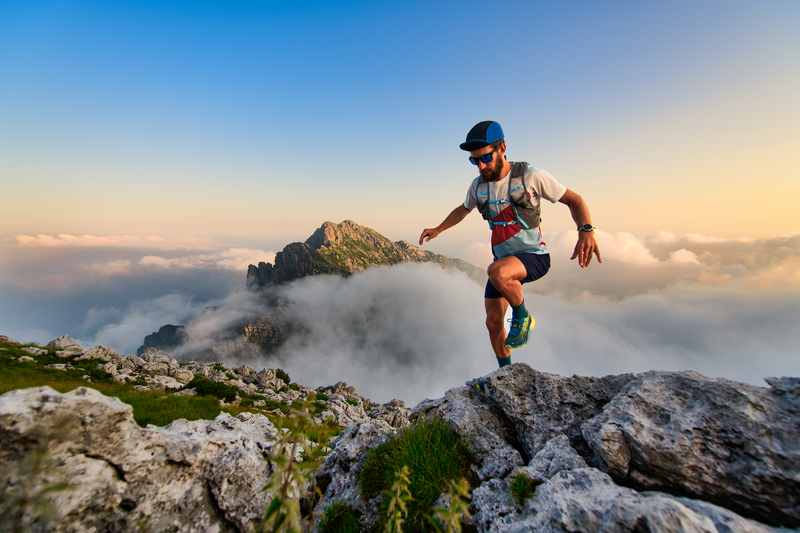
94% of researchers rate our articles as excellent or good
Learn more about the work of our research integrity team to safeguard the quality of each article we publish.
Find out more
HYPOTHESIS AND THEORY article
Front. Cell. Infect. Microbiol. , 21 August 2019
Sec. Bacteria and Host
Volume 9 - 2019 | https://doi.org/10.3389/fcimb.2019.00299
Globally, tuberculosis (TB) has reemerged as a major cause of morbidity and mortality, despite the use of the Mycobacterium bovis BCG vaccine and intensive attempts to improve upon BCG or develop new vaccines. Two lacunae in our understanding of the Mycobacterium tuberculosis (M. tb)-host pathogenesis have mitigated the vaccine efforts; the bacterial-host interaction that enables successful establishment of primary infection and the correlates of protection against TB. The vast majority of vaccine efforts are based on the premise that cell-mediated immunity (CMI) is the predominating mode of protection against TB. However, studies in animal models and in humans demonstrate that post-infection, a period of several weeks precedes the initiation of CMI during which the few inhaled bacteria replicate dramatically and disseminate systemically. The “Trojan Horse” mechanism, wherein M. tb is phagocytosed and transported across the alveolar barrier by infected alveolar macrophages has been long postulated as the sole, primary M. tb:host interaction. In the current review, we present evidence from our studies of transcriptional profiles of M. tb in sputum as it emerges from infectious patients where the bacteria are in a quiescent state, to its adaptations in alveolar epithelial cells where the bacteria transform to a highly replicative and invasive phenotype, to its maintenance of the invasive phenotype in whole blood to the downregulation of invasiveness upon infection of epithelial cells at an extrapulmonary site. Evidence for this alternative mode of infection and dissemination during primary infection is supported by in vivo, in vitro cell-based, and transcriptional studies from multiple investigators in recent years. The proposed alternative mechanism of primary infection and dissemination across the alveolar barrier parallels our understanding of infection and dissemination of other Gram-positive pathogens across their relevant mucosal barriers in that barrier-specific adhesins, toxins, and enzymes synergize to facilitate systemic establishment of infection prior to the emergence of CMI. Further exploration of this M. tb:non-phagocytic cell interaction can provide alternative approaches to vaccine design to prevent infection with M. tb and not only decrease clinical disease but also decrease the overwhelming reservoir of latent TB infection.
Tuberculosis (TB) is emerging as the most important infectious disease of our times, and is the leading cause of morbidity and mortality due to any infectious disease worldwide, surpassing even HIV. In 2015, there were >10 × 106 cases of TB and >1 × 106 TB-related deaths (Murray and Collaborators, 2018). Estimates are that ~ 2 × 109 people, a third of the global population carries a latent TB infection (LTBI). Approximately 10% of these infections will reactivate to progress to infectious clinical TB in their lifetime, thus maintaining transmission in humans. The risk for TB, both primary and reactivated, is higher in immune-compromised individuals; at least a third of HIV-related deaths are attributed to TB.
The current vaccine, Mycobacterium bovis bacilli Calmette-Guerin (BCG), developed in the 1920's, is effective in attenuating severe disseminated forms of TB in children, but not in preventing primary infection or reactivation of LTBI in adults. To improve the efficacy of BCG, approaches such as development of recombinant subunit vaccines, and engineering BCG to improve protection by inserting genes for Mycobacterium tuberculosis (M. tb) antigens, mammalian cytokines, host resistance factors, bacterial toxin-derived adjuvants etc. have been used (Nieuwenhuizen and Kaufmann, 2018). The goal of the vaccine candidates is to induce robust immune responses critical for controlling progression of M. tb infection. Candidate selection is based primarily on their ability to elicit IFN-γ from T cells in vitro and reduce bacterial burden in animal models in vivo. Unfortunately, the correlates of protection against TB are not completely defined, and the immune responses elicited by the candidate vaccines selected so far have failed to correlate with protection in humans.
The most successful anti-TB immune response in humans is the adaptive immunity elicited by natural infection, which prevents ~90–95% of infected individuals from developing active TB. This immune response prevents both progression of primary infection to clinical TB and reactivation of LTBI for long durations. So far, no candidate vaccine has achieved this. Importantly, the naturally elicited adaptive immune responses (a.k.a. cell-mediated immunity, CMI) that successfully maintain latency for the lifetime of ~90% of the infected individuals, fail to eradicate the existing infection and cannot protect against subsequent infections with M. tb (Barrios-Payán et al., 2012; Laal, 2012).
Multiple investigations of TB household-contacts performed for the identification of undiagnosed/sub-clinical cases (Beyanga et al., 2018; Fox et al., 2018; Ohene et al., 2018) as well as to specify the risk factors that influence an exposed person to active disease have been performed (Jones-Lopez et al., 2017; Stein et al., 2018). Meta-analysis of these studies demonstrates that, as measured by conversion to positive tuberculin skin test (TST), despite similar exposure, M. tb infection is established in only ~50% of the contacts (Morrison et al., 2008). Moreover, studies on transmission of TB in households indicate that <20% of TB transmission occurs via household contact, demonstrating that frequent close encounters do not necessarily result in infection (latent or active) (Martinez et al., 2017). It is unclear how contacts who do not convert to TST positivity despite frequent exposure to M. tb prevent the establishment of infection, although studies of genetic susceptibility suggest that TNF-mediated effector mechanisms may influence innate resistance to M. tb infection (Abel et al., 2018).
The precise events that occur during primary infection are poorly understood. There are no external signs and symptoms of TB infection, and it can take up to 8 weeks post-infection (p.i.) for TST reactivity to become positive and for TB-specific IFN-γ producing cells to appear (CDC, 2013). The events that occur in the lungs prior to the onset of these immune responses remain unexplored in humans where neither the time of infection nor the inhaled dose can be ascertained. In animal models, both the time and dose of infection can be controlled, but the paucity of bacterial numbers inhaled and the large pulmonary tissue volume are problematic. Yet this “Black Box” is where the dynamic events that determine the subsequent course of M. tb infection occur.
In this review, we discuss the current understanding of primary infection with M. tb and the missing information regarding the pre-CMI events that lead to establishment of infection. We discuss the features of the alveolar barrier, describe the potential mechanisms for M. tb dissemination across this barrier, and demonstrate that these are parallel to mechanisms used by other bacterial pathogens to cross their pertinent physiological barriers. Using the published transcriptional profiles of M. tb in environments relevant to those encountered during the establishment of infection, we have synthesized a narrative of how the inhaled M. tb adapts to and/or exploits each step in its infection and dissemination journey to its benefit. The focus of this review is the potentially critical role of the alveolar epithelial cell (AEC) both as a permissive niche for M. tb replication and as a portal for systemic M. tb dissemination. These interactions that occur during primary infection can be targeted in novel vaccine strategies to prevent the establishment of M. tb infection.
Only a subset of the bacteria-laden aerosol droplets (<5 μm diameter with 1–3 bacilli) inhaled actually reach any alveolar sac; the larger size droplets (>5 μm) are trapped in the upper respiratory system by mucus and ciliary action (Fernández Tena and Casan Clarà, 2012). Current understanding is that the inhaled bacteria are phagocytosed by alveolar macrophages (AM). Several mechanisms that M. tb employ to subvert being killed and to replicate intracellularly have been described, including inhibition of phagosomal maturation, de-acidification of the phagosomal vacuole, escape from the vacuole to the cytosol, and modulation of macrophage apoptosis (Awuh and Flo, 2016); understandably, almost all these studies have not been performed in AM. In any case, infected macrophages recruit additional circulating monocytes/macrophages and neutrophils to the site of infection via expression of chemokines (CCL2; CXCL10; and TNF) (Jang et al., 2008; Deshmane et al., 2009; Domingo-Gonzalez et al., 2016); and the newly recruited cells in turn phagocytose the bacteria released by lysis of the infected AM. Recent investigations of primary infection in the mouse high-dose aerosol infection model show that AMs are more permissive for M. tb replication than interstitial macrophages (Huang et al., 2018), and that M. tb-infected AM migrate to the lung interstitium where they can be visualized as small aggregates at ~2 weeks p.i. (Cohen et al., 2018); these early cellular foci are postulated to be the precursors to lung granulomas. By ~3 weeks p.i., M. tb are present in recruited monocytes and neutrophils in greater numbers than the initially infected AM (Cohen et al., 2018). The bacteria-laden phagocytic cells transport their cargo via diapedesis across the alveolar barrier to lymph nodes (“Trojan Horse” mechanism) (Nguyen and Pieters, 2005; Wolf et al., 2008) where CMI responses are initiated around 6–8 weeks p.i. in humans (Wallgren, 1948; CDC, 2013). This time point is on the early boundary of CMI and contributes to the containment of M. tb infection as measured by bacterial burden, disease progression, and ability to prevent the reactivation of LTBI (Chen et al., 2009; Lin et al., 2012).
During the first weeks of aerosol infection, dramatic bacterial replication (>20,000-fold) is reported to occur in the lung (Wolf et al., 2008). Older literature reported that M. tb spreads from the site of inoculation to multiple organs within days (Soltys and Jennings, 1950). Additional support for M. tb replication and systemic dissemination from the lungs prior to elicitation of CMI also comes from other studies in animal models. Thus, in aerosol-infected mice, M. tb is detected in the spleens and livers as early as 2 weeks p.i. (Chackerian et al., 2002), and bacteria are detected in spleens of guinea pigs within 3 weeks after a low-dose (3 CFU) aerosol-infection (Mcmurray, 2003). Tubercle lesions with granulomatous inflammation and necrosis appear in the lymph-nodes, spleen, liver, pancreas, adrenal glands and heart at ~4 weeks post—low-dose aerosol-infection with virulent strains of M. tb (Erdman K01, CDC1551, and HN878) (Palanisamy et al., 2008). In humans, evidence for systemic dissemination prior to elicitation of CMI comes from studies of autopsy tissues from individuals who died of unrelated causes in a TB-endemic setting. In these individuals, LTBI was demonstrated in the liver, kidneys, and spleen in the absence of tubercular lesions (Barrios-Payán et al., 2012). Importantly, the extrapulmonary (EP) LTBIs are in multiple non-phagocytic cell types such as Bowman's capsule parietal cells and convoluted proximal tubule epithelial cells in the kidneys, sinusoidal EC and Kupffer cells in the spleen, and hepatocytes and portal biliary duct epithelial cells in the liver. These studies demonstrate the pre-CMI systemic dissemination of M. tb in animals and humans and also highlight the breadth of cell types that M. tb infects and survives in in vivo (Barrios-Payán et al., 2012).
The alveolar barrier is the critical obstacle the inhaled M. tb must traverse to disseminate systemically (Figure 1) (Burns et al., 2003). The alveolar lumen is lined by type 1 and type 2 AECs which rest on a basement membrane (BM) composed primarily of extracellular matrix (ECM) comprised of laminin (Lm), collagen, entactin, heparan sulfate proteoglycans (HSPGs), and other additional minor components. Alveolar sacs are wrapped with alveolar capillaries lined with endothelial cells (EC) also resting on their own BM. The BMs underlying the AEC and EC are fused together in areas where gas exchange occurs (thin wall); in areas not involved in gas exchange, the two BMs are separated by ECM interspersed with interstitial fibroblasts, pericytes etc. (thick wall). The lumen of an average alveolus has ~28,000 thin and large flat type 1 AECs that cover 90–95% of the surface area (Schneeberger, 1991). The primary function of these cells is to facilitate gas exchange. About 5–10% of the alveolar lumen is occupied by ~1,500–2,000 cuboidal type 2 AEC that are responsible for secreting surfactants and restoring damaged type 1 AEC (Schneeberger, 1991). In addition, ~50 AM surveil the alveolus for foreign particles (Crystal, 1991). The lack of a type 1 AEC line has precluded studies of the host-pathogen interaction with these cells. In contrast, M. tb infection of and replication in A549 cells, a human type 2 AEC carcinoma cell-line commonly used as a model for the study of pulmonary diseases, is well-investigated (Mcdonough Kathleen and Kress, 1995; Bermudez and Goodman, 1996, Birkness et al., 1999; Dobos et al., 2000; Bermudez et al., 2002; Castro-Garza et al., 2002; Garcia-Perez et al., 2003; Chapeton-Montes et al., 2008; Ryndak et al., 2015). A549 cells maintain the type 2 AEC characteristics such as multilamellar bodies containing phospholipids similar to primary type 2 AEC and have similar surfactant protein expression (Nardone and Andrews, 1979; Cooper et al., 2016). However, while A549 cells have served as a useful type 2 AEC model across varied pulmonary disease studies (e.g., Gonzalez-Juarbe et al., 2017; Laventie et al., 2019; Nachmias et al., 2019; Nickol et al., 2019), and are capable of self-renewal, this cell line is not capable of differentiating to type 1 AEC, and therefore, stimuli that would trigger type 1 differentiation in vivo would go unnoticed (Fuchs et al., 2003). We have earlier demonstrated that lung fibroblasts (WI-26 cells) are exquisitely susceptible to M. tb-induced lysis; whether they also support M. tb replication is not known (Kinhikar et al., 2010). It may be speculated that, like pulmonary fibroblasts, type 1 AEC may also be very sensitive to M. tb-mediated lysis and could therefore present a site for enhanced traversal across the alveolar barrier.
Figure 1. Color overlay of trans-electron micrograph of the alveolar barrier. Adapted from Burns et al. (2003) with author's permission (Dr. Alan R. Burns) and the permission of Physiological Reviews (American Physiological Society). AL, alveolar lumen; CL, capillary lumen; ECM, extracellular matrix; BM, basement membrane. Blue = Type 2 AEC; Green = Type 1 AEC; Yellow = EC; Red = fibroblast; Orange = pericyte.
The accepted mechanism for M. tb dissemination across the alveolar barrier is that AM phagocytose the inhaled bacteria then traverse the barrier via diapedesis, a.k.a. the “Trojan Horse” mechanism (Nguyen and Pieters, 2005). In the last decade or so, there has been steady accumulation of evidence for a potential role for AEC in primary infection that leads us to propose an additional scenario on events that occur during this “Black Box” of primary infection. Based on our studies and those by multiple investigators discussed here, we propose that, in addition to the “Trojan Horse” mechanism, dramatic replication of M. tb in type 2 AEC and direct migration of free bacteria across the alveolar barrier into the circulation contributes significantly to the systemic dissemination from the lung during primary infection.
To cross the alveolar barrier directly, the few inhaled M. tb would have to invade AEC, replicate intracellularly, damage the BM, invade EC (and perhaps replicate in them), and then exit to the capillary lumen (Figure 2). This is in line with recent studies on mycobacterial penetration of the blood:brain barrier where Mycobacterium marinum has been demonstrated to transmigrate both via infected-macrophages and by a macrophage-independent mechanism (van Leeuwen et al., 2018). Evidence for M. tb infection of type 2 AEC has been demonstrated in humans (Hernandez-Pando et al., 2000; Eum et al., 2010; Barrios-Payán et al., 2012). Indirect evidence for massive M. tb replication in a macrophage-independent host cell in the lungs comes from studies in aerosol-infected mice that reported >20,000-fold replication at 2 weeks p.i. and prior to CMI in host cells that are “non-migrating and non-antigen presenting” (Wolf et al., 2008). While M. tb-uptake by A549 cells is less efficient compared to professional phagocytes, once inside, M. tb replicates ~15–20-fold more in the former cells over the same period of time (Mehta et al., 1996). Mechanisms by which type 2 AEC can directly internalize M. tb have been reported (Scordo et al., 2016), and some discussion on the potential mechanisms for M. tb uptake by AEC is provided later in the current review. Virulent strains of M. tb (M. tb H37Rv, Erdman, and CDC1551) have been shown to cause permeation and necrosis (cytotoxicity) and cell-cell bacterial spread in A549 monolayers (Mcdonough Kathleen and Kress, 1995; Dobos et al., 2000), while M. bovis BCG is attenuated for this phenotype (Dobos et al., 2000). M. tb-mediated cytolytic activity is critically associated with virulence, and lysis of the alveolar epithelium could have a substantial effect on downstream M. tb dissemination (Hsu et al., 2003; Lewis et al., 2003).
Figure 2. Steps in migration of M. tb across the alveolar barrier. 1 = M. tb adheres to, invades, and replicates in AEC; 2 = M. tb penetrates the BM and EC; 3 = M. tb exits the EC to enter the circulation; 4 = M. tb infects EP EC to establish LTBI in EP sites. 5 = “Trojan Horse” mechanism by which M. tb crosses the barrier via infected AM.
M. tb DNA has also been detected in both lung EC and fibroblasts of subjects with LTBI (Hernandez-Pando et al., 2000; Barrios-Payán et al., 2012). EC are reported to be relatively resistant to infection by in vitro-grown M. tb; however, bacteria that have replicated inside A549 cells are reported to be far more invasive for EC (>90-fold at 24 h p.i.) (Bermudez et al., 2002). Together, these studies indicate that during replication in AEC, M. tb acquires increased invasiveness for other non-phagocytic cells.
The strong memory B and T cell response to ESAT-6 by production of IFN-γ in individuals with LTBI is indicative of its in vivo expression during primary infection (Sebina et al., 2014; Pathakumari et al., 2017). Comparative studies identified 16 regions of difference (RD1-16) between the genomes of M. tb and BCG, of which one deletion, termed “RD1,” is absent from all BCG sub-strains used as TB vaccines globally. RD1 is part of a 15-gene locus (ESX-1), which encodes a type 7 secretion system (T7SS) that enables the secretion of several proteins including ESAT-6 and CFP10, which are also encoded in RD1. M. bovis BCG, M. tb ΔRD1 and M. tb Δesat-6 mutants are attenuated for cytolysis of type 2 AEC and macrophages, cell-to-cell spread, pulmonary necrosis, and bacterial dissemination from the lungs in vivo (Mcdonough Kathleen and Kress, 1995; Dobos et al., 2000; Hsu et al., 2003; Lewis et al., 2003; Guinn et al., 2004; Kinhikar et al., 2010). M. tb ΔRD1 is also attenuated for lethality in both SCID and BALB/c mice (Hsu et al., 2003), thus correlating the cytotoxic effect with virulence. ESAT-6 can lyse artificial lipid bilayers (Hsu et al., 2003), liposomes (de Jonge et al., 2007), RBCs (Smith et al., 2008), macrophage phagosomes (Simeone et al., 2012), fibroblasts (Tsai et al., 2009), lung fibroblasts and AEC (Kinhikar et al., 2010). Clearly, ESAT-6-mediated lysis is not restricted to phagosomal membranes. Support for ESAT-6 participation in the dissemination of free M. tb across the alveolar barrier also comes from studies with in vitro bilayer models comprised of monolayers of A549 cells and EC with or without a BM between them (Bermudez et al., 2002; Ryndak et al., 2016). The apical AEC side of these models represents the alveolar lumen, and the basolateral EC side represents the alveolar capillary lumen (entrance to the circulation). In both in vitro models, M. tb migrates across the barrier in the absence of macrophages, and BCG is attenuated for this ability. Furthermore, in the model containing a BM, in addition to BCG, M. tb Δesat-6 and M. tb ΔphoP (impaired for ESAT-6 expression and secretion, respectively) are similarly attenuated for macrophage-free transmigration (Ryndak et al., 2016). These studies collectively demonstrate that ESAT-6 secretion is an important participant in dissemination of M. tb from the lungs. Interestingly, the M. marinum macrophage-independent migration across the blood:brain barrier is also ESX-1-dependent (van Leeuwen et al., 2018), and therefore, the mechanism proposed below is not unprecedented for mycobacterial dissemination across physiological barriers.
Transcriptional analysis has provided insight into how M. tb adapts in hostile environments such as the hypoxia and starvation encountered in the granuloma (Betts et al., 2002; Gupta and Chatterji, 2005; Hudock et al., 2017), and in macrophages (Schnappinger et al., 2003; Fontán et al., 2008). Transcriptome analysis of M. tb infecting mice lungs has also described a three-stage adaptive process from acute infection (aerobic, replicating) to a transitional phase [transition to nitrate respiration, induction of DevR (DosR) regulon] to a dormant state (non-replicative, nitrate respiration) (Shi et al., 2005). Thus, transcriptional analysis provides discrimination of active and latent TB primarily by aerobic vs. alternative pathways of respiration, active vs. non-replication, and suppression vs. induction of the DevR (DosR) regulon.
To gain insight into primary infection by M. tb and its dissemination, we have examined the transcriptional states of M. tb in expectorated sputum from smear positive TB patients (Sharma et al., 2017), bacteria replicating in A549 cells (Ryndak et al., 2015), bacteria replicating ex vivo in whole blood both from HIV- and HIV+ individuals (Ryndak et al., 2014), and since earlier studies showed that the disseminated bacteria reside in non-phagocytic cells in EP sites, M. tb in a human retinal pigment epithelial cell (RPE) line (Rao et al., 2006; Barrios-Payán et al., 2012; Abhishek et al., 2018) (Table 1). The RPE cell-line has earlier been demonstrated to be an accurate functional model for studies of RPE and ocular TB (Ablonczy et al., 2011; La Distia Nora et al., 2018).
Table 1. Summary of M. tb transcriptional profiles in physiologically relevant environments encountered during establishment of infection.
The transcriptome of sputum-derived M. tb revealed a low energy, low replicating, non-aerobic, inert state (Sharma et al., 2017). These results are similar to transcriptional studies which also demonstrated that M. tb in patient sputum is in a persistent, non-replicating state (Garton et al., 2008). Other studies with sputum, broncho-alveolar lavage (BAL) fluid, and cavities from TB patients show that the most abundant cells in the sputum and the BAL are heavily bacteria-laden neutrophils (Eum et al., 2010). This intra-neutrophil localization of the sputum bacteria and their inert state suggests that after exiting the caseum of the cavities, neutrophils transport the bacilli from lesions to the pulmonary airway whereby M. tb undergoes a final adaptation, which enables it to survive in these cells as well as the external environments that may be encountered post-expectoration. Interestingly, M. tb in the sputum upregulate mprA, which encodes a “persistence”-related two-component system response regulator, but not the DevR (DosR) regulon, a hallmark of transition to dormancy/latency. The sputum M. tb also down-regulate esat-6, and genes involved in the synthesis of virulence-associated cell wall components, PDIM/PGLs. We interpret these cumulative observations to indicate a conservation of energy and weaponry not needed until actual entry into the new host and initiation of infection occurs.
When these metabolically inert bacteria are inhaled into the alveolus, they could be phagocytosed by AM and/or taken up by AEC. The transcriptome of M. tb in AM is not defined, but in THP-1 cells (Fontán et al., 2008) and in naïve and INF-γ activated murine bone marrow derived macrophages (mBMM) (Schnappinger et al., 2003), the M. tb transcriptome indicates suppression of replication and aerobic respiration, and dramatic upregulation of stress responses associated with transition to dormancy. Thus, genes for 30 and 50S ribosomal protein subunits (translational machinery) and ATP synthase (energy) are down-regulated, and genes for Universal Stress Proteins (response to hypoxia), starvation-induced genes, and the DevR (DosR) regulon (survival during hypoxia and NO stress reactive dormancy) are upregulated. The transcriptome of M. tb incubated with the lysosomal soluble fraction from activated mBMM further confirms the non-replicative and stressed state of M. tb in the intra-activated-macrophage environment (Lin et al., 2016). Transcripts for esat-6 were either not differentially expressed (in THP-1) (Fontán et al., 2008) or were down-regulated (in activated mBMM) (Schnappinger et al., 2003). Importantly, M. tb infection of primary human AM (hAM) causes them to undergo a metabolic shift to glycolysis leading to increased levels of pro-inflammatory IL-1β and decreased production of the anti-inflammatory IL-10, resulting in enhanced intracellular bacterial killing which could contribute to early clearing/control of M. tb infection in humans (Gleeson et al., 2016). Therefore, the AM may not be the most hospitable environment to promote M. tb infection.
In stark contrast, the transcriptome of M. tb in type 2 AEC offers a scenario in which M. tb perceives an environment permissive for replication, an active metabolic state, and increased virulence (Ryndak et al., 2015). Thus, indicators of enhanced replication (genes for 30 and 50S ribosomal protein subunits, ATP synthase), cell wall remodeling (mycolic acid synthesis), aerobic respiration (genes for NADH-DH1, cytochrome c reductase, and cytochrome c oxidase) and virulence (esat-6) are upregulated. Furthermore, in contrast to in macrophages, in AEC, genes involved in alternative electron transfer and non-aerobic respiration, and genes encoding Universal Stress Proteins and other hypoxia-induced genes such as those of the DevR (DosR) regulon are down-regulated indicating a perception of reduced stress by the intracellular M. tb. Conversely, genes encoding “resuscitation promoting factors” implicated in reactivation from dormancy are upregulated. It is possible that these may also promote the “resuscitation” of the “inert” bacteria expectorated from infectious individuals upon reaching an alveolus in a new host. Another striking difference between the transcriptome of M. tb replicating in AEC vs. macrophages is the upregulation of not only esat-6 but also 5 genes encoding ESAT-6-like proteins; EsxH, EsxJ, EsxK, EsxP, and EsxW, in the former cells. The ESAT-6-like family of proteins is characterized by their small size (~100 amino acids), helix-turn-helix structure, central WXG motif, and flexible N- and C-termini (Pallen, 2002). For ESAT-6, the termini serve as anchors and the helices insert to create the membrane-spanning pore (Ma et al., 2015). Since bacterial pore-forming toxins often belong to families of shared structure (Gouaux et al., 1997; Jedrzejas, 2001; Gilbert, 2010; Heuck et al., 2010), it is possible that, in addition to ESAT-6, other ESAT-6-like family members may also have toxin/pore-forming capabilities, although this remains to be investigated.
Thus, bacteria that have replicated in AEC acquire a virulent phenotype and could traverse the alveolar barrier to enter the circulation for dissemination. While M. tb-uptake by EC is enhanced after passage through AEC, it is not known if M. tb replicates further in EC prior to entering the circulation. The transcriptome of M. tb replicating in whole human blood ex vivo mirrors that of M. tb in AEC in suppression of dormancy (down-regulation of genes within the DevR (DosR) regulon), support for cell wall remodeling (upregulation of genes involved in PDIM/PGL synthesis) and enhanced virulence (upregulation of genes within the ESX-1 locus; including esat-6) (Ryndak et al., 2014). Importantly, these adaptations are more dramatic in whole blood from HIV+ patients, both in numbers of differentially expressed M. tb genes and the degree to which they are differentially expressed. Thus, while 3/48 genes from the DevR (DosR) regulon are downregulated in the HIV- blood, 21/48 are downregulated in the HIV+ blood. Also, 3 genes involved in PDIM/PGL synthesis are upregulated by M. tb in the HIV- blood while 10 are up in the HIV+ blood. Furthermore, while 4 genes of the ESX-1 locus are upregulated by M. tb in HIV- blood (including esat-6), 6 genes are upregulated in the HIV+ patient blood. esat-6 is the most upregulated M. tb gene in the HIV+ blood, and 7 genes encoding ESAT-6-like proteins that are not differentially expressed in the HIV- blood, are upregulated in the HIV+ blood (The function of these ESAT-6-like proteins is not yet known). It is after this hematogenous spread that M. tb would enter the non-phagocytic cells in multiple EP sites.
In contrast to the transcriptome in AEC where M. tb acquires a virulent and invasive phenotype and in blood where this phenotype is maintained, the M. tb transcriptome in RPE cells is that of a “quiet” but not latent state (Abhishek et al., 2018). Thus, while many stress-related genes are downregulated in RPE, these cells are not dramatically permissive for M. tb. While a small number of genes encoding the translational machinery (50 and 30S ribosomal subunits) are upregulated, none of the genes encoding ATP synthase are differentially expressed (compared to 8/8 upregulated in AEC). Several genes within the virulence-associated ESX-1 and ESX-5 T7SS loci are downregulated including esat-6, and the 5 genes encoding ESAT-6-like proteins that are upregulated in both AEC and HIV+ blood, plus an additional 9 in this family, are downregulated. This pattern of transcription indicates that while in AEC the drive is toward dramatic replication, virulence, and dissemination, and the potential for virulence and dissemination is maintained in the blood, but in the EP sites, the goal may be to lie quietly and avoid detection by the immune responses elicited. Detection of LTBI is an important component of intraocular TB diagnostic criteria since it often occurs in the absence of active pulmonary TB or other forms of EPTB. This indicates that the bacteria likely disseminated to the ocular site during primary infection.
Together, the transcriptomic studies of M. tb tell a story of M. tb adaptation from expectoration in sputum to transmission to infection and dissemination to EP sites where bacteria survive without eliciting granuloma formation (Figure 3). M. tb in sputum is an inert bacterium in terms of replication, respiration, and virulence exiting an infectious host, which remains so until it encounters cells within a recipient host alveolus. Phagocytosis by an AM will result in efforts to counter the stresses associated with this cell in order to survive and be carried to the interstitium and/or to the circulation. Alternatively, uptake by an AEC will result in an opportunity to activate and replicate dramatically as well as become more virulent and invasive allowing the bacteria to penetrate the alveolar barrier. Once in the blood circulation, the bacteria will remain active and invasive to establish infection in non-phagocytic cells at multiple EP sites. Once a non-alveolar, non-hostile niche is reached, M. tb lies quietly till the opportune conditions arise. In the blood, the bacteria may also be capable of sensing the immune status of the host (e.g., HIV status), wherein the active, virulent, and invasive adaptations are enhanced. This observation is consistent with the epidemiology associating HIV-co-infection with increased EPTB (Shafer et al., 1991; Onorato and Mccray, 1992; Yang et al., 2004; Golden and Vikram, 2005; Naing et al., 2013).
Figure 3. Proposed M. tb adaptational phenotypes in sequential environments during the course of primary infection (based upon published transcriptomic studies).
To better understand the establishment of M. tb infection, we can examine mechanisms used by other bacterial pathogens that have to cross a mucosal barrier to establish infection. The molecular mechanisms for adherence, invasion, and dissemination employed by other pathogens, e.g., Streptococci, Staphylococci, Listeria, Candida (and many more), that also translocate across mucosal barriers to establish infection have been investigated more thoroughly (Jedrzejas, 2001; Ferry et al., 2005; Bonazzi et al., 2009; Nobbs et al., 2009; Chen et al., 2010; Jensch et al., 2010; Krishnan and Narayana, 2011; Vengadesan and Narayana, 2011). The common principle that has emerged is that these pathogens use repertoires of adhesins, toxins and extracellular enzymes to establish infection. Adhesins are cell-surface or secreted proteins expressed by pathogens to attach and invade the epithelial and/or EC of the mucosal barriers by binding to receptors on their cell-membranes or to ECM proteins, e.g., Lm, fibronectin, collagens 1–4, fibrinogen, elastin, thrombospondin, vitronectin, E-cadherin, plasminogen, etc. Toxins participate by causing damage to cells to expose the underlying ECM for bacterial adhesins and increase permeabilization of the mucosal barrier for bacterial (and inflammatory cell) traversal (Jedrzejas, 2001; Ferry et al., 2005). Extracellular enzymes contribute by acting as adhesins, and by causing cell-membrane changes (Pancholi and Chhatwal, 2003). Thus, S. pneumoniae uses, cbpA (choline binding protein A; adhesin), pspA (pneumococcal surface protein A; adhesin), and Ply (pneumolysin; toxin) for infection and dissemination from the lungs (Jedrzejas, 2001; Sanchez et al., 2011). L. monocytogenes expresses several adhesins (FbpA, ActA, InlA, and InlB) that promote attachment and invasion of the intestinal barrier (Suárez et al., 2001; Dramsi et al., 2004; Bierne et al., 2007) and Listeriolysin O (LLO) which is a toxin that impairs the integrity of the intestinal barrier (Richter et al., 2009). Similarly, S. aureus uses FnBP (Fn binding protein) for adhesion to the respiratory epithelium, Spa (S. aureus protein A) to activate inflammatory responses, and α-hemolysin to damage the alveolar barrier to promote hematogenous dissemination (Ferry et al., 2005; Soong et al., 2011). Extracellular enzymes such as the Streptococcal Surface Enolase (SE) and glyceradehyde-3-phosphate degydrogenase (GAPDH) have evolved to also function as adhesins in some pathogens (Li et al., 2015). The McaP of Moraxella catarrhalis is a lipase/adhesin (Timpe et al., 2003); the Ssp of Staphylococcus saprophytycus is a surface-associated lipase that contributes to virulence (Sakinc et al., 2005, Szabados et al., 2013). The Als family of adhesins in Candida albicans are multifunctional family of proteins (Hoyer and Cota, 2016). Adhesins, toxins, and enzymes also modulate inflammatory responses to support establishment of infection; thus, the Vn-binding extracellular adherence protein (Eap) of S. aureus inhibits neutrophil recruitment in mice (Chavakis et al., 2002) as does the SLS (Streptolysin S) of S. pyogenes (Lin et al., 2009) and β-hemolysin of S. aureus (Tajima et al., 2009). Modifications of the inflammatory responses could enhance and optimize the window of time required by the pathogen to avoid/reduce elimination by other innate mechanisms like killing by neutrophils.
As in other bacteria, in addition to cytotoxins, adhesins play a role in the establishment of M. tb infection. Rv0475 (HBHA) is a well-characterized adhesin of M. tb that binds to HSPG in the cell membrane of AEC and in the BM underlying AECs (Menozzi et al., 1996). M. tb Δhbha is attenuated (~50% reduction compared to wild type) for adherence and invasion of AEC, but not of macrophages, and for dissemination from the lungs to spleen in mice (Pethe et al., 2001). Transcripts for hbha are upregulated during infection of AEC but not during infection of macrophages (Delogu et al., 2006) or RPE cells (Abhishek et al., 2018). Thus, HBHA contributes to direct dissemination of M. tb, independent of macrophages, and specifically from the lungs. Another M. tb adhesin which is CNS-specific is, Rv0931c (PknD) (Be et al., 2012). PknD is a Lm-binding adhesin that contributes to M. tb attachment and invasion of human brain microvascular EC but not to AEC or macrophages or non-CNS EC (Be et al., 2012). pknD is not differentially expressed in AEC or macrophages or RPE (Schnappinger et al., 2003; Fontán et al., 2008; Ryndak et al., 2015; Abhishek et al., 2018).
Besides functioning as a cytotoxin, ESAT-6 is also an adhesin that binds to Lm and cell-membranes of AEC (Kinhikar et al., 2010). Since in vivo, Lm is primarily concentrated at the basolateral surface of AEC and is a major component of the BM, ESAT-6 could contribute to the anchoring of intra-AEC M. tb onto the basolateral Lm-expressing BM and cause damage to the cells and the BM by pore formation, thus facilitating macrophage-independent M. tb dissemination via the alveolar wall. This is similar to the Mip adhesin/toxin of Legionella which binds collagens (i, ii, iii, iv, v, vi), lyses lung epithelial cells, and damages the BM to achieve dissemination (Wagner et al., 2007).
Unlike in other mycobacteria, Rv1837c (Malate synthase; MS, GlcB) of M. tb is expressed extracellularly, binds Lm, and serves as an adhesin that contributes to the attachment of M. tb to AEC (Kinhikar et al., 2006). The M. tb MS has a C-terminal 15 amino acid extended sequence that is absent in MS of M. avium, M. leprae or M. smegmatis. A peptide spanning this C-terminal region of M. tb MS shows binding to Lm, fibronectin, and AEC (Kinhikar et al., 2006). Like HBHA and ESAT-6, transcripts for MS are upregulated during M. tb replication in AEC (Ryndak et al., 2015). None of these adhesins are upregulated by M. tb in either the THP-1, mBMM, or RPE (Schnappinger et al., 2003; Fontán et al., 2008; Abhishek et al., 2018).
As for other bacteria, evidence for synergy between adhesins and toxins in infection/dissemination is also available for M. tb. In the complete in vitro bilayer model of the alveolar barrier where AEC and EC are separated by a BM, M. bovis BCG Δhbha, which lacks both ESAT-6 (adhesin/toxin for AEC) and HBHA (adhesin for AEC), is even more attenuated than the single esat-6 deficient mutants (an additional ~50%) for migration across the in vitro alveolar barrier (Ryndak et al., 2016).
Besides the adhesin:ECM interaction, evidence for AEC uptake of M. tb by receptor-mediated macropinocytosis involving actin-polymerization and signal transduction is now described (Scordo et al., 2016). While this mechanism is certainly less efficient than phagocytosis by AM, the far higher prevalence of AEC in the alveolus coupled to a highly permissive intracellular environment could have a profoundly significant effect during primary infection. Recently, Syndecan 4 (Sdc4) in AEC membranes has been demonstrated to be a receptor for HBHA (Zimmermann et al., 2016). The extracellular domain of Sdc4 contains heparan sulfate chains consistent with previous studies demonstrating HBHA binding to AEC depends on host cell surface heparan sulfate chains (Pethe et al., 2000). Consistent with the ~50% decrease in translocation of BCG Δhbha across an in vitro model alveolar barrier (Ryndak et al., 2016), Sdc4 knock-out AEC are impaired for M. bovis BCG uptake by about 50% (Zimmermann et al., 2016).
Bacterial uptake by non-phagocytic cells such as epithelial cells, EC, and fibroblasts can also occur by bacterial targeting of lipid rafts on the host cell membrane. Lipid rafts are isolated, tightly packed regions of eukaryotic cell membranes which are enriched with cholesterol and glycosphingolipids, and may localize certain cell signaling molecules/receptors (Pike, 2003). Bacteria, such as Pseudomonas aeruginosa, Chlamydia spp., Shigella flexneri, Salmonella typhimurium, E. coli K1, and Coxiella burnetii, target lipid rafts in host cell membranes which concentrate/localize receptors and/or key host signaling molecules to facilitate entry (Lafont et al., 2002, Zaas et al., 2005; Lim et al., 2010; Toledo and Benach, 2015; Loh et al., 2017; Samanta et al., 2017). M. tb has been shown to induce lipid raft aggregation in the membranes of AEC, and this capability is associated with its internalization (Fine-Coulson et al., 2012). Thus, in vitro M. tb infection of type 2 AEC triggered the formation of lipid raft aggregates in the cell membranes, and at 24 h p.i. ~50% M. tb was observed to co-localize with lipid raft markers. Furthermore, disruption of lipid rafts by pretreating the AEC with Filipin significantly reduced the numbers of intracellular bacteria suggesting a role for lipid raft targeting and aggregation in M. tb internalization by type 2 AEC. Whether it is M. tb adhesin:lipid raft interactions that promote uptake by AEC (or other non-phagocytic cells), either directly or through co-localized receptors, remains to be investigated; however, adhesins of other bacterial pathogens have been shown to mediate their uptake by non-phagocytic cells through lipid raft-dependent mechanisms including Chlamydia pneumoniae (Fechtner et al., 2016), Streptococcus pneumonia (Yamaguchi et al., 2013), and Shiga-toxigenic E. coli (Rogers et al., 2012).
Bacterial pore-forming toxins can also engage host cell lipid rafts (Toledo and Benach, 2015). Cholera toxin activity and internalization into epithelial cells are inhibited when the cells are pretreated with Filipin, thus indicating lipid raft involvement (Orlandi and Fishman, 1998). Furthermore, the pore-forming toxin LLO, triggers aggregation of lipid rafts in macrophage membranes (Gekara and Weiss, 2004; Gekara et al., 2005), and LLO pore-forming activity has been linked to L. monocytogenes uptake by host cells (Vadia et al., 2011). Culture filtrate proteins from M. tb H37Rv and M. tb HN878 induce significantly higher numbers of lipid raft aggregates on AEC compared to untreated cells (~30–40 aggregates per cell compared to ~5–10 aggregates per cell) and infection of AEC with M. tb pretreated with amikacin to prevent bacterial protein synthesis did not result in any increase in lipid raft aggregates (Fine-Coulson et al., 2012) indicating secreted factor(s) of M. tb contribute to the formation of lipid raft aggregates and possibly M. tb internalization as well by AEC.
The scenario described in this review suggests that likely targets for the prevention of M. tb infection would be the adhesins, toxin(s), and extracellular enzymes that promote primary infection and dissemination through their interactions with AEC. Thus, vaccination that leads to the attenuation of the interactions of HBHA with AEC, for example, has been demonstrated. Pre-incubation of wild type M. tb with anti-HBHA antibodies attenuates the dissemination of the intra-nasally administered bacteria from the lung to spleen indicating a non-macrophage and pro-AEC mechanism for dissemination during primary infection (Pethe et al., 2001). Similarly, mucosal immunization with HBHA reduces dissemination of M. Bovis BCG from the lungs in mice, and this reduction correlates with elicitation of anti-HBHA antibodies (Kohama et al., 2008). Antibodies against the CNS-specific adhesin, PknD, reduce bacterial invasion of human brain microvascular EC (Skerry et al., 2013), and guinea pigs vaccinated with PknD are protected against M. tb dissemination to the brain but not to pulmonary bacterial burden, and this protection correlated with the generation of PknD-specific antibodies (Skerry et al., 2013). PknD, therefore, is another illustration of adhesin:target specificity in M. tb. Also, antibodies against the Lm-binding domain of MS reduce M. tb adherence to AEC (Kinhikar et al., 2006). Interestingly, TB patients have high titers of antibodies to MS, but not to the Lm-binding site (unpublished data). In vivo studies of MS as a potential vaccine component have yet to be performed.
Evidence for the substantial promise of anti-adhesin/toxin/enzyme antibody-based vaccines is becoming available (Raynes et al., 2018; Solanki et al., 2018). For example, a recent study demonstrated that the intraperitoneal co-administration into mice of two Enterotoxigenic E. coli (ETEC) multi-Ag fusions, one representing two mutated ETEC toxins and the other representing the epitopes of 7 important ETEC adhesins, induced antibody responses to both the toxins and adhesins, which neutralize bacterial enterotoxicity and adherence to the human intestinal cell line, Caco-2 (Duan et al., 2018). Antibodies targeted to these toxins were also found to be present in both the serum and colostrum of intra-muscularly immunized pigs (Nandre et al., 2018), and suckling pigs born to mothers immunized during pregnancy were passively protected from ETEC-induced diarrhea. Importantly, a human Phase 1 trial in which volunteers were orally administered bovine colostral IgG antibodies to the ETEC fimbrial tip adhesin CFA/1 pilin sub-unit CfaE (or placebo) and subsequently challenged with ETEC and monitored for diarrhea, demonstrated that passive vaccination with antibodies directed against an adhesin can yield significant, protective efficacy in humans (Savarino et al., 2017).
Targeting adhesin interactions with cells of the alveolar barrier has also shown potential as a vaccine strategy in the case of Streptococcus pneumoniae (Mizrachi Nebenzahl et al., 2016). S. pneumoniae, the cause of pneumococcal disease, is spread through the inhalation of contaminated aerosols from infected individuals. PtsA is a S. pneumoniae protein that has a cytosolic enzymatic function and a cell wall adhesin function (Mizrachi Nebenzahl et al., 2016). Pre-incubation of A549 cells with recombinant PtsA (rPtsA) and pre-incubation of bacteria with anti-rPtsA antiserum inhibited the adhesion of S. pneumoniae to these cells. Similarly, pre-incubation of the bacteria with host cell protein peptides determined to bind PtsA inhibited bacterial colonization of lungs in mice. Importantly, immunization of susceptible mice with rPtsA protected the animals against intravenous lethal dose challenge with S. pneumoniae.
The mechanisms of these vaccine strategies are to disrupt the initial adherence or barrier-penetrating steps critical for the establishment of the bacterial infection. Here, we have highlighted the adhesin, HBHA, and the adhesin/lysin, ESAT-6, as vaccine targets to prevent M. tb penetration of the alveolar barrier; however, other M. tb proteins with adhesin or lytic properties may also be involved, e.g., possibly MS and the ESAT-6-like proteins whose encoding genes were upregulated in AEC. Also, bacteria:host cell adhesion is described as a multi-step process, with increasing specificities of interaction (Krachler and Orth, 2013). It is conceivable, therefore, to devise an anti-M. tb-infection vaccine based on the generation of neutralizing antibody responses against combinations of M. tb adhesins/toxins and/or their functional regions that prevent attachment/invasion of the cells in the lung that support replication, dissemination, and active disease, i.e., the AEC. Such a vaccination strategy could succeed in decreasing the overwhelming reservoir of LTBI.
TB remains a major cause of morbidity and mortality in a large proportion of our world. Our understanding of the host/pathogen factors and interactions that lead to dissemination and establishment of M. tb primary infection, however, is limited. These enigmatic steps in M. tb infection, combined with our incomplete knowledge of the true correlates of protection against M. tb infection in humans, have hindered our ability to develop strategies to prevent infection.
Once M. tb infection is established, many challenges remain to obtain accurate diagnosis and proper treatment. Notably, TB infection, after established, behaves differently in different individuals, can elude diagnosis and, if drug resistant, confound treatment. For example, the observable pathologies can depend on the immune competence of the infected individual, e.g., HIV-coinfected individuals have a higher propensity for EP/disseminated TB disease; however, lung lesions identifiable through chest X-ray may not be present and the TST may read as negative due to an impaired immune system. These issues present significant obstacles in identifying and controlling TB. Regardless of the manifestation in the infected host, for essentially all infected individuals, M. tb was acquired by inhaling contaminated aerosol droplets expelled from a person with active pulmonary TB thus bringing the common critical interaction back to the alveolus. Indirect support for the role of AEC is derived from studies of M. tb strains infecting individuals over a 5 year period in Arkansas, with patient demographics taken into consideration, which found that individuals infected with strains of the Beijing/W Lineage were 3-times more likely to have EP involvement even after controlling for patient-associated risk factors for EPTB (Kong et al., 2007). Interestingly, isolates of the highly successful M. tb strains Beijing and F15/LAM4/KZN adhere to and invade A549 cells 5 times more efficiently than other unique isolates of M. tb (Ashiru et al., 2010). There is likely a highly dynamic interplay between the consequences of M. tb encounters with the AM and AEC during primary infection. Together, these observations suggest that adaptation to the intra-AEC environment drives a more invasive and disseminative phenotype in M. tb.
Targeting of adhesins and toxins involved in the primary establishment of infection as a vaccine strategy would involve the humoral immune response. Whether it is antibodies that neutralize/attenuate adhesion/invasion of M. tb to AEC that contribute to the prevention of infection in about half of individuals with frequent and close contact with infectious TB patients remains to be investigated. Interestingly, while ESAT-6 is considered to be a promising vaccine component, the vaccine formulations, strategy, and evaluation has been designed to exploit its ability to elicit strong IFN-γ responses (van Dissel et al., 2010; Kwon et al., 2018). HBHA has been shown to induce a cytolytic CD4+ T cell subset in persons with LTBI but not active TB, suggesting a role for HBHA in the control of M. tb infection (Aerts et al., 2019), and both HBHA-induced IFN-γ responses and anti-HBHA IgA were found to be elevated in household contacts and community controls compared to untreated TB patients in TB-endemic Addis-Ababa, Ethiopia, suggesting HBHA can confer both protective cell-mediated and humoral immune responses (Belay et al., 2016). While the exact mechanisms of most vaccines are unknown, several successful vaccines have been designed to use the humoral immune response as their mode of protection, e.g., polio, tetanus, diphtheria and pertussis, hepatitis A, and MMRV vaccines. Like BCG, the live, attenuated varicella zoster vaccine relies on the CMI response as its correlate of protection (Siegrist, 2013); however, varicella zoster live has the drawback of waning efficacy (Levin et al., 2008). The newly available, Shingrix vaccine, a non-live recombinant Ag vaccine against varicella zoster, which, in addition to CMI, elicits a strong humoral response, has a more persistent efficacy than the varicella zoster live vaccine (Syed, 2018). TB generates a fine balance between humoral and CMI responses in its host, where the initial humoral responses wane as the CMI responses emerge (David, 1990). By the time a TB patient is identified, the CMI response dominates. Recent studies have reported that there are distinct differences between the humoral profiles of latently infected individuals and active TB patients that go beyond the role as mere biomarkers of disease state and indicate antibody function in controlling M. tb infection (Lu et al., 2016). Thus, antibodies from persons with LTBI were shown to be associated with increased antibody-mediated phagocytosis and cytotoxicity, increased Fc binding to the activating receptor Fcγ-RIIIa, and glycosylation structures that distinguish them from antibodies from active TB patients. Furthermore, the effect of treating primary human monocyte-derived macrophages infected with M. tb with pooled antibodies from each group (active or latent) revealed increased M. tb:lysosome co-localization, increased inflammasome activation, and decreased intracellular bacterial survival in the macrophages treated with LTBI-derived antibodies compared to those treated with active TB-associated antibodies. Therefore, antibodies can play a role in the control of M. tb infection, and we postulate that enhanced and targeted humoral immune responses to the critical mechanisms of primary infection, such as the M. tb interactions with AEC, could be exploited as a robust and novel vaccine strategy to prevent TB.
In this review, we have discussed an emerging aspect of the M. tb:host interaction that may contribute significantly to primary infection with M. tb; that interaction is with the AEC. The findings described in this review are consistent with a scenario involving AEC that is independent of macrophages and explains how M. tb replication, dissemination, and establishment of infection can outpace the CMI response. This scenario does not preclude a role for the AM in M. tb infection, but opens a whole, new way of understanding primary infection with M. tb that should be investigated in order to develop new strategies for preventing M. tb infection. Interrupting this interaction could prove crucial to averting the downstream obstacles/factors that drive TB infection, latent or active.
Based on the cumulative studies highlighted here, we suggest an explanation of the “Black Box” events of early M. tb infection during which the few, inhaled M. tb outpace the generation of adaptive immune responses to replicate and establish systemic infection. Upon entry they may invade AEC, rapidly replicate in this safe niche, and spread to neighboring cells, simultaneously fortifying themselves with the armamentarium required to migrate across the alveolar wall, spread via the circulation to various organs, and seed non-phagocytic cells, where they lie dormant, till the opportunity to reactivate arises. Therefore, we propose that the M. tb:AEC encounter may be critical to the establishment of primary infection in a naïve host and needs the focus of the scientific community to develop strategies for the prevention of M. tb infection.
SL: concept and critical revision. MR: writing and final approval. MR and SL: agreement to be accountable.
All research presented and discussed here is published, and corresponding funding can be found in the references cited. The Fogarty International Institute (FIC), National Institutes of Health (NIH) grant D43TW009588 awarded to SL covers the open access publication fees of this manuscript.
The authors declare that the research was conducted in the absence of any commercial or financial relationships that could be construed as a potential conflict of interest.
Abel, L., Fellay, J., Haas, D. W., Schurr, E., Srikrishna, G., Urbanowski, M., et al. (2018). Genetics of human susceptibility to active and latent tuberculosis: present knowledge and future perspectives. Lancet Infect. Dis. 18, e64–e75. doi: 10.1016/S1473-3099(17)30623-0
Abhishek, S., Saikia, U. N., Gupta, A., Bansal, R., Gupta, V., Singh, N., et al. (2018). Transcriptional profile of Mycobacterium tuberculosis in an in vitro model of intraocular tuberculosis. Front. Cell. Infect. Microbiol. 8:330. doi: 10.3389/fcimb.2018.00330
Ablonczy, Z., Dahrouj, M., Tang, P. H., Liu, Y., Sambamurti, K., Marmorstein, A. D., et al. (2011). Human retinal pigment epithelium cells as functional models for the RPE in vivo. Invest. Ophthalmol. Vis. Sci. 52, 8614–8620. doi: 10.1167/iovs.11-8021
Aerts, L., Selis, E., Corbière, V., Smits, K., Van Praet, A., Dauby, N., et al. (2019). HBHA-induced polycytotoxic CD4(+) T lymphocytes are associated with the control of Mycobacterium tuberculosis infection in humans. J. Immunol. 202, 421–427. doi: 10.4049/jimmunol.1800840
Ashiru, O. T., Pillay, M., and Sturm, A. W. (2010). Adhesion to and invasion of pulmonary epithelial cells by the F15/LAM4/KZN and Beijing strains of Mycobacterium tuberculosis. J. Med. Microbiol. 59, 528–533. doi: 10.1099/jmm.0.016006-0
Awuh, J. A., and Flo, T. H. (2016). Molecular basis of mycobacterial survival in macrophages. Cell. Mol. Life Sci. 74, 1625–1648. doi: 10.1007/s00018-016-2422-8
Barrios-Payán, J., Saqui-Salces, M., Jeyanathan, M., Alcántara-Vazquez, A., Castañon-Arreola, M., Rook, G., et al. (2012). Extrapulmonary locations of Mycobacterium tuberculosis DNA during latent infection. J. Infect. Dis. 206, 1194–1205. doi: 10.1093/infdis/jis381
Be, N. A., Bishai, W. R., and Jain, S. K. (2012). Role of Mycobacterium tuberculosis pknD in the pathogenesis of central nervous system tuberculosis. BMC Microbiol. 12:7. doi: 10.1186/1471-2180-12-7
Belay, M., Legesse, M., Mihret, A., Ottenhoff, T. H., Franken, K. L., Bjune, G., et al. (2016). IFN-gamma and IgA against non-methylated heparin-binding hemagglutinin as markers of protective immunity and latent tuberculosis: results of a longitudinal study from an endemic setting. J. Infect. 72, 189–200. doi: 10.1016/j.jinf.2015.09.040
Bermudez, L. E., and Goodman, J. (1996). Mycobacterium tuberculosis Invades and Replicates within Type 2 Alveolar Cells. Infect. Immun. 64, 1400–1406.
Bermudez, L. E., Sangari, F. J., Kolonoski, P., Petrofsky, M., and Goodman, J. (2002). The efficiency of the translocation of Mycobacterium tuberculosis across a bilayer of epithelial and endothelial cells as a model of the alveolar wall is a consequence of transport within mononuclear phagocytes and invasion of alveolar epithelial cells. Infect. Immun. 70, 140–146. doi: 10.1128/IAI.70.1.140-146.2002
Betts, J. C., Lukey, P. T., Robb, L. C., Mcadam, R. A., and Duncan, K. (2002). Evaluation of a nutrient starvation model of Mycobacterium tuberculosis persistence by gene and protein expression profiling. Mol. Microbiol. 43, 717–731. doi: 10.1046/j.1365-2958.2002.02779.x
Beyanga, M., Kidenya, B. R., Gerwing-Adima, L., Ochodo, E., Mshana, S. E., and Kasang, C. (2018). Investigation of household contacts of pulmonary tuberculosis patients increases case detection in Mwanza City, Tanzania. BMC Infect. Dis. 18:110. doi: 10.1186/s12879-018-3036-6
Bierne, H., Sabet, C., Personnic, N., and Cossart, P. (2007). Internalins: a complex family of leucine-rich repeat-containing proteins in Listeria monocytogenes. Microbes Infect. 9, 1156–1166. doi: 10.1016/j.micinf.2007.05.003
Birkness, K. A., Deslauriers, M., Bartlett, J. H., White, E. H., King, C. H., and Quinn, F. D. (1999). An In vitro tissue culture bilayer model to examine early events in Mycobacterium tuberculosis Infection. Infect. Immun. 67, 653–658.
Bonazzi, M., Lecuit, M., and Cossart, P. (2009). Listeria monocytogenes internalin and E-cadherin: from bench to bedside. Cold Spring Harb. Perspect. Biol. 1:a003087. doi: 10.1101/cshperspect.a003087
Burns, A. R., Smith, C. W., and Walker, D. C. (2003). Unique structural features that influence neutrophil emigration into the lung. Physiol. Rev. 83, 309–336. doi: 10.1152/physrev.00023.2002
Castro-Garza, J., King, C. H., Swords, W. E., and Quinn, F. D. (2002). Demonstration of spread by Mycobacterium tuberculosis bacilli in A549 epithelial cell monolayers. FEMS Microbiol. Lett. 212, 145–149. doi: 10.1111/j.1574-6968.2002.tb11258.x
CDC (2013). “Chapter 3: testing for tuberculosis infection and disease,” in Core Curriculum on Tuberculosis: What the Clinician Should Know, 6th Edn. Available online at: https://www.cdc.gov/tb/education/corecurr/pdf/corecurr_all.pdf
Chackerian, A. A., Alt, J. M., Perera, T. V., Dascher, C. C., and Behar, S. M. (2002). Dissemination of Mycobacterium tuberculosis is influenced by host factors and precedes the initiation of T-cell immunity. Infect. Immun. 70, 4501–4509. doi: 10.1128/IAI.70.8.4501-4509.2002
Chapeton-Montes, J. A., Plaza, D. F., Barrero, C. A., and Patarroyo, M. A. (2008). Quantitative flow cytometric monitoring of invasion of epithelial cells by Mycobacterium tuberculosis. Front. Biosci. 13, 650–656. doi: 10.2741/2709
Chavakis, T., Hussain, M., Kanse, S. M., Peters, G., Bretzel, R. G., Flock, J. I., et al. (2002). Staphylococcus aureus extracellular adherence protein serves as anti-inflammatory factor by inhibiting the recruitment of host leukocytes. Nat. Med. 8, 687–693. doi: 10.1038/nm728
Chen, C. Y., Huang, D., Wang, R. C., Shen, L., Zeng, G., Yao, S., et al. (2009). A critical role for CD8 T cells in a nonhuman primate model of tuberculosis. PLoS Pathog. 5:e1000392. doi: 10.1371/journal.ppat.1000392
Chen, S. M., Tsai, Y. S., Wu, C. M., Liao, S. K., Wu, L. C., Chang, C. S., et al. (2010). Streptococcal collagen-like surface protein 1 promotes adhesion to the respiratory epithelial cell. BMC Microbiol. 10:320. doi: 10.1186/1471-2180-10-320
Cohen, S. B., Gern, B. H., Delahaye, J. L., Adams, K. N., Plumlee, C. R., Winkler, J. K., et al. (2018). Alveolar macrophages provide an early Mycobacterium tuberculosis niche and initiate dissemination. Cell Host Microbe 24, 439–446 e4. doi: 10.1016/j.chom.2018.08.001
Cooper, J. R., Abdullatif, M. B., Burnett, E. C., Kempsell, K. E., Conforti, F., Tolley, H., et al. (2016). Long term culture of the A549 cancer cell line promotes multilamellar body formation and differentiation towards an alveolar type II pneumocyte phenotype. PLoS ONE 11:e0164438. doi: 10.1371/journal.pone.0164438
Crystal, R. J. (1991). “Alveolar macrophages,” in The Lung, eds R. J. Crystal and J. B. West (New York, NY: Raven Press, Scientific Foundations), 527–538.
David, H. L. (1990). The spectrum of tuberculosis and leprosy: what can be the significance of specific humoral responses? Res Microbiol. 141, 197–206. doi: 10.1016/0923-2508(90)90031-K
de Jonge, M. I., Pehau-Arnaudet, G., Fretz, M. M., Romain, F., Bottai, D., Brodin, P., et al. (2007). ESAT-6 from Mycobacterium tuberculosis dissociates from its putative chaperone CFP-10 under acidic conditions and exhibits membrane-lysing activity. J. Bacteriol. 189, 6028–6034. doi: 10.1128/JB.00469-07
Delogu, G., Sanguinetti, M., Posteraro, B., Rocca, S., Zanetti, S., and Fadda, G. (2006). The hbhA gene of Mycobacterium tuberculosis is specifically upregulated in the lungs but not in the spleens of aerogenically infected mice. Infect. Immun. 74, 3006–3011. doi: 10.1128/IAI.74.5.3006-3011.2006
Deshmane, S. L., Kremlev, S., Amini, S., and Sawaya, B. E. (2009). Monocyte chemoattractant protein-1 (MCP-1): an overview. J. Interferon Cytokine Res. 29, 313–326. doi: 10.1089/jir.2008.0027
Dobos, K. M., Spotts, E. A., Quinn, F. D., and King, C. H. (2000). Necrosis of lung epithelial cells during infection with Mycobacterium tuberculosis is preceded by cell permeation. Infect. Immun. 68, 6300–6310. doi: 10.1128/IAI.68.11.6300-6310.2000
Domingo-Gonzalez, R., Prince, O., Cooper, A., and Khader, S. A. (2016). Cytokines and chemokines in Mycobacterium tuberculosis infection. Microbiol Spectr. 4, 33–72. doi: 10.1128/microbiolspec.TBTB2-0018-201
Dramsi, S., Bourdichon, F., Cabanes, D., Lecuit, M., Fsihi, H., and Cossart, P. (2004). FbpA, a novel multifunctional Listeria monocytogenes virulence factor. Mol. Microbiol. 53, 639–649. doi: 10.1111/j.1365-2958.2004.04138.x
Duan, Q., Lu, T., Garcia, C., Yañez, C., Nandre, R. M., Sack, D. A., et al. (2018). Co-administered tag-less toxoid fusion 3xSTaN12S-mnLTR192G/L211A and CFA/I/II/IV MEFA (multiepitope fusion antigen) induce neutralizing antibodies to 7 adhesins (CFA/I, CS1-CS6) and both enterotoxins (LT, STa) of enterotoxigenic Escherichia coli (ETEC). Front. Microbiol. 9:1198. doi: 10.3389/fmicb.2018.01198
Eum, S. Y., Kong, J. H., Hong, M. S., Lee, Y. J., Kim, J. H., Hwang, S. H., et al. (2010). Neutrophils are the predominant infected phagocytic cells in the airways of patients with active pulmonary TB. Chest 137, 122–128. doi: 10.1378/chest.09-0903
Fechtner, T., Galle, J. N., and Hegemann, J. H. (2016). The novel chlamydial adhesin CPn0473 mediates the lipid raft-dependent uptake of Chlamydia pneumoniae. Cell. Microbiol. 18, 1094–1105. doi: 10.1111/cmi.12569
Fernández Tena, A., and Casan Clarà, P. (2012). Deposition of inhaled particles in the lungs. Arch. Bronconeumol. 48, 240–246. doi: 10.1016/j.arbr.2012.02.006
Ferry, T., Perpoint, T., Vandenesch, F., and Etienne, J. (2005). Virulence determinants in Staphylococcus aureus and their involvement in clinical syndromes. Curr. Infect. Dis. Rep. 7, 420–428. doi: 10.1007/s11908-005-0043-8
Fine-Coulson, K., Reaves, B. J., Karls, R. K., and Quinn, F. D. (2012). The role of lipid raft aggregation in the infection of type II pneumocytes by Mycobacterium tuberculosis. PLoS ONE 7:e45028. doi: 10.1371/journal.pone.0045028
Fontán, P., Aris, V., Ghanny, S., Soteropoulos, P., and Smith, I. (2008). Global transcriptional profile of Mycobacterium tuberculosis during THP-1 human macrophage infection. Infect. Immun. 76, 717–725. doi: 10.1128/IAI.00974-07
Fox, G. J., Nhung, N. V., Sy, D. N., Hoa, N. L. P., Anh, L. T. N., Anh, N. T., et al. (2018). Household-contact investigation for detection of tuberculosis in Vietnam. N. Engl. J. Med. 378, 221–229. doi: 10.1056/NEJMoa1700209
Fuchs, S., Hollins, A. J., Laue, M., Schaefer, U. F., Roemer, K., Gumbleton, M., et al. (2003). Differentiation of human alveolar epithelial cells in primary culture: morphological characterization and synthesis of caveolin-1 and surfactant protein-C. Cell Tissue Res. 311, 31–45. doi: 10.1007/s00441-002-0653-5
Garcia-Perez, B. E., Mondragon-Flores, R., and Luna-Herrera, J. (2003). Internalization of Mycobacterium tuberculosis by macropinocytosis in non-phagocytic cells. Microb. Pathog. 35, 49–55. doi: 10.1016/S0882-4010(03)00089-5
Garton, N. J., Waddell, S. J., Sherratt, A. L., Lee, S. M., Smith, R. J., Senner, C., et al. (2008). Cytological and transcript analyses reveal fat and lazy persister-like bacilli in tuberculous sputum. PLoS Med. 5:e75. doi: 10.1371/journal.pmed.0050075
Gekara, N. O., Jacobs, T., Chakraborty, T., and Weiss, S. (2005). The cholesterol-dependent cytolysin listeriolysin O aggregates rafts via oligomerization. Cell. Microbiol. 7, 1345–1356. doi: 10.1111/j.1462-5822.2005.00561.x
Gekara, N. O., and Weiss, S. (2004). Lipid rafts clustering and signalling by listeriolysin O. Biochem. Soc. Trans. 32, 712–714. doi: 10.1042/BST0320712
Gilbert, R. J. (2010). Cholesterol-dependent cytolysins. Adv. Exp. Med. Biol. 677, 56–66. doi: 10.1007/978-1-4419-6327-7_5
Gleeson, L. E., Sheedy, F. J., Palsson-Mcdermott, E. M., Triglia, D., O'leary, S. M., O'sullivan, M. P., et al. (2016). Cutting edge: Mycobacterium tuberculosis induces aerobic glycolysis in human alveolar macrophages that is required for control of intracellular bacillary replication. J. Immunol. 196, 2444–2449. doi: 10.4049/jimmunol.1501612
Golden, M. P., and Vikram, H. R. (2005). Extrapulmonary tuberculosis: an overview. Am. Fam. Phys. 72, 1761–1768.
Gonzalez-Juarbe, N., Bradley, K. M., Shenoy, A. T., Gilley, R. P., Reyes, L. F., Hinojosa, C. A., et al. (2017). Pore-forming toxin-mediated ion dysregulation leads to death receptor-independent necroptosis of lung epithelial cells during bacterial pneumonia. Cell Death Differ. 24, 917–928. doi: 10.1038/cdd.2017.49
Gouaux, E., Hobaugh, M., and Song, L. (1997). alpha-Hemolysin, gamma-hemolysin, and leukocidin from Staphylococcus aureus: distant in sequence but similar in structure. Protein Sci. 6, 2631–2635. doi: 10.1002/pro.5560061216
Guinn, K. M., Hickey, M. J., Mathur, S. K., Zakel, K. L., Grotzke, J. E., Lewinsohn, D. M., et al. (2004). Individual RD1-region genes are required for export of ESAT-6/CFP-10 and for virulence of Mycobacterium tuberculosis. Mol. Microbiol. 51, 359–370. doi: 10.1046/j.1365-2958.2003.03844.x
Gupta, S., and Chatterji, D. (2005). Stress responses in mycobacteria. IUBMB Life 57, 149–159. doi: 10.1080/15216540500090611
Hernandez-Pando, R., Jeyanathan, M., Mengistu, G., Aguilar, D., Orozco, H., Harboe, M., et al. (2000). Persistence of DNA from Mycobaterium tuberculosis in superficially normal lung tissue during latent infection. Lancet 356, 2133–2138. doi: 10.1016/S0140-6736(00)03493-0
Heuck, A. P., Moe, P. C., and Johnson, B. B. (2010). The cholesterol-dependent cytolysin family of gram-positive bacterial toxins. Subcell. Biochem. 51, 551–577. doi: 10.1007/978-90-481-8622-8_20
Hoyer, L. L., and Cota, E. (2016). Candida albicans Agglutinin-like sequence (Als) family vignettes: a review of als protein structure and function. Front. Microbiol. 7:280. doi: 10.3389/fmicb.2016.00280
Hsu, T., Hingley-Wilson, S. M., Chen, B., Chen, M., Dai, A. Z., Morin, P. M., et al. (2003). The primary mechanism of attenuation of bacillus Calmette-Guerin is a loss of secreted lytic function required for invasion of lung interstitial tissue. Proc. Natl. Acad. Sci. U.S.A. 100, 12420–12425. doi: 10.1073/pnas.1635213100
Huang, L., Nazarova, E. V., Tan, S., Liu, Y., and Russell, D. G. (2018). Growth of Mycobacterium tuberculosis in vivo segregates with host macrophage metabolism and ontogeny. J. Exp. Med. 215, 1135–1152. doi: 10.1084/jem.20172020
Hudock, T. A., Foreman, T. W., Bandyopadhyay, N., Gautam, U. S., Veatch, A. V., Lobato, D. N., et al. (2017). Hypoxia sensing and persistence genes are expressed during the intragranulomatous survival of Mycobacterium tuberculosis. Am. J. Respir. Cell Mol. Biol. 56, 637–647. doi: 10.1165/rcmb.2016-0239OC
Jang, S., Uzelac, A., and Salgame, P. (2008). Distinct chemokine and cytokine gene expression pattern of murine dendritic cells and macrophages in response to Mycobacterium tuberculosis infection. J. Leukoc. Biol. 84, 1264–1270. doi: 10.1189/jlb.1107742
Jedrzejas, M. J. (2001). Pneumococcal virulence factors: structure and function. Microbiol. Mol. Biol. Rev. 65, 187–207. doi: 10.1128/MMBR.65.2.187-207.2001
Jensch, I., Gamez, G., Rothe, M., Ebert, S., Fulde, M., Somplatzki, D., et al. (2010). PavB is a surface-exposed adhesin of Streptococcus pneumoniae contributing to nasopharyngeal colonization and airways infections. Mol. Microbiol. 77, 22–43. doi: 10.1111/j.1365-2958.2010.07189.x
Jones-Lopez, E. C., Acuna-Villaorduna, C., Fregona, G., Marques-Rodrigues, P., White, L. F., Hadad, D. J., et al. (2017). Incident Mycobacterium tuberculosis infection in household contacts of infectious tuberculosis patients in Brazil. BMC Infect. Dis. 17:576. doi: 10.1186/s12879-017-2675-3
Kinhikar, A., Vargas, D., Li, H., Mahaffey, S. B., Hinds, L., Belisle, J. T., et al. (2006). Mycobacterium tuberculosis malate synthase is a laminin binding adhesin. Mol. Microbiol. 60, 999–1013. doi: 10.1111/j.1365-2958.2006.05151.x
Kinhikar, A., Verma, I., Chandra, D., Singh, K. K., Weldingh, K., Andersen, P., et al. (2010). Potential role for ESAT-6 in dissemination of M. tuberculosis via human lung epithelial cells. Mol Microbiol. 75, 92–106. doi: 10.1111/j.1365-2958.2009.06959.x
Kohama, H., Umemura, M., Okamoto, Y., Yahagi, A., Goga, H., Harakuni, T., et al. (2008). Mucosal immunization with recombinant heparin-binding haemagglutinin adhesin suppresses extrapulmonary dissemination of Mycobacterium bovis Bacillus Calmette-Guerin (BCG) in infected mice. Vaccine 26, 924–932. doi: 10.1016/j.vaccine.2007.12.005
Kong, Y., Cave, M. D., Zhang, L., Foxman, B., Marrs, C. F., Bates, J. H., et al. (2007). Association between Mycobacterium tuberculosis Beijing/W lineage strain infection and extrathoracic tuberculosis: insights from epidemiologic and clinical characterization of the three principal genetic groups of M. tuberculosis clinical isolates. J. Clin. Microbiol. 45, 409–414. doi: 10.1128/JCM.01459-06
Krachler, A. M., and Orth, K. (2013). Targeting the bacteria-host interface: strategies in anti-adhesion therapy. Virulence 4, 284–294. doi: 10.4161/viru.24606
Krishnan, V., and Narayana, S. V. (2011). Crystallography of gram-positive bacterial adhesins. Adv. Exp. Med. Biol. 715, 175–195. doi: 10.1007/978-94-007-0940-9_11
Kwon, B. E., Ahn, J. H., Min, S., Kim, H., Seo, J., Yeo, S. G., et al. (2018). Development of new preventive and therapeutic vaccines for tuberculosis. Immune Netw. 18:e17. doi: 10.4110/in.2018.18.e17
La Distia Nora, R., Walburg, K. V., van Hagen, P. M., Swagemakers, S. M. A., van Der Spek, P. J., Quinten, E., et al. (2018). Retinal pigment epithelial cells control early Mycobacterium tuberculosis infection via interferon signaling. Invest. Ophthalmol. Vis. Sci. 59, 1384–1395. doi: 10.1167/iovs.17-23246
Laal, S. (2012). How does Mycobacterium tuberculosis establish infection? J. Infect. Dis. 206, 1157–1159. doi: 10.1093/infdis/jis382
Lafont, F., Tran Van Nhieu, G., Hanada, K., Sansonetti, P., and van Der Goot, F. G. (2002). Initial steps of Shigella infection depend on the cholesterol/sphingolipid raft-mediated CD44-IpaB interaction. EMBO J. 21, 4449–4457. doi: 10.1093/emboj/cdf457
Laventie, B. J., Sangermani, M., Estermann, F., Manfredi, P., Planes, R., Hug, I., et al. (2019). A surface-induced asymmetric program promotes tissue colonization by Pseudomonas aeruginosa. Cell Host Microbe 25, 140–152 e6. doi: 10.1016/j.chom.2018.11.008
Levin, M. J., Oxman, M. N., Zhang, J. H., Johnson, G. R., Stanley, H., Hayward, A. R., et al. (2008). Varicella-zoster virus-specific immune responses in elderly recipients of a herpes zoster vaccine. J. Infect. Dis. 197, 825–835. doi: 10.1086/528696
Lewis, K. N., Liao, R., Guinn, K. M., Hickey, M. J., Smith, S., Behr, M. A., et al. (2003). Deletion of RD1 from Mycobacterium tuberculosis mimics bacille Calmette-Guerin attenuation. J. Infect. Dis. 187, 117–123. doi: 10.1086/345862
Li, Q., Liu, H., Du, D., Yu, Y., Ma, C., Jiao, F., et al. (2015). Identification of novel laminin- and fibronectin-binding proteins by far-western blot: capturing the adhesins of Streptococcus suis type 2. Front. Cell. Infect. Microbiol. 5:82. doi: 10.3389/fcimb.2015.00082
Lim, J. S., Choy, H. E., Park, S. C., Han, J. M., Jang, I. S., and Cho, K. A. (2010). Caveolae-mediated entry of Salmonella typhimurium into senescent nonphagocytotic host cells. Aging Cell 9, 243–251. doi: 10.1111/j.1474-9726.2010.00554.x
Lin, A., Loughman, J. A., Zinselmeyer, B. H., Miller, M. J., and Caparon, M. G. (2009). Streptolysin S inhibits neutrophil recruitment during the early stages of Streptococcus pyogenes infection. Infect. Immun. 77, 5190–5201. doi: 10.1128/IAI.00420-09
Lin, P. L., Rutledge, T., Green, A. M., Bigbee, M., Fuhrman, C., Klein, E., et al. (2012). CD4 T cell depletion exacerbates acute Mycobacterium tuberculosis while reactivation of latent infection is dependent on severity of tissue depletion in cynomolgus macaques. AIDS Res. Hum. Retroviruses 28, 1693–1702. doi: 10.1089/aid.2012.0028
Lin, W., de Sessions, P. F., Teoh, G. H., Mohamed, A. N., Zhu, Y. O., Koh, V. H., et al. (2016). Transcriptional profiling of Mycobacterium tuberculosis exposed to in vitro lysosomal stress. Infect. Immun. 84, 2505–2523. doi: 10.1128/IAI.00072-16
Loh, L. N., Mccarthy, E. M. C., Narang, P., Khan, N. A., and Ward, T. H. (2017). Escherichia coli K1 utilizes host macropinocytic pathways for invasion of brain microvascular endothelial cells. Traffic 18, 733–746. doi: 10.1111/tra.12508
Lu, L. L., Chung, A. W., Rosebrock, T. R., Ghebremichael, M., Yu, W. H., Grace, P. S., et al. (2016). A functional role for antibodies in tuberculosis. Cell 167, 433–443 e14. doi: 10.1016/j.cell.2016.08.072
Ma, Y., Keil, V., and Sun, J. (2015). Characterization of Mycobacterium tuberculosis EsxA membrane insertion: roles of N- and C-terminal flexible arms and central helix-turn-helix motif. J. Biol. Chem. 290, 7314–7322. doi: 10.1074/jbc.M114.622076
Martinez, L., Shen, Y., Mupere, E., Kizza, A., Hill, P. C., and Whalen, C. C. (2017). Transmission of Mycobacterium tuberculosis in households and the community: a systematic review and meta-analysis. Am. J. Epidemiol. 185, 1327–1339. doi: 10.1093/aje/kwx025
Mcdonough Kathleen, A., and Kress, Y. (1995). Cytotoxicity for lung epithelial cells is a virulence-associated phenotype of Mycobacterium tuberculosis. Infect. Immun. 63, 4802–4811.
Mcmurray, D. N. (2003). Hematogenous reseeding of the lung in low-dose, aerosol-infected guinea pigs: unique features of the host-pathogen interface in secondary tubercles. Tuberculosis 83, 131–134. doi: 10.1016/S1472-9792(02)00079-3
Mehta, P. K., King, C. H., White, E. H., Murtagh, J. J. Jr., and Quinn, F. D. (1996). Comparison of in vitro models for the study of Mycobacterium tuberculosis invasion and intracellular replication. Infect. Immun. 64, 2673–2679.
Menozzi, F. D., Rousse, J. H., Alavi, M., Laude-Sharp, M., Muller, J., Bischoff, R., et al. (1996). Identification of heparin-binding hemmagglutinin present in mycobacteria. J. Exp. Med. 184, 993–1001. doi: 10.1084/jem.184.3.993
Mizrachi Nebenzahl, Y., Blau, K., Kushnir, T., Shagan, M., Portnoi, M., Cohen, A., et al. (2016). Streptococcus pneumoniae cell-wall-localized phosphoenolpyruvate protein phosphotransferase can function as an adhesin: identification of its host target molecules and evaluation of its potential as a vaccine. PLoS ONE 11:e0150320. doi: 10.1371/journal.pone.0150320
Morrison, J., Pai, M., and Hopewell, P. C. (2008). Tuberculosis and latent tuberculosis infection in close contacts of people with pulmonary tuberculosis in low-income and middle-income countries: a systematic review and meta-analysis. Lancet Infect. Dis. 8, 359–368. doi: 10.1016/S1473-3099(08)70071-9
Murray, C. J. L., and Collaborators, G. T. (2018). The global burden of tuberculosis: results from the Global Burden of Disease Study 2015. Lancet Infect. Dis. 18, 261–284. doi: 10.1016/S1473-3099(17)30703-X
Nachmias, N., Langier, S., Brzezinski, R. Y., Siterman, M., Stark, M., Etkin, S., et al. (2019). NLRP3 inflammasome activity is upregulated in an in-vitro model of COPD exacerbation. PLoS ONE 14:e0214622. doi: 10.1371/journal.pone.0214622
Naing, C., Mak, J. W., Maung, M., Wong, S. F., and Kassim, A. I. (2013). Meta-analysis: the association between HIV infection and extrapulmonary tuberculosis. Lung 191, 27–34. doi: 10.1007/s00408-012-9440-6
Nandre, R., Ruan, X., Lu, T., Duan, Q., Sack, D., and Zhang, W. (2018). Enterotoxigenic Escherichia coli adhesin-toxoid multiepitope fusion antigen CFA/I/II/IV-3xSTaN12S-mnLTG192G/L211A-derived antibodies inhibit adherence of seven adhesins, neutralize enterotoxicity of LT and STa toxins, and protect piglets against diarrhea. Infect. Immun. 86:e00550-17. doi: 10.1128/IAI.00550-17
Nardone, L. L., and Andrews, S. B. (1979). Cell line A549 as a model of the type II pneumocyte. Phospholipid biosynthesis from native and organometallic precursors. Biochim Biophys Acta 573, 276–295. doi: 10.1016/0005-2760(79)90061-4
Nguyen, L., and Pieters, J. (2005). The Trojan horse: survival tactics of pathogenic mycobacteria in macrophages. Trends Cell Biol. 15, 269–276. doi: 10.1016/j.tcb.2005.03.009
Nickol, M. E., Ciric, J., Falcinelli, S. D., Chertow, D. S., and Kindrachuk, J. (2019). Characterization of host and bacterial contributions to lung barrier dysfunction following co-infection with 2009 pandemic influenza and methicillin resistant Staphylococcus aureus. Viruses 11:116. doi: 10.3390/v11020116
Nieuwenhuizen, N. E., and Kaufmann, S. H. E. (2018). Next-generation vaccines based on bacille calmette-guerin. Front. Immunol. 9:121. doi: 10.3389/fimmu.2018.00121
Nobbs, A. H., Lamont, R. J., and Jenkinson, H. F. (2009). Streptococcus adherence and colonization. Microbiol. Mol. Biol. Rev. 73, 407–450. doi: 10.1128/MMBR.00014-09
Ohene, S. A., Bonsu, F., Hanson-Nortey, N. N., Sackey, A., Danso, S., Afutu, F., et al. (2018). Yield of tuberculosis among household contacts of tuberculosis patients in Accra, Ghana. Infect. Dis. Poverty 7:14. doi: 10.1186/s40249-018-0396-5
Onorato, I. M., and Mccray, E. (1992). Prevalence of human immunodeficiency virus infection among patients attending tuberculosis clinics in the United States. J. Infect. Dis. 165, 87–92. doi: 10.1093/infdis/165.1.87
Orlandi, P. A., and Fishman, P. H. (1998). Filipin-dependent inhibition of cholera toxin: evidence for toxin internalization and activation through caveolae-like domains. J. Cell Biol. 141, 905–915. doi: 10.1083/jcb.141.4.905
Palanisamy, G. S., Smith, E. E., Shanley, C. A., Ordway, D. J., Orme, I. M., and Basaraba, R. J. (2008). Disseminated disease severity as a measure of virulence of Mycobacterium tuberculosis in the guinea pig model. Tuberculosis 88, 295–306. doi: 10.1016/j.tube.2007.12.003
Pallen, M. J. (2002). The ESAT-6/WXG100 superfamily – and a new gram-positive secretion system? Trends Microbiol. 10, 209–212. doi: 10.1016/S0966-842X(02)02345-4
Pancholi, V., and Chhatwal, G. S. (2003). Housekeeping enzymes as virulence factors for pathogens. Int. J. Med. Microbiol. 293, 391–401. doi: 10.1078/1438-4221-00283
Pathakumari, B., Devasundaram, S., and Raja, A. (2017). Altered expression of antigen-specific memory and regulatory T-cell subsets differentiate latent and active tuberculosis. Immunology 153, 325–336. doi: 10.1111/imm.12833
Pethe, K., Alonso, S., Biet, F., Delogu, G., Brennan, M. J., Locht, C., et al. (2001). The heparin-binding haemagglutinin of M. tuberculosis is required for extrapulmonary dissemination. Nature 412, 190–194. doi: 10.1038/35084083
Pethe, K., Aumercier, M., Fort, E., Gatot, C., Locht, C., and Menozzi, F. D. (2000). Characterization of the heparin-binding site of the mycobacterial heparin-binding hemagglutinin adhesin. J. Biol. Chem. 275, 14273–14273. doi: 10.1074/jbc.275.19.14273
Pike, L. J. (2003). Lipid rafts: bringing order to chaos. J. Lipid Res. 44, 655–667. doi: 10.1194/jlr.R200021-JLR200
Rao, N. A., Saraswathy, S., and Smith, R. E. (2006). Tuberculous uveitis: distribution of Mycobacterium tuberculosis in the retinal pigment epithelium. Arch. Ophthalmol. 124, 1777–1779. doi: 10.1001/archopht.124.12.1777
Raynes, J. M., Young, P. G., Proft, T., Williamson, D. A., Baker, E. N., and Moreland, N. J. (2018). Protein adhesins as vaccine antigens for Group A Streptococcus. Pathog. Dis. 76:fty016. doi: 10.1093/femspd/fty016
Richter, J. F., Gitter, A. H., Günzel, D., Weiss, S., Mohamed, W., Chakraborty, T., et al. (2009). Listeriolysin O affects barrier function and induces chloride secretion in HT-29/B6 colon epithelial cells. Am. J. Physiol. Gastrointest. Liver Physiol. 296, G1350–G1359. doi: 10.1152/ajpgi.00040.2009
Rogers, T. J., Thorpe, C. M., Paton, A. W., and Paton, J. C. (2012). Role of lipid rafts and flagellin in invasion of colonic epithelial cells by Shiga-toxigenic Escherichia coli O113:H21. Infect. Immun. 80, 2858–2867. doi: 10.1128/IAI.00336-12
Ryndak, M. B., Chandra, D., and Laal, S. (2016). Understanding dissemination of Mycobacterium tuberculosis from the lungs during primary infection. J. Med. Microbiol. 65, 362–369. doi: 10.1099/jmm.0.000238
Ryndak, M. B., Singh, K. K., Peng, Z., and Laal, S. (2015). Transcriptional profile of Mycobacterium tuberculosis replicating in type II alveolar epithelial cells. PLoS ONE 10:e0123745. doi: 10.1371/journal.pone.0123745
Ryndak, M. B., Singh, K. K., Peng, Z., Zolla-Pazner, S., Li, H., Meng, L., et al. (2014). Transcriptional profiling of Mycobacterium tuberculosis replicating ex vivo in blood from HIV- and HIV+ subjects. PLoS ONE 9:e94939. doi: 10.1371/journal.pone.0094939
Sakinc, T., Woznowski, M., Ebsen, M., and Gatermann, S. G. (2005). The surface-associated protein of Staphylococcus saprophyticus is a lipase. Infect. Immun. 73, 6419–6428. doi: 10.1128/IAI.73.10.6419-6428.2005
Samanta, D., Mulye, M., Clemente, T. M., Justis, A. V., and Gilk, S. D. (2017). Manipulation of host cholesterol by obligate intracellular bacteria. Front. Cell. Infect. Microbiol. 7:165. doi: 10.3389/fcimb.2017.00165
Sanchez, C. J., Hinojosa, C. A., Shivshankar, P., Hyams, C., Camberlein, E., Brown, J. S., et al. (2011). Changes in capsular serotype alter the surface exposure of pneumococcal adhesins and impact virulence. PLoS ONE 6:e26587. doi: 10.1371/journal.pone.0026587
Savarino, S. J., Mckenzie, R., Tribble, D. R., Porter, C. K., O'dowd, A., Cantrell, J. A., et al. (2017). Prophylactic efficacy of hyperimmune bovine colostral antiadhesin antibodies against enterotoxigenic escherichia coli diarrhea: a randomized, double-blind, placebo-controlled, phase 1 trial. J. Infect. Dis. 216, 7–13. doi: 10.1093/infdis/jix144
Schnappinger, D., Ehrt, S., Voskuil, M. I., Liu, Y., Mangan, J. A., Monahan, I. M., et al. (2003). Transcriptional adaptation of mycobacterium tuberculosis within macrophages: insights into the phagosomal environment. J. Exp. Med.198, 693–704. doi: 10.1084/jem.20030846
Schneeberger, E. E. (1991). “Alveolar type I cells,” in The Lung, eds R. J. Crystal and J. B. West (New York, NY: Raven Press, Scientific Foundations), 229–234.
Scordo, J. M., Knoell, D. L., and Torrelles, J. B. (2016). Alveolar epithelial cells in Mycobacterium tuberculosis Infection: active players or innocent bystanders? J. Innate Immun. 8, 3–14. doi: 10.1159/000439275
Sebina, I., Biraro, I. A., Dockrell, H. M., Elliott, A. M., and Cose, S. (2014). Circulating B-lymphocytes as potential biomarkers of tuberculosis infection activity. PLoS ONE 9:e106796. doi: 10.1371/journal.pone.0106796
Shafer, R. W., Kim, D. S., Weiss, J. P., and Quale, J. M. (1991). Extrapulmonary tuberculosis in patients with human immunodeficiency virus infection. Medicine 70, 384–397. doi: 10.1097/00005792-199111000-00004
Sharma, S., Ryndak, M. B., Aggarwal, A. N., Yadav, R., Sethi, S., Masih, S., et al. (2017). Transcriptome analysis of mycobacteria in sputum samples of pulmonary tuberculosis patients. PLoS ONE 12:e0173508. doi: 10.1371/journal.pone.0173508
Shi, L., Sohaskey, C. D., Kana, B. D., Dawes, S., North, R. J., Mizrahi, V., et al. (2005). Changes in energy metabolism of Mycobacterium tuberculosis in mouse lung and under in vitro conditions affecting aerobic respiration. Proc. Natl. Acad. Sci. U.S.A. 102, 15629–15634. doi: 10.1073/pnas.0507850102
Siegrist, C. A. (2013). “General aspects of vaccination,” in Vaccines, 6th edn., eds S. A. Plotkin, W. A. Orenstein, and P. A. Offit (Elsevier), 14–32. doi: 10.1016/C2009-0-49973-2
Simeone, R., Bobard, A., Lippmann, J., Bitter, W., Majlessi, L., Brosch, R., et al. (2012). Phagosomal rupture by Mycobacterium tuberculosis results in toxicity and host cell death. PLoS Pathog. 8:e1002507. doi: 10.1371/journal.ppat.1002507
Skerry, C., Pokkali, S., Pinn, M., Be, N. A., Harper, J., Karakousis, P. C., et al. (2013). Vaccination with recombinant Mycobacterium tuberculosis PknD attenuates bacterial dissemination to the brain in guinea pigs. PLoS ONE 8:e66310. doi: 10.1371/journal.pone.0066310
Smith, J., Manoranjan, J., Pan, M., Bohsali, A., Xu, J., Liu, J., et al. (2008). Evidence for pore formation in host cell membranes by ESX-1-secreted ESAT-6 and its role in Mycobacterium marinum escape from the vacuole. Infect. Immun. 76, 5478–5487. doi: 10.1128/IAI.00614-08
Solanki, V., Tiwari, M., and Tiwari, V. (2018). Host-bacteria interaction and adhesin study for development of therapeutics. Int. J. Biol. Macromol. 112, 54–64. doi: 10.1016/j.ijbiomac.2018.01.151
Soltys, M. A., and Jennings, A. R. (1950). The dissemination of tubercle bacilli in experimental tuberculosis in the guinea pig. Am. Rev. Tuberc. 61, 399–406.
Soong, G., Martin, F. J., Chun, J., Cohen, T. S., Ahn, D. S., and Prince, A. (2011). Staphylococcus aureus protein A mediates invasion across airway epithelial cells through activation of RhoA GTPase signaling and proteolytic activity. J. Biol. Chem. 286, 35891–35898. doi: 10.1074/jbc.M111.295386
Stein, C. M., Nsereko, M., Malone, L. L., Okware, B., Kisingo, H., Nalukwago, S., et al. (2018). Long-term stability of resistance to latent M. tuberculosis infection in highly exposed TB household contacts in Kampala, Uganda. Clin Infect Dis. 68, 1705–1712. doi: 10.1093/cid/ciy751
Suárez, M., González-Zorn, B., Vega, Y., Chico-Calero, I., and Vázquez-Boland, J. A. (2001). A role for ActA in epithelial cell invasion by Listeria monocytogenes. Cell. Microbiol. 3, 853–864. doi: 10.1046/j.1462-5822.2001.00160.x
Syed, Y. Y. (2018). Recombinant zoster vaccine (Shingrix((R)): a review in herpes zoster. Drugs Aging 35, 1031–1040. doi: 10.1007/s40266-018-0603-x
Szabados, F., Mohner, A., Kleine, B., and Gatermann, S. G. (2013). Staphylococcus saprophyticus surface-associated protein (Ssp) is associated with lifespan reduction in Caenorhabditis elegans. Virulence 4, 604–611. doi: 10.4161/viru.25875
Tajima, A., Iwase, T., Shinji, H., Seki, K., and Mizunoe, Y. (2009). Inhibition of endothelial interleukin-8 production and neutrophil transmigration by Staphylococcus aureus beta-hemolysin. Infect. Immun. 77, 327–334. doi: 10.1128/IAI.00748-08
Timpe, J. M., Holm, M. M., Vanlerberg, S. L., Basrur, V., and Lafontaine, E. R. (2003). Identification of a Moraxella catarrhalis outer membrane protein exhibiting both adhesin and lipolytic activities. Infect. Immun. 71, 4341–4350. doi: 10.1128/IAI.71.8.4341-4350.2003
Toledo, A., and Benach, J. L. (2015). Hijacking and use of host lipids by intracellular pathogens. Microbiol Spectr. 3, 637–666. doi: 10.1128/microbiolspec.VMBF-0001-2014
Tsai, K. N., Chan, E. C., Tsai, T. Y., Chen, K. T., Chen, C. Y., Hung, K., et al. (2009). Cytotoxic effect of recombinant Mycobacterium tuberculosis CFP-10/ESAT-6 protein on the crucial pathways of WI-38 cells. J. Biomed. Biotechnol. 2009:917084. doi: 10.1155/2009/917084
Vadia, S., Arnett, E., Haghighat, A. C., Wilson-Kubalek, E. M., Tweten, R. K., and Seveau, S. (2011). The pore-forming toxin listeriolysin O mediates a novel entry pathway of L. monocytogenes into human hepatocytes. PLoS Pathog. 7:e1002356. doi: 10.1371/journal.ppat.1002356
van Dissel, J. T., Arend, S. M., Prins, C., Bang, P., Tingskov, P. N., Lingnau, K., et al. (2010). Ag85B-ESAT-6 adjuvanted with IC31 promotes strong and long-lived Mycobacterium tuberculosis specific T cell responses in naive human volunteers. Vaccine 28, 3571–3581. doi: 10.1016/j.vaccine.2010.02.094
van Leeuwen, L. M., Boot, M., Kuijl, C., Picavet, D. I., Van Stempvoort, G., Van Der Pol, S. M. A., et al. (2018). Mycobacteria employ two different mechanisms to cross the blood-brain barrier. Cell. Microbiol. 20:e12858. doi: 10.1111/cmi.12858
Vengadesan, K., and Narayana, S. V. (2011). Structural biology of Gram-positive bacterial adhesins. Protein Sci. 20, 759–772. doi: 10.1002/pro.613
Wagner, C., Khan, A. S., Kamphausen, T., Schmausser, B., Unal, C., Lorenz, U., et al. (2007). Collagen binding protein Mip enables Legionella pneumophila to transmigrate through a barrier of NCI-H292 lung epithelial cells and extracellular matrix. Cell. Microbiol.9, 450–462. doi: 10.1111/j.1462-5822.2006.00802.x
Wallgren, A. (1948). The time-table of tuberculosis. Tubercle 29, 245–251. doi: 10.1016/S0041-3879(48)80033-4
Wolf, A. J., Desvignes, L., Linas, B., Banaiee, N., Tamura, T., Takatsu, K., et al. (2008). Initiation of the adaptive immune response to Mycobacterium tuberculosis depends on antigen production in the local lymph node, not the lungs. J. Exp. Med. 205, 105–115. doi: 10.1084/jem.20071367
Yamaguchi, M., Terao, Y., Mori-Yamaguchi, Y., Domon, H., Sakaue, Y., Yagi, T., et al. (2013). Streptococcus pneumoniae invades erythrocytes and utilizes them to evade human innate immunity. PLoS ONE 8:e77282. doi: 10.1371/journal.pone.0077282
Yang, Z., Kong, Y., Wilson, F., Foxman, B., Fowler, A. H., Marrs, C. F., et al. (2004). Identification of risk factors for extrapulmonary tuberculosis. Clin. Infect. Dis. 38, 199–205. doi: 10.1086/380644
Zaas, D. W., Duncan, M. J., Li, G., Wright, J. R., and Abraham, S. N. (2005). Pseudomonas invasion of type I pneumocytes is dependent on the expression and phosphorylation of caveolin-2. J. Biol. Chem. 280, 4864–4872. doi: 10.1074/jbc.M411702200
Keywords: alveolar epithelial cells, tuberculosis, infection, adhesins, toxins, transcriptome, vaccine
Citation: Ryndak MB and Laal S (2019) Mycobacterium tuberculosis Primary Infection and Dissemination: A Critical Role for Alveolar Epithelial Cells. Front. Cell. Infect. Microbiol. 9:299. doi: 10.3389/fcimb.2019.00299
Received: 10 May 2019; Accepted: 02 August 2019;
Published: 21 August 2019.
Edited by:
Chad J. Roy, Tulane University School of Medicine, United StatesReviewed by:
Elizabeth B. Norton, Tulane University, United StatesCopyright © 2019 Ryndak and Laal. This is an open-access article distributed under the terms of the Creative Commons Attribution License (CC BY). The use, distribution or reproduction in other forums is permitted, provided the original author(s) and the copyright owner(s) are credited and that the original publication in this journal is cited, in accordance with accepted academic practice. No use, distribution or reproduction is permitted which does not comply with these terms.
*Correspondence: Suman Laal, c3VtYW4ubGFhbEBnbWFpbC5jb20=
Disclaimer: All claims expressed in this article are solely those of the authors and do not necessarily represent those of their affiliated organizations, or those of the publisher, the editors and the reviewers. Any product that may be evaluated in this article or claim that may be made by its manufacturer is not guaranteed or endorsed by the publisher.
Research integrity at Frontiers
Learn more about the work of our research integrity team to safeguard the quality of each article we publish.