- 1University of Strasbourg/CNRS, UMR7177, Institut de Chimie de Strasbourg, Strasbourg, France
- 2Institut Universitaire de France (IUF), Paris, France
Magainin 2 and PGLa are antimicrobial peptides found together in frog skin secretions. When added as a mixture they show an order of magnitude increase in antibacterial activity and in model membrane permeation assays. Here we demonstrate that both peptides can form fibers with beta-sheet/turn signature in ATR-FTIR- and CD-spectroscopic analyses, but with different morphologies in EM images. Whereas, fiber formation results in acute reduction of the antimicrobial activity of the individual peptides, the synergistic enhancement of activity remains for the equimolar mixture of PGLa and magainin 2 also after fibril formation. The biological significance and potential applications of such supramolecular aggregates are discussed.
Introduction
Magainin 2 and PGLa were among the first cationic amphipathic peptides for which the biological regulation and antimicrobial activity was explored in greater depth (Zasloff, 1987; Gibson, 1991). In nature these peptides are both produced by and stored together in the granular glands of the South African clawed frog Xenopus laevis (Giovannini et al., 1987) and from each frog up to tens of milligrams of highly concentrated gel-like vesicular skin secretions have been isolated upon stimulation of release (Kiss and Michl, 1962; Gibson et al., 1986; Giovannini et al., 1987). Thereby the frogs have a potent innate defense mechanism that can be set in action immediately to prevent bacterial and fungal infections (Zasloff, 1987, 2002). Early on biophysical investigations provided experimental evidence that the cell killing by these and other cationic amphipathic peptides can be correlated with their selective impairment of bacterial plasma membrane function (Roversi et al., 2014; Aisenbrey et al., 2019). In contrast to the formation of barrel-stave pores observed for hydrophobic antimicrobial peptides such as alamethicin (Sansom, 1991; Salnikov et al., 2016) magainins and related linear cationic amphipathic sequences preferentially align parallel to the bilayer surface and work by different mechanisms (Bechinger et al., 1991; Oren and Shai, 1998; Bechinger, 2015).
Notably, equimolar mixtures made up of magainin 2a (GIGKF LHSAK KFGKA FVGEI MNS-NH2) and PGLa (GMASK AGAIA GKIAK VALKA L-NH2) have an almost one order of magnitude increased activity in antimicrobial assays but also in fluorescent dye-release studies from lipid-only model membranes (Westerhoff et al., 1995; Matsuzaki et al., 1998; Glattard et al., 2016). The molecular mechanisms for synergistic membrane disruption and antibacterial activities remains poorly understood and a number of models have been suggested (Matsuzaki et al., 1998; Marquette et al., 2015; Pino-Angeles et al., 2016; Zerweck et al., 2017; Leber et al., 2018). The biophysical investigations of their membrane interactions have recently been reviewed (Aisenbrey et al., 2019).
These biophysical studies show that magainin 2 adopts very stable alignments parallel to the membrane surface under all conditions tested so far (Bechinger, 2011; Salnikov and Bechinger, 2011; Strandberg et al., 2013), a feature also observed for many other related peptides (Porcelli et al., 2013; Resende et al., 2014; Perrin et al., 2015; Salnikov et al., 2018). In contrast to magainin 2, the membrane topological alignment of PGLa is modulated by the detailed composition of the phospholipid membrane, its peptide-to-lipid ratio, the hydration level or the presence of magainin 2 (Tremouilhac et al., 2006; Salnikov and Bechinger, 2011; Strandberg et al., 2013).
Whereas the interactions of antimicrobial peptides with lipids and other peptides within membranes have been the focus of prior biophysical investigations (Aisenbrey et al., 2019) much less is known about their interactions in solution. This is somewhat surprising because the potential of even very short polypeptides or extended amino acids to assemble into complex supramolecular assemblies has attracted increased attention in the context of biomaterials (Kumar et al., 2016; Chakraborty and Gazit, 2018; Beesley and Woolfson, 2019; Sun et al., 2019), biomedical applications (Fitzpatrick et al., 2013; Meier et al., 2014; Vermeer et al., 2017; Hainline et al., 2018) or amyloidogenic diseases (Fusco et al., 2016; Qiang et al., 2017; Ramamoorthy, 2018). Interestingly, the amyloid-beta peptide involved in Alzheimer disease has been found to not only assemble into fibrillar structures but to also exhibit antimicrobial activity (Soscia et al., 2010; Gosztyla et al., 2018). Fiber formation of some antimicrobial sequences has been described (Auvynet et al., 2008; Jang et al., 2011; Chu et al., 2012; Caillon et al., 2013) where some of the fibers are supramolecular arrangements made of peptide and acidic phospholipids (Sood et al., 2008; Mahalka and Kinnunen, 2009). In particular, magainin 2 has early on been published to aggregate into fibers at high salt and/or low pH conditions (Urrutia et al., 1989) but to our knowledge this observation has not been followed up in further investigations. Notably, amyloid fibers can have beneficial properties in helping with the long-term storage of antimicrobial peptides in the frog skin and thereby they constitute another example of functional amyloids that have previously been described (Maji et al., 2009; Depas and Chapman, 2012; Schubeis et al., 2015; Tayeb-Fligelman et al., 2017).
Therefore, in this work we have evaluated the fibrillation capacity of the antimicrobial peptides magainin 2a and PGLa as well as their synergistic equimolar mixture under physiological conditions. Moreover, we evaluated the antimicrobial activities of such fibrillar assemblies in comparison with non-aggregated peptides in solution. We have found that both peptides can form large supramolecular assemblies including fibers under physiological conditions and that this association has a strong effect on their antimicrobial activity. In order to evaluate structural details of the fibers biophysical techniques including EM-, FTIR-, and CD-spectroscopies are used and the results correlated to antimicrobial activity. Importantly, the peptides alone but also their synergistic mixtures have been investigated.
Materials and Methods
Peptide Synthesis
PGLa (GMASK AGAIA GKIAK VALKA L-NH2) and magainin 2a (GIGKF LHSAK KFGKA FVGEI MNS-NH2; where the extension a is referring to the amidated carboxy terminus) were prepared by solid-phase synthesis using a Millipore 9050 automatic peptide synthesizer and Fmoc chemistry. The peptides were purified by reverse phase HPLC (Gilson, Villiers-le-bel, France) using a preparative C18 column (Luna, C-18-100Å-5 μm, Phenomenex, Le Pecq, France) and an acetonitrile/water gradient. Their identity and purity (>90%) were checked by MALDI-TOF mass spectrometry (MALDI-TOF Autoflex, Bruker Daltonics, Bremen Germany). The purified peptides were dissolved three times in 2 mM HCl at a 1 mg/mL concentration with subsequent lyophilization to ensure exchange of the TFA counter-ions. Quantification of peptides was based on mass and was determined for aliquots of ~10 mg peptide. Clean glass vials were weighted prior to the addition of the peptide solubilized in 2 mM HCl. The glass vials were weighted again after lyophilization. All vials were weighted 3 times before and after the addition of peptide on a 0.0001 g precision scale to account for small variations. Hereafter, the peptides were stored in aliquots of 1 mg at −20°C.
Fibrillation in Tubes
Lyophilized powder of PGLa was dissolved in 50% ethanol at a 10 mM peptide concentration before being diluted into Mueller-Hinton (MH) medium or various buffers to a 0.5 mM final peptide concentration. All buffers were 50 mM and included: sodium phosphate at pH 7.4, sodium phosphate containing 150 mM NaCl at pH 7.4, sodium phosphate at pH 8.5, sodium phosphate at pH 3.5, Tris at pH 7.4; sodium acetate at pH 4.5; Hepes at pH 7.4, and ammonium bicarbonate at pH 7.4. Also, pure unbuffered Milli-Q filtered water was tested (Merck-Millipore, Molsheim, France). Fibrillation was conducted under vigorous shaking for 1 day.
Determination of Fibrillation Yield
Fibrils prepared in tubes were centrifuged at 13,000 rpm for 30 min and the supernatants were removed. After lyophilization, the supernatants were dissolved in 2 mM HCl and analyzed by reverse phase HPLC (Bischoff chromatography, Leonberg, Germany) using an analytical C18 column (Luna, C-18-100 Å-5 μm, Phenomenex, Le Pecq, France) and an acetonitrile/water gradient. The flow rate is 1 ml/min, solvent A contains 10% acetonitrile in water, 0.1% TFA and solvent B is made of 100% acetonitrile, 0.1% TFA. The gradient for both peptides is 15% of solvent B for 3 min, an increase to 45% of solvent B within 15 min and finally to 95% for 3 min before the column is re-equilibrated with 15% B for 3 min. The peptide concentration was determined based on standard curves prepared from known peptide concentrations.
Fibrillation in 96-Well Half Area Plates
The peptides were dissolved in 50% ethanol at a 10 mM peptide concentration before being diluted into MH medium or buffers containing 40 μM ThT. For each condition, 50 μL of the samples was transferred to three different wells in 96 half area well flat clear bottom black polystyrene TC-treated microplates (Corning, Corning Incorporated, Kennebunk, ME) before the fibrillation was carried out at 37°C with 300 rpm orbital shaking. The fluorescence of ThT was monitored on a CLARIO Star plate reader (BMG Labtech, Ortenberg, Germany) with 450 nm excitation and 485 nm emission filters. For seeded experiments, fibrils prepared in phosphate buffer by the tube method (cf. above), were vortexed vigorously and then added to each well such that the seeds correspond to 10% of the peptide concentration.
Transmission Electron Microscopy
Sample material (10–20 μg) was deposited on formvar grids (CFT200-Cu, Electron Microscopy Sciences, Hatfield, PA, United States). After 2 min the grids were blotted dry and left to dry on air for another 30 min. The grids were washed twice in Milli-Q filtered water and a negative staining was made with 1% uranyl acetate in water for 1 min. TEM images were collected using a Hitachi H7500 electron microscope, operated at 80 KeV with a LaB6 filament.
FTIR and Circular Dichroism Spectroscopy
The peptides were dissolved in 50% ethanol at a 10 mM peptide concentration before being diluted 1/20 into 1X MH medium or 50 mM phosphate buffer at pH 7.4. For peptide alone or mixture at molar ratio 1:1, the peptide solutions were transferred into 1.5 mL microtubes (Vf 500 μl; Cf 0.5 mM). Fibrillation was carried out with vigorous shaking in an Eppendorf Mixer 5432 for 1 day at room temperature. After centrifugation at 13,000 g for 30 min at RT, the pellets of the fibers were washed twice in 10 mM phosphate buffer, pH 7 in D2O.
For FTIR experiments, the pellets were resuspended with 10 μL of 10 mM phosphate buffer, pH 7 in D2O, of which 2 μL were loaded and dried on the diamond crystal. FTIR spectra (12 scans, from which the background was subtracted) were recorded from 1,500 to 2,500 cm−1 with a Nicolet 6700 spectrometer (Thermo Scientific, Waltham, MA).
For CD experiments, pellets from fibers were resuspended with 250 μL of 10 mM phosphate buffer, pH 7 and tip sonicated for 1 min at 50% power during 30% of the time (Sonoplus H200, Bandelin, Berlin, Germany). A second dilution by ½ is done if necessary. CD spectra (5–10 scans, from which a blank was subtracted) were recorded from 260 to 190 nm with a Jasco J-810 spectrometer (Jasco, Tokyo, Japan) using a 1 mm thick cuvette at 25°C.
Antimicrobial Activity Assays
Fibrils of both peptides and their 1:1 mixture were prepared using the tube fibrillation in MH medium. Mueller Hinton medium (Fluka Analytical, Ref 70192, Lot BCBH7195V) contain beef infusion, casein hydrolysate and starch and it has a final pH of 7.3.
The fibrillation was initiated exactly 1 day before the antimicrobial activity assay. Similar samples were prepared half an hour before the assay. These last samples were not shaken. A gentle vortexing step was applied to all the samples prior to transferring to the microplate assuring a homogenous suspension of fibrils.
For all activity assays, E. coli bacteria (ATCC25922, Ref. 0335-CRM, Thermo Fisher Scientific, Courtaboeuf, France) were grown overnight on Mueller-Hinton agar plates. A suspension of bacteria in MH medium (Fluka Analytical, Sigma Aldrich, Saint-Louis, MO, USA) was made from the plates and used to inoculate a 10 mL preculture with a starting OD550 = 0.005. The preculture was incubated overnight and then used to inoculate a culture with a starting OD550 = 0.2 (10 mL of MH). The culture was incubated until an OD550 = 1.0 was reached (around 3 h). From this culture, a standard bacterial suspension was prepared with OD550 = 0.2 which were used to prepare the final bacterial working solution at OD550 = 0.0002.
The antimicrobial assays were performed in 96 well microplates (F-bottom sterile non-treated polystyrene, Thermo Scientific Nunc A/S, Roskilde, Denmark). All samples were submitted to the first column of the plate and subsequently exposed to a 2-fold dilution series in 10 steps. Finally, the bacterial working solution was distributed (50 μL) to each well except the blank controls. The final peptide concentration was ranging from 50 to 0.05 μM (after addition of bacteria).
The plates were incubated at 37°C for 18 h before the OD600 was measured. Resazurin was added to each well (0.033 mg/mL final dye concentration) and the plates incubated for another 2 h. The cell viability was determined based on the reduction of resazurin. The ratio of reduced resazurin was measured using the absorbance at 570 and 600 nm.
Results
PGLa and Magainin 2a Form Fibrils in Mueller Hinton Medium
The antimicrobial activities of magainin 2a and PGLa have been suggested to be associated with their cationic and amphiphilic character that allows them to partition into the interface of bacterial membranes (Bechinger, 1999; Zasloff, 2002). Later on, these and other α-helical peptides have been shown to assemble in mesophase arrangements along the membrane surface (Aisenbrey and Bechinger, 2014; Glattard et al., 2016; Marquette and Bechinger, 2018) or into fibrils (Urrutia et al., 1989; Soscia et al., 2010; Gosztyla et al., 2018) but the biological significance of such supramolecular assemblies still needs to be investigated. In this paper, we have studied the potential of magainin 2a and PGLa to form extended fibrillar structures under physiological conditions. Fibrillation assays were performed in Mueller Hinton Medium developed for antimicrobial assays or in buffers used in biochemical experiments. Furthermore, the antimicrobial activity of the resulting assemblies was tested and compared to that of non-aggregated peptides.
Fibrillation was conducted at a 1 mg/mL peptide concentration (0.5 mM) in microplates under continued shaking. The amyloidogenic dye thioflavin T was included in the samples as a reporter for fibrillar structures. After ~1 h, an increase in thioflavin T fluorescence was observed for both PGLa and an equimolar mixture of PGLa and magainin 2 (Figure 1A). The fluorescence continued to increase until a plateau was reached after ~8 h. After normalizing to the final fluorescence level, the two curves overlap (not shown). The similar fibrillation kinetics indicates that PGLa fibrillation is independent of the presence of magainin 2a. For magainin 2a alone, no increase in the ThT fluorescence was observed during the 16 h duration of the experiment.
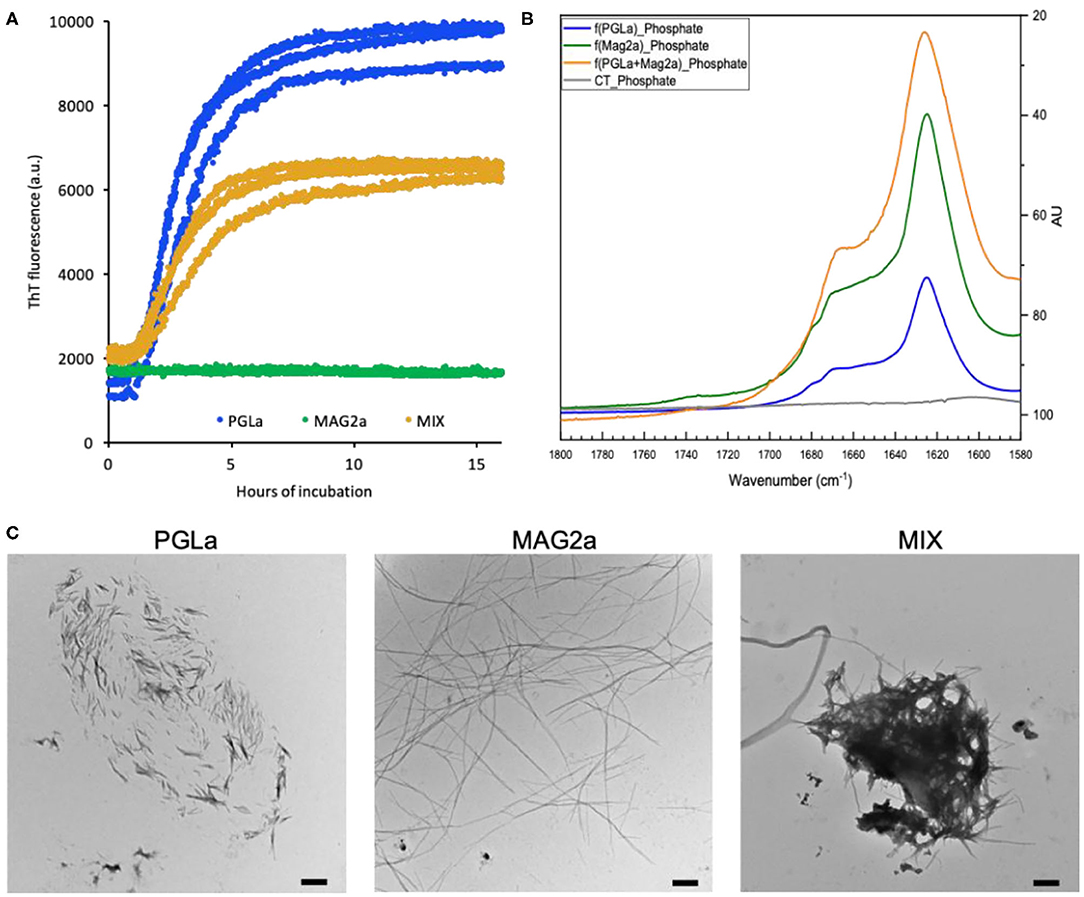
Figure 1. Fibril formation of PGLa, magainin 2 and their 1:1 mixture. (A) Fibrillation of 0.5 mM PGLa (blue), magainin 2 (green) and their 1:1 mixture (orange) in Mueller-Hinton medium monitored by ThT fluorescence. The fibrillation was conducted under constant shaking at 37°C. (B) FTIR spectra of fibrils prepared from PGLa (blue), magainin 2 (green) and their 1:1 mixture (orange) in 50 mM phosphate buffer, pH 7.4. The buffer alone is shown in gray. (C) TEM images of fibrils prepared from PGLa, magainin 2 and their 1:1 mixture in Mueller-Hinton medium. The scale bars indicate 500 nm.
The structures formed during the fibrillation assay were evaluated by electron microscopy (EM). Despite the lack of ThT fluorescence in the case of magainin 2a, all three samples exhibit assemblies of fibrillar structures (Figure 1C). Even though some inhomogeneity in the fibril morphologies was observed by EM, the fibrils generated by PGLa could be distinguished from those generated by magainin 2a. On the EM grids, PGLa appears as larger assemblies of short rigid fibril sticks while magainin 2a forms long thin fibrils, which did not seem to assemble further. The EM images of the peptide mixture appear to contain both types of fibrillar assemblies suggesting that the two peptides de-mix when forming fibrils. This is supported by the similar fibrillation kinetics observed for PGLa alone and in the peptide mixture (Figure 1A).
Thioflavin T becomes fluorescent when interacting with the repetitive sidechain arrangements on the surface of some β-sheet amyloidogenic structures (Biancalana and Koide, 2010). However, the intensity of the fluorescent signal varies substantially depending on the exact fibrillar surface defined by the peptide sequence and fibril morphology (Juhl et al., 2019). Using FTIR spectroscopy of fibrils prepared in phosphate buffer, we confirm secondary structures closely resembling those of other amyloids (Shivu et al., 2013) as the spectra are dominated by intensities around 1,623 cm−1 for all three samples (Figure 1B). Spectral deconvolution is indicative of 75–60% β-sheet/β-turn structures and ca. 20% helical conformations (not shown) (Goormaghtigh et al., 1990). CD-spectra of sonicated fibers are characterized by minima around 215 nm thus confirming the preference for β-conformations, while a quantitative analysis is hampered by light scattering artifacts (Supplementary Figure 1).
Mixed Fibrils Retain the Activity in Contrast to Fibrils of the Individual Peptides
In a next step, the effect of fibril formation was assayed by comparing the antimicrobial activity of freshly prepared peptide solutions with those from samples exposed to continuous shaking for 24 h. The antimicrobial activity assay was conducted as previously reported (Glattard et al., 2016) with the final peptide concentration ranging from 50 to 0.05 μM in a 2-fold dilution series encompassing 10 steps. The fibrils and peptides eventually remaining in solution after 24 h of incubation were not separated from each other for the antimicrobial activity test.
Formation of fibrils leads to a decrease in antimicrobial activity (Figure 2). In particular, the PGLa fibril formation completely inhibited the activity of the peptide (Figure 2A). The minimal inhibitory concentration (MIC100) of magainin 2a changed from around 15 μM before fibrillation to around 30 μM after fibrillation (Figure 2B), while the MIC100 of the 1:1 peptide mixture doubled from 3 to 6 μM (Figure 2C). Finally, we assayed the activity of a 1:1 mixture of PGLa fibrils and magainin 2a fibrils when the peptides were mixed after fibrillation (Figure 2D). Here the activity dropped slightly (MIC100 13 μM) when compared to mixtures fibrillated together, but still showed increased activities when compared to the individual peptides.
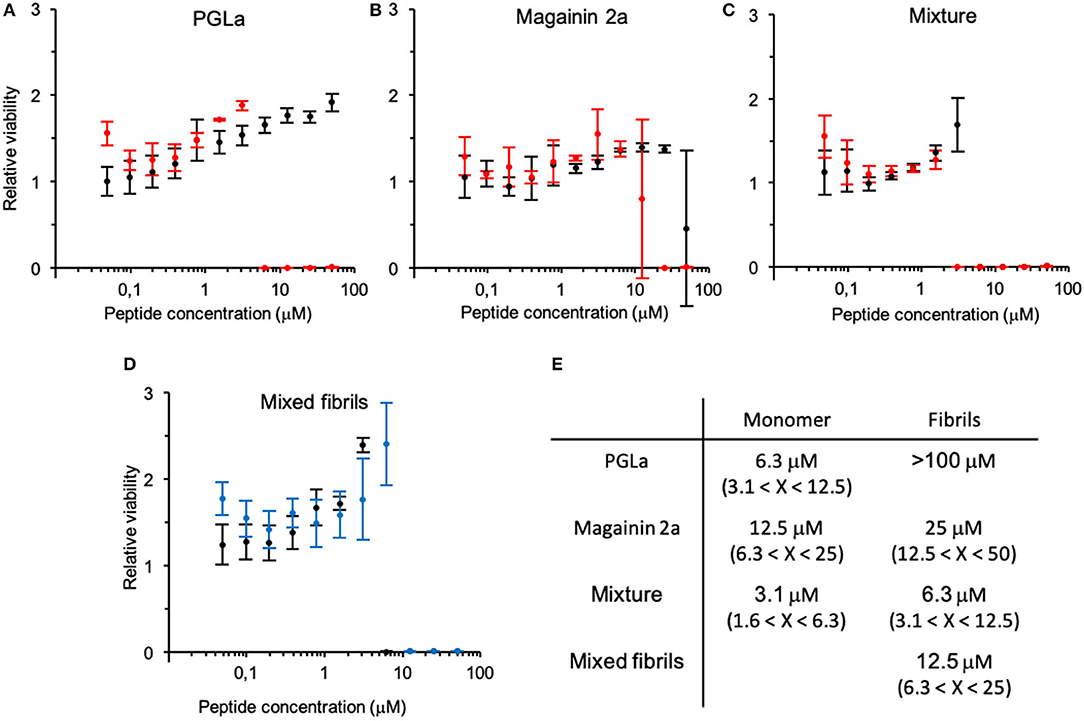
Figure 2. Change in antimicrobial activity upon peptide fibrillation. The cell viability of bacteria incubated with fibrillated (black) or monomeric (red) peptide solutions relative to bacteria grown alone is depicted at increasing concentrations of (A) PGLa, (B) magainin 2 and (C) their 1:1 mixture (top panel). (D) Antimicrobial activity assay of the 1:1 mixture of PGLa and magainin 2 mixed before (black) or after (blue) fibrillation (bottom panel). Note, the error bar at the steep transition at the MIC reflects contributions from the horizontal and vertical axis. (E) Table summarizing the MIC. Antimicrobial activity was tested against E. coli (ATCC25922). Errors arise from the 2-fold peptide dilution steps of the assay.
Measurements of antimicrobial activity during the time course of the fibrillation process were performed by taking samples at 30 min, 1, 4, and 24 h. These measurements confirmed the trends of the previous experiments. For PGLa, the activity dropped already after 1 h of shaking and disappeared completely after 4 h. For magainin 2a, the changes appeared at an intermediate time scale, while the peptide mixture only showed changes after 24 h of incubation (data not shown).
Searching the Mechanism Behind PGLa Fibrillation
To identify factors influencing fibril formation, we determined the yield of aggregation for both peptides in different buffers. The aggregation was performed in tubes and the yield was determined by quantifying the peptide in the supernatant after centrifugation. For both peptides, phosphorous ions facilitated the aggregation, while PGLa also aggregated in the presence of ammonium bicarbonate (Figure 3A). The buffers TRIS, HEPES and acetate at pH 7.4 as well as pure water did not promote aggregation within 24 h and the addition of 150 mM sodium chloride to the phosphate buffer had only little consequences. In all instances of significant aggregation, EM pictures revealed fibrillar assemblies (Supplementary Figure 2). Finally, the ThT fluorescence of PGLa fibrils was exploited to follow the fibrillation in phosphate buffers at different pH. By varying the pH from 6.5 to 8.5, a shortening of the lag time was observed when the pH was increased (Figure 3B). Plotting the logarithm of the lag time against the pH gives a linear correlation (Figure 3C).
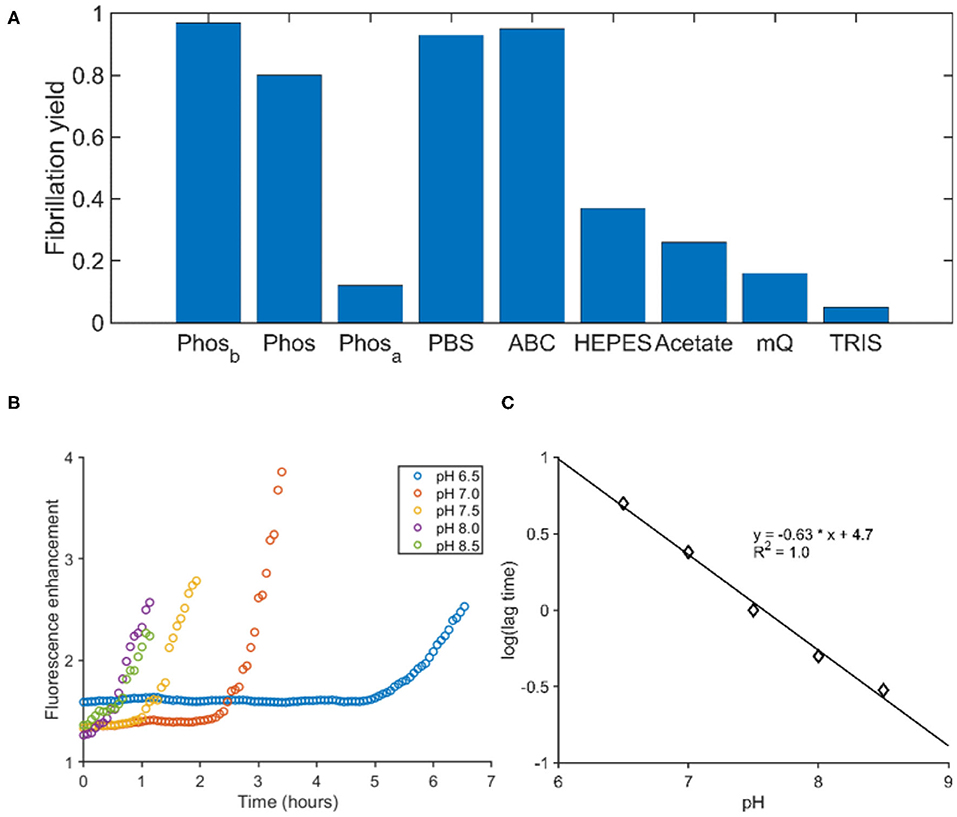
Figure 3. The influence of buffer composition on the fibrillation of PGLa. (A) The fibrillation yield of PGLa was determined by HLPC after 24 h of continuous shaking in various buffers. All buffers were at a 50 mM concentration. Phosphate (Phos), PBS, ammonium bicarbonate (ABC), HEPES and TRIS were adjusted to pH 7.4, while the acetate buffer was adjusted to pH 4.5. Phosb and Phosa were prepared like the phosphate buffer and thereafter the pH was changed outside of the buffer regime by addition of either base (Phosb) or acid (Phosa), respectively. (B) The fibrillation kinetics of PGLa was followed by ThT in phosphate buffers as a function of pH. (C) The pH of the phosphate buffer correlates linearly to the logarithm of the lag time.
When preformed PGLa fibrils prepared in phosphate were suspended into other buffers without phosphate, the fibrils disassociated (data not shown). Likewise, lowering the pH below the buffer range of phosphate (i.e., pH ≈ 3) results in the dissolution of the fibrils. The only exception was 50% ethanol which might be too hydrophobic to solvate the phosphate and lysine ions. Furthermore, when the concentration of non-aggregated peptide was tested by separating the fibers from the solution by centrifugation the supernatant contained only 10–20% of the initial peptide in phosphate buffer but a much higher fraction remained in Tris buffer solution (not shown).
Discussion
Here we demonstrate that the antimicrobial peptides magainin 2a and PGLa are prone to form fibrous molecular aggregates in the presence of bivalent anions at physiological conditions. These fibers can reversibly dissociate when exposed to aqueous environments of appropriate composition (e.g., acidic conditions, absence of phosphate or presence of detergents). The peptides investigated here are produced and stored together at high concentrations in the granular glands of the Xenopus laevis frog skin (Giovannini et al., 1987). Both peptides exhibit antimicrobial activities against a broad range of Gram-negative or Gram-positive bacteria and fungi (Soravia et al., 1988; Zasloff et al., 1988). They are released when the amphibian skin is stimulated or when exposed to bacterial infection (Giovannini et al., 1987). The supramolecular aggregate may thus help in efficient storage, protect these peptides from proteolytic degradation (Giovannini et al., 1987) and allow for their slow release over extended time periods similar to the storage of peptides and peptide hormones of the mammalian endocrine system (Maji et al., 2009). Thus, the peptides form a functional fiber beneficial to the producing organisms similar to other functional amyloids. For example, functional amyloids are formed by the phenol-soluble modulins of S. aureus, which constitute a virulence factor and help in biofilm formation of this bacterium (Tayeb-Fligelman et al., 2017).
Charges are well-known to influence the fibrillation kinetics of peptides and proteins (Zapadka et al., 2017). The charges can be soluble ions like in our study, associated with the surface of the container or be an additional fibrillation partner like Heparin sulfate (Risor et al., 2017). In all cases, the negative charges screen positive side-chains which reduces electrostatic repulsion and/or bridges cationic sites. At the same time, the arrangement of negative charges might bring the proteins closer to each other thereby improve their propensity for aggregation.
Magainin 2a and PGLa contain four lysines and a free amino terminus which, at neutral pH, nominally result in an overall positive net charge of 3–4 and 4–5, respectively. The high cationic character keeps the peptides in solution and enhances the interaction with the anionic surfaces of bacterial membranes (Matsuzaki et al., 1995; Bechinger, 1999). However, both peptides also contain a significant number of residues which confer considerable hydrophobic character driving aggregation (Torrent et al., 2011a,b; John et al., 2019). Notably, for other cationic amphipathic peptides amyloid formation has been postulated to pass through a loosely packed helical intermediate (John et al., 2019). Therefore, it is worth mentioning that both peptides can adopt amphipathic helical structures with high hydrophobic moment and a clear separation of charged/polar and hydrophobic residues (Marquette et al., 2015).
As PGLa does not contain amino acid side chains with a pK in the physiological pH range, the pH-dependent lag time and fibrillation kinetics probably mirrors the phosphate protonation state as was previously observed for another cationic peptide (Vermeer et al., 2017). For the pH range 6.5–8.5 the H2 and ions of the buffer are in equilibrium, where the divalent anion increases in concentration in a logarithmic fashion with pH. Furthermore, the amino terminus of polypeptides is typically characterized by a pK value close to the upper pH range investigated here and its deprotonation may help in the fiber assembly. Together, pH increase results in an effective reduction in peptide charge and the screening of remaining charges by phosphate ions thereby promoting the onset of fibril formation.
We show here, that divalent phosphate ions can induce fibrillation for both peptides, while carbonate works for PGLa as well (Figure 3A). The stability of the fibrils is dependent on the presence of those ions, possibly due to a cross-linking of positively charged lysine side chains from opposing peptide chains.
Fibrillation of the antimicrobial peptides results in a moderate (magainin 2a) to considerable loss in antimicrobial activities (PGLa). The reduced activity of the fibers can be explained by a number of factors. First, one can expect that the fibers face difficulties to pass the lipopolysaccharide layer, the outer membrane and peptidoglycan structures of the bacteria. Second, the β-sheet amyloids of these antimicrobial peptides form by interacting with bivalent anions. Thereby, some of the cationic charges of the peptide side chains are screened and the net charge per polymeric units is reduced. This should result in a lower local polypeptide concentration next to the plasma membrane surface (Wieprecht et al., 2000). Third, the membrane partitioning of such large complexes may be more difficult to achieve.
Based on the fibrillation yields, the activity should have decreased even further if the fibrils were completely inactive and stable. Possibly, the fibrils retain some activity on their own as has been shown for Aβ-fibers which exhibit antimicrobial action. It has been suggested that the latter are capable to interact with membranes and to trap infectious bacteria (Chairatana and Nolan, 2014; Gosztyla et al., 2018). By flocculation and sequestration the spread of pathogens is limited and they are prepared for phagocytosis (Robinson and Bishop, 2002).
The peptides' ability to re-dissolve presents another possibility on how antimicrobial activity is restored. Thus, competition between the anionic lipids and the bivalent anions that are required to glue the fibers together can be a driving force for the dissociation of the fibers. Along the same line, the multiple cationic charges of antimicrobial peptides have been suggested to compete with the bivalent cations that are required to electrostatically cross-link the bacterial lipopolysaccharide network (Hancock, 1984). Thereby the addition of bacteria to the sample might be sufficient to shift the equilibria from fibers to soluble peptide to peptides associated with the bacterial surfaces (Savini et al., 2017). The reversibility of the fibril formation could also explain the pronounced activity of the peptide mixture.
The peptides investigated here show antimicrobial activities in the 10 μM range as observed previously (Zasloff, 1987; Bevins and Zasloff, 1990), a value that drops considerably when both peptides are mixed at an equimolar ratio (Juretic et al., 1989; Matsuzaki et al., 1998; Glattard et al., 2016). The underlying reason for this synergistic enhancement remains a matter of research (Matsuzaki et al., 1998; Salnikov and Bechinger, 2011; Strandberg et al., 2013; Zerweck et al., 2017; Harmouche and Bechinger, 2018; Leber et al., 2018; Aisenbrey et al., 2019). When the fibers are tested, this synergistic enhancement is maintained. Although this synergistic effect cannot be quoted in a truly quantitative manner due to the complete lack of antimicrobial activity of the PGLa fibers at up to 50 μM concentrations, the mixed fiber retains half of the activity when compared to the non-aggregated peptide mixtures. Furthermore, a mixture of the independently prepared fibers retains considerable activity. Also, in this case the peptides can dissociate from the fiber and insert into the membrane. It has been suggested that magainin 2 and PGLa weakly interact with each other by forming parallel helical arrangements (Matsuzaki et al., 1998; Marquette and Bechinger, 2018). Although the β-sheet structure and the α-helical arrangement in membranes are different from each other it is possible that the fiber dissociates into small oligomers/dimers which may interact directly with the membrane whereby they undergo a structural transition. When added in their monomeric forms PGLa has been suggested to precondition the membrane for magainin, which then exerts its optimal activity (Matsuzaki et al., 1998; Leber et al., 2018). In an analogous manner, the presence of magainin could help PGLa to be in a more active supramolecular arrangement when encountering the bacterial membrane e.g., by dissociating into small active oligomers more easily to cite just one of many possible explanations. In this context it is worth mentioning that the 17-residue uperin 3.5, another peptide from frogs, has been shown to exist as random coil monomers, a lose helical intermediate from which fibers can form in the presence of phosphate buffer (Calabrese et al., 2016; John et al., 2019), all of which can interact with membranes (Martin et al., 2018).
So far, magainin and PGLa are thought to exert their antimicrobial and synergistic activities by partitioning into the membrane interface where they adopt amphipathic helical secondary structure (Marquette and Bechinger, 2018; Aisenbrey et al., 2019). Helices in such interfacial situations result in considerable curvature strain (Harmouche and Bechinger, 2018), but membranes can adapt until higher peptide densities cause local phase changes allowing the transient passage of ions and water (SMART model, Bechinger, 2015). Furthermore, mesophase arrangements along the membrane surface have been measured for amphipathic cationic peptide helices including magainin, PGLa and their mixtures (Aisenbrey and Bechinger, 2014; Glattard et al., 2016; Marquette and Bechinger, 2018). For mixtures of magainin 2 and PGLa parallel heterodimers have been observed (Hara et al., 2001) which can potentially enhance the membrane disruptive activities of the individual components (illustrated in Bechinger, 1999; Aisenbrey et al., 2019). Whereas, under the conditions of this study fiber formation made from β-sheets/turns has been observed in aqueous solution for PGLa and magainin 2a (Figure 1), in the presence of membranes the supramolecular ordered arrangements of the peptide-lipid complexes have been shown to encompass helical secondary structures (Matsuzaki et al., 1998; Aisenbrey et al., 2019). While for other cationic amphipathic peptide amyloid formation has been postulated to pass a loosely packed helical intermediate (John et al., 2019) future research is required to investigate the reverse process. In particular, the β-sheet fibrils in aqueous solution are capable to interact with membranes and to convert cooperatively into a helical assembly, or they have to dissociate first before interacting with lipid bilayers.
In summary, the antimicrobial peptides PGLa and magainin 2a form fibrillar aggregates in physiological aqueous solutions. Their antimicrobial activities decrease with fibril formation, but do not disappear completely. Mixing the peptides, either monomeric or in fibrillar form, results in an increased activity with little effect of the formation of fibrils. The antimicrobial activity of mixed fibrils of PGLa and magainin 2a can potentially be exploited for different future applications. Structurally, the fibrils are more resistant toward proteases and therefore constitute a possible state for long-term storage as may be the case in the granular glands. Moreover, the rapid switch to an active state upon addition of bacteria makes the fibrils ideal for surface coating of material where bacterial growth would be fatal. Finally, fibers made from peptides of similar size have been found key elements to enhance lentiviral transduction in clinical settings (Meier et al., 2014; Majdoul et al., 2016; Vermeer et al., 2017), and a number of other peptides may be suitable for the targeted delivery of macromolecules and biomolecular assemblies (Moulay et al., 2017).
Data Availability Statement
The datasets generated for this study are available on request to the corresponding author.
Author Contributions
DJ, EG, ML, and PB did the experiments. BB and DJ wrote the paper. BB acquired funding for the project. All authors contributed to the article and approved the submitted version.
Funding
The financial contributions of the Agence Nationale de la Recherche (projects MemPepSyn 14-CE34-0001-01, InMembrane 15-CE11-0017-01, Biosupramol 17-CE18-0033-3, and the LabEx Chemistry of Complex Systems 10-LABX-0026_CSC), the University of Strasbourg, the CNRS, the Région Grand-Est (Alsace), and the RTRA International Center of Frontier Research in Chemistry are gratefully acknowledged.
Conflict of Interest
The authors declare that the research was conducted in the absence of any commercial or financial relationships that could be construed as a potential conflict of interest.
Acknowledgments
The authors thank Delphine Hatey for helping with peptide synthesis and purification, Valérie Demais for the electron microscopy, the AMS and ISIS spectroscopy services for the access to the CD and FTIR spectrometers, and Peter Faller for lending the plate reader to us. BB is grateful to the Institut Universitaire de France for providing additional time to be dedicated to research.
Supplementary Material
The Supplementary Material for this article can be found online at: https://www.frontiersin.org/articles/10.3389/fcimb.2020.526459/full#supplementary-material
Abbreviations
ABC, ammonium bicarbonate; CD, circular dichroism; E. coli, Escherichia coli; EM, electron microscopy; FRET, fluorescence resonance energy transfer; FTIR, Fourier transform infrared; HCl, hydrochloric acid; HEPES, 4-(2-Hydroxyethyl)piperazine-1-ethanesulfonic acid; MALDI-TOF, matrix-assisted laser desorption ionization-time of flight; MIC, minimal inhibitory concentration; NMR, nuclear magnetic resonance; OD, optical density; PGLa, peptide starting with a glycine and ending with a leucine amide; HPLC, high performance liquid chromatography; RT, room temperature; TEM, transmission electron microscopy; TFA, trifluoroacetic acid; ThT, thioflavin T; Tris, 2-amino-2-(hydroxymethyl)-1, 3-propanediol.
References
Aisenbrey, C., and Bechinger, B. (2014). Molecular packing of amphipathic peptides on the surface of lipid membranes. Langmuir 30, 10374–10383. doi: 10.1021/la500998g
Aisenbrey, C., Marquette, A., and Bechinger, B. (2019). The mechanisms of action of cationic antimicrobial peptides refined by novel concepts from biophysical investigations. Adv. Exp. Med. Biol. 1117, 33–64. doi: 10.1007/978-981-13-3588-4_4
Auvynet, C., El Amri, C., Lacombe, C., Bruston, F., Bourdais, J., Nicolas, P., et al. (2008). Structural requirements for antimicrobial versus chemoattractant activities for dermaseptin S9. FEBS J. 275, 4134–4151. doi: 10.1111/j.1742-4658.2008.06554.x
Bechinger, B. (1999). The structure, dynamics and orientation of antimicrobial peptides in membranes by multidimensional solid-state NMR spectroscopy. Biochim. Biophys. Acta 1462, 157–183. doi: 10.1016/S0005-2736(99)00205-9
Bechinger, B. (2011). Insights into the mechanisms of action of host defence peptides from biophysical and structural investigations. J. Pept. Sci. 17, 306–314. doi: 10.1002/psc.1343
Bechinger, B. (2015). The SMART model: soft membranes adapt and respond, also transiently, to external stimuli. J. Peptide Sci. 21, 346–355 doi: 10.1002/psc.2729
Bechinger, B., Kim, Y., Chirlian, L. E., Gesell, J., Neumann, J. M., Montal, M., et al. (1991). Orientations of amphipathic helical peptides in membrane bilayers determined by solid- state NMR spectroscopy. J. Biomol. NMR 1, 167–173. doi: 10.1007/BF01877228
Beesley, J. L., and Woolfson, D. N. (2019). The de novo design of alpha-helical peptides for supramolecular self-assembly. Curr. Opin. Biotechnol. 58, 175–182. doi: 10.1016/j.copbio.2019.03.017
Bevins, C. L., and Zasloff, M. (1990). Peptides from frog skin. Ann. Rev. Biochem. 59, 395–441. doi: 10.1146/annurev.bi.59.070190.002143
Biancalana, M., and Koide, S. (2010). Molecular mechanism of Thioflavin-T binding to amyloid fibrils. Biochim. Biophys. Acta 1804, 1405–1412. doi: 10.1016/j.bbapap.2010.04.001
Caillon, L., Killian, J. A., Lequin, O., and Khemtemourian, L. (2013). Biophysical investigation of the membrane-disrupting mechanism of the antimicrobial and amyloid-like peptide dermaseptin S9. PLoS ONE 8:e75528. doi: 10.1371/journal.pone.0075528
Calabrese, A. N., Liu, Y., Wang, T., Musgrave, I. F., Pukala, T. L., Tabor, R. F., et al. (2016). The amyloid fibril-forming properties of the amphibian antimicrobial peptide uperin 3.5. Chembiochem 17, 239–246. doi: 10.1002/cbic.201500518
Chairatana, P., and Nolan, E. M. (2014). Molecular basis for self-assembly of a human host-defense peptide that entraps bacterial pathogens. J. Am. Chem. Soc. 136, 13267–13276. doi: 10.1021/ja5057906
Chakraborty, P., and Gazit, E. (2018). Amino acid based self-assembled nanostructures: complex structures from remarkably simple building blocks. Chem. Nano. Mat. 4, 730–740. doi: 10.1002/cnma.201800147
Chu, H., Pazgier, M., Jung, G., Nuccio, S. P., Castillo, P. A., De Jong, M. F., et al. (2012). Human alpha-defensin 6 promotes mucosal innate immunity through self-assembled peptide nanonets. Science 337, 477–481. doi: 10.1126/science.1218831
Depas, W. H., and Chapman, M. R. (2012). Microbial manipulation of the amyloid fold. Res. Microbiol. 163, 592–606. doi: 10.1016/j.resmic.2012.10.009
Fitzpatrick, A. W. P., Debelouchina, G. T., Bayro, M. J., Clare, D. K., Caporini, M. A., Bajaj, V. S., et al. (2013). Atomic structure and hierarchical assembly of a cross-beta amyloid fibril. Proc. Natl. Acad. Sci. U.S.A. 110, 5468–5473. doi: 10.1073/pnas.1219476110
Fusco, G., de Simone, A., Arosio, P., Vendruscolo, M., Veglia, G., and Dobson, C. M. (2016). Structural ensembles of membrane-bound alpha-synuclein reveal the molecular determinants of synaptic vesicle affinity. Sci. Rep. 6:27125. doi: 10.1038/srep27125
Gibson, B. W. (1991). “Lytic peptides from the skin secretions of Xenopus laevis: a personal perspective,” in Surface Reactive Peptides and Polymers, Peptides and Polymers, ACS Symposium series, Vol. 444, eds C.S. Sikes and A. P. Wheeler (ACS), 222–236. doi: 10.1021/bk-1991-0444.ch017
Gibson, B. W., Poulter, L., Williams, D. H., and Maggio, J. E. (1986). Novel peptide fragments originating from PGL and the Caerulein and Xenopsin precursors from Xenopus laevis. J. Biol. Chem. 261, 5341–5349.
Giovannini, M. G., Poulter, L., Gibson, B. W., and Williams, D. H. (1987). Biosynthesis and degradation of peptides derived from Xenopus laevis prohormones. Biochem. J. 243, 113–120. doi: 10.1042/bj2430113
Glattard, E., Salnikov, E. S., Aisenbrey, C., and Bechinger, B. (2016). Investigations of the synergistic enhancement of antimicrobial activity in mixtures of magainin 2 and PGLa. Biophys. Chem. 210, 35–44. doi: 10.1016/j.bpc.2015.06.002
Goormaghtigh, E., Cabiaux, V., and Ruysschaert, J. M. (1990). Secondary structure and dosage of soluble and membrane proteins by attenuated total reflection fourier-transform infrared spectroscopy on hydrated films. Eur. J. Biochem. 193, 409–420. doi: 10.1111/j.1432-1033.1990.tb19354.x
Gosztyla, M. L., Brothers, H. M., and Robinson, S. R. (2018). Alzheimer's amyloid-beta is an antimicrobial peptide: a review of the evidence. J. Alzheimers. Dis. 62, 1495–1506. doi: 10.3233/JAD-171133
Hainline, K. M., Fries, C. N., and Collier, J. H. (2018). Progress toward the clinical translation of bioinspired peptide and protein assemblies. Adv. Healthc. Mater. 7:1700930. doi: 10.1002/adhm.201700930
Hancock, R. E. (1984). Alterations in outer membrane permeability. Annu. Rev. Microbiol. 38, 237–264. doi: 10.1146/annurev.mi.38.100184.001321
Hara, T., Mitani, Y., Tanaka, K., Uematsu, N., Takakura, A., Tachi, T., et al. (2001). Heterodimer formation between the antimicrobial peptides magainin 2 and PGLa in lipid bilayers: a cross-linking study. Biochemistry 40, 12395–12399. doi: 10.1021/bi011413v
Harmouche, N., and Bechinger, B. (2018). Lipid-mediated interactions between the amphipathic antimicrobial peptides magainin 2 and PGLa in phospholipid bilayers. Biophys. J. 115, 1033–1044. doi: 10.1016/j.bpj.2018.08.009
Jang, H., Arce, F. T., Mustata, M., Ramachandran, S., Capone, R., Nussinov, R., et al. (2011). Antimicrobial protegrin-1 forms amyloid-like fibrils with rapid kinetics suggesting a functional link. Biophys. J. 100, 1775–1783. doi: 10.1016/j.bpj.2011.01.072
John, T., Dealey, T. J. A., Gray, N. P., Patil, N. A., Hossain, M. A., Abel, B., et al. (2019). The kinetics of amyloid fibrillar aggregation of uperin 3.5 is directed by the peptide's secondary structure. Biochemistry 58, 3656–3668. doi: 10.1021/acs.biochem.9b00536
Juhl, D. W., Risor, M. W., Scavenius, C., Rasmussen, C. B., Otzen, D., Nielsen, N. C., et al. (2019). Conservation of the amyloid interactome across diverse fibrillar structures. Sci. Rep. 9:3863. doi: 10.1038/s41598-019-40483-z
Juretic, D., Hendler, R. W., Zasloff, M., and Westerhoff, H. V. (1989). Cooperative action of magainins in disrupting membrane-linked free-energy transduction. Biophys. J. 55, 6597–6601.
Kiss, G., and Michl, H. (1962). Über das giftsekret der gelbbauchunke Bombina variegata L. Toxicon. 1, 33–34. doi: 10.1016/0041-0101(62)90006-5
Kumar, V. A., Wang, B. K., and Kanahara, S. M. (2016). Rational design of fiber forming supramolecular structures. Exp. Biol. Med. 241, 899–908. doi: 10.1177/1535370216640941
Leber, R., Pachler, M., Kabelka, I., Svoboda, I., Enkoller, D., Vácha, R., et al. (2018). Synergism of antimicrobial frog peptides couples to membrane intrinsic curvature strain. Biophys. J. 114, 1945–1954. doi: 10.1016/j.bpj.2018.03.006
Mahalka, A. K., and Kinnunen, P. K. (2009). Binding of amphipathic alpha-helical antimicrobial peptides to lipid membranes: lessons from temporins B and L. Biochim. Biophys. Acta 1788, 1600–1609. doi: 10.1016/j.bbamem.2009.04.012
Majdoul, S., Seye, A. K., Kichler, A., Holic, N., Galy, A., Bechinger, B., et al. (2016). Molecular determinants of vectofusin-1 and its derivatives for the enhancement of lentivirally mediated gene transfer into hematopoietic stem/progenitor cells. J. Biol. Chem. 291, 2161–2169. doi: 10.1074/jbc.M115.675033
Maji, S. K., Perrin, M. H., Sawaya, M. R., Jessberger, S., Vadodaria, K., Rissman, R. A., et al. (2009). Functional amyloids as natural storage of peptide hormones in pituitary secretory granules. Science 325, 328–332. doi: 10.1126/science.1173155
Marquette, A., and Bechinger, B. (2018). Biophysical investigations elucidating the mechanisms of action of antimicrobial peptides and their synergism. Biomolecules 8:E18. doi: 10.3390/biom8020018
Marquette, A., Salnikov, E., Glattard, E., Aisenbrey, C., and Bechinger, B. (2015). Magainin 2-PGLa interactions in membranes - two peptides that exhibit synergistic enhancement of antimicrobial activity. Curr. Top. Med. Chem. 16, 65–75. doi: 10.2174/1568026615666150703115701
Martin, L. L., Kubeil, C., Piantavigna, S., Tikkoo, T., Gray, N. P., John, T., et al. (2018). Amyloid aggregation and membrane activity of the antimicrobial peptide uperin 3.5. Pep. Sci. 110:e24052. doi: 10.1002/pep2.24052
Matsuzaki, K., Mitani, Y., Akada, K., Murase, O., Yoneyama, S., Zasloff, M., et al. (1998). Mechanism of synergism between antimicrobial peptides magainin 2 and PGLa. Biochemistry 37, 15144–15153. doi: 10.1021/bi9811617
Matsuzaki, K., Sugishita, K., Fujii, N., and Miyajima, K. (1995). Molecular basis for membrane selectivity of an antimicrobial peptide, magainin 2. Biochemistry 34, 3423–3429. doi: 10.1021/bi00010a034
Meier, C., Weil, T., Kirchhoff, F., and Munch, J. (2014). Peptide nanofibrils as enhancers of retroviral gene transfer. Wiley Interdiscip. Rev. Nanomed. Nanobiotechnol. 6, 438–451. doi: 10.1002/wnan.1275
Moulay, G., Leborgne, C., Mason, A. J., Aisenbrey, C., Kichler, A., and Bechinger, B. (2017). Histidine-rich designer peptides of the LAH4 family promote cell delivery of a multitude of cargo. J. Pept. Sci. 23, 320–328. doi: 10.1002/psc.2955
Oren, Z., and Shai, Y. (1998). Mode of action of linear amphipathic alpha-helical antimicrobial peptides. Biopolymers 47, 451–463.
Perrin, B. S. Jr., Sodt, A. J., Cotten, M. L., and Pastor, R. W. (2015). The curvature induction of surface-bound antimicrobial peptides piscidin 1 and piscidin 3 varies with lipid chain length. J. Membr. Biol. 248, 455–467. doi: 10.1007/s00232-014-9733-1
Pino-Angeles, A., Leveritt, J. M. III., and Lazaridis, T. (2016). Pore structure and synergy in antimicrobial peptides of the magainin family. PLoS Comput. Biol. 12:e1004570. doi: 10.1371/journal.pcbi.1004570
Porcelli, F., Ramamoorthy, A., Barany, G., and Veglia, G. (2013). On the role of NMR spectroscopy for characterization of antimicrobial peptides. Methods Mol. Biol. 1063, 159–180. doi: 10.1007/978-1-62703-583-5_9
Qiang, W., Yau, W. M., Lu, J. X., Collinge, J., and Tycko, R. (2017). Structural variation in amyloid-beta fibrils from Alzheimer's disease clinical subtypes. Nature 541, 217–221. doi: 10.1038/nature20814
Ramamoorthy, A. (2018). Protein aggregation and amyloidosis. Biochim. Biophys. Acta. 1860, 1601–1602. doi: 10.1016/j.bbamem.2018.05.017
Resende, J. M., Verly, R. M., Aisenbrey, C., Amary, C., Bertani, P., Pilo-Veloso, D., et al. (2014). Membrane interactions of phylloseptin-1,−2, and−3 peptides by oriented solid-state NMR spectroscopy. Biophys. J. 107, 901–911 doi: 10.1016/j.bpj.2014.07.014
Risor, M. W., Juhl, D. W., Bjerring, M., Mathiesen, J., Enghild, J. J., Nielsen, N. C., et al. (2017). Critical influence of cosolutes and surfaces on the assembly of serpin-derived amyloid fibrils. Biophys. J. 113, 580–596. doi: 10.1016/j.bpj.2017.06.030
Robinson, S. R., and Bishop, G. M. (2002). Abeta as a bioflocculant: implications for the amyloid hypothesis of Alzheimer's disease. Neurobiol. Aging 23, 1051–1072. doi: 10.1016/S0197-4580(01)00342-6
Roversi, D., Luca, V., Aureli, S., Park, Y., Mangoni, M. L., and Stella, L. (2014). How many AMP molecules kill a bacterium? Spectroscopic determination of PMAP-23 binding to E. Coli. ACS Chem. Biol. 9, 2003–2007. doi: 10.1021/cb500426r
Salnikov, E., and Bechinger, B. (2011). Lipid-controlled peptide topology and interactions in bilayers: structural insights into the synergistic enhancement of the antimicrobial activities of PGLa and magainin 2. Biophysical J. 100, 1473–1480. doi: 10.1016/j.bpj.2011.01.070
Salnikov, E. S., Anantharamaiah, G. M., and Bechinger, B. (2018). Supramolecular organization of Apolipoprotein-A-I-derived peptides within disc-like arrangements. Biophys. J. 115, 467–477. doi: 10.1016/j.bpj.2018.06.026
Salnikov, E. S., Raya, J., de Zotti, M., Zaitseva, E., Peggion, C., Ballano, G., et al. (2016). Alamethicin supramolecular organization in lipid membranes from 19F solid- state NMR. Biophys. J. 111, 2450–2459. doi: 10.1016/j.bpj.2016.09.048
Sansom, M. S. P. (1991). The biophysics of peptide models of ion channels. Prog. Biophys. Mol. Biol. 55, 139–235. doi: 10.1016/0079-6107(91)90004-C
Savini, F., Luca, V., Bocedi, A., Massoud, R., Park, Y., Mangoni, M. L., et al. (2017). Cell-density dependence of host-defense peptide activity and selectivity in the presence of host cells. ACS Chem. Biol. 12, 52–56. doi: 10.1021/acschembio.6b00910
Schubeis, T., Yuan, P., Ahmed, M., Nagaraj, M., Van Rossum, B. J., and Ritter, C. (2015). Untangling a repetitive amyloid sequence: correlating biofilm-derived and segmentally labeled curli fimbriae by solid-state NMR spectroscopy. Angew. Chem. Int. Ed. Engl. 54, 14669–14672. doi: 10.1002/anie.201506772
Shivu, B., Seshadri, S., Li, J., Oberg, K. A., Uversky, V. N., and Fink, A. L. (2013). Distinct beta-sheet structure in protein aggregates determined by ATR-FTIR spectroscopy. Biochemistry 52, 5176–5183. doi: 10.1021/bi400625v
Sood, R., Domanov, Y., Pietiainen, M., Kontinen, V. P., and Kinnunen, P. K. (2008). Binding of LL-37 to model biomembranes: insight into target vs host cell recognition. Biochim. Biophys. Acta 1778, 983–996. doi: 10.1016/j.bbamem.2007.11.016
Soravia, E., Martini, G., and Zasloff, M. (1988). Antimicrobial properties of peptides from Xenopus granular gland secretions. FEBS Lett. 228, 337–340. doi: 10.1016/0014-5793(88)80027-9
Soscia, S. J., Kirby, J. E., Washicosky, K. J., Tucker, S. M., Ingelsson, M., Hyman, B., et al. (2010). The Alzheimer's disease-associated amyloid beta-protein is an antimicrobial peptide. PLoS ONE 5:e9505. doi: 10.1371/journal.pone.0009505
Strandberg, E., Zerweck, J., Wadhwani, P., and Ulrich, A. S. (2013). Synergistic insertion of antimicrobial magainin-family peptides in membranes depends on the lipid spontaneous curvature. Biophys. J. 104, L09–L11. doi: 10.1016/j.bpj.2013.01.047
Sun, B., Tao, K., Jia, Y., Yan, X., Zou, Q., Gazit, E., et al. (2019). Photoactive properties of supramolecular assembled short peptides. Chem. Soc. Rev. 48, 4387–4400. doi: 10.1039/C9CS00085B
Tayeb-Fligelman, E., Tabachnikov, O., Moshe, A., Goldshmidt-Tran, O., Sawaya, M. R., Coquelle, N., et al. (2017). The cytotoxic Staphylococcus aureus PSMalpha3 reveals a cross-alpha amyloid-like fibril. Science 355, 831–833. doi: 10.1126/science.aaf4901
Torrent, M., Andreu, D., Nogues, V. M., and Boix, E. (2011a). Connecting peptide physicochemical and antimicrobial properties by a rational prediction model. PLoS ONE 6:e16968. doi: 10.1371/journal.pone.0016968
Torrent, M., Valle, J., Nogues, M. V., Boix, E., and Andreu, D. (2011b). The generation of antimicrobial peptide activity: a trade-off between charge and aggregation? Angew. Chem. Int. Ed. Engl. 50, 10686–10689. doi: 10.1002/anie.201103589
Tremouilhac, P., Strandberg, E., Wadhwani, P., and Ulrich, A. S. (2006). Conditions affecting the re-alignment of the antimicrobial peptide PGLa in membranes as monitored by solid state 2H-NMR. Biochim. Biophys. Acta 1758, 1330–1342. doi: 10.1016/j.bbamem.2006.02.029
Urrutia, R., Cruciani, R. A., Barker, J. L., and Kachar, B. (1989). Sponaneous polymerisation of the antibiotic peptide magainin 2. FEBS Lett. 247, 17–21. doi: 10.1016/0014-5793(89)81230-X
Vermeer, L. S., Hamon, L., Schirer, A., Schoup, M., Cosette, J., Majdoul, S., et al. (2017). The transduction enhancing peptide vectofusin-1 forms pH-dependent α-helical coiled-coil nanofibrils, trapping viral particles. Acta Biomater. 64, 259–268. doi: 10.1016/j.actbio.2017.10.009
Westerhoff, H. V., Zasloff, M., Rosner, J. L., Hendler, R. W., de Waal, A., Vaz, G., et al. (1995). Functional synergism of the magainins PGLa and magainin-2 in Escherichia coli, tumor cells and liposomes. Eur. J. Biochem. 228, 257–264. doi: 10.1111/j.1432-1033.1995.00257.x
Wieprecht, T., Apostolov, O., Beyermann, M., and Seelig, J. (2000). Membrane binding and pore formation of the antibacterial peptide PGLa: thermodynamic and mechanistic aspects. Biochemistry 39, 442–452. doi: 10.1021/bi992146k
Zapadka, K. L., Becher, F. J., Gomes Dos Santos, A. L., and Jackson, S. E. (2017). Factors affecting the physical stability (aggregation) of peptide therapeutics. Interf. Focus 7:20170030. doi: 10.1098/rsfs.2017.0030
Zasloff, M. (1987). Magainins, a class of antimicrobial peptides from Xenopus skin: isolation, characterization of two active forms, and partial cDNA sequence of a precursor. Proc. Natl. Acad. Sci. U.S.A. 84, 5449–5453. doi: 10.1073/pnas.84.15.5449
Zasloff, M. (2002). Antimicrobial peptides of multicellular organisms. Nature 415, 389–395. doi: 10.1038/415389a
Zasloff, M., Martin, B., and Chen, H. C. (1988). Antimicrobial activity of synthetic magainin peptides and several analogues. Proc. Natl. Acad. Sci. U.S.A. 85, 910–913. doi: 10.1073/pnas.85.3.910
Keywords: amyloid fiber, antimicrobial peptide, peptide-lipid interaction, supramolecular assembly, gradual release
Citation: Juhl DW, Glattard E, Lointier M, Bampilis P and Bechinger B (2020) The Reversible Non-covalent Aggregation Into Fibers of PGLa and Magainin 2 Preserves Their Antimicrobial Activity and Synergism. Front. Cell. Infect. Microbiol. 10:526459. doi: 10.3389/fcimb.2020.526459
Received: 13 January 2020; Accepted: 18 August 2020;
Published: 30 September 2020.
Edited by:
Philippe Bulet, INSERM U1209 Institut pour l'Avancée des Biosciences (IAB), FranceReviewed by:
John Adrian Carver, Australian National University, AustraliaDaniel Carvalho Pimenta, Butantan Institute, Brazil
Copyright © 2020 Juhl, Glattard, Lointier, Bampilis and Bechinger. This is an open-access article distributed under the terms of the Creative Commons Attribution License (CC BY). The use, distribution or reproduction in other forums is permitted, provided the original author(s) and the copyright owner(s) are credited and that the original publication in this journal is cited, in accordance with accepted academic practice. No use, distribution or reproduction is permitted which does not comply with these terms.
*Correspondence: Burkhard Bechinger, YmVjaGluZ2VAdW5pc3RyYS5mcg==
†Present address: Dennis Wilkens Juhl, Department of Chemistry, Aarhus University, Aarhus, Denmark