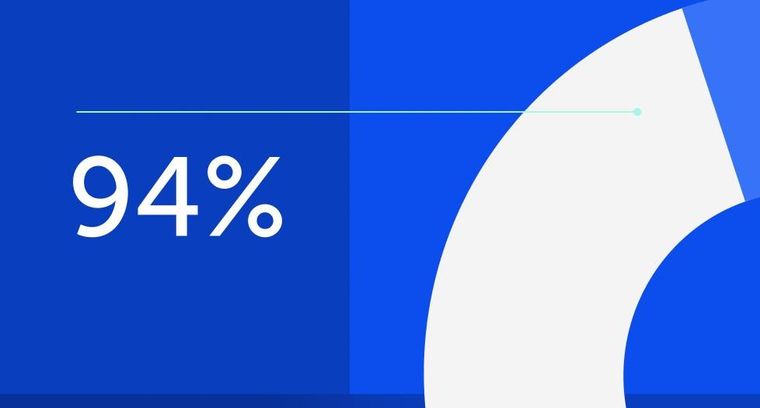
94% of researchers rate our articles as excellent or good
Learn more about the work of our research integrity team to safeguard the quality of each article we publish.
Find out more
OPINION article
Front. Cell. Infect. Microbiol., 19 November 2013
Sec. Microbiome in Health and Disease
Volume 3 - 2013 | https://doi.org/10.3389/fcimb.2013.00082
This article is part of the Research TopicModel organisms in inflammation and cancerView all 11 articles
Following the acquisition of multicellularity, organisms with increasing levels of specialized cells, tissues, and organs emerged during evolution. To coordinate specialized organs, long-distance interorgan communication systems appeared. The central nervous system evolved to regulate many organ behaviors, using hormones or neurons. In addition, organs developed systems to directly communicate their states to one another. This is illustrated by the lack of nervous systems in plants and simple animals like sponges, which can perform complex systemic functions (Lough and Lucas, 2006; Srivastava et al., 2010).
Developmental or homeostatic events within cells or tissues have been extensively studied. For example, maintenance of the integrity of the Drosophila gut involves stem cell proliferation and differentiation, partially driven by local JAK/STAT, EGF, MAPK, and Wnt signaling (Panayidou and Apidianakis, 2013). Recently, it has become clear that individual organs themselves are also able to communicate their states. However, the nature of the interorgan signaling mechanisms remains largely a mystery.
Here, we review the emerging data supporting the existence of a vast interorgan communication network (ICN). The ICN is the network of peptides, proteins, and metabolites that act between organs to coordinate essential and specialized cellular processes under homeostasis and stress (Figure 1). We propose that studies in Drosophila, where, unlike in mammals, biochemical studies can be combined with genome-wide in vivo tissue-specific genetic screens, are poised to identify many ICN components. Characterization of the ICN will further understanding of systemic diseases such as cancer-associated muscle cachexia.
Figure 1. Overview of the interorgan communication network (ICN). The ICN is the network of peptides, proteins, and metabolites that act between organs to coordinate organismal cellular processes under homeostasis and stress. Organs in the body secrete factors that act to influence the physiology of cells in distal organs. Processes that may be connected between organs include aging, protein homeostasis, nutrient uptake, metabolism, cell division, cell movement, detoxification, organelle biogenesis, and secretion of local and systemic signals. The signals may be nutrients, wastes, toxins, metabolites, nucleic acids, proteins, and peptides.
A limited number of studies in mammals, C. elegans, and Drosophila showed that perturbed tissues affect organismal growth and metabolism via largely unknown signals. The Drosophila fat-body (liver and adipose functional equivalent) responds to dietary signals by releasing factors affecting insulin secretion, growth, and metabolism (Britton and Edgar, 1998; Colombani et al., 2003; Géminard et al., 2009). For instance, in response to high dietary fat and sugar, the fat-body-derived leptin-like factor Unpaired-2 systemically controls release of insulin from insulin-producing cells in the brain (Rajan and Perrimon, 2012). Further, unknown nutrition-dependent signals control intestinal, neural, and germline stem cell division through local or systemic insulin signaling (LaFever and Drummond-Barbosa, 2005; Chell and Brand, 2010; O'Brien et al., 2011; Sousa-Nunes et al., 2011). Also, localized organ growth perturbations delay systemic development via inhibition of insulin signaling (DiAngelo et al., 2009), and insulin (Karpac et al., 2011) and ecdysteroid synthesis, partially through insulin-like Dilp8 (Colombani et al., 2012; Garelli et al., 2012).
In mammals, leptin is secreted by adipose tissue with nutritional surplus, controlling the neuroendocrine system (Zhang et al., 1994; Ahima et al., 1996). Also, exercise and muscle overexpression of PGC1-α increases the production of the secreted factor Irisin, a fragment of the transmembrane protein FNDC5, which stimulates metabolism and fat browning (Böstrom et al., 2012). Moreover, exercising muscle secretes interleukin-6 (Steensberg et al., 2000), possibly regulating systemic glucose and lipid metabolism by acting on muscle, liver, fat, intestinal L-cells, and pancreatic alpha-cells (Febbraio et al., 2004; Petersen et al., 2005; Ellingsgaard et al., 2011; Pedersen, 2011; Pedersen and Febbraio, 2012). Interestingly, liver or muscle autophagy controls whole-body glucose and fatty-acid metabolism, partially through FGF-21 (Kim et al., 2013). Finally, a number of gut-derived hormones including gastrin, ghrelin, cholecystokinin, glucagon-like peptide-1, and others affect insulin secretion, systemic fatty-acid metabolism, and feeding (Drucker, 2007). Strikingly, metabolic control is conserved, as leptin can rescue Drosophila Unpaired-2 deficiency, and both function through similar neuronal circuits (Vong et al., 2011; Rajan and Perrimon, 2012).
Intracellular pathways induce factors which regulate aging, stress resistance, and distal cellular functions. In C. elegans, germ-line absence extends life-span (Arantes-Oliveira et al., 2002) and causes systemic proteasomal activity increase, via unknown signals (Vilchez et al., 2012). In addition, tissue-specific induction of mitochondrial (Durieux et al., 2011), cytoplasmic (van Oosten-Hawle et al., 2013), and endoplasmic reticulum (ER; Taylor and Dillin, 2013) unfolded protein responses result in their systemic propagation, via poorly characterized factors. Neurotransmitter signaling partially mediates ER stress (Taylor and Dillin, 2013), but not heat-shock response propagation (van Oosten-Hawle et al., 2013). Moreover, systemic signaling to the brain causes behavioral avoidance of the stress-inducer (Melo and Ruvkun, 2012).
In Drosophila, gut, muscles, and fat-body are essential in stress resistance and aging. Gut infection or oxidative stress induces fat-body anti-microbial peptide secretion via unknown mechanisms (Foley and O'Farrell, 2003; Wu et al., 2012). Fat-body overexpression of FOXO transcription factor increases lifespan (Giannakou et al., 2004). Moreover, adult muscle-specific overexpression of FOXO prevents aging of other organs by decreasing accumulation of protein aggregates and increasing autophagy (Demontis and Perrimon, 2010). In addition, activation of muscle TOR or p38-MAPK signaling controls systemic aging and stress resistance (Vrailas-Mortimer et al., 2011). Also, muscle fatty-acid metabolism is essential for lifespan-increasing effects of dietary restriction (Katewa et al., 2012). Moreover, maintenance of gut homeostasis by stem-cell expression of PGC-1 or FOXO targets improves lifespan and metabolic homeostasis (Biteau et al., 2010; Rera et al., 2011).
Also, exposure of old mice to young blood results in restoration of muscle and liver regeneration, suggesting that systemic factors control aging (Conboy et al., 2005). For example, GDF-11 is a BMP ligand which slows myocardial aging through unknown mechanisms (Loffredo et al., 2013). Interestingly, TGF-β has been implicated in regulating reactive oxygen species production in the aorta, endothelial structure, blood-pressure, and cardiomyocyte function (Buday et al., 2010).
Systemic factors also control cell proliferation and tissue regeneration. In Drosophila, distal wounds control gut proliferative homeostasis via unknown mechanisms (Takeishi et al., 2013). Moreover, insulin regulates intestinal stem-cell proliferation (Amcheslavsky et al., 2009; Choi et al., 2011). In mammals, muscle from dystrophin-mutant mice may remotely alter wound healing (Straino et al., 2004). Also, liver-secreted betatrophin controls pancreatic beta-cell proliferation (Yi et al., 2013).
Unknown factors may also be controlled by reproduction. In insects, mating and fertilization induces numerous uncharacterized transcriptional changes in multiple organs (Rogers et al., 2008; Avila et al., 2011). In Drosophila females, mating increases mating receptivity, feeding, and egg-laying; changes movement; and decreases lifespan (Fowler and Partridge, 1988; Barnes et al., 2008; Avila et al., 2011). Some changes are associated with transfer of male accessory gland peptides (e.g., sex peptide) to females (Wigby and Chapman, 2005; Carvalho et al., 2006). Conversely, systemic factors may control reproduction. For instance, in Drosophila, insulin controls female germline stem cell proliferation (LaFever and Drummond-Barbosa, 2005). In C. elegans, oocyte and germline maintenance during aging is regulated by TGF-β and insulin via unknown relay signals (Luo et al., 2010).
In addition, systemic factors may regulate offspring fitness. In mice, paternal diet influences offspring metabolism (Carone et al., 2010; Ng et al., 2010). Moreover, the injury of fathers' and grandfathers' livers increases the regenerative capacity of their offspring's livers (Zeybel et al., 2012). Similarly, in Drosophila, tissue-specific stress causes heritable developmental alterations (Stern et al., 2012).
Finally, because alterations in its composition influence systemic physiology (e.g., metabolism; Claus et al., 2008), the microbiome is part of the ICN. For instance, obesity-induced changes in gut microbiome increase systemic deoxycholic acid that acts as a liver DNA-damaging and cancer-promoting agent (Yoshimoto et al., 2013).
In conclusion, there is growing evidence that many organismal functions mediate various aspects of interorgan communication through secreted factors. Understanding the roles of these factors, and how their activities are integrated to the organism's functions is the next big challenge. Further, as systematic screens have not been performed for such factors, it is likely that many additional ones remain to be identified.
Gene-expression analyses of organs have shown the existence of organ-to-organ coexpression networks that change in disease and aging, suggesting of unexplored interorgan processes and common responses of tissues to systemic factors (Keller et al., 2008; Dobrin et al., 2009; Huang et al., 2011). These analyses revealed that at least 40% of the interorgan features are not in single-tissue networks, and that the highly connected genes in the interorgan networks are poorly connected in the single-tissue networks (Dobrin et al., 2009).
What are the factors/nodes that connect the organs/hubs in the ICN? At their simplest and most evolutionary ancient form, signals may be nutrients, wastes, toxins, or metabolites. For instance, liver-produced beta-hydroxybutyrate inhibits histone deacetylases (Shimazu et al., 2013). Communication may also be in the form of circulating nucleic acids (e.g., miRNAs; Mitchell et al., 2008). Finally, proteins and peptides may be classical developmental regulators or novel. Intriguingly, “intracellular” proteins can be secreted outside the cell, as an isoform containing a signal sequence (e.g., PTEN-long; Hopkins et al., 2013), or through non-classical secretion (e.g., aP2; Cao et al., 2013)
An important feature that differentiates local tissue and developmental networks from the ICN, is the large distance over which signaling acts, meaning that concentration and specificity of the factors could be lower. To remedy this, a dense network of closely acting factors could exist, such that one factor acts on a neighboring tissue, which secretes a relay signal. Alternatively, signals may be carried along “molecular tracks” to their destination. These may be blood vessels or tissue regions containing “guidance factors”—putative weak affinity receptors to common structural features to groups of secreted factors. In addition, binding proteins (Mantovani et al., 2001) or proteases may be secreted to modulate local or systemic signaling. For example, Drosophila insulin-binding proteins ImpL2 (Honegger et al., 2008) or secreted decoy of insulin (Okamoto et al., 2013) bind to and inhibit insulin, locally or systemically. The mammalian ImpL2 homologs, insulin-like growth factor (IGF) binding proteins transport and regulate IGFs (Hwa et al., 1999; Honegger et al., 2008).
Factors may also be modified with fatty-acids, cholesterol, or glycans, regulating their stability, transport (Nusse, 2003; Linder and Deschenes, 2007; Moremen et al., 2012), and interaction with abundant and stable components including apolipoproteins (Panáková et al., 2005). These molecules can then deliver factors to target organs. For example, Hedgehog can be lipidated, interact with apoliproteins, and act distally (Palm et al., 2013). Finally, signaling can occur extracellularly through protease cascades (e.g., Drosophila spatzle-Toll; Morisato and Anderson, 1994) or phosphorylation (Yalak and Vogel, 2012).
Elucidation of the ICN will be valuable for disease biology. Many disorders begin locally, and ultimately involve the entire organism by affecting behavior, cell recruitment, metabolism, proliferation, and activation (McCance and Huether, 2002). For example, muscle defects are associated with alterations in wound healing (Straino et al., 2004), regeneration, hepatocyte proliferation (Conboy et al., 2005), dyslipidemia, hypertension, type 2 diabetes, cardiovascular diseases, cancer, Alzheimer's and Parkinson's diseases (Pedersen, 2011). Moreover, cachexia, wound-healing, and hematopoiesis defects occur in cancer (Devereux et al., 1979; Egeblad et al., 2010).
Also, organ failure patients who receive organ function replacement therapy eventually succumb to disease, with systemic defects. For instance, kidney failure patients receiving kidney function replacement hemodialysis suffer from malnutrition and lung defects (McCance and Huether, 2002; Doi et al., 2011; White et al., 2011). This suggests that organs have essential functions beyond their “classic” roles, for example, by regulating distal organs through secreted factors. Importantly, blood-borne signals mediate critical systemic homeostatic adjustments from local perturbations, illustrated by control of systemic physiology by electrical cycling of paralyzed muscles in spinal-cord injured tetraplegic humans (Kjaer et al., 1996; Pedersen, 2011).
Great strides are being made toward understanding intracellular and tissue homeostasis. The next step is to understand the structure, function, and components of the ICN. The main questions are the nature of the interorgan communication factors and their roles in maintaining whole-organism homeostasis. Also, how does the ICN change during development, aging, and disease? The current transcriptomic, proteomic, metabolomic, and genome-wide tissue-specific genetic manipulation technologies will allow answering these questions. Importantly, systematic in vivo identification of systemic factors is impractical in mammals. Thus, the ICN may be constructed for Drosophila, for which all of the above tools are available, and applied to mammals. Thus, “organ-sensing” RNAi screens can now be done, where genes are inactivated by tissue-specific RNAi, and function of another organ is assessed. Within the next decade, we expect a surge of interest to define the structure and function of the ICN.
Ilia A. Droujinine is in part supported by NSERC PGS-D. We thank Akhila Rajan and Edward Owusu-Ansah for their insightful comments on the manuscript.
Ahima, R. S., Prabakaran, D., Mantzoros, C., Qu, D., Lowell, B., Maratos-Flier, E., et al. (1996). Role of leptin in the neuroendocrine response to fasting. Nature 382, 250–252. doi: 10.1038/382250a0
Amcheslavsky, A., Jiang, J., and Ip, Y. T. (2009). Tissue damage-induced intestinal stem cell division in Drosophila. Cell Stem Cell 4, 49–61. doi: 10.1016/j.stem.2008.10.016
Arantes-Oliveira, N., Apfeld, J., Dillin, A., and Kenyon, C. (2002). Regulation of life-span by germ-line stem cells in Caenorhabditis elegans. Science 295, 502–505. doi: 10.1126/science.1065768
Avila, F. W., Sirot, L. K., LaFlamme, B. A., Rubinstein, C. D., and Wolfner, M. F. (2011). Insect seminal fluid proteins: identification and function. Annu. Rev. Entomol. 56, 21–40. doi: 10.1146/annurev-ento-120709-144823
Barnes, A. I., Wigby, S., Boone, J. M., Partridge., L., and Chapman, T. (2008). Feeding, fecundity and lifespan in female Drosophila melanogaster. Proc. Biol. Sci. 275, 1675–1683. doi: 10.1098/rspb.2008.0139
Biteau, B., Karpac, J., Supoyo, S., DeGennaro, M., Lehmann, R., and Jasper, H. (2010). Lifespan extension by preserving proliferative homeostasis in Drosophila. PLoS Genet. 6:e1001159. doi:10.1371/journal.pgen.1001159
Böstrom, P., Wu, J., Jedrychowski, M. P., Korde, A., Ye, L., Lo, J. C., et al. (2012). A PGC1-α-dependent myokine that drives brown-fat-like development of white fat and thermogenesis. Nature 481, 463–468. doi: 10.1038/nature10777
Britton, J. S., and Edgar, B. A. (1998). Environmental control of the cell cycle in Drosophila: nutrition activates mitotic and endoreplicative cells by distinct mechanisms. Development 125, 2149–2158.
Buday, A., Orsy, P., Godó, M., Mózes, M., Kökény, G., Lacza, Z., et al. (2010). Elevated systemic TGF-beta impairs aortic vasomotor function through activation of NADPH oxidase-driven superoxide production and leads to hypertension, myocardial remodeling, and increased plaque formation in apoE(-/-) mice. Am. J. Physiol. Heart Circ. Physiol. 299, H386–H389. doi: 10.1152/ajpheart.01042.2009
Cao, H., Sekiya, M., Ertunc, M. E., Burak, M. F., Mayers, J. R., White, A., et al. (2013). Adipocyte lipid chaperone AP2 is a secreted adipokine regulating hepatic glucose production. Cell Metab. 17, 768–778. doi: 10.1016/j.cmet.2013.04.012
Carone, B. R., Fauquier, L., Habib, N., Shea, J. M., Hart, C. E., Li, R., et al. (2010). Paternally induced transgenerational environmental reprogramming of metabolic gene expression in mammals. Cell 143, 1084–1096. doi: 10.1016/j.cell.2010.12.008
Carvalho, G. B., Kapahi, P., Anderson, D. J., and Benzer, S. (2006). Allocrine modulation of feeding behavior by the sex peptide of Drosophila. Curr. Biol. 16, 692–696. doi: 10.1016/j.cub.2006.02.064
Chell, J. M., and Brand, A. H. (2010). Nutrition-responsive glia control exit of neural stem cells from quiescence. Cell 143, 1161–1173. doi: 10.1016/j.cell.2010.12.007
Choi, N. H., Lucchetta, E., and Ohlstein, B. (2011). Nonautonomous regulation of Drosophila midgut stem cell proliferation by the insulin-signaling pathway. Proc. Natl. Acad. Sci. U.S.A. 108, 18702–18707. doi: 10.1073/pnas.1109348108
Claus, S. P., Tsang, T. M., Wang, Y., Cloarec, O., Skordi, E., Martin, F. -P., et al. (2008). Systemic multicompartmental effects of the gut microbiome on mouse metabolic phenotypes. Mol. Syst. Biol. 4, 219. doi: 10.1038/msb.2008.56
Colombani, J., Andersen, D. S., and Léopold, P. (2012). Secreted peptide Dilp8 coordinates Drosophila tissue growth with developmental timing. Science 336, 582–585. doi: 10.1126/science.1216689
Colombani, J., Raisin, S., Pantalacci, S., Radimerski, T., Montagne, J., and Léopold, P. (2003). A nutrient sensor mechanism controls Drosophila growth. Cell 114, 739–749. doi: 10.1016/S0092-8674(03)00713-X
Conboy, I. M., Conboy, M. J., Wagers, A. J., Girma, E. R., Weissman, I. L., and Rando, T. A. (2005). Rejuvenation of aged progenitor cells by exposure to a young systemic environment. Nature 433, 760–764. doi: 10.1038/nature03260
Demontis, F., and Perrimon, N. (2010). FOXO/4E-BP signaling in Drosophila muscles regulates organism-wide proteostasis during aging. Cell 143, 813–825. doi: 10.1016/j.cell.2010.10.007
Devereux, D. F., Thistlethwaite, P. A., Thibault, L. E., and Brennan, M. F. (1979). Effects of tumor bearing and protein depletion on wound breaking strength in the rat. J. Surg. Res. 27, 233–238. doi: 10.1016/0022-4804(79)90135-5
DiAngelo, J. R., Bland, M. L., Bambina, S., Cherry, S., and Birnbaum, M. J. (2009). The immune response attenuates growth and nutrient storage in Drosophila by reducing insulin signaling. Proc. Natl. Acad. Sci. U.S.A. 106, 20853–20858. doi: 10.1073/pnas.0906749106
Dobrin, R., Zhu, J., Molony, C., Argman, C., Parrish, M. L., Carlson, S., et al. (2009). Multi-tissue coexpression networks reveal unexpected subnetworks associated with disease. Genome Biol. 10, R55. doi: 10.1186/gb-2009–2010-5-r55
Doi, K., Ishizu, T., Fujita, T., and Noiri, E. (2011). Lung injury following acute kidney injury: kidney-lung crosstalk. Clin. Exp. Nephrol. 15, 464–470. doi: 10.1007/s10157-011-0459-4
Drucker, D. J. (2007). The role of gut hormones in glucose homeostasis. J. Clin. Invest. 117, 24–32. doi: 10.1172/JCI30076
Durieux, J., Wolff, S., and Dillin, A. (2011). The cell-non-autonomous nature of electron transport chain-mediated longevity. Cell 144, 79–91. doi: 10.1016/j.cell.2010.12.016
Egeblad, M., Nakasone, E. S., and Werb, Z. (2010). Tumors as organs: complex tissues that interface with the entire organism. Dev. Cell 18, 884–901. doi: 10.1016/j.devcel.2010.05.012
Ellingsgaard, H., Hauselmann, I., Schuler, B., Habib, A. M., Baggio, L. L., Meier, D. T., et al. (2011). Interleukin-6 enhances insulin secretion by increasing glucagon-like peptide-1 secretion from L cells and alpha cells. Nat. Med. 17, 1481–1489. doi: 10.1038/nm.2513
Febbraio, M. A., Hiscock, N., Sacchetti, M., Fischer, C. P., and Pedersen, B. K. (2004). Interleukin-6 is a novel factor mediating glucose homeostasis during skeletal muscle contraction. Diabetes 53, 1643–1648. doi: 10.2337/diabetes.53.7.1643
Foley, E., and O'Farrell, P. H. (2003). Nitric oxide contributes to induction of innate immune responses to gram-negative bacteria in Drosophila. Genes Dev. 17, 115–125. doi: 10.1101/gad.1018503
Fowler, K., and Partridge, L. (1988). A cost of mating in female fruitflies. Nature 338, 760–761. doi: 10.1038/338760a0
Garelli, A., Gontijo, A. M., Miguela, V., Caparros, E., and Dominguez, M. (2012). Imaginal discs secrete insulin-like peptide 8 to mediate plasticity of growth and metabolism. Science 336, 579–582. doi: 10.1126/science.1216735
Géminard, C., Rulifson, E. J., and Léopold, P. (2009). Remote control of insulin secretion by fat cells in Drosophila. Cell Metab. 10, 199–207. doi: 10.1016/j.cmet.2009.08.002
Giannakou, M. E., Goss, M., Jünger, M. A., Hafen, E., Leevers, S. J., and Partridge, L. (2004). Long-lived Drosophila with overexpressed dFOXO in adult fat body. Science 305, 361. doi: 10.1126/science.1098219
Honegger, B., Galic, M., Köhler, K., Wittwer, F., Brogiolo, W., Hafen, E., et al. (2008). Imp-L2, a putative homolog of vertebrate IGF-binding protein 7, counteracts insulin signaling in Drosophila and is essential for starvation resistance. J. Biol. 7, 10. doi: 10.1186/jbiol72
Hopkins, B. D., Fine, B., Steinbach, N., Dendy, M., Rapp, Z., Shaw, J., et al. (2013). A secreted PTEN phosphatase that enters cells to alter signaling and survival. Science 341, 399–402. doi: 10.1126/science.1234907
Huang, T., Zhang, J., Xie, L., Dong, X., Zhang, L., Cai, Y. D., et al. (2011). Crosstissue coexpression network of aging. OMICS 15, 665–671. doi: 10.1089/omi.2011.0034
Hwa, V., Oh, Y., and Rosenfeld, R. G. (1999). The insulin-like growth factor-binding protein (IGFBP) superfamily. Endocr. Rev. 20, 761–787. doi: 10.1210/er.20.6.761
Karpac, J., Younger, A., and Jasper, H. (2011). Dynamic coordination of innate immune signaling and insulin signaling regulates systemic responses to localized DNA damage. Dev. Cell 20, 841–854. doi: 10.1016/j.devcel.2011.05.011
Katewa, S. D., Demontis, F., Kolipinski, M., Hubbard, A., Gill, M. S., Perrimon, N., et al. (2012). Intramyocellular fatty-acid metabolism plays a critical role in mediating responses to dietary restriction in Drosophila melanogaster. Cell Metab. 16, 97–103. doi: 10.1016/j.cmet.2012.06.005
Keller, M. P., Choi, Y. J., Wang, P., Davis, D. B., Rabaglia, M. E., Oler, A. T., et al. (2008). A gene expression network model of type 2 diabetes links cell cycle regulation in islets with diabetes susceptibility. Genome Res. 18, 706–716. doi: 10.1101/gr.074914.107
Kim, K. H., Jeong, Y. T., Oh, H., Kim, S. H., Cho, J. M., Kim, Y. N., et al. (2013). Autophagy deficiency leads to protection from obesity and insulin resistance by inducing Fgf21 as a mitokine. Nat. Med. 19, 83–92. doi: 10.1038/nm.3014
Kjaer, M., Pollack, S. F., Mohr, T., Weiss, H., Gleim, G. M., Bach, F. W., et al. (1996). Regulation of glucose turnover and hormonal responses during electrical cycling in tetraplegic humans. Am. J. Physiol. Regul. Integr. Comp. Physiol. 271, R191–R199.
LaFever, L., and Drummond-Barbosa, D. (2005). Direct control of germline stem cell division and cyst growth by neural insulin in Drosophila. Science 309, 1071–1073. doi: 10.1126/science.1111410
Linder, M. E., and Deschenes, R. J. (2007). Palmitoylation: policing protein stability and traffic. Nat. Rev. Mol. Cell Biol. 8, 74–84. doi: 10.1038/nrm2084
Loffredo, F. S., Steinhauser, M. L., Jay, S. M., Gannon, J., Pancoast, J. R., Yalamanchi, P. et al. (2013). Growth differentiation factor 11 is a circulating factor that reverses age-related cardiac hypertrophy. Cell 153, 828–839. doi: 10.1016/j.cell.2013.04.015
Lough, T. J., and Lucas, W. J. (2006). Integrative plant biology: role of phloem long-distance macromolecular trafficking. Annu. Rev. Plant Biol. 57, 203–232. doi: 10.1146/annurev.arplant.56.032604.144145
Luo, S., Kleemann, G. A., Ashraf, J. M., Shaw, W. M., and Murphy, C. T. (2010). TGF-β and insulin signaling regulate reproductive aging via oocyte and germline quality maintenance. Cell 143, 299–312. doi: 10.1016/j.cell.2010.09.013
Mantovani, A., Locati, M., Vecchi, A., Sozzani, S., and Allavena, P. (2001). Decoy receptors: a strategy to regulate inflammatory cytokines and chemokines. Trends Immunol. 22, 328–336. doi: 10.1016/S1471-4906(01)01941-X
McCance, K. L., and Huether, S. E. (2002). Pathophysiology: The Biologic Basis for Disease in Adults and Children, 4th Edn. St. Louis, MO: Mosby.
Melo, J. A., and Ruvkun, G. (2012). Inactivation of conserved C. elegans genes engages pathogen- and xenobiotic-associated defences. Cell 149, 452–466. doi: 10.1016/j.cell.2012.02.050
Mitchell, P. S., Parkin, R. K., Kroh, E. M., Fritz, B. R., Wyman, S. K., Pogosova-Agadjanyan, E. L., et al. (2008). Circulating microRNAs as stable blood-based markers for cancer detection. Proc. Natl. Acad. Sci. U.S.A. 105, 10513–10518. doi: 10.1073/pnas.0804549105
Moremen, K. W., Tiemeyer, M., and Nairn, A. V. (2012). Vertebrate protein glycosylation: diversity, synthesis and function. Nat. Rev. Mol. Cell Biol. 13, 448–463. doi: 10.1038/nrm3383
Morisato, D., and Anderson, K. V. (1994). The spätzle gene encodes a component of the extracellular signaling pathway establishing the dorsal-ventral pattern of the Drosophila embryo. Cell 76, 677–688. doi: 10.1016/0092-8674(94)90507-X
Ng, S. F., Lin, R. C., Laybutt, D. R., Barres, R., Owens, J. A., and Morris, M. J. (2010). Chronic high-fat diet in fathers programs β -cell dysfunction in female rat offspring. Nature 467, 963–966. doi: 10.1038/nature09491
Nusse, R. (2003). Wnts and Hedgehogs: lipid-modified proteins and similarities in signaling mechanisms at the cell surface. Development 130, 5297–5305. doi: 10.1242/dev.00821
O'Brien, L. E., Soliman, S. S., Li, X., and Bilder, D. (2011). Altered modes of stem cell division drive adaptive intestinal growth. Cell 147, 603–614. doi: 10.1016/j.cell.2011.08.048
Okamoto, N., Nakamori, R., Murai, T., Yamauchi, Y., Masuda, A., and Nishimura, T. (2013). A secreted decoy of InR antagonizes insulin/IGF signaling to restrict body growth in Drosophila. Genes Dev. 27, 87–97. doi: 10.1101/gad.204479.112
Palm, W., Swierczynska, M. M., Kumari, V., Ehrhart-Bornstein, M., Bornstein, S. R., and Eaton, S. (2013). Secretion and signaling activities of lipoprotein-associated hedgehog and non-sterol-modified hedgehog in flies and mammals. PLoS Biol. 11:e1001505. doi: 10.1371/journal.pbio.1001505
Panáková, D., Sprong, H., Marois, E., Thiele, C., and Eaton, S. (2005). Lipoprotein particles are required for Hedgehog and Wingless signalling. Nature 435, 58–65. doi:10.1038/nature03504
Panayidou, S., and Apidianakis, Y. (2013). Regenerative inflammation: lessons from Drosophila intersinal epithelium in health and disease. Pathogens 2, 209–231. doi: 10.3390/pathogens2020209
Pedersen, B. K. (2011). Muscles and their myokines. J. Exp. Biol. 214, 337–346. doi: 10.1242/jeb.048074
Pedersen, B. K., and Febbraio, M. A. (2012). Muscles, exercise, and obesity: skeletal muscle as a secretory organ. Nat. Rev. Endocrinol. 8, 457–465. doi: 10.1038/nrendo.2012.49
Petersen, E. W., Carey, A. L., Sacchetti, M., Steinberg, G. R., Macaulay, S. L., Febbraio, M. A., et al. (2005). Acute IL-6 treatment increases fatty acid turnover in elderly humans in vivo and in tissue culture in vitro. Am. J. Physiol. Endocrinol. Metab. 288, E155–E162. doi: 10.1152/ajpendo.00257.2004
Rajan, A., and Perrimon, N. (2012). Drosophila cytokine unpaired 2 regulates physiological homeostasis by remotely controlling insulin secretion. Cell 151, 123–137. doi: 10.1016/j.cell.2012.08.019
Rera, M., Bahadorani, S., Cho, J., Koehler, C. L., Ulgherait, M., Hur, J. H., et al. (2011). Modulation of longevity and tissue homeostasis by the Drosophila PGC-1 homolog. Cell Metab. 14, 623–634. doi: 10.1016/j.cmet.2011.09.013
Rogers, D. W., Whitten, M. M., Thailayil, J., Soichot, J., Levashina, E. A., Catteruccia, F. (2008). Molecular and cellular components of the mating machinery in Anopheles gambiae females. Proc. Natl. Acad. Sci. U.S.A. 105, 19390–19395. doi: 10.1073/pnas.0809723105
Shimazu, T., Hirschey, M. D., Newman, J., He, W., Shirakawa, K., Le Moan, N., et al. (2013). Suppression of oxidative stress by β-hydroxybutyrate, an endogenous histone deacetylase inhibitor. Science 339, 211–214. doi: 10.1126/science.1227166
Sousa-Nunes, R., Yee, L. L., and Gould, A. P. (2011). Fat cells reactivate quiescent neuroblasts via TOR and glial insulin relays in Drosophila. Nature 471, 508–512. doi: 10.1038/nature09867
Srivastava, M., Simakov, O., Chapman, J., Fahey, B., Gauthier, M. E. A., Mitros, T., et al. (2010). The Amphimedon queenslandica genome and the evolution of animal complexity. Nature 466, 720–726. doi: 10.1038/nature09201
Steensberg, A., van Hall, G., Osada, T., Sacchetti, M., Saltin, B., and Klarlund Pedersen, B. (2000). Production of interleukin-6 in contracting human skeletal muscles can account for the exercise-induced increase in plasma interleukin-6. J. Physiol. 529, 237–242. doi: 10.1111/j.1469-7793.2000.00237.x
Stern, S., Fridmann-Sirkis, Y., Braun, E., and Soen, Y. (2012). Epigenetically heritable alteration of fly development in response to toxic challenge. Cell Rep. 1, 528–542. doi: 10.1016/j.celrep.2012.03.012
Straino, S., Germani, A., Di Carlo, A., Porcelli, D., De Mori, R., Mangoni, A., et al. (2004). Enhanced arteriogenesis and wound repair in dystrophin-deficient mdx mice. Circulation 110, 3341–3348. doi: 10.1161/01.CIR.0000147776.50787.74
Takeishi, A., Kuranaga, E., Tonoki, A., Misaki, K., Yonemura, S., Kanuka, H., et al. (2013). Homeostatic epithelial renewal in the gut is required for dampening a fatal systemic wound response in Drosophila. Cell Rep. 3, 919–930. doi: 10.1016/j.celrep.2013.02.022
Taylor, R. C., and Dillin, A. (2013). XBP-1 is a cell-nonautonomous regulator of stress resistance and longevity. Cell 153, 1435–1447. doi: 10.1016/j.cell.2013.05.042
van Oosten-Hawle, P., Porter, R. S., and Morimoto, R. I. (2013). Regulation of organismal proteostasis by transcellular chaperone signaling. Cell 153, 1366–1378. doi: 10.1016/j.cell.2013.05.015
Vilchez, D., Morantte, I., Liu, Z., Douglas, P. M., Merkwirth, C., Rodrigues, A. P. C., et al. (2012). RPN-6 determines C. elegans longevity under proteotoxic stress conditions. Nature 489, 263–268. doi: 10.1038/nature11315
Vong, L., Ye, C., Yang, Z., Choi, B., Chua, S. Jr., and Lowell, B. B. (2011). Leptin action of GABAergic neurons prevents obesity and reduces inhibitory tone to POMC neurons. Neuron 71, 142–154. doi: 10.1016/j.neuron.2011.05.028
Vrailas-Mortimer, A., del Rivero, T., Mukherjee, S., Nag, S., Gaitanidis, A., Kadas, D., et al. (2011). A muscle-specific p38 MAPK/Mef2/MnSOD pathway regulates stress, motor function, and life span in Drosophila. Dev. Cell 21, 783–785. doi: 10.1016/j.devcel.2011.09.002
White, L. E., Chaudhary, R., Moore, L. J., Moore, F. A., and Hassoun, H. T. (2011). Surgical sepsis and organ crosstalk: the role of the kidney. J. Surg. Res. 167, 306–315. doi: 10.1016/j.jss.2010.11.923
Wigby, S., and Chapman, T. (2005). Sex peptide causes mating costs in female Drosophila melanogaster. Curr. Biol. 15, 316–321. doi: 10.1016/j.cub.2005.01.051
Wu, S. C., Liao, C. W., Pan, R. L., and Juang, J. L. (2012). Infection-induced intestinal oxidative stress triggers organ-to-organ immunological communication in Drosophila. Cell Host Microbe 11, 410–417. doi: 10.1016/j.chom.2012.03.004
Yalak, G., and Vogel, V. (2012). Extracellular phosphorylation and phosphorylated proteins: not just curiosities but physiologically important. Sci. Signal. 5, re7. doi: 10.1126/scisignal.2003273
Yi, P., Park, J. S., and Melton, D. A. (2013). Betatrophin: a hormone that controls pancreatic β cell proliferation. Cell 153, 747–758. doi: 10.1016/j.cell.2013.04.008
Yoshimoto, S., Loo, T. M., Atarashi, K., Kanda, H., Sato, S., Oyadomari, S., et al. (2013). Obesity-induced gut microbial metabolite promotes liver cancer through senescence secretome. Nature 499, 97–101. doi: 10.1038/nature12347
Zeybel, M., Hardy, T., Wong, Y. K., Mathers, J. C., Fox, C. R., Gackowska, A., et al. (2012). Multigenerational epigenetic adaptation of the hepatic wound-healing response. Nat. Med. 18, 1369–1377. doi: 10.1038/nm.2893
Keywords: interorgan communication network, non-cell autonomous signaling, systemic disease, organismal homeostasis, systemic stress response
Citation: Droujinine IA and Perrimon N (2013) Defining the interorgan communication network: systemic coordination of organismal cellular processes under homeostasis and localized stress. Front. Cell. Infect. Microbiol. 3:82. doi: 10.3389/fcimb.2013.00082
Received: 20 August 2013; Accepted: 30 October 2013;
Published online: 19 November 2013.
Edited by:
Yiorgos Apidianakis, University of Cyprus, CyprusReviewed by:
Chrysoula Pitsouli, University of Cyprus, CyprusCopyright © 2013 Droujinine and Perrimon. This is an open-access article distributed under the terms of the Creative Commons Attribution License (CC BY). The use, distribution or reproduction in other forums is permitted, provided the original author(s) or licensor are credited and that the original publication in this journal is cited, in accordance with accepted academic practice. No use, distribution or reproduction is permitted which does not comply with these terms.
*Correspondence:cGVycmltb25AcmVjZXB0b3IubWVkLmhhcnZhcmQuZWR1;
ZHJvdWppbmluZUBnLmhhcnZhcmQuZWR1
Disclaimer: All claims expressed in this article are solely those of the authors and do not necessarily represent those of their affiliated organizations, or those of the publisher, the editors and the reviewers. Any product that may be evaluated in this article or claim that may be made by its manufacturer is not guaranteed or endorsed by the publisher.
Research integrity at Frontiers
Learn more about the work of our research integrity team to safeguard the quality of each article we publish.