- 1College of Life Sciences and Engineering, Foshan University, Foshan, China
- 2Key Laboratory of Zoonosis, Ministry of Education, College of Veterinary Medicine, Jilin University, Changchun, China
Toxoplasma gondii (T. gondii) can cause zoonotic toxoplasmosis worldwide. Neutrophil extracellular traps (NETs) have been known as a novel effector mechanism against T. gondii infection in the innate system of humans, cats, and sheep. Dogs are the intermediate host of T. gondii, in which the use of NETs against T. gondii infection remains unclear. Thus, this study aims to examine the effects of T. gondii on NETs release in dogs, and to further investigate the mechanism involved in the process. T. gondii-triggered NETs were analyzed by scanning electron microscopy (SEM) and fluorescence confocal microscopy, and the mechanism of T. gondii-triggered NETs release was determined by using inhibitors and a fluorometric reader. The results showed that T. gondii tachyzoites significantly triggered NETs-like structures, which consisted of DNA decorated with neutrophil elastase (NE) and myeloperoxidase (MPO). Further investigations revealed that reactive oxygen species (ROS)-, NADPH oxidase-, Rac 1- or p38 mitogen-activated protein kinase (MAPK)-signaling pathways were relevant to T. gondii tachyzoites-triggered NETs release. Moreover, zymosan-triggered NETs release was strikingly degraded by T. gondii tachyzoites treatment, indicating that T. gondii may escape from the NETs-based capture strategy. Taken together, promoting NETs release is suggested to limit motility and evade infection of T. gondii in dogs.
Introduction
Toxoplasma gondii is considered as one of the most prevalent zoonotic parasites worldwide (Tenter et al., 2000; Dubey and Jones, 2008; Dubey and Dubey, 2010; Lopes et al., 2014; Pan et al., 2017). It may cause asymptomatic infection or severe symptomatic infection including developmental defects, early embryonic death, stillbirth, or abortion to T. gondii-infected pregnancies (Dubey and Jones, 2008; Dubey and Dubey, 2010; Pan et al., 2017). Dogs are the intermediate hosts, and multiple studies have demonstrated the prevalence of T. gondii in dogs in a variety of countries or areas worldwide (Jiang et al., 2015; Zhang et al., 2015; Dubey et al., 2016; Rengifo-Herrera et al., 2017; Zarra-Nezhad et al., 2017). Although these serological surveys and diagnosis analysis of dogs infected T. gondii have been reported, the interactions between the host dog and the parasite T. gondii remain not fully understood.
Neutrophil extracellular traps (NETs) are reported as a novel effector mechanism of polymorphonuclear neutrophils (PMNs) in the host immune system against infection. Since NETs were first discovered to entrap and kill extracellular bacteria (Brinkmann et al., 2004), this novel effector mechanism has also been reported to be involved in immune system response against several pathogens, such as viruses (Wardini et al., 2010; Saitoh et al., 2012), fungi (Jin et al., 2016; Urban and Nett, 2018), or parasites (Munoz-Caro et al., 2015a; Wei et al., 2016). It also has been shown that NETs against T. gondii infection exist in a variety of species including humans (Abi Abdallah et al., 2012), sheep (Yildiz et al., 2017), mice (Abi Abdallah et al., 2012), cattle (Yildiz et al., 2017), cats (Lacerda et al., 2019), harbor seals (Reichel et al., 2015), and dolphins (Imlau et al., 2020). NETs release can damage or kill T. gondii, but whether the NETs-based effector mechanism during T. gondii infection also works in the innate immune system of dogs remains unknown.
Various studies have demonstrated that the mechanism of NETs release is associated with multiple molecular or signaling pathways, such as NADPH oxidase, Rac, or p38 MAPK signaling pathways (Lim et al., 2011; Munoz-Caro et al., 2015a; Wei et al., 2016, 2018a; Gavillet et al., 2018). Furthermore, T. gondii triggered-NETs release in harbor seals is also a NADPH oxidase-, NE- and MPO-, store-operated calcium entry (SOCE)-dependent process (Reichel et al., 2015). The key molecular and signaling pathways involved in T. gondii-triggered NETs release in dogs still needs further investigation. In this study, we examined for the first time if NETs release in dogs during T. gondii infection, and also investigated the potential mechanism undergoing these processes.
Materials and Methods
Parasites
Toxoplasma gondii tachyzoites of RH strain were inoculated and cultured in Vero cells at 37°C and 5% CO2, T. gondii tachyzoites were harvested by passing through a 20 mL syringe and a 27-gauge needle three times and was purified by filtrum (5 μm). Finally, these tachyzoites were centrifuged at 3,000 r/min for 10 min and washed two times.
Experimental Animals
Blood was collected from three healthy adult dogs, and the PMNs were isolated in the available dog PMN isolation kit® (TianJin HaoYang Biological Manufacture CO., China) as previously described (Wei et al., 2016, 2018b).
Ethics Statement
Animal experiments were approved by the Ethics Committee on the Care and Use of Laboratory Animals of Foshan University and in accordance with the current Animal Protection Laws of China.
Scanning Electron Microscopy (SEM) Analysis
T. gondii tachyzoite-triggered NETs release were examined by SEM as previously described (Wei et al., 2016). In brief, PMNs were incubated with viable T. gondii tachyzoites at the ratio of 1:1 for 90 min. After the specimens were fixed with 4.0% glutaraldehyde, and post-fixed with 1.0% osmium tetroxide (Merck), they were observed under scanning electron microscope (Hitachi S-3400N, Japan).
Fluorescence Confocal Microscopy Analysis
The components of T. gondii tachyzoite-triggered NETs were detected with fluorescence confocal microscopy analysis as described elsewhere (Wei et al., 2016, 2018b). Briefly, the newly isolated PMNs were incubated with T. gondii tachyzoites at the ratio of 1:1 for 90 min. After being fixed with paraformaldehyde, the specimens were permeabilized with Triton X-100 and blocked with goat serum/PBS. Finally, the specimens were incubated with the specific antibodies against proteins in T. gondii tachyzoites-triggered NETs structures. In parallel experiments, PMNs were co-treated with zymosan (1 mg/mL) and T. gondii tachyzoites for 120 min. The NETs specific antibodies included the anti-histone antibody (LS-C353149; Life Span BioSciences, Inc) and the anti-myeloperoxidase (MPO) antibody (Orb16003; Biorbyt). DNA in T. gondii tachyzoites-triggered NETs structures were stained with 5 μM Sytox Orange (Invitrogen), and the images were observed and analyzed with a scanning confocal microscope (Olympus FluoView FV1000).
Quantitation of T. gondii-Triggered NETs
PMNs were incubated with viable T. gondii tachyzoites (ratio 1:1, 1:3, or 1:6) for 90 min. To determine if T. gondii-triggered NETs were time-dependent, PMNs were incubated with viable T. gondii tachyzoites (ratio 1:1) for 10, 30, 60, 90, or 120 min. In inhibition tests, cells were pre-treated with 10 μM of the NADPH oxidase inhibitor Diphenyleneiodonium chloride (DPI, Sigma-Aldrich), 10 μM of the p38 MAPK (Sigma-Aldrich) signaling pathway inhibitor SB202190, 100 μM of the Rac1 activation inhibitor NSC23766, or 8.0 nM of the NLRP3 inhibitor MCC950 for 15 min. DNase I (90 U) was also pre-treated with cells for 15 min. Zymosan (1 mg/mL) was used as positive control. Finally, the release of T. gondii-triggered NETs was quantified with Pico Green® (Invitrogen) and the fluorometric reader Infiniti M200® (Tecan, Austria).
Reactive Oxygen Species (ROS) Levels Analysis
To determine ROS levels in the process of T. gondii-triggered NETs release, PMNs were incubated with viable T. gondii tachyzoites (ratio 1:1 or 1:2) for 90 min. T. gondii tachyzoites-induced ROS levels in the process of NETs release were tested by using 2, 7 dichlorofluorescein diacetate (Sigma-Aldrich) and the fluorometric reader Infiniti M200® (Tecan, Austria).
LDH Levels Analysis
To further differentiate T. gondii tachyzoites-triggered NETosis from necrosis, LDH levels were tested. PMNs were incubated with viable T. gondii tachyzoites (ratio 1:1, 1:2, or 1:4) for 90 min. T. gondii tachyzoites-induced LDH levels in the process of NETs release were tested by the LDH Cytotoxicity Assay kit® (Beyotime Biotechnology, China).
Statistical Analysis
Data were expressed as the means ± standard deviation (SD). One-way analysis of variance (ANOVA) with Tukey's multiple comparison tests was performed by using the GraphPad 5.0 software to analyze the differences among groups. The level of P ≤ 0.05 was considered as significant.
Results
T. gondii Tachyzoites Triggered Dog NETs Release
T. gondii tachyzoites significantly triggered NETs-like structures (Figure 1), and it was found that T. gondii tachyzoites were captured by thicker (Figures 1F–I) or thinner (Figures 1C–E) NETs-like structures.
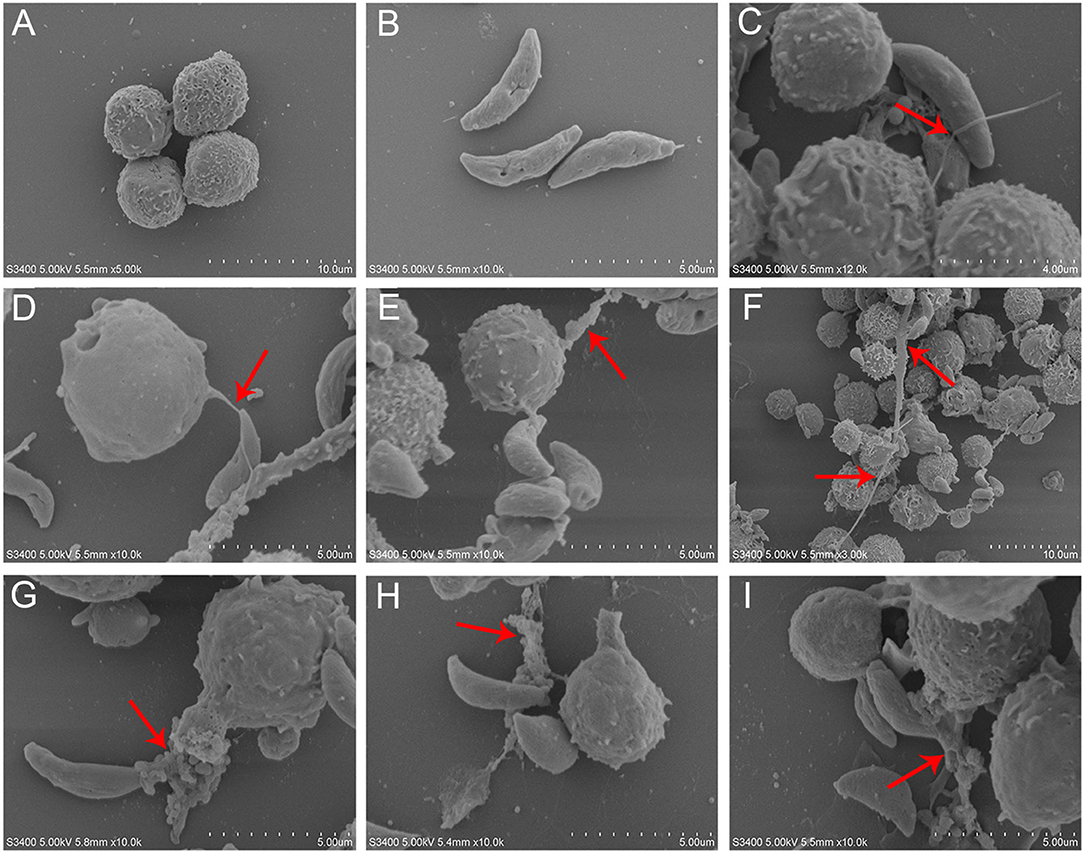
Figure 1. SEM analysis of T. gondii tachyzoites-triggered dog NETs release. (A) PMNs. (B) T. gondii tachyzoites. (C–E) Thinner NETs-like structures triggered by T. gondii tachyzoites. (F–I) thicker NETs-like structures triggered by T. gondii tachyzoites. Red arrows showed NETs-like structures triggered by T. gondii tachyzoites.
NE and MPO Co-located With DNA in T. gondii Tachyzoites-Triggered NETs Release
To confirm, if T. gondii tachyzoites-triggered NETs have similar characteristics to the typical NETs-structures, the constituent proteins in T. gondii tachyzoites-triggered NETs-like network structures were analyzed. These results showed that T. gondii tachyzoites significantly triggered NETs-like structures, and these structures consisted of DNA (Figures 2E,K) decorated with NE (Figure 2D) and MPO (Figure 2J).
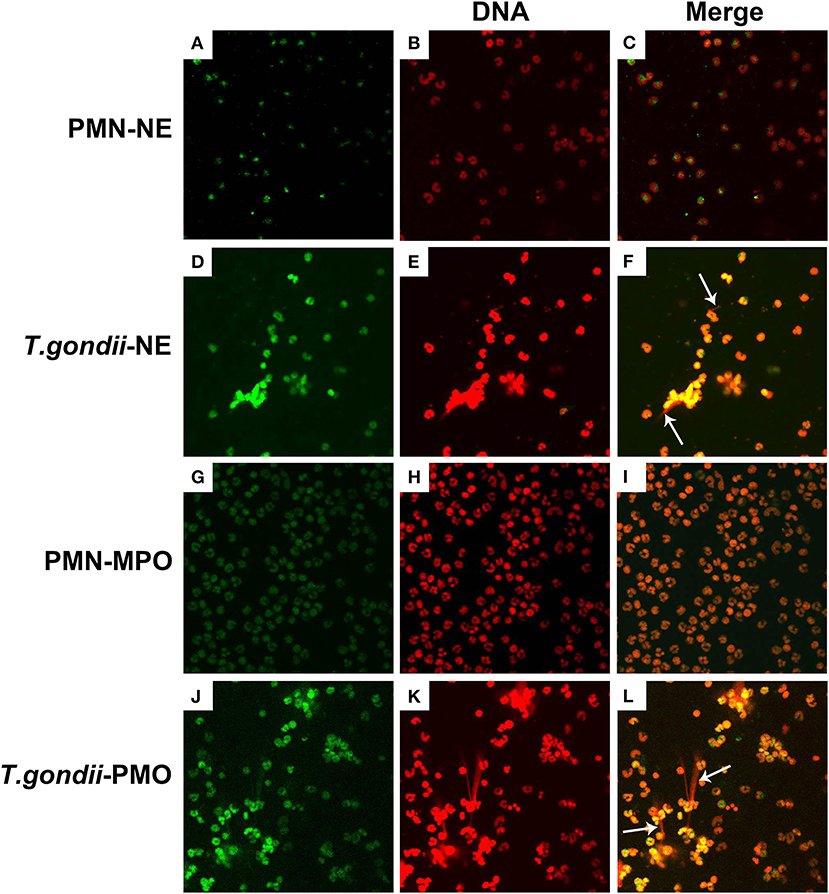
Figure 2. Fluorescence confocal microscopy analysis of T. gondii tachyzoites-triggered NETs consisting of NE and MPO with DNA. PMNs were incubated with viable T. gondii tachyzoites at a ratio of 1:1 for 90 min. The images were observed and collected by fluorescence confocal microscopy. (A,D) NE (Green). (G,J) MPO (Green). (B,E,H,K) DNA (Red). (C) Merge of (A,B). (F) Merge of (D,E). (I) Merge of (G,H). (L) Merge of (J,K). White arrow showed NETs triggered by T. gondii tachyzoites.
Quantitation of NETs Release Triggered by T. gondii Tachyzoites
As shown in Figure 3A, T. gondii tachyzoites significantly triggered NETs release compared to control groups, and the release of NETs was in a dose-dependent manner (P < 0.01, n = 3). Further experiments revealed that T. gondii tachyzoites-triggered NETs release within 60 min which was in a time-independent manner (P = 0.002, n = 5), and it was of great interest that the increasing of NETs release significantly decreased at the time point of 90 min (P = 0.034, n = 5) and 120 min (P = 0.925, n = 5; Figure 3B). It was also shown that DNase I significantly decreased T. gondii tachyzoites-triggered NETs release (P < 0.01, n = 3; Figure 4A), which further confirmed the peculiarity of DNA in these T. gondii tachyzoites-triggered NETs structures.
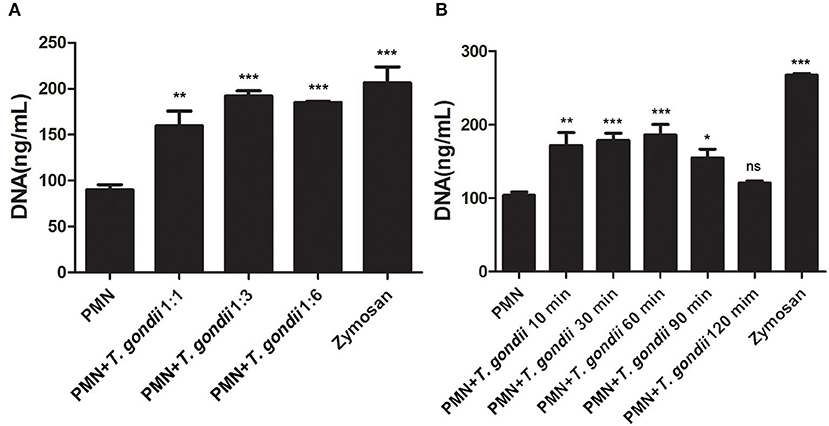
Figure 3. (A) T. gondii-triggered NETs in a dose-dependent manner. PMNs were incubated with viable T. gondii tachyzoites (ratio 1:1, 1:3, or 1:6) for 90 min. Zymosan was used as positive control. Data were expressed as mean ± SD (n = 3). (B) Dynamic analysis of T. gondii-triggered NETs release. PMNs were incubated with viable T. gondii tachyzoites (ratio 1:1) for 10, 30, 60, 90, or 120 min. The NETs release triggered by T. gondii was quantified with Pico Green and the fluorometric reader. Data were expressed as mean ± SD (n = 5). P < 0.05 were considered significant (*P < 0.05, **P < 0.01, ***P < 0.001 and “ns,” not significant).
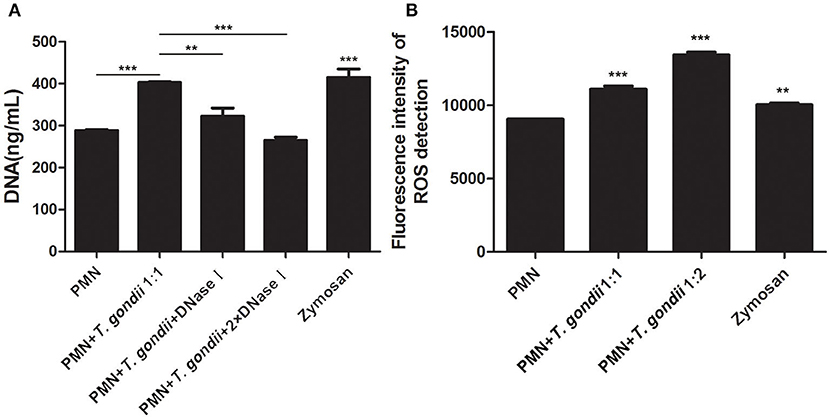
Figure 4. (A) T. gondii-triggered NETs release degraded by DNase I. PMNs were pretreated with DNase I (90 U) or DNase I (180 U) for 15 min, and then incubated with viable T. gondii tachyzoites (ratio 1:1) for 90 min. NETs release triggered by T. gondii was quantified with Pico Green and the fluorometric reader. Data were expressed as mean ± SD (n = 3). (B) Increased ROS levels in T. gondii tachyzoites-triggered NETs release. PMNs were incubated with viable T. gondii tachyzoites (ratio 1:1 or 1:2) for 90 min. NETs release triggered by T. gondii was tested by the fluorometric reader. Data were expressed as mean ± SD (n = 3). P < 0.05 were considered significant (**P < 0.01 and ***P < 0.001).
Increased ROS Levels in T. gondii Tachyzoites-Triggered NETs Release
It is reported that NETs formation has been associated with ROS production, thus we analyzed ROS levels in the process of T. gondii tachyzoites-triggered NETs release. The results showed that T. gondii tachyzoites significantly increased the levels of ROS compared to control groups (P < 0.001, n = 3; Figure 4B). Furthermore, zymosan also significantly induced ROS production in PMNs (P = 0.009, n = 3), but lower than that of T. gondii tachyzoites (Figure 4B).
T. gondii Tachyzoites-Triggered NETs Release Decreased by NADPH Oxidase-, Rac-, and p38 MAPK Signaling Pathways-Inhibitor Treatments
Inhibition experiments revealed that the NADPH oxidase inhibitor DPI (P < 0.001), the p38 MAPK signaling pathway inhibitor SB202190 (P < 0.01), and the Rac1 activation inhibitor NSC23766 (P < 0.001, n = 5) significantly decreased the release of T. gondii tachyzoites-triggered NETs in varying degrees (Figures 5A,B), while the NLRP3 inhibitor MCC950 slightly decreased NETs release but was not significant (P = 0.999, n = 5; Figure 5B).
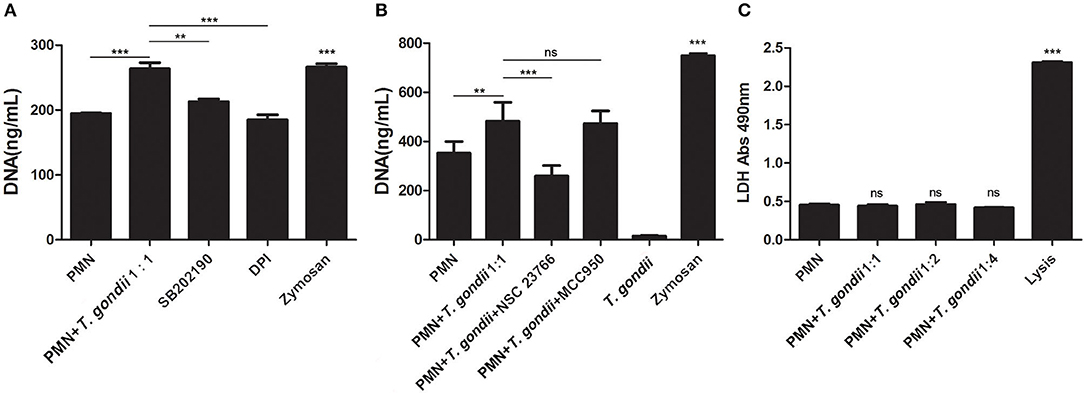
Figure 5. (A) T. gondii-triggered NETs release inhibited by DPI or SB202190. PMNs were pre-treated with the NADPH oxidase inhibitor DPI or the p38 MAPK signaling pathway inhibitor SB202190 for 15 min, and then incubated with viable T. gondii tachyzoites (ratio 1:1) for 90 min. NETs release triggered by T. gondii was quantified with Pico Green and the fluorometric reader. Data were expressed as mean ± SD (n = 3). (B) T. gondii-triggered NETs release inhibited by NSC23766. PMNs were pre-treated with the Rac1 activation inhibitor NSC23766 or the NLRP3 inhibitor MCC950 for 15 min, and then incubated with viable T. gondii tachyzoites (ratio 1:1) for 90 min. NETs release triggered by T. gondii was quantified with Pico Green and the fluorometric reader. Data were expressed as mean ± SD (n = 5). P < 0.05 were considered significant. (C). No changes of LDH levels in T. gondii tachyzoites-triggered NETs release. PMNs were incubated with T. gondii tachyzoites (ratio 1:1, 1:2, or 1:4) for 90 min, and LDH activities in supernatant were tested by the LDH kits. The lysis reagent was provided by the kit which was used as positive control. Data were expressed as mean ± SD (n = 5). P < 0.05 were considered significant (**P < 0.01, ***P < 0.001, and ns, means not significant).
No Changes of LDH Levels in T. gondii Tachyzoites-Triggered NETs Release
LDH is a typical indicator of cell necrosis. To further investigate if cell necrosis also exists in the process of T. gondii tachyzoites-triggered NETs release, LDH levels in culture supernatant were examined. As shown in Figure 5C, there was no significant changes in LDH levels were detected in the process of T. gondii tachyzoites-triggered NETs release (P > 0.05, n = 3), but LDH levels were significantly increased by the lysis (provided by LDH kits; P < 0.001, n = 3).
T. gondii Tachyzoites Escaped From NETs Release
PMNs were co-treated with zymosan and T. gondii tachyzoites to clarify if T. gondii could escape from NETs. As shown in Figures 6, 7, NETs release triggered by T. gondii tachyzoites was slightly observed at the time point of 120 min, while zymosan significantly triggered NETs compared to negative controls. However, the release of zymosan-triggered NETs was strikingly degraded by T. gondii tachyzoites treatment (Figures 6L, 7L). These NETs structures triggered by T. gondii tachyzoites consisted of NE (Figures 6D,G,J) and MPO (Figures 7D,G,J) with DNA (Figures 6E,H,K, 7E,H,K).
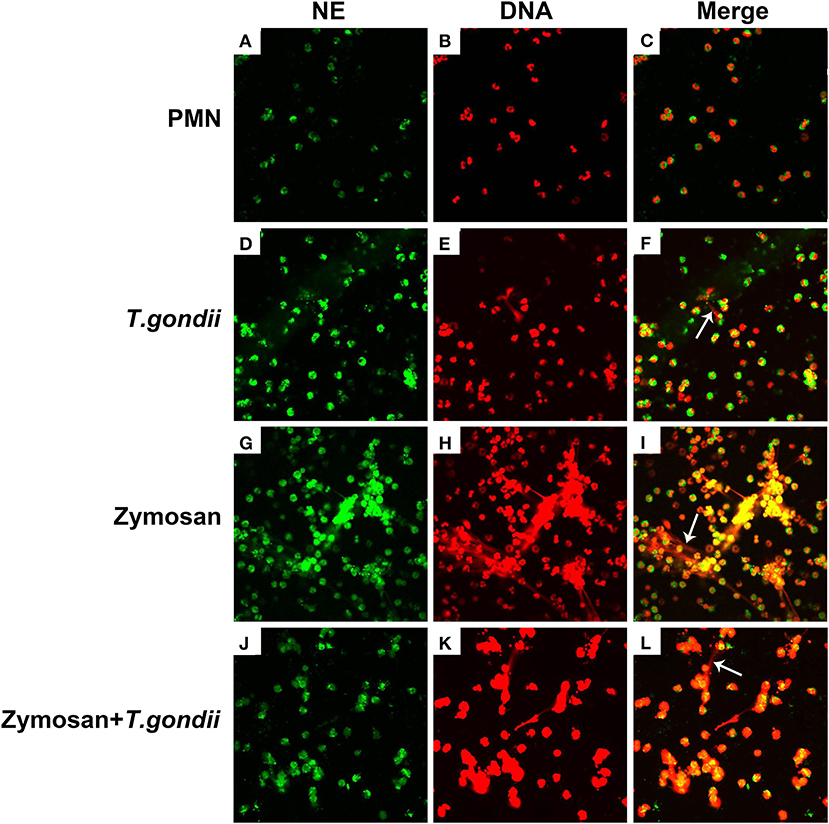
Figure 6. Zymosan-induced NETs (DNA decorated with NE) were degraded by T. gondii tachyzoites. PMNs were incubated with viable T. gondii tachyzoites (ratio 1:1) or zymosan (1 mg/mL) for 120 min. The images were observed and collected by fluorescence confocal microscopy. (A,D,G,J) NE (Green). (B,E,H,K) DNA (Red). (C) Merge of (A,B). (F) Merge of (D,E). (I) Merge of (G,H). (L) Merge of (J,K). White arrow showed NETs triggered by T. gondii tachyzoites.
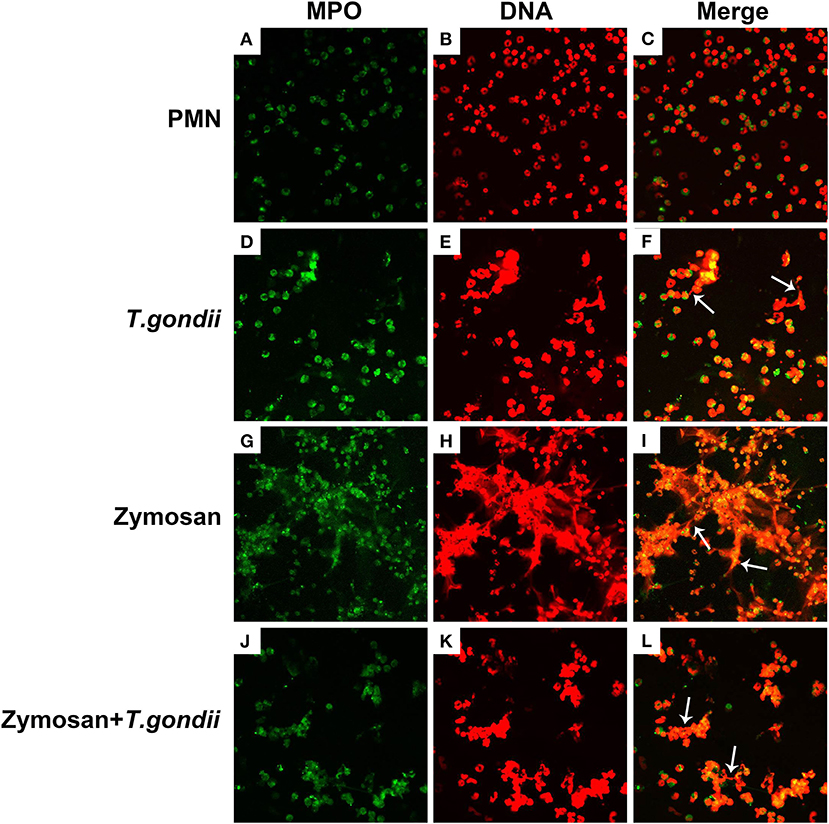
Figure 7. Zymosan-induced NETs (DNA decorated with MPO) were degraded by T. gondii tachyzoites. PMNs were incubated with viable T. gondii tachyzoites (ratio 1:1) or zymosan (1 mg/mL) for 120 min. The images were observed and collected by fluorescence confocal microscopy. (A,D,G,J) MPO (Green). (B,E,H,K) DNA (Red). (C) Merge of (A,B). (F) Merge of (D,E). (I) Merge of (G,H). (L) Merge of (J,K). White arrow showed NETs triggered by T. gondii tachyzoites.
Discussion
In the present study, NETs release triggered by T. gondii tachyzoites has been demonstrated in dogs for the first time, just like that in humans, sheep, or harbor seals (Abi Abdallah et al., 2012; Reichel et al., 2015; Yildiz et al., 2017). It was clear that T. gondii tachyzoites were captured by NETs-like structures (Figure 1), and these structures consisted of DNA decorated with NE and MPO (Figure 2), which confirmed that similar and typical features of NETs-like structures were observed in other parasites such as: Neospora caninum (Wei et al., 2016), Eimeria bovis (Munoz-Caro et al., 2015a), Cryptosporidium parvum (Munoz-Caro et al., 2015b), and Eimeria arloingi (Silva et al., 2014). Quantitation analysis demonstrated T. gondii tachyzoites triggered NETs release was in a dose-dependent manner. These results have been suggested that one part of T. gondii tachyzoites would be captured and then killed by NETs in dogs. A further quantitative experiment also revealed that T. gondii tachyzoites-triggered NETs release was in a time-independent manner, and it was of great interest that the increase in NETs release was significantly decreased at the time point of 120 min. In addition, the release of NETs triggered T. gondii tachyzoites was also degraded by DNase I (Figure 4A). All these results suggest that another part of T. gondii tachyzoites could also evade capture by these NETs after 90 min.
Given that ROS, NADPH oxidase, Rac, or p38 signaling pathways are involved in the mechanism of several pathogen-induced NETs releases (Lim et al., 2011; Munoz-Caro et al., 2015a,b; Wei et al., 2016, 2018a; Gavillet et al., 2018), and NLRP3 inflammasomes can be activated during T. gondii infection (Gorfu et al., 2014; Quan et al., 2018), we investigated if these molecular or signaling pathways also participate in T. gondii tachyzoites-triggered NETs formation. It was found that inhibitors of the NADPH oxidase, Rac 1, or p38 MAPK signaling pathways but not the inhibitor of NLRP3 significantly inhibited NETs release (Figures 5A,B), which suggested that NADPH oxidase, Rac 1, and p38 MAPK signaling pathways were relevant to T. gondii tachyzoites-triggered NETs. Further results showed that T. gondii tachyzoites significantly increased ROS levels but not LDH levels in the process of NETs release, proving that ROS was also related to T. gondii tachyzoites-triggered NETs. Zymosan used as the positive stimuli could also significantly induce ROS production in NETs release as shown in previous research (Wei et al., 2016, 2018a,b), but lower than that of T. gondii tachyzoites. We deduced that there are possibly two main reasons for the results. The first one is that the type of stimuli are different for inducing NETs release. NETs release is a ROS -dependent or -independent process, these two ways may both exist in NETs release. The other reason is that the amount of the same stimuli significantly affects ROS production in the process of NETs release. Taken together, the mechanism of T. gondii tachyzoites-triggered NETs release was a ROS-, NADPH oxidase-, Rac 1-, or p38 MAPK- signaling pathway dependent process. In this aspect, no significant species-difference of T. gondii tachyzoites-triggered NETs were found between harbor seals and dogs.
On the basis of the quantitative results that NETs release triggered by T. gondii tachyzoites was markedly decreased at the time point of 120 min, we speculated that T. gondii tachyzoites could degrade NETs structures and escape from the NETs-based capture strategy. To provide further evidence, PMNs were co-treated with zymosan and T. gondii tachyzoites for fluorescence confocal microscopy analysis. Interestingly, zymosan-triggered NETs release was strikingly degraded by T. gondii tachyzoites treatment that confirmed our suspicions. In other words, there must be one or more DNase-like enzyme existing in T. gondii to degrade NETs structures. However, predicting and identifying these DNase-like enzymes in T. gondii still needs further research, which will help understand the biological functions and mechanisms of T. gondii escape from the NETs-based capture strategy.
In conclusion, the NETs-based effector mechanism worked in dogs during T. gondii infection, and the regulatory mechanism undergoing T. gondii-triggered NETs release was associated with ROS-, NADPH oxidase-, Rac 1-, or p38 MAPK- signaling pathways. Moreover, it was found that T. gondii escaped from the NETs-based capture strategy, but the escape mechanism still needs careful study.
Data Availability Statement
All datasets generated for this study are included in the article/supplementary material.
Ethics Statement
The animal study was reviewed and approved by Animal experiments were approved by the Ethics Committee on the Care and Use of Laboratory Animals of Foshan University. Written informed consent was obtained from the owners for the participation of their animals in this study.
Author Contributions
ZY and QL conceived and designed the project and experiments. ZWe, ZWa, XL, CW, ZH, DW, and YZ carried out the experiments. ZWe and XZ performed the statistical analysis. ZWa wrote the manuscript. QL revised the manuscript. All authors reviewed the manuscript.
Funding
This work was partly supported by the National Key R&D Program of China (2017YFD0501304), the National Natural Science Foundation of China (31672542) and the Pearl River Talent Recruitment Program in Guangdong Province of China (2019CX01N111). The funders had no role in study design, data collection and analysis, decision to publish, or preparation of the manuscript.
Conflict of Interest
The authors declare that the research was conducted in the absence of any commercial or financial relationships that could be construed as a potential conflict of interest.
Acknowledgments
We acknowledge the usage of the electron microscope of the dedicated platform of the Zoonosis Institute of Jilin University.
Abbreviations
NET, neutrophils extracellular trap; SEM, scanning electron microscopy; PMN, polymorphonuclear neutrophil; NE, neutrophil elastase; MPO, myeloperoxidase; ROS, reactive oxygen species; MAPK, mitogen-activated protein kinase; LDH, lactate dehydrogenase; SOCE, store-operated calcium entry; DPI, Diphenyleneiodonium chloride.
References
Abi Abdallah, D. S., Lin, C., Ball, C. J., King, M. R., Duhamel, G. E., and Denkers, E. Y. (2012). Toxoplasma gondii triggers release of human and mouse neutrophil extracellular traps. Infect. Immun. 80, 768–777. doi: 10.1128/IAI.05730-11
Brinkmann, V., Reichard, U., Goosmann, C., Fauler, B., Uhlemann, Y., Weiss, D. S., et al. (2004). Neutrophil extracellular traps kill bacteria. Science 303, 1532–1535. doi: 10.1126/science.1092385
Dubey, J. P., and Dubey, J. P. (2010). Toxoplasmosis of Animals and Humans. Boca Raton, FL: CRC Press.
Dubey, J. P., and Jones, J. L. (2008). Toxoplasma gondii infection in humans and animals in the United States. Int. J. Parasitol. 38, 1257–1278. doi: 10.1016/j.ijpara.2008.03.007
Dubey, J. P., Verma, S. K., Villena, I., Aubert, D., Geers, R., Su, C., et al. (2016). Toxoplasmosis in the Caribbean islands: literature review, seroprevalence in pregnant women in ten countries, isolation of viable Toxoplasma gondii from dogs from St. Kitts, West Indies with report of new T. gondii genetic types. Parasitol. Res. 115, 1627–1634. doi: 10.1007/s00436-015-4900-6
Gavillet, M., Martinod, K., Renella, R., Wagner, D. D., and Williams, D. A. (2018). A key role for Rac and Pak signaling in neutrophil extracellular traps (NETs) formation defines a new potential therapeutic target. Am. J. Hematol. 93, 269–276. doi: 10.1002/ajh.24970
Gorfu, G., Cirelli, K. M., Melo, M. B., Mayer-Barber, K., Crown, D., Koller, B. H., et al. (2014). Dual role for inflammasome sensors NLRP1 and NLRP3 in murine resistance to Toxoplasma gondii. MBio 5:e01117-13. doi: 10.1128/mBio.01117-13
Imlau, M., Conejeros, I., Munoz-Caro, T., Zhou, E., Gartner, U., Ternes, K., et al. (2020). Dolphin-derived NETosis results in rapid Toxoplasma gondii tachyzoite ensnarement and different phenotypes of NETs. Dev. Comp. Immunol. 103:103527. doi: 10.1016/j.dci.2019.103527
Jiang, H. H., Li, M. W., Xu, M. J., Cong, W., and Zhu, X. Q. (2015). Prevalence of Toxoplasma gondii in dogs in Zhanjiang, Southern China. Korean J. Parasitol. 53, 493–496. doi: 10.3347/kjp.2015.53.4.493
Jin, X., Zhao, Y., Zhang, F., Wan, T., Fan, F., Xie, X., et al. (2016). Neutrophil extracellular traps involvement in corneal fungal infection. Mol. Vis. 22, 944–952.
Lacerda, L. C., dos Santos, J. L., Wardini, A. B., da Silva, A. N., Santos, A. G., Freire, H. P. S., et al. (2019). Toxoplasma gondii induces extracellular traps release in cat neutrophils. Exp. Parasitol. 207:107770. doi: 10.1016/j.exppara.2019.107770
Lim, M. B., Kuiper, J. W., Katchky, A., Goldberg, H., and Glogauer, M. (2011). Rac2 is required for the formation of neutrophil extracellular traps. J. Leukoc. Biol. 90, 771–776. doi: 10.1189/jlb.1010549
Lopes, A. P., Dubey, J. P., Darde, M. L., and Cardoso, L. (2014). Epidemiological review of Toxoplasma gondii infection in humans and animals in Portugal. Parasitology 141, 1699–1708. doi: 10.1017/S0031182014001413
Munoz-Caro, T., Lendner, M., Daugschies, A., Hermosilla, C., and Taubert, A. (2015b). NADPH oxidase, MPO, NE, ERK1/2, p38 MAPK and Ca2+ influx are essential for Cryptosporidium parvum-induced NET formation. Dev. Comp. Immunol. 52, 245–254. doi: 10.1016/j.dci.2015.05.007
Munoz-Caro, T., Mena Huertas, S. J., Conejeros, I., Alarcon, P., Hidalgo, M. A., Burgos, R. A., et al. (2015a). Eimeria bovis-triggered neutrophil extracellular trap formation is CD11b-, ERK 1/2-, p38 MAP kinase- and SOCE-dependent. Vet. Res. 46:23. doi: 10.1186/s13567-015-0155-6
Pan, M., Lyu, C., Zhao, J., and Shen, B. (2017). Sixty years (1957-2017) of research on Toxoplasmosis in China-an overview. Front. Microbiol. 8:1825. doi: 10.3389/fmicb.2017.01825
Quan, J. H., Huang, R., Wang, Z., Huang, S., Choi, I. W., Zhou, Y., et al. (2018). P2X7 receptor mediates NLRP3-dependent IL-1beta secretion and parasite proliferation in Toxoplasma gondii-infected human small intestinal epithelial cells. Parasit. Vectors 11:1. doi: 10.1186/s13071-017-2573-y
Reichel, M., Munoz-Caro, T., Sanchez Contreras, G., Rubio Garcia, A., Magdowski, G., Gartner, U., et al. (2015). Harbour seal (Phoca vitulina) PMN and monocytes release extracellular traps to capture the apicomplexan parasite Toxoplasma gondii. Dev. Comp. Immunol. 50, 106–115. doi: 10.1016/j.dci.2015.02.002
Rengifo-Herrera, C., Pile, E., Garcia, A., Perez, A., Perez, D., Nguyen, F. K., et al. (2017). Seroprevalence of Toxoplasma gondii in domestic pets from metropolitan regions of Panama. Parasite 24:9. doi: 10.1051/parasite/2017009
Saitoh, T., Komano, J., Saitoh, Y., Misawa, T., Takahama, M., Kozaki, T., et al. (2012). Neutrophil extracellular traps mediate a host defense response to human immunodeficiency virus-1. Cell Host Microbe 12, 109–116. doi: 10.1016/j.chom.2012.05.015
Silva, L. M., Caro, T. M., Gerstberger, R., Vila-Vicosa, M. J., Cortes, H. C., Hermosilla, C., et al. (2014). The apicomplexan parasite Eimeria arloingi induces caprine neutrophil extracellular traps. Parasitol. Res. 113, 2797–2807. doi: 10.1007/s00436-014-3939-0
Tenter, A. M., Heckeroth, A. R., and Weiss, L. M. (2000). Toxoplasma gondii: from animals to humans. Int. J. Parasitol. 30, 1217–1258. doi: 10.1016/S0020-7519(00)00124-7
Urban, C. F., and Nett, J. E. (2018). Neutrophil extracellular traps in fungal infection. Semin. Cell Dev. Biol. 89, 47–57. doi: 10.1016/j.semcdb.2018.03.020
Wardini, A. B., Guimaraes-Costa, A. B., Nascimento, M. T., Nadaes, N. R., Danelli, M. G., Mazur, C., et al. (2010). Characterization of neutrophil extracellular traps in cats naturally infected with feline leukemia virus. J. Gen. Virol. 91(Pt. 1), 259–264. doi: 10.1099/vir.0.014613-0
Wei, Z., Hermosilla, C., Taubert, A., He, X., Wang, X., Gong, P., et al. (2016). Canine neutrophil extracellular traps release induced by the apicomplexan parasite Neospora caninum in vitro. Front. Immunol. 7:436. doi: 10.3389/fimmu.2016.00436
Wei, Z., Wang, Y., Zhang, X., Wang, X., Gong, P., Li, J., et al. (2018a). Bovine macrophage-derived extracellular traps act as early effectors against the abortive parasite Neospora caninum. Vet. Parasitol. 258, 1–7. doi: 10.1016/j.vetpar.2018.06.002
Wei, Z., Zhang, X., Wang, J., Wang, Y., Yang, Z., and Fu, Y. (2018b). The formation of canine neutrophil extracellular traps induced by sodium arsenic in polymorphonuclear neutrophils. Chemosphere 196, 297–302. doi: 10.1016/j.chemosphere.2017.12.175
Yildiz, K., Gokpinar, S., Gazyagci, A. N., Babur, C., Sursal, N., and Azkur, A. K. (2017). Role of NETs in the difference in host susceptibility to Toxoplasma gondii between sheep and cattle. Vet. Immunol. Immunopathol. 189, 1–10. doi: 10.1016/j.vetimm.2017.05.005
Zarra-Nezhad, F., Borujeni, M. P., Mosallanejad, B., and Hamidinejat, H. (2017). A seroepidemiological survey of Toxoplasma gondii infection in referred dogs to Veterinary Hospital of Ahvaz, Iran. Int. J. Vet. Sci. Med. 5, 148–151. doi: 10.1016/j.ijvsm.2017.08.006
Keywords: Toxoplasma gondii, neutrophils extracellular traps, dogs, Rac1, ROS
Citation: Wei Z, Wang Z, Liu X, Wang C, Han Z, Wu D, Zhang Y, Zhang X, Yang Z and Liu Q (2020) Toxoplasma gondii Triggers Neutrophil Extracellular Traps Release in Dogs. Front. Cell. Infect. Microbiol. 10:429. doi: 10.3389/fcimb.2020.00429
Received: 14 May 2020; Accepted: 14 July 2020;
Published: 25 September 2020.
Edited by:
Tiago W. P. Mineo, Federal University of Uberlandia, BrazilReviewed by:
Joao Luis Garcia, State University of Londrina, BrazilMusa Hassan, University of Edinburgh, United Kingdom
Copyright © 2020 Wei, Wang, Liu, Wang, Han, Wu, Zhang, Zhang, Yang and Liu. This is an open-access article distributed under the terms of the Creative Commons Attribution License (CC BY). The use, distribution or reproduction in other forums is permitted, provided the original author(s) and the copyright owner(s) are credited and that the original publication in this journal is cited, in accordance with accepted academic practice. No use, distribution or reproduction is permitted which does not comply with these terms.
*Correspondence: Quan Liu, bGl1cXVhbjE5NzMmI3gwMDA0MDtob3RtYWlsLmNvbQ==; Zhengtao Yang, eWFuZ3poZW5ndGFvMDEmI3gwMDA0MDtzaW5hLmNvbQ==