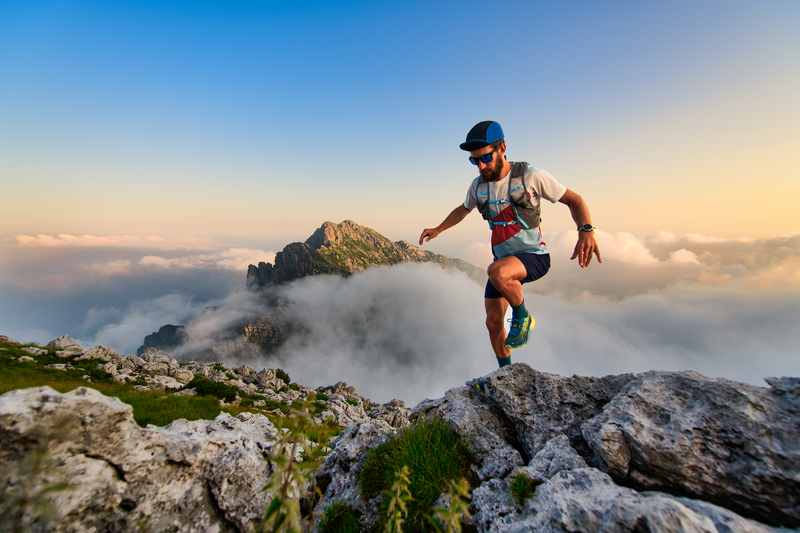
95% of researchers rate our articles as excellent or good
Learn more about the work of our research integrity team to safeguard the quality of each article we publish.
Find out more
ORIGINAL RESEARCH article
Front. Cell. Infect. Microbiol. , 24 January 2020
Sec. Parasite and Host
Volume 9 - 2019 | https://doi.org/10.3389/fcimb.2019.00479
This article is part of the Research Topic Immunobiology of Leishmaniasis View all 25 articles
Leishmaniasis is a vector-borne disease caused by Leishmania parasites. Macrophages are considered the primary parasite host cell, but dendritic cells (DCs) play a critical role in initiating adaptive immunity and controlling Leishmania infection. Accordingly, our previous study in CD11ccreIL-4Rα−/lox mice, which have impaired IL-4 receptor alpha (IL-4Rα) expression on CD11c+ cells including DCs, confirmed a protective role for IL-4/IL-13-responsive DCs in replication and dissemination of parasites during cutaneous leishmaniasis. However, it was unclear which DC subset/s was executing this function. To investigate this, we infected CD11ccreIL-4Rα−/lox and control mice with L. major GFP+ parasites and identified subsets of infected DCs by flow cytometry. Three days after infection, CD11b+ DCs and CD103+ DCs were the main infected DC subsets in the footpad and draining lymph node, respectively and by 4 weeks post-infection, Ly6C+ and Ly6C− CD11b+ DCs were the main infected DC populations in both the lymph nodes and footpads. Interestingly, Ly6C+CD11b+ inflammatory monocyte-derived DCs but not Ly6C−CD11b+ DCs hosted parasites in the spleen. Importantly, intracellular parasitism was significantly higher in IL-4Rα-deficient DCs. In terms of DC effector function, we found no change in the expression of pattern-recognition receptors (TLR4 and TLR9) nor in expression of the co-stimulatory marker, CD80, but MHCII expression was lower in CD11ccreIL-4Rα−/lox mice at later time-points compared to the controls. Interestingly, in CD11ccreIL-4Rα−/lox mice, which have reduced Th1 responses, CD11b+ DCs had impaired iNOS production, suggesting that DC IL-4Rα expression and NO production is important for controlling parasite numbers and preventing dissemination. Expression of the alternative activation marker arginase was unchanged in CD11b+ DCs in CD11creIL-4Rα−/lox mice compared to littermate controls, but RELM-α was upregulated, suggesting IL-4Rα-independent alternative activation. In summary, L. major parasites may use Ly6C+CD11b+ inflammatory DCs derived from monocytes recruited to infection as “Trojan horses” to migrate to secondary lymphoid organs and peripheral sites, and DC IL-4Rα expression is important for controlling infection.
Leishmaniasis is a vector-borne parasitic infection caused by Leishmania species, obligate intracellular protozoans that are transmitted by the bite of infected female Phlebotominae sandflies. There are over 20 Leishmania species, and over 90 sandfly species known to transmit the parasites (Burza et al., 2018; WHO, 2019). According to the World Health Organization (WHO), ~700,000-1 million new cases and 26,000-65,000 deaths occur annually (WHO, 2019). Cutaneous leishmaniasis is the most common form of the disease, causing disfiguring, often ulcerative skin lesions. Mucocutaneous leishmaniasis leads to destruction of the mucous membranes of the nose, mouth, and throat, while visceral leishmaniasis involves dissemination of the parasites to organs, such as the spleen, liver, and bone-marrow, and is usually fatal if left untreated (Burza et al., 2018). While vector control remains an important component in controlling disease transmission, other efforts have focused on the design of novel drugs or vaccines against Leishmania species (Handman, 2001).
Leishmania parasites have two morphological stages: a flagellated promastigote form that is found in the salivary glands of the insect vector and a non-motile amastigote form that is found intracellularly in the vertebrate host (Gutiérrez-Kobeh et al., 2018). Experimental infections in mouse models have shown that promastigotes infect macrophages and neutrophils that are present at the site of inoculation (Sunderkotter et al., 1993; Laskay et al., 2003; Hurdayal et al., 2013; Gutiérrez-Kobeh et al., 2018). The primary host cell for Leishmania species is considered the macrophage, wherein the parasites differentiate into amastigotes and divide within parasitophorous vacuoles (Lievin-Le Moal and Loiseau, 2016). The release of new amastigotes causes the infection to spread. Parasite killing is dependent on IFN-gamma (IFN-γ)-mediated classical activation of macrophages to induce killing effector molecules, such as nitric oxide (NO) (Liew et al., 1990; Stenger et al., 1994; Diefenbach et al., 1999; Holscher et al., 2006). Immunity to leishmaniasis therefore depends on the production of IL-12, which drives T helper 1 (Th1) responses and the production of IFN-γ. However, infection with Leishmania downregulates the capacity of macrophages to produce IL-12 (Belkaid et al., 1998). Dendritic cells (DCs), on the other hand, produce IL-12 upon taking up amastigote parasites (Woelbing et al., 2006). At the same time, they mature, upregulate MHCII and co-stimulatory molecules, and travel to the lymph nodes (LN), where they prime naïve T cells to differentiate into Th1 cells, producing IFN-γ. DCs therefore play a critical role in initiating adaptive immunity and controlling Leishmania infection.
Interestingly, optimal induction of Th1 responses by DCs requires the Th2 cytokine IL-4, which paradoxically promotes IL-12 production by dendritic cells via inhibition of IL-10 (Biedermann et al., 2001; Lutz et al., 2002; Yao et al., 2005; Hurdayal et al., 2013). In a previous study, we found that CD11ccreIL-4Rα−/lox mice, in which DCs lack IL-4Rα and are thus impaired in IL-4/IL-13 signaling, were hypersusceptible to cutaneous L. major infection in comparison to littermate control mice (Hurdayal et al., 2013). This mouse strain showed increased footpad swelling and necrosis, increased Th2 responses as well as substantially increased parasite burdens in LN, spleens and peripheral organs, such as the liver and even the brain. Importantly, we also found that DCs themselves harbored parasites, and that iNOS production was impaired in IL-4Rα deficient CD11chiMHCIIhi DCs. The observation of infected DCs at peripheral sites suggested that DCs may play a role in disseminating L. major, and their effector responses could be important in controlling disease.
However, dendritic cells are recognized as a complex array of heterogeneous cell populations, and classified into different subsets by their surface markers, effector functions and ontogeny (Steinman and Inaba, 1999; Scott and Hunter, 2002; Zhou and Wu, 2017; Gutiérrez-Kobeh et al., 2018). Thus, we aimed to determine which subset of DCs is responsible for hosting and disseminating L. major parasites. We found that CD11b+Ly6C+ inflammatory DCs were most highly infected DC subset in CD11ccreIL-4Rα−/lox mice, and that these DCs had impaired iNOS production in the absence of IL-4Rα signaling. This suggests that L. major parasites use inflammatory DCs as a “Trojan horse” to migrate to secondary lymphoid organs and peripheral sites, and that IL-4Rα signaling contributes to parasite control.
Generation, characterization and genotyping of CD11ccreIL-4Rα−/lox mice was performed as previously described (Hurdayal et al., 2013). Briefly, Cd11ccre mice were inter-crossed with IL-4Rαlox/lox BALB/c mice and homozygous IL-4Rα−/− BALB/c mice (Mohrs et al., 1999) to generate hemizygous CD11ccreIL-4Rα−/lox mice, backcrossed to a BALB/c background for nine generations to generate CD11ccreIL-4Rα−/lox BALB/c mice. Hemizygous littermates (IL-4Rα−/lox) expressing functional IL-4Rα were used as wildtype controls in all experiments. All mice were housed in specific pathogen-free barrier conditions in individually ventilated cages at the University of Cape Town biosafety level 2 animal facility. Experimental mice were age and sex matched and used between 8 and 12 weeks of age.
This study was performed in strict accordance with the recommendations of the South African national guidelines and University of Cape Town of practice for laboratory animal procedures. All mouse experiments were performed according to protocols approved by the Animal Research Ethics Committee of the Health Sciences Faculty, University of Cape Town (Permit Numbers: 009/042; 015/034). All efforts were made to minimize suffering of the animals.
Green-fluorescent protein (GFP)-labeled L. major IL81 (MHOM/IL/81/FEBNI) (Gonzalez-Leal et al., 2014) strains were maintained by continuous passage in BALB/c mice and prepared for infection as described previously (Hurdayal et al., 2013). Anesthetized mice were inoculated subcutaneously with 2 × 106 stationary phase promastigotes into the left hind footpad in a volume of 50 μl of sterile PBS. Swelling of infected footpads and weights of infected animals was monitored weekly using a Mitutoyo micrometer caliper (Brütsch, Zürich, Switzerland).
Single lymph node cell suspensions were prepared by pressing the draining popliteal lymph nodes through 40 μM cell-strainers. Single cell suspensions of spleen cells were isolated by pressing spleens through 70 μM cell-strainers followed by red blood cell lysis. To isolate a single cell population from the infected footpad, footpads were treated with DMEM medium supplemented with Collagenase IV (Sigma-Aldrich; 1 mg/ml) and DNase I (Sigma-Aldrich; 1 mg/ml) at 37°C for 60 min to digest muscle and collagen. Following incubation, single cell footpad suspensions were isolated by straining through 40 μM cell-strainers. All cell suspensions of lymph node, spleen and footpad were resuspended in complete DMEM (Gibco) supplemented with 10% FCS (Gibco) and penicillin and streptomycin (100 U/ml and 100 μg/ml, Gibco) and enumerated using trypan blue exclusion (Hurdayal et al., 2013).
Antibodies against the following extracellular markers were used for flow cytometry: CD11c, CD11b, MHCII, F4/80, CD3, CD4, CD19, CD103, and Ly6C (all BD Bioscience, Erembodegem, Belgium). Footpad, pLN, and spleen cells (1 × 106) were stained with antibody cocktails in PBS containing 1% BSA/1% rat serum for 20 min at 4°C followed by fixing in 2% [w/v] PFA. For intracellular cytokine staining of dendritic cells, popliteal lymph node cells from L. major infected mice were seeded at 2 × 106 cells/well, surface-stained, fixed and permeabilized, and stained intracellularly for iNOS using rabbit anti-mouse iNOS (Abcam) with goat anti-rabbit PE (Abcam) and goat anti-mouse arginase (Santa Cruz Biotechnology) with donkey anti-goat PE (Abcam). Staining specificity was verified by isotype-matched antibody controls and compensation performed with BD compensation beads. Acquisition was performed using BD LSRFortessa (BD Biosciences), and data were analyzed using FlowJo software (Treestar, Ashland, OR, USA).
Footpad and lymph node cells, resuspended in complete DMEM (Gibco) supplemented with 10% FCS (Gibco) and penicillin and streptomycin (100 U/ml and 100 μg/ml, Gibco) were cultured at 1 × 106 cells in 48-well plates together with 50 μg/ml soluble Leishmania antigen (SLA). Cells were incubated at 37°C in a humidified atmosphere containing 5% CO2. Supernatants were collected after 72 h and stored at −80°C for cytokine analysis.
Cytokines (IL-4, IFN-γ, and TNF-α) in supernatants from restimulated footpad or lymph node cells were measured by indirect sandwich ELISA as previously described (Mohrs et al., 1999; Hurdayal et al., 2013).
OCT-embedded lymph node and spleen tissue from mice infected with GFP-L. major IL81 parasites for 4 weeks was cut into 10 μm cryosections. Following acetone fixation, dendritic cells were stained using biotinylated anti-CD11c mAb (BD Biosciences) and visualized by staining with a streptavidin-Cy3 conjugate (Sigma). Nuclei were stained with Hoechst. Coverslips were then mounted on sections using Mowiol®4-88 mounting medium (Calbiochem) with anti-fade (Sigma). Images were acquired by Ziess LSM 510 confocal microscope and images quantified using a MatLab (MathWorks, Natick, Massachusetts) script developed for automated counting. A total of 16 fields were captured for each condition. At week 4 after GFP-L. major IL81 infection, lymph node B cells (CD19+CD3−CD11c−) were isolated by cell sorting on a FACS Vantage cell sorter. Sorted cells were viewed live, directly in suspension in chamber slides for the presence of GFP+ parasites by LSM 510 confocal microscopy.
Data is given as mean ± SEM. Statistical analysis was performed using the unpaired Student's t-test or 1-way Anova with Bonferroni's post-test, defining differences to IL-4Rα−/lox mice as significant (*p ≤ 0.05; **p ≤ 0.01; ***p ≤ 0.001) (Prism software; https://www.graphpad.com/scientific-software/prism/).
Previously, we found increased numbers of DCs containing GFP-expressing L. major parasites in CD11ccreIL-4Rα−/lox mice compared to littermate controls using flow cytometry (Hurdayal et al., 2013). To confirm the presence of intracellular parasites in DC subsets by fluorescent imaging, CD11ccreIL-4Rα−/lox and control mice were infected with GFP-expressing L. major in the hind footpad. As found previously (Hurdayal et al., 2013), by week 4 post-infection, CD11ccreIL-4Rα−/lox mice presented with fulminant cutaneous leishmaniasis, and were hypersusceptible compared to littermate control mice (Supplementary Figure S1). Representative confocal images of the pLN (Figure 1A) and spleen (Figure 1B) demonstrate the intracellular amastigote nature of most of the GFP+ parasites in CD11c+ cells, indicating that these cell populations were harboring whole parasites and not fragments of GFP. Automated quantification of intracellular GFP+ L. major amastigote parasites in the cryo-fixed tissue sections using MatLab software revealed significantly increased numbers of parasites per cell in CD11c+ cells of CD11ccreIL-4Rα−/lox mice compared to littermate control mice in both the lymph node and spleen (Figure 1C) in accordance with previous flow cytometry data (Hurdayal et al., 2013). In contrast, confocal imaging of FACS-sorted B cells demonstrated GFP+ parasites adhering to the outside of the cells and not intracellularly (Figure 1D) confirming that lymphocyte populations do not support replication of intracellular Leishmania. This illustrates the importance of confirming that GFP+ cells actually harbor intracellular pathogens. Altogether, this strengthens and confirms previous data, in which CD11c-expressing cells served as a reservoir for pathogen replication (Heyde et al., 2018), and showed increased infection in the absence of IL-4-signaling via the IL-4Rα chain (Hurdayal et al., 2013).
Figure 1. Intracellular nature of GFP+ L. major parasites in dendritic cells of lymph nodes and spleen. Mice were infected subcutaneously with 2 × 105 stationary phase GFP-expressing L. major IL81 promastigotes into the hind footpad. After 4 weeks of infection, frozen sections of lymph node and spleen were stained with a mAb against CD11c+ dendritic cells. Representative micrographs of lymph node (A) and spleen cryosections (B) showing intracellular localization of GFP+ L. major-infected (green) CD11c+ dendritic cells (red) from CD11ccreIL-4Rα−/lox and littermate mice (original magnification ×400). Insets show individual channels. The actual number of GFP+ amastigote parasites in dendritic cells from CD11ccreIL-4Rα−/lox and littermate control mice were quantified using MatLab software in 16 fields of multiple lymph node and spleen sections (C) from individual mice by confocal microscopy. At the same time-point, lymph node B cells (CD19+CD3−CD11c−) were isolated on a FACS Vantage cell sorter after staining with specific mAbs (D). Live sorted cells were viewed directly in suspension in chamber slides for the location of GFP+ parasites by LSM 510 confocal microscopy. Data is expressed as mean ± SEM. Statistical analysis was performed defining differences to IL-4Rα−/lox mice (**p ≤ 0.01; ***p ≤ 0.001) as significant.
Since DCs represent a highly heterogeneous cell population comprising various subsets defined by their effector functions and surface markers (Steinman and Inaba, 1999; Scott and Hunter, 2002; Zhou and Wu, 2017), we sought to investigate if any particular DC subset was important in harboring and disseminating L. major in infected mice. To determine which subsets harbor L. major after infection, CD11ccreIL-4Rα−/lox and littermate control mice were infected in the footpads as above and infected cell populations were tracked by flow cytometry at early (day 3) and late (week 4) stages of infection. DC subsets were identified by their expression of particular cell-surface markers, focusing on the lymphoid and myeloid DC subsets. In line with their limited phagocytic ability (Pulendran et al., 1997), lymphoid-resident DCs (CD4+CD11chiCD3− and CD8+CD11chiCD3−) DCs were the least infected DC population, both at day 3 and at week 4 post-L. major infection in both CD11ccreIL-4Rα−/lox and littermate control mice. Myeloid-derived DCs are a migratory subset of conventional DCs, known to take up antigen at the infection site and migrate to the draining LN for presentation to antigen-specific T cells, with two major subsets being CD103+ migratory tissue DCs and CD11b+ DCs (Pulendran et al., 1997; Steinman and Inaba, 1999; Martinez-Lopez et al., 2015; Mayer et al., 2017). At day 3 post-L. major infection, CD11b+ DCs (Supplementary Figure S2) were the main subset infected with L. major in the footpads of both CD11ccreIL-4Rα−/lox mice and littermate controls (Figure 2A). However, in the LN of CD11ccreIL-4Rα−/lox mice there was an increased number of GFP+ CD103+ DCs that had most likely carried L. major parasites from the tissue to the LNs (Supplementary Figure S2 and Figure 2B). CD11b+ DCs can be further divided into monocyte-derived/inflammatory DCs or non-monocyte derived, with monocyte-derived DCs (mo-DCs) typically expressing Ly6C and induced as part of the inflammatory response as infection progresses (Leon et al., 2007; Plantinga et al., 2013). Thus, at the later stage of infection (week 4), we included Ly6c as a marker to differentiate monocyte-derived DCs and non-monocyte derived DCs (Supplementary Figure S2). CD11b+ DCs (both Ly6C+ and Ly6C−) were the main infected DC populations in both the footpads and lymph nodes at week 4 post-infection, and numbers of infected CD11b+ DCs were greater in CD11ccreIL-4Rα−/lox mice compared to littermate controls (Figures 2C,D). This indicates that monocyte-derived DCs that were recruited to the footpad also became infected with L. major parasites.
Figure 2. CD11b+ inflammatory dendritic cells in CD11ccreIL-4Rα−/lox mice preferentially harbor L. major parasites during infection. CD11ccreIL-4Rα−/lox and littermate mice were infected subcutaneously with GFP-labeled L. major IL81 promastigotes into the hind footpad. Number of GFP+ L. major parasites was identified within dendritic cell subsets derived from footpad (A,C) and lymph node (B,D) at day 3 and week 4 after infection, respectively. Spleens were harvested to analyse GFP+ L. major parasites in the indicated DC subsets (E) at week 4 after infection. Dendritic cell populations were gated as shown in Supplementary Figure S1 and differentiated based on the following markers; conventional lymphoid-derived dendritic cells; CD4+ DCs (CD11c+CD4+CD3−), CD8+ DCs (CD11c+CD8+CD3−), and myeloid-derived dendritic cells; CD103+ DCs (CD11c+MHCII+CD103+), CD11b+ DCs (CD11c+CD11b+MHCII+), monocyte-derived DCs (CD11c+CD11b+MHCII+Ly6C+), and non-monocyte derived DCs (CD11c+CD11b+MHCII+Ly6C−). Statistical analysis was performed defining differences to IL-4Rα−/lox mice (*, p ≤ 0.05; **, p ≤ 0.01) as significant.
While it is known that parasites migrate from the site of infection to the lesion-draining lymph nodes, we were interested to determine if L. major parasites were using CD11b+ Mo-DCs or CD11b+ cDCs to disseminate to peripheral organs, and if IL-4/IL-13 signaling influenced this process. Therefore, we analyzed infected DC subsets in the spleens of CD11ccreIL-4Rα−/lox and littermate control mice at 4 weeks post-L. major infection. The results showed that by week 4 post-infection, CD11b+ DCs were the main subset containing GFP+ L. major in the spleen (Figure 2E). Ly6C+ CD11b+ DCs consisted of two populations, Ly6C high (Ly6Chi) and Ly6C intermediate (Ly6Cint). Both Ly6Chi and Ly6Cint populations harbored intracellular Leishmania. In contrast, Ly6C− CD11b+ DCs did not appear to be host cells for intracellular parasites. Importantly, the numbers of infected CD11b+ DCs in FPs, LNs and spleens were higher in CD11ccreIL-4Rα−/lox mice, suggesting a requirement for IL-4/IL-13 signaling in controlling dissemination of intracellular Leishmania. Taken together, these data suggest that L. major parasites utilize inflammatory CD11b+Ly6C+ DCs as “Trojan horses” to migrate to secondary lymphoid organs and that IL-4Rα-signaling contributes to parasite control in these cells.
As CD11b+ DCs were the most highly infected DC subset in CD11ccreIL-4Rα−/lox mice at day 3 (footpad) and week 4 post-L. major infection, we checked whether the higher numbers of infected cells could be due to increased numbers of these cells overall, as a consequence of IL-4Rα-deficiency. At day 3 post-infection with GFP-expressing L. major IL81 parasites, similar levels of all DC subsets, including CD11b+ DCs, was found in infected footpad (Figure 3A) and lymph node (Figure 3B). As there was no dissemination to the spleen at Day 3 post-infection (Hurdayal et al., 2013), analysis of DC subsets in the spleen was not performed at this time-point. The same trend in DC numbers was seen at week 4 post-infection, with similar numbers of all DC subsets, including CD11b+ DCs, infiltrating the FP (Figure 3C), LN (Figure 3D), and spleen (Figure 3E) in CD11ccreIL-4Rα−/lox mice and littermate controls. Moreover, analysis of CD11b+ DCs in the spleen of naïve animals and at Day 1 and 3 found no difference in infiltration of CD11b+ DCs between CD11ccreIL-4Rα−/lox mice and littermate controls (Supplementary Figure S3). This suggests that altered effector functions of CD11b+ DCs, and not increased numbers, contributed to increased parasites loads and dissemination in the absence of IL-4Rα signaling.
Figure 3. Frequency of dendritic cells in during L. major IL81 infection in CD11ccreIL-4Rα−/lox mice. CD11ccreIL-4Rα−/lox and littermate mice were infected subcutaneously with GFP-labeled L. major IL81 promastigotes into the hind footpad. At day 3 and week 4 after infection, total footpad (A,C), lymph node (B,D), and spleen (E) cells were surface stained for total frequency of dendritic cells subsets. Cell populations were differentiated based on the following markers; conventional lymphoid-derived dendritic cells; CD4+ DCs (CD11c+CD4+CD3−), CD8+ DCs (CD11c+CD8+CD3−), and myeloid-derived dendritic cells; CD103+ DCs (CD11c+MHCII+CD103+), CD11b+ DCs (CD11c+CD11b+MHCII+), monocyte-derived DCs (CD11c+CD11b+MHCII+Ly6C+), and non-monocyte derived DCs (CD11c+CD11b+MHCII+Ly6C−).
To investigate this further, we analyzed the effector functions of CD11b+ DCs at early and late stages after L. major infection in CD11ccreIL-4Rα−/lox and control mice. DC activation, differentiation into subsets and subsequent parasite control has been linked to antigen recognition via pattern-recognition receptors (PRRs), specifically the Toll-like receptors (TLRs) (Faria et al., 2012). Since TLR4 and TLR9 are reportedly required for mounting an effective Th1 response (Faria et al., 2012) and hypersusceptible CD11ccreIL-4Rα−/lox mice showed a shift toward Th2 responses in L. major infection (Hurdayal et al., 2013), we evaluated expression of these receptors on CD11b+ DCs by gating CD11c+CD11b+MHCII+ populations and analyzing mean fluorescence intensity of histograms of expression levels (Supplementary Figure S2 and Figure 4). At Day 3 post-infection in the footpad, CD11b+ DCs from CD11ccreIL-4Rα−/lox and littermate control mice expressed equivalent levels of TLR4 and TLR9 (Figure 4A). A similar trend was observed at week 4 post-infection in the footpad (Figure 4B). Once DCs are activated by pathogen products, they mature to express higher levels of MHCII and costimulatory molecules, such as CD80 and CD86, and can present antigen to prime naïve T cells (Pulendran et al., 1997), therefore we measured MHCII and CD80 expression on CD11b+ DCs in the lesion-draining lymph node. We and others have reported previously that lack of IL-4Rα signaling on DCs does not intrinsically alter expression of MHCII or co-stimulatory molecules, such as CD80, CD86, and CD40 in vivo (Cook et al., 2012; Hurdayal et al., 2013). At day 3 post-infection, CD11b+ DCs from CD11ccreIL-4Rα−/lox and littermate control mice showed equivalent expression of MHCII and CD80 (Figure 4C), demonstrating that early activation of DCs was unaltered in the absence of IL-4Rα expression. At later time-points (week 4 post-infection), MHCII expression was lower in the absence of IL-4Rα on DCs (Figures 4D,E) whilst CD80 expression was similar between CD11ccreIL-4Rα−/lox and littermate control mice (Figure 4D). Nevertheless, lower levels of MHCII expression did not correspond to differences in ability of DCs to prime differentiation of naïve Th cells into effector/memory T cells, since similar percentages and numbers of CD4+ T cells (gated FSClowSSClowCD3+CD4+ as described in Hurdayal et al., 2017) were CD44+ in the LN of CD11ccreIL-4Rα−/lox mice and littermate controls (Figures 5A,B). Similarly, we found similar levels of CD4+CD44+ T cells in the spleens of CD11ccreIL-4Rα−/lox and littermate control mice (Figures 5C,D). Taken together, these data suggest that IL-4Rα might play a role in regulation of DC maturation at the later stages of infection, but is not required for T cell activation in the lesion-draining lymph node.
Figure 4. Phenotype of CD11b+ DCs during L. major infection in CD11ccreIL-4Rα−/lox mice and controls. CD11ccreIL-4Rα−/lox and littermate mice were infected subcutaneously with GFP-labeled L. major IL81 promastigotes into the hind footpad. At week 4 after infection, total footpad cells were stained for levels of pattern-recognition receptors, TLR4 and TLR9 on CD11b+ DCs at day 3 (A) and week 4 (B) after infection. Similarly, activation markers (MHCII and CD80) on CD11c+MHCII+CD11b+ DCs in the footpad were analyzed at day 3 (C) and week 4 (D) after infection. Histogram plots of MHCII expression at week 4 after infection (E). Statistical analysis was performed defining differences to IL-4Rα−/lox mice (*, p ≤ 0.05) as significant.
Figure 5. T cell activation is unchanged in lymph node and spleen of CD11ccreIL-4Rα−/lox mice and controls. CD11ccreIL-4Rα−/lox and littermate mice were infected subcutaneously with GFP-labeled L. major IL81 promastigotes into the hind footpad. At week 4 after infection, total cells were stained for the frequency (A,C) and absolute number (B, D) of effector T helper cells (CD3+CD4+CD44+) in the lesion-draining popliteal lymph node (A,B) and spleen (C,D).
Once phagocytosed, Leishmania parasites are eliminated by iNOS-induced nitric oxide production, which occurs predominantly in classically-activated macrophages in response to IFN-γ. In contrast, IL-4/IL-13 signaling via the IL-4Rα drives alternative activation of macrophages and arginase production, and this promotes parasite survival (Hurdayal and Brombacher, 2017). We measured cytokine production by cells from FPs of L. major infected mice restimulated with SLA, and found that IFN-γ production was significantly reduced in CD11ccreIL-4Rα−/lox mice compared to littermate controls, whilst IL-4 production was dramatically increased, demonstrating a switch toward Th2-type responses at the site of infection (Figures 6A,B). Given the importance of inflammatory DCs in our model, we looked at production of the pro-inflammatory cytokine TNF-α by restimulated LN cells. Production of TNF-α was significantly higher in CD11ccreIL-4Rα−/lox mice compared to those of littermate controls (Figure 6C). It has been shown that DCs may also be alternatively activated in schistosomiasis (Cook et al., 2012), and previously we found decreased iNOS in conventional DCs in spleens of L. major infected mice (Hurdayal et al., 2013). Therefore, we aimed to determine how IL-4Rα signaling affects the killing-effector phenotype of CD11b+ DCs in L. major infection. Intracellular staining of CD11b+ DCs in infected FPs demonstrated that intracellular iNOS expression was significantly lower in CD11b+ DCs of CD11ccreIL-4Rα−/lox compared to those of littermate controls (Figure 6D). However, expression of the alternative activation marker arginase was unchanged in CD11b+ DCs in CD11ccreIL-4Rα−/lox mice compared to littermate control mice (Figure 6E). Interestingly, RELMα, another marker associated with alternative activation of macrophages (Gordon, 2003) and DCs (Cook et al., 2012), was upregulated in CD11ccreIL-4Rα−/lox mice (Figure 6F), suggesting IL-4/IL-4Rα-independent expression of RELMα on CD11b+ DCs.
Figure 6. IL-4Rα regulates the activation of CD11b+ DCs during L. major infection in CD11ccreIL-4Rα−/lox mice and controls. CD11ccreIL-4Rα−/lox and littermate mice were infected subcutaneously with GFP-labeled L. major IL81 promastigotes into the hind footpad. At week 4 after infection, total footpad cells were re-stimulated with soluble Leishmania antigen (SLA) for 72 h and levels of IFN-γ (A) and IL-4 (B) was determined in cell supernatants by ELISA. TNF-α (C) was determined in draining lymph-node cells by ELISA following SLA stimulation. To analyse classical and alternate activation of DCs, CD11b+ DCs of total footpad cells were stained for intracellular levels of inducible nitric oxide synthase (iNOS) (D), arginase (E), and resistin-like molecule alpha-RELM-α (F) at week 4 after L. major infection. Statistical analysis was performed defining differences to IL-4Rα−/lox mice (*, p ≤ 0.05; **, p ≤ 0.01) as significant.
Macrophages are considered the principal host cells of Leishmania parasites, and are key players in the parasite cycle of replication, release and entry into new host cells (Martinez-Lopez et al., 2018). However, dendritic cells can also be infected and appear to play an important role in disease progression (De Trez et al., 2009; Hurdayal et al., 2013). Dendritic cell-derived IL-12 is essential for the polarization of naïve T cells toward a Th1 subset and subsequent production of IFN-γ to control infection (Biedermann et al., 2001). In contrast, DC-derived IL-10 has been shown to dampen this process, leading to an environment that favors parasite replication and polarization of naïve T cells toward a Th2 subset (Yao et al., 2005). We previously demonstrated in vivo that IL-4 can instruct DCs to promote Th1 differentiation and effector iNOS production for killing of intracellular L. major, by using the cre/loxP recombination system to generate BALB/c mice deficient in the IL-4Rα gene under control of the cd11c locus (Hurdayal et al., 2013). In addition, we discovered that DCs function as important reservoirs for intracellular parasite replication and dissemination from the site of infection in the footpad.
DCs comprise a highly heterogeneous cell population consisting of various subsets with different characteristics (myeloid, lymphoid, migratory, plasmacytoid, and inflammatory) (Steinman and Inaba, 1999; Scott and Hunter, 2002; Zhou and Wu, 2017). They can be divided into three main groups: conventional DCs (cDCs), plasmacytoid DCs (pDCs), and monocyte-derived DCs (mo-DCs). DC-Leishmania interactions can vary depending on the different DC subsets involved, as they are equipped with different effector functions in terms of pathogen recognition, signal transduction and cytokine release. DCs are important for parasite uptake (via Toll-like receptors), processing, presentation, and subsequent activation of naïve T cells in adaptive immunity. They not only engulf apoptotic neutrophils harboring intracellular parasites but also engulf free extracellular promastigotes (Martinez-Lopez et al., 2018). It has also been shown that they can function as effector cells in killing of intracellular pathogens by expression of iNOS (De Trez et al., 2009; Hurdayal et al., 2013). Moreover, cytokine signaling has been shown to influence the effector function of DCs (Marovich et al., 2000; Girard-Madoux et al., 2015; Martinez-Lopez et al., 2015). After finding that mice with impaired IL-4Rα signaling on CD11c+ cells (CD11ccreIL-4Rα−/lox mice) were susceptible to L. major and showed increased numbers of infected DCs (Hurdayal et al., 2013), we wanted to determine which DC subset, regulated by IL-4, might be important for intracellular multiplication and the spread of L. major parasites during infection, and which effector functions were involved in the phenotype. To address this, we used GFP-labeled parasites to track infected DC populations and flow cytometry to identify subsets of dendritic cells infiltrating the footpad, LN and spleen during the early (Day 3) and late stages (week 4) of L. major infection.
During early stages of infection in the skin, recruited monocytes are believed to differentiate into effector cells for uptake, processing and transport of antigen to the draining LN. It is therefore not surprising that migratory CD103+ DCs, which are derived from monocytes (Jakubzick et al., 2008; Del Rio et al., 2010), were the main source of infected cells in the draining popliteal LN at the early stage (day 3) of infection. CD103+ DCs are reported to be the main source of IL-12 upon infection with L. major parasites, important in inducing local Th1 immunity (Martinez-Lopez et al., 2015). Interestingly, the absence of IL-4/IL-13 signaling in these cells increased the percentage of cells that contained parasites, while the number of CD103+ DCs remained similar, suggesting that IL-4/IL-13 signaling to CD103+ migratory DCs could represent an early control mechanism for establishment of infection in the LN. A previous study also reported that DCs were the primary infected cell population in the draining LN of L. major infected mice, but did not examine DC subsets (Muraille et al., 2003). Our study expands upon these findings by demonstrating that CD103+ tissue DCs may be responsible for trafficking parasites to the draining LN at the onset of infection. This concept is supported by a study involving influenza virus infection in the lung, which demonstrated that CD103+ DCs were the cells that carried intact viral protein to the draining LNs, and that this occurred as early as 12 h after inoculation, peaking at 48 h after infection (Helft et al., 2012). Previously we showed that pDCs also harbor parasites at the site of infection early (day 3) after parasite inoculation (Hurdayal et al., 2013).
During the later stage of infection (week 4), we found that CD11b+ DCs, consisting of both monocyte-derived inflammatory DCs (Ly6C+) (Leon et al., 2007) and conventional DCs (Ly6C), were the main infected cells in both the FP and LN of BALB/c mice infected with L. major. Similar to what was seen with the CD103+ DCs, the frequency of parasite-infected CD11b+ DCs increased strikingly in the absence of IL-4-responsivness, suggesting that IL-4Rα-signaling on DCs contributes to intracellular parasitism of DCs themselves. In fact, all DCs subsets analyzed had increased parasite loads in the absence of IL-4Rα on DCs, while numbers of DCs infiltrating tissues were similar between CD11ccreIL-4Rα−/lox mice and littermate controls. This suggests that differences in DC effector functions were responsible for the increased parasite replication and dissemination seen during infection. Our analysis demonstrated that hypersusceptibility, in the absence of IL-4-responsive DCs during L. major infection was not a consequence of altered expression of pattern recognition receptors (TLR4, TLR9) or expression of the co-stimulatory marker CD80. DC-derived MHCII levels were similar in CD11ccreIL-4Rα−/lox mice and littermate controls at day 3, but at week 4 they were decreased in CD11ccreIL-4Rα−/lox mice, although activation of Th cells as measured by CD44 expression was not affected. At week 4 in the spleen, L. major parasites were found primarily in Ly6C+CD11b+ DCs whereas Ly6C−CD11b+ DCs were minimally infected. This suggests that L. major uses Ly6C+ monocyte-derived inflammatory CD11b+ DCs to disseminate from primary LN organs during infection. This is in line with a previous study during Mycobacterium tuberculosis infection, in which activated inflammatory DCs (CD11c+CD11b+Ly6C+ DCs) showed a unique tendency to disseminate to systemic sites (Schreiber et al., 2011). In combination with the impaired ability to kill parasites because of reduced iNOS expression in these DCs, this would explain how CD11ccreIL-4Rα−/lox mice were more susceptible to dissemination of L. major.
The term “classical activation” is characteristically employed for IFN-dependent activation of macrophages and secretion of nitric oxide to kill intracellular pathogens, such as L. major (Gordon, 2003; Gordon and Pluddemann, 2017). However, the combined evidence of several studies demonstrates that DCs can also express iNOS in order to control intracellular parasites (Serbina et al., 2003; De Trez et al., 2009; Hurdayal et al., 2013). We demonstrated previously that iNOS expression was decreased in CD11c+ DCs from CD11ccreIL-4Rα−/lox mice compared to littermate controls, most likely as a consequence of decreased Th1 responses due to lack of IL-4 instruction of DCs (Biedermann et al., 2001; Hurdayal et al., 2013). Now we have expanded on this, showing that IL-4Rα-deficient CD11b+ DCs secrete decreased iNOS, which could play a significant role in parasite replication and indicates impaired classical activation. iNOS and arginase share the substrate L-arginine, and in macrophages, alternative activation of macrophages via IL-4Rα signaling leads to upregulation of arginase-1, which can promote parasite growth by arginase-dependent synthesis of polyamines (Kropf et al., 2005). Alternative activation of DCs by IL-4/IL-4Rα has been documented, in which DCs upregulate markers, such as RELM-α and Ym1/2 (Cook et al., 2012), However, DCs of CD11ccreIL-4Rα−/lox mice have impaired responsiveness to IL-4/IL-13. Therefore, given the impaired classical activation, we examined markers of alternative activation to see whether they were affected by the loss of DC IL-4Rα signaling. Interestingly, we found that arginase-1 expression was unchanged in CD11ccreIL-4Rα−/lox mice compared to littermate control mice, consistent with previous work showing that IL-4 does not modulate arginase-1 expression in murine DCs (Cook et al., 2012). Surprisingly however, we found that RELM-α, another marker of alternate activation (Cook et al., 2012), was upregulated in DCs of CD11ccreIL-4Rα−/lox mice compared to littermate controls. This suggests that IL-4/IL-13-independent alternative activation of DCs can occur during L. major infection. Recent studies have demonstrated other mechanisms of alternative activation of macrophages. For example, interleukin-4-indiced gene 1 (IL-4I1) has been defined as a strong modulator of alternative macrophage activation (Yue et al., 2015), and is also expressed on DCs (Aubatin et al., 2018).
Suppression of monocyte function can also occur by the IFN-induced tryptophan-catabolizing enzyme indoleamine 2,3-dioxygenase (IDO) (Musso et al., 1994). Activated IDO depletes tryptophan levels and this depletion induces cell cycle arrest of T cells, ultimately increasing their apoptosis and causing immunosuppressive effects (Bilir and Sarisozen, 2017). It has been shown that IL-4 can inhibit IDO expression (Musso et al., 1994), so in the absence of responsiveness to IL-4, it is possible that DCs of CD11ccreIL-4Rα−/lox mice would have increased IDO expression, resulting in a more suppressive phenotype. Support for this hypothesis comes from the observation of increased TNF-α production by LN cells of CD11ccreIL-4Rα−/lox mice compared to controls, as TNF-α has been shown to activate IDO secretion (Braun et al., 2005; Kim et al., 2015; Bilir and Sarisozen, 2017). However, the role of IDO remains to be investigated in hypersusceptible CD11ccreIL-4Rα−/lox mice. The function of IDO appears to be regulation of tissue inflammation (Rani et al., 2012; Bilir and Sarisozen, 2017). DCs in the CD11ccreIL-4Rα−/lox mice expressed RELM-α, another molecule that modulates inflammation (Osborne et al., 2013). IL-4Rα independent expression of RELM-α has been recently reported during hookworm infection (Sutherland et al., 2018), and was upregulated by Ym1, another molecule typically associated with alternative macrophage activation. Previously we have shown that in the absence of IL-4Rα signaling, IL-10 can induce markers of alternative macrophage activation, including Ym1 and mannose receptor (Dewals et al., 2010). Other cytokines, such as TGF-beta and activin A have also been associated with alternative macrophage activation (Ogawa et al., 2006; Gong et al., 2012; Zhang et al., 2016). Further investigations are needed in order to clarify how cytokine signaling regulates classical and alternative activation of DCs and their interaction with intracellular pathogens.
Various lines of evidence support a role for CD11c+ cells in replication of Leishmania parasites (Leon et al., 2007; De Trez et al., 2009; Hurdayal et al., 2013; Heyde et al., 2018). A recent study by Heyde et al. (2018) described CD11c+ cells as monocyte-derived dendritic cell-like, Ly6C+CCR2+ monocytes (Heyde et al., 2018). These cells were highly infected and harbored parasites with the highest proliferation rate at the site of infection. Depletion of these CD11c-expressing cells resulted in a significant reduction in pathogen burden suggesting that CD11c+ cells represent a selective niche for L. major proliferation. Our study used a different substrain of L. major, but most likely, the cells observed by Heyde et al. were the same subset of inflammatory, monocyte-derived Ly6C+ DCs that we observed in our study. In another study, CD11b+ CD11c+ Ly6C+ MHCII+ cells were the main infected population in the footpad lesions and draining LNs of L. major-infected C57BL/6 mice, and the main iNOS-producing cells (De Trez et al., 2009). Such iNOS-producing inflammatory DC have also been implicated in resistance to Listeria monocytogenes and Brucella melitensis infection, and have been termed TNF-iNOS-producing DC (TipDCs) or inflammatory DCs (Serbina et al., 2003; Copin et al., 2007; Geissmann et al., 2008). In a visceral leishmaniasis study using L. donovani, Ly6Chi inflammatory monocytes formed an important niche for parasite survival, and blocking their recruitment to the liver and spleen using CCR2 antagonists reduced bacterial burdens (Terrazas et al., 2017). In this study, the cells were identified as CD11b+Ly6ChiLy6G− and they expressed CD115, CCR2, and intermediate levels of MHCII and F4/80, but they did not express CD11c. Why such cells express CD11c during L. major infection but not L. donovani infection is not known and remains to be elucidated.
Various markers have been used to identify inflammatory DCs, including Ly6C, F4/80, CD64 (FcγRI), FcεRI, CCR2, DC-SIGN (CD209), and mannose receptor (CD206) (De Trez et al., 2009; Hammad et al., 2010; Langlet et al., 2012; Plantinga et al., 2013; Segura and Amigorena, 2013; Min et al., 2018). CD64 alone is not able to discriminate cDCs and moDCs within CD11b+ DCs (Min et al., 2018). In the lung, Ly6C was a specific but insensitive marker for discriminating cDCs and moDCs, since Ly6C expression formed a continuum within CD11b+ lung DCs, with Ly6Clow cells found to be cDCs and Ly6Chi cells found to be moDCs (Plantinga et al., 2013). The combination of CD64 and FcεRI was suggested as more specific for discriminating moDCs in lung tissue (Plantinga et al., 2013). In our study, we found clear populations of Ly6Chi and Ly6Clo CD11b+ DCs containing parasites in the LN and FP, but in the spleen we also found a population of Ly6Cint cells. Ly6Chi and Ly6Cint CD11b+ DC populations were infected with parasites in the spleen, whereas the Ly6Clo CD11b+ DC population in the spleen was not. This suggests that the Ly6Cint population we observed may also have been moDCs, and suggests that the continuum of Ly6C expression on DCs could be to some extent tissue- and/or disease-specific.
In summary, our data suggest that CD103+ DCs play an important role in trafficking parasites to the draining LN at the onset of infection, while later during infection CD11b+ DCs become infected. Importantly, dissemination to secondary lymphoid organs, such as the spleen appears to be driven by the migration of CD11b+Ly6c+ DC inflammatory DCs. In addition, control of parasites in DCs relies on IL-4Rα signaling for early priming of Th1 responses, IFN-γ release and the subsequent upregulation of iNOS. In the absence of IL-4Rα, CD11b+Ly6C+ DCs provide a safe-haven for parasite replication and dissemination in the infected host. Thus, targeting inflammatory moDC responses may represent a strategy for reducing L. major parasite burdens and dissemination.
The datasets generated for this study are available on request to the corresponding author.
The animal study was reviewed and approved by Faculty of Health Sciences, Research Animal Ethics Committee, University of Cape Town.
RH, NN, and FB designed the study. FB provided the gene-deficient mice. RH and NN performed the experiments and analyzed results. RK performed the confocal quantification. RH and FB acquired the funding. RH and NN wrote the original draft. RH, NN, and FB edited the final version of the manuscript. All authors read and approved the final manuscript.
This work was supported by grants from the National Research Foundation, South Africa and the University of Cape Town (UCT) to RH. FB was supported with grants from the South African Medical Research Council (SAMRC), National Research Foundation (NRF) of South Africa, Department of Science and Technology (DST), South African Research Chair Initiative, International Center for Genetic Engineering and Biotechnology, and Wellcome Center for Infectious Diseases. NN was funded by the Medical Research Council South Africa and the Claude Leon Foundation. RK was funded by the Department of Science and Technology of the Republic of South Africa. The funders had no role in study design, data collection and analysis or decision to publish.
The authors declare that the research was conducted in the absence of any commercial or financial relationships that could be construed as a potential conflict of interest.
We thank the animal facility staff, the genotyping staff and Mrs. Lizette Fick for their excellent technical assistance, as well as Prof. Dirk Lang and Mrs. Susan Cooper (Department of Human Biology, UCT) for assistance with confocal microscopy. We also thank Dr. Suraj Parihar for sharing his knowledge on the confocal microscopy unit.
The Supplementary Material for this article can be found online at: https://www.frontiersin.org/articles/10.3389/fcimb.2019.00479/full#supplementary-material
Supplementary Figure 1. CD11ccreIL-4Rα−/lox BALB/c are hypersusceptible to cutaneous Leishmania major IL81 infection. Mice were infected subcutaneously with 2 × 106 stationary phase virulent GFP-expressing L. major IL81 (MHOM/IL/81/FEBNI) parasite strain into the hind footpad. Footpad swelling was measured at weekly intervals. Statistical analysis was performed defining differences to IL-4Rα−/lox mice (**, p ≤ 0.01) as significant.
Supplementary Figure 2. Representative gating strategy for dendritic cell subsets during Leishmania major infection. CD11ccreIL-4Rα−/lox BALB/c and control mice were infected subcutaneously with 2 × 106 GFP-labeled L. major IL81 promastigotes into the hind footpad. At day 3 or week 4 post-infection, total cells (either footpad, lymph node or spleen) were FACS-stained and gated on FSC-H/FSC-A to obtain singlets. Macrophages and granulocytes were excluded by staining for F4/80 and Ly6G, respectively. The resulting F4/80-Ly6G-negative population was gated for various DC subsets and receptors based on cell-surface markers as depicted.
Supplementary Figure 3. Number of CD11b+ dendritic cells is unaltered in CD11ccreIL-4Rα−/lox BALB/c and control mice. CD11ccreIL-4Rα−/lox BALB/c and control mice were infected subcutaneously with 2 × 106 GFP-labeled L. major IL81 promastigotes into the hind footpad. At Day 0, 1, 3, and Week 4 after infection, total spleen cells were stained for CD11c+CD11b+ dendritic cells by flow cytometry, and total cell numbers enumerated based on spleen cell counts.
Aubatin, A., Sako, N., Decrouy, X., Donnadieu, E., Molinier-Frenkel, V., and Castellano, F. (2018). IL4-induced gene 1 is secreted at the immune synapse and modulates TCR activation independently of its enzymatic activity. Eur. J. Immunol. 48, 106–119. doi: 10.1002/eji.201646769
Belkaid, Y., Butcher, B., and Sacks, D. L. (1998). Analysis of cytokine production by inflammatory mouse macrophages at the single-cell level: selective impairment of IL-12 induction in Leishmania-infected cells. Eur. J. Immunol. 28, 1389–1400. doi: 10.1002/(SICI)1521-4141(199804)28:04<1389::AID-IMMU1389>3.0.CO;2-1
Biedermann, T., Zimmermann, S., Himmelrich, H., Gumy, A., Egeter, O., Sakrauski, A. K., et al. (2001). IL-4 instructs TH1 responses and resistance to Leishmania major in susceptible BALB/c mice. Nat. Immunol. 2, 1054–1060. doi: 10.1038/ni725
Bilir, C., and Sarisozen, C. (2017). Indoleamine 2,3-dioxygenase (IDO): only an enzyme or a checkpoint controller? J. Oncolo. Sci. 3, 52–56. doi: 10.1016/j.jons.2017.04.001
Braun, D., Longman, R. S., and Albert, M. L. (2005). A two-step induction of indoleamine 2,3 dioxygenase (IDO) activity during dendritic-cell maturation. Blood 106, 2375–2381. doi: 10.1182/blood-2005-03-0979
Burza, S., Croft, S. L., and Boelaert, M. (2018). Leishmaniasis. Lancet 392, 951–970. doi: 10.1016/S0140-6736(18)31204-2
Cook, P. C., Jones, L. H., Jenkins, S. J., Wynn, T. A., Allen, J. E., and Macdonald, A. S. (2012). Alternatively activated dendritic cells regulate CD4+ T-cell polarization in vitro and in vivo. Proc. Natl. Acad. Sci. U.S.A. 109, 9977–9982. doi: 10.1073/pnas.1121231109
Copin, R., De Baetselier, P., Carlier, Y., Letesson, J. J., and Muraille, E. (2007). MyD88-dependent activation of B220-CD11b+LY-6C+ dendritic cells during brucella melitensis infection. J. Immunol. 178, 5182–5191. doi: 10.4049/jimmunol.178.8.5182
De Trez, C., Magez, S., Akira, S., Ryffel, B., Carlier, Y., and Muraille, E. (2009). iNOS-producing inflammatory dendritic cells constitute the major infected cell type during the chronic Leishmania major infection phase of C57BL/6 resistant mice. PLoS Pathog. 5:e1000494. doi: 10.1371/journal.ppat.1000494
Del Rio, M. L., Bernhardt, G., Rodriguez-Barbosa, J. I., and Forster, R. (2010). Development and functional specialization of CD103+ dendritic cells. Immunol. Rev. 234, 268–281. doi: 10.1111/j.0105-2896.2009.00874.x
Dewals, B. G., Marillier, R. G., Hoving, J. C., Leeto, M., Schwegmann, A., and Brombacher, F. (2010). IL-4Rα-independent expression of mannose receptor and Ym1 by macrophages depends on their IL-10 responsiveness. PLoS Negl. Trop Dis. 4:e689. doi: 10.1371/journal.pntd.0000689
Diefenbach, A., Schindler, H., Rollinghoff, M., Yokoyama, W. M., and Bogdan, C. (1999). Requirement for type 2 NO synthase for IL-12 signaling in innate immunity. Science 284, 951–955. doi: 10.1126/science.284.5416.951
Faria, M. S., Reis, F. C., and Lima, A. P. (2012). Toll-like receptors in Leishmania infections: guardians or promoters? J. Parasitol. Res. 2012:930257. doi: 10.1155/2012/930257
Geissmann, F., Auffray, C., Palframan, R., Wirrig, C., Ciocca, A., Campisi, L., et al. (2008). Blood monocytes: distinct subsets, how they relate to dendritic cells, and their possible roles in the regulation of T-cell responses. Immunol. Cell Biol. 86, 398–408. doi: 10.1038/icb.2008.19
Girard-Madoux, M. J. H., Kautz-Neu, K., Lorenz, B., Ober-Blobaum, J. L., Von Stebut, E., and Clausen, B. E. (2015). IL-10 signaling in dendritic cells attenuates anti-Leishmania major immunity without affecting protective memory responses. J. Invest. Dermatol. 135, 2890–2894. doi: 10.1038/jid.2015.236
Gong, D., Shi, W., Yi, S. J., Chen, H., Groffen, J., and Heisterkamp, N. (2012). TGFbeta signaling plays a critical role in promoting alternative macrophage activation. BMC Immunol. 13:31. doi: 10.1186/1471-2172-13-31
Gonzalez-Leal, I. J., Roger, B., Schwarz, A., Schirmeister, T., Reinheckel, T., Lutz, M. B., et al. (2014). Cathepsin B in antigen-presenting cells controls mediators of the Th1 immune response during Leishmania major infection. PLoS Negl. Trop Dis. 8:e3194. doi: 10.1371/journal.pntd.0003194
Gordon, S. (2003). Alternative activation of macrophages. Nat. Rev. Immunol. 3, 23–35. doi: 10.1038/nri978
Gordon, S., and Pluddemann, A. (2017). Tissue macrophages: heterogeneity and functions. BMC Biol. 15:53. doi: 10.1186/s12915-017-0392-4
Gutiérrez-Kobeh, L., Rodríguez-González, J., Argueta-Donohué, J., Vázquez-López, R., and Wilkins-Rodríguez, A. A. (2018). Role of Dendritic Cells in Parasitic Infections. London: IntechOpen.
Hammad, H., Plantinga, M., Deswarte, K., Pouliot, P., Willart, M. A., Kool, M., et al. (2010). Inflammatory dendritic cells–not basophils–are necessary and sufficient for induction of Th2 immunity to inhaled house dust mite allergen. J. Exp. Med. 207, 2097–2111. doi: 10.1084/jem.20101563
Handman, E. (2001). Leishmaniasis: current status of vaccine development. Clin. Microbiol. Rev. 14, 229–243. doi: 10.1128/CMR.14.2.229-243.2001
Helft, J., Manicassamy, B., Guermonprez, P., Hashimoto, D., Silvin, A., Agudo, J., et al. (2012). Cross-presenting CD103+ dendritic cells are protected from influenza virus infection. J. Clin. Invest. 122, 4037–4047. doi: 10.1172/JCI60659
Heyde, S., Philipsen, L., Formaglio, P., Fu, Y., Baars, I., Hobbel, G., et al. (2018). CD11c-expressing Ly6C+CCR2+ monocytes constitute a reservoir for efficient Leishmania proliferation and cell-to-cell transmission. PLoS Pathog. 14:e1007374. doi: 10.1371/journal.ppat.1007374
Holscher, C., Arendse, B., Schwegmann, A., Myburgh, E., and Brombacher, F. (2006). Impairment of alternative macrophage activation delays cutaneous leishmaniasis in nonhealing BALB/c mice. J. Immunol. 176, 1115–1121. doi: 10.4049/jimmunol.176.2.1115
Hurdayal, R., and Brombacher, F. (2017). Interleukin-4 receptor alpha: from innate to adaptive immunity in murine models of cutaneous leishmaniasis. Front. Immunol. 8:1354. doi: 10.3389/fimmu.2017.01354
Hurdayal, R., Ndlovu, H. H., Revaz-Breton, M., Parihar, S. P., Nono, J. K., Govender, M., et al. (2017). IL-4-producing B cells regulate T helper cell dichotomy in type 1- and type 2-controlled diseases. Proc. Natl. Acad. Sci. USA, 114, E8430–E8439. doi: 10.1073/pnas.1708125114
Hurdayal, R., Nieuwenhuizen, N. E., Revaz-Breton, M., Smith, L., Hoving, J. C., Parihar, S. P., et al. (2013). Deletion of IL-4 receptor alpha on dendritic cells renders BALB/c mice hypersusceptible to Leishmania major infection. PLoS Pathog. 9:e1003699. doi: 10.1371/journal.ppat.1003699
Jakubzick, C., Tacke, F., Ginhoux, F., Wagers, A. J., Van Rooijen, N., Mack, M., et al. (2008). Blood monocyte subsets differentially give rise to CD103+ and CD103− pulmonary dendritic cell populations. J. Immunol. 180, 3019–3027. doi: 10.4049/jimmunol.180.5.3019
Kim, S., Miller, B. J., Stefanek, M. E., and Miller, A. H. (2015). Inflammation-induced activation of the indoleamine 2,3-dioxygenase pathway: relevance to cancer-related fatigue. Cancer 121, 2129–2136. doi: 10.1002/cncr.29302
Kropf, P., Fuentes, J. M., Fahnrich, E., Arpa, L., Herath, S., Weber, V., et al. (2005). Arginase and polyamine synthesis are key factors in the regulation of experimental leishmaniasis in vivo. FASEB J. 19, 1000–1002. doi: 10.1096/fj.04-3416fje
Langlet, C., Tamoutounour, S., Henri, S., Luche, H., Ardouin, L., Gregoire, C., et al. (2012). CD64 expression distinguishes monocyte-derived and conventional dendritic cells and reveals their distinct role during intramuscular immunization. J. Immunol. 188, 1751–1760. doi: 10.4049/jimmunol.1102744
Laskay, T., Van Zandbergen, G., and Solbach, W. (2003). Neutrophil granulocytes–trojan horses for Leishmania major and other intracellular microbes? Trends Microbiol. 11, 210–214. doi: 10.1016/S0966-842X(03)00075-1
Leon, B., Lopez-Bravo, M., and Ardavin, C. (2007). Monocyte-derived dendritic cells formed at the infection site control the induction of protective T helper 1 responses against Leishmania. Immunity 26, 519–531. doi: 10.1016/j.immuni.2007.01.017
Lievin-Le Moal, V., and Loiseau, P. M. (2016). Leishmania hijacking of the macrophage intracellular compartments. FEBS J. 283, 598–607. doi: 10.1111/febs.13601
Liew, F. Y., Millott, S., Parkinson, C., Palmer, R. M., and Moncada, S. (1990). Macrophage killing of Leishmania parasite in vivo is mediated by nitric oxide from L-arginine. J. Immunol. 144, 4794–4797.
Lutz, M. B., Schnare, M., Menges, M., Rossner, S., Rollinghoff, M., Schuler, G., et al. (2002). Differential functions of IL-4 receptor types I and II for dendritic cell maturation and IL-12 production and their dependency on GM-CSF. J. Immunol. 169, 3574–3580. doi: 10.4049/jimmunol.169.7.3574
Marovich, M. A., Mcdowell, M. A., Thomas, E. K., and Nutman, T. B. (2000). IL-12p70 production by Leishmania major-harboring human dendritic cells is a CD40/CD40 ligand-dependent process. J. Immunol. 164, 5858–5865. doi: 10.4049/jimmunol.164.11.5858
Martinez-Lopez, M., Iborra, S., Conde-Garrosa, R., and Sancho, D. (2015). Batf3-dependent CD103+ dendritic cells are major producers of IL-12 that drive local Th1 immunity against Leishmania major infection in mice. Eur. J. Immunol. 45, 119–129. doi: 10.1002/eji.201444651
Martinez-Lopez, M., Soto, M., Iborra, S., and Sancho, D. (2018). Leishmania hijacks myeloid cells for immune escape. Front. Microbiol. 9:883. doi: 10.3389/fmicb.2018.00883
Mayer, J. U., Demiri, M., Agace, W. W., Macdonald, A. S., Svensson-Frej, M., and Milling, S. W. (2017). Different populations of CD11b(+) dendritic cells drive Th2 responses in the small intestine and colon. Nat. Commun. 8:15820. doi: 10.1038/ncomms15820
Min, J., Yang, D., Kim, M., Haam, K., Yoo, A., Choi, J. H., et al. (2018). Inflammation induces two types of inflammatory dendritic cells in inflamed lymph nodes. Exp. Mol. Med. 50:e458. doi: 10.1038/emm.2017.292
Mohrs, M., Ledermann, B., Kohler, G., Dorfmuller, A., Gessner, A., and Brombacher, F. (1999). Differences between IL-4- and IL-4 receptor alpha-deficient mice in chronic Leishmaniasis reveal a protective role for IL-13 receptor signaling. J. Immunol. 162, 7302–7308.
Muraille, E., De Trez, C., Pajak, B., Torrentera, F. A., De Baetselier, P., Leo, O., et al. (2003). Amastigote load and cell surface phenotype of infected cells from lesions and lymph nodes of susceptible and resistant mice infected with Leishmania major. Infect Immun. 71, 2704–2715. doi: 10.1128/IAI.71.5.2704-2715.2003
Musso, T., Gusella, G. L., Brooks, A., Longo, D. L., and Varesio, L. (1994). Interleukin-4 inhibits indoleamine 2,3-dioxygenase expression in human monocytes. Blood 83, 1408–1411. doi: 10.1182/blood.V83.5.1408.1408
Ogawa, K., Funaba, M., Chen, Y., and Tsujimoto, M. (2006). Activin A functions as a Th2 cytokine in the promotion of the alternative activation of macrophages. J. Immunol. 177, 6787–6794. doi: 10.4049/jimmunol.177.10.6787
Osborne, L. C., Joyce, K. L., Alenghat, T., Sonnenberg, G. F., Giacomin, P. R., Du, Y., et al. (2013). Resistin-like molecule alpha promotes pathogenic Th17 cell responses and bacterial-induced intestinal inflammation. J. Immunol. 190, 2292–2300. doi: 10.4049/jimmunol.1200706
Plantinga, M., Guilliams, M., Vanheerswynghels, M., Deswarte, K., Branco-Madeira, F., Toussaint, W., et al. (2013). Conventional and monocyte-derived CD11b(+) dendritic cells initiate and maintain T helper 2 cell-mediated immunity to house dust mite allergen. Immunity 38, 322–335. doi: 10.1016/j.immuni.2012.10.016
Pulendran, B., Lingappa, J., Kennedy, M. K., Smith, J., Teepe, M., Rudensky, A., et al. (1997). Developmental pathways of dendritic cells in vivo: distinct function, phenotype, and localization of dendritic cell subsets in FLT3 ligand-treated mice. J. Immunol. 159, 2222–2231. doi: 10.1016/S0165-2478(97)86082-8
Rani, R., Jordan, M. B., Divanovic, S., and Herbert, D. R. (2012). IFN-gamma-driven IDO production from macrophages protects IL-4Rα-deficient mice against lethality during Schistosoma mansoni infection. Am. J. Pathol. 180, 2001–2008. doi: 10.1016/j.ajpath.2012.01.013
Schreiber, H. A., Harding, J. S., Hunt, O., Altamirano, C. J., Hulseberg, P. D., Stewart, D., et al. (2011). Inflammatory dendritic cells migrate in and out of transplanted chronic mycobacterial granulomas in mice. J. Clin. Invest. 121, 3902–3913. doi: 10.1172/JCI45113
Scott, P., and Hunter, C. A. (2002). Dendritic cells and immunity to leishmaniasis and toxoplasmosis. Curr. Opin. Immunol. 14, 466–470. doi: 10.1016/S0952-7915(02)00353-9
Segura, E., and Amigorena, S. (2013). Inflammatory dendritic cells in mice and humans. Trends Immunol. 34, 440–445. doi: 10.1016/j.it.2013.06.001
Serbina, N. V., Salazar-Mather, T. P., Biron, C. A., Kuziel, W. A., and Pamer, E. G. (2003). TNF/iNOS-producing dendritic cells mediate innate immune defense against bacterial infection. Immunity 19, 59–70. doi: 10.1016/S1074-7613(03)00171-7
Steinman, R. M., and Inaba, K. (1999). Myeloid dendritic cells. J. Leukoc. Biol. 66, 205–208. doi: 10.1002/jlb.66.2.205
Stenger, S., Thuring, H., Rollinghoff, M., and Bogdan, C. (1994). Tissue expression of inducible nitric oxide synthase is closely associated with resistance to Leishmania major. J. Exp. Med. 180, 783–793. doi: 10.1084/jem.180.3.783
Sunderkotter, C., Kunz, M., Steinbrink, K., Meinardus-Hager, G., Goebeler, M., Bildau, H., et al. (1993). Resistance of mice to experimental leishmaniasis is associated with more rapid appearance of mature macrophages in vitro and in vivo. J. Immunol. 151, 4891–4901.
Sutherland, T. E., Ruckerl, D., Logan, N., Duncan, S., Wynn, T. A., and Allen, J. E. (2018). Ym1 induces RELMalpha and rescues IL-4Ralpha deficiency in lung repair during nematode infection. PLoS Pathog. 14:e1007423. doi: 10.1371/journal.ppat.1007423
Terrazas, C., Varikuti, S., Oghumu, S., Steinkamp, H. M., Ardic, N., Kimble, J., et al. (2017). Ly6C(hi) inflammatory monocytes promote susceptibility to Leishmania donovani infection. Sci. Rep. 7:14693. doi: 10.1038/s41598-017-14935-3
WHO (2019). Leishmaniasis. Available online at: https://www.who.int/en/news-room/fact-sheets/detail/leishmaniasis (accessed August 5, 2019).
Woelbing, F., Kostka, S. L., Moelle, K., Belkaid, Y., Sunderkoetter, C., Verbeek, S., et al. (2006). Uptake of Leishmania major by dendritic cells is mediated by Fcgamma receptors and facilitates acquisition of protective immunity. J. Exp. Med. 203, 177–188. doi: 10.1084/jem.20052288
Yao, Y., Li, W., Kaplan, M. H., and Chang, C. H. (2005). Interleukin (IL)-4 inhibits IL-10 to promote IL-12 production by dendritic cells. J. Exp. Med. 201, 1899–1903. doi: 10.1084/jem.20050324
Yue, Y., Huang, W., Liang, J., Guo, J., Ji, J., Yao, Y., et al. (2015). IL4I1 is a novel regulator of M2 macrophage polarization that can inhibit T cell activation via L-tryptophan and arginine depletion and IL-10 production. PLoS ONE 10:e0142979. doi: 10.1371/journal.pone.0142979
Zhang, F., Wang, H., Wang, X., Jiang, G., Liu, H., Zhang, G., et al. (2016). TGF-beta induces M2-like macrophage polarization via SNAIL-mediated suppression of a pro-inflammatory phenotype. Oncotarget 7, 52294–52306. doi: 10.18632/oncotarget.10561
Keywords: Leishmania major, IL-4Rα, IL-4, dendritic cell, mice
Citation: Hurdayal R, Nieuwenhuizen NE, Khutlang R and Brombacher F (2020) Inflammatory Dendritic Cells, Regulated by IL-4 Receptor Alpha Signaling, Control Replication, and Dissemination of Leishmania major in Mice. Front. Cell. Infect. Microbiol. 9:479. doi: 10.3389/fcimb.2019.00479
Received: 30 August 2019; Accepted: 27 December 2019;
Published: 24 January 2020.
Edited by:
Claudia Ida Brodskyn, Gonçalo Moniz Institute (IGM), BrazilReviewed by:
Vanessa Carregaro, University of São Paulo, BrazilCopyright © 2020 Hurdayal, Nieuwenhuizen, Khutlang and Brombacher. This is an open-access article distributed under the terms of the Creative Commons Attribution License (CC BY). The use, distribution or reproduction in other forums is permitted, provided the original author(s) and the copyright owner(s) are credited and that the original publication in this journal is cited, in accordance with accepted academic practice. No use, distribution or reproduction is permitted which does not comply with these terms.
*Correspondence: Ramona Hurdayal, ci5odXJkYXlhbEB1Y3QuYWMuemE=; Frank Brombacher, YnJvbWJhY2hlcmZyYW5rQGdtYWlsLmNvbQ==
†These authors have contributed equally to this work
Disclaimer: All claims expressed in this article are solely those of the authors and do not necessarily represent those of their affiliated organizations, or those of the publisher, the editors and the reviewers. Any product that may be evaluated in this article or claim that may be made by its manufacturer is not guaranteed or endorsed by the publisher.
Research integrity at Frontiers
Learn more about the work of our research integrity team to safeguard the quality of each article we publish.