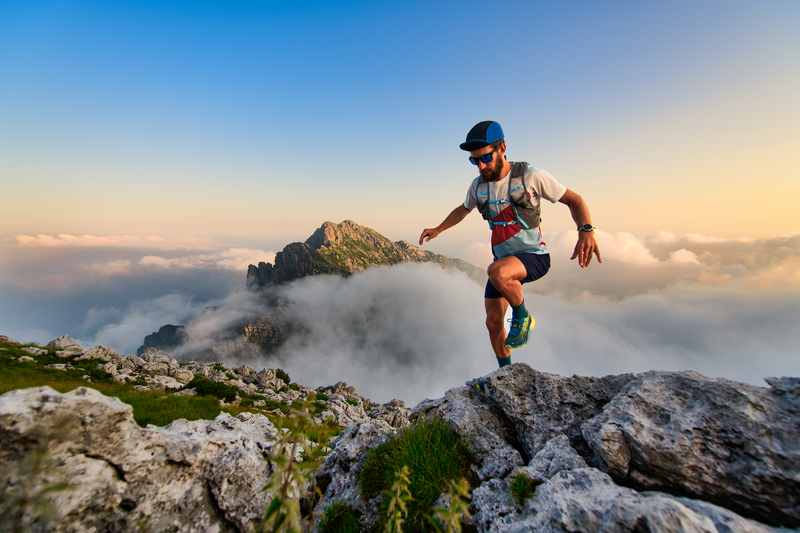
95% of researchers rate our articles as excellent or good
Learn more about the work of our research integrity team to safeguard the quality of each article we publish.
Find out more
ORIGINAL RESEARCH article
Front. Cell. Infect. Microbiol. , 08 August 2017
Sec. Bacteria and Host
Volume 7 - 2017 | https://doi.org/10.3389/fcimb.2017.00359
This article is part of the Research Topic Neutrophils and Microbial Pathogens: The Never Ending Battle View all 23 articles
Porphyromonas gingivalis is a gram-negative anaerobic periodontal pathogen that persists in dysbiotic mixed-species biofilms alongside a dense inflammatory infiltrate of neutrophils and other leukocytes in the subgingival areas of the periodontium. Toll-like receptor 2 (TLR2) mediates the inflammatory response to P. gingivalis and TLR2-deficient mice resist alveolar bone resorption following oral challenge with this organism. Although, MyD88 is an adaptor protein considered necessary for TLR2-induced inflammation, we now report for the first time that oral challenge with P. gingivalis leads to alveolar bone resorption in the absence of MyD88. Indeed, in contrast to prototypical TLR2 agonists, such as the lipopeptide Pam3CSK4 that activates TLR2 in a strictly MyD88-dependent manner, P. gingivalis strikingly induced TLR2 signaling in neutrophils and macrophages regardless of the presence or absence of MyD88. Moreover, genetic or antibody-mediated inactivation of TLR2 completely reduced cytokine production in P. gingivalis-stimulated neutrophils or macrophages, suggesting that TLR2 plays a non-redundant role in the host response to P. gingivalis. In the absence of MyD88, inflammatory TLR2 signaling in P. gingivalis-stimulated neutrophils or macrophages depended upon PI3K. Intriguingly, TLR2-PI3K signaling was also critical to P. gingivalis evasion of killing by macrophages, since their ability to phagocytose this pathogen was reduced in a TLR2 and PI3K-dependent manner. Moreover, within those cells that did phagocytose bacteria, TLR2-PI3K signaling blocked phago-lysosomal maturation, thereby revealing a novel mechanism whereby P. gingivalis can enhance its intracellular survival. Therefore, P. gingivalis uncouples inflammation from bactericidal activity by substituting TLR2-PI3K in place of TLR2-MyD88 signaling. These findings further support the role of P. gingivalis as a keystone pathogen, which manipulates the host inflammatory response in a way that promotes bone loss but not bacterial clearance. Modulation of these host response factors may lead to novel therapeutic approaches to improve outcomes in disease conditions associated with P. gingivalis.
Bone resorption is a prominent feature of chronic conditions of microbial etiology, such as osteomyelitis and periodontitis. In periodontitis, polymicrobial dysbiotic communities induce inflammation that leads to erosion of the soft and hard tissues that support the teeth, and eventually to tooth loss (Armitage, 1996; Pihlstrom et al., 2005). Porphyromonas gingivalis, a Gram-negative asaccharolytic anaerobic oral bacterium, was shown to remodel a symbiotic community into a dysbiotic one, thereby driving the inflammatory process and leading to osteoclast activation and bone resorption in the oral cavity (Holt and Bramanti, 1991; Socransky and Haffajee, 1992; Hajishengallis et al., 2011; Barth et al., 2012). In chronic periodontitis, bacteria persist within an environment rich in activated neutrophils and monocyte/macrophages, inflammatory cytokines, enzymes, and reactive oxygen species (Delima and Van Dyke, 2003; Ryder, 2010; Nussbaum and Shapira, 2011). In fact, inflammation provides the nutritional support for the asaccharolytic communities of bacteria such as P. gingivalis by releasing tissue breakdown products such as peptides and heme-containing compounds (Hajishengallis, 2014). Considering inflammation as a nutrient source can explain the clinical observation that bacterial numbers increase in accordance with the degree of host inflammation. However, activated immune cells kill bacteria by intracellular and extracellular mechanisms, so to thrive in a highly inflamed milieu, P. gingivalis must dissociate the beneficial effects of inflammation from bactericidal activity (Nussbaum and Shapira, 2011; Hajishengallis et al., 2012; Hajishengallis, 2014). The role of the innate immune receptor Toll-Like Receptor 2 (TLR2) in the host response to P. gingivalis exemplifies this dissociation—sensing of infection and the inflammatory response are driven by TLR2, however, the response does not lead to killing of P. gingivalis. Indeed, the absence of TLR2, rather than its presence, confers resistance to disease (Burns et al., 2006), although the underlying mechanisms are incompletely understood. TLR2-driven inflammation is normally linked to bactericidal activity and to osteoclast activation and bone loss through signaling initiated by the intracellular adaptor protein MyD88 (Pandey et al., 2014). Therefore, to evade bactericidal activity, P. gingivalis disrupts MyD88 activity, as demonstrated in neutrophils (Maekawa et al., 2014) and in vivo (Mizraji et al., 2017), but surprisingly it does not disrupt TLR2-driven inflammation (Burns et al., 2010). Although, MyD88 has been linked to a number of pathological inflammatory conditions (Kfoury et al., 2014; Kassem et al., 2015a), we demonstrate here that P. gingivalis induces bone resorption independently of MyD88. Moreover, the MyD88-independent TLR2 activation induced by P. gingivalis stimulates PI3K signaling that drives inflammation but at the same time depresses phagocytosis and enables phagocytosed bacteria to escape lysosomal degradation. Therefore, activation of TLR2-PI3K occurs independently of MyD88 and is critical for P. gingivalis to escape immunity and drive inflammatory bone resorption. Understanding the mechanisms that uncouple inflammation from bactericidal activity can lead to novel therapeutic approaches for chronic inflammatory tissue damage associated with dysbiotic microbiota.
LY 294002 and LY 303511 were from Sigma-Aldrich (Rehovot, Israel). Pam3CSK4 was obtained from InvivoGen (San Diego, CA). T2.5 monoclonal antibody (mAb) against mouse and human TLR2 was from Hycult Biotech (Uden, Netherlands), mAb 1A6 was a gift from Greg Elson (NovImmune, Geneva, Switzerland), and isotype control mAbs were from BioLegend (San Diego, USA). Recombinant proteins were from Peprotech (Rehovot, Israel). LysoTracker® Red DND-99 was from ThermoFischer Scientific (MA, USA).
P. gingivalis (ATCC strains 381 and 53,977 were used in this study) was cultured for 48 h in Wilkins broth (Oxoid, Hampshire, England) without additional nutrients, under anaerobic conditions in Oxoid™ AnaeroJar™ 2.5 L at 37°C. An OD value of 0.1 (650 nm) was determined to correlate to 1010 CFU per ml.
C57BL/6 were from Envigo (Rehovot, Israel), and Tlr2−/−, and Myd88−/− mice backcrossed to the C57BL/6 background were a kind gift from Dr. S. Akira (Osaka University, Osaka, Japan). All mice were housed at the SPF unit of Hebrew University. Tlr2−/−/Myd88−/− double-knockout mice were generated by screening F2 progeny of Tlr2−/− females crossed with Myd88−/− males as described previously (Burns et al., 2010). The institutional animal care and use committee of the Hebrew University of Jerusalem approved all experiments and experiments complied with the guidelines of the National Research Council Guide for the Care and Use of Laboratory Animals (NIH Publication no. 85-23, revised 1996).
RAW 264.7 and THP1 cell lines were obtained from the American Type Culture Collection (ATCC, USA). RAW264.7 cells were maintained in DMEM and THP-1 cells in RPMI (Sigma-Aldrich, Israel) and both were supplemented with 10% fetal calf serum, 2 mM L-glutamine, penicillin (100 units/ml), streptomycin (100 μg/ml; Biological Industries, Israel). In addition, 1% 1M HEPES, and sodium pyruvate were added to the culture medium of THP-1 cells. The cell lines were cultured at 37°C and 5% CO2. THP-1 cells were differentiated to mature macrophages by the addition of PMA each for 72 h.
Mice were sacrificed and the tibia and femur were extracted and cleaned from excess flesh. The ends of each tibia and femur were clipped with dissecting scissors and the bone marrow cells were flushed with ice cold PBS−/− using a syringe with 27-G needle. The pooled bone marrow was then separated by gentle pipetting, followed by filtration through a sterile 70-μm nylon cell strainer to remove cell clumps and bone particles. The filtrated cells were centrifuged for 5 min at 1,500 rpm and resuspended in complete DMEM. To prepare Bone Marrow Macrophages (BMM), cells were plated 5 × 106 cells/ plate and cultured in complete DMEM supplemented with 15% L cell supernatant as a source of M-CSF, and 15% horse serum. Media was replaced after 3 days and cells were collected for use after 1 week. To stimulate, BMM were plated in triplicate at 400,000 cells/well, in 96-well flat bottom plates in complete DMEM. Bone marrow neutrophils were prepared by negative or positive selection from pooled bone marrow cells. Negative selection was performed using the EASYSEP® magnet cell selection system (STEMCELL Technologies, Vancouver, Canada) according to the manufacturer's instructions. Positive selection was performed using the MACS® mouse Anti-Ly-6G MicroBead Kit (Miltenyi Biotec Inc., CA, USA) according to manufacturer's instructions.
Mice were administered 1 ml Glycogen (Sigma) and after 4 h the mice were sacrificed and PECs were collected from the peritoneal cavity by washing with cold / free PBS.
Alveolar bone loss was induced by P. gingivalis oral infection as previously described (Baker et al., 2000). Briefly, mice (n ≥ 8) were treated with Sulfamethoxazole (0.4% solution in drinking water) for 10 days, followed by 3-days without antibiotics. Mice were infected with live P. gingivalis in PBS (4 × 109 CFU) containing 2% carboxymethylcellulose (“vehicle”) using a round-tipped feeding needle three times at 2-day intervals. Control groups were treated with vehicle alone. In experiments for locating P. gingivalis in host tissues, 1, 7, and 14 days after the last challenge mice were sacrificed and gingiva and lung were obtained. Tissue samples were immediately frozen in −20°C until they were processed for DNA isolation. In experiments measuring alveolar bone resorption 6 weeks after the first challenge mice were sacrificed and maxillae were scanned by μCT (SCANCO Medical, Switzerland). Three-dimensional alveolar bone loss was quantified as reported (Wilensky et al., 2005; Steinmetz et al., 2016; Mizraji et al., 2017). Approximately 180 slices 12 μm wide were scanned for each sample, covering the entire bucco-palatal aspect.
Tissue samples were incubated in lysis buffer (200 mM NaCl, 5 mM EDTA, 0.2% SDS, 100 mM Tris pH 8, 100 μm/ml Proteinase K) overnight at 55°C. After centrifugation and transfer of clear supernatant, DNA was precipitated by adding isopropanol. DNA was washed, dried, and resuspended in double-distilled water. P. gingivalis was identified in host tissue by using specific primers recognizing a 432 bp fragment of the P. gingivalis 16S gene. Forward primer sequence 5′-AGAGTTTGATCCTGCTCAG-3′ and reverse primer sequence 5′-CAATACTCGTATCGCCCGTTATTC -3′. Reaction conditions used were: 94°C for 2 min followed by 40 cycles of 30 s at 94°C, 30 s at 63°C, 1 min at 72°C. PCR products were analyzed on 1% agarose gel with 100 bp DNA ladder (NEB, USA).
Cytokine levels were determined by ELISA using mouse and human Elisa MAX TM sets (Biolegend, San Diego, CA), according to the manufacturer's instructions.
Macrophages were seeded in 96 well flat bottom plates and challenged with FITC-labeled P. gingivalis. To label the bacteria, P. gingivalis was incubated with 0.1 mg/ml FITC (Sigma, Israel) in carbonate buffer (pH 9.5) for 20 min at RT and then extensively washed in PBS. Following challenge, macrophages were washed twice with PBS and then incubated with 1 ml of 1X trypan blue for 1 min to quench extracellular fluorescence from bacteria attached to the external surface of the macrophages. Cells were washed twice with PBS−/− and fluorescence was determined using a GENios Microplate Reader (Tecan, Männedorf, Switzerland).
Intracellular survival was determined using an antibiotic protection assay. 106 macrophages/well were plated in 6-well plates and challenged with live P. gingivalis at multiplicity of infection (MOI) of 10 for 1 h. Cells were then washed twice and treated with metronidazole and gentamycin for an additional hour to kill all remaining extracellular bacteria. Following antibiotic treatment, cells were washed twice and incubated in medium without antibiotics for an additional hour. Cells were then lysed by incubation in ice cold DDW for 20 min. Serial dilutions of lysates were plated on blood agar plates (Novamed, Jerusalem, Israel), and incubated under anaerobic conditions for 7 days to determine the number of Colony Forming Units (CFU).
Thirty thousand cells/well were plated in U-Slide 8 well-IBIDI tissue culture treated chamber slides (Ibidi, Martinsried, Germany). Cells were blocked as indicated for 1 h prior to challenge with FITC-labeled P. gingivalis for another hour. Lysotracker red (50 mM) was added to cells 10 min prior to fixing. Cells were washed twice, fixed with 2% formaldehyde for 10 min and then washed with PBS for 5 min and IBIDI mounting medium was applied (Ibidi). Multi-projection images were obtained using a NIKON confocal fluorescent microscope at 60X magnification.
The 2-Tailed t-test was used for statistical evaluation of all the results. Values are shown for data that reached a significance of P ≤ 0.05 (*), P ≤ 0.01 (**), P ≤ 0.005 (***). Bars show mean and standard deviation (s.d.; Prism v.5, GraphPad Software Inc. San Diego, USA).
Repeated oral challenge of mice with P. gingivalis leads to its colonization followed by chronic inflammation, osteoclast activation, and resorption of alveolar bone surrounding the teeth (Baker et al., 2000). We and others demonstrated that TLR2-deficient mice are resistant to alveolar bone loss following oral challenge with P. gingivalis (Burns et al., 2006; Papadopoulos et al., 2013), consistent with the role of TLR2, together with C5aR, in mediating the inflammatory response to P. gingivalis that leads to osteoclast differentiation (Maekawa et al., 2014). Since the canonical signaling pathway of TLR2 requires the adaptor protein MyD88, we set out to test if the phenotype of resistance to alveolar bone resorption would be recapitulated in MyD88-deficient mice. Surprisingly, in contrast to TLR2-deficient mice, the absence of MyD88 did not prevent P. gingivalis from inducing alveolar bone resorption, as shown for a non-encapsulated P. gingivalis strain (ATCC 381), and an additional strain that expresses a polysaccharide capsule (ATCC 53977; Figures 1A,B). We next tracked the clearance of P. gingivalis by PCR from mouse tissues 24 h and 7 days following the last oral challenge. Consistent with the importance of TLR2 in bacterial immune evasion, P. gingivalis was not detectable in the gingival tissue of most Tlr2−/− mice at 24 h following the third oral challenge, and was not detected in any mice 7 days later (Table 1). In contrast, P. gingivalis was detected in all WT and MyD88-deficient mice 24 h following the third challenge, and was still detectable in the gingiva of most mice 7 days later. We also tested for P. gingivalis in the lungs of orally-infected mice as a measure of bacterial dissemination (either via aspiration during oral challenge or through spreading infection). Whereas, P. gingivalis was detected in the lungs of all WT and Myd88−/− mice 24 h after the last challenge, there was no evidence of bacteria in the lungs of Tlr2−/− mice. These data collectively suggested that MyD88 does not mediate the TLR2-dependent evasive effects that promote P. gingivalis persistence, required for disease. Accordingly, to examine whether P. gingivalis infection leads to TLR2-dependent but MyD88-independent bone resorption, we infected TLR2/MyD88 double knock-out mice. First, we observed that P. gingivalis could be detected in the double knock-out mice where its survival was similar to the single MyD88 knock-out mice (Table 1), indicating that the absence of TLR2 (and hence the ability of P. gingivalis to evade immunity) is without serious consequences when MyD88 is also absent (hence MyD88 is required for immune clearance). Strikingly, despite the fact that the double knock-out mice were colonized by P. gingivalis, they were completely resistant to infection-driven bone resorption (Figure 1A), suggesting an absolute requirement for TLR2 in pathologic bone loss in this model. Taken together, these results demonstrate that MyD88-independent TLR2 signaling is necessary for bacterial persistence, and that TLR2-driven inflammation promotes oral infection-driven bone resorption even in the absence of the canonical TLR2 adaptor protein MyD88.
Figure 1. Experimental periodontitis induced by oral challenge with P. gingivalis. Groups of mice were administered P. gingivalis in CMC vs. CMC alone by repeated oral gavage. Six weeks later, the maxillae were harvested and alveolar bone volume was measured by μCT from the cemento-enamel junction to a reference line. The residual bone volume of P. gingivalis infected mice was compared to vehicle-treated mice (n = 8–10 per group). (A) WT, Tlr2−/−, Myd88−/−, and Tlr2/Myd88 double knock-out mice (DKO) infected with P. gingivalis ATCC 381. (B) An independent experiment examined bone loss in WT vs. Myd88−/− mice infected with P. gingivalis ATCC 381 vs. P. gingivalis ATCC 53977. Ns, non-significant. **P ≤ 0.01, ***P ≤ 0.005.
To dissect the mechanism by which P. gingivalis can induce a TLR2-dependent but MyD88-independent inflammatory response, we next studied the role of MyD88 in response to infection with P. gingivalis in vitro, and compared this response to that induced by the prototypical lipopeptide Pam3CSK4, which stimulates TLR2 in a strictly MyD88-dependent manner. We previously reported that in-vivo, the cytokine response to subcutaneous challenge with P. gingivalis is similar in WT and Myd88−/− mice (Burns et al., 2010). Since >70% of the cells responding to subcutaneous challenge are neutrophils (Steinmetz et al., 2016), we hypothesized that TLR2-dependent, MyD88-independent signaling may be most prominent in this cell type. In vitro activation of a mixed population of bone marrow (BM) cells from MyD88-deficient mice with P. gingivalis induced significant cytokine production (Figure 2A). Consistently, moreover, enrichment of the neutrophil population by negative selection (achieving roughly 60% Ly6G+ cells) further enhanced the response (Figure 2A). Myd88−/− peritoneal exudate cells (PECs) collected 4 h following glycogen administration (~89% neutrophils), and positively-selected Ly6G+ bone marrow neutrophils (>96% purity) also responded to P. gingivalis challenge in vitro, but notably failed to respond to either Pam3CSK4 or E. coli LPS (Figures 2B,C). However, WT BM cells secreted more TNF when stimulated with P. gingivalis than Myd88−/− BM cells (Figure 2D), suggesting that both MyD88-dependent and independent pathways are activated in response to challenge in vitro.
Figure 2. Myd88−/− neutrophils respond to challenge with P. gingivalis. (A) Total Myd88−/− bone marrow cells and neutrophil-enriched bone marrow cells were plated 4*105 cells/well in a 96-well plate and incubated with P. gingivalis (MOI 100). (B) Glycogen-induced Myd88−/− PECs, or naïve bone marrow Myd88−/− Ly6G+ neutrophils isolated by positive selection (C) produce TNF-α in response to P. gingivalis challenge but not in response to S. minnesota LPS (1 μg/ml) or Pam3CSK4 (10 μg/ml), or buffer control. (D) The response of Myd88−/− total and neutrophil enriched BM was compared to the response of WT BM cells. (E) BM cells were collected from WT and Myd88−/− mice and primed with GM-CSF or IFN-γ for 2 h prior to challenge with P. gingivalis (E). The percent increase in TNF produced in response to P. gingivalis challenge in primed cells vs. unprimed cells is shown. (A–E) Supernatants were collected after overnight challenge and the level of TNF in the supernatants was measured by ELISA. One representative experiment is shown in each case. Experiments were repeated 3–5 times. *P ≤ 0.05, **P ≤ 0.01, ***P ≤ 0.005.
We next hypothesized that factors known to prime cells responding to infection, such as IFN-γ and Granulocyte-Macrophage Colony-Stimulating Factor (GM-CSF), may contribute to the ability of P. gingivalis to induce TLR2-dependent, MyD88-independent inflammatory signaling. To test this notion, WT and Myd88−/− BM neutrophils were exposed to GM-CSF or IFN-γ for 2 h prior to challenge with live P. gingivalis. Priming cells with either compound alone did not produce significant amounts of TNF. Although GM-CSF priming had no effect on the Myd88−/− neutrophil response to P. gingivalis, IFN-γ priming significantly increased the response to bacterial challenge (Figure 2E). In contrast, IFN-γ priming did not induce MyD88-independent TNF production in response to Pam3CSK4 (data not shown). Thus, IFN-γ enhances TLR2, MyD88-independent signaling in neutrophils in response to P. gingivalis but not necessarily to other TLR2 agonists.
In addition to neutrophils, macrophages predominate in the host response to subcutaneous or oral infection with P. gingivalis, where they regulate the neutrophil response (Lam et al., 2014; Steinmetz et al., 2016). Interestingly, Myd88−/− BM-derived macrophages (BMM) required IFNγ priming in order to respond to increasing MOI of P. gingivalis infection (Figures 3A,B). Consistent with the Myd88−/− neutrophil response, priming did not enable Myd88−/− BMM to respond to Pam3CSK4 (Figure 3A). We previously showed that P. gingivalis promotes its survival in vivo by cross-talk signaling between TLR2 and C5aR1 in a manner dependent on phosphatidylinositol-3-OH kinase (PI3K; Maekawa et al., 2014). We now show that inhibition of PI3K completely abrogated MyD88-independent cytokine production in response to P. gingivalis in vitro (Figures 4A–C), suggesting that PI3K is a crucial component of the MyD88-independent TLR2 pathway. Inhibition of p38K, a downstream MAPK, also blocked cytokine production in Myd88−/− cells (Figures 4A,B), whereas blocking other factors implicated in TLR2 signaling such as mTORC1 (Lorne et al., 2009) or RAC1 (Arbibe et al., 2000; Harokopakis et al., 2006) either partially disrupted (mTORC1) or did not affect (RAC1) MyD88-independent signaling (Figures 4A,B). We further confirmed that MyD88-independent signaling in response to P. gingivalis is dependent on TLR2 using specific blocking antibodies. Indeed, blocking TLR2 or inhibition of PI3K on Myd88−/− BMM reduced the P. gingivalis-induced response in a dose-dependent manner (Figure 4C), and to background levels, whereas blocking TLR4 had no effect (Figure 4D). Therefore, IFNγ priming facilitates a distinctive TLR2-PI3K signaling pathway that is completely independent of MyD88, leading to inflammatory cytokine production in response to P. gingivalis.
Figure 3. IFN-γ priming enables TLR2-dependent, MYD88-independent signaling in macrophages in response to P. gingivalis challenge. (A) Naïve Myd88−/− BMM vs. BMM primed with IFN-γ (100 ng/ml) for 2 h were challenged with P. gingivalis (MOI 100) vs. Pam3CSK4 (10 μg/ml). In (B) Myd88−/− BMM primed with IFN-γ were challenged with increasing MOI of P. gingivalis. (A,B) Supernatants were collected after overnight stimulation and tested for TNF by ELISA. **P ≤ 0.01, ***P ≤ 0.005.
Figure 4. Kinase involvement in the Myd88−/− response to P. gingivalis. (A) Ly6C+ BM neutrophils or (B–D) BMM were prepared from Myd88−/− mice and primed with IFN-γ (100 ng/ml for 2 h). (A,B) Inhibitors for PI3K (LY 2940002 100 μM), p38 MAPK (SB 202190 50 μM), mTORC1 (RaPamycin 60 nM) and RAC1 inhibitor (NSC 23766, 50 μM) were added 30 min before challenge with P. gingivalis (MOI 100). DMSO was used as a control at the highest concentration used in the inhibitor wells. Supernatants were collected after overnight stimulation and the percent inhibition of TNF production is shown. (C) Myd88−/− BMM were similarly primed and Ly294 was added at increasing concentrations 30 min prior to challenge with P. gingivalis. (D) Myd88−/− BMM were primed with IFN-γ and antibodies (20 μg/ml anti-TLR2 or TLR4 vs. isotype control, I.C.) or LY294 were added prior to challenge with P. gingivalis. *P ≤ 0.05, **P ≤ 0.01, ***P ≤ 0.005.
Previous studies using macrophages from gene knockout mice suggested that MyD88-dependent TLRs, such as TLR4 (Papadopoulos et al., 2013) or TLR9 (Kim et al., 2015), contribute to cytokine production in response to P. gingivalis. In line with these findings, even though MyD88-deficient cells responded to P. gingivalis in the present study, the response is diminished compared to WT cells. These studies suggest that cells can respond to P. gingivalis through multiple redundant pathways. We therefore next determined the importance of TLR2-PI3K signaling in response to P. gingivalis using WT mouse and human macrophages. Blocking TLR2, but not TLR4, and inhibition of PI3K, reduced cytokine production to near background levels in primary BMM and the murine RAW264.7 macrophage cell line responding to infection with P. gingivalis (Figures 5A,B). The specificity of the blocking antibodies to TLR2 and TLR4 was confirmed using murine and human macrophages challenged with Pam3CSK4 (for the inhibitory antibody to TLR2) and LPS (for the antibody to TLR4). As expected, inhibition of PI3K did not affect cytokine production induced by Pam3CSK4 (data not shown). The role of TLR2 and PI3K in the response to P. gingivalis was also confirmed in the human THP-1 macrophage cell line primed with IFNγ (Figure 5C). Therefore, in WT cells with functional TLRs and MyD88, the TLR2-PI3K pathway induced by P. gingivalis plays a non-redundant role in cytokine production.
Figure 5. TLR2-PI3K plays a non-redundant role in the murine and human macrophage response to P. gingivalis. WT murine BMM (A), RAW264.7 macrophages (B), and PMA-differentiated human THP-1 cells (C) were primed with IFN-γ. TLR2 and TLR4 were inhibited with blocking antibodies vs. isotype control (I.C.) for 1 h and PI3K was blocked with LY294 prior to challenge with P. gingivalis (MOI 10). Supernatants were collected after overnight incubation and TNF was measured by ELISA. Background (BG) represents IFN-γ primed cells not challenged with P. gingivalis. Cells challenged with P. gingivalis without any blocker are referred to in the graphs as (–). Representative graphs of >3 repeats are shown. *P ≤ 0.05, **P ≤ 0.01, ***P ≤ 0.005.
In vivo, TLR2 signaling promotes the survival of P. gingivalis and enables infection to drive dysbiosis and alveolar bone resorption (Maekawa et al., 2014). The results above demonstrate that the TLR2-PI3K pathway drives inflammation in response to P. gingivalis, but the inflammatory response is disconnected from immune bacterial clearance in vivo. To identify the mechanism underlying the TLR2-dependent evasion of P. gingivalis clearance, we next determined the effects of TLR2-PI3K signaling in vitro on the ability of macrophages to phagocytose and eradicate P. gingivalis. Phagocytosis was measured in a florescence plate-based assay by labeling the bacteria with FITC and quenching extracellular florescence with Trypan Blue after phagocytosis. In stark contrast to results with other organisms whose phagocytosis is promoted by TLR2 and/or PI3K signaling (Shin et al., 2009; Giraldo et al., 2010; Fang et al., 2014), blocking TLR2 or PI3K, but not TLR4, significantly enhanced WT murine and human macrophage phagocytosis of P. gingivalis (Figures 6A,B). Next, we tracked the fate of phagocytosed bacteria using an antibiotic protection assay (Hajishengallis et al., 2017). Despite reduced entry of P. gingivalis into the macrophages when the TLR2-PI3K pathway is active, we observed higher intracellular bacterial burden under this condition than when the TLR2-PI3K pathway was blocked (Figure 6), suggesting that the P. gingivalis-induced TLR2-PI3K pathway suppresses phagocytosis but, even if phagocytosed, the bacteria have a way to escape killing. Indeed, blocking either TLR2 or PI3K significantly improved eradication of phagocytosed bacteria, whereas blocking TLR4 had no effect (Figure 6C). TLR4 does not mediate the bactericidal activity observed when TLR2 is inhibited since co-inhibition of TLR2 and TLR4 led to similar diminished P. gingivalis survival as when TLR2 is blocked alone (Figure 6D). Thus, the TLR2-PI3K pathway is responsible for both inflammatory signaling and bacterial evasion from killing via a two-pronged mechanism; (i) reduced phagocytosis and (ii) enhanced intracellular survival within those cells that do phagocytose bacteria.
Figure 6. TLR2-PI3K signaling suppresses phagocytosis and enhances intracellular survival. (A) RAW264.7 or (B) PMA-differentiated THP-1 cells were treated with blocking antibodies or PI3K inhibitor and then challenged with FITC-labeled P. gingivalis at MOI 10 for 1 h. Cells were then washed, extracellular fluorescence was quenched with trypan blue, and phagocytosis was determined using a fluorescence plate reader (RFU, relative fluorescence units). (C,D) RAW 264.7 cells were treated with TLR blocking antibodies or the PI3K inhibitor prior to challenge with P. gingivalis at MOI 10 for 1 h. Cells were then washed and extracellular bacteria were killed by incubating the cells with Metronidazole and Gentamycin for 1 h. Cells were allowed to recover in fresh media for an additional hour after which they were lysed by DDW for 20 min and lysates were plated on blood agar plates in serial dilution. CFU were enumerated after 7 days of anaerobic growth. *P ≤ 0.05, **P ≤ 0.01, ***P ≤ 0.005.
To investigate the mechanism by which the TLR2-PI3K pathway promotes the survival of internalized P. gingivalis bacteria, we tracked the intracellular fate of P. gingivalis in the presence or absence of TLR2-PI3K signaling. To this end, we labeled bacteria with FITC and labeled acidified vacuoles with the lysosomal marker lyso-tracker red, a dye that fluoresces in a low pH environment. Phagosome maturation and lysosomal fusion would lead to co-localization of the FITC signal with the LysoTracker signal. Cells were treated with inhibitors prior to challenge with FITC-labeled P. gingivalis, and Lyso-tracker was added 15 min prior to fixing the cells. When TLR2 or PI3K were inhibited, there were more internalized bacteria, consistent with the enhanced phagocytosis shown in Figure 6. However, in stark contrast to untreated or isotype control treated cells, when TLR2 or PI3K were inhibited most internalized bacteria were found in lysosomes (Figure 7). Therefore, TLR2-PI3K signaling enhances P. gingivalis intracellular survival by inhibiting phago-lysosomal maturation.
Figure 7. TLR2-PI3K signaling enhances intracellular survival by blocking phago-lysosomal maturation. RAW 264.7 cells were seeded at 3 × 104 cells/ well in Ibidi 8 well m-slides. The cells were untreated (A) or treated with anti-TLR2 (B), anti-TLR4 (C), or with the PI3K inhibitor LY 294002 (D) for an hour. Cells were then infected with FITC-labeled P. gingivalis at MOI 10 for 1 h. LysoTracker red was added at 50 nM for the last 10 min of infection. Cells were washed and fixed with 2% formaldehyde and mounted with mounting media. Images were captured using a NIKON confocal microscope at 60X magnification. Yellow color indicates co-localization of P. gingivalis (green) with lysosomes (red). In each field (A–D) the cell in the box is further magnified and shown in the upper right corner. (E) The percent of co-localization was determined by counting cells that demonstrate co-localization as a percentage of all FITC positive cells. (F) Schematic representation of the pathway used by P. gingivalis to evade bactericidal activity without preventing inflammation. ***P ≤ 0.005.
Repeated oral inoculation of mice with P. gingivalis leads to its colonization, quantitative and compositional alterations to the periodontal microbiota and the induction of an acute inflammatory infiltrate in the gingival tissue dominated by neutrophils and monocyte/macrophages, which in turn contribute to bone resorption surrounding the teeth (Baker et al., 2000; Burns et al., 2006; Hajishengallis et al., 2011; Papadopoulos et al., 2013). Our study shows for the first time that P. gingivalis-induced bone loss is TLR2-dependent but MyD88-independent. Our findings stand in contrast to the dominant role of MyD88 in TLR2-driven responses in general (Pandey et al., 2014), and in particular in the setting of osteoclastogenesis. MyD88 is essential for osteoclastogenesis induced by IL-1, and ligands of TLR4 or TLR2 (Sato et al., 2004; Kim et al., 2013). TLR2-dependent osteoclast differentiation and bone resorption in response to infection with S. aureus and B. abortus were also shown to be MyD88 dependent (Delpino et al., 2012; Kim et al., 2013). Finally, MyD88 is essential for TLR2-driven osteoclastogenesis in vitro in response to P. gingivalis or its isolated LPS (Zhang et al., 2011; Kassem et al., 2015b), and for alveolar bone loss induced by LPS from other oral pathogens (Madeira et al., 2013). Although, MyD88 plays a role in in vitro osteoclastogenesis involving direct interactions of P. gingivalis with osteoclast precursors (Zhang et al., 2011), the significance of MyD88 may be different in biologically more relevant settings that include all cellular players (i.e., both immune and bone cells). Indeed, the ability of live P. gingivalis to manipulate MyD88 in immune cells and induce TLR2 signaling via alternative adaptors results in the induction of TLR2-dependent, MyD88-independent inflammation that leads to bone loss. We earlier showed that MyD88 promotes immune clearance of P. gingivalis (Burns et al., 2010), which is consistent with our subsequent findings that the same pathogen induces MyD88 degradation in neutrophils (Maekawa et al., 2014) and in the oral tissue (Mizraji et al., 2017) to suppress the bactericidal activity mediated by MyD88. In the absence of both TLR2 and MyD88, P. gingivalis persists in the oral cavity but is unable to induce bone loss, further confirming the essential role of TLR2-dependent, MyD88-independent inflammation in bone loss that develops in response to infection.
Although P. gingivalis induced Myd88−/− neutrophils to produce TNF in vitro, the cells remained unresponsive to challenge with the triacylated lipopeptide Pam3CSK4. Furthermore, exposure to IFNγ enhanced the neutrophil cytokine response to P. gingivalis without enabling the cells to respond to Pam3CSK4. Interestingly, Myd88−/− macrophages required priming with IFNγ to respond to challenge with P. gingivalis, but still did not respond to Pam3CSK4. These findings suggest that the repertoire of available signaling molecules downstream to TLR2 is determined by the nature of the ligand and the type of the responding cell. P. gingivalis induces TLR2 crosstalk with complement and chemokine receptors (e.g., C5aR and CXCR4), expanding potential signaling pathways (Hajishengallis et al., 2008; Maekawa et al., 2014). Since they are the most abundant cell type responding to infection, neutrophils may be the prime target of immune evasion by P. gingivalis. To avoid killing, P. gingivalis degrades neutrophil MyD88 (Maekawa et al., 2014); nevertheless, we show that even unprimed neutrophils maintain inflammatory signaling in the absence of MyD88. Thus, inflammation, which benefits P. gingivalis by providing a nutrient source, is uncoupled from bactericidal activity mediated by MyD88 (Figure 7F).
As shown here, PI3K plays a central role in the MyD88-independent TLR2 response of neutrophils and macrophages challenged with P. gingivalis. PI3K may be recruited to TLR2 by the adaptor protein Mal/TIRAP (MyD88-adapter-like/TIR-domain-containing adaptor protein; Maekawa et al., 2014). Mal/TIRAP bridges between TLR2 and MyD88 (Pandey et al., 2014). However, since Mal/TIRAP can also interact with PI3K (Strassheim et al., 2004; Santos-Sierra et al., 2009), it may serve to bridge between TLR2 and PI3K in the absence of MyD88 (Maekawa et al., 2014).
The ability of P. gingivalis to exploit TLR2 signaling to block phagosome maturation and lysosomal fusion, thereby escaping intracellular killing in macrophages, constitutes a hitherto undescribed mechanism that can readily explain earlier observations. For instance, P. gingivalis was shown to persist at higher intracellular viable counts in WT than in Tlr2−/− macrophages, although the mechanistic basis for this effect was uncertain (Hajishengallis et al., 2007). Moreover, disruption of membrane lipid rafts by cholesterol depletion was shown to promote the colocalization of P. gingivalis with lysosomes in macrophages (Wang and Hajishengallis, 2008). Although, the same study showed that lipid raft disruption impairs TLR2 signaling, the latter was not linked to alterations in phagolysosomal fusion. Our findings are consistent with TLR2-mediated escape of certain mycobacterial species from phagosomal degradation in monocytes (Weiss et al., 2008), although TLR2 engagement by other species promotes their phagosomal degradation (Gomes et al., 2008). Similarly, distinct PI3K classes modulate various steps of phagosome maturation, although in most cases PI3K activation promotes, rather than inhibits, maturation, and fusion to lysosomes (Thi and Reiner, 2012). Therefore, when PI3K acts to inhibit phagolysosomal fusion, this likely implies proactive microbial manipulation of host signaling, as shown in the present study. Identification of the particular PI3K classes induced by P. gingivalis downstream of TLR2 may shed light on the mechanism through which bacteria prevent lysosomal degradation.
In addition to its well-established pro-inflammatory properties, MyD88 signaling is critical to phagocytosis and phagosome maturation in response to gram-negative and gram-positive bacteria, as shown by numerous reports (Blander and Medzhitov, 2004; Yates and Russell, 2005). Therefore, besides serving as an alternative pathway for induction of inflammation, TLR2-PI3K signaling enables bacteria to escape phagocytosis and, even when phagocytosed, to escape lysosomal degradation. These results of the present study are consistent with, and explain mechanistically the reduced phagocytosis and enhanced P. gingivalis survival in Myd88−/− mice (as compared to WT mice) in the subcutaneous chamber infection model where neutrophils and macrophages constitute the vast majority of cells responding to challenge (Burns et al., 2010; Steinmetz et al., 2016). Since neutrophils and monocyte/macrophages predominate in the oral niche where P. gingivalis thrives, it makes sense that P. gingivalis evolved to escape killing by these “professional killers.” However, TLR2 may play an opposite role in dendritic cells where TLR2 signaling was shown to enhance bactericidal activity (El-Awady et al., 2015; Hajishengallis et al., 2017). The cell-type specific factors that enable P. gingivalis to hijack TLR2 signaling in macrophages and neutrophils, but not in dendritic cells, have yet to be identified. P. gingivalis also invades and survives within epithelial and endothelial cells via manipulation of vesicle trafficking pathways (Dorn et al., 2001; Takeuchi et al., 2011) or through survival mechanisms within phagolysosomes (Yamatake et al., 2007). Although, the role of TLR2 in directing these events has not been fully explored, P. gingivalis stimulates and exploits PI3K signaling in gingival epithelial cells to promote its intracellular persistence (Yilmaz et al., 2004), suggesting that a similar evasion pathway exists in these diverse cell types.
P. gingivalis uncouples TLR2-driven inflammation from bactericidal activity by substituting PI3K in place of MyD88 signaling. Beyond the advantage to P. gingivalis itself, the creation of an inflammatory environment with impaired immunity benefits other inflammophilic anaerobic bacteria, further supporting the role of P. gingivalis as a keystone pathogen (Hajishengallis et al., 2012). Targeting the TLR2-PI3K escape pathway may improve therapeutic outcomes in P. gingivalis-driven periodontal bone loss.
GN, GH, and CK conceived and designed experiments. HM, SH, EB, performed and analyzed in vivo and in vitro experiments. HM and GN wrote the first draft of the manuscript. GN, GH, KH edited text and figures.
This work was supported by grants from the German-Israel Foundation (grant G-1126-202.13/2010 to GN and CK), the Israel Science Foundation (grant 1396/12 to GN), and Grant DE015254 from the National Institutes of Health (to GH). HM gratefully acknowledges the STEP-GTP foundation for a student stipend.
The authors declare that the research was conducted in the absence of any commercial or financial relationships that could be construed as a potential conflict of interest.
The reviewer JP and handling Editor declared their shared affiliation, and the handling Editor states that the process nevertheless met the standards of a fair and objective review.
Arbibe, L., Mira, J. P., Teusch, N., Kline, L., Guha, M., Mackman, N., et al. (2000). Toll-like receptor 2-mediated NF-κ B activation requires a Rac1-dependent pathway. Nat. Immunol. 1, 533–540. doi: 10.1038/82797
Armitage, G. C. (1996). Periodontal diseases: diagnosis. Ann. Periodontol. 1, 37–215. doi: 10.1902/annals.1996.1.1.37
Baker, P. J., Dixon, M., Evans, R. T., and Roopenian, D. C. (2000). Heterogeneity of Porphyromonas gingivalis strains in the induction of alveolar bone loss in mice. Oral Microbiol. Immunol. 15, 27–32. doi: 10.1034/j.1399-302x.2000.150105.x
Barth, K., Remick, D. G., and Genco, C. A. (2012). Disruption of immune regulation by microbial pathogens and resulting chronic inflammation. J. Cell Physiol. 228, 1413–1422. doi: 10.1002/jcp.24299
Blander, J. M., and Medzhitov, R. (2004). Regulation of phagosome maturation by signals from toll-like receptors. Science 304, 1014–1018. doi: 10.1126/science.1096158
Burns, E., Bachrach, G., Shapira, L., and Nussbaum, G. (2006). Cutting edge: TLR2 is required for the innate response to Porphyromonas gingivalis: activation leads to bacterial persistence and TLR2 deficiency attenuates induced alveolar bone resorption. J. Immunol. 177, 8296–8300. doi: 10.4049/jimmunol.177.12.8296
Burns, E., Eliyahu, T., Uematsu, S., Akira, S., and Nussbaum, G. (2010). TLR2-dependent inflammatory response to Porphyromonas gingivalis is MyD88 independent, whereas MyD88 is required to clear infection. J. Immunol. 184, 1455–1462. doi: 10.4049/jimmunol.0900378
Delima, A. J., and Van Dyke, T. E. (2003). Origin and function of the cellular components in gingival crevice fluid. Periodontol. 2000 31, 55–76. doi: 10.1034/j.1600-0757.2003.03105.x
Delpino, M. V., Barrionuevo, P., Macedo, G. C., Oliveira, S. C., Genaro, S. D., Scian, R., et al. (2012). Macrophage-elicited osteoclastogenesis in response to Brucella abortus infection requires TLR2/MyD88-dependent TNF-α production. J. Leukoc. Biol. 91, 285–298. doi: 10.1189/jlb.04111185
Dorn, B. R., Dunn, W. A. Jr., and Progulske-Fox, A. (2001). Porphyromonas gingivalis traffics to autophagosomes in human coronary artery endothelial cells. Infect. Immun. 69, 5698–5708. doi: 10.1128/IAI.69.9.5698-5708.2001
El-Awady, A. R., Miles, B., Scisci, E., Kurago, Z. B., Palani, C. D., Arce, R. M., et al. (2015). Porphyromonas gingivalis evasion of autophagy and intracellular killing by human myeloid dendritic cells involves DC-SIGN-TLR2 crosstalk. PLoS Pathog. 10:e1004647. doi: 10.1371/journal.ppat.1004647
Fang, L., Wu, H. M., Ding, P. S., and Liu, R. Y. (2014). TLR2 mediates phagocytosis and autophagy through JNK signaling pathway in Staphylococcus aureus-stimulated RAW264.7 cells. Cell. Signal. 26, 806–814. doi: 10.1016/j.cellsig.2013.12.016
Giraldo, E., Martin-Cordero, L., Garcia, J. J., Gehrmann, M., Multhoff, G., and Ortega, E. (2010). Exercise-induced extracellular 72 kDa heat shock protein (Hsp72) stimulates neutrophil phagocytic and fungicidal capacities via TLR-2. Eur. J. Appl. Physiol. 108, 217–225. doi: 10.1007/s00421-009-1201-8
Gomes, M. S., Sousa Fernandes, S., Cordeiro, J. V., Silva Gomes, S., Vieira, A., and Appelberg, R. (2008). Engagement of Toll-like receptor 2 in mouse macrophages infected with Mycobacterium avium induces non-oxidative and TNF-independent anti-mycobacterial activity. Eur. J. Immunol. 38, 2180–2189. doi: 10.1002/eji.200737954
Hajishengallis, G. (2014). Immunomicrobial pathogenesis of periodontitis: keystones, pathobionts, and host response. Trends Immunol. 35, 3–11. doi: 10.1016/j.it.2013.09.001
Hajishengallis, G., Darveau, R. P., and Curtis, M. A. (2012). The keystone-pathogen hypothesis. Nat. Rev. Microbiol. 10, 717–725. doi: 10.1038/nrmicro2873
Hajishengallis, G., Krauss, J. L., Jotwani, R., and Lambris, J. D. (2017). Differential capacity for complement receptor-mediated immune evasion by Porphyromonas gingivalis depending on the type of innate leukocyte. Mol. Oral Microbiol. 32, 154–165. doi: 10.1111/omi.12161
Hajishengallis, G., Liang, S., Payne, M. A., Hashim, A., Jotwani, R., Eskan, M. A., et al. (2011). Low-abundance biofilm species orchestrates inflammatory periodontal disease through the commensal microbiota and complement. Cell Host Microbe 10, 497–506. doi: 10.1016/j.chom.2011.10.006
Hajishengallis, G., Shakhatreh, M. A., Wang, M., and Liang, S. (2007). Complement receptor 3 blockade promotes IL-12-mediated clearance of Porphyromonas gingivalis and negates its virulence in vivo. J. Immunol. 179, 2359–2367. doi: 10.4049/jimmunol.179.4.2359
Hajishengallis, G., Wang, M., Liang, S., Triantafilou, M., and Triantafilou, K. (2008). Pathogen induction of CXCR4/TLR2 cross-talk impairs host defense function. Proc. Natl. Acad. Sci. U.S.A. 105, 13532–13537. doi: 10.1073/pnas.0803852105
Harokopakis, E., Albzreh, M. H., Martin, M. H., and Hajishengallis, G. (2006). TLR2 transmodulates monocyte adhesion and transmigration via Rac1- and PI3K-mediated inside-out signaling in response to Porphyromonas gingivalis fimbriae. J. Immunol. 176, 7645–7656. doi: 10.4049/jimmunol.176.12.7645
Holt, S. C., and Bramanti, T. E. (1991). Factors in virulence expression and their role in periodontal disease pathogenesis. Crit. Rev. Oral Biol. Med. 2, 177–281. doi: 10.1177/10454411910020020301
Kassem, A., Henning, P., Kindlund, B., Lindholm, C., and Lerner, U. H. (2015a). TLR5, a novel mediator of innate immunity-induced osteoclastogenesis and bone loss. FASEB J. 29, 4449–4460. doi: 10.1096/fj.15-272559
Kassem, A., Henning, P., Lundberg, P., Souza, P. P., Lindholm, C., and Lerner, U. H. (2015b). Porphyromonas gingivalis stimulates bone resorption by enhancing RANKL (Receptor Activator of NF-κB Ligand) through activation of toll-like receptor 2 in Osteoblasts. J. Biol. Chem. 290, 20147–20158. doi: 10.1074/jbc.M115.655787
Kfoury, A., Virard, F., Renno, T., and Coste, I. (2014). Dual function of MyD88 in inflammation and oncogenesis: implications for therapeutic intervention. Curr. Opin. Oncol. 26, 86–91. doi: 10.1097/CCO.0000000000000037
Kim, J., Yang, J., Park, O. J., Kang, S. S., Kim, W. S., Kurokawa, K., et al. (2013). Lipoproteins are an important bacterial component responsible for bone destruction through the induction of osteoclast differentiation and activation. J. Bone Miner. Res. 28, 2381–2391. doi: 10.1002/jbmr.1973
Kim, P. D., Xia-Juan, X., Crump, K. E., Abe, T., Hajishengallis, G., and Sahingur, S. E. (2015). Toll-like receptor 9-mediated inflammation triggers alveolar bone loss in experimental murine periodontitis. Infect. Immun. 83, 2992–3002. doi: 10.1128/IAI.00424-15
Lam, R. S., O'brien-Simpson, N. M., Lenzo, J. C., Holden, J. A., Brammar, G. C., Walsh, K. A., et al. (2014). Macrophage depletion abates Porphyromonas gingivalis-induced alveolar bone resorption in mice. J. Immunol. 193, 2349–2362. doi: 10.4049/jimmunol.1400853
Lorne, E., Zhao, X., Zmijewski, J. W., Liu, G., Park, Y. J., Tsuruta, Y., et al. (2009). Participation of mammalian target of rapamycin complex 1 in toll-like receptor 2- and 4-induced neutrophil activation and acute lung injury. Am. J. Respir. Cell Mol. Biol. 41, 237–245. doi: 10.1165/rcmb.2008-0290OC
Madeira, M. F., Queiroz-Junior, C. M., Cisalpino, D., Werneck, S. M., Kikuchi, H., Fujise, O., et al. (2013). MyD88 is essential for alveolar bone loss induced by Aggregatibacter actinomycetemcomitans lipopolysaccharide in mice. Mol. Oral Microbiol. 28, 415–424. doi: 10.1111/omi.12034
Maekawa, T., Krauss, J. L., Abe, T., Jotwani, R., Triantafilou, M., Triantafilou, K., et al. (2014). Porphyromonas gingivalis manipulates complement and TLR signaling to uncouple bacterial clearance from inflammation and promote dysbiosis. Cell Host Microbe 15, 768–778. doi: 10.1016/j.chom.2014.05.012
Mizraji, G., Nassar, M., Segev, H., Sharawi, H., Eli-Berchoer, L., Capucha, T., et al. (2017). Porphyromonas gingivalis promotes unrestrained type I interferon production by dysregulating TAM signaling via MYD88 degradation. Cell Rep. 18, 419–431. doi: 10.1016/j.celrep.2016.12.047
Nussbaum, G., and Shapira, L. (2011). How has neutrophil research improved our understanding of periodontal pathogenesis? J. Clin. Periodontol. 38, 49–59. doi: 10.1111/j.1600-051X.2010.01678.x
Pandey, S., Kawai, T., and Akira, S. (2014). Microbial sensing by Toll-like receptors and intracellular nucleic acid sensors. Cold Spring Harb. Perspect. Biol. 7:a016246. doi: 10.1101/cshperspect.a016246
Papadopoulos, G., Weinberg, E. O., Massari, P., Gibson, F. C. III, Wetzler, L. M., Morgan, E. F., et al. (2013). Macrophage-specific TLR2 signaling mediates pathogen-induced TNF-dependent inflammatory oral bone loss. J. Immunol. 190, 1148–1157. doi: 10.4049/jimmunol.1202511
Pihlstrom, B. L., Michalowicz, B. S., and Johnson, N. W. (2005). Periodontal diseases. Lancet 366, 1809–1820. doi: 10.1016/S0140-6736(05)67728-8
Ryder, M. I. (2010). Comparison of neutrophil functions in aggressive and chronic periodontitis. Periodontol. 2000 53, 124–137. doi: 10.1111/j.1600-0757.2009.00327.x
Santos-Sierra, S., Deshmukh, S. D., Kalnitski, J., Kuenzi, P., Wymann, M. P., Golenbock, D. T., et al. (2009). Mal connects TLR2 to PI3Kinase activation and phagocyte polarization. EMBO J. 28, 2018–2027. doi: 10.1038/emboj.2009.158
Sato, N., Takahashi, N., Suda, K., Nakamura, M., Yamaki, M., Ninomiya, T., et al. (2004). MyD88 but not TRIF is essential for osteoclastogenesis induced by lipopolysaccharide, diacyl lipopeptide, and IL-1α. J. Exp. Med. 200, 601–611. doi: 10.1084/jem.20040689
Shin, O. S., Miller, L. S., Modlin, R. L., Akira, S., Uematsu, S., and Hu, L. T. (2009). Downstream signals for MyD88-mediated phagocytosis of Borrelia burgdorferi can be initiated by TRIF and are dependent on PI3K. J. Immunol. 183, 491–498. doi: 10.4049/jimmunol.0900724
Socransky, S. S., and Haffajee, A. D. (1992). The bacterial etiology of destructive periodontal disease: current concepts. J. Periodontol. 63, 322–331. doi: 10.1902/jop.1992.63.4s.322
Steinmetz, O., Hoch, S., Avniel-Polak, S., Gavish, K., Eli-Berchoer, L., Wilensky, A., et al. (2016). CX3CR1hi monocyte/macrophages support bacterial survival and experimental infection-driven bone resorption. J. Infect. Dis. 213, 1505–1515. doi: 10.1093/infdis/jiv763
Strassheim, D., Asehnoune, K., Park, J. S., Kim, J. Y., He, Q., Richter, D., et al. (2004). Phosphoinositide 3-kinase and Akt occupy central roles in inflammatory responses of Toll-like receptor 2-stimulated neutrophils. J. Immunol. 172, 5727–5733. doi: 10.4049/jimmunol.172.9.5727
Takeuchi, H., Furuta, N., Morisaki, I., and Amano, A. (2011). Exit of intracellular Porphyromonas gingivalis from gingival epithelial cells is mediated by endocytic recycling pathway. Cell. Microbiol. 13, 677–691. doi: 10.1111/j.1462-5822.2010.01564.x
Thi, E. P., and Reiner, N. E. (2012). Phosphatidylinositol 3-kinases and their roles in phagosome maturation. J. Leukoc. Biol. 92, 553–566. doi: 10.1189/jlb.0212053
Wang, M., and Hajishengallis, G. (2008). Lipid raft-dependent uptake, signalling and intracellular fate of Porphyromonas gingivalis in mouse macrophages. Cell. Microbiol. 10, 2029–2042. doi: 10.1111/j.1462-5822.2008.01185.x
Weiss, D. J., Souza, C. D., Evanson, O. A., Sanders, M., and Rutherford, M. (2008). Bovine monocyte TLR2 receptors differentially regulate the intracellular fate of Mycobacterium avium subsp. paratuberculosis and Mycobacterium avium subsp. avium. J. Leukoc. Biol. 83, 48–55. doi: 10.1189/jlb.0707490
Wilensky, A., Gabet, Y., Yumoto, H., Houri-Haddad, Y., and Shapira, L. (2005). Three-dimensional quantification of alveolar bone loss in Porphyromonas gingivalis-infected mice using micro-computed tomography. J Periodontol. 76, 1282–1286. doi: 10.1902/jop.2005.76.8.1282
Yamatake, K., Maeda, M., Kadowaki, T., Takii, R., Tsukuba, T., Ueno, T., et al. (2007). Role for gingipains in Porphyromonas gingivalis traffic to phagolysosomes and survival in human aortic endothelial cells. Infect. Immun. 75, 2090–2100. doi: 10.1128/IAI.01013-06
Yates, R. M., and Russell, D. G. (2005). Phagosome maturation proceeds independently of stimulation of toll-like receptors 2 and 4. Immunity 23, 409–417. doi: 10.1016/j.immuni.2005.09.007
Yilmaz, O., Jungas, T., Verbeke, P., and Ojcius, D. M. (2004). Activation of the phosphatidylinositol 3-kinase/Akt pathway contributes to survival of primary epithelial cells infected with the periodontal pathogen Porphyromonas gingivalis. Infect. Immun. 72, 3743–3751. doi: 10.1128/IAI.72.7.3743-3751.2004
Keywords: P. gingivalis, immune evasion, TLR2 signaling, MyD88, PI3 kinase, neutrophils, macrophages
Citation: Makkawi H, Hoch S, Burns E, Hosur K, Hajishengallis G, Kirschning CJ and Nussbaum G (2017) Porphyromonas gingivalis Stimulates TLR2-PI3K Signaling to Escape Immune Clearance and Induce Bone Resorption Independently of MyD88. Front. Cell. Infect. Microbiol. 7:359. doi: 10.3389/fcimb.2017.00359
Received: 28 March 2017; Accepted: 25 July 2017;
Published: 08 August 2017.
Edited by:
Silvia Mercedes Uriarte, University of Louisville, United StatesReviewed by:
Jan Potempa, University of Louisville, United StatesCopyright © 2017 Makkawi, Hoch, Burns, Hosur, Hajishengallis, Kirschning and Nussbaum. This is an open-access article distributed under the terms of the Creative Commons Attribution License (CC BY). The use, distribution or reproduction in other forums is permitted, provided the original author(s) or licensor are credited and that the original publication in this journal is cited, in accordance with accepted academic practice. No use, distribution or reproduction is permitted which does not comply with these terms.
*Correspondence: Gabriel Nussbaum, Z2FicmllbG5AZWttZC5odWppLmFjLmls
Disclaimer: All claims expressed in this article are solely those of the authors and do not necessarily represent those of their affiliated organizations, or those of the publisher, the editors and the reviewers. Any product that may be evaluated in this article or claim that may be made by its manufacturer is not guaranteed or endorsed by the publisher.
Research integrity at Frontiers
Learn more about the work of our research integrity team to safeguard the quality of each article we publish.