- 1Molecular Biomedicine Department, Centro de Investigación y de Estudios Avanzados del Instituto Politécnico Nacional, Mexico City, Mexico
- 2Infectious Diseases Research Unit, Hospital General O'Horan, Mérida, Mexico
- 3Department of Epidemiology and Biostatistics, Michigan State University, Lansing, MI, United States
- 4Centro de Ciencias Genómicas, Universidad Nacional Autónoma de México, Cuernavaca, Mexico
- 5Department of Pediatrics, University of Virginia, Charlottesville, VI, United States
Diarrheagenic E. coli can be separated into six distinct pathotypes, with enteroaggregative (EAEC) and diffusely-adherent E. coli (DAEC) among the least characterized. To gain additional insights into these two pathotypes we performed whole genome sequencing of ten DAEC, nine EAEC strains, isolated from Mexican children with diarrhea, and one EAEC plus one commensal E. coli strains isolated from an adult with diarrhea and a healthy child, respectively. These genome sequences were compared to 85 E. coli genomes available in public databases. The EAEC and DAEC strains segregated into multiple different clades; however, six clades were heavily or exclusively comprised of EAEC and DAEC strains, suggesting a phylogenetic relationship between these two pathotypes. EAEC strains harbored the typical virulence factors under control of the activator AggR, but also several toxins, bacteriocins, and other virulence factors. DAEC strains harbored several iron-scavenging systems, toxins, adhesins, and complement resistance or Immune system evasion factors that suggest a pathogenic paradigm for this poorly understood pathotype. Several virulence factors for both EAEC and DAEC were associated with clinical presentations, not only suggesting the importance of these factors, but also potentially indicating opportunities for intervention. Our studies provide new insights into two distinct but related diarrheagenic organisms.
Introduction
Worldwide, diarrheal disease remains a major cause of morbidity and is the eighth leading cause of mortality, among children under 5 years of age from less developed regions of the world (GBD 2016 Diarrhoeal Disease Collaborators, 2018). Diarrheagenic Escherichia coli pathotypes (DEP) are important etiological agents of acute and persistent diarrhea in children (Ochoa et al., 2009; Patzi-Vargas et al., 2015; Zhou et al., 2018), that can lead to death (Lanata et al., 2013; GBD 2016 Diarrhoeal Disease Collaborators, 2018), and cause traveler's diarrhea in adults (Paredes-Paredes et al., 2011; Jennings et al., 2017). Recent studies by our group have shown that DEP have become the main etiologic agents of diarrheal disease among children requiring hospitalization; DAEC and EAEC are the most prevalent pathotypes, surpassing Salmonella and Shigella (Patzi-Vargas et al., 2015).
DEP are classified into six well-described categories: shiga toxin producing E. coli (STEC), which encompasses the enterohemorrhagic E. coli group (EHEC); enterotoxigenic E. coli (ETEC); enteroaggregative E. coli (EAEC); enteroinvasive E. coli (EIEC); diffusely adherent E. coli (DAEC); and enteropathogenic E. coli (EPEC). The latter is subdivided into typical EPEC (tEPEC) and atypical EPEC (aEPEC) (Kaper et al., 2004). Overall, pathogenic E. coli use adhesins, sugars (capsular polysaccharides and lipopolysaccharides), toxins, invasion-promoting proteins, iron acquisition systems, complement resistance factors or immune evasion factors, to colonize, survive in, and injure their hosts (Kaper et al., 2004; Nataro, 2005; Meza-Segura and Estrada-Garcia, 2016). EAEC strains are characterized by their ability to form a stacked-brick or aggregative adherence (AA) pattern on HEp-2 cells, which is mediated by the aggregative adherence fimbriae (AAF) (Kaper et al., 2004). AAF transcription and that of at least other 44 EAEC chromosomal (aaiC) and plasmid-borne genes (the anti-aggregative secreted protein dispersin), are regulated by the Aggregative Adherence Regulator (AggR) (Nataro et al., 1994; Estrada-Garcia and Navarro-Garcia, 2012).
Due to the importance of AggR, EAEC isolates were subdivided into typical (tEAEC), i.e., aagR-positive, and atypical (aEAEC), i.e., aagR-negative, groups (Nataro, 2005). The recognition of tEAEC as strains carrying the aggR regulon was a major step in EAEC molecular identification, since it was shown that most EAEC strains previously characterized by the HEp-2 adherence assay harbored the aggR gene (Cerna et al., 2003). It is noteworthy that tEAEC, as well as other DEP, may also acquire other virulence factors (VF) by horizontal transfer and increase their virulence. In 2011, for example, a hybrid pathogenic EAEC-STEC O104:H4 that was responsible for a massive outbreak of bloody diarrhea in 16 countries, mostly harbored tEAEC (aggA, aggR, set1, pic, and aap) virulence genes and produced shiga toxin 2 (stx2) (World Health Organization, 2018).
Like EAEC, DAEC was classified based on its diffusely adherent (DA) pattern on HEp-2 cells, promoted by Afa, F1845, and Dr adhesins encoded by the afa, daa, and dra operons, respectively. Most of these adhesins have affinity for the human decay accelerating factor (hDAF; also known as CD55) or for carcinoembryonic antigen cell adhesion molecules (hCEACAMs), and both molecules are localized on the surface of intestinal epithelial cells (Servin, 2014; Meza-Segura and Estrada-Garcia, 2016). In contrast with EAEC, DAEC strains, aside from the adhesins, have only been associated with one other VF, the secreted autotransporter toxin (Sat) (Servin, 2014; Meza-Segura and Estrada-Garcia, 2016).
With the exception of tEPEC, aEPEC, STEC, and ETEC strains, the phylogenetic relationship among different DEP remains unclear (Lacher et al., 2007; Hazen et al., 2016; Montero et al., 2019; Rasko et al., 2019).
To date, not a single DAEC genome, and only a few different tEAEC genomes have been sequenced. Whole genome sequencing (WGS) of these pathotypes, in conjunction with previously reported E. coli genomes, will contribute to a greater understanding of the E. coli pan- and core-genomes. The pan-genome comprises the whole repertoire of genes identified in a bacterial species or group, while the core-genome includes the genes shared by all strains that encode for the proteins necessary for basic biological functions. E. coli species are considered to have an infinitely open pan-genome (Rouli et al., 2015), as it includes commensals and several pathogenic groups of strains capable of causing a wide variety of diseases, such as diarrhea, urinary tract infection, sepsis, and neonatal meningitis (Kaper et al., 2004). Therefore, E. coli phylogenetic analysis needs recognize E. coli pathogenic groups (Rasko et al., 2011; Rouli et al., 2015). WGS of DAEC and tEAEC may also help to identify new VF and potential novel pathogenetic mechanisms.
In this study, we sequenced the whole genome of ten DAEC and ten tEAEC strains identified as the sole pathogens isolated from the stools of patients with diarrhea, as well as one commensal E. coli strain isolated from a healthy child. These genomes were analyzed for their phylogenetic association with other E. coli strains and for the presence of genes encoding for VF. The results revealed that DAEC and tEAEC are phylogenetically related. However, strains of the different pathotypes harbor genes encoding for different sets of VF. DAEC carry more genes encoding for iron acquisition factors, while tEAEC harbor genes encoding toxins and bacteriocins. These findings will help to understand the molecular mechanisms that underlie the ability of DAEC and tEAEC to cause disease and will facilitate the identification of virulence gene profiles associated with more virulent strains. Moreover, this study will give insight into the origin and diversification of DAEC and tEAEC in reference to other DEP and ExPEC genomes.
Materials and Methods
Study Population
A 4-year study was conducted from March 2010 to July 2014 on 1,043 children < 10 years of age who sought medical care for acute community-acquired diarrhea at the Hospital O'Horan in Merida, Yucatan. The clinical severity of each diarrheal episode was determined by the Ruuska-Vesikari scoring system (RVSS) (Ruuska and Vesikari, 1990). In addition, children who presented bloody diarrhea but no shock, were classified as moderate diarrhea, and those who presented hypovolemic shock, severe electrolyte imbalance and/or seizures were classified as severe. On admission, a trained nurse obtained demographic and clinical information for each child on a standardized questionnaire. Stools from each child were collected and screened for Escherichia coli, Campylobacter spp., Salmonella sp., Shigella spp., Vibrio spp., and rotavirus, as previously described (Patzi-Vargas et al., 2015).
Ethics Statement
This study was approved by both the Hospital General O'Horan Ethics Committee and the CINVESTAV Committee of Bioethics for Human Research. Legal guardians were required to sign an informed consent form. All children received medical treatment according to the hospital protocols.
Identification of Diarrheagenic E. coli
From each patient, five E. coli like strains were selected. All isolates were both biochemically confirmed as E. coli and screened by two multiplex PCR for DEP genes. The first multiplex PCR identifies the presence of distinctive loci defining ETEC, EPEC, STEC and EIEC (Lopez-Saucedo et al., 2003), while the second multiplex PCR specific genes for DAEC and tEAEC (modified from Patzi-Vargas et al., 2013), including two plasmid-borne (aap and aatA) and one chromosomal (aaiC) EAEC genes (Supplementary Table 1).
DAEC and EAEC Strains Included for the VF -Clinical Severity Study
Thirty-eight DAEC and 30 EAEC strains isolated as the sole pathogen from children with diarrhea were selected for the characterization of 29 VF-encoding genes by four different multiplex PCR; one previously described (Guzman-Hernandez et al., 2016) and three newly developed in this study. The full description of the VF identified by each PCR assay, primers and PCR conditions, are described in Supplementary Table 1.
DAEC and tEAEC Strains Included for WGS
For WGS, ten DAEC and nine tEAEC isolates were selected from those children with the most severe diarrhea who were not malnourished (Table 1). A tEAEC strain isolated as the only enteric pathogen from a 56-year-old woman with acute diarrhea, and a commensal E. coli strain from a healthy 2-year-old girl, both from Mexico City, were included for comparison.
Genomic DNA was extracted by a phenol/chloroform method (Neumann et al., 1992). DNA libraries were prepared using the illumine TrueSeq DNA nano sample preparation kit and sequenced on the Illumina MiSeq platform at CINVESTAV-Langebio to generate tagged paired-end reads of 250 bases in length. Quality control, trimming and filtering of raw sequencing data was performed using Trim Galore v0.4.11. Genomes were assembled with SPAdes v3.9.0 (Nurk et al., 2013; Prjibelski et al., 2014; Vasilinetc et al., 2015), and the accuracy of the assemblies was evaluated with REAPR 1.0.16 (Hunt et al., 2013). Pre-assembled contigs were scaffolded using SSPACE_Standard_v3.0.pl (Boetzer et al., 2011) and GapFiller_v1-10 (Boetzer and Pirovano, 2012).
Phylogenomic Analysis
Phylogenetic trees were constructed using a total of 107 genomes, including the 21 genomes sequenced in this study and 79 publicly available genomes for pathogenic E. coli, six commensal E. coli and one Escherichia fergusonii (Supplementary Table 2). Open reading frames (ORFs) of all genomes were predicted by GeneMark (Besemer and Borodovsky, 2005) and a Maximum Parsimony pan-genome phylogenetic tree was constructed with GetHomologues (Contreras-Moreira and Vinuesa, 2013), based on the translated protein sequences of each genome, using a combination of the orthoMCL and COGS as clustering algorithms. Single-copy genes of the E. coli core-genome were retrieved with a custom Bash/Perl script deposited in Github2 MAFFT 7.3.10 was used to align all sequences (Katoh and Standley, 2013). After alignment all sequences were concatenated using the multigenomes2blocks pipeline (Vera-Ponce de Leon et al., 2020) and the GTR+F+R10 nucleotide substitution model was obtained by ModelFinder (Kalyaanamoorthy et al., 2017). The maximum-likelihood (ML) phylogenetic tree was then calculated by IQtree v1.6.12 (Minh et al., 2020) with 1,000 Bootstrap replicates for internal branch support. Population structure was calculated using a Bayesian analysis approach (BAPS) by RhierBAPS package (Tonkin-Hill et al., 2018) in R 3.6.3. For this, two depth levels and a maximum clustering size of 20 (default = number of isolates/5; 107/5 = 21.4) were specified. Seven different sequence clusters (SC) were identified, which were further divided into 24 lineages (Supplementary Figure 1). All scripts to replicate this experiment are deposited in Github2. Pan- and core-genome phylogenetic trees were visualized and edited using iTOL (Letunic and Bork, 2016).
Identification of ORFs Encoding for Virulence Factors
For this study, we defined VF as molecules that enable a microorganism to establish itself on or within a host of a particular species and enhance its potential to cause disease. A commensal E. coli strain was defined as an isolate from the stool of subjects exhibiting no signs of diarrheal illness and that did not display any known molecular markers that are associated with DEP. The presence of genes encoding for bacterial VF was analyzed by a Protein-Protein BLAST (BLAST 2.2.28+), using the translated protein sequences of all predicted ORFs and the virulence factor database (VFDB) (Liu et al., 2019). VF were annotated when their coverage and identities were ≥ 80%, after BLAST searches. VF were included for the analysis only when all genes or operons involved in their production or secretion were identified (i.e., the Sit iron/manganese transport system was only included when the genes encoding for sitA, sitB, sitC, and sitD were present). Individual VF were grouped together based on their function, including adhesins, bacteriocins, toxins, iron acquisition systems (IAS), and complement resistance or immune system evasion factors (CR/ISEF). The relative frequency (RF) of these groups was calculated by adding together the individual frequency of each VF in that group and dividing the result by the total number of genomes.
Statistical Analysis
All statistical analyses were performed using GraphPad PRISM® version 5.0 software. The incidence of individual VF was evaluated by Fisher's exact test (FET) and the RF of VF groups by Mann-Whitney U-Test (MWUT). P < 0.05 were considered statistically significant.
Data Availability
The genome sequence assemblies generated in this study have been deposited in GenBank as part of the Bioproject PRJNA588567 under the accession numbers listed in Supplementary Table 2. The remaining data that support the findings of this study are available from the corresponding author upon request.
Results and Discusion
Pan-Genome and Core-Genome of E. coli, DAEC, and EAEC
The clinical characteristics of each subject and the general genomic features of their sequenced strains are summarized in Table 1 and Supplementary Table 3, respectively. Almost all children presented with moderate to severe diarrhea (95%) and were < 5 years of age (95%). E. coli genome sizes obtained ranged from 4.9 to 5.5 Mbp, similar to those reported for other DEP genomes (Lukjancenko et al., 2010). To our knowledge, these are the first DAEC genomes to be reported.
In order to establish the phylogenetic relationships among the 21 genomes described in this work, 85 previously sequenced E. coli genomes were used for comparison, including six tEAEC, one aEAEC and ten tEAEC-STEC O104:H4, forty-nine DEP, ten uropathogenic E. coli (UPEC), three neonatal meningitis-causing E. coli (NMEC), six commensal and MG1655 K12 genomes (Supplementary Table 2). E. fergusonni (ATCC® 35469™) (Farmer et al., 1985) was used as an outgroup to root the phylogenetic trees. The comparative genomic analysis revealed a pan-genome containing 25,726 genes, including 1,452 core-genes, of which only 1,144 were single copy. This pan-genome contained more genes than those previously described with a similar number of genomes analyzed, reported with 13,000–16,373 genes (Rasko et al., 2008; Lukjancenko et al., 2010; Kaas et al., 2012). The number of genes included in the core-genome, however, were comparable to previously published studies, in which an average number of 1,631 genes was reported (Rasko et al., 2008; Lukjancenko et al., 2010; Kaas et al., 2012).
Pan- and Core-Genome Phylogenetic Clades Revealed DAEC and EAEC Relatedness
For the pan-genome tree we selected those clades harboring more than one DAEC or tEAEC genomes (I–VII), each of which included some of the sequenced strains (Figure 1). Clade VI contained only one tEAEC strain sequenced in this study, as well as one hybrid EAEC-STEC and one aEAEC reference strain, revealing that these three EAEC groups share a common set of genes.
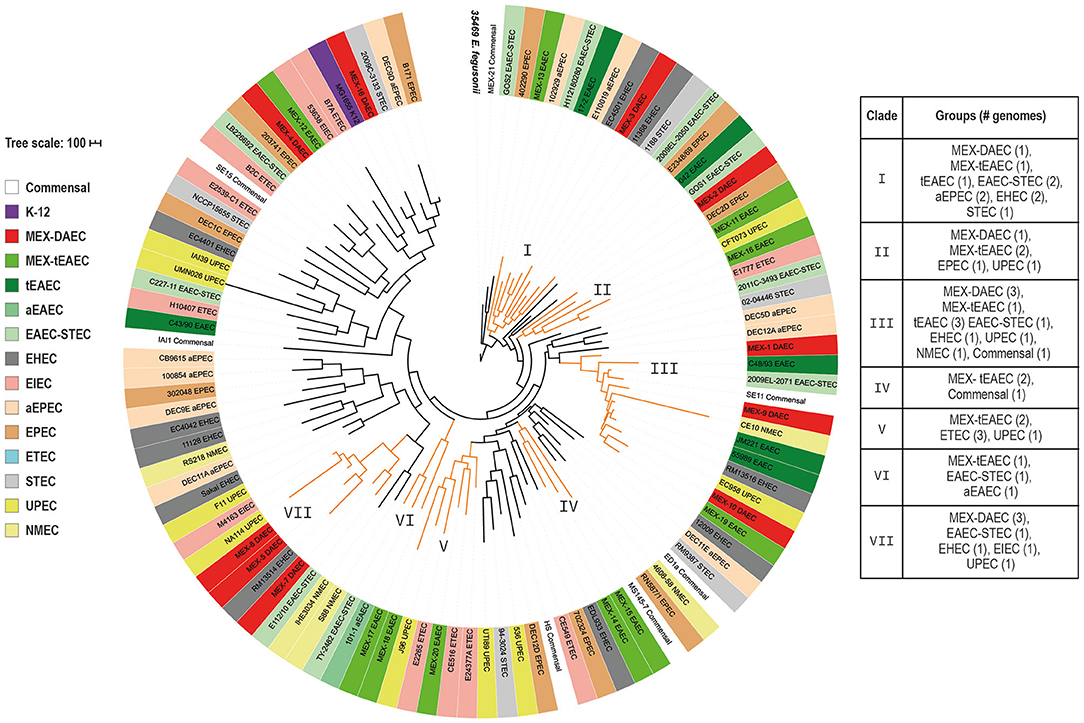
Figure 1. Pan-genome phylogenetic tree. The tree was built up based on the presence or absence of 25,726 genes comprised in the E. coli pan-genome. E. fergusonii 35,469 (in bold) was included to root the tree. Each E. coli group is indicated by a different color listed in the left box. Clades including more than one DAEC or tEAEC genomes are highlighted in orange and the roman numeral indicates the clade number. The scale bar represents the number of differences in the presence or absence of genes among all genomes.
To analyze the evolutionary relationships among DEP we built a core-genome phylogenetic tree, and the population structure was analyzed using a Bayesian approach. All 107 genomes were grouped into seven sequence clusters (SC), which were further divided into 24 lineages (Supplementary Figure 1). Nineteen of these lineages were selected and illustrated in the core-genome tree as clades I-VI and a-m (Figure 2). The tree revealed six different phylogenetic origins for each DAEC and tEAEC pathotypes. Even though these two DEP were outnumbered by other E. coli genomes, clades I–VI mostly comprised DAEC or tEAEC strains. Clades I and IV exclusively contained DAEC and tEAEC genomes. Additionally, clade V, which mostly harbored these two pathotypes (two DAEC and nine tEAEC), also contained one aEPEC, one commensal and K12 strains. Altogether, this results strongly suggest that DAEC and tEAEC are phylogenetically related. Of note, nine of the 14 genomes (64.3%) included in clade V were isolated from Mexican children, suggesting a geographical cluster: two MEX-DAEC, five MEX-tEAEC, the commensal MEX-21 and the JM221 tEAEC reference strain, which was isolated from a Mexican child with diarrhea in the 1980s (Mathewson et al., 1987). Clade VI solely contained tEAEC and EAEC-STEC O104:H4 genomes, confirming that EAEC-STEC O104:H4 is more closely related to tEAEC than to EHEC 0157:H7 (Rasko et al., 2011).
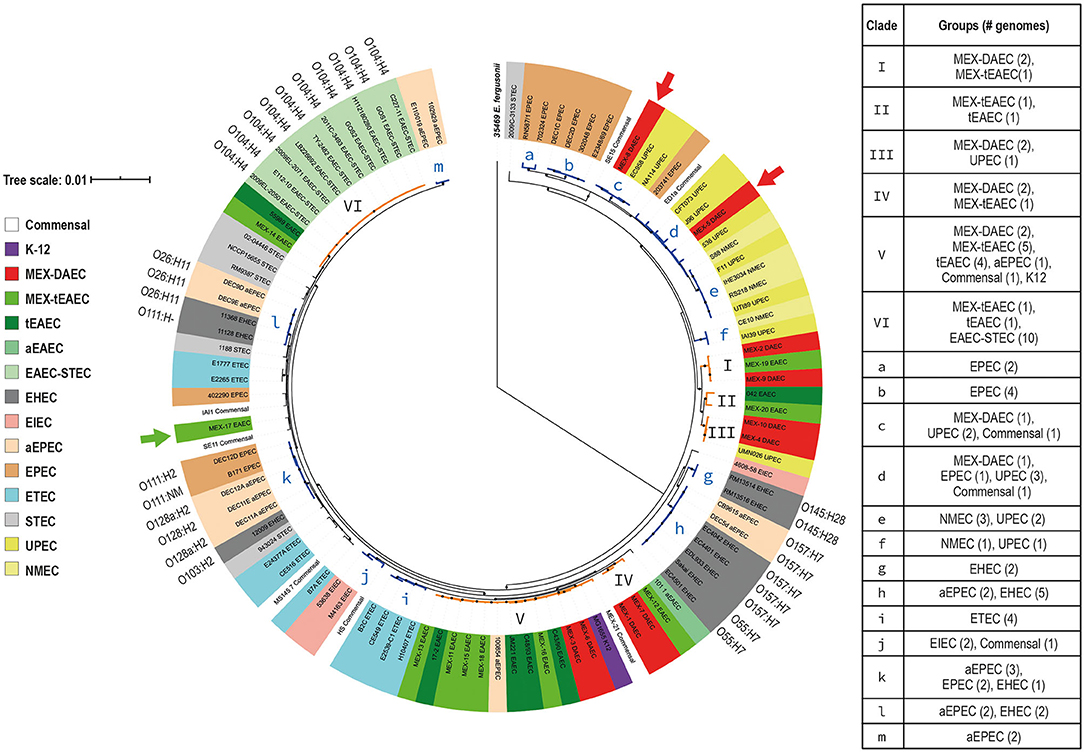
Figure 2. Core-genome (GTR + F + R10) phylogenetic tree. A maximum likelihood tree was constructed based on 1,144 single copy genes from the E. coli core-genome. E. fergusonii 35,469 (in bold) was used as an out-group. The tree was then calculated by IQtree v1.6.12 (Minh et al., 2020) with 1,000 Bootstrap replicates for internal branch support and values over 70% are indicated at each node with a black circle. Population structure was calculated using a Bayesian approach by RhierBAPS package (Tonkin-Hill et al., 2018) in R 3.6.3. E. coli groups are indicated by different color labels listed in the left chart. Clades including more than one DAEC or tEAEC genomes are highlighted in orange and the roman numerals in front of them indicate the clade number. Two DAEC (red arrows) and one tEAEC (green arrow) genomes were located outside these clades. Clades highlighted in blue (a–m) contain other pathogenic E. coli genomes that had important associations. The scale bar represents the number of nucleotide substitutions per site.
The core-genome tree also revealed that DAEC clustered together with UPEC strains (clades III, c, and d). Clade III and c harbored exclusively DAEC and UPEC genomes, whereas clade d also included an aEPEC and a commensal strain. This was not unexpected, given that UPEC strains carrying afa/daa/dra operons have been identified among UTI patients (Servin, 2014).
Additionally, the core-genome tree revealed associations between other E. coli groups (clades a, b, e–m). Clades a and b encompassed typical EPEC strains that resemble previously described EPEC9 and EPEC1 phylogenomic lineages, respectively, whereas clade m belongs to the B1 phylogroup and comprises two aEPEC strains, including the prototype E110019 strain (Lacher et al., 2007; Hazen et al., 2016). Moreover, clade h supports the notion that EHEC O157:H7 evolved from O55:H7 aEPEC (Zhou et al., 2010). Clade k harbors EPEC, aEPEC and EHEC genomes, similar to EPEC2 phylogenomic lineage (Lacher et al., 2007; Hazen et al., 2016). Phylogenetic relatedness was also observed between aEPEC and EHEC strains belonging to the O26:H11 serotype (clade L); these strains undergo transient interconversions via loss and gain of Stx-encoding phages (Bielaszewska et al., 2007).
Of interest, four ETEC (40%) and two EIEC (66.6%) genomes formed clades i and j, respectively, suggesting a specific phylogenetic origin for each pathotype (Rasko et al., 2019). In addition, clades e and f, containing NMEC and UPEC strains, revealed that these ExPEC groups are phylogenetically related. The fact that commensal E. coli genomes were widely distributed and clustered with strains from different pathogenic groups, confirms the great diversity of E. coli genomes (Figure 2).
Analysis of DAEC and tEAEC VF by Function Reveals Different and Novel Pathogenic Mechanisms
In order to identify new DAEC and tEAEC pathogenic mechanisms, virulence factors were grouped by function: adhesins, bacteriocins, toxins, iron acquisition factors (IAF), complement resistance or Immune system evasion factors (CR/ISEF), and bacterial secretion systems. With the exception of adhesins, all genes identified among these groups are mentioned in Table 2. In addition, the genes encoding for the following 14 adhesins were identified: antigen 43 (Agn43), calcium-binding antigen 43 homolog (Cah), curli, EaeH surface protein, E. coli common pilus (ECP), EHEC autotransporter encoding genes A (EhaA) and B (EhaB), E. coli YcbQ laminin-binding fimbriae (Elf/Ycb), pyelonephritis-associated pilus (Pap), Stg fimbria, toxigenic invasion loci A/haemagglutinin from E. coli K1 (Tia/Hek), type 1 fimbria (TIF), trimeric autotransporter adhesin UpaG, and autotransporter adhesin UpaH. Most of these adhesins have been implicated in adhesion to epithelial cells, autoaggregation and biofilm formation. The relative frequency (RF) of adhesins was similar among DAEC (RF 7.4), tEAEC (RF 7.5), and commensal (RF 7.0) E. coli strains, revealing that adherence is a key event for initiating E. coli commensal and pathogen colonization, and that its success relies on multiple adhesins that recognize receptors on the intestinal and bladder epithelium (Kaper et al., 2004; Flores-Mireles et al., 2015).
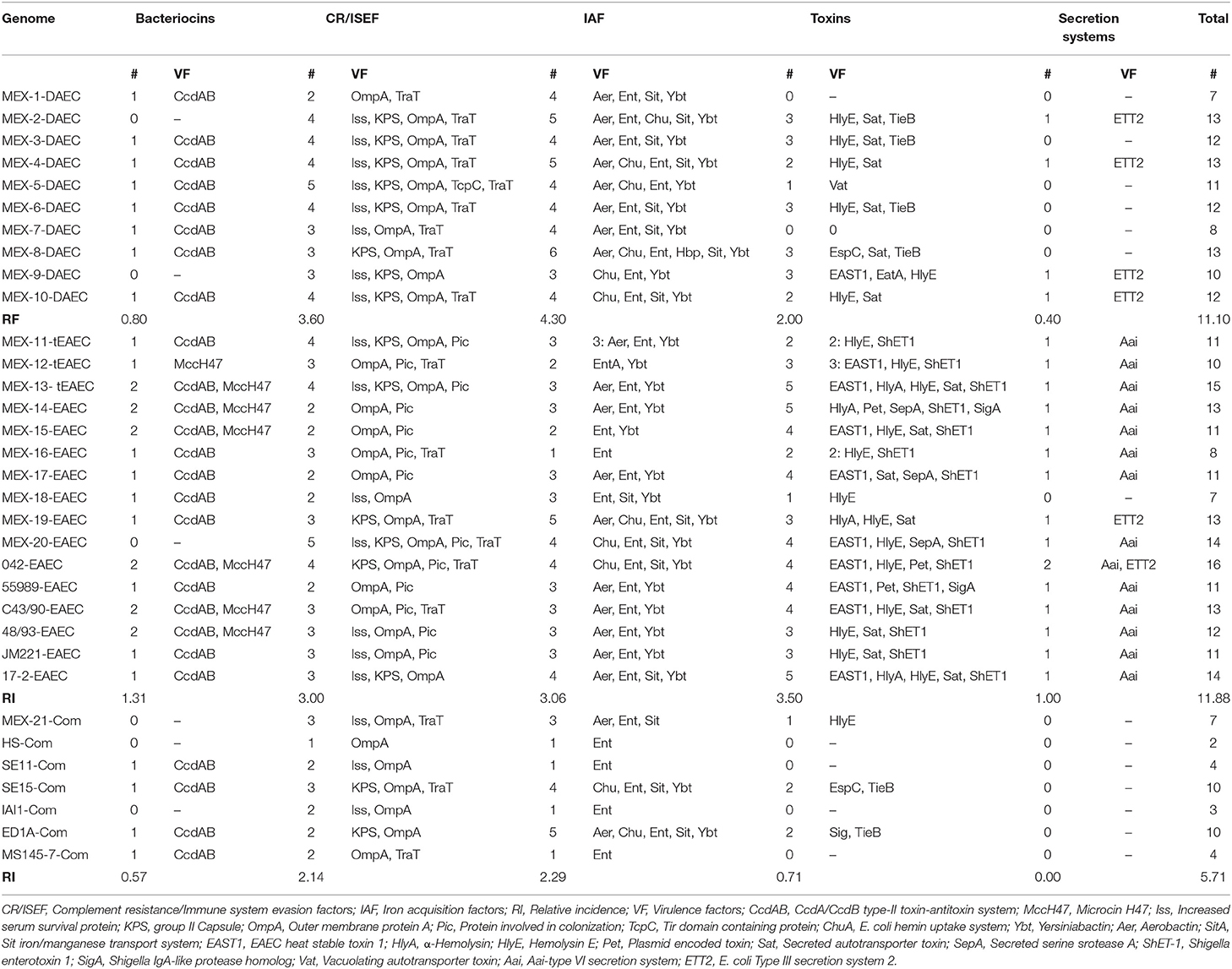
Table 2. Distribution of groups of virulence factors among DAEC, EAEC, and commensal E. coli genomes.
Since the information on DAEC VF is scarce, it was interesting to find that DAEC harbored significantly more IAF than both tEAEC (4.30 vs. 3.06 p = 0.0023) and commensal E. coli (4.30 vs. 2.29 p = 0.0256), suggesting that IAF may play an important role in DAEC virulence (Table 2). Iron is essential for E. coli survival, facilitating numerous essential cellular activities, such as peroxide reduction, electron transport, and nucleotide biosynthesis (Gao et al., 2012). In other pathogenic E. coli groups, such as UPEC, IAS play an important role in virulence in vivo (Torres et al., 2001; Flores-Mireles et al., 2015). Shigella has also evolved multiple strategies for sequestering iron and other elements from the host (Wei and Murphy, 2016).
As shown in Table 2, tEAEC carried significantly more bacteriocins than DAEC (p = 0.0005) and commensal E. coli (p = 0.0167). tEAEC genomes were the only ones to harbor the Microcin H47 (43%) system. Microcin H47 was first found in the non-pathogenic, probiotic Nissle 1917 E. coli strain and is highly prevalent among ExPEC strains (Micenkova et al., 2014). It exhibits antibacterial activity against enteric pathogens such as Adherent Invasive E. coli (AIEC) and S. enterica serovar Typhimurium (Sassone-Corsi et al., 2016; Palmer et al., 2018). It is plausible that DAEC, and particularly tEAEC, use bacteriocins to eliminate competing bacteria in the gut, including other pathogenic species. Likewise, tEAEC had significantly more toxins (Figure 2) than DAEC (3.50 vs. 2.0, p = 0.007) and commensal E. coli (3.50 vs. 0.71, p = 0.0321), providing evidence that toxins play an important role in tEAEC virulence (Estrada-Garcia and Navarro-Garcia, 2012). DAEC had significantly more toxins than commensal strains (2.00 vs. 071, P = 0.0418), thus maybe toxins play a role in DAEC pathogenesis as well.
Two secretion systems were identified among the sequenced genomes: (1) the E. coli Type III secretion system 2 (ETT2), which was first discovered through the genome analysis of enterohemorrhagic E. coli O157:H7 (Ren et al., 2004), and (2) the tEAEC Aai type six secretion system (T6SS), which is regulated by AggR and its function is as yet unknown (Dudley et al., 2006).
ETT2 was more prevalent in DAEC (40%) than in tEAEC (12.5%) and was absent in all commensal strains (Table 2). With exception of MEX-20 tEAEC genome, the whole ETT2 gene cluster was identified in all strains of clades I, II, III, g and h. It has been reported that ETT2 participates in the invasion of endothelial cells by NMEC strain EC10 (Yao et al., 2009) and may have functional importance in infection among human pathogenic E. coli strains isolated from blood samples (Fox et al., 2020). Aai-T6SS was only identified among tEAEC genomes (P < 0.0001). T6SS was initially associated with bacterial virulence in eukaryotic host cells, but currently the main role of T6SS is thought to involve bacterial competition via killing of neighboring bacteria by the injection of antibacterial proteins directly into their periplasm after cell-cell contact (Navarro-Garcia et al., 2019).
CR/ISEF were significantly more prevalent in DAEC (RF 3.60 vs. 2.14, P = 0.0043) and tEAEC (RF 3.00 vs. 2.14, P = 0.0008) than among commensal strains, but there was no difference between these two pathotypes. CR/ISEF may play an essential role in DAEC pathogenesis, since the initial binding of Afa/F1845/Dr adhesins to hDAF could potentially result in a decreased inactivation of the complement cascade, thus production of CR/ISEF could protect DAEC from the action of complement (Duan and Mukherjee, 2016; Meza-Segura and Estrada-Garcia, 2016).
Novel and Common VF Genes Found Among DAEC and tEAEC Strains
Because very little is known about DAEC VF this section highlights the new VF genes identified for this pathotype. We also describe common tEAEC and commensal VF genes (Table 3). When compared to tEAEC, Cah was highly prevalent among DAEC genomes (90% vs. 37.5%, p = 0.0143), and was absent from commensal bacteria (Table 3). cah has been described to be widespread among STEC and has only been identified in one commensal E. coli strain (Torres et al., 2002; Montero et al., 2014; Carter et al., 2018). Cah shares high sequence similarity with AIDA-1, an adhesin that mediates DAEC diffuse adherence pattern to HeLa cells, and with Antigen 43. In E. coli O157:H7, Cah is known to be involved in autoaggregation and biofilm formation (Carter et al., 2018). The gene encoding for the enterotoxin TieB (senB) was also significantly more prevalent in DAEC, since it was absent in tEAEC genomes (p = 0.0140, Table 3); there was no significant difference between DAEC and commensal E. coli genomes in the frequency of senB. TieB is an enterotoxin that was initially described in EIEC (Nataro et al., 1995). Additionally, UPEC strains carrying senB have been associated with both pyelonephritis and urosepsis, suggesting that TieB may play a role in UPEC virulence in humans (Mao et al., 2012).
Similarly, the gene encoding for the plasmid-encoded outer membrane protein TraT was more prevalent in DAEC than in tEAEC (90 vs. 37.5%, P = 0.0143). TraT is a complement-resistance molecule that is believed to enhance E. coli serum resistance by inhibiting later stages of the membrane attack complex activity or by altering C3 deposition on the bacterial surface and affecting outer membrane permeability (Miajlovic and Smith, 2014). traT was detected in a significantly higher proportion of E. coli strains isolated from patients with bacteraemia/septicaemia and enteric infections when compared to E. coli strains isolated from healthy subjects (Montenegro et al., 1985).
IAS is the most prevalent functional group associated with DAEC and we identified two genes that were significantly more prevalent in DAEC than in tEAEC genomes: Chu, which binds host hemoproteins (60 vs. 18.8%, p = 0.0461), and Sit iron/manganese transport system, for the utilization of ferric iron (80 vs. 31.25%, p = 0.0414) (Table 3). Mice infected with chuA-inactivated UPEC strains resulted in attenuation of UPEC virulence in vivo, indicating that this heme receptor is a VF in UPEC (Torres et al., 2001). Sit has been described as the sole iron-uptake system in S. flexneri that allows this strain to survive and form plaques in a monolayer of eukaryotic cells, suggesting a direct role in S. flexneri pathogenesis (Runyen-Janecky et al., 2003). Furthermore, in S. enterica serovar Typhimurium the Sit system transports manganese (Kehres et al., 2002).
In contrast with DAEC, toxins play an important role in tEAEC virulence (Estrada-Garcia and Navarro-Garcia, 2012). As shown in Table 3, ShET1 (81%) and HlyA (25%) were only identified among tEAEC genomes, as both toxins play a role in tEAEC pathogenesis. ShET1 is an oligomeric toxin that induces intestinal secretion via intracellular increase of cAMP and cGMP (Fasano et al., 1997). Sat toxin, previously reported to be significantly associated with DAEC strains (Meza-Segura and Estrada-Garcia, 2016), was similarly distributed among the genomes of both pathotypes (Table 3).
The Protein involved in colonization (Pic) was exclusively found and highly prevalent (81%) in tEAEC. Pic and ShET1 are encoded on the same chromosomal locus, encoded on opposite strands. Pic was first described in tEAEC (Henderson et al., 1999), since then it has been identified in EHEC, EPEC, and UPEC, as well as in other pathogens of the Enterobacteriaceae family (Abreu and Barbosa, 2017). Pic is a secreted autotransporter able to cleave C2, C3/C3b, and C4/C4b, and works synergistically with human Factor I and Factor H (FH), thereby promoting inactivation of C3b. It is thereby considered a CR/ISEF (Abreu and Barbosa, 2017). Also, E. coli Ycb laminin-binding fimbriae (Elf/Ycb) was significantly more frequent among tEAEC than DAEC genomes (87.5 vs. 30%, P = 0085). Elf contributes to the adherence of STEC to human intestinal epithelial cells, and to cow and pig gut tissue in vitro (Samadder et al., 2009). To the best of our knowledge, this is the first time Elf/Ycb has been described among DAEC and tEAEC strains. As expected, the genes encoding for dispersin (aap) and its translocator (aatA) were significantly associated with tEAEC (Patzi-Vargas et al., 2015). Obviously, the presence of these virulence genes should be corroborated among tEAEC and DAEC strains from other regions of the world, to confirm its importance in the pathogenesis of these strains.
Association of DAEC and tEAEC Virulence Genes With Clinical Severity of Diarrheal Disease
The presence of 29 E. coli virulence genes, identified by PCR, among the 38 DAEC and 30 tEAEC strains is shown in Table 4. In agreement with the comparative genome analysis, genes encoding for ChuA and SitA were more prevalent among DAEC isolates, while AaiC (T6SS), AatA, dispersin (aap), EAST1 (astA), MccH47 (mchB) and Pic, among tEAEC isolates. In addition, PCR results revealed for the first time the presence of five genes significantly associated with DAEC strains: iss, kps MII, fyuA, and iutA. On the other hand, hlyA, papC, pet and sepA were significantly associated with tEAEC isolates.
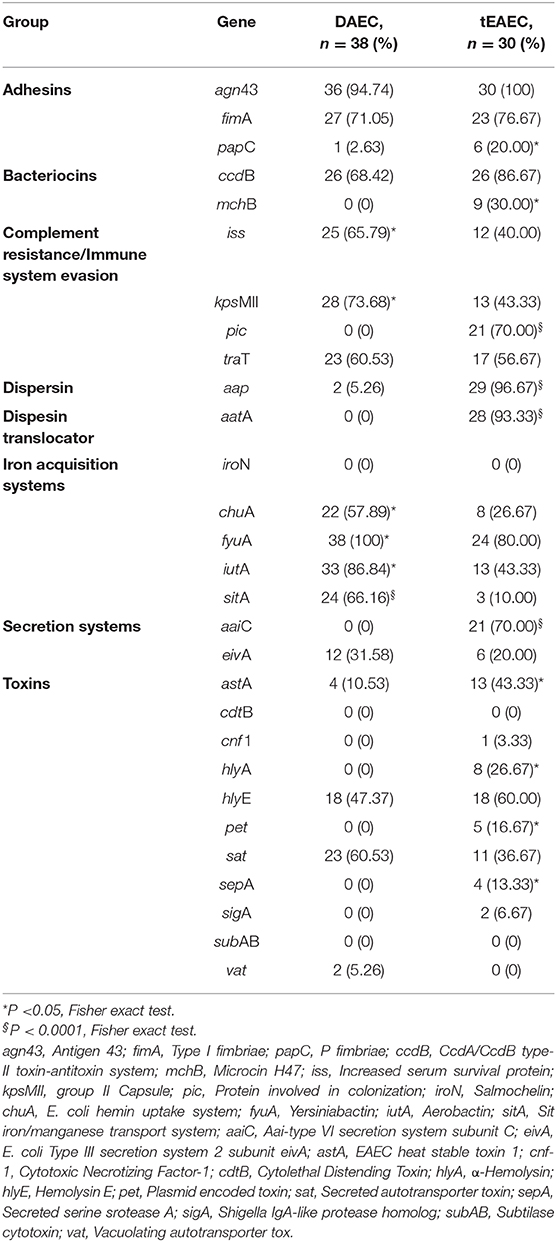
Table 4. Prevalence of 29 selected virulence genes among DAEC and tEAEC strains identified as the only pathogens isolated from children with diarrhea.
DAEC strains harbored significantly more IAS encoding genes, while tEAEC strains harbored more toxin and bacteriocin genes (Figure 3), as previously shown in the ORF genome analysis (Table 2).
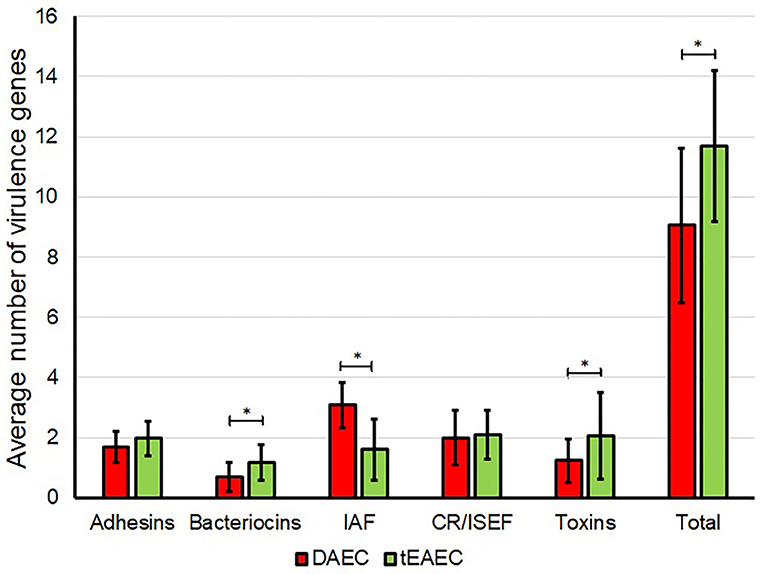
Figure 3. Distribution of virulence genes in DAEC and tEAEC strains. The average number of different groups of virulence genes detected by PCR in DAEC (red) and tEAEC (green) strains are shown. Asterisks indicate a significant difference in the number of virulence genes between both pathotypes (P < 0.05, MWUT). CR/ISEF, Complement resistance/Immune system evasion factors; IAF, Iron acquisition factors.
Of the 38 DAEC strains, 20 (52.6%) had the afaC-ang43-fyuA-iutA-kpsMII-sat-fimA virulence gene profile (VGP), which harbors two genes encoding for IAS yersianiabactin (fyuA) and aerobactin (iutA) siderophore receptors. Both yersiniabactin and aerobactin genes are found more frequently among pathogenic E. coli strains and have been involved in UPEC virulence (Garcia et al., 2011; Flores-Mireles et al., 2015). Yersiniabactin binds copper to protect pathogens during infection; direct mass spectrometroscopy showed that it also binds nickel, cobalt, and chromium, and transports these metals by its receptor FyuA (Koh et al., 2017). Detection of urinary Ybt and serological detection of the outer membrane Ybt importer (fyuA) have been implicated in clinical UTI (Koh et al., 2017). It also appears that the aerobactin system is important in UPEC virulence (Gao et al., 2012). Therefore, the presence of several IAF in DAEC genomes should be advantageous not only for its virulence, but to overcome the host “nutritional immunity” which limits bacterial growth by sequestering metals in the intestine (Lopez and Skaar, 2018). sat, kpsMII, and fimA, also included in this common VGP, belong to the functional groups of toxins, CR/ISEF and adhesins, respectively. In animal models, SAT-producing UPEC and DAEC strains may cause damage to the renal and intestinal epithelium, respectively (Guyer et al., 2002; Taddei et al., 2005). Seventy percent of NMEC strains harbored the kpsMII gene that encodes for the K2 capsule (Wijetunge et al., 2015). K2 provides protection against complement-mediated killing of UPEC CFT073 strain and has been shown to be important for UTI pathogenesis (Buckles et al., 2009). Among UPEC strains it has been shown that type 1 pilus (fimA) participates in the colonization of both the uroepithelium and the intestinal epithelium (Flores-Mireles et al., 2015; Spaulding et al., 2017). Furthermore, the type 1 pilus plays an essential role in ETEC virulence, acting in concert with specific ETEC colonization factors (CF) promoting optimal bacterial adhesion to cultured intestinal epithelium and to epithelial monolayers differentiated from human small intestinal stem cells (Sheikh et al., 2017).
Of the 30 tEAEC strains, aggR-ang43-aap-aatA-aaiC-pic-ccdB was the predominant VGP (56.67%). pic also comprised part of the most prevalent gene profile (set1A-set1B-pic) identified in tEAEC strains isolated from Peruvian children with diarrhea, but not in strains isolated from asymptomatic children, and was also significantly associated with both acute diarrhea and prolonged diarrhea (Duan and Mukherjee, 2016). Pic's role in EAEC pathogenesis is multi-factorial: it is a mucinase, induces intestinal mucus hypersecretion, and is involved in gut colonization and complement inactivation; thus it appears that pic is a potential marker for virulent tEAEC strains (Estrada-Garcia and Navarro-Garcia, 2012; Abreu and Barbosa, 2017). CcdA/CcdB toxin-antitoxin system may increase the probability of tEAEC colonization by inhibiting the growth of competing organisms in the gut. To the best of our knowledge, this is the first study to identify this bacteriocin among tEAEC strains.
Specific DAEC or tEAEC VGP and Adhesins Were Associated With Fever, Age, Hyponatremia, and Disease Severity
We explored associations between the clinical characteristics of the diarrheal episode and specific VGP in DAEC and tEAEC. The agn43-ccdB-fyuA-iutA-kpsMII-sat-sitA-traT DAEC VGP was significantly associated with fever ≥ 38°C (7/12, 58.3% vs. 3/26, 11.5% p = 0.0047). It is unknown whether any of the molecules encoded by these genes directly induces secretion of IL-1, the cytokine that induces fever (Endres et al., 1987). However, we have previously shown that both DAEC reference strain C1845 and a clinical DAEC isolate induce the production of IL-1ß from Caco-2 confluent cells in vitro (Patzi-Vargas et al., 2013). Furthermore, DAEC and tEAEC strains isolated from patients with fever had a higher number of toxin-encoding genes (RF 2.0 vs. 1.3, P = 0.0231) and overall more virulence genes (RF 11.5 vs. 9.43, P = 0.0037) than strains isolated from children without fever.
The aap-aatA-agn43-fimA-fyuA VGP was only found among tEAEC isolates from children aged < 2-years (18/24, 75%, P = 0.0157), while the mchB-hlyE-iutA-kpsMII VGP was exclusive to older children (3/5, 60%, P = 0.0027). It has been suggested that DAEC strains from children and adults constitute two different populations (Mansan-Almeida et al., 2013); this may be the case for tEAEC strains as well. Sat was found to be associated with hyponatremia in tEAEC diarrhea (4/5, 80% vs. 7/25, 28%, p = 0.0472). In a ligated rabbit ileal loop assay, SAT produced a copious amount of fluid similar to that observed with ETEC LT toxin, as well as villous edema, vacuolization and loss of internal villous structure (Taddei et al., 2005), that may result in loss of electrolytes and hyponatremia. In both DAEC and tEAEC strains, fimA (type 1 pilus) was more common in isolates from children ≤ 24 months than in older children (22/27, 81.5% vs. 5/11, 45.45%, P = 0.0471; and 22/24, 91.67% vs. 1/6,16.67%, P = 0.0008; respectively). As observed for ETEC, type 1 pilus may enable more effective bacterial adhesion of DAEC and tEAEC strains to the intestinal epithelium of younger children (Sheikh et al., 2017). tEAEC strains isolated from cases with dehydration signs had a higher number of genes encoding for adhesins (RF 3.43 vs. RF 2.74, P = 0.0126).
Hypothetical DAEC Model of Pathogenesis
Based on the variety of novel genes identified among DAEC genomes, we propose the following model for DAEC pathogenesis (Figure 4). Afa/F1845/Dr adhesins bind to hDAF/CD55 and hCEACAMs, localized on the apical pole of human intestinal epithelial cells, which results in DAEC characteristic diffuse adherence pattern. A novel adhesin, Cah, was found among most DAEC strains, suggesting that it could also participate in the initial adherence of DAEC, in the aggregation of bacteria and in the formation of biofilm, as it has been described before for STEC (Torres et al., 2002; Carter et al., 2018). Also, Cah shares high sequence similarity with AIDA-1, an adhesin that also mediates DAEC diffuse adherence pattern to HeLa cells (Torres et al., 2002). DAEC intrinsically has an effect on the host complement system since it binds hDAF/C55, hampering the capacity of this molecule to inhibit the complement-cascade amplification. The expression of CR/ISEF by DAEC could protect the bacteria from the action of the complement. For example, traT, which inhibits later stages of the membrane attack complex activity, was highly prevalent among DAEC strains. Moreover, these strains also express a wide variety of iron acquisition systems, such as Chu, which binds host hemoproteins, and Sit iron/manganese transport system for the utilization of ferric iron, allowing DAEC to capture these elements that are indispensable for bacterial growth and to replicate faster than resident microbiota. Also, the most common DAEC VGP afaC-ang43-fyuA-iutA-kpsMII-sat-fimA, contained two IAS, the yersianiabactin and aerobactin siderophore receptors.
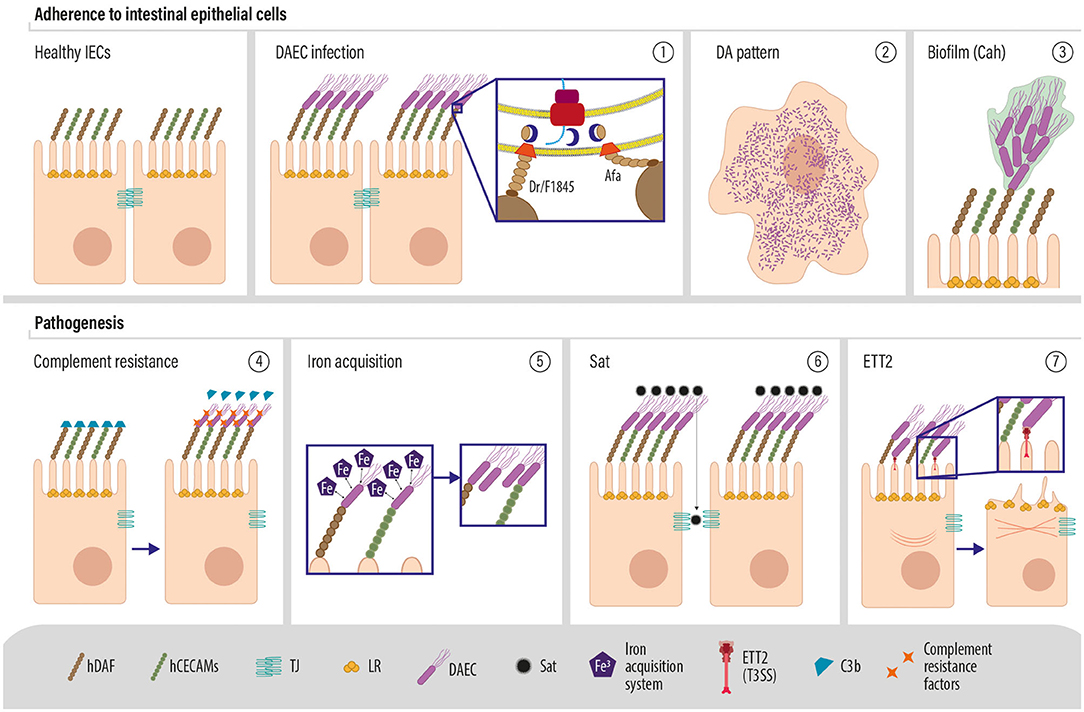
Figure 4. Molecular pathogenesis of DAEC (1). DAEC expresses Afa/F1845/Dr adhesins that interact with the decay accelerating factor (also known as Dr or CD55) and (2) are responsible for the characteristic diffuse adherence (DA) pattern exhibited by this pathotype (3). The production of Cah may assist in the initial adherence to enterocytes or with production of biofilm (3). Adherence of the bacteria may dampen the inhibitory activity of hDAF, which could result in a decreased inactivation of the complement. However, DAEC strains carry a wide variety of complement resistance factors, which might protect the bacteria (4). Iron acquisition systems are common among DAEC, suggesting an important role for iron scavenging in DAEC infection, which may facilitate bacterial replication and colonization (5). The serine protease Sat is also common among DAEC strains and it is associated with the loss of cell shape and potentially detachment from epithelial layers. Finally, the ETT2 type 3 secretion system may deliver intracellular effectors to the enterocytes, which may induce cytoskeletal modifications.
Among DAEC strains, we found the whole gene cluster encoding ETT2, which could be potentially involved in the polymerization of actin observed during DAEC infection (Riveros et al., 2011), as it has been shown for the type three secretion system of EPEC and EHEC (Kaper et al., 2004). Sat, the only previously described DAEC VF, was also identified in the most common DAEC VGP. Sat may be responsible for tight junction alteration and increased secretion of fluids into the lumen during the course of DAEC infection. We identified a gene encoding for a second toxin, TieB, for which a mechanism of action has not yet been described.
Conclusions
Comprehensive WGS and identification of VF for DAEC and tEAEC strains reveal for the first time that these two pathotypes are phylogenetically related. Given this relationship, we hypothesized that the two pathotypes would harbor a shared set of VF (as do EPEC and STEC, for example), supplemented by different factors that mediate their divergent pathogenic lifestyles. This was in fact the case, as most VF were shared between the two pathogens with few exceptions. Moreover, our work is the first comprehensive genomic analysis of DAEC, and as such identified several novel VF groups and genes among DAEC strains, including the ones encoding for Cah, Iss, Kps MII, Sit, TieB, and TraT; the analysis has allowed us to suggest for the first time a pathogenetic paradigm for this pathotype. Lastly, our work suggests clinical application by identifying common and conserved VF that may offer promise as immunogens to control DAEC and tEAEC infections.
Data Availability Statement
The genome sequence assemblies generated in this study have been deposited in GenBank under the accession numbers listed in Supplementary Table 2. The remaining data that support the findings of this study are available from the corresponding author upon request.
Ethics Statement
The studies involving human participants were reviewed and approved by both the Hospital General O'Horan Ethics Committee and the CINVESTAV Committee of Bioethics for Human Research. Legal guardians were required to sign an informed consent form. All children received medical treatment according to the hospital protocols. Written informed consent to participate in this study was provided by the participants' legal guardian/next of kin.
Author Contributions
MM-S and TE-G conceived the experiments. TE-G was Co-PI of the epidemiological study. MM-S and NM-G performed the experiments. MM-S, AV-P, and EM-R performed all bioinformatic analysis. MBZ was Co-PI of the epidemiological study, collected clinical samples data, and strains data. MM-S, MBZ, JPN, and TE-G wrote the manuscript, which was reviewed and approved by all authors. All authors contributed to the article and approved the submitted version.
Conflict of Interest
The authors declare that the research was conducted in the absence of any commercial or financial relationships that could be construed as a potential conflict of interest.
Acknowledgments
CONACYT scholarship 368026 to MM-S, 261884 to AV-P, and 368716 to NM-G. TE-G was recipient of CONACYT Grant 254990. We thank Diego Meza-Segura for realization of drawings.
Supplementary Material
The Supplementary Material for this article can be found online at: https://www.frontiersin.org/articles/10.3389/fcimb.2020.572951/full#supplementary-material
Footnotes
1. ^Krueger F. Trim Galore 0.4.1. Available online at: https://www.bioinformatics.babraham.ac.uk/projects/trim_galore/ (accessed on November 12th, 2015).
2. ^Available online at: https://github.com/avera1988/E.coli_PopulationStructure.
References
Abreu, A. G., and Barbosa, A. S. (2017). How Escherichia coli circumvent complement-mediated killing. Front. Immunol. 8:452. doi: 10.3389/fimmu.2017.00452
Besemer, J., and Borodovsky, M. (2005). GeneMark: web software for gene finding in prokaryotes, eukaryotes and viruses. Nucleic Acids Res. 33, W451–454. doi: 10.1093/nar/gki487
Bielaszewska, M., Prager, R., Kock, R., Mellmann, A., Zhang, W., Tschape, H., et al. (2007). Shiga toxin gene loss and transfer in vitro and in vivo during enterohemorrhagic Escherichia coli O26 infection in humans. Appl. Environ. Microbiol. 73, 3144–3150. doi: 10.1128/AEM.02937-06
Boetzer, M., Henkel, C. V., Jansen, H. J., Butler, D., and Pirovano, W. (2011). Scaffolding pre-assembled contigs using SSPACE. Bioinformatics 27, 578–579. doi: 10.1093/bioinformatics/btq683
Boetzer, M., and Pirovano, W. (2012). Toward almost closed genomes with GapFiller. Genome Biol. 13:R56. doi: 10.1186/gb-2012-13-6-r56
Buckles, E. L., Wang, X., Lane, M. C., Lockatell, C. V., Johnson, D. E., Rasko, D. A., et al. (2009). Role of the K2 capsule in Escherichia coli urinary tract infection and serum resistance. J. Infect. Dis. 199, 1689–1697. doi: 10.1086/598524
Carter, M. Q., Brandl, M. T., Kudva, I. T., Katani, R., Moreau, M. R., and Kapur, V. (2018). Conditional function of autoaggregative protein cah and common cah mutations in shiga toxin-producing Escherichia coli. Appl. Environ. Microbiol. 84, e01739–17. doi: 10.1128/AEM.01739-17
Cerna, J. F., Nataro, J. P., and Estrada-Garcia, T. (2003). Multiplex PCR for detection of three plasmid-borne genes of enteroaggregative Escherichia coli strains. J. Clin. Microbiol. 41, 2138–2140. doi: 10.1128/JCM.41.5.2138-2140.2003
Contreras-Moreira, B., and Vinuesa, P. (2013). GET_HOMOLOGUES, a versatile software package for scalable and robust microbial pangenome analysis. Appl. Environ. Microbiol. 79, 7696–7701. doi: 10.1128/AEM.02411-13
Duan, L., and Mukherjee, E. (2016). Janeway's immunobiology, ninth edition. Yale J. Biol. Med. 89, 424–425.
Dudley, E. G., Thomson, N. R., Parkhill, J., Morin, N. P., and Nataro, J. P. (2006). Proteomic and microarray characterization of the AggR regulon identifies a pheU pathogenicity island in enteroaggregative Escherichia coli. Mol. Microbiol. 61, 1267–1282. doi: 10.1111/j.1365-2958.2006.05281.x
Endres, S., van der Meer, J. W., and Dinarello, C. A. (1987). Interleukin-1 in the pathogenesis of fever. Eur. J. Clin. Invest. 17, 469–474. doi: 10.1111/j.1365-2362.1987.tb01144.x
Estrada-Garcia, T., and Navarro-Garcia, F. (2012). Enteroaggregative Escherichia coli pathotype: a genetically heterogeneous emerging foodborne enteropathogen. FEMS Immunol. Med. Microbiol. 66, 281–298. doi: 10.1111/j.1574-695X.2012.01008.x
Farmer, J. J. III., Fanning, G. R., Davis, B. R., O'Hara, C. M., Riddle, C., Hickman-Brenner, F. W., et al. (1985). Escherichia fergusonii and enterobacter taylorae, two new species of enterobacteriaceae isolated from clinical specimens. J. Clin. Microbiol. 21, 77–81. doi: 10.1128/JCM.21.1.77-81.1985
Fasano, A., Noriega, F. R., Liao, F. M., Wang, W., and Levine, M. M. (1997). Effect of Shigella Enterotoxin 1 (ShET1) on rabbit intestine in vitro and in vivo. Gut 40, 505–511. doi: 10.1136/gut.40.4.505
Flores-Mireles, A. L., Walker, J. N., Caparon, M., and Hultgren, S. J. (2015). Urinary tract infections: epidemiology, mechanisms of infection and treatment options. Nat. Rev. Microbiol. 13, 269–284. doi: 10.1038/nrmicro3432
Fox, S., Goswami, C., Holden, M., Connolly, J. P. R., Mordue, J., O'Boyle, N., et al. (2020). A highly conserved complete accessory Escherichia coli type III secretion system 2 is widespread in bloodstream isolates of the ST69 lineage. Sci. Rep. 10:4135. doi: 10.1038/s41598-020-61026-x
Gao, Q., Wang, X., Xu, H., Xu, Y., Ling, J., Zhang, D., et al. (2012). Roles of iron acquisition systems in virulence of extraintestinal pathogenic Escherichia coli: salmochelin and aerobactin contribute more to virulence than heme in a chicken infection model. BMC Microbiol. 12:143. doi: 10.1186/1471-2180-12-143
Garcia, E. C., Brumbaugh, A. R., and Mobley, H. L. (2011). Redundancy and specificity of Escherichia coli iron acquisition systems during urinary tract infection. Infect. Immun. 79, 1225–1235. doi: 10.1128/IAI.01222-10
GBD 2016 Diarrhoeal Disease Collaborators (2018). Estimates of the global, regional, and national morbidity, mortality, and aetiologies of diarrhoea in 195 countries: a systematic analysis for the Global Burden of Disease Study 2016. Lancet. Infect. Dis. 18, 1211–1228. doi: 10.1016/S1473-3099(18)30362-1
Guyer, D. M., Radulovic, S., Jones, F. E., and Mobley, H. L. (2002). Sat, the secreted autotransporter toxin of uropathogenic Escherichia coli, is a vacuolating cytotoxin for bladder and kidney epithelial cells. Infect. Immun. 70, 4539–4546. doi: 10.1128/IAI.70.8.4539-4546.2002
Guzman-Hernandez, R., Contreras-Rodriguez, A., Hernandez-Velez, R., Perez-Martinez, I., Lopez-Merino, A., Zaidi, M. B., et al. (2016). Mexican unpasteurised fresh cheeses are contaminated with salmonella spp., non-O157 shiga toxin producing Escherichia coli and potential uropathogenic E. coli strains: a public health risk. Int. J. Food Microbiol. 237, 10–16. doi: 10.1016/j.ijfoodmicro.2016.08.018
Hazen, T. H., Donnenberg, M. S., Panchalingam, S., Antonio, M., Hossain, A., Mandomando, I., et al. (2016). Genomic diversity of EPEC associated with clinical presentations of differing severity. Nat. Microbiol. 1:15014. doi: 10.1038/nmicrobiol.2015.14
Henderson, I. R., Czeczulin, J., Eslava, C., Noriega, F., and Nataro, J. P. (1999). Characterization of pic, a secreted protease of shigella flexneri and enteroaggregative Escherichia coli. Infect. Immun. 67, 5587–5596. doi: 10.1128/IAI.67.11.5587-5596.1999
Hunt, M., Kikuchi, T., Sanders, M., Newbold, C., Berriman, M., and Otto, T. D. (2013). REAPR: a universal tool for genome assembly evaluation. Genome Biol. 14:R47. doi: 10.1186/gb-2013-14-5-r47
Jennings, M. C., Tilley, D. H., Ballard, S. B., Villanueva, M., Costa, F. M., Lopez, M., et al. (2017). Case-case analysis using 7 years of travelers' diarrhea surveillance data: preventive and travel medicine applications in cusco, Peru. Am. J. Trop. Med. Hyg. 96, 1097–1106. doi: 10.4269/ajtmh.16-0633
Kaas, R. S., Friis, C., Ussery, D. W., and Aarestrup, F. M. (2012). Estimating variation within the genes and inferring the phylogeny of 186 sequenced diverse Escherichia coli genomes. BMC Genomics 13:577. doi: 10.1186/1471-2164-13-577
Kalyaanamoorthy, S., Minh, B. Q., Wong, T. K. F., von Haeseler, A., and Jermiin, L. S. (2017). ModelFinder: fast model selection for accurate phylogenetic estimates. Nat. Methods 14, 587–589. doi: 10.1038/nmeth.4285
Kaper, J. B., Nataro, J. P., and Mobley, H. L. (2004). Pathogenic Escherichia coli. Nat. Rev. Microbiol. 2, 123–140. doi: 10.1038/nrmicro818
Katoh, K., and Standley, D. M. (2013). MAFFT multiple sequence alignment software version 7: improvements in performance and usability. Mol. Biol. Evol. 30, 772–780. doi: 10.1093/molbev/mst010
Kehres, D. G., Janakiraman, A., Slauch, J. M., and Maguire, M. E. (2002). SitABCD is the alkaline Mn2+ transporter of Salmonella enterica serovar typhimurium. J. Bacteriol. 184, 3159–3166. doi: 10.1128/JB.184.12.3159-3166.2002
Koh, E. I., Robinson, A. E., Bandara, N., Rogers, B. E., and Henderson, J. P. (2017). Copper import in Escherichia coli by the yersiniabactin metallophore system. Nat. Chem. Biol. 13, 1016–1021. doi: 10.1038/nchembio.2441
Lacher, D. W., Steinsland, H., Blank, T. E., Donnenberg, M. S., and Whittam, T. S. (2007). Molecular evolution of typical enteropathogenic Escherichia coli: clonal analysis by multilocus sequence typing and virulence gene allelic profiling. J. Bacteriol. 189, 342–350. doi: 10.1128/JB.01472-06
Lanata, C. F., Fischer-Walker, C. L., Olascoaga, A. C., Torres, C. X., Aryee, M. J., Black, R. E., et al. (2013). Global causes of diarrheal disease mortality in children <5 years of age: a systematic review. PLoS ONE. 8, e72788. doi: 10.1371/journal.pone.0072788
Letunic, I., and Bork, P. (2016). Interactive tree of life (iTOL) v3: an online tool for the display and annotation of phylogenetic and other trees. Nucleic Acids Res. 44, W242–245. doi: 10.1093/nar/gkw290
Liu, B., Zheng, D., Jin, Q., Chen, L., and Yang, J. (2019). VFDB 2019: a comparative pathogenomic platform with an interactive web interface. Nucleic Acids Res. 47, D687–D692. doi: 10.1093/nar/gky1080
Lopez, C. A., and Skaar, E. P. (2018). The impact of dietary transition metals on host-bacterial interactions. Cell Host Microbe 23, 737–748. doi: 10.1016/j.chom.2018.05.008
Lopez-Saucedo, C., Cerna, J. F., Villegas-Sepulveda, N., Thompson, R., Velazquez, F. R., Torres, J., et al. (2003). Single multiplex polymerase chain reaction to detect diverse loci associated with diarrheagenic Escherichia coli. Emerg. Infect. Dis. 9, 127–131. doi: 10.3201/eid0901.010507
Lukjancenko, O., Wassenaar, T. M., and Ussery, D. W. (2010). Comparison of 61 sequenced Escherichia coli genomes. Microb. Ecol. 60, 708–720. doi: 10.1007/s00248-010-9717-3
Mansan-Almeida, R., Pereira, A. L., and Giugliano, L. G. (2013). Diffusely adherent Escherichia coli strains isolated from children and adults constitute two different populations. BMC Microbiol. 13:22. doi: 10.1186/1471-2180-13-22
Mao, B. H., Chang, Y. F., Scaria, J., Chang, C. C., Chou, L. W., Tien, N., et al. (2012). Identification of Escherichia coli genes associated with urinary tract infections. J. Clin. Microbiol. 50, 449–456. doi: 10.1128/JCM.00640-11
Mathewson, J. J., Oberhelman, R. A., Dupont, H. L., Javier de la Cabada, F., and Garibay, E. V. (1987). Enteroadherent Escherichia coli as a cause of diarrhea among children in Mexico. J. Clin. Microbiol. 25, 1917–1919. doi: 10.1128/JCM.25.10.1917-1919.1987
Meza-Segura, M., and Estrada-Garcia, T. (2016). “Diffusely adherent Escherichia coli,” in Escherichia coli in the Americas, ed A. G. Torres (Cham: Springer International Publishing), 125–147. doi: 10.1007/978-3-319-45092-6_6
Miajlovic, H., and Smith, S. G. (2014). Bacterial self-defence: how Escherichia coli evades serum killing. FEMS Microbiol. Lett. 354, 1–9. doi: 10.1111/1574-6968.12419
Micenkova, L., Staudova, B., Bosak, J., Mikalova, L., Littnerova, S., Vrba, M., et al. (2014). Bacteriocin-encoding genes and ExPEC virulence determinants are associated in human fecal Escherichia coli strains. BMC Microbiol. 14:109. doi: 10.1186/1471-2180-14-109
Minh, B. Q., Schmidt, H. A., Chernomor, O., Schrempf, D., Woodhams, M. D., von Haeseler, A., et al. (2020). IQ-TREE 2: new models and efficient methods for phylogenetic inference in the genomic era. Mol. Biol. Evol. 37, 1530–1534. doi: 10.1093/molbev/msaa015
Montenegro, M. A., Bitter-Suermann, D., Timmis, J. K., Aguero, M. E., Cabello, F. C., Sanyal, S. C., et al. (1985). traT gene sequences, serum resistance and pathogenicity-related factors in clinical isolates of Escherichia coli and other gram-negative bacteria. J. Gen. Microbiol. 131, 1511–1521. doi: 10.1099/00221287-131-6-1511
Montero, D., Orellana, P., Gutierrez, D., Araya, D., Salazar, J. C., Prado, V., et al. (2014). Immunoproteomic analysis to identify Shiga toxin-producing Escherichia coli outer membrane proteins expressed during human infection. Infect. Immun. 82, 4767–4777. doi: 10.1128/IAI.02030-14
Montero, D. A., Canto, F. D., Velasco, J., Colello, R., Padola, N. L., Salazar, J. C., et al. (2019). Cumulative acquisition of pathogenicity islands has shaped virulence potential and contributed to the emergence of LEE-negative Shiga toxin-producing Escherichia coli strains. Emerg. Microbes Infect. 8, 486–502. doi: 10.1080/22221751.2019.1595985
Nataro, J. P. (2005). Enteroaggregative Escherichia coli pathogenesis. Curr. Opin. Gastroenterol. 21, 4–8.
Nataro, J. P., Seriwatana, J., Fasano, A., Maneval, D. R., Guers, L. D., Noriega, F., et al. (1995). Identification and cloning of a novel plasmid-encoded enterotoxin of enteroinvasive Escherichia coli and shigella strains. Infect. Immun. 63, 4721–4728. doi: 10.1128/IAI.63.12.4721-4728.1995
Nataro, J. P., Yikang, D., Yingkang, D., and Walker, K. (1994). AggR, a transcriptional activator of aggregative adherence fimbria I expression in enteroaggregative Escherichia coli. J. Bacteriol. 176, 4691–4699. doi: 10.1128/JB.176.15.4691-4699.1994
Navarro-Garcia, F., Ruiz-Perez, F., Cataldi, A., and Larzabal, M. (2019). Type VI secretion system in pathogenic Escherichia coli: structure, role in virulence, and acquisition. Front. Microbiol. 10:1965. doi: 10.3389/fmicb.2019.01965
Neumann, B., Pospiech, A., and Schairer, H. U. (1992). Rapid isolation of genomic DNA from gram-negative bacteria. Trends Genet. 8, 332–333. doi: 10.1016/0168-9525(92)90269-A
Nurk, S., Bankevich, A., Antipov, D., Gurevich, A. A., Korobeynikov, A., Lapidus, A., et al. (2013). Assembling single-cell genomes and mini-metagenomes from chimeric MDA products. J. Comput. Biol. 20, 714–737. doi: 10.1089/cmb.2013.0084
Ochoa, T. J., Ecker, L., Barletta, F., Mispireta, M. L., Gil, A. I., Contreras, C., et al. (2009). Age-related susceptibility to infection with diarrheagenic Escherichia coli among infants from periurban areas in Lima, Peru. Clin. Infect. Dis. 49, 1694–1702. doi: 10.1086/648069
Palmer, J. D., Piattelli, E., McCormick, B. A., Silby, M. W., Brigham, C. J., and Bucci, V. (2018). Engineered probiotic for the inhibition of salmonella via tetrathionate-induced production of microcin H47. ACS Infect. Dis. 4, 39–45. doi: 10.1021/acsinfecdis.7b00114
Paredes-Paredes, M., Okhuysen, P. C., Flores, J., Mohamed, J. A., Padda, R. S., Gonzalez-Estrada, A., et al. (2011). Seasonality of diarrheagenic Escherichia coli pathotypes in the US students acquiring diarrhea in Mexico. J. Travel Med. 18, 121–125. doi: 10.1111/j.1708-8305.2010.00488.x
Patzi-Vargas, S., Zaidi, M., Bernal-Reynaga, R., Leon-Cen, M., Michel, A., and Estrada-Garcia, T. (2013). Persistent bloody diarrhoea without fever associated with diffusely adherent Escherichia coli in a young child. J. Med. Microbiol. 62(Pt 12), 1907–1910. doi: 10.1099/jmm.0.062349-0
Patzi-Vargas, S., Zaidi, M. B., Perez-Martinez, I., Leon-Cen, M., Michel-Ayala, A., Chaussabel, D., et al. (2015). Diarrheagenic Escherichia coli carrying supplementary virulence genes are an important cause of moderate to severe diarrhoeal disease in Mexico. PLoS Negl. Trop. Dis. 9:e0003510. doi: 10.1371/journal.pntd.0003510
Prjibelski, A. D., Vasilinetc, I., Bankevich, A., Gurevich, A., Krivosheeva, T., Nurk, S., et al. (2014). ExSPAnder: a universal repeat resolver for DNA fragment assembly. Bioinformatics 30, i293–301. doi: 10.1093/bioinformatics/btu266
Rasko, D. A., Del Canto, F., Luo, Q., Fleckenstein, J. M., Vidal, R., and Hazen, T. H. (2019). Comparative genomic analysis and molecular examination of the diversity of enterotoxigenic Escherichia coli isolates from Chile. PLoS Negl. Trop. Dis. 13:e0007828. doi: 10.1371/journal.pntd.0007828
Rasko, D. A., Rosovitz, M. J., Myers, G. S., Mongodin, E. F., Fricke, W. F., Gajer, P., et al. (2008). The pangenome structure of Escherichia coli: comparative genomic analysis of E. coli commensal and pathogenic isolates. J. Bacteriol. 190, 6881–6893. doi: 10.1128/JB.00619-08
Rasko, D. A., Webster, D. R., Sahl, J. W., Bashir, A., Boisen, N., Scheutz, F., et al. (2011). Origins of the E. coli strain causing an outbreak of hemolytic-uremic syndrome in Germany. N Engl. J. Med. 365, 709–717. doi: 10.1056/NEJMoa1106920
Ren, C. P., Chaudhuri, R. R., Fivian, A., Bailey, C. M., Antonio, M., Barnes, W. M., et al. (2004). The ETT2 gene cluster, encoding a second type III secretion system from Escherichia coli, is present in the majority of strains but has undergone widespread mutational attrition. J. Bacteriol. 186, 3547–3560. doi: 10.1128/JB.186.11.3547-3560.2004
Riveros, M., Barletta, F., Cabello, M., Durand, D., Mercado, E. H., Contreras, C., et al. (2011). Adhesion patterns in diffusely adherent Escherichia coli (DAEC) strains isolated from children with and without diarrhea. Rev. Peru Med. Exp. Salud. Publica 28, 21–28. doi: 10.1590/S1726-46342011000100004
Rouli, L., Merhej, V., Fournier, P. E., and Raoult, D. (2015). The bacterial pangenome as a new tool for analysing pathogenic bacteria. N Microbes N Infect. 7, 72–85. doi: 10.1016/j.nmni.2015.06.005
Runyen-Janecky, L. J., Reeves, S. A., Gonzales, E. G., and Payne, S. M. (2003). Contribution of the shigella flexneri sit, Iuc, and Feo iron acquisition systems to iron acquisition in vitro and in cultured cells. Infect. Immun. 71, 1919–1928. doi: 10.1128/IAI.71.4.1919-1928.2003
Ruuska, T., and Vesikari, T. (1990). Rotavirus disease in finnish children: use of numerical scores for clinical severity of diarrhoeal episodes. Scand. J. Infect. Dis. 22, 259–267. doi: 10.3109/00365549009027046
Samadder, P., Xicohtencatl-Cortes, J., Saldana, Z., Jordan, D., Tarr, P. I., Kaper, J. B., et al. (2009). The Escherichia coli ycbQRST operon encodes fimbriae with laminin-binding and epithelial cell adherence properties in Shiga-toxigenic E. coli O157:H7. Environ. Microbiol. 11, 1815–1826. doi: 10.1111/j.1462-2920.2009.01906.x
Sassone-Corsi, M., Nuccio, S. P., Liu, H., Hernandez, D., Vu, C. T., Takahashi, A. A., et al. (2016). Microcins mediate competition among enterobacteriaceae in the inflamed gut. Nature 540, 280–283. doi: 10.1038/nature20557
Servin, A. L. (2014). Pathogenesis of human diffusely adhering Escherichia coli expressing Afa/Dr adhesins (Afa/Dr DAEC): current insights and future challenges. Clin. Microbiol. Rev. 27, 823–869. doi: 10.1128/CMR.00036-14
Sheikh, A., Rashu, R., Begum, Y. A., Kuhlman, F. M., Ciorba, M. A., Hultgren, S. J., et al. (2017). Highly conserved type 1 pili promote enterotoxigenic E. coli pathogen-host interactions. PLoS Negl. Trop. Dis. 11:e0005586. doi: 10.1371/journal.pntd.0005586
Spaulding, C. N., Klein, R. D., Ruer, S., Kau, A. L., Schreiber, H. L., Cusumano, Z. T., et al. (2017). Selective depletion of uropathogenic E. coli from the gut by a FimH antagonist. Nature 546, 528–532. doi: 10.1038/nature22972
Taddei, C. R., Fasano, A., Ferreira, A. J., Trabulsi, L. R., and Martinez, M. B. (2005). Secreted autotransporter toxin produced by a diffusely adhering Escherichia coli strain causes intestinal damage in animal model assays. FEMS Microbiol. Lett. 250, 263–269. doi: 10.1016/j.femsle.2005.07.013
Tonkin-Hill, G., Lees, J. A., Bentley, S. D., Frost, S. D. W., and Corander, J. (2018). RhierBAPS: an R implementation of the population clustering algorithm hierBAPS. Wellcome Open Res. 3:93. doi: 10.12688/wellcomeopenres.14694.1
Torres, A. G., Perna, N. T., Burland, V., Ruknudin, A., Blattner, F. R., and Kaper, J. B. (2002). Characterization of Cah, a calcium-binding and heat-extractable autotransporter protein of enterohaemorrhagic Escherichia coli. Mol. Microbiol. 45, 951–966. doi: 10.1046/j.1365-2958.2002.03094.x
Torres, A. G., Redford, P., Welch, R. A., and Payne, S. M. (2001). TonB-dependent systems of uropathogenic Escherichia coli: aerobactin and heme transport and TonB are required for virulence in the mouse. Infect. Immun. 69, 6179–6185. doi: 10.1128/IAI.69.10.6179-6185.2001
Vasilinetc, I., Prjibelski, A. D., Gurevich, A., Korobeynikov, A., and Pevzner, P. A. (2015). Assembling short reads from jumping libraries with large insert sizes. Bioinformatics 31, 3262–3268. doi: 10.1093/bioinformatics/btv337
Vera-Ponce de Leon, A., Jahnes, B. C., Duan, J., Camuy-Velez, L. A., and Sabree, Z. L. (2020). Cultivable, host-specific bacteroidetes symbionts exhibit diverse polysaccharolytic strategies. Appl. Environ. Microbiol. 86, e00091–20. doi: 10.1128/AEM.00091-20
Wei, Y., and Murphy, E. R. (2016). Shigella iron acquisition systems and their regulation. Front. Cell. Infect. Microbiol. 6:18. doi: 10.3389/fcimb.2016.00018
Wijetunge, D. S., Gongati, S., DebRoy, C., Kim, K. S., Couraud, P. O., Romero, I. A., et al. (2015). Characterizing the pathotype of neonatal meningitis causing Escherichia coli (NMEC). BMC Microbiol. 15:211. doi: 10.1186/s12866-015-0547-9
World Health Organization. (2018). Outbreaks of E. coli O104: H4 infection: update 30. Available online at: https://www.euro.who.int/en/countries/germany/news/news/2011/07/outbreaks-of-e.-coli-o104h4-infection-update-30 (accessed June 12, 2020).
Yao, Y., Xie, Y., Perace, D., Zhong, Y., Lu, J., Tao, J., et al. (2009). The type III secretion system is involved in the invasion and intracellular survival of Escherichia coli K1 in human brain microvascular endothelial cells. FEMS Microbiol. Lett. 300, 18–24. doi: 10.1111/j.1574-6968.2009.01763.x
Zhou, Y., Zhu, X., Hou, H., Lu, Y., Yu, J., Mao, L., et al. (2018). Characteristics of diarrheagenic Escherichia coli among children under 5 years of age with acute diarrhea: a hospital based study. BMC Infect. Dis. 18:63. doi: 10.1186/s12879-017-2936-1
Keywords: DAEC, EAEC, genomes, phylogeny, virulence factors, DAEC pathogenesis
Citation: Meza-Segura M, Zaidi MB, Vera-Ponce de León A, Moran-Garcia N, Martinez-Romero E, Nataro JP and Estrada-Garcia T (2020) New Insights Into DAEC and EAEC Pathogenesis and Phylogeny. Front. Cell. Infect. Microbiol. 10:572951. doi: 10.3389/fcimb.2020.572951
Received: 15 June 2020; Accepted: 09 September 2020;
Published: 15 October 2020.
Edited by:
Roxane M. Piazza, Butantan Institute, BrazilReviewed by:
David A. Montero, University of Chile, ChileBrandon Luedtke, University of Nebraska at Kearney, United States
Claire Jenkins, Public Health England, United Kingdom
Copyright © 2020 Meza-Segura, Zaidi, Vera-Ponce de León, Moran-Garcia, Martinez-Romero, Nataro and Estrada-Garcia. This is an open-access article distributed under the terms of the Creative Commons Attribution License (CC BY). The use, distribution or reproduction in other forums is permitted, provided the original author(s) and the copyright owner(s) are credited and that the original publication in this journal is cited, in accordance with accepted academic practice. No use, distribution or reproduction is permitted which does not comply with these terms.
*Correspondence: Teresa Estrada-Garcia, dGVzdHJhZGFAY2ludmVzdGF2Lm14