- 1College of Life Science and Technology, Huazhong University of Science and Technology, Wuhan, China
- 2National Engineering Research Center for Nanomedicine, Huazhong University of Science and Technology, Wuhan, China
The skin represents the exterior interface between the human body with the environment while providing a home to trillions of the commensal microorganisms—collectively referred to as the skin microbiota. These microbes that coexist in an established balance play a pivotal role in the protection of cutaneous health and the orchestration of skin homeostasis. However, the well-controlled but delicate balance can be perturbed by alterations in the skin microbial communities, namely, dysbiosis, often due to commensals defeated by pathogens competing for space and nutrients, which leads to the occurrence of multiple cutaneous disorders. In view of this, the analysis of skin microbiota constituents in skin diseases is crucial for defining the role of commensal microbes and treatment of skin diseases. Emerging evidence shows that the ecology-based therapy of microbial transplantation has been proven as a valid therapeutic strategy for cutaneous disorders caused by skin microbial dysbiosis. Although its mechanism is not well-understood, there are already some applications for ecology-based therapy with the aim of correcting the imbalances on the cutaneous ecosystem. In this review, we summarize the interactions between dysbiosis and the cutaneous disorders, including homeostasis and dysbiosis of skin microbiota, microbial composition in skin diseases, and the mechanisms and applications of reversing or ameliorating the dysbiosis by the targeted manipulation of the skin microbiota, which may contribute to aid development of therapeutic modality for ecology-based therapy.
Introduction
The skin is a dynamic and steady ecology that is populated with millions of microbes, such as bacteria, fungi, and viruses, which were collectively termed the skin microbiota (Figure 1; Grice and Segre, 2011). Although the skin, the largest and most exposed organ in the body (Gallo, 2017), acquires plenty of transient species of microbe by constant dialogue of different people and the external environments (Oh et al., 2016), the composition of the skin microbiota remains surprisingly stable over time (Dorrestein et al., 2016; Lax et al., 2017; Sohn, 2018). However, the diversity and relative abundance of the body's microbial communities vary in both the individual and the physiology of the skin sites, which has been categorized into four major skin microenvironment: oily, moist, dry, and foot (Figure 1; Belkaid and Segre, 2014; Byrd et al., 2018). Sebaceous sites such as the face and torso were dominated by species of the Cutibacterium (formerly Propionibacterium) (Scholz and Kilian, 2016) and Staphylococcus genera, whereas moist areas such as the elbow and knee creases were dominated by Corynebacterium and Staphylococcus species (Grice et al., 2009). In contrast to bacterial communities, fungi of the genus Malassezia are found throughout the body (Oh et al., 2014) but predominated at oily sites such as the face and back (Findley et al., 2013). Although microbial variations of these sites are highly consistent between people, the relative abundance of the individual skin microbiota, especially low-abundance microbial species, can be differentiated by metagenomic shotgun sequencing of high-resolution and multikingdom analyses (Oh et al., 2016), which hints that the individual may be identified from any part of the skin (Lax et al., 2017). For instance, although the taxa of bacteria that colonize the skin of normal individuals is generally similar, the skin microbiota of individuals with primary immunodeficiency displays more ecological permissiveness with altered population structures (Oh et al., 2013; Lehman, 2014).
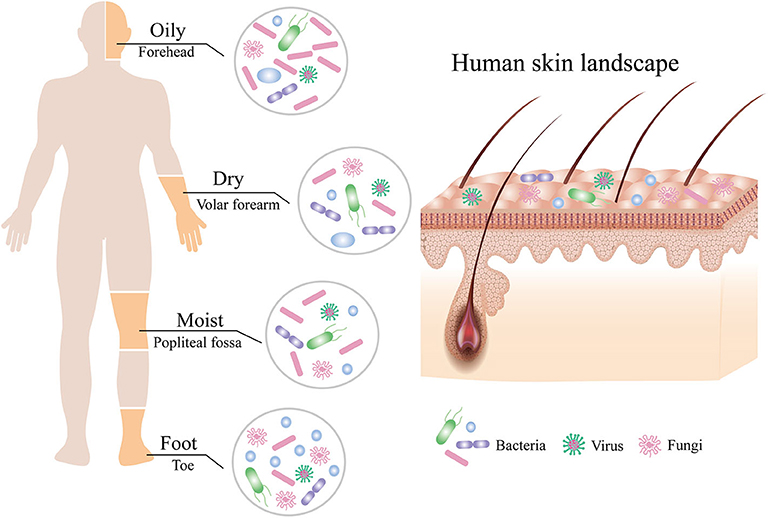
Figure 1. Composition of the human skin microbiota. The human skin is inhabited by millions of bacteria, fungi, and viruses that compose the skin microbiota. The composition of human skin microbiota is shaped by biogeography of the skin site including oily, moist, dry, and foot sites. Bacteria are the most abundant at all sites, followed by fungi, with the fewest viruses.
Actually, it is still unknown how the homeostasis is maintained and shaped by the skin microbiota, but the balance between members of skin microbial communities plays a pivotal role in guarding against cutaneous disorders (Dreno et al., 2016). The opportunistic pathogen Staphylococcus aureus asymptomatically colonizes more than 30% of the individuals under steady-state conditions in a relatively low colonization rate and barely detectable abundance levels (Totté et al., 2016; Totte et al., 2016), indicating that potentially pathogenic bacteria that colonize the skin of healthy individuals are influenced by the native microbial community. However, its expansion in certain contexts is able to counteract promptly the equilibrium state (Parlet et al., 2019), ultimately resulting in several skin disorders such as atopic dermatitis (AD) (Kobayashi et al., 2015) and systemic lupus erythematosus (Conti et al., 2016). Furthermore, Staphylococcus epidermidis, the most prominent of the coagulase-negative Staphylococcus (CoNS) species (Sohn, 2018), which is generally beneficial to the host, appears unexpectedly in AD flares and predominates in less severe flares (Byrd et al., 2017), implying that a disease could arise from dysbiosis of the microbial community without an invading pathogen prevailing in the community. Of note, heterogeneous S. epidermidis strain communities in less severe AD flares are genomically similar to nosocomial strains that are a leading cause of mortality of sepsis in newborn infants (Meisel et al., 2016). In addition to bacterial dysbiosis, many reports have suggested that fungi and viruses have an impact on skin conditions by their own changes in cutaneous microbial communities, such as Malassezia and eczema (Darabi et al., 2009; Chng et al., 2016) and herpesviruses and chickenpox (Chen et al., 2018). Therefore, observing disease-associated dysbiosis is profound since these interactions may augment disease severity or susceptibility or facilitate transitions from opportunistic or commensal to pathogenic.
There is no doubt that the composition of the skin microbiota can shift dramatically during the disease progression (Kong et al., 2012). Although it is not yet known that those alterations are a cause or consequence of the skin disease, certain specific species or strains of microbes have been substantially linked with specific cutaneous disorders such as eczema (Chng et al., 2016), psoriasis (Quan et al., 2019), and acne vulgaris (AV) (Szegedi et al., 2019). For example, the appearance of AD has been tightly associated with dysbiosis in the skin microbiota (Kobayashi et al., 2015). In contrast to the skin microbiota of healthy adult, this distortion in patients with more severe AD flares is mainly caused by a single clade of S. aureus and demonstrates a strong correlation with S. aureus relative abundances (Byrd et al., 2017), suggesting that disease states of skin have a specific microbiota composition differing from that of healthy skin. These findings lead to a corollary that disease-associated changes in the skin microbial community can sever itself as a “pathogen” and then conjure up a potential therapeutic strategy that adjusting the skin microbiota reverts the consistency of premorbid state by a microbial transplant on the skin. Akin to the therapeutic principle appearing in the gut, manipulating microbiota to modulate dysbiosis has been successful in fecal microbiota transplantation (FMT) for the control of the antibiotic-resistant bacteria Clostridioides difficile (formerly Clostridium difficile) infection (Allegretti et al., 2019) and more recently in leveraging probiotics such as Lactobacillus plantarum to prevent neonatal sepsis (Panigrahi et al., 2017). With an increasing appreciation in the contribution of microbes to skin diseases, ecology-based therapy of microbial transplantation that exploits the preferred niche of skin microbiota has also been developed for the treatment and study of diseases. Compared to traditional antibiotic therapy of directly eradicating pathogens by brute force, the advantage of this methodology is not only to reduce the formation of drug-resistant bacteria but also to avoid destroying the indigenous microbiota of patients. Therefore, finding out the extent to which microbial composition at the site of skin disorders is deciphered is remarkably important for ecology-based therapy.
Microbial Composition in Skin Diseases
The development of high-throughput microbial genomic sequencing technique, including amplicon sequencing and whole genome sequencing (shotgun metagenomic sequencing), makes it possible to thoroughly characterize skin microbiota constituents (Byrd et al., 2018; Grogan et al., 2019). Traditionally, due to the selectivity of artificial growth conditions, capitalizing on culture-based approaches to explore skin microbiota components can underestimate the total community diversity (Kong and Segre, 2012). Hence, to bypass the biases and selection caused by strain isolation and culture (Goodman et al., 2011), next-generation sequencing techniques are prescribed to capture the complete diversity of the skin microbiome (Neuman and Koren, 2016). Amplicon sequencing is the most commonly used for characterizing skin microbiota communities by the 16S ribosomal RNA (rRNA) gene for bacteria (Jo et al., 2016) or the internal transcribed spacer 1 (ITS1) region of the eukaryotic ribosomal gene for fungi (Schoch et al., 2012). It is able to give a classification at the relative abundance of the genus and, when possible, the species level. On the other hand, shotgun metagenomic sequencing provides much higher resolution to differentiate species-, strain- and even single-nucleotide variant (SNV)-level diversification, as all genetic material in the sample is simultaneously sequenced (Oh et al., 2014, 2016; Grogan et al., 2019). Considering that most amplicon sequencing approaches are insufficient to recapitulate skin microbiome community composition at the species level, especially members of the Staphylococcus genus (Meisel et al., 2016), and that the important functional differences existing among strains within a species are not being resolved (Bosi et al., 2016) due to modest gene gain or loss events or even differences in gene expression among strains (Chen et al., 2018), the ability of species- and strain-level analysis of the skin microbiome population structures is crucial for defining the role of commensals and explaining the pathogenesis of skin diseases.
Skin Microbiota Constituents in AD
AD (also known as eczema) is a paradigmatic chronic inflammatory cutaneous disease that affects 10–30% of children in industrialized countries and has a lifetime prevalence of up to a fifth of the population in the developed world (Weidinger and Novak, 2016; Tsakok et al., 2018; Guttman-Yassky et al., 2019). The skin condition is clinically characterized by intense pruritus, relapsing eczematous lesions, and a fluctuating course (Eyerich et al., 2015; Geoghegan et al., 2018), whose manifestations not only have a substantial effect on quality of life by sleep deprivation and profoundly diminished self-esteem (Simpson et al., 2016; Weidinger et al., 2018) but also increase the risk of infection and other atopic disorders (Thaçi et al., 2016). Recent work provided evidence that patients with AD have an increased likelihood of developing asthma, allergic rhinitis, and chronic sinusitis with nasal polyposis, which were previously regarded as a symbol of overall immune-system dysfunction (Thaçi et al., 2016; Sohn, 2018). The complexity of AD onset is highlighted by the heterogeneity of course, clinical severity, and treatment responses (Byrd et al., 2017; Sun et al., 2019) and has closely evolved with multiple contributing factors, including impairment of epidermal barrier function, the immunity response of T-helper 2 cell-mediated lymphocyte skewing and immunoglobulin E (IgE)-mediated sensitization, neuroinflammation involved in itch, and dysbiosis of the skin microbiota (Weidinger et al., 2018). The potential genetic determinants of AD predisposition have been identified in relation to variants of more than 30 host gene loci, including the gene encoding filaggrin (FLG, a key component of terminal differentiation and skin barrier function) (Palmer et al., 2006) and genes linked to the immune system (Paternoster et al., 2015).
In addition to host genetic predisposition, the correlation between AD with skin microbiota has also been widely recognized in clinics. As seen in healthy individuals, bacteria communities are the most abundant kingdom across time points and body sites for AD patients, whereas fungal communities are the least abundant and mainly Malassezia species, particularly Malassezia restricta and Malassezia globose (Findley et al., 2013; Chng et al., 2016; Oh et al., 2016). Human polyomaviruses and papillomaviruses prevailing in the eukaryotic DNA viral communities depend on the individual rather than anatomical site (Byrd et al., 2017). In longitudinal surveys of a cohort of pediatric AD patients, shotgun metagenomic sequencing of clinical skin sample throughout the disease course revealed that bacterial communities shifted markedly in this cohort, although the fungal or viral components had no significant differences over time (Byrd et al., 2017). For bacterial communities, investigation of AD flares demonstrated a decline in the total bacterial diversity and the dramatic increase in relative abundance of specific staphylococcal species S. aureus in the flare vs. the healthy (or post-flare) state (Figure 2). In addition, the relative abundance of staphylococci evolved closely with AD flare severity (Salava and Lauerma, 2014; Drago et al., 2016; Gonzalez et al., 2016). For example, individuals with less severe AD flares had relative abundances of 3.8 ± 1.7% S. aureus with 13 ± 3.9% S. epidermidis; individuals with strong AD flares had relative abundances of 34 ± 8.7% S. aureus with 7.4 ± 4.2% S. epidermidis averaged across all sites (Byrd et al., 2017). As such, these flares in eczema patients are thought to be driven by S. aureus skin colonization and exacerbating eczema flare-ups are associated with an increase in the number of S. aureus on affected region and a decrease in S. epidermidis that produce S. aureus-targeting bacteriocins. Intriguingly, a prior study had demonstrated that an increased abundance of S. aureus preceded flares in AD patients (Kennedy et al., 2017), hinting that S. aureus may contribute to the initiation of the disease rather than the consequence of the outbreak. At the strain level, a single clade of S. aureus primarily colonizes more severe AD patients during disease flares, and these same strains at the postflare still persisted in 80% severe AD patients but at markedly lower mean relative abundances (Byrd et al., 2017). The results of strain tracking were well confirmed using a complementary approach in which SNVs were identified in the 1.9 Mbp core genome shared between all sequenced S. aureus. In contrast to clonal S. aureus strain communities, AD patients' heterogeneous S. epidermidis strain communities in both flares and post-flares were composed of multiple different strains from diverse clades (Byrd et al., 2017). Strikingly, in addition to strain-specific differences during the course of AD, whole genome sequencing profiling of strain-level gene variation showed that individuals with less severe AD were colonized with more methicillin-resistant strains, whereas individuals with more severe AD were predominantly colonized with methicillin-sensitive strains (Hsiang et al., 2012; Chaptini et al., 2016).
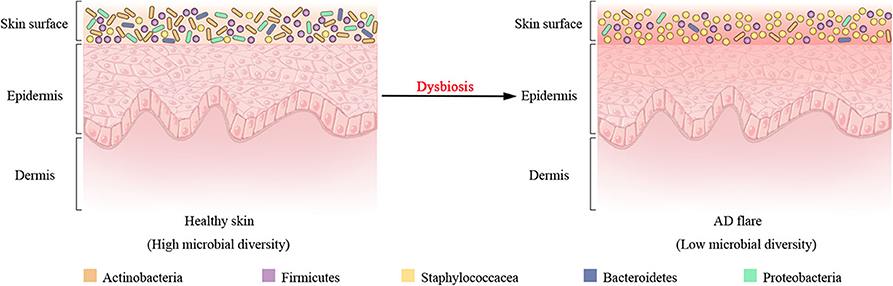
Figure 2. Shift of bacterial communities during atopic dermatitis (AD) disease progression. Four most prominent bacterial taxa (Actinobacteria, Firmicutes, Bacteroidetes, and Proteobacteria) and the Staphylococcaceae (a Firmicute) are found on the surface of healthy skin. Dysbiosis in AD flares is characterized by a decline in bacterial diversity and the dramatic augment in relative abundance of Staphylococcaceae.
However, recent studies hint clues that fungi might also play a fundamental role in the protection of skin from AD. Malassezia is a typically dominant skin-dwelling fungal class comprising 17 species and linked with AD (Sohn, 2018). One cue is that Malassezia peaks in greasy skin in infants or adolescence, but AD becomes less common (Havlickova et al., 2008; Seebacher et al., 2008). Malassezia leverages the lipids of sebum and the stratum corneum to generate their own lipids in the carbohydrate-deficient and lipid-rich skin setting (Scharschmidt and Fischbach, 2013), which concurs with an enrichment of lipase genes and a depletion of carbohydrate-utilizing enzyme genes in Malassezia spp. genomes (Wu et al., 2015). This indicates that the decrease in surface lipids may restrict Malassezia's ecological competitiveness. Another clue found a decreased relative abundance of Malassezia as the population of S. aureus rise in AD flares (Chng et al., 2016). Noteworthy, Malassezia species (such as Malassezia dermatitis and Malassezia sympodialis) were enriched in AD-prone skin by whole metagenome profiling (Casagrande et al., 2006; Chng et al., 2016), suggesting that the relative abundance of Malassezia species shifts in association with the AD disease course. Additionally, a recent study shows that Malassezia globosa can secrete protease that attenuates S. aureus biofilm associated with increased severity and barrier dysfunction of the progression of AD (Li et al., 2018).
Additional clinical studies showed that the composition of the skin microbiota is different in pediatric vs. adult AD (Shi et al., 2016), suggesting that the disease prevalence of AD may have a linkage with age. AD most often develops in early childhood, and up to 70% of AD children demonstrate clearing of the disease or a spontaneous resolution until late childhood even in patients with FLG mutations (Margolis et al., 2014; Mortz et al., 2015; Bieber et al., 2017). AD can persist into adulthood in certain AD children or begin in adulthood (Garmhausen et al., 2013). The most recent estimate of prevalence of AD in the adult population is ~10% (Weidinger et al., 2018). In terms of the overall diversity of the skin microbiota, AD is significantly lower in adults than in children. Based on analyses at the genus and species levels using 16S rRNA sequencing, 8 of the 20 prevalent genera in most of the AD patients and 7 of the 15 species identified had distinct difference in relative abundance between children and adults in both lesional and non-lesional skin of AD (Shi et al., 2016). In a separate study, whole metagenome sequencing analysis that compared the non-flare skin of AD adults with that of a control cohort revealed skin microbiome-dependent susceptibility in AD flares and identified a distinct AD-associated skin microbiota signature, which had an enrichment of Streptococcus and Gemella reported to be originally enriched in children and a depletion of Dermacoccus often present on healthy human skin (Oh et al., 2012; Chng et al., 2016; Kennedy et al., 2017).
Skin Microbiota Constituents in AV
AV (also commonly called acne) is one of the most ubiquitary non-communicable inflammatory dermatoses of the pilosebaceous unit (Williams et al., 2012; Lichtenberger et al., 2017), characterized by both specific localization on skin regions of abundant sebaceous glands (such as the face, neck, and back) and manifestations within a narrow age range in association with adolescence followed by frequent resolution (Szegedi et al., 2019). AV affects up to 85% of adolescents of varying ethnicities and persist into adulthood with a prevalence in 11% (Cunliffe and Gould, 1979; James, 2005; Jahns et al., 2012). The severity of AV clinical symptoms depends on the number of non-inflammatory lesions (closed and open comedones), inflammatory lesions (pustules and papules), and the residual pathology of nodules and cysts (Shalita, 2004; Ghodsi et al., 2009). Severe manifestations of AV not only have an impact on patients' physical and psychological health including abscess, permanent scarring, and depression (Zaenglein et al., 2016) but also produce social handicaps such as higher rates of unemployment (Lichtenberger et al., 2017). Although the etiology and pathogenesis of AV are not well-understood, multifaceted involvements encompassing androgen-induced increased sebum secretion and sebaceous gland proliferation, hypercornification of the pilosebaceous follicle, inflammation involving innate and acquired immunity response, skin microbiota colonization and proliferation in the pilosebaceous unit, and external factors and genetics are considered as the mechanisms contributing to the development of AV (Agak et al., 2018; O'Neill and Gallo, 2018; Chien et al., 2019).
However, with the further development of sequencing technology and the current understanding of AV pathogenesis continuously evolving, skin microbiota are considered to play a major role in the occurrence of acne. Several notable observations that the composition of the skin microbiota in acne is disturbed highlight the importance of studying diseases in the context of the microbial dysbiosis (Lomholt and Kilian, 2010; Fitz-Gibbon et al., 2013). In particular, Cutibacterium acnes, the most abundant commensal (up to 90%) in the skin-residing microorganisms of healthy adults, has been viewed as an important pathogenic factor accompanying the development of AV (Melnik, 2015; Barnard et al., 2016; Lomholt et al., 2017). In a case–control study utilizing immunofluorescent labeling to visualize different C. acnes phylotypes in macrocolonies/biofilms in sebaceous follicles of facial skin biopsies from AV patients, AV development was unequivocally linked with the occurrence and localization of C. acnes in follicles, and the formation of C. acnes biofilms attached to the hair shaft and follicular epithelial wall increased morbidity of AV patients (Jahns et al., 2012). A recent comprehensive review proposes a new concept that AV may be a naturally developing, transient inflammatory interplay of adolescent facial skin with its new microbiota (C. acnes), replacing a state of previous skin homeostasis in childhood (Szegedi et al., 2019). It is worth noting that initial attempts failed to consolidate the relationship between AV and the skin microflora. Almost all adults are colonized with C. acnes, but only a minority suffer from acne. Comparing the makeup of the skin microbial communities between control and AV-prone groups by next-generation sequencing technology, the results demonstrated the relative abundance of C. acnes tending to be consistent in both, which also implied that the skin microbiota might not play a role in AV after all (Sohn, 2018).
In contrast to the interspecies dysbiosis of skin microbiota in AD, the distortion of AV is caused by the shifts of intraspecies population structures of skin bacterium C. acnes (Fitz-Gibbon et al., 2013). Comparing the population structure of C. acnes strains in pilosebaceous units from 48 acne patients and 51 normal individuals using a combination of metagenomics and genome sequencing, metagenomic data analysis demonstrated that the strain-level distribution of C. acnes was different enough to distinguish acne skin from healthy skin (Figure 3; Fitz-Gibbon et al., 2013). Moreover, the severity of acne caused by C. acnes is typically strain specific (Allhorn et al., 2016). According to the top 10 most abundant ribotypes (RT) showing healthy and acne-specific associations, the three most abundant ribotypes (RT1, RT2, and RT3) had a similar relative abundance and evenly distributed in acne and normal pilosebaceous units (Fitz-Gibbon et al., 2013; O'Neill and Gallo, 2018). However, RT4 and RT5 were significantly enriched in up to 40% of AV individuals but rarely found in normal individuals. In contrast, RT6 was found to be enriched in 99% of individuals with healthy skin. At the clade level, RT4 and RT5 are categorized as the type IA-2 phylogroup that have been consistently associated with acne by increasing inflammation based on the presence of putative virulence factors (Fitz-Gibbon et al., 2013; O'Neill and Gallo, 2018). At genome and function level, the potential genetic elements and gene expression profiles of healthy and acne-associated C. acnes strains were revealed. The unique genome regions of acne-enriched RT4 and RT5 strains are implicated in a linear plasmid (loci 3) encoding a tight adhesion (Tad) locus in relation to virulence affecting bacterial adhesion and host immune responses and two unique loci of genomic islands (loci 1 and 2) encoding a Sag gene cluster in association with hemolytic activity in pathogens (Fuller et al., 2002; Humar et al., 2002; Tomich et al., 2007; Fitz-Gibbon et al., 2013; Kasimatis et al., 2013). All the genomes of healthy-enriched RT6 strain can encode Clustered Regularly Interspaced Short Palindromic Repeats (CRISPRs), which are present in health-associated type II C. acnes phenotypes, confer protective immunity against the invasion of viruses, phages, and plasmids or other exotic DNA, and avoid the acquisition of extra genetic elements that foster virulence and acne pathogenesis (Bolotin et al., 2005; Brouns et al., 2008; Bruggemann et al., 2012; O'Neill and Gallo, 2018). These are consistent with the results obtained from the strain-level distribution of C. acnes.
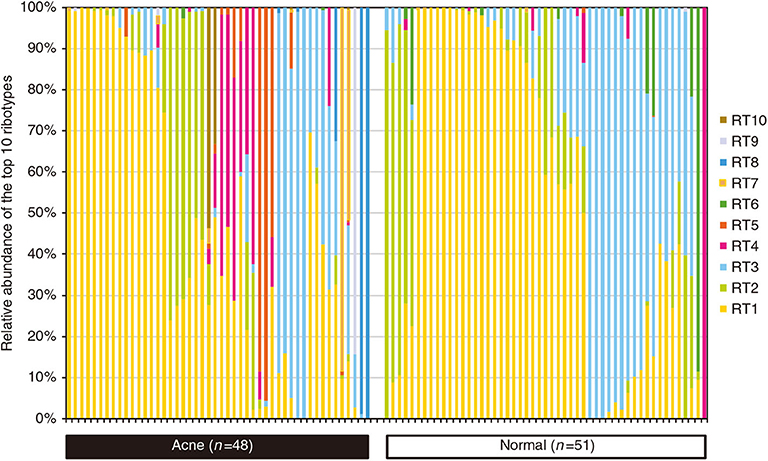
Figure 3. Percentage of relative abundance of diverse C. acnes strains in the pilosebaceous units between acne patients and normal individuals. C. acnes predominates the microbiota of pilosebaceous units, accounting for up to 90%. There was no significant difference in the relative abundance of C. acnes between acne patients and normal individuals. However, the distributions of distinct C. acnes strain populations in acne and normal skin are dramatically different. Dysbiosis in acne vulgaris (AV) is characterized by a decrease in the proportion of C. acnes strains RT6 and the significant increase in the proportion of C. acnes strains RT4, RT5, RT7, RT8, RT9, and RT10. Reprinted with permission from Fitz-Gibbon et al. (2013). Copyright (2013) The Society for Investigative Dermatology, Inc.
Applications for Ecology-Based Therapy
The understanding of the essential role of the homeostasis and dysbiosis of skin microbiota in skin disorders is rapidly expanding, with a major contributory factor being the potentiated awareness of the cutaneous ecosystem (Grice and Segre, 2011). Although there is no consistent definition of a healthy cutaneous ecosystem, some of the main parameters, such as microbial composition, diversity, and stability, have been established as key markers of homeostasis (Vandegrift et al., 2017). Disturbances of the skin microbiota have been directly correlated with multiple diseases. As a result, the novel concept of restoring the composition and functionality of the skin microbiota to the previous indigenous state by targeted manipulation of the skin microbiota, on the basis of the skin ecosystem, has been proposed as a potential therapeutic approach in the last few years (Paetzold et al., 2019; Stacy and Belkaid, 2019). As initial studies of microbiota focused on the gut, which has the largest microbial community in the human body, FMT, a synthetic reproduction of the complex gut ecosystem remaining as most effective therapeutic option with remarkable safety performance, has been applied into clinical practice although not approved by the US Food and Drug Administration (FDA) for clinical use (Cammarota et al., 2017; Allegretti et al., 2019). At present, reintroduction of living microbiota (a single or cocktail of microbiota) to modulate skin microbiota composition from disease microbiota states to healthy ones may represent a valid target for ecology-based therapy (Stacy and Belkaid, 2019). Recent advances in unlocking a key understanding of the cellular mechanisms through which the microbiota implement both the establishment and restoration of cutaneous homeostasis highlight three indispensable essential interactions (Figure 4): (i) antimicrobial peptides (AMPs) or other metabolites produced by reintroduction of a single of microbiota directly inhibit or kill pathogenic microorganisms (Nakatsuji et al., 2017, 2018; Williams et al., 2019); (ii) reintroduction of living microbiota induces keratinocytes and sebocytes to produce AMPs to shape microbial communities (Nagy et al., 2005, 2006; Naik et al., 2015); and (iii) reintroduction of a cocktail of microbiota has a synergistic effect on ameliorating the ecology of skin microbial communities (Paetzold et al., 2019). However, the extensive communication at the molecular level in the ecology of skin microbial communities is not well-understood, especially the intercellular communication of multidirectional signals. Although research on the skin microbiota lags behind studies of gut flora, there are already some applications for ecology-based therapy with the aim of correcting the imbalances on the cutaneous ecosystem.
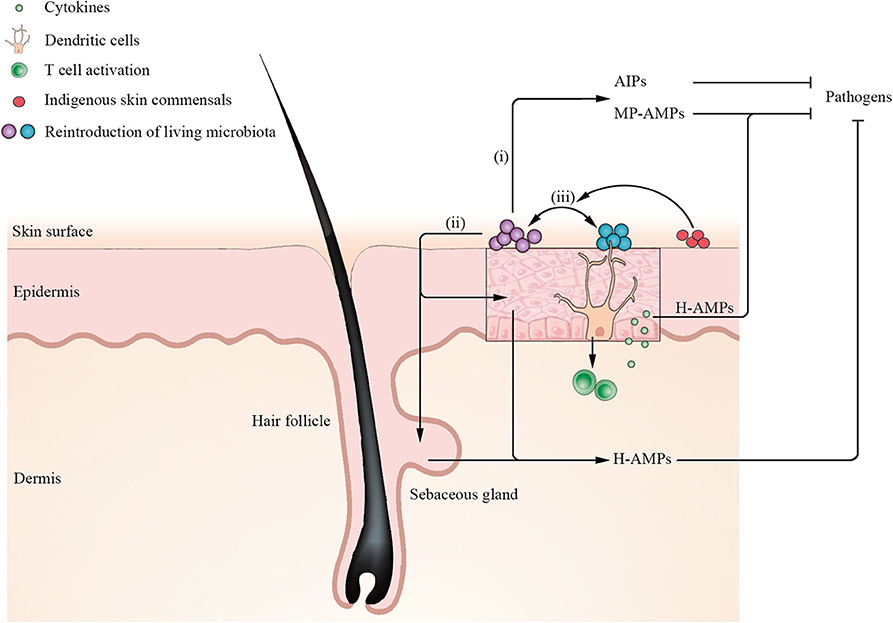
Figure 4. Mechanisms for establishment and restoration of cutaneous homeostasis by reintroduction of living microbiota. Application of a single of microbiota such as S. hominis secretes microbiota-produced antimicrobial peptides (MP-AMPs) or other metabolites [such as autoinducing peptides (AIPs)] to inhibit pathogen S. aureus. Moreover, reintroduction of living microbiota induces keratinocytes and sebocytes to secrete human AMPs (H-AMPs) to directly inhibit pathogens. Notably, dendritic cells (DCs) extend their dendrites into the stratum corneum to acquire microbial antigens and active T cells to release cytokines. Keratinocytes-produced H-AMPs LL-37 induced by cytokines synergize with MP-MAPs (such as S. epidermidis lantibiotics), which can kill S. aureus more effectively. In addition, reintroduction of a cocktail of microbiota has a synergistic effect on ameliorating the ecology of skin microbial communities. When certain indigenous skin commensals are rich in recipient skin, reintroduction of a cocktail of microbiota has a higher engraftment.
As mentioned earlier, S. aureus has been implicated in the pathogenesis of AD, and the clinical severity of AD is positively correlated with carriage of S. aureus. Therefore, defeating the microbes in competition might contribute to treating the cutaneous disorder. Nakatsuji et al. (2017) isolated the CoNS collected from the skin of normal and AD individuals using high-throughput screening for antimicrobial activity against S. aureus. The predominant species of CoNS with antimicrobial activity were identified as S. epidermidis and Staphylococcus hominis, although many strains of the CoNS species did not produce antimicrobial activity. A single application of S. hominis A9 with anti-S. aureus activity confirmed a significant decrease in S. aureus colonizing mice and pig skin, comparing with application of S. hominis isolates without antimicrobial activity. Then, reintroduction of antimicrobial CoNS strains to human skin of AD subjects diminished S. aureus colonization. The interspecies interaction largely depends on antimicrobial CoNS strains producing novel AMPs (such as lantibiotic, bacteriocin, and lugdunin) and synergizing with microbiota-produced AMPs and the human AMP LL-37 or/and DCD-1(L) to selectively kill or inhibit S. aureus (Figure 4; Lee et al., 2008; Nakatsuji et al., 2017; Bitschar et al., 2019). Subsequently, Gallo et al. (Sohn, 2018) has devised a cream that contains antimicrobial CoNS strains isolated from human skin to inhibit S. aureus. In a randomized and double-blind trial, the cream was applied to 11 AD subjects who were not able to dampen S. aureus before accepting Gallo's intervention. The result uncovered a more than 90% decrease in the number of S. aureus on their skin by a single application. In follow-up studies, it was revealed that the colonization by S. aureus was almost completely eliminated, and clinical symptoms in AD severity declined by up to 30% when the product was applied twice a day for a week. Then, he has founded a company with the aim of bringing this product to market in the next 2–3 years. This is also basically the first time that a microbial transplantation on human skin has been useful for treatment (Sohn, 2018).
The molecular mechanism of accessory gene regulatory (agr) quorum sensing system plays a pivotal role in the orchestration of cellular behavior between CoNS species and S. aureus via an autoinducing peptide (AIP) signaling molecule. The full length of AIP is composed of 7–12 amino acids with the last 5 amino acids forming a cyclic (thio)lactone ring between the C-terminal and a cysteine or serine side chain (Janek et al., 2016; Zipperer et al., 2016; Nakatsuji et al., 2017). Prior studies from Dr Richard Novick's group have established the central role of agr quorum sensing signaling in S. aureus skin pathogenesis by several notable observations (Parlet et al., 2019), implying that the quorum sensing interaction may lead to disease or promote remissions. Otto et al. (2001) and Olson et al. (2014) applied the CoNS strains S. epidermidis agr type I to successfully suppress S. aureus agr function. Recently, Paharik et al. (2017) found that the CoNS species Staphylococcus caprae isolated from goat milk secreted an AIP (YSTCSYYF) that could block the agr-mediated quorum sensing of all classes of S. aureus (type I–IV) to prohibit their colonization and skin infection. Although a number of animal CoNS species have been identified to have inhibitory effects on S. aureus quorum sensing, there are still few studies on quorum sensing between CoNS species on human skin. In a more recent study, Williams et al. (2019) applied the CoNS strains collected from human subjects to identify the molecular mechanism for the effects of S. aureus on the epidermal barrier disruption in the development of AD and further determine how dysbiosis on the skin surface permits the bacteria to induce inflammation. Under the control of the agr quorum sensing system, the human CoNS species produced AIP to suppress the expression of S. aureus PSMα to prevent S. aureus-mediated epithelial damage and inflammation. In addition, the topical application of live CoNS strain S. hominis C5 producing an AIP (SYNVCGGYF) to murine skin resulted in potent inhibition of S. aureus agr activity, demonstrating that the human skin microbiota can contribute to epithelial barrier homeostasis by using quorum sensing to dampen S. aureus virulence factors (Williams et al., 2019).
Previous studies have illustrated that dysbiosis may lead to carcinogenesis. For instance, the abnormal proliferation of Fusobacterium nucleatum in the gut has been linked to colorectal cancer through their adherence and invasion into intestinal epithelial cells, ultimately resulting in increased oncogenic and inflammatory responses (Song et al., 2019), which suggests that certain members of human skin microbiota may curb tumor growth. Recently, Nakatsuji et al. (2018) found that a strain of S. epidermidis, which is common on human skin, produces 6-N-hydroxyaminopurine (6-HAP) that has selective antiproliferative activity against tumor lines by interfering with the essential process of DNA replication but did not inhibit primary keratinocytes. A single application of S. epidermidis strain producing 6-HAP to mouse impaired the incidence of ultraviolet-induced skin tumors. However, further research is needed to validate whether the dysbiosis caused by the loss of such strains elevates the risk of human skin cancer.
In addition to CoNS, the potential role of another Gram-negative skin bacteria in AD is also investigated. Roseomonas mucosa is one particular commensal found on the skin of healthy and AD individuals (Paller et al., 2019). In mouse and cell culture models of AD, R. mucosa isolated from healthy individuals improved the prognosis, but those collected from AD patients worsened the prognosis, whose interventions of targeting the microbiome could provide therapeutic benefit for AD patients (Myles et al., 2018). Subsequently, Myles et al. (2018) tested the therapeutic potential of topical live R. mucosa in humans for the first time. In an open-label phase I/II safety and activity trial in 10 adult and 5 pediatric patients, topical microbiome transplantation with R. mucosa led to a prominent reduction in AD severity and S. aureus burden without any negative reactions or treatment complications. Besides, metabolites of R. mucosa on the non-lesional and lesional skin of AD patients demonstrated that differences at strain level might contribute to disease pathology. These observations mirror AD treatment effects of other skin bacteria present in healthy and AD individuals but lack known cellular and molecular mechanisms.
Human AMPs (such as cathelicidins and β-defensins) are produced by keratinocytes and sebocytes to potentiate the skin resistance against pathogens. Except for the constitutively expressed AMPs, others can be agitated by cues from certain members of the skin microbiota, a phenomenon known as heterologous protection. For example, these cues can be directly stimulated by S. epidermidis TLR2 signaling or induced by activation of S. epidermidis-specific IL-17+CD8+ T cells that confer protection against skin infection by inducing keratinocytes to secrete cathelicidin and kill distinct pathogens (Figure 4; Naik et al., 2015). Furthermore, S. epidermidis decreases the anionic charge of its surface and membrane by an AMP-sensing system called aps, thereby avoiding harmful effects on itself via decreased attraction or repulsion of human AMPs (Otto, 2009). In another in vitro study, two clinical isolates of C. acnes type IA and IB significantly induced the expression of β-defensin-2 in human sebocytes and keratinocytes (Nagy et al., 2005, 2006). The additional observation that distinct strains of C. acnes may differ in contribution to the pathogenesis of AV raises the exciting possibility that investigators need to identify C. acnes at the strain level with a greater potential to be implicated in the condition (Nagy et al., 2006).
In contrast to transplantation of a single microbiota, reintroduction of a cocktail of microbiota has a greater advantage in the level of engraftment. As investigators usually focus only on the most critical microbe that causes disease, dysbiosis on the surface of skin is thought to be the loss and increase in a single commensal microbe rather than a mix of different skin microbiota. Paetzold et al. (2019) prepared probiotic solutions that were mixtures of different skin microbiome components taken from a healthy donor to modulate the subpopulation of skin microbiota on sebaceous-gland-rich skin regions. After sequential applications of probiotic solutions containing different combinations from one strain to multiple strains of C. acnes on 18 subjects in the first 3 days, the observation demonstrated that the level of engraftment was positively correlated with the presence of several different strains in the probiotic solution, implicating that different strain combinations have synergistic effects on colonization of recipient skin surface and modulation of the C. acnes population at the strain level is feasible by microbiome transplantation. Notably, patients with C. acnes subtype H1 and Leifsonia being more abundant showed a markedly higher transplantation by using a multistrain donor solution, suggesting that different microbiota might have a synergistic effect (Figure 4; Paetzold et al., 2019). However, the correlation of multidirectional signals in the ecology of skin microbiota is poorly understood.
Concluding Remarks and Future Perspectives
The examples discussed herein illustrate that determining the fate of the healthy or disease states of skin may need to be updated to include the skin microbiota constituents. The available evidence has clearly indicated that the dysbiosis of cutaneous microbiota is substantially associated with multiple skin disorders, and the degree of disorder has a positive correlation with the severity of skin disease. One limitation of the current study is that the skin microbiota in dysbiosis do not differentiate well from that in healthy states. This information would provide a supporting evidence that the differences in species- and strain-level composition for the effects on the host and other skin microbiota have been largely unexplored, only mainly focusing on common skin diseases such as AD and AV (Fitz-Gibbon et al., 2013; Nakatsuji et al., 2017). Thus, further studies are needed to establish standards for skin microbial healthy states and to conduct a broader and more accurate analysis to determine the differences in multiple levels and functions of skin microbiota between dysbiosis and healthy conditions. In addition, advanced sequencing technology makes it possible to provide an analysis of the skin microbiota composition in healthy or disease states at previously unexplored resolution even including the strain level within a species (Byrd et al., 2018). With increasing identification of highly personalized skin microbial communities (Oh et al., 2016), the presence of specific strains in patients emphasizes the importance of the individuality in the disease process and in response to diagnosis and treatment, which may provide an opportunity for precision medicine in the field of skin microbiota.
Due to the high prevalence and ability to affect physical and psychological health for human (Williams et al., 2012; Weidinger and Novak, 2016), certain common cutaneous diseases related to skin microbiota remain a frontier for research. While conventional antimicrobial therapies such as oral and topical antibiotic remain the primary therapeutic method for sufferers with the cutaneous disorders, indiscriminately targeting skin microbiota and eliminating important indigenous commensal microbes remain a major drawback (O'Neill and Gallo, 2018). Recent advances, showing that the application of natural bacteria to the human skin modulates skin microbiota composition, underscore the importance for the manipulation in the indigenous microbiota of patients and highlight an opportunity to develop an ecology-based therapeutic modality that specifically target the invading pathogens while preserving indigenous commensal bystanders in diseases affecting the skin (Parlet et al., 2019; Stacy and Belkaid, 2019). As we learn more about how commensal microbiota shape the cutaneous ecosystem, we may be able to leverage this specificity by the reintroduction of living microbiota to govern the skin homeostasis. Moreover, not all strains of one species have antimicrobial activity, and the ability of inhibiting microbiota between active strains are different (Williams et al., 2019). Understanding reintroduction of living microbiota at the strain level may be critically important for correcting dysbiosis. Furthermore, it is not clear how microbiome transplantation shapes microbial communities on diseased skin via cell-to-cell communication, but the complexity of communication from intraspecies to interkingdom dense network does play an important role in maintaining homeostasis of the cutaneous ecosystem. For instance, an AIP from a CoNS species found on human skin can suppress the growth of S. aureus by interspecies agr quorum sensing mechanisms to protect against epidermal injury in AD, and application of a synthetic AIP to mouse skin colonized with S. aureus inhibited agr activity (Williams et al., 2019). It is possible that heightened understanding of the communication network of skin microbiota in molecular mechanisms will contribute to the amelioration of dysbiosis and may provide more targeted therapies for dysbiosis.
Moving forward, it is clear that the technique of skin microbiome transplantation is only at the initial stages. A temporary modulation of the skin microbiota population at the species and strain level is feasible in patients with skin disorders without an adverse event. The application of living microbiota is largely limited by in vitro models (such as murine skin models) that differ immunologically and structurally from the human cutaneous milieu. As investigations in the field grows, efforts will be needed to identify the efficacy of long-term application of living microbiota onto epidermal models (Williams et al., 2019) and provide a promising in vitro model for human therapeutic applications (Summerfield et al., 2015; Bitschar et al., 2019).
Author Contributions
WL and ZL conceived the structure of the review. HZ drafted the manuscript. LS, YR, and XT carried out revisions of the manuscript. All authors read and approved the final manuscript. All authors contributed to the article and approved the submitted version.
Funding
This work was supported by the National Natural Science Foundation of China (31470968).
Conflict of Interest
The authors declare that the research was conducted in the absence of any commercial or financial relationships that could be construed as a potential conflict of interest.
Acknowledgments
We would like to thank all the members of Liu Group who gave valuable suggestions on this manuscript.
References
Agak, G. W., Kao, S., Ouyang, K., Qin, M., Moon, D., Butt, A., et al. (2018). Phenotype and antimicrobial activity of Th17 cells induced by propionibacterium acnes strains associated with healthy and acne skin. J. Invest. Dermatol. 138, 316–324. doi: 10.1016/j.jid.2017.07.842
Allegretti, J. R., Mullish, B. H., Kelly, C., and Fischer, M. (2019). The evolution of the use of faecal microbiota transplantation and emerging therapeutic indications. Lancet 394, 420–431. doi: 10.1016/S0140-6736(19)31266-8
Allhorn, M., Arve, S., Bruggemann, H., and Lood, R. (2016). A novel enzyme with antioxidant capacity produced by the ubiquitous skin colonizer Propionibacterium acnes. Sci. Rep. 6:36412. doi: 10.1038/srep36412
Barnard, E., Shi, B., Kang, D., Craft, N., and Li, H. (2016). The balance of metagenomic elements shapes the skin microbiome in acne and health. Sci. Rep. 6:39491. doi: 10.1038/srep39491
Belkaid, Y., and Segre, J. A. (2014). Dialogue between skin microbiota and immunity. Science 346, 954–959. doi: 10.1126/science.1260144
Bieber, T., D'Erme, A. M., Akdis, C. A., Traidl-Hoffmann, C., Lauener, R., Schappi, G., et al. (2017). Clinical phenotypes and endophenotypes of atopic dermatitis: where are we, and where should we go? J. Allergy Clin. Immunol. 139, S58–S64. doi: 10.1016/j.jaci.2017.01.008
Bitschar, K., Sauer, B., Focken, J., Dehmer, H., Moos, S., Konnerth, M., et al. (2019). Lugdunin amplifies innate immune responses in the skin in synergy with host- and microbiota-derived factors. Nat. Commun. 10:2730. doi: 10.1038/s41467-019-10646-7
Bolotin, A., Quinquis, B., Sorokin, A., and Ehrlich, S. D. (2005). Clustered regularly interspaced short palindrome repeats (CRISPRs) have spacers of extrachromosomal origin. Microbiology 151, 2551–2561. doi: 10.1099/mic.0.28048-0
Bosi, E., Monk, J. M., Aziz, R. K., Fondi, M., Nizet, V., and Palsson, B. O. (2016). Comparative genome-scale modelling of Staphylococcus aureus strains identifies strain-specific metabolic capabilities linked to pathogenicity. Proc. Natl. Acad. Sci. U.S.A. 113, E3801–E3809. doi: 10.1073/pnas.1523199113
Brouns, S. J., Jore, M. M., Lundgren, M., Westra, E. R., Slijkhuis, R. J., Snijders, A. P., et al. (2008). Small CRISPR RNAs guide antiviral defense in prokaryotes. Science 321, 960–964. doi: 10.1126/science.1159689
Bruggemann, H., Lomholt, H. B., Tettelin, H., and Kilian, M. (2012). CRISPR/cas loci of type II Propionibacterium acnes confer immunity against acquisition of mobile elements present in type I P. acnes. PLoS ONE 7:e34171. doi: 10.1371/journal.pone.0034171
Byrd, A. L., Belkaid, Y., and Segre, J. A. (2018). The human skin microbiome. Nat. Rev. Microbiol. 16, 143–155. doi: 10.1038/nrmicro.2017.157
Byrd, A. L., Deming, C., Cassidy, S. K. B., Harrison, O. J., Ng, W. I., Conlan, S., et al. (2017). Staphylococcus aureus and Staphylococcus epidermidis strain diversity underlying pediatric atopic dermatitis. Sci. Transl. Med. 9:eaal4651. doi: 10.1126/scitranslmed.aal4651
Cammarota, G., Ianiro, G., Tilg, H., Rajilic-Stojanovic, M., Kump, P., Satokari, R., et al. (2017). European consensus conference on faecal microbiota transplantation in clinical practice. Gut 66, 569–580. doi: 10.1136/gutjnl-2016-313017
Casagrande, B. F., Fluckiger, S., Linder, M. T., Johansson, C., Scheynius, A., Crameri, R., et al. (2006). Sensitization to the yeast Malassezia sympodialis is specific for extrinsic and intrinsic atopic eczema. J. Invest. Dermatol. 126, 2414–2421. doi: 10.1038/sj.jid.5700431
Chaptini, C., Quinn, S., and Marshman, G. (2016). Methicillin-resistant Staphylococcus aureus in children with atopic dermatitis from 1999 to 2014: a longitudinal study. Australas. J. Dermatol. 57, 122–127. doi: 10.1111/ajd.12371
Chen, Y. E., Fischbach, M. A., and Belkaid, Y. (2018). Skin microbiota-host interactions. Nature 553, 427–436. doi: 10.1038/nature25177
Chien, A. L., Tsai, J., Leung, S., Mongodin, E. F., Nelson, A. M., Kang, S., et al. (2019). Association of systemic antibiotic treatment of acne with skin microbiota characteristics. JAMA Dermatol. 155, 425–434. doi: 10.1001/jamadermatol.2018.5221
Chng, K. R., Tay, A. S. L., Li, C., Ng, A. H. Q., Wang, J., Suri, B. K., et al. (2016). Whole metagenome profiling reveals skin microbiome-dependent susceptibility to atopic dermatitis flare. Nat. Microbiol. 1:106. doi: 10.1038/nmicrobiol.2016.106
Conti, F., Ceccarelli, F., Iaiani, G., Perricone, C., Giordano, A., Amori, L., et al. (2016). Association between Staphylococcus aureus nasal carriage and disease phenotype in patients affected by systemic lupus erythematosus. Arthritis Res. Ther 18:177. doi: 10.1186/s13075-016-1079-x
Cunliffe, W. J., and Gould, D. J. (1979). Prevalence of facial acne vulgaris in late adolescence and in adults. Br. Med. J. 1, 1109–1110. doi: 10.1136/bmj.1.6171.1109
Darabi, K., Hostetler, S. G., Bechtel, M. A., and Zirwas, M. (2009). The role of Malassezia in atopic dermatitis affecting the head and neck of adults. J. Am. Acad. Dermatol. 60, 125–136. doi: 10.1016/j.jaad.2008.07.058
Dorrestein, P. C., Gallo, R. L., and Knight, R. (2016). Microbial skin inhabitants: friends forever. Cell 165, 771–772. doi: 10.1016/j.cell.2016.04.035
Drago, L., De Grandi, R., Altomare, G., Pigatto, P., Rossi, O., and Toscano, M. (2016). Skin microbiota of first cousins affected by psoriasis and atopic dermatitis. Clin. Mol. Allergy 14:2. doi: 10.1186/s12948-016-0038-z
Dreno, B., Araviiskaia, E., Berardesca, E., Gontijo, G., Sanchez Viera, M., Xiang, L. F., et al. (2016). Microbiome in healthy skin, update for dermatologists. J. Eur. Acad. Dermatol. Venereol. 30, 2038–2047. doi: 10.1111/jdv.13965
Eyerich, K., Eyerich, S., and Biedermann, T. (2015). The multi-modal immune pathogenesis of atopic eczema. Trends Immunol 36, 788–801. doi: 10.1016/j.it.2015.10.006
Findley, K., Oh, J., Yang, J., Conlan, S., Deming, C., Meyer, J. A., et al. (2013). Topographic diversity of fungal and bacterial communities in human skin. Nature 498, 367–370. doi: 10.1038/nature12171
Fitz-Gibbon, S., Tomida, S., Chiu, B. H., Nguyen, L., Du, C., Liu, M., et al. (2013). Propionibacterium acnes strain populations in the human skin microbiome associated with acne. J. Invest. Dermatol. 133, 2152–2160. doi: 10.1038/jid.2013.21
Fuller, J. D., Camus, A. C., Duncan, C. L., Nizet, V., Bast, D. J., Thune, R. L., et al. (2002). Identification of a streptolysin S-associated gene cluster and its role in the pathogenesis of Streptococcus iniae disease. Infect. Immun. 70, 5730–5739. doi: 10.1128/IAI.70.10.5730-5739.2002
Gallo, R. L. (2017). Human skin is the largest epithelial surface for interaction with microbes. J. Invest. Dermatol. 137, 1213–1214. doi: 10.1016/j.jid.2016.11.045
Garmhausen, D., Hagemann, T., Bieber, T., Dimitriou, I., Fimmers, R., Diepgen, T., et al. (2013). Characterization of different courses of atopic dermatitis in adolescent and adult patients. Allergy 68, 498–506. doi: 10.1111/all.12112
Geoghegan, J. A., Irvine, A. D., and Foster, T. J. (2018). Staphylococcus aureus and atopic dermatitis: a complex and evolving relationship. Trends Microbiol. 26, 484–497. doi: 10.1016/j.tim.2017.11.008
Ghodsi, S. Z., Orawa, H., and Zouboulis, C. C. (2009). Prevalence, severity, and severity risk factors of acne in high school pupils: a community-based study. J. Invest. Dermatol. 129, 2136–2141. doi: 10.1038/jid.2009.47
Gonzalez, M. E., Schaffer, J. V., Orlow, S. J., Gao, Z., Li, H., Alekseyenko, A. V., et al. (2016). Cutaneous microbiome effects of fluticasone propionate cream and adjunctive bleach baths in childhood atopic dermatitis. J. Am. Acad. Dermatol. 75, 481–493.e488. doi: 10.1016/j.jaad.2016.04.066
Goodman, A. L., Kallstrom, G., Faith, J. J., Reyes, A., Moore, A., Dantas, G., et al. (2011). Extensive personal human gut microbiota culture collections characterized and manipulated in gnotobiotic mice. Proc. Natl. Acad. Sci. U.S.A. 108, 6252–6257. doi: 10.1073/pnas.1102938108
Grice, E. A., Kong, H. H., Conlan, S., Deming, C. B., Davis, J., Young, A. C., et al. (2009). Topographical and temporal diversity of the human skin microbiome. Science 324, 1190–1192. doi: 10.1126/science.1171700
Grice, E. A., and Segre, J. A. (2011). The skin microbiome. Nat. Rev. Microbiol. 9, 244–253. doi: 10.1038/nrmicro2537
Grogan, M. D., Bartow-McKenney, C., Flowers, L., Knight, S. A. B., Uberoi, A., and Grice, E. A. (2019). Research techniques made simple: profiling the skin microbiota. J. Invest. Dermatol. 139, 747–752.e741. doi: 10.1016/j.jid.2019.01.024
Guttman-Yassky, E., Bissonnette, R., Ungar, B., Suarez-Farinas, M., Ardeleanu, M., Esaki, H., et al. (2019). Dupilumab progressively improves systemic and cutaneous abnormalities in patients with atopic dermatitis. J. Allergy Clin. Immunol. 143, 155–172. doi: 10.1016/j.jaci.2018.08.022
Havlickova, B., Czaika, V. A., and Friedrich, M. (2008). Epidemiological trends in skin mycoses worldwide. Mycoses 51(Suppl 4), 2–15. doi: 10.1111/j.1439-0507.2008.01606.x
Hsiang, M. S., Shiau, R., Nadle, J., Chan, L., Lee, B., Chambers, H. F., et al. (2012). Epidemiologic similarities in pediatric community-associated methicillin-resistant and methicillin-sensitive Staphylococcus aureus in the san francisco bay area. J. Pediatr. Infect. Dis. Soc. 1, 200–211. doi: 10.1093/jpids/pis061
Humar, D., Datta, V., Bast, D. J., Beall, B., De Azavedo, J. C. S., and Nizet, V. (2002). Streptolysin S and necrotising infections produced by group G streptococcus. Lancet 359, 124–129. doi: 10.1016/S0140-6736(02)07371-3
Jahns, A. C., Lundskog, B., Ganceviciene, R., Palmer, R. H., Golovleva, I., Zouboulis, C. C., et al. (2012). An increased incidence of Propionibacterium acnes biofilms in acne vulgaris: a case-control study. Br. J. Dermatol. 167, 50–58. doi: 10.1111/j.1365-2133.2012.10897.x
Janek, D., Zipperer, A., Kulik, A., Krismer, B., and Peschel, A. (2016). High frequency and diversity of antimicrobial activities produced by nasal staphylococcus strains against bacterial competitors. PLoS Pathog 12:e1005812. doi: 10.1371/journal.ppat.1005812
Jo, J. H., Kennedy, E. A., and Kong, H. H. (2016). Research techniques made simple: bacterial 16S ribosomal RNA gene sequencing in cutaneous research. J. Invest. Dermatol. 136, e23–e27. doi: 10.1016/j.jid.2016.01.005
Kasimatis, G., Fitz-Gibbon, S., Tomida, S., Wong, M., and Li, H. (2013). Analysis of complete genomes of Propionibacterium acnes reveals a novel plasmid and increased pseudogenes in an acne associated strain. Biomed Res. Int. 2013:918320. doi: 10.1155/2013/918320
Kennedy, E. A., Connolly, J., Hourihane, J. O., Fallon, P. G., McLean, W. H. I., Murray, D., et al. (2017). Skin microbiome before development of atopic dermatitis: early colonization with commensal staphylococci at 2 months is associated with a lower risk of atopic dermatitis at 1 year. J. Allergy Clin. Immunol. 139, 166–172. doi: 10.1016/j.jaci.2016.07.029
Kobayashi, T., Glatz, M., Horiuchi, K., Kawasaki, H., Akiyama, H., Kaplan, D. H., et al. (2015). Dysbiosis and Staphylococcus aureus colonization drives inflammation in atopic dermatitis. Immunity 42, 756–766. doi: 10.1016/j.immuni.2015.03.014
Kong, H. H., Oh, J., Deming, C., Conlan, S., Grice, E. A., Beatson, M. A., et al. (2012). Temporal shifts in the skin microbiome associated with disease flares and treatment in children with atopic dermatitis. Genome Res. 22, 850–859. doi: 10.1101/gr.131029.111
Kong, H. H., and Segre, J. A. (2012). Skin microbiome: looking back to move forward. J. Invest. Dermatol. 132, 933–939. doi: 10.1038/jid.2011.417
Lax, S., Sangwan, N., Smith, D., Larsen, P., Handley, K. M., Richardson, M., et al. (2017). Bacterial colonization and succession in a newly opened hospital. Sci Transl. Med. 9:eaah6500. doi: 10.1126/scitranslmed.aah6500
Lee, D. Y., Yamasaki, K., Rudsil, J., Zouboulis, C. C., Park, G. T., Yang, J. M., et al. (2008). Sebocytes express functional cathelicidin antimicrobial peptides and can act to kill propionibacterium acnes. J. Invest. Dermatol. 128, 1863–1866. doi: 10.1038/sj.jid.5701235
Lehman, H. (2014). Skin manifestations of primary immune deficiency. Clin. Rev. Allergy Immunol. 46, 112–119. doi: 10.1007/s12016-013-8377-8
Li, H., Goh, B. N., Teh, W. K., Jiang, Z., Goh, J. P. Z., Goh, A., et al. (2018). Skin commensal malassezia globosa secreted protease attenuates Staphylococcus aureus biofilm formation. J. Invest. Dermatol. 138, 1137–1145. doi: 10.1016/j.jid.2017.11.034
Lichtenberger, R., Simpson, M. A., Smith, C., Barker, J., and Navarini, A. A. (2017). Genetic architecture of acne vulgaris. J. Eur. Acad. Dermatol. Venereol. 31, 1978–1990. doi: 10.1111/jdv.14385
Lomholt, H. B., and Kilian, M. (2010). Population genetic analysis of Propionibacterium acnes identifies a subpopulation and epidemic clones associated with acne. PLoS ONE 5:e12277. doi: 10.1371/journal.pone.0012277
Lomholt, H. B., Scholz, C. F. P., Bruggemann, H., Tettelin, H., and Kilian, M. (2017). A comparative study of Cutibacterium (Propionibacterium) acnes clones from acne patients and healthy controls. Anaerobe 47, 57–63. doi: 10.1016/j.anaerobe.2017.04.006
Margolis, J. S., Abuabara, K., Bilker, W., Hoffstad, O., and Margolis, D. J. (2014). Persistence of mild to moderate atopic dermatitis. JAMA Dermatol. 150, 593–600. doi: 10.1001/jamadermatol.2013.10271
Meisel, J. S., Hannigan, G. D., Tyldsley, A. S., SanMiguel, A. J., Hodkinson, B. P., Zheng, Q., et al. (2016). Skin microbiome surveys are strongly influenced by experimental design. J. Invest. Dermatol. 136, 947–956. doi: 10.1016/j.jid.2016.01.016
Melnik, B. (2015). Linking diet to acne metabolomics, inflammation, and comedogenesis: an update. Clin. Cosmet. Investig. Dermatol. 8, 371–388. doi: 10.2147/CCID.S69135
Mortz, C. G., Andersen, K. E., Dellgren, C., Barington, T., and Bindslev-Jensen, C. (2015). Atopic dermatitis from adolescence to adulthood in the TOACS cohort: prevalence, persistence and comorbidities. Allergy 70, 836–845. doi: 10.1111/all.12619
Myles, I. A., Earland, N. J., Anderson, E. D., Moore, I. N., Kieh, M. D., Williams, K. W., et al. (2018). First-in-human topical microbiome transplantation with Roseomonas mucosa for atopic dermatitis. JCI Insight 3:e120608. doi: 10.1172/jci.insight.120608
Nagy, I., Pivarcsi, A., Kis, K., Koreck, A., Bodai, L., McDowell, A., et al. (2006). Propionibacterium acnes and lipopolysaccharide induce the expression of antimicrobial peptides and proinflammatory cytokines/chemokines in human sebocytes. Microbes Infect. 8, 2195–2205. doi: 10.1016/j.micinf.2006.04.001
Nagy, I., Pivarcsi, A., Koreck, A., Szell, M., Urban, E., and Kemeny, L. (2005). Distinct strains of Propionibacterium acnes induce selective human beta-defensin-2 and interleukin-8 expression in human keratinocytes through toll-like receptors. J. Invest. Dermatol. 124, 931–938. doi: 10.1111/j.0022-202X.2005.23705.x
Naik, S., Bouladoux, N., Linehan, J. L., Han, S. J., Harrison, O. J., Wilhelm, C., et al. (2015). Commensal-dendritic-cell interaction specifies a unique protective skin immune signature. Nature 520, 104–108. doi: 10.1038/nature14052
Nakatsuji, T., Chen, T. H., Butcher, A. M., Trzoss, L. L., Nam, S. J., Shirakawa, K. T., et al. (2018). A commensal strain of Staphylococcus epidermidis protects against skin neoplasia. Sci. Adv. 4:eaao4502. doi: 10.1126/sciadv.aao4502
Nakatsuji, T., Chen, T. H., Narala, S., Chun, K. A., Two, A. M., Yun, T., et al. (2017). Antimicrobials from human skin commensal bacteria protect against Staphylococcus aureus and are deficient in atopic dermatitis. Sci. Transl. Med. 9:eaah4680. doi: 10.1126/scitranslmed.aah4680
Neuman, H., and Koren, O. (2016). The gut microbiome. Encyclopedia Cell Biol. 2, 799–808. doi: 10.1016/B978-0-12-394447-4.20083-7
Oh, J., Byrd, A. L., Deming, C., Conlan, S., Program, N. C. S., Kong, H. H., et al. (2014). Biogeography and individuality shape function in the human skin metagenome. Nature 514, 59–64. doi: 10.1038/nature13786
Oh, J., Byrd, A. L., Park, M., Program, N. C. S., Kong, H. H., and Segre, J. A. (2016). Temporal stability of the human skin microbiome. Cell 165, 854–866. doi: 10.1016/j.cell.2016.04.008
Oh, J., Conlan, S., Polley, E. C., Segre, J. A., and Kong, H. H. (2012). Shifts in human skin and nares microbiota of healthy children and adults. Genome Med. 4, 77–87. doi: 10.1186/gm378
Oh, J., Freeman, A. F., Program, N. C. S., Park, M., Sokolic, R., Candotti, F., et al. (2013). The altered landscape of the human skin microbiome in patients with primary immunodeficiencies. Genome Res. 23, 2103–2114. doi: 10.1101/gr.159467.113
Olson, M. E., Todd, D. A., Schaeffer, C. R., Paharik, A. E., Van Dyke, M. J., Buttner, H., et al. (2014). Staphylococcus epidermidis agr quorum-sensing system: signal identification, cross talk, and importance in colonization. J. Bacteriol. 196, 3482–3493. doi: 10.1128/JB.01882-14
O'Neill, A. M., and Gallo, R. L. (2018). Host-microbiome interactions and recent progress into understanding the biology of acne vulgaris. Microbiome 6:177. doi: 10.1186/s40168-018-0558-5
Otto, M. (2009). Staphylococcus epidermidis–the 'accidental' pathogen. Nat. Rev. Microbiol. 7, 555–567. doi: 10.1038/nrmicro2182
Otto, M., Echner, H., Voelter, W., and Gotz, F. (2001). Pheromone cross-inhibition between Staphylococcus aureus and Staphylococcus epidermidis. Infect. Immun. 69, 1957–1960. doi: 10.1128/IAI.69.3.1957-1960.2001
Paetzold, B., Willis, J. R., Pereira de Lima, J., Knodlseder, N., Bruggemann, H., Quist, S. R., et al. (2019). Skin microbiome modulation induced by probiotic solutions. Microbiome 7:95. doi: 10.1186/s40168-019-0709-3
Paharik, A. E., Parlet, C. P., Chung, N., Todd, D. A., Rodriguez, E. I., Van Dyke, M. J., et al. (2017). Coagulase-negative staphylococcal strain prevents Staphylococcus aureus colonization and skin infection by blocking quorum sensing. Cell Host Microbe 22, 746–756.e745. doi: 10.1016/j.chom.2017.11.001
Paller, A. S., Kong, H. H., Seed, P., Naik, S., Scharschmidt, T. C., Gallo, R. L., et al. (2019). The microbiome in patients with atopic dermatitis. J. Allergy Clin. Immunol. 143, 26–35. doi: 10.1016/j.jaci.2018.11.015
Palmer, C. N., Irvine, A. D., Terron-Kwiatkowski, A., Zhao, Y., Liao, H., Lee, S. P., et al. (2006). Common loss-of-function variants of the epidermal barrier protein filaggrin are a major predisposing factor for atopic dermatitis. Nat. Genet. 38, 441–446. doi: 10.1038/ng1767
Panigrahi, P., Parida, S., Nanda, N. C., Satpathy, R., Pradhan, L., Chandel, D. S., et al. (2017). A randomized synbiotic trial to prevent sepsis among infants in rural India. Nature 548, 407–412. doi: 10.1038/nature23480
Parlet, C. P., Brown, M. M., and Horswill, A. R. (2019). Commensal staphylococci influence Staphylococcus aureus skin colonization and disease. Trends Microbiol. 27, 497–507. doi: 10.1016/j.tim.2019.01.008
Paternoster, L., Standl, M., Waage, J., Baurecht, H., Hotze, M., Strachan, D. P., et al. (2015). Multi-ancestry genome-wide association study of 21,000 cases and 95,000 controls identifies new risk loci for atopic dermatitis. Nat. Genet. 47, 1449–1456. doi: 10.1038/ng.3424
Quan, C., Chen, X. Y., Li, X., Xue, F., Chen, L. H., Liu, N., et al. (2019). Psoriatic lesions are characterized by higher bacterial load and imbalance between Cutibacterium and Corynebacterium. J. Am. Acad. Dermatol. 82, 955–961. doi: 10.1016/j.jaad.2019.06.024
Salava, A., and Lauerma, A. (2014). Role of the skin microbiome in atopic dermatitis. Clin. Transl. Allergy 4, 33–38. doi: 10.1186/2045-7022-4-33
Scharschmidt, T. C., and Fischbach, M. A. (2013). What lives on our skin: ecology, genomics and therapeutic opportunities of the skin microbiome. Drug Discov. Today Dis. Mech. 10, e83–e89. doi: 10.1016/j.ddmec.2012.12.003
Schoch, C. L., Seifert, K. A., Huhndorf, S., Robert, V., Spouge, J. L., Levesque, C. A., et al. (2012). Nuclear ribosomal internal transcribed spacer (ITS) region as a universal DNA barcode marker for Fungi. Proc. Natl. Acad. Sci. U.S.A. 109, 6241–6246. doi: 10.1073/pnas.1117018109
Scholz, C. F. P., and Kilian, M. (2016). The natural history of cutaneous propionibacteria, and reclassification of selected species within the genus Propionibacterium to the proposed novel genera Acidipropionibacterium gen. nov., Cutibacterium gen. nov. and Pseudopropionibacterium gen. nov. Int. J. Syst. Evol. Microbiol. 66, 4422–4432. doi: 10.1099/ijsem.0.001367
Seebacher, C., Bouchara, J. P., and Mignon, B. (2008). Updates on the epidemiology of dermatophyte infections. Mycopathologia 166, 335–352. doi: 10.1007/s11046-008-9100-9
Shalita, A. R. (2004). Acne: clinical presentations. Clin. Dermatol. 22, 385–386. doi: 10.1016/j.clindermatol.2004.03.012
Shi, B., Bangayan, N. J., Curd, E., Taylor, P. A., Gallo, R. L., Leung, D. Y. M., et al. (2016). The skin microbiome is different in pediatric versus adult atopic dermatitis. J. Allergy Clin. Immunol. 138, 1233–1236. doi: 10.1016/j.jaci.2016.04.053
Simpson, E. L., Bieber, T., Guttman-Yassky, E., Beck, L. A., Blauvelt, A., Cork, M. J., et al. (2016). Two phase 3 trials of dupilumab versus placebo in atopic dermatitis. N. Engl. J. Med. 375, 2335–2348. doi: 10.1056/NEJMoa1610020
Sohn, E. (2018). Skin microbiota's community effort. Nature 563, S91–S93. doi: 10.1038/d41586-018-07432-8
Song, W., Anselmo, A. C., and Huang, L. (2019). Nanotechnology intervention of the microbiome for cancer therapy. Nat. Nanotechnol. 14, 1093–1103. doi: 10.1038/s41565-019-0589-5
Stacy, A., and Belkaid, Y. (2019). Microbial guardians of skin health. Science 363, 227–228. doi: 10.1126/science.aat4326
Summerfield, A., Meurens, F., and Ricklin, M. E. (2015). The immunology of the porcine skin and its value as a model for human skin. Mol. Immunol. 66, 14–21. doi: 10.1016/j.molimm.2014.10.023
Sun, Z., Huang, S., Zhu, P., Yue, F., Zhao, H., Yang, M., et al. (2019). A microbiome-based index for assessing skin health and treatment effects for atopic dermatitis in children. mSystems 4:e00293–19. doi: 10.1128/mSystems.00293-19
Szegedi, A., Dajnoki, Z., Biro, T., Kemeny, L., and Torocsik, D. (2019). Acne: transient arrest in the homeostatic host-microbiota dialog? Trends Immunol. 40, 873–876. doi: 10.1016/j.it.2019.08.006
Thaçi, D., Simpson, E. L., Beck, L. A., Bieber, T., Blauvelt, A., Papp, K., et al. (2016). Efficacy and safety of dupilumab in adults with moderate-to-severe atopic dermatitis inadequately controlled by topical treatments: a randomised, placebo-controlled, dose-ranging phase 2b trial. Lancet 387, 40–52. doi: 10.1016/S0140-6736(15)00388-8
Tomich, M., Planet, P. J., and Figurski, D. H. (2007). The tad locus: postcards from the widespread colonization island. Nat. Rev. Microbiol. 5, 363–375. doi: 10.1038/nrmicro1636
Totte, J. E., van der Feltz, W. T., Bode, L. G., van Belkum, A., van Zuuren, E. J., and Pasmans, S. G. (2016). A systematic review and meta-analysis on Staphylococcus aureus carriage in psoriasis, acne and rosacea. Eur. J. Clin. Microbiol. Infect. Dis. 35, 1069–1077. doi: 10.1007/s10096-016-2647-3
Totté, J. E. E., van der Feltz, W. T., Hennekam, M., van Belkum, A., van Zuuren, E. J., and Pasmans, S. G. M. A. (2016). Prevalence and odds of Staphylococcus aureuscarriage in atopic dermatitis: a systematic review and meta-analysis. Br. J. Dermatol. 175, 687–695. doi: 10.1111/bjd.14566
Tsakok, T., Woolf, R., Smith, C. H., Weidinger, S., and Flohr, C. (2018). Atopic dermatitis: the skin barrier and beyond. Br. J. Dermatol. 180, 464–474. doi: 10.1111/bjd.16934
Vandegrift, R., Bateman, A. C., Siemens, K. N., Nguyen, M., Wilson, H. E., Green, J. L., et al. (2017). Cleanliness in context: reconciling hygiene with a modern microbial perspective. Microbiome 5:76. doi: 10.1186/s40168-017-0294-2
Weidinger, S., Beck, L. A., Bieber, T., Kabashima, K., and Irvine, A. D. (2018). Atopic dermatitis. Nat. Rev. Dis. Primers 4:1. doi: 10.1038/s41572-018-0001-z
Weidinger, S., and Novak, N. (2016). Atopic dermatitis. Lancet 387, 1109–1122. doi: 10.1016/S0140-6736(15)00149-X
Williams, H. C., Dellavalle, R. P., and Garner, S. (2012). Acne vulgaris. Lancet 379, 361–372. doi: 10.1016/S0140-6736(11)60321-8
Williams, M. R., Costa, S. K., Zaramela, L. S., Khalil, S., Todd, D. A., Winter, H. L., et al. (2019). Quorum sensing between bacterial species on the skin protects against epidermal injury in atopic dermatitis. Sci. Transl. Med. 11:eaat8329. doi: 10.1126/scitranslmed.aat8329
Wu, G., Zhao, H., Li, C., Rajapakse, M. P., Wong, W. C., Xu, J., et al. (2015). Genus-wide comparative genomics of malassezia delineates its phylogeny, physiology, and niche adaptation on human skin. PLoS Genet 11:e1005614. doi: 10.1371/journal.pgen.1005614
Zaenglein, A. L., Pathy, A. L., Schlosser, B. J., Alikhan, A., Baldwin, H. E., Berson, D. S., et al. (2016). Guidelines of care for the management of acne vulgaris. J. Am. Acad. Dermatol. 74, 945–973.e933. doi: 10.1016/j.jaad.2015.12.037
Keywords: human skin microbiota, cutaneous disorders, dysbiosis, ecology-based therapy, applications
Citation: Zhou H, Shi L, Ren Y, Tan X, Liu W and Liu Z (2020) Applications of Human Skin Microbiota in the Cutaneous Disorders for Ecology-Based Therapy. Front. Cell. Infect. Microbiol. 10:570261. doi: 10.3389/fcimb.2020.570261
Received: 07 June 2020; Accepted: 18 September 2020;
Published: 22 October 2020.
Edited by:
Jun Zhu, University of Pennsylvania, United StatesReviewed by:
Alinne Castro, Dom Bosco Catholic University, BrazilZhigang Zhou, Feed Research Institute (CAAS), China
Copyright © 2020 Zhou, Shi, Ren, Tan, Liu and Liu. This is an open-access article distributed under the terms of the Creative Commons Attribution License (CC BY). The use, distribution or reproduction in other forums is permitted, provided the original author(s) and the copyright owner(s) are credited and that the original publication in this journal is cited, in accordance with accepted academic practice. No use, distribution or reproduction is permitted which does not comply with these terms.
*Correspondence: Wei Liu, d2xpdSYjeDAwMDQwO2h1c3QuZWR1LmNu; Zhi Liu, emhpbGl1JiN4MDAwNDA7aHVzdC5lZHUuY24=