- 1Laboratório de Pesquisa em Malária, Instituto Oswaldo Cruz, Fundação Oswaldo Cruz (Fiocruz), Rio de Janeiro, Brazil
- 2Instituto de Ciência e Tecnologia em Biomodelos, Fiocruz, Rio de Janeiro, Brazil
- 3Laboratório de Patologia, Instituto Oswaldo Cruz, Fiocruz, Rio de Janeiro, Brazil
- 4Vac4All Initiative, Pepinière Paris Biotech Santé, Paris, France
A major constraint in the study of Plasmodium falciparum malaria, including vaccine development, lies on the parasite's strict human host specificity and therefore the shortage of animal experimental models able to harbor human plasmodia. The best experimental models are neo-tropical primates of the genus Saimiri and Aotus, but they require splenectomy to reduce innate defenses for achieving high and consistent parasitemias, an important limitation. Clodronate-liposomes (CL) have been successfully used to deplete monocytes/macrophages in several experimental models. We investigated whether a reduction in the numbers of phagocytic cells by CL would improve the development of P. falciparum parasitemia in non-splenectomized Saimiri sciureus monkeys. Depletion of S. sciureus splenocytes after in vitro incubation with CL was quantified using anti-CD14 antibodies and flow cytometry. Non-infected and P. falciparum-infected S. sciureus were injected intravenously twice a week with either CL at either 0.5 or 1 mL (5 mg/mL) or phosphate buffered saline (PBS). Animals were monitored during infection and treated with mefloquine. After treatment and euthanasia, spleen and liver were collected for histological analysis. In vitro CL depleted S. sciureus splenic monocyte/macrophage population in a dose- and time-dependent manner. In vivo, half of P. falciparum-infected S. sciureus treated with CL 0.5 mL, and two-thirds of those treated with CL 1 mL developed high parasitemias requiring mefloquine treatment, whereas all control animals were able to self-control parasitemia without the need for antimalarial treatment. CL-treated infected S. sciureus showed a marked decrease in the degree of splenomegaly despite higher parasitemias, compared to PBS-treated animals. Histological evidence of partial monocyte/macrophage depletion, decreased hemozoin phagocytosis and decreased iron recycling was observed in both the spleen and liver of CL-treated infected S. sciureus. CL is capable of promoting higher parasitemia in P. falciparum-infected S. sciureus, associated with evidence of partial macrophage depletion in the spleen and liver. Macrophage depletion by CL is therefore a practical and viable alternative to surgical splenectomy in this experimental model.
Introduction
According to the World Health Organization (WHO) estimates, 212 million cases of malaria occurred in 2015, with 429,000 deaths (WHO, 2016). Among the five Plasmodium species capable of causing human malaria, Plasmodium falciparum is associated with more severe and lethal forms of the disease (Rowe et al., 2009; Quintero et al., 2011). Due to increasing parasite resistance to currently available antimalarial drugs (Chrubasik and Jacobson, 2010; Dondorp et al., 2010), development of an effective vaccine against malaria is urgently needed. Currently, one of the promising vaccine candidates (RTS,S/AS01) is being tested in a Phase III clinical trial in Africa in order to inform a decision regarding its deployment (Clemens and Moorthy, 2016; Olotu et al., 2016; Otieno et al., 2016). Another candidate, the MSP3 experimental vaccine, had a promising performance in African children in a double-blind follow-up of a preliminary Phase I study (Sirima et al., 2011). However, there is no assurance that these candidates will become indeed effective vaccines in the near future. Continued evaluation of potential vaccines is therefore expected and necessary.
Animal models for pre-clinical studies are an important component in vaccine development, but the limited availability of experimental models that can harbor human malaria is a critical constraint. The neo-tropical primates of the genus Saimiri and Aotus are experimental models recommended by WHO for pre-clinical testing of malaria vaccine candidates (WHO, 2004). Studies using the Saimiri sciureus model showed that immunization against P. falciparum blood stage vaccine candidate antigens such as the glutamate-rich protein (GLURP), the merozoite surface protein-3 (MSP3), or the SE36 antigen, using different adjuvants, may induce potent antibody responses and elicit partially protective immunity upon challenge (Carvalho et al., 2004, 2005; Tougan et al., 2013). Besides being susceptible to P. vivax and to P. falciparum infection (Gysin and Fandeur, 1983; Carvalho et al., 2000, 2002, 2004, 2005; Contamin et al., 2000; Herrera et al., 2002; Collins et al., 2005), these primates are abundant in nature and can be easily handled in captivity due to their relatively small size. Furthermore, they are able to reproduce clinical and pathological manifestations of human malaria such as thrombocytopenia, changes in leukocyte counts and anemia, offering an appropriate model to study the immunopathogenesis of malaria (Contamin et al., 2000; Carvalho et al., 2003). The limited availability of immunological tools to study the immune response in these animals imposes some constraints, but human reagents can be used to some extent and specific Saimiri reagents have been generated (Garraud et al., 1998; Contamin et al., 2005; Alves et al., 2010; Riccio et al., 2015). However, these models also have disadvantages. One of the major constraints is the need for splenectomy to achieve high and consistent parasitemias (Collins, 1992). The immune response against the erythrocytic forms of the parasite is largely mediated by resident cells in the spleen (Criswell et al., 1971; Achtman et al., 2003; Leisewitz et al., 2004) and this organ has critical roles in the immune response during malarial infections. Therefore, radical surgical splenectomy poses a strong limitation for testing malaria vaccines, as the very immune responses the vaccines are intended to elicit may be affected by the intervention. Therefore, alternative approaches to allow increased P. falciparum parasitemias in S. sciureus without the need for surgical splenectomy are necessary for proper evaluation of potential vaccines.
Liposomes containing a toxic chemical induce macrophage “suicide,” depleteing phagocytes in specific tissues. Clodronate, a bisphosphonate drug that activates apoptosis, has been the most widely employed compound in this respect. It has been demonstrated in several animal models, including mice, dogs, and pigs, that macrophages ingest the liposome particles by phagocytosis and are destroyed or become functionally inactivated (van Rooijen and van Nieuwmegen, 1984; Mathes et al., 2006; Kim et al., 2008). The spleen is a site of important macrophage activity and destruction of blood-borne microorganisms including Plasmodium, senescent red blood cells, and clodronate-encapsulated liposomes (van Rooijen and van Nieuwmegen, 1984; van Rooijen and Kors, 1989; van Rooijen et al., 1990; van Rooijen and van Kesteren-Hendrikx, 2002; Abbas et al., 2008). Chemical “splenectomy” by clodronate liposomes is expected to induce higher P. falciparum parasitemias as it depletes monocyte/macrophages in large numbers while preserving spleen structure and functions. Indeed, in immunocompromised mice lacking B, T, and NK cells, P. falciparum growth was fully controlled by macrophages. Conversely, P. falciparum could successfully replicate at very high parasite densities for several weeks when macrophage populations were controlled by repeated administration of clodronate-encapsulated liposomes (Badell et al., 2000; Arnold et al., 2010, 2011). If the same system can be used in P. falciparum infections in S. sciureus monkeys, it can provide a much improved model for testing malaria vaccines. In such a system, the phagocytic activity of monocytes/macrophages would be decreased allowing increased parasitemia in naive animals, but it is expected that effective vaccines inducing protective adaptative immune responses, in a scenario of preserved spleen structure and function, will be able to contain parasite growth.
In this study, we investigated whether a decrease in the number of monocytes/macrophages by using clodronate-encapsulated liposomes would favor the development of consistent P. falciparum parasitemias in S. sciureus monkeys without the need for surgical splenectomy, through a “chemical splenectomy.” This procedure would also be more respectful of ethical considerations than surgery, because of its transient nature resulting in fast restitutio ad integrum.
Materials and Methods
Animals
The animals used in this study were male and female S. sciureus, karyotype 14-7, ranging in age from 3 to 19 years and in weight from 0.548 to 0.926 kg. The monkeys, non-splenectomized and malaria-naïve, were obtained from the breeding colony of the Primatology Service (CECAL/Fiocruz), Rio de Janeiro, Brazil. In total, 34 animals were used in three independent experiments (Table 1). This study was carried out in accordance with the recommendations and approved by the Fiocruz Ethics Committee on Animal Use (CEUA Licences L-0062/08 and LW-9/14).
Clodronate-Encapsulated Liposomes
Clodronate-encapsulated liposomes (CL, 5 mg/mL clodronate) were obtained from ClodronateLiposome.org (Department of Molecular Cell Biology and Immunology of the Vrije Universiteit Medisch Centrum - University Amsterdam, VUmc - Netherlands).
Evaluation of Clodronate-Liposome on Macrophages/Monocytes in Vitro
Cryopreserved Saimiri splenocytes obtained during splenectomy of three naïve animals were thawed, washed twice with pure RPMI medium (Gibco, Grand Island, New York, EUA), resuspended in RPMI supplemented with 10% fetal bovine serum (FBS, Invitrogen, Grand Island, New York, EUA) at a concentration of 5 × 105 cells/ 500 μL and incubated in 5 mL culture tubes (Falcon, BD Biosciences, San Jose, CA, EUA) in RPMI medium containing 15 mM glutamine (Gibco), 10 mM Hepes (Sigma-Aldrich, St. Louis, MO, EUA), 200 U/mL penicillin (Gibco), 200 μg/mL streptomycin (Gibco), 3 mg/mL gentamicin (Sigma-Aldrich) and 2 g/L sodium bicarbonate (Sigma-Aldrich) supplemented with 10% inactivated fetal bovine serum (Invitrogen). CL suspension was added at concentrations of 1, 10, and 100 μg/mL and incubated for 4, 8, 16, or 24 h at 37°C and 5% CO2. After culture, cells were labeled with anti-CD14-APC antibody (clone 61D3, eBioscience, Science Center Dr. San Diego, CA, EUA), a marker of macrophages and monocytes, and quantified by flow cytometry in the CyAn cell analyser (Dako Cytomation Inc., Carpinteria, CA, EUA) by counting 60,000 events. Data were analyzed using the software Summit (Dako Cytomation).
Evaluation of Clodronate-Liposome in Vivo
Plasmodium falciparum FUP strain (Carvalho et al., 2004) was recovered from cryostabilates and the parasitized red blood cells (pRBCs) were passaged by intravenous inoculation in a splenectomized donor monkey. pRBCs from the passage animal were used to infect the experimental group animals intravenously with an inoculum of 106 pRBC. The animals were divided into six groups, three with infected (Inf) animals and three control, non-infected (NInf) groups: (1) NInf receiving 1 mL of phosphate buffered saline (PBS - Sigma-Aldrich); (2) NInf receiving 0.5 mL of clodronate-encapsulated liposome (CL); (3) NInf receiving 1 mL of CL; (4) Inf receiving 1 mL of PBS; (5) Inf receiving 0.5 mL of CL; (6) Inf receiving 1 mL of CL. CL injections were performed intravenously two times a week, from day 0 of infection. Three independent experiments were performed using a total of 34 animals (Table 1).
The follow up of infection included a daily evaluation of parasitemia by thin Giemsa-stained blood films, hematocrit (microhematocrit method) and hemoglogin levels twice a week (using the HemoCue Hb301 system, HemoCue AB, Ängelholm, Sweden) as well as daily measurement of body (rectal) temperature. Blood monocyte count was done, twice a week starting from day 0 of infection, by flow cytometry using anti-CD14-APC antibody (clone 61D3, eBioscience) in a CyAn cell analyser (Dako Cytomation) and the data were analyzed using the software Summit (Dako Cytomation). An experienced veterinarian performed daily clinical examinations. Monkeys were treated with mefloquine (15 mg/kg—Biomanguinhos, Fiocruz, Rio de Janeiro, RJ, Brazil) when parasitemia reached 20% or above or in case the hematocrit reached 20% or below or when the monkeys presented manifestations of severe disease (prostration, anorexia). Monkeys that spontaneously controlled their parasitemia also received mefloquine on day 17 to ensure complete parasite clearance. Four days after mefloquine treatment, with animals no longer presenting patent parasitemia, all animals (including the uninfected controls) received a last injection of CL (or PBS) and were euthanized 24 h later. The animals were euthanized with 7 mg/kg thiopental (Cristália, Itapira, SP, Brazil) inoculated directly into the heart muscle, after being anesthesized with ketamine (Cristália) 100 mg/kg plus midazolam (União Química, Embu-Guaçu, SP, Brazil) 10 mg/kg. After the death of the animal, liver and spleen were harvested for histological analysis.
Spleen and Liver Analysis
Immediately after liver and spleen harvest, macroscopic evaluation was performed and the spleen was weighted. Samples of the liver and spleen were fixed using a 4% formaldehyde solution (Merk - Darmstadt, Germany) in Millonig's buffer (0.1 M sodium hydroxide, 0.13 M sodium phosphate monobasic—Sigma-Aldrich) and later processed for histology. Paraffin-embedded sections (5 μm) were stained with hematoxylin and eosin (HE), Giemsa or Prussian blue. The slides were analyzed with the aid of a microscope (AxioImager A2, Zeiss, Oberkochen, Germany) and images were captured using an Axiocam HRM (Zeiss) and the software AxionVision Release 4.8.2 (Zeiss).
For hemozoin quantitation in the spleen, the histology slices were dyed with Nuclear Fast Red and mounted with coverslips. Each slide was then completely scanned using VSlide (Metasystems, Germany), generating high definition images (0.8 NA) of all the slices. Because the original images were too big, they were divided into three parts for analysis, by using ImageJ, where the red (nuclei) and brown/black (hemozoin) colors were separated with the color threshold function. The hemozoin areas and the total tissue area in each image were measured in square pixels. These results were then used to estimate the relative hemozoin area in each slide.
Statistical Analysis
The significance of the differences between the results or means of all variables was examined by the nonparametric Kruskal-Wallis analysis followed by Dunn test (GraphPad Prism 6, GraphPad Software Inc., San Diego, CA, USA). Results were considered to be statistically significant when p < 0.05.
Results
Effect of Clodronate-Encapsulated Liposomes on S. sciureus Monocytes in Vitro
Even though CL has been used as a rapid inducer of apoptosis of monocytes/macrophages in vivo and in vitro, especially in mice, there are no available data on the effect of CL in S. sciureus monocytes. In vitro incubation of S. sciureus splenocytes with CL induced death of CD14+ cells (monocyte/macrophage) in a dose-dependent manner, with marked depletion at 100 μg/mL (Figure 1).
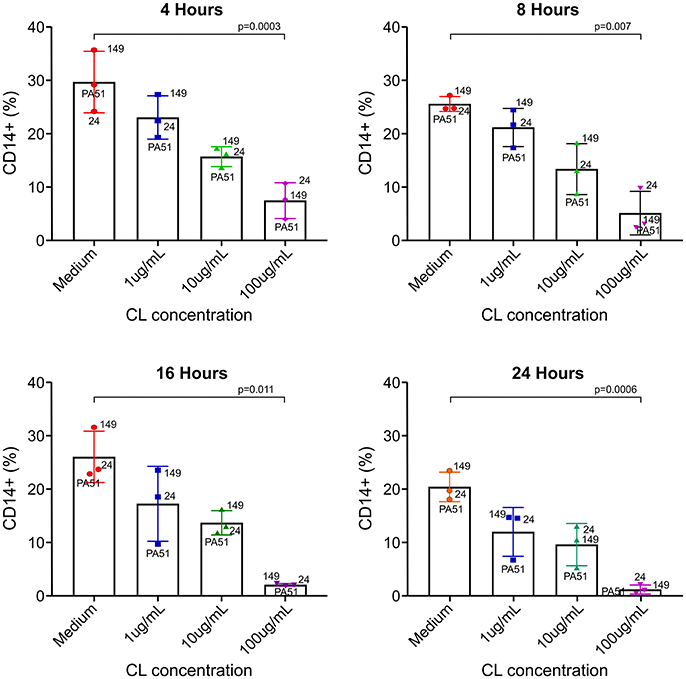
Figure 1. Effect of CL on S. sciureus splenocyte viability in vitro. CL induced dose-dependent cytotoxicity in monocytes/macrophages. 24, 149, and PA51 refer to S. sciureus identification. Medium vs. 100 μg/ml: 4 h (p = 0.0003), 8 h (p = 0.007), 16 h (p = 0.011), and 24 h (p = 0.0006). %: percentage of CD14+ cells in total number of splenocytes.
Effect of Clodronate-Encapsulated Liposomes on P. falciparum Infection in S. sciureus Monkeys
Parasitemia
Saimiri sciureus monkeys infected with P. falciparum and receiving PBS showed variable courses of parasitemia (Figure 2A). The maximum parasite density varied between 4.8 and 17.7% (peak average 8.1%). Most animals kept parasitemia below 10%, and one showed extremely low parasitemia throughout the follow up. The peak parasitemia was reached between days 11 and 15. All animals were able to control parasitemia without the need for antimalarial drug treatment. The infected animals receiving 0.5 mL CL also showed variable courses of parasitemia, which reached peak levels between days 10 and 15, with parasite densities ranging between 3.9 and 26.7% (average 16.4%) (Figure 2B). Of the six animals in this group, three (50%) required treatment with mefloquine due to high parasitemia (26.7, 19.5, and 26.7%). In the group of infected animals receiving 1 mL CL the maximum parasite density varied between 4.7 and 31.2% (average 18.5%) and peak parasitemia was reached between days 11 and 15. Of the nine animals in the group, six (66.6%) required antimalarial treatment due to high parasitemia (22; 26; 31.2; 25; 20, and 21.5%). Depending on the need for treatment and treatment time, animals received a minimum of four and a maximum of seven CL injections.
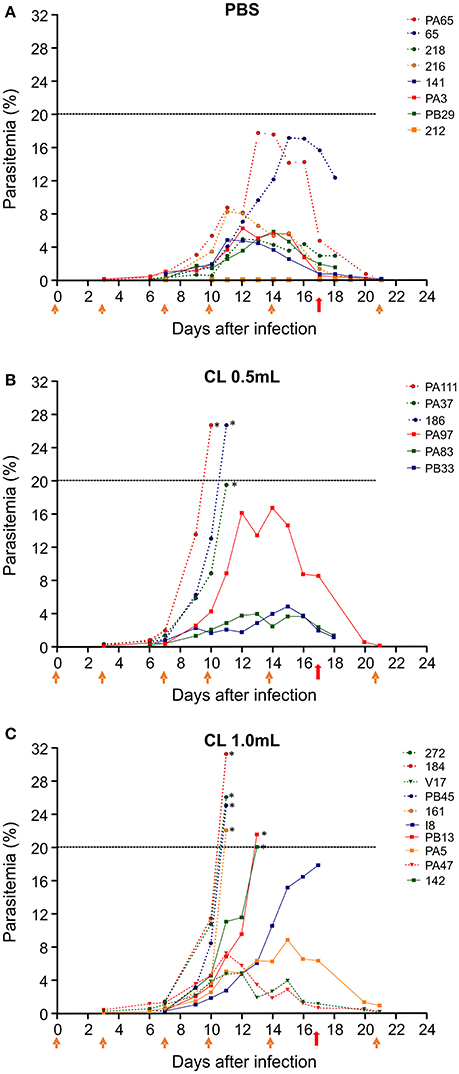
Figure 2. Course of parasitemia after infection of S. sciureus with 106 pRBC/mL by FUP strain of P. falciparum. Parasitemia is shown for animals that received PBS (controls) (A); clodronate liposomes (CL) 0.5 mL (B); or CL 1.0 mL (C). Data are from three separate experiments, with 6–9 animals per group. Orange arrows indicate the timepoints when PBS or CL was given to the animals. Red arrows indicate treatment with mefloquine. Dashed line represents the limit of parasitemia established for treatment (20%) with mefloquine. *Animals that received treatment with mefloquine before day 17 for reaching 20% parasitemia.
CD14+ Cell Count in Peripheral Blood
There was no apparent effect of P. falciparum infection or CL injections on circulating monocytes, as verified by CD14+ cell counts in peripheral blood (Figure 3).
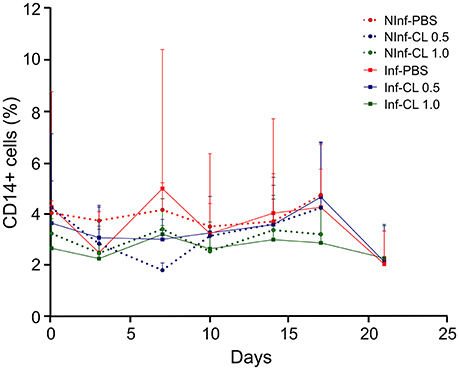
Figure 3. Changes in blood monocyte counts during P. falciparum infection and CL administration. Monocyte quantification was performed by flow cytometry using anti-CD14-APC antibodies. Solid lines represent the mean and standard deviation. %: percentage of CD14+ cells in total number of peripheral blood monocuclear cells (PBMC). NInf, non-infected control groups; Inf, P. falciparum-infected groups.
Anemia and Other Clinical Parameters
In P. falciparum-infected animals, hemoglobin concentration and hematocrit decreased reaching minimum values during or after parasite clearance (Figure 4). No weight loss greater than 10% was observed. Body temperature increased 1–2°C during infection and decreased after antimalarial treatment (data not shown). Some animals showed loss of appetite and prostration at the time of peak parasitemia. Two animals that received CL 1 mL and presented high parasitemias showed hematuria at the time of peak parasitemia that reversed after mefloquine treatment. Results of experiments 1, 2, and 3 showing the data of parasitemia, CD14 counting, hematocrit, hemoglobin and rectal temperature are shown in Supplemental Table 1.
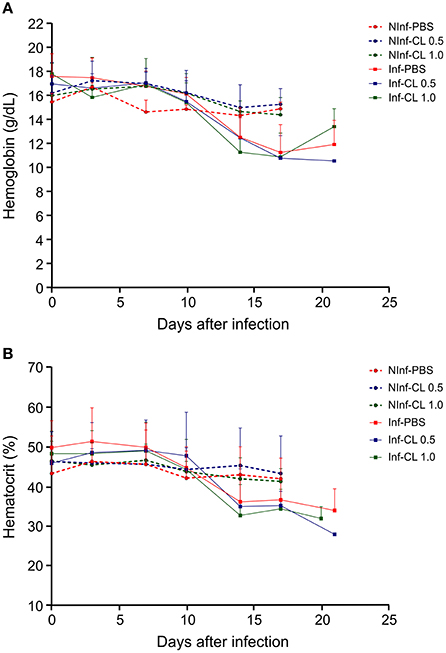
Figure 4. Hemoglobin concentration in the blood (A) and hematocrit (B) of S. sciureus. NInf, non-infected control groups; Inf, P. falciparum-infected groups. Solid lines represent the mean and standard deviation.
Changes in the Structure of the Spleen and Liver
All infected animals that developed parasitemia over 20% were treated with mefloquine between days 11 and 13 of infection, whereas all other infected animals were treated on day 17. All uninfected and infected-treated animals received a last injection of CL (or PBS) four days after mefloquine treatment, when parasitemia had cleared, and were euthanized 24 h later. Spleen and liver were harvested and processed for histological analysis.
Spleen
Treatment of uninfected S. sciureus with CL caused no perceivable changes in spleen weight in relation to uninfected animals that received PBS (Figure 5). Plasmodium falciparum infection, as expected, led to splenomegaly, with an average 5-fold increase in spleen/body weight ratios compared to uninfected animals. Treatment of P. falciparum-infected S. sciureus with CL 1 mL led to substantial reduction (average of 55%) in the degree of splenomegaly compared to infected animals that received only PBS (Figure 5 and Supplemental Table 1). The decrease in spleen/body weight ratios in animals treated with CL 0.5 mL was less prominent and did not reach significance compared to PBS-treated animals, although the lack of significance is likely due to the small sample size.
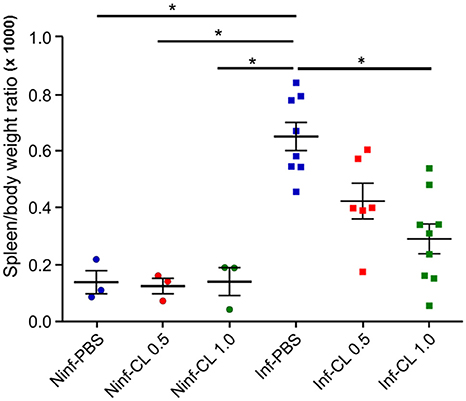
Figure 5. Effect of P. falciparum infection and/or CL administration on spleen/body weight ratios. Solid lines represent the mean and standard deviation. *P < 0.05.
The spleen of uninfected, control animals that received PBS showed typical S. sciureus splenic architecture, with well-defined limits between the red and white pulps, resting T cell areas, mostly resting follicles, and in some cases with phase I and phase II germinal centers containing a few apoptotic centers, absence of pigment and plasmacytes, and areas of monocyte accumulation in the marginal zone and the red pulp (Figure 6A), as previously described (Alves et al., 2015). Uninfected animals that received CL (0.5 or 1 mL) showed decreased number of clusters of monocyte/macrophages in red pulp and of apoptotic centers in the follicles compared to animals that received PBS (Figure 6B).
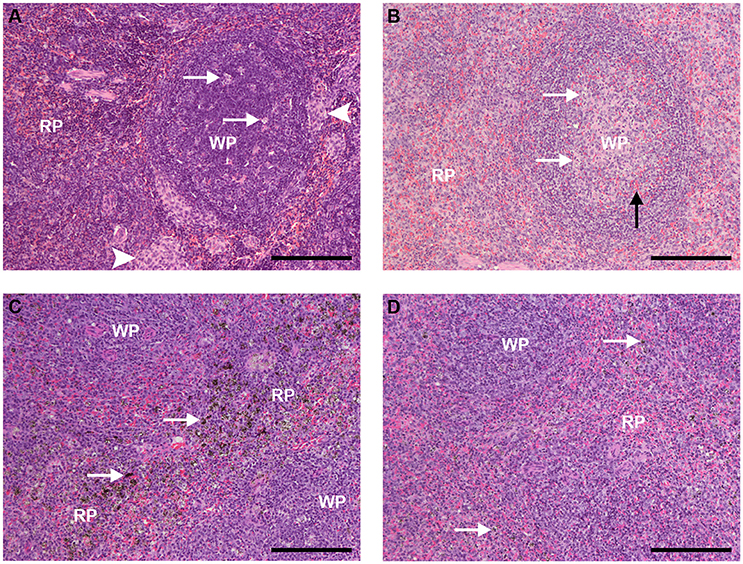
Figure 6. Spleen sections HE staining (bar: 200 μm). (A) Spleen of an uninfected, control animal that received PBS (code PA11). (A) B-cell follicle (WP, white pulp) is shown, with a few apoptotic centers (arrows); clusters of monocyte/macrophages, typical of Saimiri spleens, are present (arrowheads). The red pulp (RP) shows typical architecture, densily populated with small-nuclei, dark-stained cells, and red blood cells (red stain) flowing through. (B) Spleen of an uninfected Saimiri inoculated with CL 1 mL (code V3). In general, the splenic structure was preserved, but cell density was apparently smaller as compared to animals that received PBS, with predominance of cells with light-stained nuclei; penetration of red blood cells inside B-cell follicle was observed (black arrow); apoptotic centers (arrows), which are dependent on macrophages, and clusters of monocyte/macrophages were decreased (clusters of monocytes absent in this image). (C) Spleen of a P. falciparum-infected Saimiri that received PBS (code 141: treated at day 17, with 0.69% parasitemia, killed 5 days later). Emphasis is given to the distribution of hemozoin (seen as dark, granulated stain - arrows), which could be found throughout the red pulp (RP); loss of well-defined limits between the RP and the white pulp (WP) was observed. (D) Spleen of a P. falciparum-infected Saimiri that received CL 1 mL (code 272: treated at day 11, with 22 % parasitemia, killed 5 days later). The amount of hemozoin (arrows) was substantially decreased compared to infected animals that received PBS, and seen as small black dots. As in (C), loss of well-defined limits between the red pulp (RP) and the white pulp (WP) was observed.
Infected animals that received PBS showed phagocitosed malarial pigment (hemozoin) throughout the red pulp (Figure 6C). Erythroid precursors and plasmacytes were observed, and the marginal zone was in disarray (Figure 6C). Infected animals that received CL 1 mL showed substantial reduction in the accumulation of malarial pigment in the red pulp (Figure 6D), despite the fact that these animals in general showed much higher parasitemias (average 18.5%) as compared to infected animals that received PBS (average 8.1%), although for shorter periods of time (Figure 2).
The analysis of spleen sections stained with Perls, which shows ferric iron (Fe3+) within macrophages and can be used not only to observe the physiology of iron recycling but also to estimate the distribution of these cells, revealed that uninfected, control animals showed clusters of iron-containing macrophages distributed throughout the red pulp, and mild staining within follicles (Figure 7A). Uninfected, control animals that received CL 0.5 and 1 mL showed reduced amounts of iron-containing macrophages (Figure 7B).
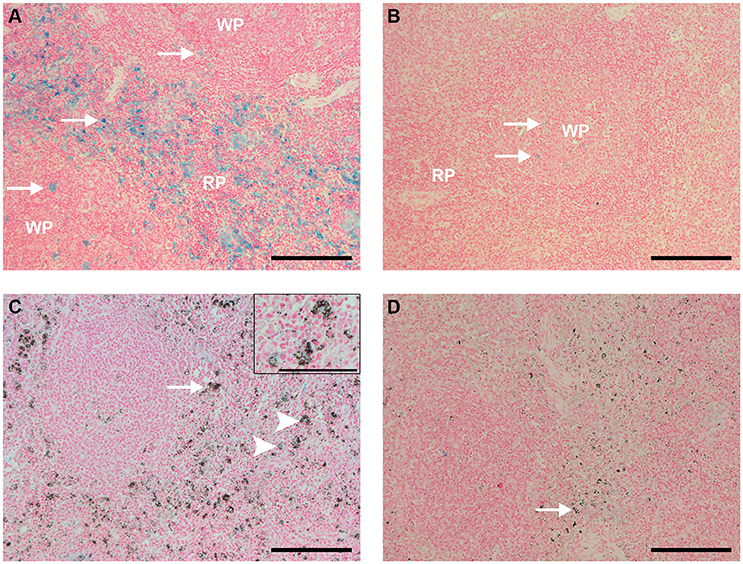
Figure 7. Spleen sections Perls staining (bar: 200 μm, except in C, bar: 100 μm). (A) Spleen of an uninfected, control animal that received PBS (code PA11). Large amounts of ferric iron-containing macrophages (blue staining) were observed throughout the red pulp (RP), and also sparsed in follicles (arrows). (B) Spleen of an uninfected Saimiri inoculated with CL 1 mL (code PB75). Blue staining, representing ferric iron-containing macrophages, nearly disappeared from the red pulp (RP), and was faint in follicles (arrows). (C) Spleen of a P. falciparum-infected Saimiri that received PBS (code 216: treated at day 17, with 1,3% parasitemia, killed 5 days later). Blue staining, representing ferric iron-containing macrophages, was much fainter in the spleen of infected, compared to uninfected, S. sciureus; on the other hand, macrophages were laden with hemozoin (arrow); only few macrophages showed colocalization of iron staining and hemozoin (arrowheads and magnified insert on top right). (D) Spleen of a P. falciparum-infected Saimiri that received CL 1 mL (code 272: treated at day 11, with 22% parasitemia, killed 5 days later). Presence of iron staining was nearly absent, and presence of hemozoin (arrow) was much decreased in relation to (C).
Infected animals treated with PBS showed iron staining in the red pulp, but substantially less than uninfected controls (Figure 7C). However, as mentioned above (Figure 6C), large numbers of hemozoin-containing macrophages were observed. As a rule, there was little or absent colocalization of iron staining and hemozoin (macrophages showed only one staining, Figure 7C). Infected animals treated with CL showed less iron staining as compared to animals that received PBS, and also less hemozoin staining (Figures 7D, 10).
Liver
With HE staining, livers of uninfected control animals that received PBS showed typical structure, with healthy hepatocytes and absence of necrosis or vacuolization and Kupffer cells without pigment (Figure 8A and Supplemental Figure 1A). Mononuclear cell infiltrates in the portal space were occasionally observed. Livers of animals that received CL 0.5 or 1 mL showed either no significant changes or, in some cases, evidence of hepatocyte vacuolization. Figure 8B shows one of these areas of hepatocyte vacuolization, an event that was only occasionally found but may indicate some degree of toxicity induced by CL treatment.
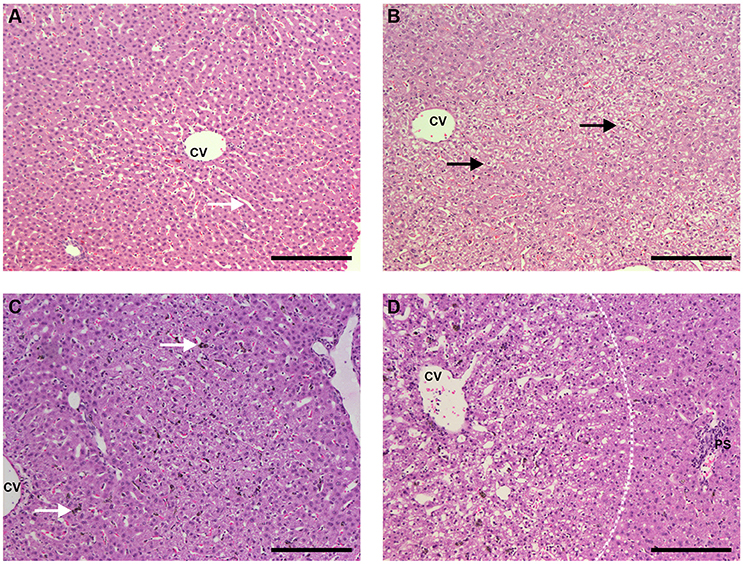
Figure 8. Liver sections HE staining (bar: 200 μm). (A) Liver of an uninfected, control animal that received PBS (code PA67), showing typical architecture, healthy hepatocytes and clean synusoids (arrows); CV, centrilobular vein. (B) Liver of an uninfected Saimiri inoculated with CL 1 mL (code PB75). The general aspect of most sections was rather normal (similar to the pattern of A), but eventually areas of hepatocyte vacuolization, as depicted, were observed; CV, centrilobular vein. (C) Liver of a P. falciparum-infected Saimiri that received PBS (code 141: treated at day 17, with 0.69 % parasitemia, killed 5 days later). Kupffer cells laden with hemozoin (arrow); CV, centrilobular vein. (D) Liver of a P. falciparum-infected Saimiri that received CL 1 mL (code 161: treated at day 11, with 22% parasitemia, killed 5 days later). Intense hepatocyte vacuolization was observed, evidenced particularly in the left half of the image, closer to the centrilobular vein (CV) and away from the portal space (PS) (more vacuolized area and more preserved area separated by a dashed line).
Infected animals that received PBS showed large numbers of mononuclear cells and also erythroid cells, neutrophils and plasmacytes within sinusoids, mononuclear cell infiltration in the portal space, and Kupffer cells containing malarial pigment (Figure 8C and Supplemental Figures 1B–D). Diffuse vacuolization of hepatocytes was observed in two thirds of the animals. Infected animals that received CL showed areas of intense hepatocyte vacuolization, and there were apparently fewer Kupffer cells with malarial pigment than in infected animals that received PBS (Figure 8D).
Perls staining of liver sections revealed that uninfected animals that received PBS showed strong iron staining throughout the organ, with granules within hepatocytes and Kupffer cells (Figure 9A). Iron staining in liver sections of uninfected animals that received CL 0.5 or 1 mL was less intense than in those that received PBS (Figure 9B).
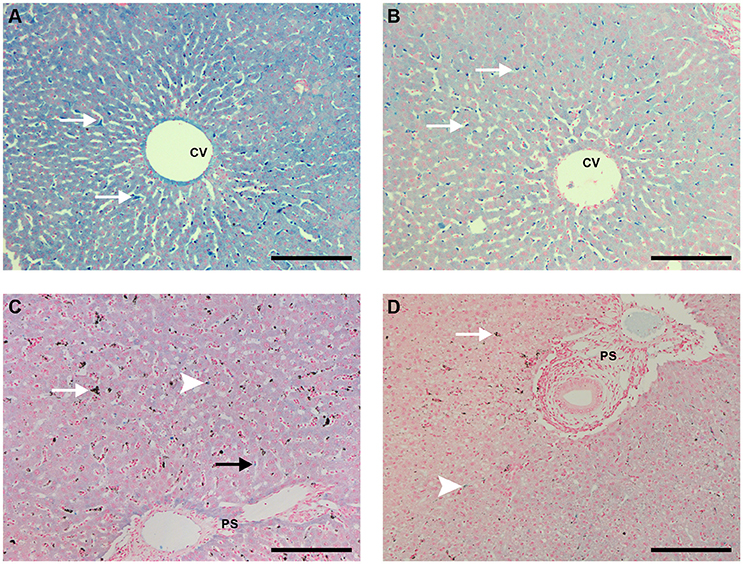
Figure 9. Liver sections Perls staining (bar: 200 μm). (A) Liver of an uninfected, control animal that received PBS (code PA11), showing blue staining throughout the hepatocytes, with stronger staining in Kupffer cells (arrows); CV: centrilobular vein (B) Liver of an uninfected Saimiri inoculated with CL 1 mL (code V3). The blue stainingwas lighter, especially in hepatocytes, with Kupffer cells still showing more pronounced staining (arrows). CV, centrilobular vein. (C) Liver of a P. falciparum-infected Saimiri that received PBS (code 216: treated at day 17, with 1,3% parasitemia, killed 5 days later). Iron staining was lighter than (A) in both hepatocytes and Kupffer cells, but still visible; Kupffer cells were laden with hemozoin (arrow); in only sporadic cases colocalization of hemozoin and iron staining was observed in Kupffer cells (arrowhead). PS: portal space. (D) Liver of a P. falciparum-infected Saimiri that received CL 1 mL (code 161: treated at day 11, with 22% parasitemia, killed 5 days later). Both iron staining (arrowhead) and hemozoin (arrow) were decreased in relation to (C). PS: portal space.
Infected animals that received PBS showed large amounts of hemozoin. Iron staining was weak throughout the organ, but still present in hepatocytes and isolated Kupffer cells or adherent macrophages within sinusoids, with little or no colocalization with hemozoin (Figure 9C and Supplemental Figures 2A,B). Infected animals that received CL 0.5 or 1 mL showed less intense hemozoin and iron staining, and Kupffer cells or macrophages with strong iron staining were rarely observed or not at all (Figure 9D).
Estimating the Efficacy of CL Treatment in Depleting Macrophages through Splenic Hemozoin Quantification
Qualitative analysis of spleen and liver, as shown in Figures 6–9, and decreased spleen/body weight ratios (Figure 5) indicated that CL treatment led to a decrease in macrophage population in these organs. Attempts to quantify macrophages using immunostaining with anti-human CD14, CD68, and HAM-56 monoclonal antibodies were unsucessful, probably due to the prolonged period in formalin. The effect of CL treatment on splenic macrophage numbers was therefore further estimated by quantifying hemozoin accumulation in this organ, which increases with increasing parasitemia (Sullivan et al., 1996), and hemozoin-laden macrophages remain in the spleen for several weeks (Frita et al., 2012). Although, CL-treated monkeys showed about half the splenic hemozoin accumulation as compared to PBS-treated animals (Figure 10A), this difference did not reach statistical significance due to small sample size and to some degree of variation between animals. However, when hemozoin concentration was adjusted to the level of parasitemia (hemozoin concentration/peak parasitemia, Figure 10B; hemozoin concentration/area under the curve of parasitemia, Figure 10C), marked differences were observed between the two groups, indicating that despite sustaining much higher parasite loads CL-treated animals showed less hemozoin accumulation in the spleen. These data indicate that splenic macrophages of CL-treated animals are either markedly decreased in numbers, as also suggested by HE staining and spleen weights, or are functionally impaired, in any case resulting in decreased capacity to phagocytose parasitized red blood cells.
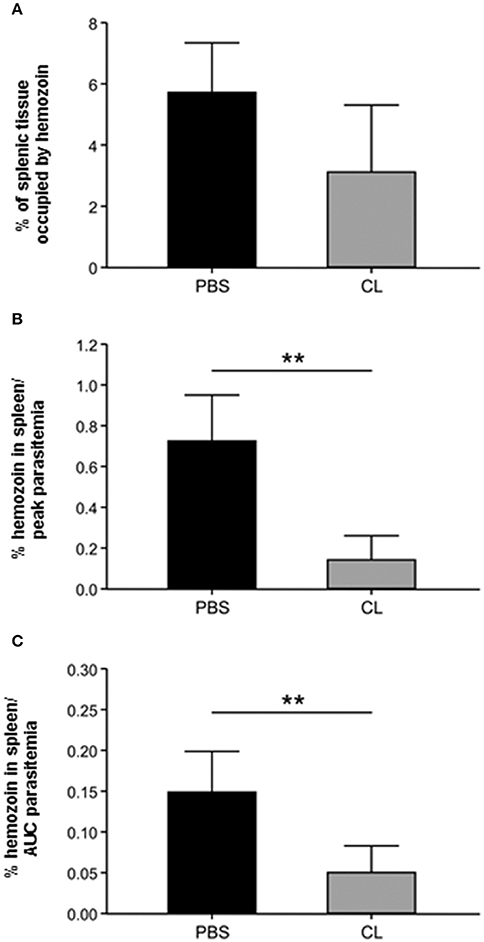
Figure 10. Estimation of the effect of CL treatment on splenic macrophage population through hemozoin quantification. (A) Percentage of the splenic tissue occupied by hemozoin in PBS-treated and CL-treated infected animals. (B,C) Amount of splenic hemozoin adjusted by parasitemia in PBS-treated and CL-treated infected animals. The percentage of splenic tissue occupied by hemozoin (as in A) was divided by the peak parasitemia (B) or by the area under the curve (AUC) of parasitemia (C) of each animal. **P < 0.01.
Discussion
Saimiri monkeys are susceptible to infection by Plasmodium falciparum and, together with Aotus, constitute unique experimental models for malaria (WHO, 2004), making these animals particularly useful in preclinical trials of potential malaria vaccines and for studies on pathogenesis (Carvalho et al., 2000, 2004, 2005). Pre-clinical evaluation of vaccines in these experimental models can provide valuable information about the immunogenicity, efficacy and safety of a variety of formulations, facilitating selection for humans trials. Since access to this valuable resource is restricted, with limited number of animals available for research, optimization of the model is needed to allow the design of experiments with reduced sample sizes. This goal faces two important limitations regarding the use of non-splenectomized animals: (1) they usually develop low parasitemias, which makes it more difficult to detect the effect of interventions, such as vaccines, on the course of infection; and (2) there is substantial interindividual variability in the levels of parasitemias (Contamin et al., 2000). These two factors underscore the need to increase sample sizes in experiments using these animals. The alternative to overcome these limitations has been the use of splenectomized animals, which achieve higher and more consistent parasitemias, allowing smaller experimental groups to be formed. However, this strategy also faces important setbacks: (a) the spleen plays a central role in immunity against plasmodial infections and (b) non-human primates (NHP) are chosen as models for experimental studies with human plasmodia, mainly vaccines, because of their close filogenetic relationship to man. Splenectomy pushes back the model from the natural human situation. For these reasons, splenectomy constitutes a severe handicap especially in immunity and vaccine studies. The use of the “chemical (temporary) splenectomy” described here offers therefore an animal model closer to the target population condition.
In plasmodial infections, the spleen helps to control parasite burden through innate and adaptative immune responses (Yazdani et al., 2006; Portillo et al., 2012; Gazzinelli et al., 2014). Splenic macrophages are major players in this system, clearing parasitized red blood cells through phagocytic activity. Because S. sciureus are not P. falciparum's natural host, splenic clearance seems to be particularly effective, preventing higher parasitemias. We asked whether partial depletion of the macrophage population in the spleen and other sites would result in higher and more consistent parasitemias in S. sciureus while maintaining not only the structure but also the function of the spleen. Together, our results indicate that this goal was largely achieved in this study.
Saimiri sciureus monkeys infected with Plasmodium falciparum and treated with periodic intravenous injections of clodronate-encapsulated liposomes (CL) in general developed higher parasitemias requiring antimalarial treatment than infected monkeys treated with PBS. It is not clear why this effect was not observed in three out of 9 (33.3%) infected S. sciureus treated with CL 1 mL, as they were able to self-control parasitemia, behaving closer to PBS-treated animals. Three independent experiments were performed, and these three animals were from the same experiment, suggesting that the lot of CL used may not have been optimal. Alternatively, this result would confirm that there is some degree of individual animal variation, either in the efficacy of CL treatment or in the pre-existing state of non-adaptive immunity. It is likely that the number of macrophages will vary from one animal to the other. It is advisable that each lot of CL be assayed in vitro for monocyte killing capacity prior to the conduction of in vivo experiments.
In any case, the efficacy of CL 1 mL treatment was evident, as two thirds (6 out of 9) of the treated animals developed parasitemias over 20% requiring mefloquine treatment, whereas all eight PBS-treated animals self-controled their parasitemias. Administration of a smaller amount of CL (0.5 mL) also resulted in higher parasitemias overall, with half (3 out of 6) of the animals showing parasitemias over 20%. Results are in agreement with those recorded with P. falciparum in immunocompromised mice grafted with human RBCs (Badell et al., 2000; Arnold et al., 2010). Thus, it is very likely that higher CL doses would result in improved macrophage control, and thereby in higher parasitemias. This remains to be investigated in future experiments.
From an ethical point of view CL treatment offers the paramount advantage of avoiding heavy surgery and allowing full recovery of animals. It thus constitutes a major improvement from a scientific as well as from an ethical viewpoint over splenectomy.
Plasmodium falciparum infection and/or CL treatment had little effect on peripheral blood monocyte counts, which is not a surprise as young monocytes have limited phagocytic activity and CL targets preferentially large and active macrophages, which ingest a larger number of liposomes per cell. Indeed, CL injections resulted in marked effects in the spleen and the liver. In uninfected animals, no evident effect of CL injections was observed in spleen/body weight ratios, but the number and distribution of ferric iron-containing cells in the spleen were markedly reduced. Macrophages are involved in senescent red blood cell phagocytosis and iron recycling, and therefore CL injections largely depleted this cell population in the spleen. In Plasmodium falciparum-infected animals, CL injections resulted in milder splenomegaly and this effect was associated histologically with marked reductions in hemozoin-containing cells and further reductions in iron-containing cells. Efforts to further substantiate the histological findings by specific macrophage staining with commercially available anti-human CD14, CD68, and HAM-56 monoclonal antibodies were unsucessful, probably due to the prolonged period the biological material was kept in formalin.
Our results also indicate that P. falciparum infection in S. sciureus causes impaired iron-recycling by macrophages. Infected animals showed large numbers of hemozoin-containing macrophages but decreased numbers of ferric iron-containing cells in the spleen. An effect on iron homeostasis was also observed in the liver. It is known that macrophages are very important actors in controlling the iron upload and metabolism in hepatocytes, mostly via hepcidin. Therefore, it is possible that the reduction in iron staining in hepatocytes after CL treatment resulted from the instability of the hepcidin production due to Kupffer cell destruction (Fleming, 2005; Makui et al., 2005). However, additional studies are needed in order to clarify the mechanisms behind this phenomenon. In addition, colocalization of hemozoin and iron was a rare event, indicating that phagocytes were busy processing hemozoin from infected red blood cells and their capacity to carry out phagocytosis of uninfected red blood cells was impaired. Although the dark and gross pattern of hemozoin might actually prevent visualization of the light blue iron staining and therefore colocalization might be underestimated, some facts suggest that iron uptake by macrophages was impaired: (i) even macrophages with little, sparse amount of hemozoin showed no iron staining; (ii) colocalization was observed in some cells heavily laden with hemozoin. Since it has been shown that hemozoin may persist for weeks to months after parasite clearance (Frita et al., 2012; Alves et al., 2015), it may negatively impact the immune responses and iron recycling of the affected individuals for long periods of time after treatment has been implemented and therefore, contribute significantly to increasing malaria morbidity. Interestingly, in infected animals, Kupffer cells and sinusoidal macrophages heavily laden with iron were observed. This finding may indicate that young cells from the bone marrow repopulate the liver in an effort to restore iron recycling and other functions.
Depletion of macrophages by CL shows a number of advantages over surgical splenectomy for the study of malaria vaccines in Saimiri monkeys. However, macrophages are very important in the immune responses against P. falciparum through direct infected red blood cell phagocytosis or antibody-mediated parasite opsonization or inhibition (Bouharoun-Tayoun et al., 1990; Buffet et al., 2011). Therefore, sharp decreases in macrophage numbers might affect the antiplasmodial immune responses a given pre-clinical vaccine trial may be attempting to evaluate. The same limitation can be considered for dendritic cells, which also play relevant roles in antiplasmodial immunity (Urban et al., 1999, 2001). Indeed, phagocytic marginal dendritic cells but not interdigitating dendritic cells are known to be depleted by CL treatment (Leenen et al., 1998), which can affect immune responses to particulate antigens (Delemarre et al., 1990). These limitations need to be taken into account when using CL treatment as the strategy to induce higher P. falciparum parasitemias.
It has been shown that plasmodial infections in humans (Urban et al., 2005), Saimiri (Alves et al., 2015) and mice (Achtman et al., 2003; Carvalho et al., 2007; Martins et al., 2009) induce disarray of the spleen and other lymphoid organs, with disturbance of germinal center architecture. This finding was confirmed in the present study in S. sciureus, and CL administration had no apparent effect on this event. The splenic changes observed in P. falciparum-infected, saline-treated Saimiri 5 days after mefloquine treatment were similar to those recently reported for P. falciparum-infected Saimiri at peak parasitemia before antimalarial treatment (Alves et al., 2015). These changes included the presence of large numbers of phagocytes in the red pulp heavily laden with malaria pigment, disarray of B-cell follicles, blurred limits between the red and white pulps, and penetration of RBCs into follicles. In that study, 14 days after chloroquine treatment, spleens showed better white pulp organization and persistence of hemozoin in the red pulp but with a different pattern with more compacted, less granulous pigment (Alves et al., 2015). Therefore, the pattern of splenic changes observed in infected animals 5 days after mefloquine treatment in the present study was more similar to the changes observed at peak parasitemia prior to antimalarial treatment than after an extended period (2 weeks) after antimalarial treatment. This finding suggests that 5 days following antimalarial drug treatment is insufficient to reverse the pathological changes in spleen structure induced by P. falciparum infection.
Finally, infected animals that received CL showed more intense hepatocyte vacuolization. This effect was most likely a result of a combination of CL injection with higher parasitemias in these animals, because both uninfected animals receiving CL and infected animals receiving PBS showed some degree of hepatocyte vacuolization, milder than when the events were combined.
In conclusion, these results indicate that CL depleted splenic macrophages leading to decreased parasite phagocytosis, decreased splenic congestion, and increased parasitemias. CL administration is therefore a practical and viable alternative to surgical splenectomy in this experimental model.
Author Contributions
JC participated in study design, carried out the experiments and helped LC in drafting the manuscript; CBJ participated in study design and carried out the experiments, MA, LP-R, ER, and MP carried out the experiments and reviewed the manuscript; IdS carried out the experiments; LC, CD-R, and PD conceived the study, participated in its design and coordination, and reviewed the manuscript. All authors have read and approved the final manuscript.
Funding
This study was supported by Coordenação de Aperfeiçoamento de Pessoal de Nível Superior (CAPES), Conselho Nacional de Desenvolvimento Científico e Tecnológico (CNPq, Brazil), Fundação Carlos Chagas Filho de Apoio de Amparo à Pesquisa do Estado do Rio de Janeiro (FAPERJ, Brazil), Instituto Oswaldo Cruz (FIOCRUZ, Brazil) and Programa de Apoio a Núcleos de Excelência-PRONEX (DECIT/CNPq/FAPERJ). CD-R and LC are recipients of Research Productivity fellowships from CNPq and are supported by FAPERJ as “Cientistas do Nosso Estado.”
Conflict of Interest Statement
The authors declare that the research was conducted in the absence of any commercial or financial relationships that could be construed as a potential conflict of interest.
Supplementary Material
The Supplementary Material for this article can be found online at: http://journal.frontiersin.org/article/10.3389/fcimb.2017.00408/full#supplementary-material
Supplemental Figure 1. Liver sections, HE (bar: 100 μm). (A) Liver of an uninfected, control animal that received PBS (code PA67), in higher magnification than Figure 8A, showing Kupffer cells (arrows). (B–D) Liver of a P. falciparum-infected Saimiri that received PBS (code 141: treated at day 17, with 0.69% parasitemia, killed 5 days later), in greater detail compared to Figure 8C. Portal infiltrates (black arrow), increased cellularity in sinusoids with presence of mononuclear cells (white arrowhead), erythroid cells (thin arrow) and plasma cells (black arrowhead).
Supplemental Figure 2. Liver sections, Perls (bar: 100 μm). (A,B) Liver of a P. falciparum-infected Saimiri that received PBS (code 216: treated at day 17, with 1.3% parasitemia, killed 5 days later), in greater detail compared to Figure 9C. Intrasinusoidal macrophages, circulating or attached to the sinusoidal wall, laden with malarial pigment (white arrows). There was little or no colocalization of hemozoin and iron staining (black arrow).
Supplemental Table 1. Results of experiments 1, 2, and 3 showing the data of parasitemia, CD14 counting, hematocrit, hemoglobin, rectal temperature, weight and spleen/body weight ratios.
References
Abbas, A. K., Lichtman, A. H., and Pillai, S. (2008). Imunologia Celular e Molecular, 6th Edn. Rio de Janeiro: Elsevier.
Achtman, A. H., Khan, M., MacLennan, I. C., and Langhorne, J. (2003). Plasmodium chabaudi chabaudi infection in mice induces strong B cell responses and striking but temporary changes in splenic cell distribution. J. Immunol. 171, 317–324. doi: 10.4049/jimmunol.171.1.317
Alves, F. A., Pelajo-Machado, M., Totino, P. R., Souza, M. T., Gonçalves, E. C., Schneider, M. P., et al. (2015). Splenic architecture disruption and parasite-induced splenocyte activation and anergy in Plasmodium falciparum-infected Saimiri sciureus monkeys. Malar. J. 14:128. doi: 10.1186/s12936-015-0641-3
Alves, F. A., Souza, M. T., Gonçalves, E. C., Schneider, M. P., Marinho, A. M., Muniz, J. A., et al. (2010). DNA sequencing of 13 cytokine gene fragments of Aotus infulatus and Saimiri sciureus, two non-human primate models for malaria. Cytokine 52, 151–155. doi: 10.1016/j.cyto.2010.09.004
Arnold, L., Tyagi, R. K., Mejia, P., Swetman, C., Gleeson, J., Perignon, J. L., et al. (2011). Further improvements of the P. falciparum humanized mouse model. PLoS ONE 6:e18045. doi: 10.1371/journal.pone.0018045
Arnold, L., Tyagi, R. K., Mejia, P., van Rooijen, N., Perignon, J. L., and Druilhe, P. (2010). Analysis of innate defences against Plasmodium falciparum in immunodeficient mice. Malar. J. 9:197. doi: 10.1186/1475-2875-9-197
Badell, E., Oeuvray, C., Moreno, A., Soe, S., van Rooijen, N., Bouzidi, A., et al. (2000). Human malaria in immunocompromised mice: an in vivo model to study defense mechanisms against Plasmodium falciparum. J. Exp. Med. 192, 1653–1659. doi: 10.1084/jem.192.11.1653
Bouharoun-Tayoun, H., Attanath, P., Sabchareon, A., Chongsuphajaisiddhi, T., and Druilhe, P. (1990). Antibodies that protect humans against Plasmodium falciparum blood stages do not on their own inhibit parasite growth and invasion in vitro, but act in cooperation with monocytes. J. Exp. Med. 172, 1633–1641.
Buffet, P. A., Safeukui, I., Deplaine, G., Brousse, V., Prendki, V., Thellier, M., et al. (2011). The pathogenesis of Plasmodium falciparum malaria in humans: insights from splenic physiology. Blood 117, 381–392. doi: 10.1182/blood-2010-04-202911
Carvalho, L. J., Ferreira-da-Cruz, M. F., Daniel-Ribeiro, C. T., Pelajo-Machado, M., and Lenzi, H. L. (2007). Germinal center architecture disturbance during Plasmodium berghei ANKA infection in CBA mice. Malar. J. 16:59. doi: 10.1186/1475-2875-6-59
Carvalho, L. J. M., Alves, F. A., Bianco-Jr, C., Oliveira, S. G., Zanini, G. M., Soe, S., et al. (2005). Immunization of Saimiri sciureus monkeys with a recombinant hybrid protein derived from the Plasmodium falciparum antigens glutamate-rich protein and merozoite surface protein-3 can induce partial protection with Freund's and Montanide ISA720 adjuvants. Clin. Diagn. Lab. Immunol. 12, 242–248. doi: 10.1128/CDLI.12.2.242-248.2005
Carvalho, L. J., Alves, F. A., de Oliveira, S. G., do Valle, R. R., Fernandes, A. A., Muniz, J. A., et al. (2003). Severe anemia affects both splenectomized and non-splenectomized Plasmodium falciparum-infected Aotus infulatus monkeys. Mem. Inst. Oswaldo Cruz. 98, 679–686.
Carvalho, L. J. M., Daniel-Ribeiro, C. T., and Goto, H. (2002). Malaria vaccine: candidate antigens, mechanisms, constraints and prospects. Scand. J. Immunol. 56, 327–343. doi: 10.1046/j.1365-3083.2002.01160.x
Carvalho, L. J. M., Oliveira, S. G., Alves, F. A., Brígido, M. C. O., Muniz, J. A. P. C., and Daniel-Ribeiro, C. T. (2000). Aotus infulatus is susceptible to Plasmodium falciparum infection and may constitute an alternative experimental model for malaria. Mem. Inst. Oswaldo Cruz 95, 363–365. doi: 10.1590/S0074-02762000000300011
Carvalho, L. J. M., Oliveira, S. G., Theisen, M., Alves, F. A., Andrade, M. C. R., Zanini, G. M., et al. (2004). Immunization of Saimiri sciureus monkeys with Plasmodium falciparum merozoite surface protein-3 and glutamate-rich protein suggests that protection is related to antibody levels. Scand. J. Immunol. 59, 363–372. doi: 10.1111/j.0300-9475.2004.01409.x
Chrubasik, C., and Jacobson, R. L. (2010). The development of artemisinin resistance in malaria: reasons and solutions. Phytother. Res. 24, 1104–1106. doi: 10.1002/ptr.3133
Clemens, J., and Moorthy, V. (2016). Implementation of RTS,S/AS01 malaria vaccine – the need for further evidence. N. Engl. J. Med. 374, 2596–2597. doi: 10.1056/NEJMe1606007
Collins, W. (1992). South american monkeys in the development and testing of malaria vaccines. Mem. Inst. Oswaldo Cruz 87(Suppl. 3), 401–406. doi: 10.1590/S0074-02761992000700068
Collins, W. E., Galland, G. G., Barnwell, J. W., Udhayakumar, V., Sullivan, J. S., Nace, D., et al. (2005). Preliminary observations on the efficacy of a recombinant multistage Plasmodium falciparum vaccine in Aotus nancymai monkeys. Am. J. Trop. Med. Hyg. 73, 686–693.
Contamin, H., Behr, C., Mercereau-Puijalon, O., and Michel, J. C. (2000). Plasmodium falciparum in the squirrel monkey (Saimiri sciureus): infection of nonsplenectomised animals as a model for exploring clinical manifestations of malaria. Microbes Infect. 2, 945–954. doi: 10.1016/S1286-4579(00)00401-9
Contamin, H., Loizon, S., Bourreau, E., Michel, J. C., Garraud, O., Mercereau-Puijalon, O., et al. (2005). Flow cytometry identification and characterization of mononuclear cell subsets in the neotropical primate Saimiri sciureus (squirrel monkey). J. Immunol. Methods 297, 61–71. doi: 10.1016/j.jim.2004.11.019
Criswell, B. S., Butler, W. T., Rossen, R. D., and Knight, V. (1971). Murine malaria: the role of humoral factors and macrophages in destruction of parasitized erythrocytes. J. Immunol. 107, 212–221.
Delemarre, F. G., Kors, N., and van Rooijen, N. (1990). Elimination of spleen and of lymph node macrophages and its difference in the effect on the immune response to particulate antigens. Immunobiology 182, 70–78. doi: 10.1016/S0171-2985(11)80584-X
Dondorp, A. M., Yeung, S., White, L., Nguon, C., Day, N. P., Socheat, D., et al. (2010). Artemisinin resistance: current status and scenarios for containment. Nat. Rev. Microbiol. 9, 272–280. doi: 10.1038/nrmicro2331
Fleming, R. E. (2005). The iron sensor: macrophage, hepatocyte, both? Blood 106, 1893–1894. doi: 10.1182/blood-2005-07-2673
Frita, R., Carapau, D., Mota, M. M., and Hänscheid, T. (2012). In vivo hemozoin kinetics after clearance of Plasmodium berghei infection in mice. Malar. Res. Treat. 2012:373086. doi: 10.1155/2012/373086
Garraud, O., Contamin, H., Perraut, R., Behr, C., and Gysin, J. (1998). Analysis on reactivity of human lymphocyte and monocyte-specific antibodies with peripheral blood mononuclear cells from squirrel monkeys (Saimiri sciureus). J. Med. Primatol. 73, 686–693. doi: 10.1111/j.1600-0684.1998.tb00074.x
Gazzinelli, R. T., Kalantari, P., Fitzgerald, K. A., and Golenbock, D. T. (2014). Innate sensing of malaria parasites. Nat. Rev. Immunol. 14, 744–757. doi: 10.1038/nri3742
Gysin, J., and Fandeur, T. (1983). Saimiri sciureus (karyotype 14-7): an alternative experimental model of Plasmodium falciparum infection. Am. J. Trop. Med. Hyg. 32, 461–467. doi: 10.4269/ajtmh.1983.32.461
Herrera, S., Perlaza, B. L., Bonelo, A., and Arévalo-Herrera, M. (2002). Aotus monkeys: their great value for anti-malaria vaccines and drug testing. Int. J. Parasitol. 32, 1625–1635. doi: 10.1016/S0020-7519(02)00191-1
Kim, H. M., Lee, Y. W., Lee, K. J., Kim, H. S., Cho, S. W., van Rooijen, N., et al. (2008). Alveolar macrophages are indispensable for controlling influenza viruses in lungs of pigs. J. Virol. 82, 4265–4274. doi: 10.1128/JVI.02602-07
Leenen, P. J., Radosević, K., Voerman, J. S., Salomon, B., van Rooijen, N., Klatzmann, D., et al. (1998). Heterogeneity of mouse spleen dendritic cells: in vivo phagocytic activity, expression of macrophage markers, and subpopulation turnover. J. Immunol. 160, 2166–2173.
Leisewitz, A. L., Rockett, K. A., Gumede, B., Jones, M., Urban, B., and Kwiatkowski, D. P. (2004). Response of the splenic dendritic cell population to malaria infection. Infect. Immun. 72, 4233–4239. doi: 10.1128/IAI.72.7.4233-4239.2004
Makui, H.;, Soares, R. J.;, Jiang, W., Constante, M., and Santos, M. M. (2005). Contribution of Hfe expression in macrophages to the regulation of hepatic hepcidin levels and iron loading. Blood 106, 2189–2195. doi: 10.1182/blood-2005-02-0629
Martins, Y. C., Smith, M. J., Pelajo-Machado, M., Werneck, G. L., Lenzi, H. L., Daniel-Ribeiro, C. T., et al. (2009). Characterization of cerebral malaria in the outbred Swiss Webster mouse infected by Plasmodium berghei ANKA. Int. J. Exp. Pathol. 90, 119–130. doi: 10.1111/j.1365-2613.2008.00622.x
Mathes, M., Jordan, M., and Dowa, S. (2006). Evaluation of liposomal clodronate in experimental spontaneous autoimmune hemolytic anemia in dogs. Exp. Hematol. 34, 1393–1402. doi: 10.1016/j.exphem.2006.05.014
Olotu, A., Fegan, G., Wanbua, J., Nyangweso, G., Leach, A., and Lievens, M. (2016). Seven-years efficacy of RTS,S/AS01 malaria vaccine among young african children. N. Engl. J. Med. 374, 2519–2529. doi: 10.1056/NEJMoa1515257
Otieno, L., Oneko, M., Otieno, W., Abuodha, J., Owino, E., Odero, C., et al. (2016). Safety and immunogenicity of RTS,S/AS01 malaria vaccine in infants and children with WHO stage 1 or 2 HIV disease: a randomised, double-blind, controlled trial. Lancet Infect. Dis. 16, 1134–1144. doi: 10.1016/S1473-3099(16)30161-X
Portillo, H. A., Ferrer, M., Brugat, T., Martin-Jaular, L., Langhorne, J., Lacerda, M. V. G., et al. (2012). The role of the spleen in malaria. Cell. Microbiol. 14, 343–355. doi: 10.1111/j.1462-5822.2011.01741.x
Quintero, J. P., Siqueira, A. M., Tobón, A., Blair, S., Moreno, A., Arévalo-Herrera, M., et al. (2011). Malaria-related anaemia: a Latin American perspective. Mem. Inst. Oswaldo Cruz 106(Suppl. 1), 91–104. doi: 10.1590/S0074-02762011000900012
Riccio, E. K., Pratt-Riccio, L. R., Bianco-Junior, C., Sanchez, V., Totino, P. R., and Carvalho, L. J. (2015). Molecular and immunological tools for the evaluation of the cellular immune response in the neotropical monkeys Saimiri sciureus, a non-human primate model for malaria research. Malar. J. 14:166. doi: 10.1186/s12936-015-0688-1
Rowe, J. A., Claessens, A., Corrigan, R. A., and Arman, M. (2009). Adhesion of Plasmodium falciparum infected erythrocytes to human cells: molecular mechanisms and therapeutic implications. Expert Rev Mol. Med. 11:e16. doi: 10.1017/S1462399409001082
Sirima, S. B., Cousens, S., and Druilhe, P. (2011). Protection against malaria by MSP3 candidate vaccine. N. Engl. J. Med. 365, 1062–1064. doi: 10.1056/NEJMc1100670
Sullivan, A. D., Ittarat, I., and Meshnick, S. R. (1996). Patterns of haemozoin accumulation in tissue. Parasitology 112(Pt 3), 285–294.
Tougan, T., Aoshi, T., Coban, C., Katakai, Y., Kai, C., Yasutomi, Y., et al. (2013). TLR9 adjuvants enhance immunogenicity and protective efficacy of the SE36/AHG malaria vaccine in nonhuman primate models. Hum. Vaccin. Immunother. 9, 283–290. doi: 10.4161/hv.22950
Urban, B. C., Ferguson, D. J., Pain, A., Willcox, N., Plebanski, M., Austyn, J. M., et al. (1999). Plasmodium falciparum-infected erythrocytes modulate the maturation. Nature 400, 73–77.
Urban, B. C., Willcox, N., and Roberts, D. J. (2001). A role for CD36 in the regulation of dendritic cell function. Proc. Natl. Acad. Sci. U.S.A. 98, 8750–8755. doi: 10.1073/pnas.151028698
Urban, B. C., Hien, T. T., Day, N. P., Phu, N. H., Roberts, R., Pongponratn, E., et al. (2005). Fatal Plasmodium falciparum malaria causes specific patterns of splenic architectural disorganization. Infect. Immun. 73, 1986–1994. doi: 10.1128/IAI.73.4.1986-1994.2005
van Rooijen, N., and Kors, N. (1989). Effects of intracellular diphosphonates on cells of the mononuclear phagocyte system: in vivo effects of liposome-encapsulated diphosphonates on different macrophage subpopulations in the spleen. Calcif. Tis. Int. 45, 153–156. doi: 10.1007/BF02556058
van Rooijen, N., Kors, N., vd Ende, M., and Dijkstra, C. D. (1990). Depletion and repopulation of macrophages in spleen and liver of rat after intravenous treatment with liposome-encapsulated dichloromethylene diphosphonate. Cell Tis. Res. 260, 215–222.
van Rooijen, N., and van Kesteren-Hendrikx, E. (2002). Clodronate liposomes: perspectives in research and therapeutics. J. Liposome Res. 12, 81–94. doi: 10.1081/LPR-120004780
van Rooijen, N., and van Nieuwmegen, R. (1984). Elimination of phagocytic cells in the spleen after intravenous injection of liposome-encapsulated dichloromethylene diphosphonate. an enzyme-histochemical study. Cell Tis. Res. 238, 355–358.
WHO (2004). MALVAC Meeting 2004: Evaluation of Malaria Vaccines. Pre-clinical Evaluation Group: Optimizing the Developmental Pathway from the Lab to the Clinic. Report from a technical consultation at WHO/IVR Malaria Vaccine Advisory Committee meeting Montreux; 2004.
WHO (2016). World Malaria Report 2016. World Health Organization: 2015. Available online at: http://www.who.int/malaria/publications/world-malaria-report-2016/report/en/
Keywords: malaria, Plasmodium falciparum, Saimiri sciureus, clodronate liposomes, macrophages, spleen, liver
Citation: Cunha JA, Carvalho LJM, Bianco-Junior C, Andrade MCR, Pratt-Riccio LR, Riccio EKP, Pelajo-Machado M, da Silva IJ, Druilhe P and Daniel-Ribeiro CT (2017) Increased Plasmodium falciparum Parasitemia in Non-splenectomized Saimiri sciureus Monkeys Treated with Clodronate Liposomes. Front. Cell. Infect. Microbiol. 7:408. doi: 10.3389/fcimb.2017.00408
Received: 09 February 2017; Accepted: 04 September 2017;
Published: 21 September 2017.
Edited by:
Kai Matuschewski, Humboldt-Universität zu Berlin, GermanyReviewed by:
Ashley Vaughan, Center for Infectious Disease Research, United StatesJodi L. McGill, Kansas State University, United States
Copyright © 2017 Cunha, Carvalho, Bianco-Junior, Andrade, Pratt-Riccio, Riccio, Pelajo-Machado, da Silva, Druilhe and Daniel-Ribeiro. This is an open-access article distributed under the terms of the Creative Commons Attribution License (CC BY). The use, distribution or reproduction in other forums is permitted, provided the original author(s) or licensor are credited and that the original publication in this journal is cited, in accordance with accepted academic practice. No use, distribution or reproduction is permitted which does not comply with these terms.
*Correspondence: Cláudio Tadeu Daniel-Ribeiro, bWFsYXJpYUBmaW9jcnV6LmJy
†These authors have contributed equally to this work.