- 1Centre for Vaccines and Immunology, National Institute for Communicable Diseases, Johannesburg, South Africa
- 2Department of Chemical Pathology, Faculty of Health Sciences, School of Pathology, University of the Witwatersrand, Johannesburg, South Africa
Progression from latency to active Tuberculosis (TB) disease is mediated by incompletely understood host immune factors. The definitive characteristic of progressive human immunodeficiency virus (HIV) disease is a severe loss in number and function of T lymphocytes. Among the many possible mediators of T lymphocyte loss and ineffective function is the activity of the immune-modulatory enzyme indoleamine 2,3-dioxygenase (IDO). IDO is the rate-limiting enzyme converting tryptophan to kynurenine. IDO activity was initially recognized to mediate tolerance at the foeto-maternal interface. Recently, IDO activity has also been noted to play a critical role in immune tolerance to pathogens. Studies of host immune and metabolic mediators have found IDO activity significantly elevated in HIV and TB disease. In this review, we explore the link between IDO-mediated tryptophan catabolism and the presence of active TB disease in HIV-infected patients. We draw attention to increased IDO activity as a key factor marking the progression from latent to active TB disease in HIV-infected patients.
Introduction
Recently, the effect of indoleamine 2,3-dioxygenase-1 (IDO) activity in various diseases, including tuberculosis (TB) and human immunodeficiency virus (HIV) disease has generated considerable interest. Tryptophan, an essential amino acid in the body, can either be converted to serotonin or oxidized to kynurenines. IDO is the rate-limiting enzyme in the conversion of tryptophan to kynurenines (Higuchi and Hayaishi, 1967; Katz et al., 2008). IDO activation plays a crucial role in macrophage and dendritic cell modulation toward alternate activation and immune tolerance (Nagamatsu and Schust, 2010; Martinez and Gordon, 2014). Two theories have been postulated to explain the mechanism of tolerance induced by IDO. Firstly, depletion of tryptophan in the microenvironment starves T cells of a vital micronutrients, which results in the inability of T cells to proliferate and function effectively (Fallarino et al., 2006; Sharma et al., 2009; Yan et al., 2010). Secondly, the accumulation of kynurenine and its downstream metabolites promotes T cell stress, anergy and apoptosis (Frumento et al., 2002; Fallarino et al., 2006; Mezrich et al., 2010; Platten et al., 2012). IDO activity plays a crucial role in T cell hypo-responsiveness during pregnancy and evasion of malignant cells from immune surveillance (Munn et al., 1998; Mellor and Munn, 1999, 2004; Nagamatsu and Schust, 2010; Platten et al., 2012). IDO is expressed in many malignancies and high IDO expression is associated with poor prognosis in a variety of cancer types (Brandacher et al., 2006; Ino et al., 2006; Creelan et al., 2013; Wei et al., 2018). Prior to 1998, IDO activity was considered to be anti-microbial, acting via depletion of available tryptophan, which is required for microbial growth (Däubener and Mackenzie, 1999). IDO activity, however, may have opposing roles, either by dominantly suppressing pathogen replication or providing a comfortable immunological niche for the pathogen to thrive in and to evade immune-mediated killing (Leonhardt et al., 2007; Almeida et al., 2009; Divanovic et al., 2011; Makala et al., 2011; Yeung et al., 2012). Both HIV-infection and TB disease are associated with upregulation of IDO activity (Divanovic et al., 2011; Blumenthal et al., 2012; Chen et al., 2014). In this review, we highlight evidence examining the role of elevated IDO activity in HIV infection and active TB disease.
Tryptophan and Its Cellular Metabolism
Essential micronutrients are those which cannot be produced endogenously and must be acquired through dietary sources. Humans, like other mammals, cannot synthesize tryptophan, and therefore, must obtain it from their diet and internal protein turn-over (Brown, 1996). Foods such as banana, spinach, soya-beans, fish, egg, milk, and cheese are rich sources of tryptophan (Sainio et al., 1996; Friedman, 2018). Human beings require about 150–250 mg tryptophan from the diet, and this amount is exceeded in regular household meals (Peters, 1991). Dietary tryptophan is absorbed and delivered through the hepatic portal system, where it enters the bloodstream for protein synthesis and other cellular functions.
In certain cell types, tryptophan can be converted to serotonin or melatonin by tryptophan hydroxylase (Ruddick et al., 2006). Alternatively, tryptophan can be catabolised to kynurenines (Higuchi and Hayaishi, 1967; Takikawa, 2005; Katz et al., 2008). Two cytosolic enzymes, IDO and Tryptophan 2,3-dioxygenase (TDO), are responsible for tryptophan catabolism in specific cell types (Takikawa, 2005). IDO and TDO are intracellular, non-secreted enzymes (Yamazaki et al., 1985). While TDO expression and activity is confined to hepatocytes, IDO is expressed by a wider variety of cells, but most potently by macrophages, dendritic and mesenchymal stem cells (Thomas et al., 2001; Dai and Zhu, 2010; Francois et al., 2010). IDO comprises two related, but distinct enzymes encoded by two different genes, namely IDO1 and IDO2 (Yamazaki et al., 1985; Takikawa, 2005; Bilir and Sarisozen, 2017). Both enzymes are expressed by epithelial and antigen-presenting cells (Munn et al., 1998; Nagamatsu and Schust, 2010; Zhu, 2010). In this review, IDO1 is referred to as IDO. IDO or TDO initiate catabolism of tryptophan by oxidizing the indole ring to produce N-formylkynurenine (Liu et al., 2016). N-formylkynurenine can be further converted to stable end-products including kynurenic acid, anthranilic acid and 3-hydroxykynurenine. N-formylkynurenine can also be further oxidized to nicotinamide adenine dinucleotide (NAD+), a vital co-factor in energy production, DNA synthesis and cellular homeostasis (Bryleva and Brundin, 2017). The tryptophan-kynurenine-NAD+ pathway represents the de novo pathway for NAD+ synthesis when niacin intake is limited in the diet (Higuchi and Hayaishi, 1967; Takikawa, 2005).
IDO Expression and Function
Munn and colleagues observed IDO expression and activity at the foeto-maternal interface in pregnant mice (Munn et al., 1998). Their findings suggested that IDO activity mediated immune tolerance to the fetus by the mother. Interestingly, blocking IDO activity in allogeneic pregnancy led to a break in the tolerogenic state that protected the fetus from the maternal immune system (Munn et al., 1998; Mellor and Munn, 1999). Since then, several studies have shown that IDO is expressed in various tissues including liver, lymph nodes, spleen and tonsils (Mellor and Munn, 2004; Bilir and Sarisozen, 2017). Antigen-presenting cells, particularly macrophages and dendritic cells, can express IDO in response to cytokines such as IFN-γ and TNF-α (Robinson et al., 2005; Nagamatsu and Schust, 2010). During infection or inflammation, IDO is stimulated in polymorphonuclear cells, endothelial cells, epithelial cells, eosinophils and fibroblasts by pro-inflammatory cytokines (Hayashi et al., 2004; Robinson et al., 2005; Xu et al., 2008).
IDO-mediated Tryptophan Metabolism and Host Immunity
Tryptophan depletion and subsequent generation of kynurenine metabolites have considerable effects on the host's immunity. The IDO pathway plays a significant role in T cell hypofunctionality, a critical concept in immune tolerance to pathogens (Mellor et al., 2017). In acute inflammation, induction of IDO may act as a regulatory feedback loop to prevent over-reaction of the immune response (Grohmann et al., 2003). In chronic inflammatory conditions, elevated IDO activity may dampen the host's protective immunity (Leonhardt et al., 2007; Divanovic et al., 2011).
Immune cells, in particular macrophages and dendritic cells, are sites of IDO production. IDO expression and activation creates a local immunosuppressive micro-environment, which allows pathogens to escape sterilizing immunity or containment (Nagamatsu and Schust, 2010). IDO induction is a feature of alternative macrophage activation, which favors a tolerogenic or anti-inflammatory state, rather than a pro-inflammatory phenotype. While a pro-inflammatory phenotype leads to killing and elimination of a pathogen, an alternatively activated phenotype leads to immune tolerance, induction of regulatory T cells, tissue repair and fibrosis (Nagamatsu and Schust, 2010; Mellor et al., 2017). Macrophage phenotypes have been termed M1 and M2, however, plasticity and intermediate types exist (Das et al., 2015). The alternative pathway of macrophage activation is shown in Figure 1.
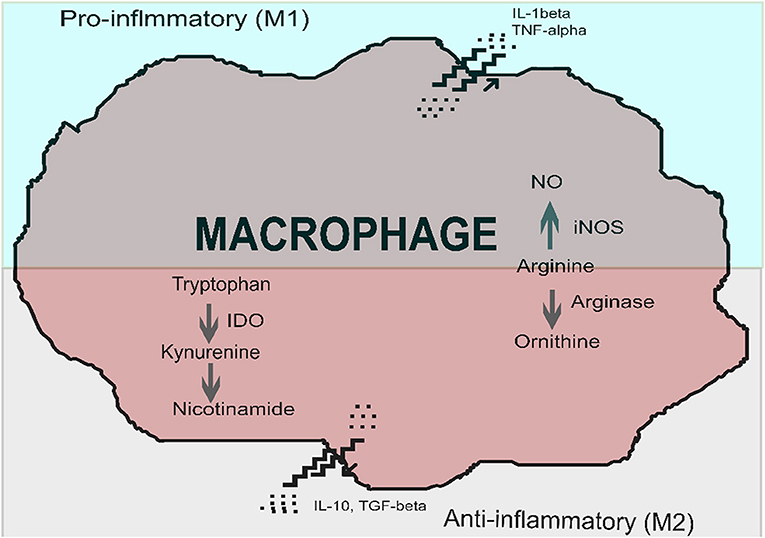
Figure 1. Macrophage activation, in the presence of indoleamine 2,3-dioxygenase (IDO), favors polarization to an immunosuppressive phenotype (alternatively activated macrophage) over that of a pro-inflammatory macrophage (classically activated macrophage). Classically activated macrophages produce pro-inflammatory cytokines such as interleukin 1-β (IL-1β) at the time of macrophage activation. Alternatively activated macrophages produce immune-suppressive cytokines such as interleukin 10 (IL-10) and transforming growth factor-beta (TGF-β). Key enzymes involved in classical activation include inducible nitric oxide synthase (iNOS), which converts arginine into nitric oxide. Two critical enzymes are involved in the alternative activation pathway: arginase converts arginine to ornithine, and indoleamine 2,3-dioxygenase converts tryptophan to kynurenines. Image adapted and modified from Nagamatsu and Schust (2010) with permission.
Downstream metabolites of the IDO pathway such as quinolinic acid, are neurotoxic and have been implicated in HIV-associated dementia (Gostner et al., 2015). Increased tryptophan oxidation leads to a net increase in circulating niacin, which increases the basal metabolic rate (Murray, 2003a). Kynurenines also activate the aryl hydrocarbon receptor (AhR), a transcription factor, key in sensing external environmental triggers and modulating gene expression in response (Lewis et al., 2014; Schmidt and Schultze, 2014; Salazar et al., 2017). Lipopolysaccharides, viral proteins such as HIV-Tat, HIV-Nef, and HIV-p17, and pathogen-associated molecular patterns, stimulate IDO expression to influence survival and pathogenesis (Murray, 2003b, Schmidt and Schultze, 2014). In malignancies, where IDO activity has been extensively studied, IDO activity correlates with dampened immunity, and poor prognosis (Ino et al., 2006; Hornyák et al., 2018).
Tryptophan Metabolism in Microbial Infections
Amino acids are critical micronutrients for all pathogens. Microbes either synthesize amino acids or depend on their hosts for amino acids for growing and multiplying. The ability of the host to deprive pathogens of essential micronutrients is a crucial feature of innate defense. Tryptophan, just like carbon, iron, and nitrogen are critical for microbial survival (Rohmer et al., 2011). Conventionally, tryptophan depletion has long been known to have anti-microbial actions for pathogens, which cannot synthesize it from smaller molecules (Russell, 2013; Zhang and Rubin, 2013; Zhang et al., 2013). Tryptophan deprivation has been shown to inhibit Chlamydia and Toxoplasmosis in vitro (Byrne et al., 1986; Murray et al., 1989). Several in vitro studies have reported that measles, influenza, cytomegalovirus, and herpes simplex viral infections are also susceptible to tryptophan depletion (Obojes et al., 2005; Zhang and Rubin, 2013; Schmidt and Schultze, 2014). On the other hand, mycobacteria possess all the complete set of tools to synthesize any essential amino acid, including tryptophan, required for their survival, a feature which gives them an evolutionary advantage (Zhang and Rubin, 2013; Zhang et al., 2013).
Animal Studies of IDO Activity in TB Pathogenesis
In animal models, increased tryptophan metabolism via the IDO pathway has been associated with poor immune responses to M. TB (O'Connor et al., 2009; Mehra et al., 2012; Zhang and Rubin, 2013; Zhang et al., 2013). IDO activation has been reported in mycobacterial infection in both mice and non-human primates (Mehra et al., 2012; Foreman et al., 2016; Gautam et al., 2018). In mice, increased IDO activation has been associated with poor TB outcomes (Moreau et al., 2005; O'Connor et al., 2009). In macaques, Mehra and colleagues demonstrated that IDO induction in the periphery of the granuloma correlates with active TB disease (Mehra et al., 2012). IDO induction was significantly higher in mock-vaccinated compared with BCG-vaccinated animals, and was seen predominantly in non-lymphocytic cells within the ring structure of the granuloma, lining the area of central necrosis (Mehra et al., 2012). Gautam et al. (2018) also showed that M. TB induces IDO expression and activity in the lung macrophages of macaques with active TB disease (Mehra et al., 2012). Based on evidence from both human and animal studies, M. TB infection is associated with high expression of IDO in the granuloma, leading to compromised T cell function, which promotes mycobacterial survival and growth (Mehra et al., 2012; Seo et al., 2015; Gautam et al., 2018). Blocking IDO expression and activity led to reduced pathogen burden, minimal TB pathology and mproved survival (Gautam et al., 2018). Furthermore, IDO inhibition was accompanied by restoration of T cell quantity and functions (Gautam et al., 2018). Collectively, these findings indicate that IDO activation plays a crucial role in M. TB pathogenesis in animal models.
Tryptophan Catabolism in HIV Infection
When co-infecting a host, HIV and M. TB influence each other, accelerating deterioration of immune function. HIV infection causes severe loss of T cells, both in number and function (Douek et al., 2001; Alimonti et al., 2003; Schindler et al., 2006). Specific mechanisms of immune impairment are beyond the scope of this review; however, it is noted that aspects of T cell exhaustion persist in the face of chronic immune activation (Brenchley et al., 2006; Doitsh et al., 2014; Doitsh and Greene, 2016; Sokoya et al., 2017). In the last three decades, a wealth of information has indicated that IDO activation and its associated metabolites play a role in human HIV infection and early progression to AIDS (Fuchs et al., 1990; Hunt et al., 2014; Jenabian et al., 2015; Mehraj and Routy, 2015; Gelpi et al., 2017). Tryptophan catabolism and its immune-regulatory effect are notable during HIV progression (Almeida et al., 2009; Mehraj and Routy, 2015; Gelpi et al., 2017; Gautam et al., 2018). There is a strong association of elevated IDO activity with AIDS-defining illnesses (Nagamatsu and Schust, 2010; Catalfamo et al., 2012; Martinez and Gordon, 2014; Sokoya et al., 2017). Furthermore, gut microbial translocation sustains increased immune activation, and chronic immune activation results in a pro-inflammatory cytokine storm, which activates the IDO pathway (Vazquez-Castellanos et al., 2015; Chen et al., 2018; Lu et al., 2018).
Werner et al. (1988) reported that HIV-infected patients have high serum kynurenine-to-tryptophan ratio (IDO activity). The elevated kynurenine-tryptophan ratio in HIV-infected patients correlates with advanced HIV disease (Huengsberg et al., 1998). Huengsberg et al. (1998) observed a striking association between the kynurenine-tryptophan ratio, declining CD4 T cells and progression to AIDS. Antiretroviral therapy (ART) improved the kynurenine-tryptophan ratio and correlated with decreased viral load and improved CD4 cell count (Zangerle et al., 2002). Hunt et al. (2014) showed that the kynurenine-tryptophan ratio was an independent predictor of death. Multiple studies have reported a similar finding of increased IDO activity or kynurenine metabolites in HIV infection (Table 1). We speculate that increased IDO-mediated tryptophan catabolism in HIV infection may be more than merely an association, but play a causal role in susceptibility to disease, particularly active TB.
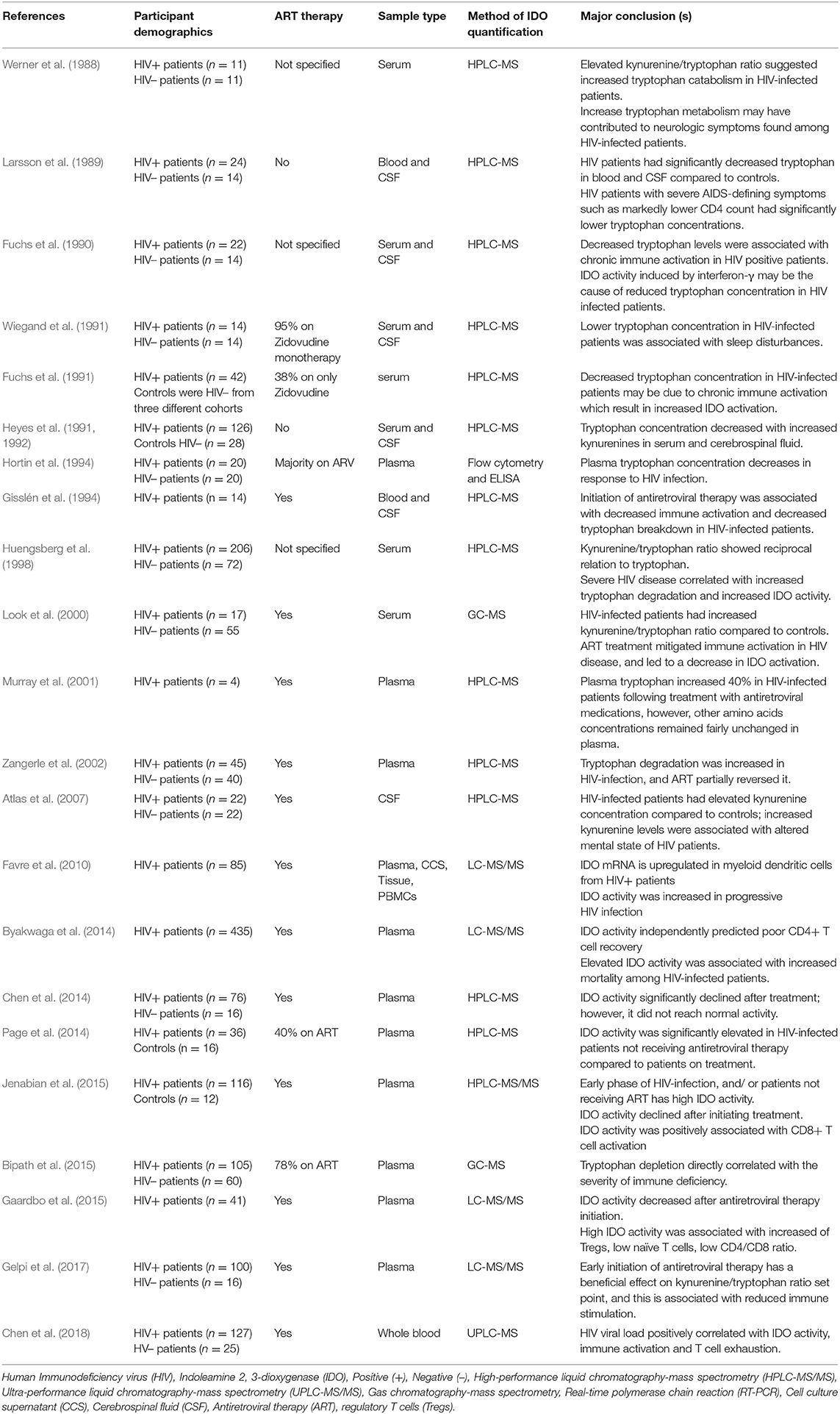
Table 1. Studies reporting elevated IDO activity, increased kynurenine or decreased tryptophan in human HIV infection.
IDO Activity in Human Tuberculosis Disease
Despite extensive work in murine and macaque models of TB, only a few studies have investigated IDO-mediated tryptophan catabolism and its metabolites in human TB (Table 2). M. TB has been shown to induce IDO activity in vitro (Blumenthal et al., 2012) and in vivo (Gautam et al., 2018). Almeida et al. (2009) studied 20 immunological mediators by reverse transcriptase-polymerase chain reaction (rt-PCR) in induced sputa of patients with active TB disease, patients with other lung diseases and controls. The authors found significantly elevated IDO expression in TB patients. The IDO levels decreased more than 500-fold within 2 weeks of beginning of TB treatment. Their work suggested that IDO might be a useful tool to diagnose active disease and monitor the efficacy of TB treatment; however, their studies did not include HIV-infected patients (Almeida et al., 2009). Weiner et al. explored more than 400 metabolites from the serum of latently infected and active TB patients and found that IDO activity was elevated in active TB patients (Weiner 3rd et al., 2012). Li et al. found increased IDO expression and activity in pleural fluid from TB patients (Li et al., 2011). We and others have shown that IDO activity is elevated at the time of TB diagnosis and declines after TB treatment (Almeida et al., 2009; Suzuki et al., 2012, 2013; Adu-Gyamfi et al., 2017) (Table 2). Both HIV-infected and uninfected patients with active TB had elevated IDO activity compared with latent TB infection (Almeida et al., 2009; Suzuki et al., 2012; Adu-Gyamfi et al., 2017; Chen et al., 2018). Additionally, IDO activity showed potential to predict the onset of active disease ahead of major TB symptoms (Adu-Gyamfi et al., 2017) and could be useful in early diagnosis of multi-drug resistance TB (Shi et al., 2019).
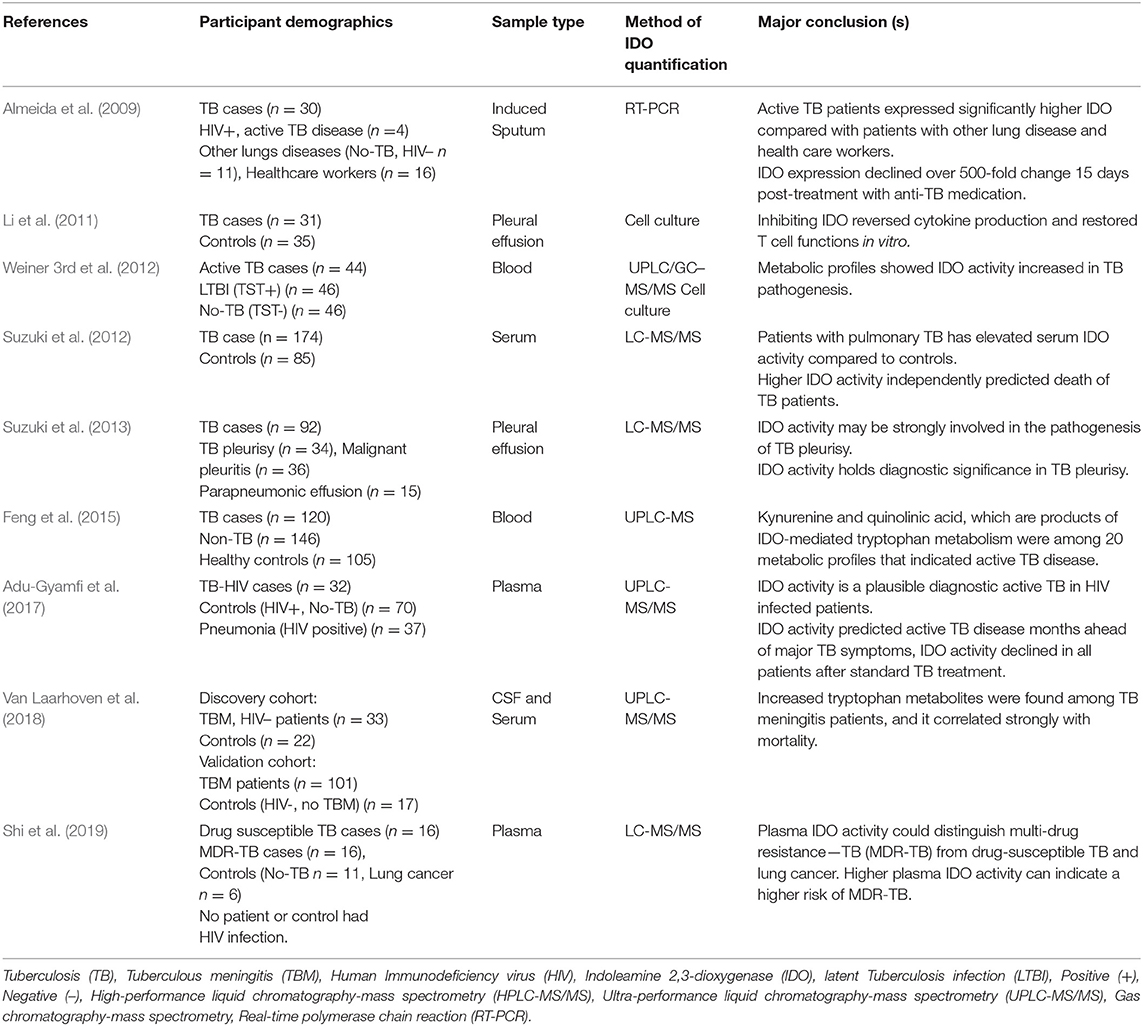
Table 2. Studies reporting IDO activity or either kynurenine or tryptophan in human active TB disease.
Laboratory Measurement of IDO Activity
IDO can be studied quantitatively by measuring mRNA expression or protein levels or enzymatic activity (product-to-substrate ratio; Li et al., 2011; Mehra et al., 2012; Suzuki et al., 2012, 2013; Huang et al., 2013; Bipath et al., 2015; Zhang et al., 2019). IDO activity can be detected in host fluids including blood, sputum, pleural fluid, cerebrospinal fluid and urine (Almeida et al., 2009; Li et al., 2011; Suzuki et al., 2012, 2013; Bipath et al., 2015; Adu-Gyamfi et al., 2017; Gautam et al., 2018; Yarbrough et al., 2018). IDO mRNA expression has been studied using rt-PCR, and IDO protein has been measured by western blot, radioimmunoassay and immunofluorescence methods (Almeida et al., 2009; Soliman et al., 2013; Zhang et al., 2019). Recently, intracellular staining methods have also been described (Sørensen et al., 2011). For purposes of clinical diagnosis, the current gold standard method for measuring IDO activity is using liquid chromatography-mass spectrometry (Suzuki et al., 2012; Huang et al., 2013; Adu-Gyamfi et al., 2017). Even though the chromatographic-mass spectrometry method has been reliable, it requires substantial laboratory infrastructure and high-grade expertise; hence the facility is not available in frontline diagnostics, especially in resource-constrained, high burden countries (Tiwari et al., 2007). Newer, higher throughput, lower-cost method–enzyme-linked immunosorbent assay (ELISA) are available (Ziklo et al., 2018). The quantitative ELISA method can be performed using cell culture supernatant, serum or plasma. We recently evaluated the ELISA method in comparison with the mass spectrometry method for measuring IDO activity. The ELISA showed good reproducibility and agreement, and could replace mass spectrometry as the analytical method for clinical use. ELISA has an added advantage of small sample volume and the potential of being fully automated compared with mass spectrometry. IDO detection in plasma has shown good discrimination between patients with active compared to latent TB, and may reflect a cumulative picture of intracellular IDO activity over time (Adu-Gyamfi et al., 2017).
IDO Activity as a Novel Active TB Biomarker in HIV-infection
A biomarker is defined as any characteristic that can be objectively measured as an indicator of health or disease process or as a response to a therapeutic intervention (Group et al., 2001; Phillips et al., 2010; Mcnerney et al., 2012; Walzl et al., 2018). In an infectious disease, biomarkers can be host or pathogen-derived parameters (Phillips et al., 2010; Mcnerney et al., 2012; Wallis et al., 2013). In TB, the lack of a biomarker indicating risk of progression to active disease, particularly in individuals with compromised immunity, presents a massive gap in consolidating efforts to eliminate the disease. Similarly, the absence of a diagnostic TB biomarker or a marker to monitor response to anti-TB therapy cripples the efficiency of global TB control strategies. Despite increased research aimed at finding a reliable, inexpensive and easily quantitated TB biomarker, our lack of full understanding of factors mediating the switch of latent TB to active TB disease is partly to be blamed for lack of an improved TB vaccine and new therapeutics.
Most TB studies have relied on animal models or ex vivo M. TB challenge in cells or tissue from active or latently infected patients to understand TB pathology. Some studies have examined metabolic profiles or genes from active TB patients to identify metabolites or genes that are switched on or off, which may be implicated in the transition from latency to active disease in both HIV-infected and uninfected individuals (Weiner 3rd et al., 2012; Scriba et al., 2017; Suliman et al., 2018). Despite dynamic efforts in the search for a sensitive, specific, and reliable TB biomarker, there has been insufficient attention paid to the host pathway of tryptophan metabolism, which is a central player in both HIV infection and TB disease. M. TB, an organism able to synthesize tryptophan, thrives during tryptophan depletion in its microenvironment, while proliferating T cells die in vitro (Zhang et al., 2013; Berney and Berney-Meyer, 2017; Gautam et al., 2018). The high level of interferon-γ during TB infection activates IDO-mediated tryptophan catabolism in macrophages (Giacomini et al., 2001; Khan et al., 2016). This phenomenon may result in M. TB's ability to hijack IDO's immunosuppressive actions on the host immune system to cause pathology.
It remains to be determined whether elevated IDO activity is causative of, or only associated with, progressive HIV and TB disease. Some researchers postulate that human co-existence with latent TB infection may be a human evolutionary adaptation to increase host tryptophan in times of low dietary tryptophan availability (Williams and Dunbar, 2013; Williams and Hill, 2017). Intriguingly, M. TB can produce nicotinamide from tryptophan (Fricker et al., 2018). An older laboratory test for distinguishing M. TB from non-tuberculous mycobacteria was known as the niacin test (Ogawa et al., 1968). Isoniazid (INH), one of the most effective anti-TB drugs was developed as a nicotinamide analog for the treatment of TB (Kushner et al., 1952; Murray, 2003a, Mckenzie et al., 1948). Serendipitously, nicotinamide was discovered to be anti-tuberculous even though the mechanism of action remained unknown (Mckenzie et al., 1948; Kushner et al., 1952; Murray, 2003a). Accordingly, these pieces of evidence support the fact that elevated flux through the tryptophan-nicotinamide pathway results in M. TB inhibition.
Currently, several clinical trials are exploring blocking IDO activity for therapeutic use. During infection with Chlamydia trachomatis, an intra-macrophage pathogen, which can synthesize tryptophan, blocking IDO activity with levo-1-methyl-tryptophan led to improved susceptibility to doxycycline (Ibana et al., 2011). In TB, mechanistic studies have demonstrated that blocking IDO activity in Simian immunodeficiency virus (SIV) infection improved T cell number and functions (Boasso and Shearer, 2007; Boasso et al., 2009). Blocking IDO activity in combination with antiviral therapy significantly reduced the SIV viral load in plasma and lymph nodes of treated animals (Boasso et al., 2009). Recently, Gautam et al. (2018) demonstrated in vivo that M. TB induces IDO expression in the lungs of macaques and mice with active TB disease. In the macaque, inhibition of IDO activity led to a reduced M. TB burden, pathology and improved animal survival (Gautam et al., 2018). Together, these results suggest that there exists a potential for using IDO inhibitors as an effective and host-driven therapy in TB. IDO inhibition as a therapeutic strategy against various cancers has yielded encouraging results (O'brien et al., 2003; Muller et al., 2005; Hanafi et al., 2014; Spranger et al., 2014; Austin and Rendina, 2015; Prendergast et al., 2017, 2018). IDO inhibitors are thus readily available and include some agents already licensed by the US Food and Drug Administration (Jeong et al., 2013).
In summary, IDO-mediated tryptophan catabolism via the kynurenine-nicotinamide pathway represents a common axis upregulated in HIV infection and TB disease. Rather than being uniquely elevated in TB, cumulative elevation of IDO in HIV and TB may explain the extraordinarily high rate of progression to active TB in HIV-infected patients. It is unclear whether elevated IDO is causative of progression to active disease or a compensatory response to the microbe. There is much promise in using metabolites from the IDO-mediated tryptophan pathway to diagnose active TB and monitor anti-TB treatment. IDO holds promise as a TB biomarker in both HIV-positive and negative patients, but further confirmatory studies are urgently needed. Regarding new TB drug targets, it remains speculative whether IDO inhibition would be beneficial. Further exploration of this pathway is necessary to better understand TB pathogenesis and discover novel host biomarkers and potential drug targets for either TB or HIV disease.
Author Contributions
All authors listed have made a substantial, direct and intellectual contribution to the work, and approved it for publication.
Funding
This research was supported by the Strategic Health Innovation Partnerships Unit of the South African Medical Research Council (MRC-SHIP, Grant no. MRC-RFA-SHIP 02-2018) with funds received from the South African Department of Science and Technology, and the International Society of Infectious Diseases (ISID, Grant no. 188T942242NI1D02).
Conflict of Interest
The authors declare that the research was conducted in the absence of any commercial or financial relationships that could be construed as a potential conflict of interest.
References
Adu-Gyamfi, C. G., Snyman, T., Hoffmann, C. J., Martinson, N. A., Chaisson, R. E., George, J. A., et al. (2017). Plasma indoleamine 2, 3-dioxygenase, a biomarker for tuberculosis in human immunodeficiency virus-infected patients. Clin. Infect. Dis. 65, 1356–1358. doi: 10.1093/cid/cix550
Alimonti, J. B., Ball, T. B., and Fowke, K. R. (2003). Mechanisms of CD4+ T lymphocyte cell death in human immunodeficiency virus infection and AIDS. J. Gen. Virol. 84, 1649–1661. doi: 10.1099/vir.0.19110-0
Almeida, A. S., Lago, P. M., Boechat, N., Huard, R. C., Lazzarini, L. C., Santos, A. R., et al. (2009). Tuberculosis is associated with a down-modulatory lung immune response that impairs Th1-type immunity. J. Immunol. 183, 718–731. doi: 10.4049/jimmunol.0801212
Atlas, A., Gisslén, M., Nordin, C., Lindström, L., and Schwieler, L. (2007). Acute psychotic symptoms in HIV-1 infected patients are associated with increased levels of kynurenic acid in cerebrospinal fluid. Brain Behav. Immun. 21, 86–91. doi: 10.1016/j.bbi.2006.02.005
Austin, C. J., and Rendina, L. M. (2015). Targeting key dioxygenases in tryptophan–kynurenine metabolism for immunomodulation and cancer chemotherapy. Drug Discov. Today 20, 609–617. doi: 10.1016/j.drudis.2014.11.007
Berney, M., and Berney-Meyer, L. (2017). Mycobacterium tuberculosis in the face of host-imposed nutrient limitation. Microbiol. Spectr. 5:TBTB2-0030-2016. doi: 10.1128/microbiolspec.TBTB2-0030-2016
Bilir, C., and Sarisozen, C. (2017). Indoleamine 2, 3-dioxygenase (Ido): only an enzyme or a checkpoint controller? J. Oncol. Sci. 3, 52–56. doi: 10.1016/j.jons.2017.04.001
Bipath, P., Levay, P. F., and Viljoen, M. (2015). The kynurenine pathway activities in a sub-Saharan HIV/AIDS population. BMC Infect. Dis. 15:346. doi: 10.1186/s12879-015-1087-5
Blumenthal, A., Nagalingam, G., Huch, J. H., Walker, L., Guillemin, G. J., Smythe, G. A., et al. (2012). M. tuberculosis induces potent activation of IDO-1, but this is not essential for the immunological control of infection. PloS ONE 7:e37314. doi: 10.1371/journal.pone.0037314
Boasso, A., and Shearer, G. M. (2007). How does indoleamine 2, 3-dioxygenase contribute to HIV-mediated immune dysregulation. Curr. Drug Metab. 8, 217–223. doi: 10.2174/138920007780362527
Boasso, A., Vaccari, M., Fuchs, D., Hardy, A. W., Tsai, W.-P., Tryniszewska, E., et al. (2009). Combined effect of antiretroviral therapy and blockade of IDO in SIV-infected rhesus macaques. J. Immunol. 182, 4313–4320. doi: 10.4049/jimmunol.0803314
Brandacher, G., Perathoner, A., Ladurner, R., Schneeberger, S., Obrist, P., Winkler, C., et al. (2006). Prognostic value of indoleamine 2, 3-dioxygenase expression in colorectal cancer: effect on tumor-infiltrating T cells. Clin. Cancer Res. 12, 1144–1151. doi: 10.1158/1078-0432.CCR-05-1966
Brenchley, J. M., Price, D. A., Schacker, T. W., Asher, T. E., Silvestri, G., Rao, S., et al. (2006). Microbial translocation is a cause of systemic immune activation in chronic HIV infection. Nat. Med. 12:1365. doi: 10.1038/nm1511
Brown, R. (1996). “Metabolism and biology of tryptophan,” in Recent Advances in Tryptophan Research, eds G. A. Filippini, C. V. L. Costa, and A. Bertazzo (Podova: Springer), 15–25.
Bryleva, E. Y., and Brundin, L. (2017). Kynurenine pathway metabolites and suicidality. Neuropharmacology 112, 324–330. doi: 10.1016/j.neuropharm.2016.01.034
Byakwaga, H., Boum, Y., Huang, Y., Muzoora, C., Kembabazi, A., Weiser, S. D., et al. (2014). The kynurenine pathway of tryptophan catabolism, CD4+ T-cell recovery, and mortality among HIV-infected Ugandans initiating antiretroviral therapy. J. Infect. Dis. 210, 383–391. doi: 10.1093/infdis/jiu115
Byrne, G. I., Lehmann, L. K., and Landry, G. J. (1986). Induction of tryptophan catabolism is the mechanism for gamma-interferon-mediated inhibition of intracellular Chlamydia psittaci replication in T24 cells. Infect. Immun. 53, 347–351.
Catalfamo, M., Le Saout, C., and Lane, H. C. (2012). The role of cytokines in the pathogenesis and treatment of HIV infection. Cytokine Growth Factor Rev. 23, 207–214. doi: 10.1016/j.cytogfr.2012.05.007
Chen, J., Shao, J., Cai, R., Shen, Y., Zhang, R., Liu, L., et al. (2014). Anti-retroviral therapy decreases but does not normalize indoleamine 2, 3-dioxygenase activity in HIV-infected patients. PLoS ONE 9:e100446. doi: 10.1371/journal.pone.0100446
Chen, J., Xun, J., Yang, J., Ji, Y., Liu, L., Qi, T., et al. (2018). Plasma indoleamine 2,3-dioxygenase activity is associated with the size of the human immunodeficiency virus reservoir in patients receiving antiretroviral therapy. Clin. Infect. Dis. 68, 1274–1281. doi: 10.1093/cid/ciy676
Creelan, B. C., Antonia, S. J., Bepler, G., Garrett, T. J., Simon, G. R., and Soliman, H. H. (2013). Indoleamine 2, 3-dioxygenase activity and clinical outcome following induction chemotherapy and concurrent chemoradiation in Stage III non-small cell lung cancer. Oncoimmunology 2:e23428. doi: 10.4161/onci.23428
Dai, X., and Zhu, B. T. (2010). Indoleamine 2, 3-dioxygenase tissue distribution and cellular localization in mice: implications for its biological functions. J. Histochem. Cytochem. 58, 17–28. doi: 10.1369/jhc.2009.953604
Das, A., Sinha, M., Datta, S., Abas, M., Chaffee, S., Sen, C. K., et al. (2015). Monocyte and macrophage plasticity in tissue repair and regeneration. Am. J. Pathol. 185, 2596–2606. doi: 10.1016/j.ajpath.2015.06.001
Däubener, W., and Mackenzie, C. R. (1999). IFN-γ activated indoleamine 2, 3-dioxygenase activity in human cells is an antiparasitic and an antibacterial effector mechanism. Adv. Exp. Med. Biol. 467, 517–524. doi: 10.1007/978-1-4615-4709-9_64
Divanovic, S., Sawtell, N. M., Trompette, A., Warning, J. I., Dias, A., Cooper, A. M., et al. (2011). Opposing biological functions of tryptophan catabolizing enzymes during intracellular infection. J. Infect. Dis. 205, 152–161. doi: 10.1093/infdis/jir621
Doitsh, G., Galloway, N. L., Geng, X., Yang, Z., Monroe, K. M., Zepeda, O., et al. (2014). Cell death by pyroptosis drives CD4 T-cell depletion in HIV-1 infection. Nature 505, 509–514. doi: 10.1038/nature12940
Doitsh, G., and Greene, W. C. (2016). Dissecting how CD4 T cells are lost during HIV infection. Cell Host Microbe 19, 280–291. doi: 10.1016/j.chom.2016.02.012
Douek, D. C., Betts, M. R., Hill, B. J., Little, S. J., Lempicki, R., Metcalf, J. A., et al. (2001). Evidence for increased T cell turnover and decreased thymic output in HIV infection. J. Immunol. 167, 6663–6668. doi: 10.4049/jimmunol.167.11.6663
Fallarino, F., Grohmann, U., You, S., Mcgrath, B. C., Cavener, D. R., Vacca, C., et al. (2006). The combined effects of tryptophan starvation and tryptophan catabolites down-regulate T cell receptor ζ-chain and induce a regulatory phenotype in naive T cells. J. Immunol. 176, 6752–6761. doi: 10.4049/jimmunol.176.11.6752
Favre, D., Mold, J., Hunt, P. W., Kanwar, B., Seu, L., Barbour, J. D., et al. (2010). Tryptophan catabolism by indoleamine 2, 3-dioxygenase 1 alters the balance of TH17 to regulatory T cells in HIV disease. Sci. Transl. Med. 2, 32ra36. doi: 10.1126/scitranslmed.3000632
Feng, S., Du, Y.-Q., Zhang, L., Zhang, L., Feng, R.-R., and Liu, S.-Y. (2015). Analysis of serum metabolic profile by ultra-performance liquid chromatography-mass spectrometry for biomarkers discovery: application in a pilot study to discriminate patients with tuberculosis. Chin. Med. J. 128, 159–168. doi: 10.4103/0366-6999.149188
Foreman, T. W., Mehra, S., Lobato, D. N., Malek, A., Alvarez, X., Golden, N. A., et al. (2016). CD4+ T-cell–independent mechanisms suppress reactivation of latent tuberculosis in a macaque model of HIV coinfection. Proc. Natl. Acad. Sci. U.S.A. 113, E5636–E5644. doi: 10.1073/pnas.1611987113
Francois, M., Romieu, R., Li, M., and Galipeau, J. (2010). IDO Expression in human mesenchymal stromal cells mediates T cell suppression and leads to monocyte differentiation into IL-10 secreting immunosuppressive CD206+ M2 macrophages. Am. Soc. Hematol. doi: 10.1182/blood.V116.21.2784.2784
Fricker, R. A., Green, E. L., Jenkins, S. I., and Griffin, S. M. (2018). The influence of nicotinamide on health and disease in the central nervous system. Int. J. Tryptophan Res. 11:1178646918776658. doi: 10.1177/1178646918776658
Friedman, M. (2018). Analysis, nutrition, and health benefits of tryptophan. Int. J. Tryptophan Res. 11:1178646918802282. doi: 10.1177/1178646918802282
Frumento, G., Rotondo, R., Tonetti, M., Damonte, G., Benatti, U., and Ferrara, G. B. (2002). Tryptophan-derived catabolites are responsible for inhibition of T and natural killer cell proliferation induced by indoleamine 2, 3-dioxygenase. J. Exp. Med. 196, 459–468. doi: 10.1084/jem.20020121
Fuchs, D., Forsman, A., Hagberg, L., Larsson, M., Norkrans, G., Reibnegger, G., et al. (1990). Immune activation and decreased tryptophan in patients with HIV-1 infection. J. Interferon Res. 10, 599–603. doi: 10.1089/jir.1990.10.599
Fuchs, D., Möller, A. A., Reibnegger, G., Werner, E. R., Werner-Felmayer, G., Dierich, M. P., et al. (1991). Increased endogenous interferon-gamma and neopterin correlate with increased degradation of tryptophan in human immunodeficiency virus type 1 infection. Immunol. Lett. 28, 207–211. doi: 10.1016/0165-2478(91)90005-U
Gaardbo, J. C., Trøsied, M., Stiksrud, B., Midttun, Ø., Ueland, P. M., Ullum, H., et al. (2015). Increased tryptophan catabolism is associated with increased frequency of CD161+ Tc17/MAIT cells and lower CD4+ T-cell count in HIV-1 infected patients on cART after 2 years of follow-up. J. Acquir. Immune Defic. Syndr. 70, 228–235. doi: 10.1097/QAI.0000000000000758
Gautam, U. S., Foreman, T. W., Bucsan, A. N., Veatch, A. V., Alvarez, X., Adekambi, T., et al. (2018). In vivo inhibition of tryptophan catabolism reorganizes the tuberculoma and augments immune-mediated control of Mycobacterium tuberculosis. Proc. Natl. Acad. Sci. U.S.A. 115, E62–E71. doi: 10.1073/pnas.1711373114
Gelpi, M., Hartling, H. J., Ueland, P. M., Ullum, H., Trøseid, M., and Nielsen, S. D. (2017). Tryptophan catabolism and immune activation in primary and chronic HIV infection. BMC Infect. Dis. 17:349. doi: 10.1186/s12879-017-2456-z
Giacomini, E., Iona, E., Ferroni, L., Miettinen, M., Fattorini, L., Orefici, G., et al. (2001). Infection of human macrophages and dendritic cells with Mycobacterium tuberculosis induces a differential cytokine gene expression that modulates T cell response. J. Immunol. 166, 7033–7041. doi: 10.4049/jimmunol.166.12.7033
Gisslén, M., Larsson, M., Norkrans, G., Fuchs, D., Wachter, H., and Hagberg, L. (1994). Tryptophan concentrations increase in cerebrospinal fluid and blood after zidovudine treatment in patients with HIV type 1 infection. AIDS Res. Hum. Retroviruses 10, 947–951. doi: 10.1089/aid.1994.10.947
Gostner, J. M., Becker, K., Kurz, K., and Fuchs, D. (2015). Disturbed amino acid metabolism in HIV: association with neuropsychiatric symptoms. Front. Psychiatry 6:97. doi: 10.3389/fpsyt.2015.00097
Grohmann, U., Fallarino, F., and Puccetti, P. (2003). Tolerance, DCs and tryptophan: much ado about IDO. Trends Immunol. 24, 242–248. doi: 10.1016/S1471-4906(03)00072-3
Group, B. D. W., Atkinson, A. J. Jr, Colburn, W. A., Degruttola, V. G., Demets, D. L., Downing, G. J., et al. (2001). Biomarkers and surrogate endpoints: preferred definitions and conceptual framework. Clin. Pharmacol. Therap. 69, 89–95. doi: 10.1067/mcp.2001.113989
Hanafi, L.-A., Gauchat, D., Godin-Ethier, J., Possamaï, D., Duvignaud, J.-B., Leclerc, D., et al. (2014). Fludarabine downregulates indoleamine 2, 3-dioxygenase in tumors via a proteasome-mediated degradation mechanism. PLoS ONE 9:e99211. doi: 10.1371/journal.pone.0099211
Hayashi, T., Beck, L., Rossetto, C., Gong, X., Takikawa, O., Takabayashi, K., et al. (2004). Inhibition of experimental asthma by indoleamine 2, 3-dioxygenase. J. Clin. Invest. 114, 270–279. doi: 10.1172/JCI21275
Heyes, M. P., Brew, B. J., Martin, A., Price, R. W., Salazar, A. M., Sidtis, J. J., et al. (1991). Quinolinic acid in cerebrospinal fluid and serum in HIV-1 infection: relationship to clinical and neurological status. Ann. Neurol. 29, 202–209. doi: 10.1002/ana.410290215
Heyes, M. P., Brew, B. J., Saito, K., Quearry, B. J., Price, R. W., Lee, K., et al. (1992). Inter-relationships between quinolinic acid, neuroactive kynurenines, neopterin and β2-microglobulin in cerebrospinal fluid and serum of HIV-1-infected patients. J. Neuroimmunol. 40, 71–80. doi: 10.1016/0165-5728(92)90214-6
Higuchi, K., and Hayaishi, O. (1967). Enzymic formation of D-kynurenine from D-tryptophan. Arch. Biochem. Biophys. 120, 397–403. doi: 10.1016/0003-9861(67)90256-1
Hornyák, L., Dobos, N., Koncz, G., Karányi, Z., Páll, D., Szabó, Z., et al. (2018). The role of indoleamine-2, 3-dioxygenase in cancer development, diagnostics, and therapy. Front. Immunol. 9, 151. doi: 10.3389/fimmu.2018.00151
Hortin, G. L., Landt, M., and Powderly, W. G. (1994). Changes in plasma amino acid concentrations in response to HIV-1 infection. Clin. Chem. 40, 785–789.
Huang, Y., Louie, A., Yang, Q., Massenkoff, N., Xu, C., Hunt, P. W., et al. (2013). A simple LC–MS/MS method for determination of kynurenine and tryptophan concentrations in human plasma from HIV-infected patients. Bioanalysis 5, 1397–1407. doi: 10.4155/bio.13.74
Huengsberg, M., Winer, J. B., Gompels, M., Round, R., Ross, J., and Shahmanesh, M. (1998). Serum kynurenine-to-tryptophan ratio increases with progressive disease in HIV-infected patients. Clin. Chem. 44, 858–862.
Hunt, P. W., Sinclair, E., Rodriguez, B., Shive, C., Clagett, B., Funderburg, N., et al. (2014). Gut epithelial barrier dysfunction and innate immune activation predict mortality in treated HIV infection. J. Infect. Dis. 210, 1228–1238. doi: 10.1093/infdis/jiu238
Ibana, J. A., Belland, R. J., Zea, A. H., Schust, D. J., Nagamatsu, T., Abdelrahman, Y. M., et al. (2011). Inhibition of indoleamine 2, 3-dioxygenase activity by levo-1-methyl tryptophan blocks gamma interferon-induced Chlamydia trachomatis persistence in human epithelial cells. Infect. Immun. 79, 4425–4437. doi: 10.1128/IAI.05659-11
Ino, K., Yoshida, N., Kajiyama, H., Shibata, K., Yamamoto, E., Kidokoro, K., et al. (2006). Indoleamine 2, 3-dioxygenase is a novel prognostic indicator for endometrial cancer. Br. J. Cancer 95, 1555–1561. doi: 10.1038/sj.bjc.6603477
Jenabian, M.-A., El-Far, M., Vyboh, K., Kema, I., Costiniuk, C. T., Thomas, R., et al. (2015). Immunosuppressive tryptophan catabolism and gut mucosal dysfunction following early HIV infection. J. Infect. Dis. 212, 355–366. doi: 10.1093/infdis/jiv037
Jeong, W., Doroshow, J. H., and Kummar, S. (2013). US FDA approved oral kinase inhibitors for the treatment of malignancies. Curr. Probl. Cancer 37, 110–144. doi: 10.1016/j.currproblcancer.2013.06.001
Katz, J. B., Muller, A. J., and Prendergast, G. C. (2008). Indoleamine 2, 3-dioxygenase in T-cell tolerance and tumoral immune escape. Immunol. Rev. 222, 206–221. doi: 10.1111/j.1600-065X.2008.00610.x
Khan, T. A., Mazhar, H., Saleha, S., Tipu, H. N., Muhammad, N., and Abbas, M. N. (2016). Interferon-gamma improves macrophages function against M. tuberculosis in multidrug-resistant tuberculosis patients. Chemother. Res. Pract. 2016:7295390. doi: 10.1155/2016/7295390
Kushner, S., Dalalian, H., Sanjurjo, J., Bach, F. Jr., Safir, S., Smith, V. Jr, et al. (1952). Experimental chemotherapy of tuberculosis. II. The synthesis of pyrazinamides and related compounds1. J. Am. Chem. Soc. 74, 3617–3621. doi: 10.1021/ja01134a045
Larsson, M., Hagberg, L., Norkrans, G., and Forsman, A. (1989). Indole amine deficiency in blood and cerebrospinal fluid from patients with human immunodeficiency virus infection. J. Neurosci. Res. 23, 441–446. doi: 10.1002/jnr.490230410
Leonhardt, R. M., Lee, S.-J., Kavathas, P. B., and Cresswell, P. (2007). Severe tryptophan starvation blocks onset of conventional persistence and reduces reactivation of Chlamydia trachomatis. Infect. Immun. 75, 5105–5117. doi: 10.1128/IAI.00668-07
Lewis, C. N., Chinnadurai, R., and Galipeau, J. (2014). Tryptophan catabolites directly modulate the immunosuppressive effects of MSCs via activation of the endogenous aryl hydrocarbon receptor. Am. Soc. Hematol. 124:1593. doi: 10.1182/blood.V124.21.1593.1593
Li, Q., Li, L., Liu, Y., Fu, X., Qiao, D., Wang, H., et al. (2011). Pleural fluid from tuberculous pleurisy inhibits the functions of T cells and the differentiation of Th1 cells via immunosuppressive factors. Cell. Mol. Immunol. 8, 172–180. doi: 10.1038/cmi.2010.80
Liu, Y., Zhang, Y., Zheng, X., Zhang, X., Wang, H., Li, Q., et al. (2016). Gene silencing of indoleamine 2, 3-dioxygenase 2 in melanoma cells induces apoptosis through the suppression of NAD+ and inhibits in vivo tumor growth. Oncotarget 7, 32329–32340. doi: 10.18632/oncotarget.8617
Look, M. P., Altfeld, M., Kreuzer, K. A., Riezler, R., Stabler, S. P., Allen, R. H., et al. (2000). Parallel decrease in neurotoxin quinolinic acid and soluble tumor necrosis factor receptor p75 in serum during highly active antiretroviral therapy of HIV type 1 disease. AIDS Res. Hum. Retroviruses 16, 1215–1221. doi: 10.1089/08892220050116989
Lu, W., Feng, Y., Jing, F., Han, Y., Lyu, N., Liu, F., et al. (2018). Association between gut microbiota and cd4 recovery in HIV-1 infected patients. Front. Microbiol. 9:1451. doi: 10.3389/fmicb.2018.01451
Makala, L. H., Baban, B., Lemos, H., El-Awady, A. R., Chandler, P. R., Hou, D. Y., et al. (2011). Leishmania major attenuates host immunity by stimulating local indoleamine 2,3-dioxygenase expression. J. Infect. Dis. 203, 715–725. doi: 10.1093/infdis/jiq095
Martinez, F. O., and Gordon, S. (2014). The M1 and M2 paradigm of macrophage activation: time for reassessment. F1000prime Rep. 6:13. doi: 10.12703/P6-13
Mckenzie, D., Malone, L., Kushner, S., Oleson, J., and Subbarow, Y. (1948). The effect of nicotinic acid amide on experimental tuberculosis of white mice. J. Lab. Clin. Med. 33, 1249–1253.
Mcnerney, R., Maeurer, M., Abubakar, I., Marais, B., Mchugh, T. D., Ford, N., et al. (2012). Tuberculosis diagnostics and biomarkers: needs, challenges, recent advances, and opportunities. J. Infect. Dis. 205, S147–S158. doi: 10.1093/infdis/jir860
Mehra, S., Alvarez, X., Didier, P. J., Doyle, L. A., Blanchard, J. L., Lackner, A. A., et al. (2012). Granuloma correlates of protection against tuberculosis and mechanisms of immune modulation by Mycobacterium tuberculosis. J. Infect. Dis., 207, 1115–1127. doi: 10.1093/infdis/jis778
Mehraj, V., and Routy, J.-P. (2015). Tryptophan catabolism in chronic viral infections: handling uninvited guests. Int. J. Tryptophan Res. 8:S26862. doi: 10.4137/IJTR.S26862
Mellor, A. L., Lemos, H., and Huang, L. (2017). Indoleamine 2, 3-Dioxygenase and Tolerance: where Are we now? Front. Immunol. 8:1360. doi: 10.3389/fimmu.2017.01360
Mellor, A. L., and Munn, D. H. (1999). Tryptophan catabolism and T-cell tolerance: immunosuppression by starvation? Immunol. Today 20, 469–473. doi: 10.1016/S0167-5699(99)01520-0
Mellor, A. L., and Munn, D. H. (2004). IDO expression by dendritic cells: tolerance and tryptophan catabolism. Nat. Rev. Immunol. 4, 762–774. doi: 10.1038/nri1457
Mezrich, J. D., Fechner, J. H., Zhang, X., Johnson, B. P., Burlingham, W. J., and Bradfield, C. A. (2010). An interaction between kynurenine and the aryl hydrocarbon receptor can generate regulatory T cells. J. Immunol. 185, 3190–3198. doi: 10.4049/jimmunol.0903670
Moreau, M., Lestage, J., Verrier, D., Mormede, C., Kelley, K. W., Dantzer, R., et al. (2005). Bacille Calmette-Guerin inoculation induces chronic activation of peripheral and brain indoleamine 2, 3-dioxygenase in mice. J. Infect. Dis. 192, 537–544. doi: 10.1086/431603
Muller, A. J., Duhadaway, J. B., Donover, P. S., Sutanto-Ward, E., and Prendergast, G. C. (2005). Inhibition of indoleamine 2, 3-dioxygenase, an immunoregulatory target of the cancer suppression gene Bin1, potentiates cancer chemotherapy. Nat. Med. 11, 312–319. doi: 10.1038/nm1196
Munn, D. H., Zhou, M., Attwood, J. T., Bondarev, I., Conway, S. J., Marshall, B., et al. (1998). Prevention of allogeneic fetal rejection by tryptophan catabolism. Science 281, 1191–1193. doi: 10.1126/science.281.5380.1191
Murray, H. W., Szuro-Sudol, A., Wellner, D., Oca, M. J., Granger, A. M., Libby, D. M., et al. (1989). Role of tryptophan degradation in respiratory burst-independent antimicrobial activity of gamma interferon-stimulated human macrophages. Infect. Immun. 57, 845–849.
Murray, M. F. (2003a). Nicotinamide: an oral antimicrobial agent with activity against both Mycobacterium tuberculosis and human immunodeficiency virus. Clin. Infect. Dis. 36, 453–460. doi: 10.1086/367544
Murray, M. F. (2003b). Tryptophan depletion and HIV Tryptophan depletion and HIV infection: a metabolic link to pathogenesis. Lancet Infect. Dis. 3, 644–652. doi: 10.1016/S1473-3099(03)00773-4
Murray, M. F., Langan, M., and Macgregor, R. R. (2001). Increased plasma tryptophan in HIV-infected patients treated with pharmacologic doses of nicotinamide. Nutrition 17, 654–656. doi: 10.1016/S0899-9007(01)00568-8
Nagamatsu, T., and Schust, D. J. (2010). The contribution of macrophages to normal and pathological pregnancies. Am. J. Reprod. Immunol. 63, 460–471. doi: 10.1111/j.1600-0897.2010.00813.x
Obojes, K., Andres, O., Kim, K. S., Däubener, W., and Schneider-Schaulies, J. (2005). Indoleamine 2, 3-dioxygenase mediates cell type-specific anti-measles virus activity of gamma interferon. J. Virol. 79, 7768–7776. doi: 10.1128/JVI.79.12.7768-7776.2005
O'brien, S. G., Guilhot, F., Larson, R. A., Gathmann, I., Baccarani, M., Cervantes, F., et al. (2003). Imatinib compared with interferon and low-dose cytarabine for newly diagnosed chronic-phase chronic myeloid leukemia. N. Engl. J. Med. 348, 994–1004. doi: 10.1056/NEJMoa022457
O'Connor, J. C., Lawson, M. A., André, C., Briley, E. M., Szegedi, S. S., Lestage, J., et al. (2009). Induction of IDO by bacille Calmette-Guerin is responsible for development of murine depressive-like behavior. J. Immunol. 182, 3202–3212. doi: 10.4049/jimmunol.0802722
Ogawa, T., Hatanaka, E., and Nishimura, S. (1968). Niacin test for differentiation of Mycobacterium tuberculosis var. hominis from other acid-fast bacilli. XII. Clinical and bacteriological examination of 2 cases with pulmonary tuberculosis which had excreted niacin test negative Mycobacterium tuberculosis var. hominis for a long period. 3. Animal inoculation of the detected acid-fast bacilli. Kitasato Arch. Exp. Med. 41, 99–112.
Page, E. E., Greathead, L., Metcalf, R., Clark, S.-A., Hart, M., Fuchs, D., et al. (2014). Loss of Th22 cells is associated with increased immune activation and IDO-1 activity in HIV-1 infection. J. Acquir. Immune Def. Syndr. 67, 227–235. doi: 10.1097/QAI.0000000000000294
Peters, J. (1991). Tryptophan nutrition and metabolism: an overview. Adv. Exp. Med. Biol. 294, 345–358. doi: 10.1007/978-1-4684-5952-4_32
Phillips, P. P. J., Davies, G. R., and Mitchison, D. A. (2010). Biomarkers for tuberculosis disease activity, cure, and relapse. Lancet Infect. Dis. 10, 69–70. doi: 10.1016/S1473-3099(09)70256-7
Platten, M., Wick, W., and Van Den Eynde, B. J. (2012). Tryptophan catabolism in cancer: beyond IDO and tryptophan depletion. Cancer Res. 72, 5435–5440. doi: 10.1158/0008-5472.CAN-12-0569
Prendergast, G. C., Malachowski, W. J., Mondal, A., Scherle, P., and Muller, A. J. (2018). Indoleamine 2, 3-dioxygenase and its therapeutic inhibition in cancer. Int. Rev. Cell Mol. Biol. 336, 175–203. doi: 10.1016/bs.ircmb.2017.07.004
Prendergast, G. C., Malachowski, W. P., Duhadaway, J. B., and Muller, A. J. (2017). Discovery of IDO1 inhibitors: from bench to bedside. Cancer Res. 77, 6795–6811. doi: 10.1158/0008-5472.CAN-17-2285
Robinson, C. M., Hale, P. T., and Carlin, J. M. (2005). The role of IFN-γ and TNF-α-responsive regulatory elements in the synergistic induction of indoleamine dioxygenase. J. Interferon Cytokine Res. 25, 20–30. doi: 10.1089/jir.2005.25.20
Rohmer, L., Hocquet, D., and Miller, S. I. (2011). Are pathogenic bacteria just looking for food? Metabolism and microbial pathogenesis. Trends Microbiol. 19, 341–348. doi: 10.1016/j.tim.2011.04.003
Ruddick, J. P., Evans, A. K., Nutt, D. J., Lightman, S. L., Rook, G. A., and Lowry, C. A. (2006). Tryptophan metabolism in the central nervous system: medical implications. Expert Rev. Mol. Med. 8, 1–27. doi: 10.1017/S1462399406000068
Sainio, E.-L., Pulkki, K., and Young, S. N. (1996). L-Tryptophan: Biochemical, nutritional and pharmacological aspects. Amino Acids 10, 21–47. doi: 10.1007/BF00806091
Salazar, F., Awuah, D., Negm, O. H., Shakib, F., and Ghaemmaghami, A. M. (2017). The role of indoleamine 2,3-dioxygenase-aryl hydrocarbon receptor pathway in the TLR4-induced tolerogenic phenotype in human DCs. Sci. Rep. 7:43337. doi: 10.1038/srep43337
Schindler, M., Münch, J., Kutsch, O., Li, H., Santiago, M. L., Bibollet-Ruche, F., et al. (2006). Nef-mediated suppression of T cell activation was lost in a lentiviral lineage that gave rise to HIV-1. Cell 125, 1055–1067. doi: 10.1016/j.cell.2006.04.033
Schmidt, S. V., and Schultze, J. L. (2014). New insights into IDO biology in bacterial and viral infections. Front. Immunol. 5:384. doi: 10.3389/fimmu.2014.00384
Scriba, T. J., Penn-Nicholson, A., Shankar, S., Hraha, T., Thompson, E. G., Sterling, D., et al. (2017). Sequential inflammatory processes define human progression from M. tuberculosis infection to tuberculosis disease. PLoS Pathog. 13:e1006687. doi: 10.1371/journal.ppat.1006687
Seo, K.-J., Yoo, C.-Y., Im, S.-Y., Yeo, C.-D., Jung, J.-H., Choi, H.-J., et al. (2015). A possible complementary tool for diagnosing tuberculosis: a feasibility test of immunohistochemical markers. Int. J. Clin. Exp. Pathol. 8, 13900–13910.
Sharma, M. D., Hou, D.-Y., Liu, Y., Koni, P. A., Metz, R., Chandler, P., et al. (2009). Indoleamine 2, 3-dioxygenase controls conversion of Foxp3+ Tregs to TH17-like cells in tumor-draining lymph nodes. Blood 113, 6102–6111. doi: 10.1182/blood-2008-12-195354
Shi, W., Wu, J., Tan, Q., Hu, C.-M., Zhang, X., Pan, H.-Q., et al. (2019). Plasma indoleamine 2, 3-dioxygenase activity as a potential biomarker for early diagnosis of multidrug-resistant tuberculosis in tuberculosis patients. Infect. Drug Resist. 12, 1265–1276. doi: 10.2147/IDR.S202369
Sokoya, T., Steel, H. C., Nieuwoudt, M., and Rossouw, T. M. (2017). HIV as a cause of immune activation and immunosenescence. Mediators Inflamm. 2017:6825493. doi: 10.1155/2017/6825493
Soliman, H., Rawal, B., Fulp, J., Lee, J.-H., Lopez, A., Bui, M. M., et al. (2013). Analysis of indoleamine 2-3 dioxygenase (IDO1) expression in breast cancer tissue by immunohistochemistry. Cancer Immunol. Immunother. 62, 829–837. doi: 10.1007/s00262-013-1393-y
Sørensen, R. B., Hadrup, S. R., Svane, I. M., Hjortsø, M. C., Thor Straten, P., and Andersen, M. H. (2011). Indoleamine 2, 3-dioxygenase specific, cytotoxic T cells as immune regulators. Blood 117, 2200–2210. doi: 10.1182/blood-2010-06-288498
Spranger, S., Koblish, H., Horton, B., Scherle, P., Newton, R., and Gajewski, T. (2014). Mechanism of tumor rejection with doublets of CTLA-4, PD-1/PD-L1, or IDO blockade involves restored IL-2 production and proliferation of CD8(+) T cells directly within the tumor microenvironment. J. Immunother. Cancer 2:3. doi: 10.1186/2051-1426-2-3
Suliman, S., Thompson, E. G., Sutherland, J., Weiner 3rd, J., Ota, M. O., Shankar, S., et al. (2018). Four-gene pan-African blood signature predicts progression to tuberculosis. Am. J. Respir. Crit. Care Med. 197, 1198–1208. doi: 10.1164/rccm.201711-2340OC
Suzuki, Y., Miwa, S., Akamatsu, T., Suzuki, M., Fujie, M., Nakamura, Y., et al. (2013). Indoleamine 2, 3-dioxygenase in the pathogenesis of tuberculous pleurisy. Int. J. Tuberculosis Lung Dis. 17, 1501–1506. doi: 10.5588/ijtld.13.0082
Suzuki, Y., Suda, T., Asada, K., Miwa, S., Suzuki, M., Fujie, M., et al. (2012). Serum indoleamine 2, 3-dioxygenase activity predicts prognosis of pulmonary tuberculosis. Clin. Vaccine Immunol. 19, 436–442. doi: 10.1128/CVI.05402-11
Takikawa, O. (2005). Biochemical and medical aspects of the indoleamine 2, 3-dioxygenase-initiated L-tryptophan metabolism. Biochem. Biophys. Res. Commun. 338, 12–19. doi: 10.1016/j.bbrc.2005.09.032
Thomas, S. R., Salahifar, H., Mashima, R., Hunt, N. H., Richardson, D. R., and Stocker, R. (2001). Antioxidants inhibit indoleamine 2, 3-dioxygenase in IFN-γ-activated human macrophages: posttranslational regulation by pyrrolidine dithiocarbamate. J. Immunol. 166, 6332–6340. doi: 10.4049/jimmunol.166.10.6332
Tiwari, R. P., Hattikudur, N. S., Bharmal, R. N., Kartikeyan, S., Deshmukh, N. M., and Bisen, P. S. (2007). Modern approaches to a rapid diagnosis of tuberculosis: promises and challenges ahead. Tuberculosis 87, 193–201. doi: 10.1016/j.tube.2006.07.005
Van Laarhoven, A., Dian, S., Aguirre-Gamboa, R., Avila-Pacheco, J., Ricaño-Ponce, I., Ruesen, C., et al. (2018). Cerebral tryptophan metabolism and outcome of tuberculous meningitis: an observational cohort study. Lancet Infect. Dis. 18, 526–535. doi: 10.1016/S1473-3099(18)30053-7
Vazquez-Castellanos, J. F., Serrano-Villar, S., Latorre, A., Artacho, A., Ferrus, M. L., Madrid, N., et al. (2015). Altered metabolism of gut microbiota contributes to chronic immune activation in HIV-infected individuals. Mucosal Immunol. 8, 760–772. doi: 10.1038/mi.2014.107
Wallis, R. S., Kim, P., Cole, S., Hanna, D., Andrade, B. B., Maeurer, M., et al. (2013). Tuberculosis biomarkers discovery: developments, needs, and challenges. Lancet Infect. Dis. 13, 362–372. doi: 10.1016/S1473-3099(13)70034-3
Walzl, G., Mcnerney, R., Du Plessis, N., Bates, M., Mchugh, T. D., Chegou, N. N., et al. (2018). Tuberculosis: advances and challenges in development of new diagnostics and biomarkers. Lancet Infect. Dis. 18, e199–e210. doi: 10.1016/S1473-3099(18)30111-7
Wei, L., Zhu, S., Li, M., Li, F., Wei, F., Liu, J., et al. (2018). High indoleamine 2, 3-dioxygenase is correlated with microvessel density and worse prognosis in breast cancer. Front. Immunol. 9:724. doi: 10.3389/fimmu.2018.00724
Weiner 3rd, J., Parida, S. K., Maertzdorf, J., Black, G. F., Repsilber, D., Telaar, A., et al. (2012). Correction: biomarkers of Inflammation, immunosuppression and stress with active disease are revealed by metabolomic profiling of tuberculosis patients. PloS ONE 7:e14954d6a01d. doi: 10.1371/annotation/b7f554bc-ad78-4745-9cd6-e14954d6a01d
Werner, E. R., Fuchs, D., Hausen, A., Jaeger, H., Reibnegger, G., Werner-Felmayer, G., et al. (1988). Tryptophan degradation in patients infected by human immunodeficiency virus. Biol. Chem. Hoppe-Seyler, 369, 337–340. doi: 10.1515/bchm3.1988.369.1.337
Wiegand, M., Möller, A. A., Schreiber, W., Krieg, J. C., Fuchs, D., Wachter, H., et al. (1991). Nocturnal sleep EEG in patients with HIV infection. Eur. Arch. Psychiatry Clin. Neurosci. 240, 153–158. doi: 10.1007/BF02190756
Williams, A. C., and Dunbar, R. I. (2013). Big brains, meat, tuberculosis, and the nicotinamide switches: co-evolutionary relationships with modern repercussions? Int. J. Tryptophan Res. 6:S12838. doi: 10.4137/IJTR.S12838
Williams, A. C., and Hill, L. J. (2017). Meat and nicotinamide: a causal role in human evolution, history, and demographics. Int. J. Tryptophan Res. 10:1178646917704661. doi: 10.1177/1178646917704661
Xu, H., Oriss, T. B., Fei, M., Henry, A. C., Melgert, B. N., Chen, L., et al. (2008). Indoleamine 2, 3-dioxygenase in lung dendritic cells promotes Th2 responses and allergic inflammation. Proc. Natl. Acad. Sci. U.S.A. 105, 6690–6695. doi: 10.1073/pnas.0708809105
Yamazaki, F., Kuroiwa, T., Takikawa, O., and Kido, R. (1985). Human indolylamine 2, 3-dioxygenase. Its tissue distribution, and characterization of the placental enzyme. Biochem. J. 230, 635–638. doi: 10.1042/bj2300635
Yan, Y., Zhang, G.-X., Gran, B., Fallarino, F., Yu, S., Li, H., et al. (2010). IDO upregulates regulatory T cells via tryptophan catabolite and suppresses encephalitogenic T cell responses in experimental autoimmune encephalomyelitis. J. Immunol. 185, 5953–5961. doi: 10.4049/jimmunol.1001628
Yarbrough, M. L., Briden, K. E., Mitsios, J. V., Weindel, A. L., Terrill, C. M., Hunstad, D. A., et al. (2018). Mass spectrometric measurement of urinary kynurenine-to-tryptophan ratio in children with and without urinary tract infection. Clin. Biochem. 56, 83–88. doi: 10.1016/j.clinbiochem.2018.04.014
Yeung, A. W., Wu, W., Freewan, M., Stocker, R., King, N. J., and Thomas, S. R. (2012). Flavivirus infection induces indoleamine 2, 3-dioxygenase in human monocyte-derived macrophages via tumor necrosis factor and NF-κB. J. Leukoc. Biol. 91, 657–666. doi: 10.1189/jlb.1011532
Zangerle, R., Widner, B., Quirchmair, G., Neurauter, G., Sarcletti, M., and Fuchs, D. (2002). Effective antiretroviral therapy reduces degradation of tryptophan in patients with HIV-1 infection. Clin. Immunol. 104, 242–247. doi: 10.1006/clim.2002.5231
Zhang, W., Zhang, J., Zhang, Z., Guo, Y., Wu, Y., Wang, R., et al. (2019). Overexpression of indoleamine 2, 3-dioxygenase 1 promotes epithelial-mesenchymal transition by activation of the IL-6/STAT3/PD-L1 pathway in bladder cancer. Transl. Oncol. 12, 485–492. doi: 10.1016/j.tranon.2018.11.012
Zhang, Y. J., Reddy, M. C., Ioerger, T. R., Rothchild, A. C., Dartois, V., Schuster, B. M., et al. (2013). Tryptophan biosynthesis protects mycobacteria from CD4 T-cell-mediated killing. Cell 155, 1296–1308. doi: 10.1016/j.cell.2013.10.045
Zhang, Y. J., and Rubin, E. J. (2013). Feast or famine: the host–pathogen battle over amino acids. Cell. Microbiol. 15, 1079–1087. doi: 10.1111/cmi.12140
Zhu, B. T. (2010). Development of selective immune tolerance towards the allogeneic fetus during pregnancy: role of tryptophan catabolites. Int. J. Mol. Med. 25, 831–835. doi: 10.3892/ijmm_00000411
Keywords: IDO, latent TB, active TB, kynurenine/tryptophan ratio, nicotinamide
Citation: Adu-Gyamfi CG, Savulescu D, George JA and Suchard MS (2019) Indoleamine 2, 3-Dioxygenase-Mediated Tryptophan Catabolism: A Leading Star or Supporting Act in the Tuberculosis and HIV Pas-de-Deux? Front. Cell. Infect. Microbiol. 9:372. doi: 10.3389/fcimb.2019.00372
Received: 07 August 2019; Accepted: 14 October 2019;
Published: 29 October 2019.
Edited by:
Chad J. Roy, Tulane University School of Medicine, United StatesReviewed by:
Sergio Rutella, Nottingham Trent University, United KingdomOleh Andrukhov, University Dental Clinic Vienna, Austria
Copyright © 2019 Adu-Gyamfi, Savulescu, George and Suchard. This is an open-access article distributed under the terms of the Creative Commons Attribution License (CC BY). The use, distribution or reproduction in other forums is permitted, provided the original author(s) and the copyright owner(s) are credited and that the original publication in this journal is cited, in accordance with accepted academic practice. No use, distribution or reproduction is permitted which does not comply with these terms.
*Correspondence: Clement Gascua Adu-Gyamfi, Y2xlbWVudGdhc2N1YSYjeDAwMDQwO3lhaG9vLmNvbQ==