- 1Institute of Veterinary Medicine, Jiangsu Academy of Agricultural Sciences, Nanjing, China
- 2Jiangsu Co-Innovation Center for the Prevention and Control of Important Animal Infectious Disease and Zoonose, Yangzhou University, Yangzhou, China
- 3College of Veterinary Medicine, Nanjing Agricultural University, Nanjing, China
- 4Key Lab of Food Quality and Safety of Jiangsu Province, State Key Laboratory Breeding Base, Nanjing, China
Streptococcus suis serotype 2 (SS2) is an important zoonotic pathogen responsible for septicemia and meningitis. The redox-sensing regulator Rex has been reported to play critical roles in the metabolism regulation, oxidative stress response, and virulence of various pathogens. In this study, we identified and characterized a Rex ortholog in the SS2 virulent strain SS2-1 that is involved in bacterial pathogenicity and stress environment susceptibility. Our data show that the Rex-knockout mutant strain Δrex exhibited impaired growth in medium with hydrogen peroxide or a low pH compared with the wildtype strain SS2-1 and the complementary strain CΔrex. In addition, Δrex showed a decreased level of survival in whole blood and in RAW264.7 macrophages. Further analyses revealed that Rex deficiency significantly attenuated bacterial virulence in an animal model. A comparative proteome analysis found that the expression levels of several proteins involved in virulence and oxidative stress were significantly different in Δrex compared with SS2-1. Electrophoretic mobility shift assays revealed that recombinant Rex specifically bound to the promoters of target genes in a manner that was modulated by NADH and NAD+. Taken together, our data suggest that Rex plays critical roles in the virulence and oxidative stress response of SS2.
Introduction
Streptococcus suis is an important zoonotic pathogen that has caused severe economic losses in the swine industry and endangered public health worldwide (Lun et al., 2007). Among the 33 serotypes defined based on capsular polysaccharide (CPS), serotype 2 (SS2) is the most virulent and the most frequently isolated in association with diseases in the majority of countries (Hill et al., 2005). The first human case of S. suis infection was reported in Denmark in 1968; by 2014, the total number of S. suis infections in humans was over 1600 (Goyette-Desjardins et al., 2014). In China, two large outbreaks of SS2 occurred in 1998 and 2005, resulting in 25 human cases with 14 deaths and 215 human cases with 38 deaths, respectively (Tang et al., 2006; Yu et al., 2006). During the past decades, numerous studies on S. suis have been performed; however, the pathogenesis of S. suis infection is still not entirely known (Segura et al., 2017).
During the infection process, pathogens encounter changing environments and host immune systems (Richardson et al., 2015). To deal with these hostile environments, pathogenic bacteria have evolved or acquired regulatory networks to sense and respond to environmental signals by modulating the expression of related genes. In S. suis, the signal regulatory systems contained within the two-component systems (TCSs) such as SalK/R (Li et al., 2008), NisKR (Xu et al., 2014), CiaRH (Li et al., 2011), and SsSTK/SsSTP (Zhu et al., 2011, 2014; Fang et al., 2017; Zhang et al., 2017), stand-alone regulators (SARs) such as CcpA (Willenborg et al., 2011, 2014) and Rgg (Zheng et al., 2011), and other regulators such as CodY (Feng et al., 2016), PerR (Zhang et al., 2012), AdcR, and Fur (Aranda et al., 2010) have been shown to be involved in bacterial metabolism and virulence. To gain further insight into the global regulatory networks of SS2, the role of other uncharacterized regulators should be investigated.
The redox-sensing regulator Rex was first discovered in Streptomyces coelicolor and is widely distributed among Gram-positive bacteria (Richardson et al., 2015). The crystal structures of Rex proteins from Thermus aquaticus and Bacillus subtilis in complex with NADH, NAD+, and/or DNA operator have been determined (Mclaughlin et al., 2010; Wang et al., 2011). Rex is composed of two domains, an N-terminal winged-helix DNA-binding domain and a C-terminal Rossmann-like domain involved in NADH binding and subunit dimerization. The DNA-binding activity of Rex proteins is modulated by the ratio of NADH to NAD+ concentrations (Brekasis and Paget, 2003; Mclaughlin et al., 2010). When the NADH/NAD+ ratio is low, Rex binds to target genes and represses the transcription of genes involved in NAD+ regeneration. In contrast, a high NADH/NAD+ ratio inhibits the DNA-binding activity of Rex and regulates the transcription of its target genes (Brekasis and Paget, 2003; Gyan et al., 2006; Pagels et al., 2010). The relationship between pathogenesis and the maintenance of an appropriate balance of reduced and oxidized NAD/NADH is not yet clear, but in some bacteria, such as Staphylococcus aureus (Pagels et al., 2010), Enterococcus faecalis (Vesić and Kristich, 2013), and Streptococcus mutans (Bitoun et al., 2012), the metabolic pathways under Rex control are implicated in virulence. In S. aureus, Rex is intimately involved in the survival of cells exposed to nitric oxide (NO), and it regulates genes involved in anaerobic respiration and fermentation (Pagels et al., 2010). The Rex ortholog in S. mutans contributes to the oxidative stress response and biofilm formation of bacteria (Bitoun et al., 2012; Bitoun and Wen, 2016). In E. faecalis, Rex has been shown to influence hydrogen peroxide (H2O2) accumulation (Vesić and Kristich, 2013).
Here, we characterized a Rex ortholog in S. suis (designed as SsRex) and examined the roles of SsRex in the oxidative stress tolerance and virulence of SS2. The isogenic mutant Δrex strain exhibited increased susceptibility to oxidative stress agents, decreased survival in blood and macrophages, and attenuated virulence in murine infection models, suggesting that SsRex plays important roles in the pathogenicity of S. suis.
Materials and Methods
Bacterial Strains, Plasmids, and Growth Conditions
The bacterial strains and plasmids used in this study are listed in Table 1. SS2 strain SS2-1 was isolated from a pig with a fatal case of septicemia in Jiangsu province in 1998 and has been confirmed as virulent by animal experiments (Zhu et al., 2014). SS2 strains were grown in Todd-Hewitt broth (THB) medium (Difco Laboratories) or plated on THB agar (THA) at 37°C. Escherichia coli strains were cultured in Luria-Bertani broth liquid medium or plated on Luria-Bertani agar. SS2 strains were grown in THB supplemented with 2% yeast extract (THY) for the preparation of competent cells. Culture media was supplemented with antibiotics (Sigma) as required at the following concentrations: spectinomycin (Spc), 100 μg/ml for SS2 and 50 μg/ml for E. coli; chloramphenicol (Cm), 4 μg/ml for SS2 and 8 μg/ml for E. coli.
Gene Inactivation and Functional Complementation
The thermosensitive suicide vector pSET4s was used for gene replacement in S. suis through homologous recombination (Takamatsu et al., 2001b). All the primers and plasmids used in this study are listed in Table 2. Two pairs of specific primers (L1/L2 and R1/R2) were used for cloning the Rex upstream and downstream homologous regions carrying HindIII/SalI and BamHI/EcoRI restriction enzyme sites, respectively. DNA fragments were digested with the corresponding restriction enzymes and directionally cloned into vector pSET4s. The cat gene expression cassette was amplified from pBR326 with primers CAT1/CAT2 and inserted at the SalI/BamHI sites to obtain the knockout vector pSET4sΔRex. To obtain the isogenic mutant Δrex, SS2-1 competent cells were electrotransformed with pSET4sΔRex as described previously (Takamatsu et al., 2001b). SS2-1 containing pSET4sΔRex were grown at 28°C in the presence of Cm and Spc. Cells in mid-log growth phase were diluted 1,000-fold with THY broth containing Cm and cultured at 28°C to the early logarithmic growth phase. The culture was next moved to 37°C and incubated for 4 h before subsequent dilution and plating on THY-Cm plates and non-selective plates, which were then incubated at 37°C. Cultures were screened for vector-loss mutants that had exchanged their wild-type (WT) allele for a genetic segment containing the rex gene after homologous recombination via a double crossover. For all CmR transformants, colony PCR assays with primers IN1/IN2 were performed to detect the presence of rex in the genome. Candidate mutants in which the rex failed to be amplified were further verified by PCR assays with primers CAT1/CAT2, OUT1/CAT2, and CAT1/OUT2 and then confirmed by DNA sequencing.
The SsRex promoter sequence was predicted based on computer analysis by using BPROM (http://www.softberry.com/berry.phtml). For functional complementation, the promoter region (372 bp) and open reading frame of the Rex sequence (639 bp) were amplified by PCR using specific primers C1/C2 (carrying SphI/EcoRI restriction enzyme sites) and then cloned into pSET2 (Takamatsu et al., 2001a) to obtain the recombinant plasmid pSET2-Rex, which was subsequently electroporated into Δrex. The complemented strain CΔrex was screened on THY plates with double selection pressure of Spc and Cm and further confirmed by PCR assays using primers IN1/IN2, CAT1/CAT2, and Spc1/Spc2.
Adherence and Invasion Assays
The adherence and invasion assays were performed using HEp-2 cells as described previously, with some modifications (Feng et al., 2016). HEp-2 cells were cultured in DMEM medium (Hyclone), maintained at 37°C, and supplemented with 10% (vol/vol) fetal bovine serum (FBS) (Sijiqing, Hangzhou, China). In the adherence assays, log-phase bacteria (107 CFU) were added to the wells of a 24-well tissue culture plate containing a monolayer (105 cells) of HEp-2 cells (multiplicity of infection [MOI] of 100 bacteria/HEp-2 cell). The plates were incubated for 2 h at 37°C in 5% CO2 to allow cell adherence by the bacteria. The monolayers were then washed four times with PBS, digested with 100 μl of trypsin for 15 min at 37°C, and then disrupted by the addition of 900 μl of sterile deionized water on ice followed by repeated pipetting to release all bacteria. Aliquots (100 μl) diluted 102 to 104 in PBS were used for quantitative plating.
For the invasion assays, log-phase SS2 (107 CFU) at a MOI of 100 were incubated with HEp-2 cells at 37°C for 2 h, and then the culture suspensions were removed. DMEM culture medium containing ampicillin (100 μg/ml) was added into the wells of cells infected with SS2 for 2 h to kill the bacteria outside the host cells. The cells were then washed four times with PBS. A 100-μl sample of the last PBS wash solution was plated on THA to test whether 100% of extracellular bacteria were killed after the antibiotic treatment. The cells were treated with 100 μl of trypsin for 15 min and then disrupted by the addition of 900 μl of sterile deionized water on ice. Aliquots (100 μl) of serial dilutions in PBS were used for quantitative plating. The experiments also included negative control cells without SS2 infection, which were plated on THA. The adherence and invasion assays were each performed in triplicate and repeated three times.
Acid and Oxidative Stress Challenges
To evaluate the role of SsRex in acid and oxidative stress, SS2 WT and its mutant strains were challenged with HCl and H2O2 according to previously described protocols (Han et al., 2012; Li et al., 2014). In the acid tolerance assays, log-phase bacteria cultured in THB (pH 7.0) were harvested by centrifugation at 3000 ×g at 4°C for 10 min, washed once with 0.1 M glycine buffer (pH 7.0), and then resuspended using THB with various pH values (4.0, 5.0, 6.0, and 7.0), which were achieved by adjustment with HCl. The suspensions were incubated for up to 4 h at 37°C, and the numbers of surviving cells were determined by plating them on THA plates in triplicate, followed by incubation at 37°C for 24 h (Han et al., 2012).
In the oxidative stress tolerance assays, log-phase bacteria were pelleted, washed, and resuspended in 0.1 M glycine buffer, pH 7.0. H2O2 was added to the cell suspension to create a final concentration of 10 mM (Han et al., 2012), and the cells were incubated for 15 min, 30 min, or 45 min. The catalase was then added immediately (5 mg/ml; Sigma) to the samples to inactive the H2O2 (Li et al., 2014). Lastly, the survival rate was calculated by plating the samples in triplicate on THA plates. These experiments were performed in triplicate and repeated three times.
Intracellular Survival Assays
Murine macrophage RAW264.7 cells were cultured in DMEM supplemented with 10% FBS in 24-well tissue culture plates at a concentration of 4 × 105 cells/well for intracellular survival assays as described previously, with some modifications (Tang et al., 2012). Log-phase bacteria were pelleted, washed twice with sterile PBS, and resuspended in fresh serum-free DMEM without antibiotics. The RAW264.7 cells were infected at a MOI of 10. After being co-cultured with the SS2 strains for 1 h at 37°C in 5% CO2, the macrophages were washed four times with PBS and then incubated in DMEM with 1% FBS containing ampicillin (100 μg/ml) for the duration of the assays. For measuring the survival of intracellular bacteria, infected cells were sampled at 1 h after the addition of antibiotics (time 0) and again at 2, 4, 6, 8, and 10 h. The cells were washed three times with sterile PBS and then incubated with 0.02% Triton X-100 for 15 min at 37°C to lyse the macrophages and release intracellular bacteria. Serial dilutions of these lysates were plated on THA plates and incubated overnight at 37°C. Colonies were counted to determine the number of intracellular bacteria. To analyze the number of bacteria surviving over time compared with the initial number of intracellular bacteria, the relative CFU (rCFU) was calculated as follows: rCFU at time point x/CFU at time point 0 (Cumley et al., 2012). These experiments were performed with three biological replicates, each with two technical replicates.
Survival in Blood
The survival of SS2 in whole blood was determined as described in previous studies (Feng et al., 2016). Briefly, 50 μl of mid-log-phase cultures of the SS2-1 and Δrex strains, which had been pelleted, washed twice with sterile PBS, and adjusted to an OD600 of 0.1, were inoculated into sterile Eppendorf tubes pre-filled with 450 μl of fresh heparinized pig blood from clinically healthy pigs. The blood-bacteria mixtures were incubated at 37°C for 2 h. Surviving bacteria were then diluted and plated on THA. These experiments were performed in duplicate and repeated three times.
Mouse Infections
All animal experiments were approved by the Science and Technology Agency of Jiangsu Province. Approval (ID: XYSK 2015-0020) was also granted by the Jiangsu Academy of Agricultural Sciences Experimental Animal ethics committee. All efforts were made to minimize the suffering of the animals. The BALB/c mice (female, 4–6 weeks old) were obtained from Yangzhou Laboratory Animal Research Center, Yangzhou, China. A total of 80 female BALB/c mice were randomly classified into 10 groups with 8 mice per group. Log-phase cultures of SS2 strains were centrifuged, and the resulting cell pellets were washed twice in PBS and then suspended in THY. For each strain, three groups of experimental mice were injected intraperitoneally (ip) with 1.0 ml of bacterial suspension at the following concentrations: 4.20 × 107 CFU/ml, 8.40 × 106 CFU/ml, and 1.68 × 106 CFU/ml. The last group mice (8 control mice) were inoculated with only the medium solution (THY). Mortality was monitored until 7 days post-infection, after which the 50% lethal dose (LD50) value was calculated using the method of Reed and Muench (Reed Lj, 1938).
Protein Digestion and iTRAQ Labeling
All the SS2 strains were grown in THB in triplicate (three WT strain SS2-1 and three mutant strain Δrex).When the SS2 cultures reached an OD600 of 0.7, 80 ml of sample were taken from each culture and pooled (240 ml total volume) (Redding et al., 2006). The samples were centrifuged at 10,000 × g for 5 min at 4°C, and the resulting cell pellets were then washed twice with PBS (Shen et al., 2013). iTRAQ analysis was carried out at Wuhan GeneCreate Biological Engineering Co., Ltd. (Wuhan, GeneCreate, China). Protein digestion was performed as previously described (Jing et al., 2008) with some modifications. Briefly, bacterial cell pellets were ground to powder in liquid nitrogen and incubated in dissolution buffer (8 M urea/100 mM triethylammonium hydrogen carbonate buffer [TEAB], pH 8.0) containing 1 mM PMSF and 2 mM EDTA (final concentration) for 5 min, and 10 mM DTT (final concentration) was then added to the sample. The suspension was sonicated for 15 min and then centrifuged at 4°C at 14,000 ×g for 20 min. The resulting supernatant was mixed with four volumes of precooled acetone at −20°C overnight. After another centrifugation, the resulting protein pellets were air-dried and resuspended in 8 M urea/100 mM TEAB (pH 8.0). Protein samples were reduced with 10 mM DTT at 56°C for 30 min and then alkylated with 50 mM iodoacetamide (IAM) for 30 min in the dark. The protein concentration was measured using the Bradford Protein Assay Kit (Beyotime,Shanghai,China). After being diluted 5× with 100 mM TEAB, equal amount of proteins from each sample were used for tryptic digestion. Trypsin was added at an enzyme protein ratio of 1:50 (w/w), and the digest reaction was performed at 37°C for 12–16 h. After digestion, peptides were desalted using C18 columns, and the resulting desalted peptides were dried with a vacuum concentration meter. The dried peptide powder was later re-dissolved with 0.5 M TEAB and processed according to the manufacturer's instructions for the iTRAQ Reagent-8 plex Multiplex Kit (AB Sciex U.K. Limited). The peptides from the WT strain SS2-1 were labeled with iTRAQ tag 119, and the peptides from the mutant strain Δrex were labeled with tag 121. The peptide samples were fractionated using a Durashell C18 column (5 μm, 100 Å, 4.6 × 250 mm) on an Ultimate 3000 HPLC system (Thermo DINOEX, USA) operating at 1 ml/min. Peptides were separated by increasing acetonitrile (ACN) concentrations under high pH (pH 10) conditions. Fractions were collected at 1-ml intervals and pooled into 12 fractions. Each fraction was dried with a vacuum concentration meter.
LC-ESI-MS/MS Analysis
The peptide samples were dissolved in 2% acetonitrile/0.1% formic acid and analyzed using a Triple TOF 5600+ mass spectrometer coupled with the Eksigent nanoLC System (SCIEX, USA) as previously described (Lin et al., 2015). The raw files collected from the Triple TOF 5600 were interpreted by ProteinPilot version 4.5 (July 2012, Applied Biosystems; Foster City, CA, USA). MS/MS spectra were searched against the Uniprot S. suis database (80,299 items, updated Jan 2017). The following search parameters were used: the instrument was set as TripleTOF 5600 plus with cysteine carbamidomethylation and 8 multiplex iTRAQ labeling was set as a fixed modification, methionine oxidation as a variable modification, and digestion by trypsin with at less one missed cleavage. The ratio of label 119 to label 121 represents the expression of proteins with the protein identification confidence of a 1% false discovery rate (Unwin et al., 2010). The proteins were considered overexpressed when the iTRAQ ratio was above 1.5 and under-expressed when the iTRAQ ratio was lower than 0.67 (Yu et al., 2018).
Transcriptional Analysis
Total RNA was extracted from in vitro late log-phase (OD600, 0.6–0.8) bacterial culture using the EZNA bacterial RNA kit (Omega, Beijing, China) according to the manufacturer's protocol. cDNA was reverse transcribed using a PrimeScript RT reagent kit with gDNA Eraser (TaKaRa, Dalian, China). The two-step relative quantitative real time PCR (qRT-PCR) was performed to analyze the expression profile of these selected genes using a SYBR Premix Ex Taq kit (TaKaRa). The ABI 7500 RT-PCR system was used for relative qRT-PCR. The gene-specific primers used for the qRT-PCR assays are listed in Table 2. The housekeeping gene aroA (encoding 5-enolpyruvylshikimate 3-phosphate synthase) used as the internal control was also amplified under the same conditions to normalize reactions. Reactions were performed in triplicate. A dissociation analysis of amplification products was performed at the end of each PCR assay to confirm that only one PCR product was amplified and detected. The relative fold change was calculated based on the 2−ΔΔCt method (Livak and Schmittgen, 2001).
Expression and Purification of the Recombinant Protein SsREX
The whole open reading frame of SsRex was amplified by PCR with primers Rex-F and Rex-R containing added BamHI and XhoI recognition sites (listed in Table 2). The PCR product was restriction-enzyme digested with BamHI and XhoI and then inserted into the similarly digested pET-28a vector (Novagen) to generate the recombinant plasmid pET28a-Rex, which was then transformed into E. coli BL21 (DE3) cells. The recombinant Rex protein was expressed via IPTG (isopropyl–D-thiogalactopyranoside) induction. The resulting fusion protein was purified using a HisTrap HP column (GE Healthcare, Shanghai, China) according to the manufacturer's guidelines. The expression and purity of the fusion protein were determined by sodium dodecyl sulfate-polyacrylamide gel electrophoresis (SDS-PAGE). The purified protein was stored at 4°C for later use in electrophoretic mobility shift assays.
Electrophoretic Mobility Shift Assays (EMSAs)
Protein–DNA interactions were analyzed by performing EMSAs according to previously described protocols with some modifications (Pagels et al., 2010; Bitoun et al., 2012). The promoter regions of the potential target genes, which were selected according to our comparative proteomics results and findings from previous studies in other pathogens, were amplified by PCR from SS2-1 gDNA using the specific primers shown in Table 2 and purified by using the High Pure PCR product purification kit (Axygen, Hangzhou, China). The purified PCR fragments were incubated with increasing amounts of rSsRex in reaction buffer containing 20 mM Tris pH 8.0, 1 mM EDTA, 75 mM KCl, 2 mM DTT, and 10% glycerol for 20 min at 30°C. When NAD+ or NADH was included for EMSAs, the samples were incubated for an additional 15 min (Pagels et al., 2010; Bitoun et al., 2012). Promoter fragments of impdh (Inosine 5-monophosphate dehydrogenase) (Zhou et al., 2014) lacking a putative Rex-binding site and 16sRNA were used as negative controls. Another two control reactions in which only included the DNA fragment or rRex protein were also performed. The reaction mixtures were separated on a 6% polyacrylamide gel in 0.5× Tris-borate-EDTA for 60 min. After electrophoresis, the DNA mobility shift was visualized by staining with ethidium bromide using a Tanon 2500R imager.
Statistical Analysis
All the statistical analyses were performed using GraphPad Prism 5. One-way analysis of variance (ANOVA) tests were used to analyze the oxidative stress, bacterial adherence, and survival in whole blood assays. Mann-Whitney tests were used to analyze the bacterial load in all organs examined. Two-way ANOVAs were performed on the qRT-PCR results. Values of P that were <0.05 were considered significant.
Results
Bioinformatics Analysis of Rex in the S. suis Genome
The rex gene of SS2-1 has a 639-bp open reading frame and encodes a protein composed of 213 amino acids with a predicted molecular mass of 23.7 kDa and an isolectric point of 8.27. BlastN analysis using the rex sequence of SS2-1 confirmed the presence of rex in all 35 complete S. suis genomes available in the National Centre for Biotechnology Information database as of 31 Dec 2017 (data not shown). BlastP analysis revealed that SsRex (possessing N-terminal DNA-binding sites and a C-terminal Rossmann-fold NAD(P)H/NAD(P)(+)-binding [NADB] domain) exhibited more than 78% amino acid sequence identity with the Rex homolog proteins in other Streptococci. Multiple sequence alignments of the redox-sensing regulator Rex from S. suis and other Streptococci revealed that Rex is highly conserved among Streptococcus species. Protein homology modeling was performed to predict the structure of SsRex, which may be useful for studying its active sites and designing therapeutics against S. suis infection. The secondary structure of SsRex is predicted to consist of nine α-helices, eight β-sheets, and three coils (Data not shown).
The rex gene of SS2-1 is flanked downstream by chp, which encodes a conserved hypothetical protein, and upstream by pp, which encodes a putative peptidase (Figure 1A). Computer based analysis using BPROM (www.softberry.com), a bacterial sigma70 promoter recognition program, identified a putative −10 and −35 element of the rex promoter situated 97 nucleotides upstream of the translational start site (GTG) of Rex. The transcriptional terminator signal was predicted by an online tool (http://rna.igmors.u-psud.fr/toolbox/arnold/). It identified an inverted repeat sequence (TGAATATAAAACAGGGGATCATACAGAACCCCTGCTTTCTTATACCCA) (underline nucleotides denote palindrome sequence) situated 36 nucleotides downstream of the stop coden (TAA) of rex that may be a transcriptional terminator, suggesting that rex is monocistronically transcribed. Reverse transcription-PCR (RT-PCR) was performed using the primers CT-P1/P2 and CT-P3/P4 to analyze the transcription of genes adjacent to rex and the results showed that rex was a single transcript unit, confirming the predicted results of online tools (Supplementary Figure 1). The binding sequence of Rex is highly conserved in Gram-positive bacteria. The reported consensus sequences in S. coelicolor (5′-TGTGAACNNNTTCACA-3′) (Brekasis and Paget, 2003), B. subtilis (5′ -WWTGTGAANTNNTNNNCAAW-3′; W represents either A or T) (Wang et al., 2008), S. aureus (Pagels et al., 2010) (5′ -TTGTGAAWWWWTTCACAA-3′), and S. mutans (TTGTGAANNNNTTCACAA) (Bitoun et al., 2012) are very similar. In the SS2-1 strain, a putative Rex-binding sequence (TGTTGATTTTTTCACAA) in the Rex promoter region, which overlapped the −35 element, provides a feasible mechanism by which Rex feedback autoregulation could occur (Figure 1C). The EMSA results show that recombinant Rex (rRex) was able to bind to the promoter regions of rex(Prex), and displayed a dose-dependent mobility shift to the higher molecular mass of rRex-Prex complexes. However, the rRex protein did not bind to the control DNA fragment from 16SrRNA, and no binding was observed under these conditions, even with 150 ng of rRex protein (Figure 1B). These results further suggest that SsRex is an authentic Rex transcriptional factor that is poised for autoregulation.
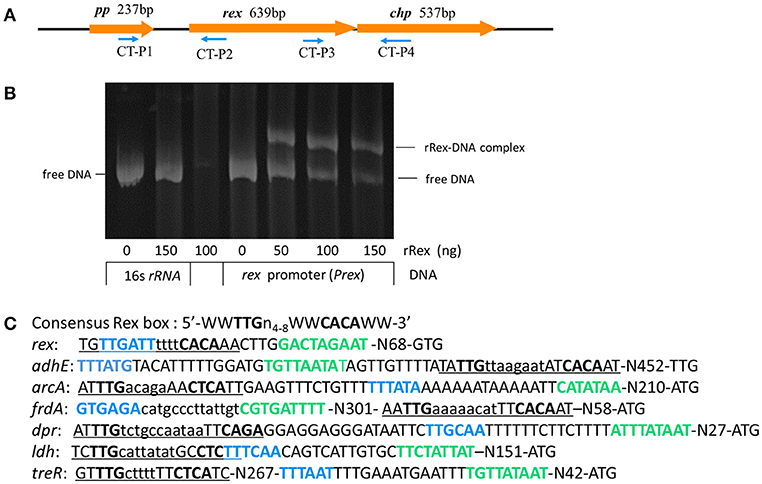
Figure 1. Identification and characterization of redox-sensing regulator Rex in SS2. (A) Schematic diagram of regions flanking rex, with the arrows indicating the direction of transcription and the numbers above indicating the sizes of the respective open reading frames in base pairs. (B) EMSA analysis shows interaction of rex promoter (Prex) with recombinant Rex (rRex) protein. Inclusion of rRex resulted in mobility shift and such interaction was concentration-dependent. (C) The promoter regions of selected genes identified in SS2-1 that contain putative Rex binding sites. The−35 regions (blue font) and−10 regions (green font) as determined by BPROM programs are shown in bold type and putative Rex-binding sites are underlined. W indicates A or T.
Construction of Rex Knockout Mutant and Complemented Strains
An isogenic rex mutant of SS2 strain SS2-1 was constructed through homologous recombination (Supplementary Figure 2A). One candidate mutant in which the rex gene failed to be amplified was found from more than 180 CmR transformants. The allelic replacement of the rex gene by the CatR in the mutant strain was confirmed by multiple PCR analysis (Supplementary Figure 2B), and DNA sequencing (data not shown). The complemented strain was screened on THY plates with double selection pressure of Spc and Cm and further confirmed by PCR analysis (Supplementary Figure 2C), and DNA sequencing (data not shown). A previous study reported that the bacterial cells carrying the pSET vectors should be grown under appropriate antibiotic pressures, especially when the recA mutant strains are used as the hosts (Takamatsu et al., 2001a). So we detected the expression levels of rex in SS2-1, Δrex, and CΔrex in presence or absence of antibiotic pressure by using qRT-PCR. The expression level value of rex in the WT SS2-1 was set as 1.0 and the results were shown as relative expression ratios compared to SS2-1. The qRT-PCR analysis showed that the expression level of rex in Δrex was not detectable, and that of in CΔrex with antibiotic, and CΔrex in the absence of antibiotic pressure were decreased by 0.32, and 0.45, respectively, compared with the WT strain (Supplementary Figure 2D). There was no significant difference between the CΔrex strain under the two conditions with or without antibiotic. The qRT-PCR results confirmed the rex was transcribed after complementation regardless of the antibiotic pressure.
Δrex Demonstrates Reduced Tolerance to Acid and Oxidative Stress Agents
During the infection process, S. suis is exposed to a hostile environment with various stress factors, including nutritional deprivation, increased osmolality, lowered pH, and reactive oxygen species (ROS) generated by host phagocytes (Zhu et al., 2014). Rex regulators play important roles in acid and oxidative stress tolerance in several pathogens (Pagels et al., 2010; Bitoun et al., 2012). To test whether SsRex affects the acid and oxidative tolerance of SS2, we compared the survival rates of SS2-1, Δrex, and CΔrex strains under these stress conditions in vitro. In a neutral pH environment, the survival rates of these three SS2 strains (WT SS2-1, Δrex, and CΔrex) were similar; in contrast, as the pH of the medium was lowered, the bacterial survival of Δrex rapidly decreased compared with the WT and the CΔrex strains (Figure 2A). The results show that Δrex had reduced tolerance to acidic pHs (p < 0.01). In addition, when these three SS2 strains were challenged with H2O2 (10 mM H2O2 in THB), Δrex was significantly more susceptible to H2O2 compared with the WT and CΔrex strains. However, there was no significant difference between the H2O2 susceptibility of Δrex and CΔrex following a 30 min-incubation in the oxidative stress assay (Figure 2B). These results suggest that the expression of SsRex contributes to the resistance of SS2 to environmental stresses.
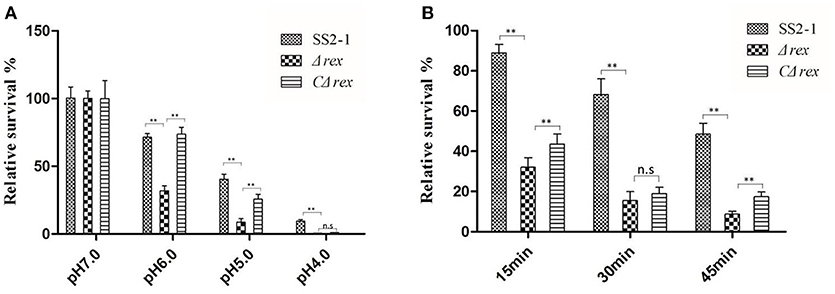
Figure 2. (A) In acid challenge assay, log phase SS2 cultures were harvested and washed once with 0.1 M glycine buffer (pH 7.0), and then resuspended using THB with various pH values (4.0, 5.0, 6.0, and 7.0), which were achieved by adjustment with HCl. The suspensions were incubated for up to 4 h at 37°C and the numbers of surviving cells were determined by plating them on THA plates in triplicate. (B) In H2O2 challenge assay, log phase SS2 were pelleted, washed and resuspended in 0.1 M glycine buffer (pH 7.0). H2O2 was added to the cell suspension to create a final concentration of 10 mM and incubation for 15, 30, and 45 min, respectively. Then catalase was added immediately (5 mg/mL; Sigma) to the samples to inactive H2O2. Surviving cells were diluted appropriately, plated on THA plates. The percentage of the CFU was normalized to WT group designed as 100% (n. s, p > 0.05, **p < 0.01).
SsRex Did Not Affect the SS2 Adherence to or Invasion of HEp-2 Cells
Successful establishment of infection by S.suis requires adhesion to host cells, colonization of tissues, and in certain cases, cellular invasion followed by intracellular multiplication, dissemination to other tissues (Tang et al., 2006). HEp-2 cells were used to evaluate the effcts of SsRex on the SS2 adhesion to and invasion of mammalian cells. Compared with the WT strain SS2-1, Δrex showed similar adherence and invasion levels (p > 0.05) (Supplementary Figure 3). These results indicate that SsRex was not crucial for SS2 adherence to or invasion of HEp-2 cells.
SsRex Deficiency Reduced Ss2 Survival in Whole Blood and Macrophages
S. suis invasion from the mucosal surface into deeper tissues and its blood circulation are critical events in the development of disease. Therefore, S. suis survival in the blood is central to its pathogenesis (Fittipaldi et al., 2012). To determine whether the deletion of SsRex affected the survival of SS2 in whole blood, we measured the survival ability of SS2 strains in whole pig blood. The survival rate of the WT strain SS2-1 was 45.0%, which was significantly higher than that of Δrex, which had a survival rate of 11.1% (p < 0.01) (Figure 3A). Compared with the mutant strain Δrex, the survival rate of the complemented strain CΔrex was increased up to 16.2%. However, although the entire rex gene is complemented in CΔrex (Supplementary Figure 2D), its survival rate in the blood bacterial assay was not as high as that of the WT strain (Figure 3A).Similar finding had also been reported in other study (Ju et al., 2012).
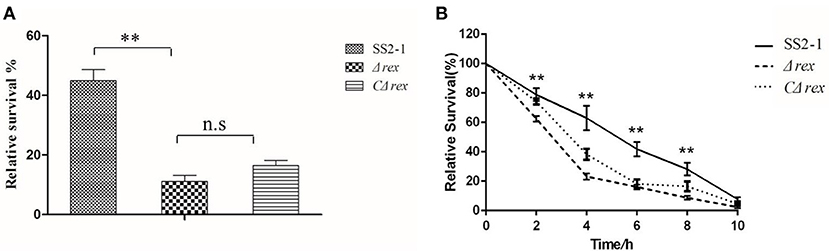
Figure 3. (A) Survival of WT SS2-1 in pig whole blood compared to that of the mutant strain Δrex and complemented strain CΔrex. Mixtures were incubated at 37°C for 2 h. A value of 100% was given to the CFU at time 0 h. The survival rate of Δrex was significantly reduced compared to SS2-1. (B) Intracellular growth of SS2 in RAW264.7 macrophages. The macrophages cells were infected with mid-log growth phase SS2 strains at a MOI of 10 (bacteria: macrophage).The samples were taken 1 h after the addition of antibiotics (time zero) and then at 2, 4, 6, 8, and 10 h. The relative numbers of CFU (rCFU) were estimated by plating out the lysates of infected macrophages and counting the numbers of CFU at each time point. Asterisks indicate the time points when the intracellular bacteria survival rates elicited by the Δrex were significantly lower than those produced by WT infection.
Once S. suis reaches deep tissues and/or the bloodstream, phagocytic cells play a pivotal role in the host defense against these invading pathogens during infection (Fang et al., 2017). Phagocytosis and intracellular survival assays were performed in RAW264.7 cells to assess the role of SsRex in the context of S. suis–phagocyte interactions. As shown in Figure 3B, there were no significant differences in the phagocytosis rates among SS2-1, Δrex, and CΔrex at timepoint of 0 h. However, significant differences were observed in the intracellular survival of SS2-1, Δrex, and CΔrex when they were co-incubated with macrophages for another 10 h (Figure 3B). Our results show that the mutant strain Δrex exhibited significantly reduced resistance to killing after ingestion by macrophages in a time-dependent manner (Figure 3B), indicating that SsRex significantly contributes to bacterial survival in macrophages and that its deletion results in the attenuated virulence of SS2.
Deletion of SsRex Attenuates SS2 Virulence in Mice
Previous studies have indicated that Rex proteins are important for the virulence of bacterial pathogens (Bitoun et al., 2012; Bitoun and Wen, 2016). To determine whether SsRex is involved in the virulence of SS2, we compared the LD50 values of SS2-1, its mutant Δrex, and the complementation strain CΔrex in BALB/c mice. As shown in Table 3, at a high bacterial dose (4.20 × 107 CFU per animal), mice in the WT and mutant groups all presented severe clinical symptoms associated with septicemia during the first 36 hpi, including depression, swollen eyes, weakness, and prostration. The death rate was 100% (8/8) in the WT group and 87.5% (7/8) in both the Δrex and CΔrex groups in the high dose trials. At an intermediate bacterial dose (8.40 × 106 CFU per animal), most mice in the WT group also presented severe clinical symptoms and prostration during the first 36 hpi, and seven mice (87.5%) died from septicemia. Mice in the Δrex group presented moderate clinical symptoms, and only one mouse (12.5%) died; four mice (50%) died from septicemia in the CΔrex group. At a low bacterial dose (1.68 × 106 CFU per animal), three mice died in the WT group. In contrast, no clinical symptoms were observed in the Δrex and CΔrex groups, and all the mice in these two groups survived until the end of the infection experiments. The LD50 value was 1.81 × 107 CFU/mouse for the Δrex group, which is 7-fold higher than that of the WT SS2-1 group (2.51 × 106 CFU/mouse), the LD50 value was 8.40 × 106 CFU/mouse for the CΔrex group. The results suggest that Rex deficiency impaired the virulence of SS2 in a BALB/c mouse infection model.
Differentially Expressed Proteins in the Δrex Mutant Strain
To determine which proteins are regulated by Rex, an iTRAQ analysis characterizing the differences in protein expression between the WT and Δrex strains was performed. Compared with the WT strain SS2-1, 39 proteins were upregulated and 57 proteins were downregulated in Δrex. The differentially expressed proteins (DEPs) between Δrex and the WT strain are summarized in Supplementary Table 1. (I) KEGG pathway analysis of DEPs. To obtain an overview of the major perturbed functions in Δrex compared with the WT SS2-1, a KEGG pathway enrichment analysis was conducted. Some of the DEPs in Δrex were involved in metabolic pathways (7 proteins [46.67%] upregulated; 14 proteins [73.68%] downregulated), biosynthesis of secondary metabolites (5 proteins [33.33%] upregulated; 8 proteins [42.11%] downregulated), and microbial metabolism in diverse environments (5 proteins [33.33%] upregulated; 7 proteins [36.84%] downregulated) (Figure 4). Three metabolic pathways were significantly altered by rex deletion, including glycolysis/gluconeogenesis, the pentose phosphate pathway, and the citrate cycle (TCA cycle) (Figure 5), which were all associated with central metabolism (Richardson et al., 2015). Five proteins, including 6-phosphogluconate dehydrogenase (6Pgd), phosphomannomutase (Pgm), glucose-6-phosphate 1-dehydrogenase (G6pdh), L-lactate dehydrogenase (Ldh), and ribose-phosphate pyrophosphokinase (Prs), were significantly downregulated, and five proteins, including ATP-dependent 6-phosphofructokinase (PfkA), pyruvate/2-oxoglutarate dehydrogenase complex, dehydrogenase (E1) component (Pdh), fumarate reductase flavoprotein subunit (FrdA), and alcohol dehydrogenases (AdhE, AdhP) were significantly upregulated (Figure 5). (II) SsRex regulates known virulence factors. The SsRex deletion significantly reduced the virulence of SS2. Among the 32 virulence factors (VFs) predicted by the Virulence Factor Database (VFDB) (Chen et al., 2005), 14 known VFs of SS were among the DEPs in the Δrex strain (Fittipaldi et al., 2012), including 9 downregulated proteins and 5 upregulated proteins, such as the transcriptional regulators TreR and Dpr, adenylosuccinate synthetase (PurA), autolysins (Ju et al., 2012), peptidase T (PepT) (Yu et al., 2016), type I restriction enzyme protein (HsdS) (Xu et al., 2017), aldehyde-alcohol dehydrogenase (AdhE) (Yu et al., 2018), foldase protein PrsA (Jiang et al., 2016), sialic acid synthase (NeuB), and arginine deiminase (ArcA) (Table 4). Another 18 VFs that have been reported in other pathogens were identified as DEPs in the Δrex strain, including ATP-dependent zinc metalloprotease FtsH (Choi et al., 2015), metalloendopeptidas (PepO) (Agarwal et al., 2013, 2014), branched-chain-amino-acid aminotransferase (IlvE) (Santiago et al., 2012), adenylosuccinate lyase (PurB) (Connolly et al., 2017), and ATP-dependent protease ATP-binding subunit ClpL (Kwon et al., 2003) (Table 4, Supplementary Table 1).
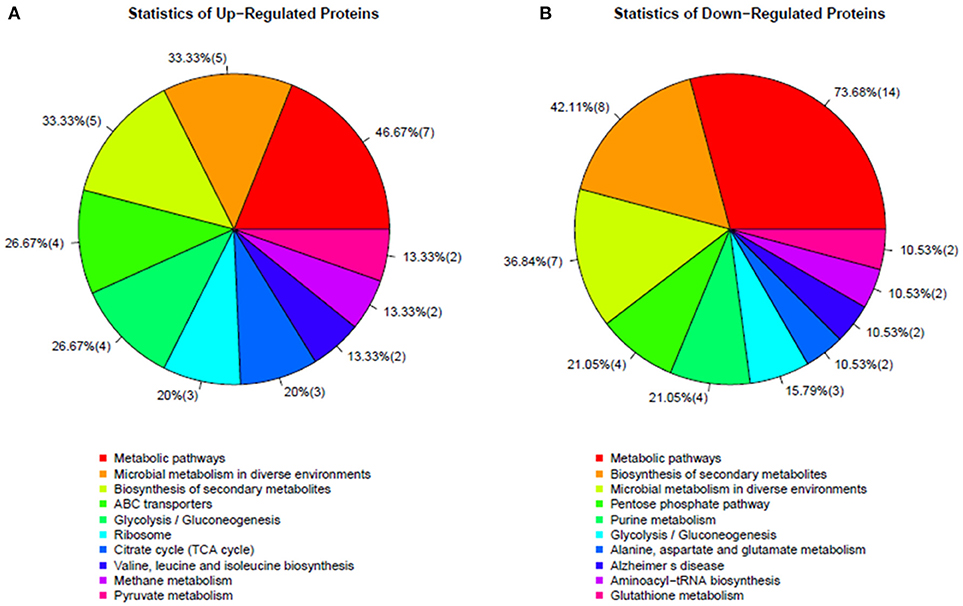
Figure 4. KEGG pathways enrichments in altered proteins in mutant strain Δrex. (A) Up-regulated proteins and (B) Down-regulated proteins.
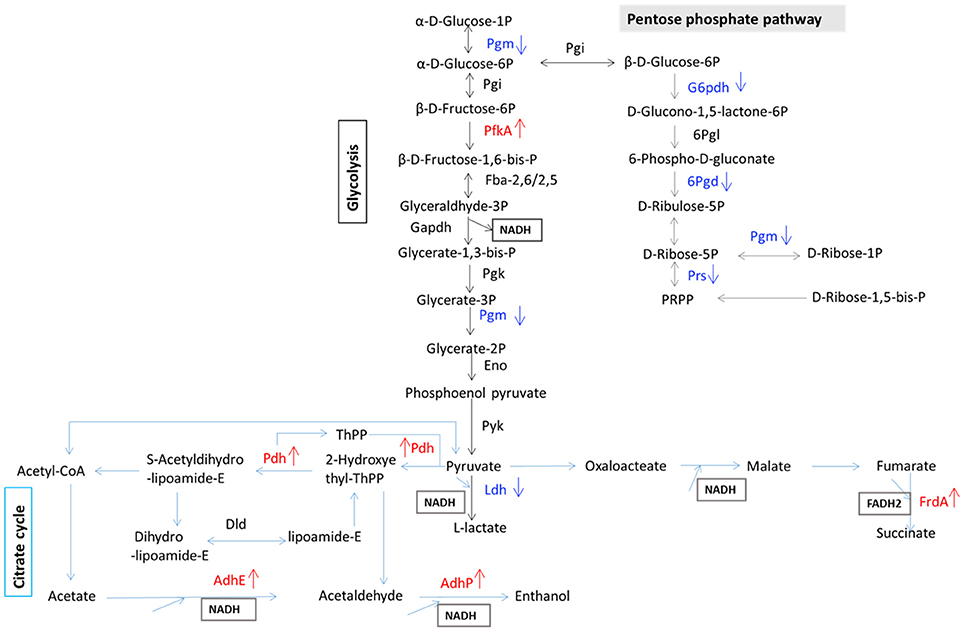
Figure 5. Schematic representation of SS2 metabolic pathways differentially regulated in carbohydrate metabolism. Differentially expressed proteins are involved in glycolysis, citrate cycle, and pentose phosphate pathway. Red color, up-regulated proteins; blue color, down-regulated proteins.
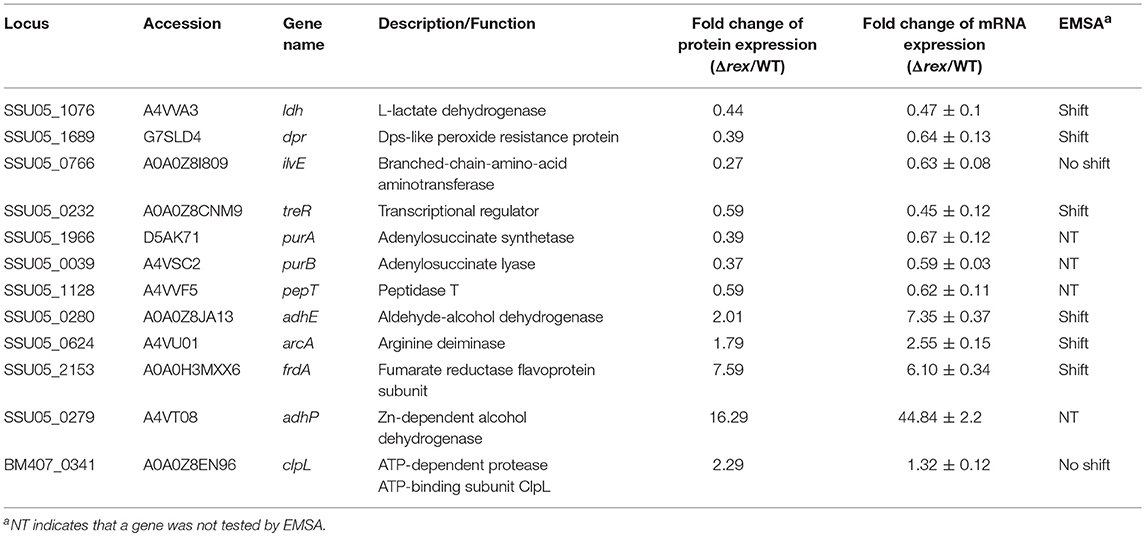
Table 4. Virulence associated factors identified by iTRAQ in Δrex and analyzed by qRT-PCR and EMSAs.
Transcriptional Analysis
Alterations in the expression level of a protein may be due to a change in its mRNA level. Twelve genes selected based on the DEPs in the Δrex strain, including seven downregulated genes and five upregulated genes, were measured via qRT-PCR in the mutant and WT strains. The aroA gene was used as a control housekeeping gene. As shown in Table 4, the transcript levels of the genes dpr, ilvE, pepT,treR, purA, purB, and ldh were significantly downregulated and those of adhE, adhP, clpL, frdA, and arcA were significantly upregulated in the mutant strain Δrex, which matched well with the comparative proteomics data.
DNA Binding Activity of SsRex
Rex is an autoregulation repressor that binds to the operators of its target genes, modulating them in response to the cellular NADH/NAD+ level (Brekasis and Paget, 2003). To determine if SsRex is an authentic Rex transcriptional factor, we performed EMSAs to evaluate the binding ability of rRex to its promoter sequence (Rex-promoter, Prex). As shown in Figure 1B, recombinant Rex (rRex) can bind to DNA fragments containing Prex, in the same manner as that reported for S. mutans (Bitoun et al., 2012). To verify the direct role of Rex in the regulation of the altered genes, we used EMSAs to assess the interactions between rRex and the promoters of selected genes identified from the comparative proteome and transcriptional analyses. We tested the promoters of four upregulated genes (adhE, arcA, clpL, and frdA) and four downregulated genes (dpr,ldh,ilvE,and treR). Six promoters that contained known consensus Rex-binding motifs (shown in Figure 1C) displayed a dose-dependent mobility shift to higher molecular mass, indicating the formation of rRex–promoter complexes (Figure 6A). However, no mobility shift was observed when rRex was mixed with the promoter of clpL and ilvE, which demonstrated that the two genes were not directly regulated by Rex and whose promoter does not have an apparent Rex-box (Figure 6A). The results showed that adhE,arcA, frdA, and ldh were the Rex targets for SS2, similar to the previous reported in S. aureus (Pagels et al., 2010) and S. mutans (Bitoun et al., 2012; Bitoun and Wen, 2016). In addition, we found that the promoter regions of the two regulators (dpr and treR) also could be bound by rRex. To the best of our knowledge, these two new genes promoters were identified as the targets of Rex which has been reported for the first time. It was also worth noting that Rex may also act as a transcriptional activator, directly binding to the promoters of down-regulated genes, as well as a repressor which had been extensively reported in some bacteria (Brekasis and Paget, 2003; Gyan et al., 2006; Pagels et al., 2010).
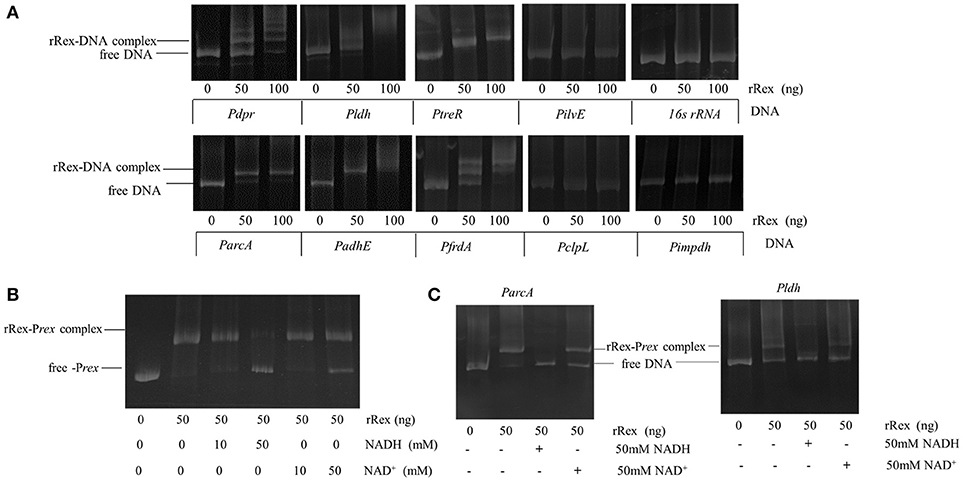
Figure 6. Determination of Rex binding abilities by EMSAs. (A) Binding of purified rRex to the promoter regions of different genes using EMSAs. The resulting protein–DNA complexes were separated from unbound DNA fragments using native polyacrylamide gels. The DNA fragments were visualized by ethidium bromide staining. Formation of stable Rex–DNA complexes resulted in one or more distinct shifted DNA bands. The promoter region of impdh, which is not regulated by Rex and whose promoter sequence lacks a putative Rex binding box, and 16sRNA DNA fragments were used as negative controls. (B) EMSAs were performed with PCR products of the promoter regions of rex incubated with 50 ng purified Rex protein and different concentrations of NAD+ and NADH. At lower concentration, NADH and NAD+ did not influence the rRex affinity to Prex and not change the mobility pattern. A higher concentration of NADH (50 mM) completely prevents the formation of rRex–Prex complexes, and 50 mM of NAD+ causes the complexes to dissociate slightly. (C) EMSAs were performed with PCR products of the promoter regions of arcA and ldh incubated with 50 ng purified rRex protein and 50 mM NAD+ or 50 mM NADH. The presence of 50 mM NADH inhibits promoter-DNA complex formation for ParcA and Pldh. However, the binding affinity between rRex and ParcA is slightly decreased in the presence of 50 mM NAD+, and rRex binds better to the Pldh when compared to ParcA under the same conditions.
To test if NADH and NAD+ affect the interaction between S. suis Rex and its cognate operators, EMSAs were performed using the target promoters Prex, ParcA, and Pldh. In the EMSA pre-reaction buffer, the rRex and the target promoters were pre-incubated for 20 min, and NADH and/or NAD+ (10 mM, and 50 mM, final concentration) were then added. As shown in Figure 6B, rSsRex can still bind to Prex with 10 mM of NAD+ or 10 mM of NADH in the EMSA reaction system, as evidenced by the mobility shift. The presence of 50 mM of NADH nearly completely prevents the formation of rRex–Prex complexes, whereas the addition of 50 mM of NAD+ did not result in a noticeable enhancement of Rex binding to the DNA fragment and exhibited a slight interference to the binding activity (Figure 6B). Additionally, rRex bound the arcA and ldh promoters under the similar conditions (Figure 6C). Although inclusion of lower concentration of NADH and NAD+ did not have a major effect on the mobility shift, the addition of higher concentration of NADH nearly completely abolished such binding activity (Figures 6B,C). It was unexpected that high concentration of NAD+ slightly impaired the formation of rRex-DNA complexes for some genes in S. suis. Similar findings had been reported for the Rex-family protein RSP in Thermoanaerobacter ethanolicus (Pei et al., 2011). The results show that SsRex could bind to its promoter region as an authentic Rex family protein and that the interaction was modulated by NADH and NAD+.
Discussion
Bacterial pathogens use global regulatory networks to sense and modify gene expression in response to changing environments. In some Gram-positive bacteria, including S. aureus (Pagels et al., 2010), B. subtilis (Gyan et al., 2006; Wang et al., 2008), E. faecalis (Vesić and Kristich, 2013), and S. mutans (Bitoun et al., 2012; Bitoun and Wen, 2016), Rex proteins are global transcriptional regulators that play a pivotal role in the regulation of energy metabolism, stress tolerance, and virulence (Pagels et al., 2010; Bitoun et al., 2012; Vesić and Kristich, 2013; Laouami et al., 2014; Zhang et al., 2014; Hu et al., 2016; Liu et al., 2017). However, the role of Rex in SS2 had not been investigated in detail.
A bioinformatics analysis revealed that SsRex (possessing N-terminal DNA-binding sites and a C-terminal Rossmann-fold NAD(P)H/NAD(P)(+)-binding (NADB) domain) exhibits >78% amino acid sequence identity with the Rex homolog proteins in other Streptococci. This conserved structural feature ensures that the Rex protein can to bind specific DNA sequences to regulate the expression of target genes. In the present study, EMSAs revealed that both NADH and NAD+ could bind to rSsRex (Figures 6B,C). This finding suggests that SsRex is a typical member of the Rex family of regulatory proteins in that it has common structural features and similar regulatory mechanisms with other homolog proteins of the Rex family. Furthermore, our EMSA analysis of selected promoters (adhE, arcA, frdA, dpr, ldh, and treR) with known Rex-binding sites also demonstrated that these DNA sequences interact with rSsRex (Figure 6A) and the interaction was modulated by NADH and NAD+ (Figures 6B,C). Our additional analysis of these promoter regions/Rex-binding sites further showed that differences exist among various promoters in their nucleotide compositions and positions to the translation initiation site (ATG or GTG or TTG) (Figure 1C).Microarray data in S. mutans showed that Rex-deficiency effected transcription dissimilarly, suggesting either the involvement of other regulator(s) and/or regulation levels that depend on the nucleotide composition of the Rex-binding site (Bitoun et al., 2012). In S. aureus, Rex binding sites are localized within the promoter regions of its target genes or are only a few base pairs upstream or downstream of the promoter regions. This finding supports the idea that the binding of Rex at these sites prevents the binding of the RNA polymerase and hence hinders transcriptional initiation (Pagels et al., 2010). However, further studies are needed to elucidate the molecular mechanism of the Rex regulation involvement of SS2.
Rex proteins are important for the virulence of S. mutans (Bitoun et al., 2012; Bitoun and Wen, 2016). Here, the LD50 values of Δrex calculated from BALB/c mouse infection model studies and in vivo colonization experiments (data not shown) show that the deletion of rex in S. suis resulted in altered bacterial pathogenicity and that SsRex facilitates the virulence of SS2. The attenuation of Δrex may be due to a direct effect of rex on the expression of VFs. The comparative protein expression profiles showed that, among the 96 DEPs in Δrex, there were 32 VFs predicted by the VFDB, including 20 downregulated and 12 upregulated proteins (Table 4, Supplementary Table 1) such as L-lactate dehydrogenase (Ldh), aminopeptidase T (AmpT), adenylosuccinate lyase (PurB), and metalloendopeptidase (PepO). In Listeria monocytogenes, AmpT is a member of the M29 family aminopeptidases that is involved in invasion and intracellular survival inside the host cells and is required for full virulence in a murine infection model (Cheng et al., 2015). In S. aureus, purine biosynthesis (PurA, PurB) is indispensable for its growth in human blood and in vivo pathogenicity in a zebrafish embryo model (Connolly et al., 2017). In Streptococcus pneumoniae, PepO is a multifunctional plasminogen- and fibronectin-binding protein that modulates the complement attack by binding to the complement component C1q (Agarwal et al., 2013, 2014).
Our iTRAQ analysis also identified 9 known S. suis VFs (Fittipaldi et al., 2012) among the 32 VFs with repressed expression in Δrex, such as the transcriptional regulators TreR (Wilson et al., 2007) and Dpr (Zhang et al., 2012), PurA (Wilson et al., 2007), autolysins (Ju et al., 2012), PepT (Yu et al., 2016), and HsdS (Xu et al., 2017) (Table 4, Supplementary Table 1). In the SS2 virulent strain S735, treR and purA were identified as VFs by a novel signature-tagged mutagenesis system, and S735 with mutations in these genes had attenuated virulence in both mouse and piglet infection models (Wilson et al., 2007). In the SS2 virulent strain ZY05719, hsdS was reported to facilitate phagocytosis and survival in whole blood and to enhance the bacterial survival ability against a peroxidation environment (Xu et al., 2017). Furthermore, PepT was identified as a protein that is unique to a virulent strain, and its mutant strain ΔpepT had attenuated virulence in a zebrafish model (Yu et al., 2016). Therefore, downregulation of these known VFs may be associated with the decreased level of survival in whole blood and macrophages cells and the attenuated virulence of Δrex.
In some bacteria, the metabolic pathways under Rex control are implicated in virulence (Richardson et al., 2015). The GO term and KEGG pathway analyses showed that several DEPs, such as 6Pgd, Pgm,G6pdh, Ldh, PfkA, Pdh, FrdA, and Adh, contribute to complicated cellular metabolic pathways (Figure 5), including glycolysis/gluconeogenesis, the pentose phosphate pathway, and the TCA cycle (Richardson et al., 2015). In the present study, the EMSAs showed that rRex can bind to the promoters of ldh and frdA (Figure 6A). Previous studies reported that LDH and ADH are also Rex targets (Larsson et al., 2005; Pagels et al., 2010; Mehmeti et al., 2011). In S. aureus, Rex is intimately involved in the survival of cells exposed to NO. Because NO inhibits the activities of terminal respiratory oxidases, nitrate reductase, and pyruvate formate lyase, it leaves LDH, a target of Rex, as the major means of regenerating NAD+ (Pagels et al., 2010). LDH enables the bacteria to resist host innate immunity, which means that modulating Rex function appropriately is a critical factor in the pathogenesis of S. aureus (Richardson et al., 2008). In S. pneumoniae, LDH, which is a key enzyme for pyruvate metabolism, was reported to be important for pneumococcal survival in blood, metabolism, and virulence (Gaspar et al., 2014). In SS2, 6PGD was found to be a good adhesin, and it induced protective immune responses in both mice and piglets (Tan et al., 2008, 2009).
During infection, phagocytic cells play a pivotal role against invading pathogens. The process of phagocytic killing begins with the engulfment of bacteria by endocytosis into phagosomes, which are then fused with lysosomes to form phagolysosomes (Chen et al., 2008). The formation of phagolysosomes, a hostile environment with various reactive oxygen species (ROS) and a lower pH, is essential for the intracellular destruction of pathogens. In our studies, Δrex displayed defects in its ability to adapt to oxidative and acidic environmental conditions and had a decreased level of survival in macrophages. Similar results have been reported in other pathogens; for example, Rex-deficiency in S. mutans causes increased sensitivity to exogenous H2O2-mediated killing (Bitoun et al., 2012). In E. faecalis, a Rex-deficient mutant had an impaired ability to cope with oxidative stress (Mehmeti et al., 2011; Vesić and Kristich, 2013). Here, our iTRAQ results showed the downregulation of several proteins (such as Dpr, IlvE) that are involved in the stress response in Δrex; these might be partly responsible for the phenotypes of defective growth under conditions of oxidative and acidic stresses. Dpr is especially crucial for the H2O2 resistance of SS2, and inactivation of dpr led to a nearly complete loss of the SS2 H2O2 defensive capability (Zhang et al., 2012). The EMSAs also revealed that rSsRex can bind to the promoters of dpr (Figure 6A), suggesting that Rex may directly regulate oxidative stress response of SS2. In S. mutans, IlvE was upregulated in a proteome analysis under acidic stress conditions, and a deletion of ilvE caused a decrease in acid tolerance (Santiago et al., 2012). In SS2, a group of global regulators such as Spx proteins (Zheng et al., 2014), SalK/SalR (Li et al., 2008), Ihk/Irr (Han et al., 2012) were positively related to oxidative stress tolerance of bacterial, these genes mutants exhibited impaired growth in the presence of H2O2 in vitro and significantly decreased survival in vivo. These findings suggest that the Rex regulator in SS2 might not only be responsible for the defective growth of the mutant strain under stress conditions, but also facilitate the survival of SS2 within the host.
In our study, we found that a Rex mutation made the SS2 more susceptible to acidic and oxidative environments and easily to be eliminated in whole blood. However, there was no significant difference between mutant strain Δrex and complemented strain CΔrex in the resistance to an H2O2 environment (for a 30-min incubation) and the whole blood environment. It is interesting that the complementation of Δrex was able to restore the WT phenotype in the acid stress assay, but, it was not able to rescue the mutant phenotypes in the oxidative stress assay (for a 30-min incubation) or in the blood survival assay. Similar observations have been reported in other studies (Behlau and Miller, 1993; Ju et al., 2012; Mcgillivray et al., 2014; Lewis et al., 2015). The most likely reasons are that (i) Rex functional complementation is mediated by a plasmid, which might be different from complementing the target genes into the genome of bacteria (Dortet et al., 2011; Lewis et al., 2015). A previous study reported that the plasmid-mediated complementation for Rv2745c gene in Mycobacterium tuberculosis resulted in either a partial or, a delayed complementation of the phenotypes (Mcgillivray et al., 2014), and (ii) the transcription level of rex and/or that of some genes within Rex regulon of the complemented strain CΔrex may occur within a few microenvironments (such as in whole blood), which might be due to a secondary response of genes repression. Further researches are needed to identify that the genes expression patterns under Rex regulation in whole blood and the regulatory elements within Rex regulation networks that impact expression of rex at different environmental conditions.
In summary, bioinformatic, mutational, and proteomic analyses as well as EMSAs were used to identify and characterize the Rex regulator of SS2. The present study has clearly demonstrated that SsRex is an authentic Rex transcriptional factor based on the following evidence: (i) it was able to bind to promoter fragments in vitro, indicating that, at least in some cases, the effect of Rex is mediated by direct interaction with promoters such as those for Rex, ArcA, and AdhE, and (ii) it impacted the cellular metabolism, oxidative stress tolerance, and virulence, as previously reported for Rex homolog proteins in other pathogens (Pagels et al., 2010; Bitoun et al., 2012; Laouami et al., 2014; Bitoun and Wen, 2016). We also demonstrated that the Rex mutant strain showed a reduced ability to colonize host tissues and a decreased survival in whole blood or following phagocytosis, which partially explains the attenuated bacterial pathogenicity of the Rex mutant strain in animal models. Further investigations of the Rex-related protein expression patterns under in vivo infection conditions or host environment mimics are needed; however, our study provides novel insights into the requirement for Rex in the pathogenesis of SS2 infection.
Author Contributions
HZ, YW, KH conceived and designed the experiments. HZ, YW, LH performed the experiments. HZ, YW, JZ analyzed the data. YN, ZY, DW, AM, HF contributed reagents materials analysis tools. HZ, YW, KH wrote the paper. All authors read, advised, and approved the final manuscript.
Funding
This work was supported by the National Natural Sciences Foundation of China (31302114), the Special Fund for Public Welfare Industry of Chinese Ministry of Agriculture (201303041), the National Transgenic Major Program (2014ZX0800946B), the Innovation of Agricultural Sciences in Jiangsu province (CX(14)5042). The funders had no role in study design, data collection and analysis, decision to publish, or preparation of the manuscript.
Conflict of Interest Statement
The authors declare that the research was conducted in the absence of any commercial or financial relationships that could be construed as a potential conflict of interest.
Acknowledgments
We are grateful to Dr. Takamatsu and colleagues (National Institute of Animal Health, Japan) for supplying the plasmid pSET4s and their precious guidance for correctly using this plasmid. Comparative proteomics were performed with the help of Wuhan GeneCreate Biological Engineering Co., Ltd. We thank Katie Oakley, PhD, from Liwen Bianji, Edanz Editing China (www.liwenbianji.cn/ac), for editing the English text of a draft of this manuscript.
Supplementary Material
The Supplementary Material for this article can be found online at: https://www.frontiersin.org/articles/10.3389/fcimb.2018.00317/full#supplementary-material
References
Agarwal, V., Kuchipudi, A., Fulde, M., Riesbeck, K., Bergmann, S., and Blom, A. M. (2013). Streptococcus pneumoniae endopeptidase O (PepO) is a multifunctional plasminogen- and fibronectin-binding protein, facilitating evasion of innate immunity and invasion of host cells. J. Biol. Chem. 288, 6849–6863. doi: 10.1074/jbc.M112.405530
Agarwal, V., Sroka, M., Fulde, M., Bergmann, S., Riesbeck, K., and Blom, A. M. (2014). Binding of Streptococcus pneumoniae endopeptidase O (PepO) to complement component C1q modulates the complement attack and promotes host cell adherence. J. Biol. Chem. 289, 15833–15844. doi: 10.1074/jbc.M113.530212
Aranda, J., Garrido, M. E., Fittipaldi, N., Cortes, P., Llagostera, M., Gottschalk, M., et al. (2010). The cation-uptake regulators AdcR and Fur are necessary for full virulence of Streptococcus suis. Vet Microbiol. 144, 246–249. doi: 10.1016/j.vetmic.2009.12.037
Behlau, I., and Miller, S. I. (1993). A PhoP-repressed gene promotes Salmonella typhimurium invasion of epithelial cells. J. Bacteriol. 175, 4475–4484. doi: 10.1128/jb.175.14.4475-4484.1993
Bitoun, J. P., Liao, S., Yao, X., Xie, G. G., and Wen, Z. T. (2012). The redox-sensing regulator Rex modulates central carbon metabolism, stress tolerance response and biofilm formation by Streptococcus mutans. PLoS ONE 7:e44766. doi: 10.1371/journal.pone.0044766
Bitoun, J. P., and Wen, Z. T. (2016). Transcription factor Rex in regulation of pathophysiology in oral pathogens. Mol. Oral Microbiol. 31, 115–124. doi: 10.1111/omi.12114
Brekasis, D., and Paget, M. S. (2003). A novel sensor of NADH/NAD+ redox poise in Streptomyces coelicolor A3(2). EMBO J. 22, 4856–4865. doi: 10.1093/emboj/cdg453
Chen, L., Yang, J., Yu, J., Yao, Z., Sun, L., Shen, Y., et al. (2005). VFDB: a reference database for bacterial virulence factors. Nucleic Acids Res. 33, D325–D328. doi: 10.1093/nar/gki008
Chen, Z. H., Kim, H. P., Sciurba, F. C., Lee, S. J., Feghali-Bostwick, C., Stolz, D. B., et al. (2008). Egr-1 regulates autophagy in cigarette smoke-induced chronic obstructive pulmonary disease. PLoS ONE 3:e3316. doi: 10.1371/journal.pone.0003316
Cheng, C., Wang, X., Dong, Z., Shao, C., Yang, Y., Fang, W., et al. (2015). Aminopeptidase T of M29 family acts as a novel intracellular virulence factor for Listeria Monocytogenes infection. Sci. Rep. 5:17370. doi: 10.1038/srep17370
Choi, E., Kwon, K., and Lee, E. J. (2015). A single amino acid of a Salmonella virulence protein contributes to pathogenicity by protecting from the FtsH-mediated proteolysis. FEBS Lett. 589, 1346–1351. doi: 10.1016/j.febslet.2015.04.014
Connolly, J., Boldock, E., Prince, L. R., Renshaw, S. A., Whyte, M. K., and Foster, S. J. (2017). Identification of Staphylococcus aureus Factors required for pathogenicity and growth in human blood. Infect. Immun. 85, e00337–e00317. doi: 10.1128/IAI.00337-17
Cumley, N. J., Smith, L. M., Anthony, M., and May, R. C. (2012). The CovS/CovR acid response regulator is required for intracellular survival of group B Streptococcus in macrophages. Infect. Immun. 80, 1650–1661. doi: 10.1128/IAI.05443-11
Dortet, L. S., Mostowy, A., Samba-Louaka, E., Gouin, M. A., Nahori, E. A., Wiemer, E. A., et al. (2011). Recruitment of the major vault protein by InlK: a Listeria monocytogenes strategy to avoid autophagy. PLoS Pathog 7:e1002168. doi: 10.1371/annotation/a70544fc-6d8b-4549-921a-9e86557b0ffc
Fang, L., Fan, P., Yang, Y., Zhou, J., Shen, H., and Fang, W. (2017). A serine/threonine phosphatase 1 of Streptococcus suis type 2 is an important virulence factor. J. Vet. Sci. 18, 439–447. doi: 10.4142/jvs.2017.18.4.439
Feng, L., Zhu, J., Chang, H., Gao, X., Gao, C., Wei, X., et al. (2016). The CodY regulator is essential for virulence in Streptococcus suis serotype 2. Sci. Rep. 6:21241. doi: 10.1038/srep21241
Fittipaldi, N., Segura, M., Grenier, D., and Gottschalk, M. (2012). Virulence factors involved in the pathogenesis of the infection caused by the swine pathogen and zoonotic agent Streptococcus suis. Future Microbiol. 7, 259–279. doi: 10.2217/fmb.11.149
Gaspar, P., Al-Bayati, F. A., Andrew, P. W., Neves, A. R., and Yesilkaya, H. (2014). Lactate dehydrogenase is the key enzyme for pneumococcal pyruvate metabolism and pneumococcal survival in blood. Infect. Immun. 82, 5099–5109. doi: 10.1128/IAI.02005-14
Goyette-Desjardins, G., Auger, J. P., Xu, J., Segura, M., and Gottschalk, M. (2014). Streptococcus suis, an important pig pathogen and emerging zoonotic agent-an update on the worldwide distribution based on serotyping and sequence typing. Emerg. Microbes Infect. 3:e45. doi: 10.1038/emi.2014.45
Gyan, S., Shiohira, Y., Sato, I., Takeuchi, M., and Sato, T. (2006). Regulatory loop between redox sensing of the NADH/NAD(+) ratio by Rex (YdiH) and oxidation of NADH by NADH dehydrogenase Ndh in Bacillus subtilis. J. Bacteriol. 188, 7062–7071. doi: 10.1128/JB.00601-06
Han, H., Liu, C., Wang, Q., Xuan, C., Zheng, B., Tang, J., et al. (2012). The two-component system Ihk/Irr contributes to the virulence of Streptococcus suis serotype 2 strain 05ZYH33 through alteration of the bacterial cell metabolism. Microbiology 158, 1852–1866. doi: 10.1099/mic.0.057448-0
Hill, J. E., Gottschalk, M., Brousseau, R., Harel, J., Hemmingsen, S. M., and Goh, S. H. (2005). Biochemical analysis, cpn60 and 16S rDNA sequence data indicate that Streptococcus suis serotypes 32 and 34, isolated from pigs, are Streptococcus orisratti. Vet. Microbiol. 107, 63–69. doi: 10.1016/j.vetmic.2005.01.003
Hu, L., Huang, H., Yuan, H., Tao, F., Xie, H., and Wang, S. (2016). Rex in Clostridium kluyveri is a global redox-sensing transcriptional regulator. J. Biotechnol. 233, 17–25. doi: 10.1016/j.jbiotec.2016.06.024
Jiang, X., Yang, Y., Zhou, J., Zhu, L., Gu, Y., Zhang, X., et al. (2016). Roles of the putative type IV-like secretion system key component VirD4 and PrsA in pathogenesis of Streptococcus suis type 2. Front. Cell Infect. Microbiol. 6:172. doi: 10.3389/fcimb.2016.00172
Jing, H. B., Yuan, J., Wang, J., Yuan, Y., Zhu, L., Liu, X. K., et al. (2008). Proteome analysis of Streptococcus suis serotype 2. Proteomics 8, 333–349. doi: 10.1002/pmic.200600930
Ju, C. X., Gu, H. W., and Lu, C. P. (2012). Characterization and functional analysis of atl, a novel gene encoding autolysin in Streptococcus suis. J. Bacteriol. 194, 1464–1473. doi: 10.1128/JB.06231-11
Kwon, H. Y., Kim, S. W., Choi, M. H., Ogunniyi, A. D., Paton, J. C., Park, S. H., et al. (2003). Effect of heat shock and mutations in ClpL and ClpP on virulence gene expression in Streptococcus pneumoniae. Infect. Immun. 71, 3757–3765. doi: 10.1128/IAI.71.7.3757-3765.2003
Laouami, S., Clair, G., Armengaud, J., and Duport, C. (2014). Proteomic evidences for rex regulation of metabolism in toxin-producing Bacillus cereus ATCC 14579. PLoS ONE 9:e107354. doi: 10.1371/journal.pone.0107354
Larsson, J. T., Rogstam, A., and Von Wachenfeldt, C. (2005). Coordinated patterns of cytochrome bd and lactate dehydrogenase expression in Bacillus subtilis. Microbiology 151, 3323–3335. doi: 10.1099/mic.0.28124-0
Lewis, A. M., Matzdorf, S. S., Endres, J. L., Windham, I. H., Bayles, K. W., and Rice, K. C. (2015). Examination of the Staphylococcus aureus nitric oxide reductase (saNOR) reveals its contribution to modulating intracellular NO levels and cellular respiration. Mol. Microbiol. 96, 651–669. doi: 10.1111/mmi.12962
Li, J., Tan, C., Zhou, Y., Fu, S., Hu, L., Hu, J., et al. (2011). The two-component regulatory system CiaRH contributes to the virulence of Streptococcus suis 2. Vet. Microbiol. 148, 99–104. doi: 10.1016/j.vetmic.2010.08.005
Li, M., Wang, C., Feng, Y., Pan, X., Cheng, G., Wang, J., et al. (2008). SalK/SalR, a two-component signal transduction system, is essential for full virulence of highly invasive Streptococcus suis serotype 2. PLoS ONE 3:e2080. doi: 10.1371/journal.pone.0002080
Li, Y., Zheng, J. X., Zhang, H., Fan, H. J., and Lu, C. P. (2014). Effect of Tran on virulence through regulating metabolism and stress tolerance of Streptococcus suis serotype 2. Microbiol. Res. 169, 666–674. doi: 10.1016/j.micres.2014.03.002
Lin, X., Lin, L., Yao, Z., Li, W., Sun, L., Zhang, D., et al. (2015). An integrated quantitative and targeted proteomics reveals fitness mechanisms of Aeromonas hydrophila under oxytetracycline stress. J. Proteome Res. 14, 1515–1525. doi: 10.1021/pr501188g
Liu, X., Cheng, Y., Lyu, M., Wen, Y., Song, Y., Chen, Z., et al. (2017). Redox-sensing regulator Rex regulates aerobic metabolism, morphological differentiation, and avermectin production in Streptomyces avermitilis. Sci. Rep. 7:44567. doi: 10.1038/srep44567
Livak, K. J., and Schmittgen, T. D. (2001). Analysis of relative gene expression data using real-time quantitative PCR and the 2(-delta delta C(T)) method. Methods 25, 402–408. doi: 10.1006/meth.2001.1262
Lun, Z. R., Wang, Q. P., Chen, X. G., Li, A. X., and Zhu, X. Q. (2007). Streptococcus suis: an emerging zoonotic pathogen. Lancet Infect. Dis. 7, 201–209. doi: 10.1016/S1473-3099(07)70001-4
Mcgillivray, A., Golden, N. A., Gautam, U. S., Mehra, S., and Kaushal, D. (2014). The Mycobacterium tuberculosis Rv2745c plays an important role in responding to redox stress. PLoS ONE 9:e93604. doi: 10.1371/journal.pone.0093604
Mclaughlin, K. J., Strain-Damerell, C. M., Xie, K., Brekasis, D., Soares, A. S., Paget, M. S., et al. (2010). Structural basis for NADH/NAD+ redox sensing by a Rex family repressor. Mol. Cell 38, 563–575. doi: 10.1016/j.molcel.2010.05.006
Mehmeti, I., Jonsson, M., Fergestad, E. M., Mathiesen, G., Nes, I. F., and Holo, H. (2011). Transcriptome, proteome, and metabolite analyses of a lactate dehydrogenase-negative mutant of Enterococcus faecalis V583. Appl. Environ. Microbiol. 77, 2406–2413. doi: 10.1128/AEM.02485-10
Pagels, M., Fuchs, S., Pane-Farre, J., Kohler, C., Menschner, L., Hecker, M., et al. (2010). Redox sensing by a Rex-family repressor is involved in the regulation of anaerobic gene expression in Staphylococcus aureus. Mol. Microbiol. 76, 1142–1161. doi: 10.1111/j.1365-2958.2010.07105.x
Pei, J. Q., Zhou, Q., Jing, L., Li, C., Dai, H., Li, H., et al. (2011).The mechanism for regulating ethanol fermentation by redox levels in Thermoanaerobacter ethanolicus. Metab. Eng. 13, 186–193. doi: 10.1016/j.ymben.2010.12.006
Redding, A. M., Mukhopadhyay, A., Joyner, D. C., Hazen, T. C., and Keasling, J. D. (2006). Study of nitrate stress in Desulfovibrio vulgaris Hildenborough using iTRAQ proteomics. Brief Funct. Genomic Proteomic 5, 133–143. doi: 10.1093/bfgp/ell025
Reed Lj, M. H. (1938). A simple method of estimating fifty percent endpoints. Am. J. Hyg. 27, 493–497.
Richardson, A. R., Libby, S. J., and Fang, F. C. (2008). A nitric oxide-inducible lactate dehydrogenase enables Staphylococcus aureus to resist innate immunity. Science 319, 1672–1676. doi: 10.1126/science.1155207
Richardson, A. R., Somerville, G. A., and Sonenshein, A. L. (2015). Regulating the intersection of metabolism and pathogenesis in gram-positive bacteria. Microbiol. Spectr. 3:MBP-0004–2014. doi: 10.1128/microbiolspec.MBP-0004-2014
Santiago, B., Macgilvray, M., Faustoferri, R. C., and Quivey, R. G. Jr. (2012). The branched-chain amino acid aminotransferase encoded by ilvE is involved in acid tolerance in Streptococcus mutans. J. Bacteriol. 194, 2010–2019. doi: 10.1128/JB.06737-11
Segura, M., Fittipaldi, N., Calzas, C., and Gottschalk, M. (2017). Critical Streptococcus suis virulence factors: are they all really critical? Trends Microbiol. 25, 585–599. doi: 10.1016/j.tim.2017.02.005
Shen, X., Zhong, Q., Zhao, Y., Yin, S., Chen, T., Hu, F., et al. (2013). Proteome analysis of the two-component SalK/SalR system in epidemic Streptococcus suis serotype 2. Curr. Microbiol. 67, 118–122. doi: 10.1007/s00284-013-0343-4
Takamatsu, D., Osaki, M., and Sekizaki, T. (2001a). Construction and characterization of Streptococcus suis-Escherichia coli shuttle cloning vectors. Plasmid 45, 101–113. doi: 10.1006/plas.2000.1510
Takamatsu, D., Osaki, M., and Sekizaki, T. (2001b). Thermosensitive suicide vectors for gene replacement in Streptococcus suis. Plasmid 46, 140–148. doi: 10.1006/plas.2001.1532
Tan, C., Fu, S., Liu, M., Jin, M., Liu, J., Bei, W., et al. (2008). Cloning, expression and characterization of a cell wall surface protein, 6-phosphogluconate-dehydrogenase, of Streptococcus suis serotype 2. Vet. Microbiol. 130, 363–370. doi: 10.1016/j.vetmic.2008.02.025
Tan, C., Liu, M., Liu, J., Yuan, F., Fu, S., Liu, Y., et al. (2009). Vaccination with Streptococcus suis serotype 2 recombinant 6PGD protein provides protection against S. suis infection in swine. FEMS Microbiol. Lett. 296, 78–83. doi: 10.1111/j.1574-6968.2009.01617.x
Tang, J., Wang, C., Feng, Y., Yang, W., Song, H., Chen, Z., et al. (2006). Streptococcal toxic shock syndrome caused by Streptococcus suis serotype 2. PLoS Med. 3:e151. doi: 10.1371/journal.pmed.0030151
Tang, Y., Zhang, X., Wu, W., Lu, Z., and Fang, W. (2012). Inactivation of the sodA gene of Streptococcus suis type 2 encoding superoxide dismutase leads to reduced virulence to mice. Vet. Microbiol. 158, 360–366. doi: 10.1016/j.vetmic.2012.02.028
Unwin, R. D., Griffiths, J. R., and Whetton, A. D. (2010). Simultaneous analysis of relative protein expression levels across multiple samples using iTRAQ isobaric tags with 2D nano LC-MS/MS. Nat. Protoc. 5, 1574–1582. doi: 10.1038/nprot.2010.123
Vesić, D., and Kristich, C. J. (2013). A Rex family transcriptional repressor influences H2O2 accumulation by Enterococcus faecalis. J. Bacteriol. 195, 1815–1824. doi: 10.1128/JB.02135-12
Wang, E., Bauer, M. C., Rogstam, A., Linse, S., Logan, D. T., and Von Wachenfeldt, C. (2008). Structure and functional properties of the Bacillus subtilis transcriptional repressor Rex. Mol. Microbiol. 69, 466–478. doi: 10.1111/j.1365-2958.2008.06295.x
Wang, E., Ikonen, T. P., Knaapila, M., Svergun, D., Logan, D. T., and Von Wachenfeldt, C. (2011). Small-angle X-ray scattering study of a Rex family repressor: conformational response to NADH and NAD+ binding in solution. J. Mol. Biol. 408, 670–683. doi: 10.1016/j.jmb.2011.02.050
Willenborg, J., De Greeff, A., Jarek, M., Valentin-Weigand, P., and Goethe, R. (2014). The CcpA regulon of Streptococcus suis reveals novel insights into the regulation of the streptococcal central carbon metabolism by binding of CcpA to two distinct binding motifs. Mol. Microbiol. 92, 61–83. doi: 10.1111/mmi.12537
Willenborg, J., Fulde, M., De Greeff, A., Rohde, M., Smith, H. E., Valentin-Weigand, P. et al. (2011). Role of glucose and CcpA in capsule expression and virulence of Streptococcus suis. Microbiology 157, 1823–1833. doi: 10.1099/mic.0.046417-0
Wilson, T. L., Jeffers, J., Rapp-Gabrielson, V. J., Martin, S., Klein, L. K., Lowery, D. E., et al. (2007). A novel signature-tagged mutagenesis system for Streptococcus suis serotype 2. Vet. Microbiol. 122, 135–145. doi: 10.1016/j.vetmic.2006.12.025
Xu, B., Zhang, P., Li, W., Liu, R., Tang, J., and Fan, H. (2017). hsdS, Belonging to the type I restriction-modification system, contributes to the Streptococcus suis serotype 2 survival ability in phagocytes. Front. Microbiol. 8:1524. doi: 10.3389/fmicb.2017.01524
Xu, J., Fu, S., Liu, M., Xu, Q., Bei, W., Chen, H., et al. (2014). The two-component system NisK/NisR contributes to the virulence of Streptococcus suis serotype 2. Microbiol. Res. 169, 541–546. doi: 10.1016/j.micres.2013.11.002
Yu, H., Jing, H., Chen, Z., Zheng, H., Zhu, X., Wang, H., et al. (2006). Human Streptococcus suis outbreak, Sichuan, China. Emerg. Infect. Dis. 12, 914–920. doi: 10.3201/eid1206.051194
Yu, Y., Qian, Y., Du, D., Li, Q., Xu, C., Liu, H., et al. (2018). Infection and adaption-based proteomic changes of Streptococcus suis serotype 2 in a pig model. J. Proteomics 180, 41–52. doi: 10.1016/j.jprot.2017.12.001
Yu, Y., Qian, Y., Du, D., Xu, C., Dai, C., Li, Q., et al. (2016). SBP2 plays an important role in the virulence changes of different artificial mutants of Streptococcus suis. Mol. Biosyst. 12, 1948–1962. doi: 10.1039/C6MB00059B
Zhang, C., Sun, W., Tan, M., Dong, M., Liu, W., Gao, T., et al. (2017). The eukaryote-like serine/threonine kinase STK regulates the growth and metabolism of zoonotic Streptococcus suis. Front. Cell Infect. Microbiol. 7:66. doi: 10.3389/fcimb.2017.00066
Zhang, L., Nie, X., Ravcheev, D. A., Rodionov, D. A., Sheng, J., Gu, Y., et al. (2014). Redox-responsive repressor Rex modulates alcohol production and oxidative stress tolerance in Clostridium acetobutylicum. J. Bacteriol. 196, 3949–3963. doi: 10.1128/JB.02037-14
Zhang, T., Ding, Y., Li, T., Wan, Y., Li, W., Chen, H., et al. (2012). A Fur-like protein PerR regulates two oxidative stress response related operons dpr and metQIN in Streptococcus suis. BMC Microbiol. 12:85. doi: 10.1186/1471-2180-12-85
Zheng, C., Xu, J., Li, J., Hu, L., Xia, J., Fan, J., et al. (2014). Two Spx regulators modulate stress tolerance and virulence in Streptococcus suis serotype 2. PLoS ONE 9:e108197. doi: 10.1371/journal.pone.0108197
Zheng, F., Ji, H., Cao, M., Wang, C., Feng, Y., Li, M., et al. (2011). Contribution of the Rgg transcription regulator to metabolism and virulence of Streptococcus suis serotype 2. Infect. Immun. 79, 1319–1328. doi: 10.1128/IAI.00193-10
Zhou, J., Zhang, X., He, K., Wang, W., Ni, Y., Zhu, H., et al. (2014). Characterization and proteome analysis of inosine 5-monophosphate dehydrogenase in epidemic Streptococcus suis serotype 2. Curr. Microbiol. 68, 663–669. doi: 10.1007/s00284-014-0527-6
Zhu, H., Huang, D., Zhang, W., Wu, Z., Lu, Y., Jia, H., et al. (2011). The novel virulence-related gene stp of Streptococcus suis serotype 9 strain contributes to a significant reduction in mouse mortality. Microb. Pathog. 51, 442–453. doi: 10.1016/j.micpath.2011.08.002
Keywords: Streptococcus suis, redox-sensing regulator Rex, virulence, oxidative stress, electrophoretic mobility shift assays
Citation: Zhu H, Wang Y, Ni Y, Zhou J, Han L, Yu Z, Mao A, Wang D, Fan H and He K (2018) The Redox-Sensing Regulator Rex Contributes to the Virulence and Oxidative Stress Response of Streptococcus suis Serotype 2. Front. Cell. Infect. Microbiol. 8:317. doi: 10.3389/fcimb.2018.00317
Received: 10 April 2018; Accepted: 20 August 2018;
Published: 18 September 2018.
Edited by:
Justin Merritt, Oregon Health and Science University, United StatesReviewed by:
Zezhang Tom Wen, LSU Health Sciences Center New Orleans, United StatesJessica Kajfasz, University of Florida, United States
Copyright © 2018 Zhu, Wang, Ni, Zhou, Han, Yu, Mao, Wang, Fan and He. This is an open-access article distributed under the terms of the Creative Commons Attribution License (CC BY). The use, distribution or reproduction in other forums is permitted, provided the original author(s) and the copyright owner(s) are credited and that the original publication in this journal is cited, in accordance with accepted academic practice. No use, distribution or reproduction is permitted which does not comply with these terms.
*Correspondence: Kongwang He, a3doMjAwM0AyNjMubmV0
†These authors have contributed equally to the work