CFUs in lungs were analyzed by culturing lung homogenates and enumerating the bacteria. Each bar represent the median (CFU/lung for each group of mice). Comparisons among groups were determined by one-way ANOVA followed by Holm-Sidak's multiple comparison test. A value of p < 0.05 was considered statistically significant. *p < 0.05 and **p < 0.01. (B) The presence of Mtb in spleens was analyzed in all the studied groups. The graph shows the percentage of mice displaying bacteria in their spleens. Comparisons with control groups were determined by Fisher's exact test, #p < 0.05. A representative example of three assays is shown.
Nowadays, Mycobacterium bovis BCG displays 90% of effectiveness in childhood tuberculous meningitis and miliary TB (Mangtani et al., 2014). However, regarding pulmonary TB, the most common form of the disease in adults and teenagers (World Health Organization, 2019), BCG confers 59% of protection in children (Mangtani et al., 2014). Furthermore, BCG does not protect after 10–15 years of vaccination in endemic countries (Sterne et al., 1998; Abubakar et al., 2013). Thus, in order to eradicate TB, new vaccines conferring protection during the whole life of the individual are required.
Preventive vaccines against TB can be classified into four broad categories: viable whole-cell vaccines (such as VPM1002, MTBVAC or BCG revaccination), inactivated whole-cell vaccines (such as Vaccae™, RUTI, DAR-901, MIP, or AEC/BCO2), protein subunit vaccine (such as ID93/GLA-SE, H56:IC31, M72/AS01E, or GamTBVac), and viral-vectored vaccines (such as ChAdOx185A-MVA85A, TB/FLU-04L, or Ad5Ag85A) (Home—ClinicalTrials.gov1). Among these 15 vaccine candidates in clinical phase, the adjuvants employed include GLA-SE, AS01E, IC31, and CpG plus alum salt-based adjuvant or DEAE-dextran core. All of the mentioned adjuvants are included in protein subunits vaccines formulations, and there are currently no clinical trials reports using DNA vaccines. Given the importance of IL-12 during the immune response of the host against Mtb infection, its use as a vaccine adjuvant has been previously proposed (Ha et al., 2006; Yoshida et al., 2006).
IL-12 helps naïve T cells to switch to Th1 lymphocytes and increases T cells cytotoxicity. The use of IL-12 as an adjuvant has been investigated in vaccines against several pathogens (Chow et al., 1998; Gherardi et al., 2000, 2001, 2003; Du et al., 2003; Tapia et al., 2003; Abaitua et al., 2006; Rodríguez et al., 2012; Kalams et al., 2013; Maeto et al., 2014; Elizaga et al., 2018). However, the study of IL-12 as an adjuvant in TB vaccines has been scarcely explored. This might be partly because recombinant IL-12 is quickly removed and inactivated in vivo (Ha et al., 2006). In fact, to avoid IL-12 removal, Ha et al. (2006) encapsulated recombinant IL-12 in microspheres to achieve its sustained local expression. The authors showed that the combination of IL-12 plus AS01B adjuvants in a TB subunit vaccine-induced mice immunity and protection against Mtb challenge. In addition, Yoshida et al. used the hemagglutinating virus of Japan (HVJ)-liposome method to encapsulate mouse IL-12 expression vector (mIL-12). Then, the authors immunized mice with mIL-12/HVJ plus DNA expressing IgHsp65 (Yoshida et al., 2006). However, no significant differences in the number of CFU per lung were detected between the mIL-12/HVJ (control) and the IgHsp65+mIL-12/HVJ groups (Yoshida et al., 2006). Therefore, the protection described by these authors would not be associated with the host immune response against Hsp65 antigen immunization.
Cytokines are crucial initiators and regulators of the immune response. In TB, key effectors related to host protection include IL-12 and IFN-γ (North and Jung, 2004; Cooper, 2009). IL-12 displays multiple biological functions in immunity modulation, linking the early innate immune response with the subsequent antigen-specific adaptive immunity (Hamza et al., 2010). Accordingly, it has been demonstrated that mice deficient in IL-12 were more susceptible to Mtb infection than wild-type animals (Hölscher et al., 2001). Moreover, IL-12 is critical for activation of CD4+T cells, leading to the protective Th1 response that promotes IFN-γ secretion by T lymphocytes. Therefore, given the crucial role of IL-12 in TB infection, in this study, we evaluated the incorporation of plasmid DNA encoding murine IL-12 during Ag85A DNA prime followed by a heterologous boost with MVA85A. In line with our work, other authors have previously evaluated the modulation of the response induced by DNA vaccines co-injected with pro-inflammatory cytokines in individual vectors (Kim et al., 1998; Tapia et al., 2003; Rodríguez et al., 2012). Initially, we analyzed the adjuvant effect of IL-12 DNA on Ag85A DNA immunization as compared to mice that received Ag85A DNA alone (Figures 2A,C). We observed that IL-12 DNA co-administration significantly increased both the levels of IgG and IFN-γ in response to Ag85A. Furthermore, when mice received a boost of MVA85A, even higher levels of IgG and IFN-γ against Ag85A were detected (Figure 2). Moreover, the IFN-γ secretion augment was a reflection of the increase of CD4+ and CD8+ IFN-γ producing lymphocytes (Figures 3B,C). In addition, the co-administration of IL-12 adjuvant induced the expansion of a higher proportion of cytotoxic CD8 T cells (Figures 3D,E). Even more, mice that received a vaccination scheme augmenting the host immune response showed the highest protection against Mtb challenge. However, in order to further analyze bacterial load, future studies including staining of Mtb (such as acid-fast staining) in tissue samples should be performed.
In this study, we employed Ag85A and IL-12 in different vectors. We had previously used this same strategy using either IL-12 or GM-CSF in trans-expression, with optimal results (Rodríguez et al., 2012). Considering that IL-12 is a soluble mediator required in the microenvironment, the use of individual vectors might have a positive effect without the need to be expressed in the same cell as the antigen. Other authors have used different cytokines expressed by DNA in trans as adjuvants in DNA vaccines (Hanlon et al., 2001; Tapia et al., 2003; Abaitua et al., 2006). However, in the context of TB disease, Sun et al. (2017) have previously employed the cis-expression of Ag85A and IL-15 with encouraging results.
During clinical trials, MVA85A was commonly administered by the intradermal route (Tameris et al., 2013; Ndiaye et al., 2015). Nevertheless, when Tchilian et al. (2009) used the intradermal route to apply MVA85A as a BCG boost in the murine model, they were unable to observe any improved effect beyond the protection conferred by BCG. Furthermore, MVA85A immunogenicity and efficacy in mice have been demonstrated using nasal (Goonetilleke et al., 2003) or intravenous (McShane et al., 2002; Romano et al., 2006) routes. Thus, since immunization with DNA vaccines is usually performed by i.m. route while MVA boost is normally achieved by i.p. route (Rodríguez et al., 2012; Pérez et al., 2020) we used those immunization routes in our mice model. Importantly, this immunization strategy using an adjuvant plus a viral vector was used with the ultimate aim of eliciting efficient humoral and cellular immune responses against the selected antigen.
Several cytokines have a critical function in the immune response of the host against Mtb (Domingo-Gonzalez et al., 2016). The central role of IFN-γ in the immune response against Mtb infection has been widely demonstrated both in mouse models (Flynn et al., 1993) and humans. Accordingly, we previously reported that TB patients with a weak immune response against Mtb and impaired IFN-γ production displayed the most severe disease (Pasquinelli et al., 2004). Moreover, we demonstrated that IFN-γ promotes autophagy in infected monocytes from TB patients contributing to the elimination of Mtb (Tateosian et al., 2017). In the present work, we focused on IFN-γ determination, although the role of other crucial cytokines in TB infection should be evaluated in the future. In fact, Sakai et al. reported that IFN-γ from CD4+ T lymphocytes were responsible for only one third of the total anti-bacterial effects of CD4+ T cells in the lungs (Sakai et al., 2016). Furthermore, increasing IFN-γ production led to the early death of the host in mice infection models (Sakai et al., 2016). Additionally, it has been reported that a polyfunctional Mtb specific CD4+ T cell immune response is required for protective pulmonary immunity during TB (Wu et al., 2017). In fact, IFN-γ could be a part of the response of polyfunctional Mtb specific lymphocytes (IFN-γ/IL-2/IL-17/TNF-α) but the role of the other cytokines in the immune response of the host against Mtb has to be considered as well. Nevertheless, Beveridge et al. (2007) reported that MVA85A immunization induced polyfunctional CD4+ T lymphocytes producing TNF-α, IFN-γ, and IL-2 in humans, but no increase in protection was observed. Moreover, the development of phase I of the AERAS-402 candidate vaccine (a recombinant adenovirus which expresses the Mtb antigens Ag85A, Ag85B, and TB10.4) demonstrated that polyfunctional T cell response were not directly associated with the ability of these lymphocytes to identify Mtb infected cells (Nyendak et al., 2016). In any case, the role of polyfunctional T cells in our present model should be investigated in future experiments.
The adjuvant potential of IL-12 to induce cytotoxicity has been previously demonstrated (Okada et al., 1997; Tsuji et al., 1997; Belyakov et al., 1998; Tatsumi et al., 2001; Moore et al., 2002; Matsui et al., 2003). Mtb can infect epithelial and endothelial cells, and CD8 lymphocytes (together with NK cells) have the ability to eliminate infected cells. Furthermore, cytotoxic CD8 cells have a critical role in killing infected macrophages during TB. Our findings showed that immunization with IL-12 and Ag85A as DNA plus a boost of MVA85A induced a significant increment in the percentage of Ag85A specific CD8+ CD107a/b+ cells (Figure 3D).
Immune response against Mtb is complex, but the participation of macrophages and T lymphocytes in the defense of the host against the pathogen has been extensively demonstrated (Chai et al., 2020). In contrast, the role of humoral immunity in the fight against Mtb has been ignored for many years. For this reason, most vaccine candidates focus on the development of appropriate cellular immune responses against Mtb. Nevertheless, there are studies demonstrating that polyfunctional and neutralizing antibodies might potentiate humoral effector functions of macrophages and NK cells (Choreño-Parra et al., 2020). By evaluating the production of antibodies, significantly higher levels of anti-Ag85A IgG were detected in mice that received three-doses of Ag85A DNA plus IL-12 DNA as compared to mice immunized with three-doses of Ag85A DNA alone (Figure 2B). Moreover, we observed a significant increase in serum IgG against Ag85A in mice immunized with pCI-Ag85A and MVA85A that also received pCI-IL12 as an adjuvant (Figure 2D). These results are in line with previous reports showing that administration of IL-12 as an adjuvant improves the humoral response of vaccine candidates (McKnight et al., 1994; Germann et al., 1995; Metzger et al., 1996). Importantly, a retrospective analysis in BCG-vaccinated children who received MVA85A showed that elevated anti-Ag85A IgG levels at 28 days after MVA85A boost, reduced the risk of TB development (Fletcher et al., 2016). Therefore an association between the levels of antibodies against Ag85A and the probability of Mtb disease in infants was suggested (Fletcher et al., 2016).
Cytokines present in the environment impact on maturation of DCs by modulating their functions during the maturation and migration processes (Banchereau and Steinman, 1998; Fukao et al., 2001). DCs are professional antigen-presentation cells and their main functions are antigen capture and processing, migration to secondary lymphoid organs, and T cell priming. It has been demonstrated that DCs express a single class of high-affinity IL-12R (functionally distinct from those in T and NK cells) that upon binding IL-12 activates members of the NF-κB family and leads to endogenous IL-12 production (Grohmann et al., 1998). Therefore, we hypothesized that in our model, the adjuvant effect of IL-12 DNA on the immunogenicity of the DNA vaccine might be related to the local production of IL-12 by a mechanism similar to the one described by Grohmann et al. In fact, IL-12 produced by IL-12 DNA at the site of injection would induce DCs to secrete more IL-12 eliciting a Th1 profile and activating CD8 cells at the draining lymph node. Furthermore, there is evidence that IL-12 and antigen presentation can be produced by different cells (Bianchi et al., 1999). On the other hand, CDs and monocytes were shown to be capable of producing IFN-γ when cultured in the presence of IL-12, playing an important role in innate immunity and subsequent T helper cell type 1 development in vivo (Ohteki et al., 1999).
Taken together, our results demonstrate the ability of IL-12 DNA to improve the humoral and cellular immune responses against Ag85A induced by DNA/MVA85A immunization. Thus, IL-12 DNA might be considered as a tool for designing vaccine strategies to control Mtb infection.
All datasets generated for this study are included in the article/Supplementary Material.
This animal study was reviewed and approved by Operative Unit of the Biological Containment Center from the National Administration of Laboratories and Health Institutes Dr. Carlos G. Malbrán (UOCCCB-ANLIS).
The writing—original draft preparation as well as the writing—review and editing of the manuscript was in charge of MPM and VEG. Resources were provided by VEG, MMG, and GC. MPM and MPD were in charge of the development of the methodology. MPM, MPD, and JMP performed the experiments and analysis of the data. NOA and NLT performed formal analyses. VEG was responsible for the supervision, project administration, and conceptualization of the present work. All authors have read and agreed to the published version of the manuscript.
This work was supported by grants from Agencia Nacional de Promoción Científica y Tecnológica (ANPCyT) (PICT2015-0611 and PICT2017-1451 to VEG), Universidad de Buenos Aires (UBACyT) (20020170100127BA to VEG), and Instituto Nacional de Tecnología Agropecuaria (INTA) (2019-PD-I102). The funders had no role in the design of the study; in the collection, analyses, or interpretation of data; in the writing of the manuscript, or in the decision to publish the results.
The authors declare that the research was conducted in the absence of any commercial or financial relationships that could be construed as a potential conflict of interest.
We thank Dr. Agustin Rolandelli for continuous support and Licenciado Guillermo Piazza for technical assistance. We also thank all members of the UOCCCB-ANLIS and Central animal FCEN-UBA for advice and technical assistance.
The Supplementary Material for this article can be found online at: https://www.frontiersin.org/articles/10.3389/fcimb.2020.581812/full#supplementary-material
BCG, Bacillus Calmette-Guérin; ConA, Concanavalin A; IL-12, interleukin-12; Mtb, Mycobacterium tuberculosis; MVA, Modified Vaccinia Ankara; MVA85A, MVA expressing the mycobacterial antigen 85A; pCI, empty pCI plasmid; pCI-Ag85A, pCI plasmid expressing Ag85A; pCI-IL12, pCI plasmid expressing murine IL-12; TB, tuberculosis.
1. ^https://clinicaltrials.gov/ (accessed June 19, 2020).
Abaitua, F., Rodríguez, J. R., Garzón, A., Rodríguez, D., and Esteban, M. (2006). Improving recombinant MVA immune responses: potentiation of the immune responses to HIV-1 with MVA and DNA vectors expressing Env and the cytokines IL-12 and IFN-gamma. Virus Res. 116, 11–20. doi: 10.1016/j.virusres.2005.08.008
Abubakar, I., Pimpin, L., Ariti, C., Beynon, R., Mangtani, P., Sterne, J., et al. (2013). Systematic review and meta-analysis of the current evidence on the duration of protection by bacillus Calmette-Guérin vaccination against tuberculosis. Health Technol. Assess. 17, 1–372. doi: 10.3310/hta17370
Banchereau, J., and Steinman, R. M. (1998). Dendritic cells and the control of immunity. Nature 392, 245–252. doi: 10.1038/32588
Belyakov, I. M., Ahlers, J. D., Brandwein, B. Y., Earl, P., Kelsall, B. L., Moss, B., et al. (1998). The importance of local mucosal HIV-specific CD8(+) cytotoxic T lymphocytes for resistance to mucosal viral transmission in mice and enhancement of resistance by local administration of IL-12. J. Clin. Invest. 102, 2072–2081. doi: 10.1172/JCI5102
Betts, M. R., Brenchley, J. M., Price, D. A., De Rosa, S. C., Douek, D. C., Roederer, M., et al. (2003). Sensitive and viable identification of antigen-specific CD8+ T cells by a flow cytometric assay for degranulation. J. Immunol. Methods 281, 65–78. doi: 10.1016/S0022-1759(03)00265-5
Beveridge, N. E. R., Price, D. A., Casazza, J. P., Pathan, A. A., Sander, C. R., Asher, T. E., et al. (2007). Immunisation with BCG and recombinant MVA85A induces long-lasting, polyfunctional Mycobacterium tuberculosis-specific CD4+ memory T lymphocyte populations. Eur. J. Immunol. 37, 3089–3100. doi: 10.1002/eji.200737504
Bianchi, R., Grohmann, U., Vacca, C., Belladonna, M. L., Fioretti, M. C., and Puccetti, P. (1999). Autocrine IL-12 is involved in dendritic cell modulation via CD40 ligation. J. Immunol. 163, 2517–2521.
Chai, Q., Lu, Z., and Liu, C. H. (2020). Host defense mechanisms against Mycobacterium tuberculosis. Cell. Mol. Life Sci. 77, 1859–1878. doi: 10.1007/s00018-019-03353-5
Choreño-Parra, J. A., Weinstein, L. I., Yunis, E. J., Zúñiga, J., and Hernández-Pando, R. (2020). Thinking outside the box: innate- and B cell-memory responses as novel protective mechanisms against tuberculosis. Front. Immunol. 11:226. doi: 10.3389/fimmu.2020.00226
Chow, Y.-H., Chiang, B.-L., Lee, Y.-L., Chi, W.-K., Lin, W.-C., Chen, Y.-T., et al. (1998). Development of Th1 and Th2 populations and the nature of immune responses to hepatitis B virus DNA vaccines can be modulated by codelivery of various cytokine genes. J. Immunol. 160, 1320–1329.
Cooper, A. M. (2009). Cell-mediated immune responses in tuberculosis. Annu. Rev. Immunol. 27, 393–422. doi: 10.1146/annurev.immunol.021908.132703
Cotter, C. A., Earl, P. L., Wyatt, L. S., and Moss, B. (2017). “Preparation of cell cultures and vaccinia virus stocks: preparation of cell cultures and vaccinia virus stocks,” in Current Protocols in Protein Science, eds J. E. Coligan, B. M. Dunn, D. W. Speicher, and P. T. Wingfield (Hoboken, NJ: John Wiley & Sons, Inc.), 5.12.1–5.12.18. doi: 10.1002/cpps.34
Domingo-Gonzalez, R., Prince, O., Cooper, A., and Khader, S. A. (2016). Cytokines and chemokines in Mycobacterium tuberculosis infection. Microbiol. Spectr. 4. doi: 10.1128/microbiolspec.TBTB2-0018-2016
Du, D.-W., Jia, Z.-S., Li, G.-Y., and Zhou, Y.-Y. (2003). HBV DNA vaccine with adjuvant cytokines induced specific immune responses against HBV infection. World J. Gastroenterol. 9, 108–111. doi: 10.3748/wjg.v9.i1.108
Elizaga, M. L., Li, S. S., Kochar, N. K., Wilson, G. J., Allen, M. A., Tieu, H. V. N., et al. (2018). Safety and tolerability of HIV-1 multiantigen pDNA vaccine given with IL-12 plasmid DNA via electroporation, boosted with a recombinant vesicular stomatitis virus HIV Gag vaccine in healthy volunteers in a randomized, controlled clinical trial. PLoS ONE 13:e0202753–e0202753. doi: 10.1371/journal.pone.0202753
European Parliament European Council (2010). Directive 2010/63/EU of the European Parliament and of the Council of 22 September 2010 on the Protection of Animals Used for Scientific Purposes Text with EEA Relevance. Available online at: https://eur-lex.europa.eu/eli/dir/2010/63/oj (accessed June 19, 2020).
Fletcher, H. A., Snowden, M. A., Landry, B., Rida, W., Satti, I., Harris, S. A., et al. (2016). T-cell activation is an immune correlate of risk in BCG vaccinated infants. Nat. Commun. 7:11290. doi: 10.1038/ncomms11290
Flynn, J. L., Chan, J., Triebold, K. J., Dalton, D. K., Stewart, T. A., and Bloom, B. R. (1993). An essential role for interferon gamma in resistance to Mycobacterium tuberculosis infection. J. Exp. Med. 178, 2249–2254. doi: 10.1084/jem.178.6.2249
Fukao, T., Frucht, D. M., Yap, G., Gadina, M., O'Shea, J. J., and Koyasu, S. (2001). Inducible expression of Stat4 in dendritic cells and macrophages and its critical role in innate and adaptive immune responses. J. Immunol. 166, 4446–4455. doi: 10.4049/jimmunol.166.7.4446
García-Arriaza, J., and Esteban, M. (2014). Enhancing poxvirus vectors vaccine immunogenicity. Hum. Vaccines Immunother. 10, 2235–2244. doi: 10.4161/hv.28974
Gately, M. K., Renzetti, L. M., Magram, J., Stern, A. S., Adorini, L., Gubler, U., et al. (1998). The interleukin-12/interleukin-12-receptor system: role in normal and pathologic immune responses. Annu. Rev. Immunol. 16, 495–521. doi: 10.1146/annurev.immunol.16.1.495
Germann, T., Bongartz, M., Dlugonska, H., Hess, H., Schmitt, E., Kolbe, L., et al. (1995). Interleukin-12 profoundly up-regulates the synthesis of antigen-specific complement-fixing IgG2a, IgG2b and IgG3 antibody subclasses in vivo. Eur. J. Immunol. 25, 823–829. doi: 10.1002/eji.1830250329
Gherardi, M. M., Ramírez, J. C., and Esteban, M. (2000). Interleukin-12 (IL-12) enhancement of the cellular immune response against human immunodeficiency virus type 1 Env antigen in a DNA prime/vaccinia virus boost vaccine regimen is time and dose dependent: suppressive effects of IL-12 boost are mediated by nitric oxide. J. Virol. 74, 6278–6286. doi: 10.1128/JVI.74.14.6278-6286.2000
Gherardi, M. M., Ramírez, J. C., and Esteban, M. (2001). Towards a new generation of vaccines: the cytokine IL-12 as an adjuvant to enhance cellular immune responses to pathogens during prime-booster vaccination regimens. Histol. Histopathol. 16, 655–667. doi: 10.14670/HH-16.655
Gherardi, M. M., Ramírez, J. C., and Esteban, M. (2003). IL-12 and IL-18 act in synergy to clear vaccinia virus infection: involvement of innate and adaptive components of the immune system. J. Gen. Virol. 84, 1961–1972. doi: 10.1099/vir.0.19120-0
Gilbert, S. C. (2013). Clinical development of modified vaccinia virus ankara vaccines. Vaccine 31, 4241–4246. doi: 10.1016/j.vaccine.2013.03.020
Goonetilleke, N. P., McShane, H., Hannan, C. M., Anderson, R. J., Brookes, R. H., and Hill, A. V. S. (2003). Enhanced immunogenicity and protective efficacy against Mycobacterium tuberculosis of bacille Calmette-Guérin vaccine using mucosal administration and boosting with a recombinant modified vaccinia virus Ankara. J. Immunol. 171, 1602–1609. doi: 10.4049/jimmunol.171.3.1602
Grohmann, U., Belladonna, M. L., Bianchi, R., Orabona, C., Ayroldi, E., Fioretti, M. C., et al. (1998). IL-12 acts directly on DC to promote nuclear localization of NF-κB and primes DC for IL-12 production. Immunity 9, 315–323. doi: 10.1016/S1074-7613(00)80614-7
Ha, S.-J., Park, S.-H., Kim, H.-J., Kim, S.-C., Kang, H.-J., Lee, E.-G., et al. (2006). Enhanced immunogenicity and protective efficacy with the use of interleukin-12-encapsulated microspheres plus AS01B in tuberculosis subunit vaccination. Infect. Immun. 74, 4954–4959. doi: 10.1128/IAI.01781-05
Hamza, T., Barnett, J. B., and Li, B. (2010). Interleukin 12 a key immunoregulatory cytokine in infection applications. Int. J. Mol. Sci. 11, 789–806. doi: 10.3390/ijms11030789
Hanlon, L., Argyle, D., Bain, D., Nicolson, L., Dunham, S., Golder, M. C., et al. (2001). Feline leukemia virus DNA vaccine efficacy is enhanced by coadministration with interleukin-12 (IL-12) and IL-18 expression vectors. J. Virol. 75, 8424–8433. doi: 10.1128/JVI.75.18.8424-8433.2001
Hatherill, M., White, R. G., and Hawn, T. R. (2020). Clinical development of new TB vaccines: recent advances and next steps. Front. Microbiol. 10:3154. doi: 10.3389/fmicb.2019.03154
Hölscher, C., Atkinson, R. A., Arendse, B., Brown, N., Myburgh, E., Alber, G., et al. (2001). A protective and agonistic function of IL-12p40 in mycobacterial infection. J. Immunol. 167, 6957–6966. doi: 10.4049/jimmunol.167.12.6957
Kalams, S. A., Parker, S. D., Elizaga, M., Metch, B., Edupuganti, S., Hural, J., et al. (2013). Safety and comparative immunogenicity of an HIV-1 DNA vaccine in combination with plasmid interleukin 12 and impact of intramuscular electroporation for delivery. J. Infect. Dis. 208, 818–829. doi: 10.1093/infdis/jit236
Kim, J. J., Trivedi, N. N., Nottingham, L. K., Morrison, L., Tsai, A., Hu, Y., et al. (1998). Modulation of amplitude and direction of in vivo immune responses by co-administration of cytokine gene expression cassettes with DNA immunogens. Eur. J. Immunol. 28, 1089–1103. doi: 10.1002/SICI1521-414119980328:03<1089::AID-IMMU1089>3.0.CO;2-L
Maeto, C., Rodríguez, A. M., Holgado, M. P., Falivene, J., and Gherardi, M. M. (2014). Novel mucosal DNA-MVA HIV vaccination in which DNA-IL-12 plus cholera toxin B subunit (CTB) cooperates to enhance cellular systemic and mucosal genital tract immunity. PLoS ONE 9:e107524. doi: 10.1371/journal.pone.0107524
Maeto, C. A. (2015). Optimización de un esquema de inmunización de mucosas frente al HIV basado en la combinación de los vectores ADN y del virus Vaccinia Ankara Modificado (MVA) (ADN/MVA): ADN-IL-12 junto con la subunidad B de la toxina colérica (CTB) cooperan en el incremento de la respuesta celular a nivel sistémico y de la mucosa del tracto genita. Buenos Aires.
Mangtani, P., Abubakar, I., Ariti, C., Beynon, R., Pimpin, L., Fine, P. E., et al. (2014). Protection by BCG vaccine against tuberculosis: a systematic review of randomized controlled trials. Clin. Infect. Dis. 58, 470–480. doi: 10.1093/cid/cit790
Matsui, M., Moriya, O., and Akatsuka, T. (2003). Enhanced induction of hepatitis C virus-specific cytotoxic T lymphocytes and protective efficacy in mice by DNA vaccination followed by adenovirus boosting in combination with the interleukin-12 expression plasmid. Vaccine 21, 1629–1639. doi: 10.1016/S0264-410X(02)00704-1
McKnight, A. J., Zimmer, G. J., Fogelman, I., Wolf, S. F., and Abbas, A. K. (1994). Effects of IL-12 on helper T cell-dependent immune responses in vivo. J. Immunol. 152, 2172–2179.
McShane, H., Behboudi, S., Goonetilleke, N., Brookes, R., and Hill, A. V. S. (2002). Protective Immunity against Mycobacterium tuberculosis induced by dendritic cells pulsed with both CD8+- and CD4+-T-cell epitopes from antigen 85A. Infect. Immun. 70, 1623–1626. doi: 10.1128/IAI.70.3.1623-1626.2002
Metzger, D. W., Buchanan, J. M., Collins, J. T., Lester, T. L., Murray, K. S., Cleave, V. H. V., et al. (1996). Enhancement of humoral immunity by interleukin-12. Ann. N. Y. Acad. Sci. 795, 100–115. doi: 10.1111/j.1749-6632.1996.tb52659.x
Moore, A. C., Kong, W., Chakrabarti, B. K., and Nabel, G. J. (2002). Effects of antigen and genetic adjuvants on immune responses to human immunodeficiency virus DNA vaccines in mice. J. Virol. 76, 243–250. doi: 10.1128/JVI.76.1.243-250.2002
National Research Council (2011). Guide for the Care and Use of Laboratory Animals. 8th Edn, Washington, DC: The National Academies Press.
Ndiaye, B. P., Thienemann, F., Ota, M., Landry, B. S., Camara, M., Dièye, S., et al. (2015). Safety, immunogenicity, and efficacy of the candidate tuberculosis vaccine MVA85A in healthy adults infected with HIV-1: a randomised, placebo-controlled, phase 2 trial. Lancet Respir. Med. 3, 190–200. doi: 10.1016/S2213-2600(15)00037-5
North, R. J., and Jung, Y. J. (2004). Immunity to tuberculosis. Annu Rev Immunol 22, 599–623. doi: 10.1146/annurev.immunol.22.012703.104635
Nyendak, M., Swarbrick, G. M., Duncan, A., Cansler, M., Huff, E. W., Hokey, D., et al. (2016). Adenovirally-induced polyfunctional T cells do not necessarily recognize the infected target: lessons from a phase I trial of the AERAS-402 vaccine. Sci. Rep. 6:36355. doi: 10.1038/srep36355
Ohteki, T., Fukao, T., Suzue, K., Maki, C., Ito, M., Nakamura, M., et al. (1999). Interleukin 12-dependent interferon gamma production by CD8alpha+ lymphoid dendritic cells. J. Exp. Med. 189, 1981–1986. doi: 10.1084/jem.189.12.1981
Okada, E., Sasaki, S., Ishii, N., Aoki, I., Yasuda, T., Nishioka, K., et al. (1997). Intranasal immunization of a DNA vaccine with IL-12- and granulocyte-macrophage colony-stimulating factor (GM-CSF)-expressing plasmids in liposomes induces strong mucosal and cell-mediated immune responses against HIV-1 antigens. J. Immunol. 159, 3638–3647.
Pasquinelli, V., Quiroga, M. F., Martinez, G. J., Zorrilla, L. C., Musella, R. M., Bracco, M. M., et al. (2004). Expression of signaling lymphocytic activation molecule-associated protein interrupts IFN-gamma production in human tuberculosis. J. Immunol. 172, 1177–1185. doi: 10.4049/jimmunol.172.2.1177
Pérez, P., Marín, M. Q., Lázaro-Frías, A., Sorzano, C. Ó. S., Gómez, C. E., Esteban, M., et al. (2020). Deletion of vaccinia virus A40R gene improves the immunogenicity of the HIV-1 vaccine candidate MVA-B. Vaccines 8:70. doi: 10.3390/vaccines8010070
Revelli, D. A., Boylan, J. A., and Gherardini, F. C. (2012). A non-invasive intratracheal inoculation method for the study of pulmonary melioidosis. Front. Cell. Infect. Microbiol. 2:164. doi: 10.3389/fcimb.2012.00164
Rodríguez, A. M., Pascutti, M. F., Maeto, C., Falivene, J., Holgado, M. P., Turk, G., et al. (2012). IL-12 and GM-CSF in DNA/MVA immunizations against HIV-1 CRF12_BF Nef induced T-cell responses with an enhanced magnitude, breadth and quality. PLoS ONE 7:e0037801. doi: 10.1371/journal.pone.0037801
Romano, M., D'Souza, S., Adnet, P.-Y., Laali, R., Jurion, F., Palfliet, K., et al. (2006). Priming but not boosting with plasmid DNA encoding mycolyl-transferase Ag85A from Mycobacterium tuberculosis increases the survival time of Mycobacterium bovis BCG vaccinated mice against low dose intravenous challenge with M. tuberculosis H37Rv. Vaccine 24, 3353–3364. doi: 10.1016/j.vaccine.2005.12.066
Sakai, S., Kauffman, K. D., Sallin, M. A., Sharpe, A. H., Young, H. A., Ganusov, V. V., et al. (2016). CD4 T cell-derived IFN-γ plays a minimal role in control of pulmonary Mycobacterium tuberculosis infection and must be actively repressed by PD-1 to prevent lethal disease. PLoS Pathog. 12:e1005667. doi: 10.1371/journal.ppat.1005667
Sterne, J. A. C., Rodrigues, L. C., and Guedes, I. N. (1998). Does the efficacy of BCG decline with time since vaccination? Int. J. Tuberc. Lung Dis. 2, 200–207.
Sun, L., Yuan, Q., Xu, T., Yao, L., Feng, J., Ma, J., et al. (2017). Novel adjuvant for immunization against tuberculosis: DNA vaccine expressing Mycobacterium tuberculosis antigen 85A and interleukin-15 fusion product elicits strong immune responses in mice. Biotechnol. Lett. 39, 1159–1166. doi: 10.1007/s10529-017-2342-1
Tameris, M. D., Hatherill, M., Landry, B. S., Scriba, T. J., Snowden, M. A., Lockhart, S., et al. (2013). Safety and efficacy of MVA85A, a new tuberculosis vaccine, in infants previously vaccinated with BCG: a randomised, placebo-controlled phase 2b trial. Lancet 381, 1021–1028. doi: 10.1016/S0140-6736(13)60177-4
Tapia, E., Pérez-Jiménez, E., López-Fuertes, L., Gonzalo, R., Gherardi, M. M., and Esteban, M. (2003). The combination of DNA vectors expressing IL-12 + IL-18 elicits high protective immune response against cutaneous leishmaniasis after priming with DNA-p36/LACK and the cytokines, followed by a booster with a vaccinia virus recombinant expressing p36/LACK. Microbes Infect. 5, 73–84. doi: 10.1016/S1286-4579(02)00077-1
Tateosian, N. L., Pellegrini, J. M., Amiano, N. O., Rolandelli, A., Casco, N., Palmero, D. J., et al. (2017). IL17A augments autophagy in Mycobacterium tuberculosis-infected monocytes from patients with active tuberculosis in association with the severity of the disease. Autophagy 13, 1191–1204. doi: 10.1080/15548627.2017.1320636
Tatsumi, T., Takehara, T., Kanto, T., Miyagi, T., Kuzushita, N., Sugimoto, Y., et al. (2001). Administration of interleukin-12 enhances the therapeutic efficacy of dendritic cell-based tumor vaccines in mouse hepatocellular carcinoma. Cancer Res. 61, 7563–7567.
Tchilian, E. Z., Desel, C., Forbes, E. K., Bandermann, S., Sander, C. R., Hill, A. V. S., et al. (2009). Immunogenicity and protective efficacy of prime-boost regimens with recombinant (delta)ureC hly+ Mycobacterium bovis BCG and modified vaccinia virus ankara expressing M. tuberculosis antigen 85A against murine tuberculosis. Infect. Immun. 77, 622–631. doi: 10.1128/IAI.00685-08
Trinchieri, G. (2003). Interleukin-12 and the regulation of innate resistance and adaptive immunity. Nat. Rev. Immunol. 3, 133–146. doi: 10.1038/nri1001
Tsuji, T., Hamajima, K., Fukushima, J., Xin, K. Q., Ishii, N., Aoki, I., et al. (1997). Enhancement of cell-mediated immunity against HIV-1 induced by coinnoculation of plasmid-encoded HIV-1 antigen with plasmid expressing IL-12. J. Immunol. 158, 4008–4013.
World Health Organization (2019). Global Tuberculosis Report 2019. Geneva. Available online at: https://apps.who.int/iris/bitstream/handle/10665/329368/9789241565714-eng.pdf?ua=1 (accessed June 19, 2020).
Wu, M., Zhao, H., Li, M., Yue, Y., Xiong, S., and Xu, W. (2017). Intranasal vaccination with mannosylated chitosan formulated DNA vaccine enables robust IgA and cellular response induction in the lungs of mice and improves protection against pulmonary mycobacterial challenge. Front. Cell. Infect. Microbiol. 7:445. doi: 10.3389/fcimb.2017.00445
Yoshida, S., Tanaka, T., Kita, Y., Kuwayama, S., Kanamaru, N., Muraki, Y., et al. (2006). DNA vaccine using hemagglutinating virus of Japan-liposome encapsulating combination encoding mycobacterial heat shock protein 65 and interleukin-12 confers protection against Mycobacterium tuberculosis by T cell activation. Vaccine 24, 1191–1204. doi: 10.1016/j.vaccine.2005.08.103
Keywords: Ag85A, DNA vaccine, IL-12, MVA, tuberculosis, vaccine
Citation: Morelli MP, Del Medico Zajac MP, Pellegrini JM, Amiano NO, Tateosian NL, Calamante G, Gherardi MM and García VE (2020) IL-12 DNA Displays Efficient Adjuvant Effects Improving Immunogenicity of Ag85A in DNA Prime/MVA Boost Immunizations. Front. Cell. Infect. Microbiol. 10:581812. doi: 10.3389/fcimb.2020.581812
Received: 09 July 2020; Accepted: 18 August 2020;
Published: 23 September 2020.
Edited by:
Natarajaseenivasan Kalimuthusamy, Bharathidasan University, IndiaReviewed by:
Zhidong Hu, Fudan University, ChinaCopyright © 2020 Morelli, Del Medico Zajac, Pellegrini, Amiano, Tateosian, Calamante, Gherardi and García. This is an open-access article distributed under the terms of the Creative Commons Attribution License (CC BY). The use, distribution or reproduction in other forums is permitted, provided the original author(s) and the copyright owner(s) are credited and that the original publication in this journal is cited, in accordance with accepted academic practice. No use, distribution or reproduction is permitted which does not comply with these terms.
*Correspondence: Verónica Edith García, dmdhcmNpYUBxYi5mY2VuLnViYS5hcg==
Disclaimer: All claims expressed in this article are solely those of the authors and do not necessarily represent those of their affiliated organizations, or those of the publisher, the editors and the reviewers. Any product that may be evaluated in this article or claim that may be made by its manufacturer is not guaranteed or endorsed by the publisher.
Research integrity at Frontiers
Learn more about the work of our research integrity team to safeguard the quality of each article we publish.