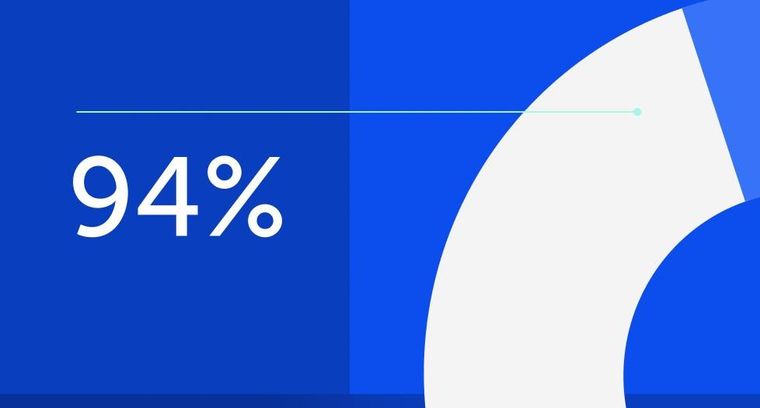
94% of researchers rate our articles as excellent or good
Learn more about the work of our research integrity team to safeguard the quality of each article we publish.
Find out more
REVIEW article
Front. Chem., 05 November 2024
Sec. Organic Chemistry
Volume 12 - 2024 | https://doi.org/10.3389/fchem.2024.1485301
This article is part of the Research TopicSynthesis, Structural Analysis, and Conformational Behavior of BODIPY Dyes and Their CongenersView all 4 articles
Icosahedral boron clusters-based BODIPY dyes represent a cutting-edge class of compounds that merge the unique properties of boron clusters with the exceptional fluorescence characteristics of BODIPY dyes. These kinds of molecules have garnered substantial interest due to their potential applications across various fields, mainly including optoelectronics, bioimaging, and potential use as boron carriers for Boron Neutron Capture Therapy (BNCT). Carborane clusters are known for their exceptional stability, rigid geometry, and 3D-aromaticity, while BODIPY dyes are renowned for their strong absorption, high fluorescence quantum yields, and photostability. The integration of carborane into BODIPY structures leverages the stability and versatility of carboranes while enhancing the photophysical properties of BODIPY-based fluorophores. This review explores the synthesis and structural diversity of boron clusters-based BODIPY dyes, highlighting how carborane incorporation can lead to significant changes in the electronic and optical properties of the dyes. We discuss the enhanced photophysical characteristics, such as red-shifted absorption and emission poperties, charge and electronic transfer effects, and improved cellular uptake, resulting from carborane substitution. The review also delves into the diverse applications of these compounds. In bioimaging, carborane-BODIPY dyes offer superior fluorescence properties and cellular internalization, making them ideal for cell tracking. In photodynamic therapy, (PDT) these dyes can act as potent photosensitizers capable of generating reactive oxygen species (ROS) for targeted cancer treatment making them excellent candidates for PDT. Additionally, their unique electronic properties make them suitable candidates for optoelectronic applications, including organic light-emitting diodes (OLEDs) and sensors. Overall, carborane-BODIPY dyes represent a versatile and promising class of materials with significant potential for innovation in scientific and technological applications. This review aims to provide a comprehensive overview of the current state of research on carborane-BODIPY dyes, highlighting their synthesis, properties, and broad application spectrum.
Among the vast array of known fluorescent dyes, the 4,4-difluoro-4-bora-3a,4a-diaza-s-indacene more known as boro-dipyrromethene (abbreviated as BODIPY; Chart 1) has emerged as one of the most versatile reagents and has garnered popularity among the scientific community. BODIPYs have intrinsic unique properties, including strong electron donating properties, excellent chemical, thermal and optical stability, high molar absorptivity and quantum yield (QY or φF) and versatility for their functionalization at different positions. BODIPY dyes show a strong and broad absorption band at around 500–550 nm, corresponding to the S0-S1 transition (π-π*) with high molar extinction coefficients (4000–110000 M−1cm−1), emit a narrow spectrum between 530–560 nm and present high fluorescence QY. Additionally, they exhibit thermal and photochemical stability and good solubility in various organic solvents; some derivatives are also soluble in water.
Chart 1. (A) Structure of BODIPY, IUPAC and common nomenclature and main accessible routes for the functionalization. (B) (Top) Isomeric neutral icosahedral boron clusters: o-carborane, m-carborane and p-carborane. (Bottom) Anionic boron clusters with their vertexes numbering.
These dyes are relatively easy to synthesize, and various methods can be employed to modify the BODIPY core at the α, β, meso position, and at the boron atom employing adequate conditions (Chart 1A). (Loudet and Burgess, 2007) These methods encompass Knoevenagel-type condensation, nucleophilic and electrophilic substitutions, Pd-catalyzed C-C cross-coupling reactions, Liebeskind-Srogl cross-coupling, Grignard reactions, direct styrylation, replacement of fluorine atoms at the boron centre, etc. All these synthetic techniques can be utilized to synthesize BODIPY derivatives, allowing for precise adjustment of their photonic and electronic properties. Due to all the characteristics described above, in the last 3 decades, a significant number of BODIPY derivatives have been designed and synthesized for their use in material science and biomedical applications (Li et al., 2024; Das et al., 2023), such as fluorescent switches, electroluminescent films, laser dyes (Avellanal-Zaballa et al., 2022), energy transfer cassettes, light harvesting, triplet photosensitizers (for solar cells, organo photocatalysis and of singlet oxygen production for photodynamic therapy) (Yadav and Misra, 2023), nonlinear optics, bioimaging, and chemosensing (Bumagina and Antina, 2024).
Carboranes are a unique class of inorganic polyhedral clusters composed primarily of boron, carbon, and hydrogen atoms. Their distinct structural characteristics and remarkable chemical stability have garnered significant interest across various fields of chemistry and materials science. Among the carborane cluster families, the twelve-vertex icosahedral dicarba-closo-dodecaborane, also known as closo-carborane (C2B10H12), and its derivatives are the most extensively studied (Chart 1B). (Grimes, 2016; Scholz and Hey-Hawkins, 2011), These clusters, considered analogues to arenes, show three dimensional (3D) geometry and σ-aromaticity (Poater et al., 2014; Poater et al., 2020). The ortho-carborane (o-carborane, 1,2-C2B10H12) can be thermally isomerized to produce meta-carborane (m-carborane, 1,7-C2B10H12) (Chart 1B) and finally, para-carborane (p-carborane, 1,12-C2B10H12) (Grimes, 2016). These neutral carboranes are remarkably robust, with unique properties such as hydrophobicity, electronic and thermal stability, chemical versatility to be functinalized both at C and B atoms of the cluster) and low toxicity in biological systems. Moreover, the closo-o-carborane can be partially deboronated in basic media, by removing formally a BH+ vertex to give the corresponding anionic species nido-o-carborane (Chart 1B). Deprotonation of nido-o-carborane and further reaction with transition metal salts (mainly NiCl2, CoCl2 and FeCl2) led to the formation of anionic θ-shape metallacarboranes (Chart 1B). These metallacarboranes have high molecular volume, low nucleophilic character, low charge densities, as well as extraordinary high chemical, thermal and photochemical stability, and excellent biocompatibility (Housecroft, 2011). All these properties make these boron clusters promising building blocks for a wide variety of applications (Fisher et al., 2019; Sivaev, I. B. and Bregadze, 2019) such as optoelectronic materials (Mukherjee and Thilagar, 2016; Núñez et al., 2016b; Ochi et al., 2020; Parejo et al., 2021), polymers (Núñez et al., 2016a), and biomedical applications (Valliant et al., 2002; Sivaev and Bregadze, 2009; Issa et al., 2011; Vinas, 2013; Leśnikowski, 2016; Stockmann et al., 2019), among others.
In recent years, the luminescent properties of carborane-containing compounds have become a focal point of research, particularly due to their potential applications in optoelectronics, sensing, and bioimaging. The incorporation of carborane moieties into organic and inorganic frameworks can profoundly influence the photophysical properties of the resulting materials. This influence is attributed to the electron-deficient nature of boron atoms within the carborane cluster, which can modulate electronic transitions and enhance luminescent efficiency. Regarding the electronic effect, o-carborane behaves as a strong electron-withdrawing group (similar to fluorinated aryl) on a substituent at one of the cluster carbons (Ccluster, Cc). When o-carborane is linked to an aromatic donor group, the intramolecular charge transfer (ICT) process occurs from the donor moiety to the acceptor boron cluster, which is influenced by the Cc-Cc bond vibration (Oliva et al., 2005), usually producing the quenching of the fluorescence in solution. Moreover, carboranes are known to impart unique photophysical behaviors such as aggregation-induced emission (AIE) and thermally activated delayed fluorescence (TADF) (Ochi et al., 2020). These phenomena are of considerable interest for developing advanced luminescent materials. The rigidity and electron-withdrawing nature of carboranes stabilize excited states, thereby extending emission lifetimes and improving QYs in aggregate and solid state (Naito et al., 2017; Tu et al., 2019; Wei et al., 2019; Ochi et al., 2020; Cabrera-Gonzalez et al., 2016; 2017; Chaari et al., 2018b; 2019; 2020; Cabrera-González et al., 2020).
Carborane-BODIPY dyes represent an innovative class of luminescent compounds that uniquely combine the exceptional properties of boron-rich carborane clusters with the renowned fluorescence characteristics of BODIPY fluorophores. Carborane clusters, known for their chemical stability and electron-withdrawing capabilities, provide a robust and versatile framework that significantly enhances the optical and electronic properties of BODIPY dyes. The photophysical behavior of these carborane-based BODIPY dyes is primarily shaped by the strong electronic interactions between the carborane cluster and the BODIPY core. These interactions often result in notable modifications to the absorption and emission spectra, most prominently inducing a red-shift (bathochromic shift) in the fluorescence emission. This shift is attributed to the stabilization of the lowest unoccupied molecular orbital (LUMO) by the electron-withdrawing effect of the carborane unit, thereby narrowing the HOMO-LUMO energy gap. The integration of carborane clusters not only enhances fluorescence but also improves the overall chemical stability and bio-compatibility of the molecules, making them highly promising for a range of cutting-edge applications, particularly in biomedicine, where targeted imaging and therapy are crucial, as well as in advanced optoelectronic devices. Despite the interesting synergy in the properties of both fragments, BODIPY and boron clusters, the small number of examples of these systems in the literature is surprising as shown in graph depicted in Chart 2.
Chart 2. Number of publications of BODIPYs (blue bars) and BODIPYs containing boron clusters (orange bars) since 1990. The data was taken from the Web of Science.
This review aims to describe the various synthetic routes for incorporating different boron clusters (carboranes and metallacarboranes) into BODIPY cores, delve into the mechanisms by which carborane clusters affect the photoluminescence properties, explore recent advancements in luminescent carborane-based BODIPY dyes, and highlight their potential applications. By understanding the interplay between carborane structure and luminescent properties, we can pave the way for materials with tailored photophysical characteristics for cutting-edge technological applications. The review is organized into several sections, classifying carborane-BODIPY dyes based on their properties and potential applications. Most of the BODIPYs featuring boron clusters identified in the literature can be gathered into two main categories. The first category includes BODIPY systems with notable photophysical properties, in which the presence of the boron cluster can influence the fluorescence resonance energy transfer or Förster resonance energy transfer (FRET), photoinduced electron transfer (PET), or AIE processes. In this category we have also included nanocar-type systems suitable for single-molecule fluorescence microscopy (SMFM) experiments. The second large section includes those designed boron cluster containing BODIPY for biological and biomedical applications, mainly in vitro bioimaging and Boron Neutron Capture Therapy (BNCT).
Ziessel and coworkers synthesized a series of fluorescent dyads using a p-carborane as a linker between different fluorophores, with one acting as the electron-donor group and the other as the electron-acceptor group (Scheme 1). These systems demonstrated spatial energy transfer through the carborane linker making them promising candidates for optoelectronic devices, sensors, data storage or data computing.
Scheme 1. Synthesis of BDP-pCB-1. Steps: (i) nBuLi, THF, −78°C, CuBr, 4-(bromoethynyl)iodobenzene, 35%; (ii) BDP-1, benzene, triethylamine, [Pd(PPh3)4], 60°C, overnight, 33%; (iii) BDP-2, benzene, Et3N, [Pd(PPh3)4], 60°C, 36 h, 70%.
In 2010, They synthesized a rigid molecular donor-acceptor dyad, BDP-pCB-1, arranged in a rod-like structure using a 1,12-dicarba-closo-dodecaborane (p-carborane) core to link two distinct BODIPY modules. This design was intended to facilitate through-space energy transfer (Ziessel et al., 2010). The target molecule BDP-pCB-1 was synthesized by metalation of p-carborane employing n-BuLi and CuBr under kinetical control followed by adding 4-(bromoethynyl)iodobenzene to provide the disubstituted compound 1. Taking advantage of the presence of two iodo groups at the vertices of compound 1, it is possible to link ethynyl-substituted BODIPY dyes by a sequence of two Sonogashira cross-coupling reactions to yield rigid dyad BDP-pCB-1.
Two years later, unsymmetrical BDP-pCB-2-4 derivatives were synthesized by the same authors (Schemes 2, 3). In these donor-acceptor dyads, the BODIPY (λabs/λem at 645/659 nm) was chosen as acceptor unit and subphthalocyanine (SubPc: λabs/λem at 565/574 nm) in BDP-pCB-2 and diketopyrrolopyrrole (DPP: λabs/λem at 645/659 nm) in BDP-pCB-3 and BDP-pCB-4 as donor cores (Hablot et al., 2012). Dyads BDP-pCB-2 and BDP-pCB-3 were obtained from common intermediate 4 (Scheme 2); firstly, bonding the BDP-6 with 4 by Sonogashira cross-coupling reaction followed by deprotection of triethylsilyl (TES) group with KF to yield BDP-7. Finally, compound BDP-7 was linked to a second fluorophore, such as A or B, by other Sonoghashira reactions to produce the dyads BDP-pCB-2 and BDP-pCB-3. Additionally, dyad BDP-pCB-4 with two p-carborane linkers between BODIPY and SubPc cores was prepared through a sequence of Pd-catalyzed couplings (Scheme 3), to compare the effect of increasing the length of the spacing unit on the efficiency of electronic energy transfer between the donor to the acceptor systems.
Scheme 2. Steps: (i) nBuLi, CuBr, 4-(bromoethynyl)iodobenzene; (ii) thiethylsilylacetylene, [Pd(PPh3)2Cl2]/CuI, 92%; (iii) nBuLi, CuBr, 4-(bromoethynyl)iodobenzene; (iv) 1) [Pd(PPh3)4]/CuI, compound BDP-6, 93%, 2) KF, 92%; (v) [Pd(PPh3)4]/CuI, compound B, 85%, (vi) [Pd(PPh3)4]/CuI, compound A, 86%.
Scheme 3. Steps: (i) trimethylsilylacetylene, [Pd(PPh3)2Cl2]/CuI, 91%; (ii) K2CO3 (1 equiv.), rt, 86%; (iii) [Pd(PPh3)4], triethylamine, compound A, 86%; (iv) 1) KF, 50%, 2) [Pd(PPh3)4], Et3N, compound 7, 78% 3) KF, 52% (v) [Pd(PPh3)4], compound BDP-4, 78%.
In 2013, Ziessel, Harriman, and coworkers reported two other donor-acceptor dyads, BDP-pCB-5 and BDP-oCB-6 (Schemes 4, 5), employing para- and ortho-carborane as linkers, respectively (Harriman et al., 2013). In each compound, the acceptor unit is a BODIPY (BDP: λabs/λem at 672/687 nm) conjugated to two ethenylthiophene groups, and the donor unit is a diketopyrrolopyrrole (DPP: λabs/λem at 605/627 nm). Detailed synthetic steps for synthesizing these dyads are shown in Schemes 4, 5, respectively. Intermediate BDP-10 was synthesized in three steps. Firstly, a Knoevenagel-type condensation reaction between BDP-8 and thiophene derivative 9 gives BDP-9. Reaction of BDP-9 with trimethylsilylacetylene under Pd-catalyzed cross-coupling reaction conditions followed by subsequent removal of the trimethylsilane (TMS) group yielded BDP-10. The rest of the synthesis routes to obtain dyads BDP-pCB-5 and BDP-oCB-6 followed the same strategy based on Pd-catalyzed cross-coupling reactions between pre-functionalized carborane clusters and fluorophores.
Scheme 4. Steps: (i) piperidine, pTSOH cat., toluene, reflux, 52%; (ii) 1) [Pd(PPh3)2Cl2], CuI, trimethylsilylacetylene, benzene/Et3N, 60°C, 97%, 2) K2CO3, THF/MeOH/H2O, room temperature (r.t.), 94%. (iii) 4, [Pd(PPh3)4], benzene/Et3N, 60°C, 97%; (iv) 1) NaOH/H2O, 60°C, 79%; 2) DPP, [Pd(PPh3)4], benzene/Et3N, 60°C, 78%.
Scheme 5. Steps: (i) BDP-10, [Pd(PPh3)4], benzene/Et3N, 60°C, 72%; (ii) 1) [Pd(PPh3)2Cl2], CuI, trimethylsilylacetylene, benzene/Et3N, 50°C, 84%, 2) K2CO3, THF/MeOH/H2O, r.t, 92%; 3) DPP, benzene/Et3N, 60°C, 84%.
The same year, Ziessel and Harriman reported a series of linear donor-spacer acceptor compounds, including the previously reported BDP-pCB-2 and new dyads BDP-pCB-7, BDP-pCB-8, BDP-pCB-9 and BDP-pCB-10 (Scheme 6) (Hablot et al., 2013). The spacer was built by increasing of p-ethynylene-carborane units, which provided acceptor-donor separation distances of 38, 57, 76, 96, and 115 Å along the series. All dyads were synthesized using the building blocks shown in Scheme 6, following an iterative synthetic protocol. The first step involves a Pd-catalyzed cross-coupling reaction between BDP-13 and compound 11. Deprotection of the triethysilyl group using NaOH as base afforded the BDP-pCB(H) cross-linking to DPP(Br) to obtain BDP-pCB-8 with n = 1. BDP-pCB(H) was coupled to a second compound 4 and then deprotected and involved in further cross-linking with DPP(Br) yielding dyad BDP-pCB-7 with n = 2. The rest of the dyads BDP-pCB9 and BDP-pCB-10 were prepared following the same strategy using BDP-pCB(H) as a critical component to extend the bridge.
In BDP-pCB-1, the BDP-1 core acts as an electron donor unit; it contains two dimethylamino groups sensitive to pH, enabling variation of the spectral overlap to the donor module, BDP-2 core acts as an electron acceptor unit, and p-carborane has no significant electronic influence. Optical data for all compounds in this section are summarized in Supplementary Table S1. Protonation of BDP-pCB-1 (in dioxane) with HCl (g) induces a strong hypsochromic shift, shifting the absorption band from 694 nm to 628 nm and the emission band from 728 to 638 nm, while increasing the QY from 30% to 42%. Remarkably, the absorption and emission spectra of BDP-pCB-1 in its deprotonated and protonated forms are linear combinations of the absorption and emission of BDP-1 (deprotonated and protonated) and BDP-2 (λabs = 525 nm and λem = 538 nm). Förster Resonance Energy Transfer (FRET) efficiency between two BODIPY modules in dyad BDP-pCB-1 in its deprotonated and protonated forms was calculated from measured fluorescence QYs to be 82% and 89%, with constant for FRET of 6.2 × 108 and 1.7 × 109 S−1 respectively, and a center D-A distance of 36.8 Å.
Compounds BDP-pCB 2, BDP-pCB 3 and BDP-pCB-4 exhibited absorption and emission spectra show the bands typical of each photoactive module with no significant influence of the p-carborane linker (Supplementary Table S1). Dyads BDP-pCB-2 and BDP-pCB-3 present a very efficient electronic energy transfer (EET>99%) with rate constants calculated for EET of 1.2 × 1010 s−1 and 1.7 × 109 s−1, respectively. Compound BDP-pCB-4 reaches 76% of EET with a rate constant of 3.2 × 108 s−1, indicating that increasing the distance between donor-acceptor groups decreases EET efficiency.
The excitation spectra confirmed that intramolecular EET occurs from DPP to BODIPY on BDP-pCB5 and BDP-oCB6 dyads. The donor’s emission spectrum and the acceptor’s absorption spectrum showed excellent spectral overlap, resulting in high efficiency for EET. BDP-pCB-5 has a KEET of 3.1 ± 0.2 × 109 s−1 and an efficiency EET process >90% with a donor-acceptor distance of 36 Å. The dyad BDP-oCB-6 has a KEET of 1.9 ± 0.2 × 1010 s−1 and an efficiency EET process >95% with a donor-acceptor distance of 19 Å. In line with the previous report, it is worth noting that the EET efficiency and the KET increased when the acceptor and the donor units were closer to each other.
In compounds BDP-pCB-2 and BDP-pCB-7-10, the intensity of both absorption and emission as well as fluorescent QY increase with the length of dyads (Supplementary Table S1). It is noteworthy that BDP-pCB-2 produces poor fluorescent QY (0.09), which is much lower than the larger dyads (QY = 0.44, 0.73, 0.81, and 0.84). Computational calculations for all dyads established that the Förster critical distance of the two isolated chromophores is 54.4 Å at room temperature and 52.8 Å at 77 K, which supports the experimental data. The efficiency parameters of EET between acceptor-donor units depend on the length of the spacer and external factors such as temperature or pressure. As the temperature decreases (spectra recorded in MTHF), the emission maxima band of the BODIPY acceptor is red-shifted while the emission maxima band of the acceptor is blue-shifted; consequently, there is a progressive decrease in the integral of the spectral overlap, and therefore it decreases EET efficiency from DPP to BODIPY. Additionally, when pressure increases, there is a decrease in the fluorescence of BODIPY and an increase in the fluorescence of the DPP, which reduces the probabilities of EET.
In 2015, Hawthorne et al. reported the synthesis of a nickelacarborane complex (BDP-NiMCB-1, Scheme 7) bearing two fluorophore molecules capable of Förster resonance energy transfer (FRET). In this dye, the N-boc-tryptophan and 8-(40-bromophenyl)-4,4-difluoro-4-bora-3a, 4a-diaza-s-indacene were chosen as the FRET donor and acceptor groups, respectively (Shlyakhtina et al., 2015). The first attempt to prepare BDP-NiMCB-1 was through the synthesis of BDP-16 upon deboronation reaction from BDP-15 using different nucleophiles (Scheme 7A), however only traces of the target BDP-16 were obtained. The second synthetic strategy is presented in Scheme 7B, C. Successful deboronation of compounds 14 and 16 led to the formation of the corresponding nido-carborane derivatives 15 and 17 (Scheme 7B). The synthesis of the mixed-ligand Ni(III) nickelacarborane 18 was carried out by the reaction of stoichiometric amounts of tetrabutylammonium salts of nido-carboranes 15 and 17 with excess butyllithium and then with Ni(acac)2 in THF (Scheme 7B). After a complicated separation of complexes 18, 19, and 20 by chromatography, paramagnetic compound 21 was formed in excellent yield (92%) by deprotonation reaction of 18 with TBAF in THF (Scheme 7B). The reaction of 21 with previously esterified N-boc-triptophan in the presence of Et3N in dichloromethane (DCM) gave the corresponding intermediate in 76% yield (Scheme 7C). The final step was the Sonogashira cross-coupling reaction of the intermediate with BDP-14 in the presence of Pd(PPh3)4 catalyst to give BDP-NiMCB-1 in 5.5% yield (Scheme 7C).
Scheme 7. (A) Reaction to attempt the synthesis of BDP-16 containing nido-o-carborane. (B, C) Synthetic procedure for the metallacarborane-based BODIPY BDP-NiMCB-1.
The absorption spectrum of BDP-NiMCB-1 showed characteristic bands for all parts of the molecules. The absorption band centered at around 300 nm corresponds to tryptophan and the linker, whereas the maximum of absorption at 500 nm and the broad band in the 350–400 nm region corresponds to the BODIPY. The emission spectrum showed a maximum centered at 532 nm, a low-intensity band at 345 nm, attributed to the residual fluorescence of tryptophan, and a broad emission in the range 450–500 nm, with difficult assignation. The presence of the BODIPY emission band at 532 nm can only be explained by FRET in solution.
The development of electron donor-acceptor systems that exhibit photoinduced electron transfer (PET) have attracted significant attention in many areas, including charge separation processes in photovoltaics, molecular electronics, light-emitting diodes, photoluminescence sensors and switches. In solar energy conversion and storage, and photovoltaics. Low-energy photosensitization using electron donor-acceptor dyads is crucial for efficient solar light harvesting in artificial photosynthesis systems and solar cells (Kulinich and Ishchenko, 2024; Tan et al., 2023; Imahori and Akiyama, 2024; Feng et al., 2023). In the following section, we will describe a series of BODIPY functionalized with carborane derivatives that exhibited photoinduced electron transfer (PET) processes.
In 2018, E. Hamuryudan et al. (Berksun et al., 2018) synthesized the porphyrin–o-carborane–BODIPY triad BDP-oCB-11 by the reaction of a previously prepared porphyrin-BDP-17 dyad with decaborane (B10H14), using N,N-dimethylaniline in toluene at reflux for 12 h. After extraction and purification by column chromatography on silica gel, hexane/DCM (2:1, v/v) was used as the eluent, and compound BDP-oCB-11 was obtained in 46% yield (Scheme 8A).
The UV-Vis spectrum of the compound BDP-oCB-11 in toluene showed two separated bands at 424 and 506 nm, which correspond to the absorption bands of the porphyrin and BODIPY fragments, respectively (Berksun et al., 2018). The spectrum of the compound was very similar to their independent precursors, the BODIPY and the porphyrin, which suggested that the presence of the carborane as a linker between both fluorophores does not affect the electronic properties of the triad, and there is no significant ground state interaction between the fluorophores. BDP-oCB-11 exhibited weak fluorescence in THF with low quantum yield of 0.028 that was attributed to the quenching caused by the o-carborane. Due to the electron-withdrawing character of the o-carborane through the Cc (Núñez et al., 2006), when the porphyrin or the BODIPY in the BDP-oCB-11 were selectively excited, an efficient PET occurs through the antibonding orbitals of the C–C bonds of the o-carborane, producing the quenching of the BDP-oCB-11. When the BODIPY unit in triad BDP-oCB-11 was selectively excited, was observed a resonance energy transfer (RET) to the corresponding porphyrin unit. However, it was not possible to calculate the energy transfer efficiency for the triad, as the fluorescence of the donor unit was almost completely quenched, due to the PET process in the presence of o-carborane.
In 2015, Kang and coworkers reported on two V-shaped triads, BDP-oCB-12 and BDP-oCB-13, designed for low-energy photosensitization (Jin et al., 2015). These dyads comprise two BODIPY electron donor units attached to two adjacent carbon atoms of the central o-carborane, which serve as an acceptor group. Detailed synthetic routes for BDP-oCB-12 and BDP-oCB-13 are described in Scheme 8B. Through the reaction between the decaborane-Lewis base complex arachno-B10H12(SEt2)2 and alkyne derivatives BDP-18 and BDP-19, it is possible to form the o-carborane moiety, resulting in the generation of BDP-oCB-12 and BDP-oCB-13, respectively. The intermediate BDP-18 and BDP-19 were obtained following a literature procedure reported by Ziessel (Ulrich and Ziessel, 2004).
In both BDP-oCB-12 and BDP-oCB-13, the electron-acceptor o-carborane group reduces the fluorescence intensities and lifetimes (Supplementary Table S2) of two electron-donor BODIPYs units through PET exergonic process, mainly from S1 to CT states. The calculated efficiencies were 63% and 71%, respectively. Theoretical calculations and cyclic voltammetry were also performed, supporting the PET hypothesis. Additionally, BODIPY.+ and CB.- species generated through the PET process were identified via spectroelectrochemical (SEC) measurements.
Three years later, Kang and colleagues elucidated the dynamics of photoinduced electron transfer (PET) of BDP-oCB-13 using femtosecond time-resolved transient absorption spectroscopy (Kim et al., 2018). The PET process occurs in the S1 state (Marcus normal region) and is strongly influenced by the driving force (-ΔG), which is controlled by solvent polarity; so, PET in the S1 state is faster in polar solvents than in nonpolar ones (τs1 = 0.72 ns in CH3CN, 1.22 ns in CH2Cl2 and 2.72 ns in n-hexane). On the other hand, deactivation via internal conversion prevents the PET process occurring from the higher energy S2 state.
In recent decades, significant effort has been dedicated to designing, synthesizing, and operating synthetic molecular machines. Tour and coworkers have designed nanocars that can observe and track molecular motion using scanning tunneling microscopy (STM) and single-molecule fluorescence microscopy (SMFM), offering unparalleled atomic resolution (Morin et al., 2007; Khatua et al., 2009; Vives and Tour, 2009; Claytor et al., 2009). In 2010 they designed and synthesized three highly fluorescent nanocars: BDP-pCB-14 (Scheme 9A), BDP-pCB-15, and BDP-pCB-16 (Scheme 9B). (Godoy et al., 2010) The nanocar’s axle consists of two units of BODIPY, and four p-carborane units form part of the wheels, which are important in the translational motion. Nanocars BDP-pCB-14 and BDP-pCB-15 move in a straight line on surfaces. In contrast, nanocar BDP-pCB-16 exhibits circular surface motion that could be detected by measuring the polarization anisotropy distribution. The compounds were prepared following a modular and convergent synthesis (Scheme 9). Axle BDP-23 was obtained through a sequence of 4 steps with an overall yield of 58%. The first step involved a Sonogashira reaction between known BDP-8 (Burghart et al., 1999) and ethynyltrimethylsilane resulting in compound BDP-20. Next, BDP-20 underwent iodination at positions 2 and 6 using N-iodosuccinimide (NIS) to yield BDP-21. A double Sonogashira cross-coupling reaction between BDP-22 and 1-ethynyl-p-carborane (22), followed by deprotection of the TMS group under basic conditions, allowed the formation of axle BDP-23. The homocoupling Eglinton-Glaser-type reaction, using PdCl2(PPh3)2 as a catalyst of BDP-23, provides nanocar BDP-pCB-14. For the synthesis of nanocars BDP-pCB-15 and 16, compound BDP-23 was also used as an axle. Both compounds were synthesized by means of a double Sonogashira reaction using 1,4-diiodo-2,5-dimethoxybenzene (23) and 9-butyl-2,6-diiodo-9H-carbazole (24) as coupling patterns, respectively.
Scheme 9. (A) Synthesis of BDP-pCB-14. Steps: (i) NIS, dimethylformamide (DMF), CH2Cl2, 84%; (ii) THF, Et3N, 60°C, PdCl2(PPh3)2, CuI, 88%; (iii) K2CO3, THF/MeOH, 79%; (iv) PdCl2(PPh3)2, CuI, air, THF, Et3N, 85%. (B) Synthesis of BDP-pCB-15 and BDP-pCB-16. Reaction conditions: (i) PdCl2(PPh3)2, CuI, THF, Et3N, 50°C.
The optical properties of axle BDP-23 and all nanocars were analyzed using UV-Vis and fluorescence spectroscopy, and the summarized data is provided in Supplementary Table S3. The three nanocars displayed intense absorption bands, and the values of extinction coefficients varied depending on the substitution pattern. The fluorescent QY of all nanocars was found to be slightly smaller than that of axle BDP-23, possibly due to through-bond energy transfer between the BODIPYs cores. The high fluorescent QYs make all the nanocars suitable for single-molecule fluorescence microscopy (SMFM) experiments.
Some organic π-conjugated molecules exhibit aggregation-caused quenching (ACQ) (Jenekhe and Osaheni, 1994). Conversely, some organic π-conjugated molecules exhibit AIE, wherein non-emissive molecules in solution are induced to emit light under aggregate formation conditions; due to these characteristics, AIE-active compounds are top candidates for responsive materials and solid-state luminescent devices (Xie et al., 2024; Rana et al., 2023; Bhosle et al., 2024). It is well known that in solution, the o-carborane, acts as an electron-withdrawing group (Teixidor et al., 2000; Núñez et al., 2006), and triggers the PET process in the system, (Ferrer-Ugalde et al., 2014), when attaching to aromatic units through carborane Cc bonds. In addition, the phenyl units rotate around the Cc-C bond. These two mechanisms, PET and rotation, increase the nonradiative decay, producing a quenching of the fluorescence emission. On the contrary, after aggregation in solution, the efficiency of PET and rotation decrease, and the phenomenon of AIE takes place, leading to an increase of the emission, confirming that the o-carborane acts as a good aggregation-induced emission generator (AIE-gen).
In 2014, Chujo and coworkers synthesized a series of organic dyes based on o-carborane with the aim of studying emission behaviors, intramolecular charge transfer (ICT), energy transfer, AIE, and ACQ (Tominaga et al., 2014). Two organic dyes, BDP-o-CB-17 and BDP-oCB-18 (Scheme 10A), contain tolane-BODIPY units linked to the 9,12-boron position of o-carborane were prepared. Additionally, BDP-oCB-17 has a phenanthrene unit linked to the Cc position of o-carborane. Compound 25 (Tominaga et al., 2013) was treated with nBuLi to deprotonate the Cc. Subsequent Ullman-type coupling with 9-iodophenanthrene formed compound 26. The removal of trimethylsilyl (TMS) groups of 26 using K2CO3 as a base provided compound 27. Finally, a Sonogashira cross-coupling reaction between 27 and BDP-8 afforded the target molecule BDP-oCB-17. On the other hand, the BDP-oCB-18 was synthesized via a Sonogashira coupling between compound 28 and BDP-8.
Scheme 10. (A) Synthesis of BDP-oCB-17 and BDP-oCB-18. Keys: (i) 1) nBuLi, MeOCH2CH2OMe, 0°C, 30 min, 2) CuCl, room temperature, 2 h, 3) 9-iodophenanthene, pyridine, reflux, 5 h, 27% for 3 steps; (ii) K2CO3, THF/MeOH, room temperature, 2 h, 93%; (iii) BDP-8, Pd(PPh3)4, CuI, THF/Et3N, 40°C, 15 h, 33%; (iv) BDP-8, Pd(PPh3)4, CuI, THF/Et3N, 40°C, 15 h, 33%. (B) Synthesis of BDP-oCB-19.
Supplementary Table S4 outlines the results from the absorption and photoluminescence spectroscopy analyses. BDP-oCB-17 and BDP-oCB-18 have similar emission behaviors. In a THF dilute solution, both exhibited intense photoluminescence upon excitation at 310 nm, emitting green light from the BODIPY moiety. BDP-oCB-17 and BDP-oCB-18 have fluorescence QYs of 0.33 and 0.40, respectively. In contrast, neither dye emits in both aggregate and solid state due to energy transfer from tolanes and phenanthrene (in BDP-oCB-17) to the BODIPY cores, and the subsequent ACQ mechanism.
In 2019, Hamuryudan et al. synthesized the BODIPY triad BDP-oCB-19 (Nar et al., 2019) following a similar process to one previously reported by the same authors (Berksun et al., 2018). The decaborane insertion to the ethynyl derivative TPE-BDP-24 in dry toluene gave the BODIPY triad BDP-oCB-19 in a 47% yield (Scheme 10B).
BDP-oCB-19 displayed two absorption bands in THF, the one at 311 nm was attributed to an electronic transition located at the tetraphenylethylene (TPE), whereas the band at 502 nm was associated with BODIPY absorption (Nar et al., 2019). The absorption spectrum was similar to their precursors, TPE and BODIPY, suggesting that there is not considerable ground-state interaction between the fluorophores through the o-carborane. Furthermore, the emission properties of the compound in mixtures of THF/water indicated that the emission intensity increased by the increasing upon adding water to THF due to the formation of aggregates, what suggested that BDP-oCB-19 exhibits AIE properties in THF/water solutions. BDP-oCB-19 did not show AIE properties in the mixture of solvents, but an aggregation-caused quenching (ACQ) was observed with a subsequent decrease in the emission. The observed ACQ effect was explained by structural considerations (vide infra). In one hand, methyl groups on the BODIPY unit suppress an intramolecular charge transfer (ICT) between BODIPY and TPE, due to the electron donating character of the methyl groups to the BODIPY moiety. On the other hand, crystal packing shows face-to-face π–π stacking of BODIPY units with a short distance of 3.97 Å between BODIPY–BODIPY mean planes for both compounds, which could explain the formation of aggregates that lead to ACQ behavior.
Carborane-BODIPY dyes have emerged as promising candidates for biological applications due to their unique photophysical properties and biocompatibility. These dyes combine the strong fluorescence and stability of BODIPY compounds with the advantageous features of carborane clusters, such as increased lipophilicity and enhanced cellular uptake. Recent studies have demonstrated their effectiveness as fluorescent markers in imaging techniques, enabling visualization of biological processes at the cellular level. Additionally, the incorporation of boron cluster structures can improve the dyes’ stability and resistance to photobleaching, making them suitable for long-term observations. As a result, carborane-BODIPY dyes are being explored for use in diagnostic tools, targeted drug delivery, and real-time tracking of biological events.
In 2015, M.G. Vicente et al. synthesized the BODIPY BDP-Me-oCB-20 by Suzuki coupling reaction between the diiodo BODIPY BDP-25 with 1.5 equiv. of o-carborane-containing boronic acid (29) (Hao et al., 2007), in the presence of Na2CO3 and Pd(PPh3)4 (Gibbs et al., 2015). The reaction afforded BDP-Me-oCB-20 in 89% yield after purification (Scheme 11). In parallel, the reaction of 29 with the corresponding 8-chloro-BODIPY BDP-26 under the same conditions led to the formation of BCP-Me-oCB-21 (Gibbs et al., 2015) and BCP-Me-oCB-22 (Xuan et al., 2016) in 47% and 45% yield, respectively (Scheme 11).
Scheme 11. Synthesis of BDP-Me-oCB-20, BDP-Me-oCB-21, BDP-Me-oCB-22. Reactions conditions: (i) Na2CO3, Pd(PPh3)4, toluene.
Later in 2018, R. Núñez, C. Prandi and coworkers synthesized a family of carborane-BODIPY dyes (Bellomo et al., 2018) by Heck cross-coupling reaction between the green emitting brominated BDP-27 (Gibbs et al., 2013) and styrene-substituted Me-o-carborane (30), Me-m-carborane (31) or Ph-m-carborane (32) (Ferrer-Ugalde et al., 2012), in the presence of Pd2 (dba)3/Pd(P(tBu)3)2 (1.2 and 1.6 mol%, respectively) as catalysts, and Cy2NMe as a base (Littke and Fu, 2001) in 1,4-dioxane at 100°C for 12 h. After an appropriate purification process, the formation of the expected carboraryl-BODIPY dyes BDP-Me-oCB-23, BDP-Me-mCB-24 and BDP-Ph-mCB-25 in moderate to good yields (46%, 51%, and 67%, respectively) (Scheme 12A) took place. In addition, the reaction of BDP-28 and 31 under the same conditions yielded BDP-Me-mCB-26 with a 55% yield (Scheme 12B).
Scheme 12. (A) Synthesis of BDP-Me-oCB-23, BDP-Me-mCB-24 and BDP-Ph-mCB-25. Reaction conditions: (i) [Pd2 (dba)3] (1.2 mol%), Pd [P (t-Bu)3]2 (1.6 mol%), Cy2NMe (1.4 eq.), dry 1,4-dioxane, 100°C, overnight. (B) Synthesis of BDP-Me-mCB-26. Reaction conditions: (i) [Pd2 (dba)3] (1.2 mol%), Pd [P (t-Bu)3]2 (1.6 mol%), Cy2NMe (1.4 eq.), dry 1,4-dioxane, 100°C, 12 h.
Later, in 2021, the same group synthesized a set of carborane-BODIPY conjugates (Bellomo et al., 2021). The Heck coupling reaction between the 2,6-diiodo BODIPY BDP-29 and two equivalents of 31 in refluxing 1,4-dioxane using the [Pd2 (dba)3] and [Pd (tBu3P)2] as catalysts, in the presence of Cy2NMe overnight at reflux, yielded BDP-Me-mCB-27 in 43% yield, along with its β,β-isomer (Scheme 13A). (Bellomo et al., 2021). The same conditions were successfully applied to the reaction of BDP-29 or BDP-30, which incorporate an amphiphile oligoethylene glycol alkyl chain at the meso-position, with the styrenyl substituted m-carborane derivative 32 to achieve symmetric dyes BDP-Ph-mCB-28 and BDP-Ph-mCB-29, in 57% and 52%, respectively (Scheme 13A).
Scheme 13. (A) Synthesis of carborane-BODIPY dyes BDP-Me-mCB-27, BDP-Ph-mCB-28 and BDP-Ph-mCB-29. Reaction conditions: (i) styrenyl-CB: 31 or 32 (1 eq.), [Pd2(dba)3] (3 mol%), Pd[P(t-Bu)3]2 (6 mol%), Cy2NMe (5 eq.), dry 1,4-dioxane, 100°C, 12 h dry 1,4-dioxane, 100°C, overnight. (B) Synthesis of carborane-BODIPY dyes BDP-Me-mCB-Ph-oCB-32 and BDP-Me-mCB-Ph-mCB-33. Reaction conditions: (i) 1) [Pd2(dba)3] (1.2 mol%), Pd[P(t-Bu)3]2 (1.6 mol%), Cy2NMe (1.4 eq.), dry 1,4-dioxane, 100°C, overnight, 2) N-iodosuccinimide (2 eq.), DCM, r.t, 12 h (ii) [Pd2(dba)3] (1.2 mol%), Pd[P(t-Bu)3]2 (1.6 mol%), Cy2NMe (1.4 eq.), dry 1,4-dioxane, 100°C, overnight. (C) Synthesis of BDP-Ph-mCB-34. Reaction conditions: (i) [Pd2(dba)3] (3 mol%), Pd[P(t-Bu)3]2 (6 mol%), Cy2NMe (4.8 eq.), dry 1,4-dioxane, 100°C, overnight.
Once the reaction was optimized, the procedure was extended to the synthesis of asymmetric compounds by attaching two different carboranyl units to the starting BODIPY. The mono-iodinated 1,3,5,7-tetramethyl BODIPY, BDP-31 was used as starting compound, that reacted in a first step with 31 in the presence of [Pd2 (dba)3], [Pd (tBu3P)2] and Cy2NMe (1.4 eq.) in refluxing dry 1,4-dioxane to give intermediate BDP-Me-mCB-30 in 72% (Scheme 13B). The reaction of BDP-Me-mCB-30 with N-iodosuccinimide at room temperature overnight leads to the formation of compounds BDP-Me-mCB-31, that after the reactions with 33 and 32 under Heck conditions gave asymmetric dyes BDP-Me-mCB-Ph-oCB-32 and BDP-Me-mCB-Ph-mCB-33 in 55% and 35%, respectively (Scheme 13B). Following similar conditions as before, BDP-Ph-mCB-34 was synthesized by the Heck-coupling reaction between BDP-32 and styrenyl-carborane 32 (Scheme 13C).
BODIPY dye BDP-33 reacted with carborane derivatives 34, 35 and 36 (Ferrer-Ugalde et al., 2017), under Sonogashira cross-coupling reaction conditions, by using Pd(PPh3)2Cl2 and CuI, in a solution of THF and triethylamine at reflux for 2 h (Scheme 14). After that time, the solvent was evaporated and the residue was dissolved in DCM and washed with saturated ammonium chloride. Finally, compounds were purified through a silica gel chromatographic column using hexane/acetone mixtures as eluent to give BDP-Me-oCB-35, BDP-Ph-oCB-36 and BDP-Ph-mCB-37 in 56, 81, 61% yield, respectively (Labra-Vázquez et al., 2020).
Scheme 14. Synthesis of BDP-Me-oCB-35, BDP-Ph-oCB-36 and BDP-Ph-mCB-37. Reaction conditions: (i) carboranyl benzyl iodide (1 mmol), Pd(PPh3)2Cl2 (0.05 mmol), CuI (0.1 mmol), THF, Et3N, 2h reflux.
Furthermore, the synthesis of red/NIR emitting carboranyl-aza-BODIPY derivatives was also performed by Bellomo et al. (Bellomo et al., 2018), following the Heck coupling reaction; however, due to the tendency of the aza-BODIPY to dechelate under the cross-coupling conditions (Parisotto et al., 2017; Bellier et al., 2011), this reaction was initiated using the brominated 3,3′,5,5′-tetraarylazadipyrromethene dye aza-DIPY that reacted with 30, 31 and 37 (Scheme 15), in the presence of Pd2 (dba)3/Pd(P(tBu)3)2, Cy2Me in 1,4-dioxane at reflux overnight to give the corresponding carboranyl-substituted aza-DIPY aza-DP-Me-oCB-1, aza-DP-Me-mCB-2 and aza-DP-Ph-oCB-3. The last step was the reaction with BF3·OEt2 using diisopropylethylamine (DIPEA) in DCM to give compounds aza-BDP-Me-oCB-38, aza-BDP-Me-mCB-39 and aza-BDP-Ph-oCB-40 in 93%, 88%, and 86% yields, respectively) (Scheme 15).
Scheme 15. Synthesis of carborane-aza-BODIPY dyes aza-BDP-Me-oCB-38, aza-BDP-Me-mCB-39 and aza-BDP-Ph-oCB-40. Reaction conditions: (i) [Pd2 (dba)3] (1.2 mol%), Pd [P (t-Bu)3]2 (1.6 mol%), Cy2NMe (1.4 eq.), dry 1,4-dioxane, 100°C, 12 h; (ii) BF3·OEt2, DIPEA, DCM.
All these carborane-BODIPY and aza-BODIPY derivatives exhibited characteristic absorption spectra of BODIPY dyes with strong absorption bands corresponding to the S0-S1 (π-π*) transitions. These bands are accompanied by a shoulder at slightly lower wavelength attributed to vibrational transitions. The maximum absorption (λmax) for these compounds ranged from 501 to 641 nm (Supplementary Table S5). For the same set of compounds, similar absorption spectra were obtained and no significant effect of the different carboranes were observed. Notably, BODIPY BDP-Me-oCB-34 (Scheme 13C) showed the largest red-shift (up to 140 nm) compared to the others BODIPYs, attributed to the substitution at the 3,5 or α positions, followed by those BODIPYs with 2,6-aryl substitution (Jiao et al., 2011). Those BODIPYs substituted with carboranes at the meso position exhibited a hypsochromic shift in the absorption maxima (Supplementary Table S6). The same trend was observed in the emission spectra with λem ranging from 508 nm for BDP-Me-oCB-21 (Scheme 11) to 641 nm for BDP-Me-oCB-34 (Scheme 13C). In the case of BDP-Me-mCB-26 (Scheme 12B), as expected, a significant red-shift was observed when compared with its homologous, 23, 24 and 25 (Scheme 12A), due to the conjugation of the thiophene group in 3 and 5 positions. The large ranges of absorption and emission is reflected in the different Stokes shifts observed from 7 to 65 nm (Supplementary Table S5). Regarding the fluorescence QYs, BDP-Me-oCB-20 and BDP-Me-mCB-21 (Scheme 11) exhibited the highest values of around 99% (Gibbs et al., 2015), which indicated that the o-carborane cluster not only did not quench the fluorescence but also enhanced the luminescence properties of the fluorophores. (Ferrer-Ugalde et al., 2012; Ferrer-Ugalde et al., 2014). On the contrary, the decrease of fluorescence QY observed in BDP-Me-oCB-22 (Scheme 11) was attributed to a higher rotational freedom of the 8-aryl group in the absence of methyl groups. BDP-Me-oCB-23, BDP-Me-mCB-24, and BDP-Ph-mCB-25 (Scheme 12A) showed good to moderate QYs, ranging from 0.40 to 0.44, which was attributed to the restricted rotation of the meso-aryl group (Karatay et al., 2015). However, the rest of BODIPYs exhibited low quantum efficiencies, specially BDP-Me-oCB-35, BDP-Ph-oCB-36 and BDP-Ph-mCB-37 (Scheme 14) with QYs ∼ 0.01. The solvatochromic analysis of BDP-Me-mCB-27, BDP-Ph-mCB-28 (Scheme 13A), BDP-Me-mCB-Ph-oCB-32 (Scheme 13B) and BDP-Ph-mCB-34 (Scheme 13C) indicated that a very minor solvent effect was observed both in absorption and emission maxima for the four compounds due to their low intrinsic molecular dipole moment. Aggregation studies in water for BDP-Ph-mCB-28 and BDP-Ph-mCB-29 (Scheme 13A) showed a similar photophysical behavior upon increasing amount of water. Up to 40% of water in THF, a hypochromic effect in the absorption spectra occurred, whereas similar emission spectra to those exhibited in pure THF were observed. In those THF:water mixtures (60/80 v/v), the absorption spectra showed large, red-shifted peaks suggesting the formation of aggregates, and the emission bands were weak most likely due to aggregation phenomena. A clear absorption band was observed in water indicating the formation of stable aggregates, making these BODIPYs excellent candidates as fluorogenic probes for bio-supramolecular assays, in which the fluorescence features might get restored upon binding or intercalation events with biological relevant molecules. For the aza-BODIPYs, both absorption and emission maxima were characteristics for these kind of dyes with maxima centered at 665 and 706 nm, respectively, exhibiting a noticeable bathochromic shift when compared to their parent tetraphenyl-BODIPYs and very low fluorescence quantum yields (Supplementary Table S5).
In 2015, M. G. Vicente reported the synthesis of a family of mercaptocarborane-BODIPY dyes as boron delivery agents for their potential application in BNCT (vide infra). The substitution reaction of the corresponding 8-chloro-BODIPY BDP-33 with 1-mercapto-o-carborane (38) in the presence of K2CO3 in THF to obtain BDP-oCB-41 in 94% yield (Scheme 16A). (Gibbs et al., 2015) Later, the same synthetic methodology was used to prepare the family of compounds BDP-oCB-42 - BDP-oCB-46, with yields ranging from 74% to 95% (Xuan et al., 2016). Following the same procedure and conditions, the reaction of the BDP-30 with 1-mercaptomethyl-p-carborane (39) led to BDP-pCB-47 in 92% yield. (Wang et al., 2014) (Scheme 16A). On the other hand, BDP-pCB-48 was prepared in 67% yield from BDP-34 via two successive regioselective reactions, a Stille cross-coupling at the 8-position using 1 equiv of tributylphenylstannane and Pd(PPh3)4, followed by substitution using an excess of 37 (Scheme 16B). (Zhao et al., 2015)
Scheme 16. (A) Synthesis of BDP-oCB-41, BDP-oCB-42, BDP-oCB-43, BDP-oCB-44, BDP-oCB-45, BDP-oCB-46 and BDP-pCB-47. Reaction conditions: (i) K2CO3, THF, r.t. (B) Synthesis of BCP-oCB-48.
Later in 2022, Ol’shevskaya and coworkers reported another set of mercaptocarborane-BODIPY conjugates for biological applications (vide infra). The reaction of BDP-35 that contain triazole linkers modified with maleimides, with the 9-mercapto-o-carborane (40) or 9-mercapto-m-carborane (41) (Plesek et al., 1978) takes place at the maleimide double bonds in the presence of NaOAc in THF, to produce thiosuccinimide compounds BDP-oCB-49, BDP-oCB-50, BDP-mCB-51 and BDP-mCB-52 in 40%–75% yields as red crystalline solids (Scheme 17A). (Zaitsev et al., 2022b)
Scheme 17. (A) Synthesis of BDP-oCB-49, BDP-oCB-50, BDP-mCB-51 and BDP-mCB-52. (B) Synthesis of BDP-oCB-53, BDP-oCB-54, BDP-mCB-55, BDP-mCB-56, BDP-monoCB-57 and BDP-monoCB-58.
The same authors have demonstrated that the thiolation reaction of pentafluorophenyl groups in BDP-36 (Alamiry et al., 2012) with mercaptocarboranes 40 or 41 in the presence of NaOAc in DMF for 1 h at r.t. produced the corresponding carborane-substituted BODIPYs BDP-oCB-53, BDP-oCB-54, BDP-mCB-55 and BDP-mCB-56 in 60%–68% yields (Scheme 17B). (Zaitsev et al., 2022a) Besides, two water soluble boronated BODIPYs BDP-monoCB-57 and BDP-monoCB-58 were also prepared in 72% and 83% yields respectively, by reaction of BDP-36 and the anionic 1-mercapto-1-carba-closo-dodecaborate (42) (Jelínek et al., 1986) using K2CO3 in anhydrous DMF at room temperature for 8 h (Scheme 17B).
Following a similar procedure with slight modifications, A. Ol’shevskaya and coworkers have recently prepared a new family of carborane-BODIPY derivatives (Zaitsev et al., 2023). The reaction of BDP-37 (Sekhar et al., 2014) with mercaptocarboranes 40 or 41 in the presence of NaOAc in boiling CH3CN under argon for 2 h afforded BDP-oCB-59, BDP-mCB-60 in 85% and 92% yields, respectively (Scheme 18). A similar reaction of BDP-37 with water-soluble 42 in the presence of K2CO3 in CH3CN at reflux for 4 h led to the formation of BDP-monoCB-61 in 70% yield (Scheme 18). It is important to note that these reaction conditions did not produce p-fluorine substitution, so it is necessary to modify the conditions to introduce the carborane clusters at the pentafluorophenyl group in the new BODIPYs. The reaction of BDP-oCB-59 or BDP-mCB-60 with the corresponding mercapta-carboranes in the presence of NaOAc in DMF at room temperature for 1 h gave BDP-oCB-62 and BDP-mCB-63 in 80% and 75% yields, respectively. In the same way, compound BDP-monoCB-64 was obtained in 71% yield by reaction of BDP-monoCB-61 with 42 and K2CO3 in DMF at room temperature for 4 h (Scheme 18).
Scheme 18. Synthesis of BDP-oCB-62, BDP-mCB-63 and BDP-monoCB-64. Reaction conditions (i) NaOAc, CH3CN, reflux 2h; (ii) K2CO3, CH3CN, reflux 4h; (iii) NaOAc, DMF, r.t 1 h; (iv) K2CO3, DMF, r.t. 4 h.
Dyes BDP-oCB-41–48 (Scheme 16) displayed characteristic absorption spectra of BODIPYs with maxima in the range 521–582 nm (Supplementary Table S6). The introduction of the o-carboranylthio group at the meso (8) position (Wang et al., 2014) caused a significant red-shift (up to 57 nm) in the maximum absorption compared to analogs BDP-oCB-21 and BDP-oCB-22 (Scheme 11). Regarding emission properties, these BODIPYs exhibited emission maxima ranging from 540 to 609 nm. As observed in their absorption properties, BODIPYs BDP-oCB-45 and BDP-oCB-46 (Scheme 16A) showed a significant red-shift in emission (up to 61 nm) compared to the others BODIPYs (Supplementary Table S6). This red shift is likely due to the stabilization of the LUMO by the o-carboranylthio group, which decreases the HOMO–LUMO gap. It is well known that the replacement of the meso-chloro by meso-thiol produces an important decrease in the fluorescence. While BDP-oCB-41 and BDP-oCB-44 to BDP-oCB-47 are poorly emissive, (Wang et al., 2014), BDP-oCB-42 showed higher fluorescence quantum yield, suggesting limited rotational freedom for the 8-carboranylthio group (Supplementary Table S6). The yields decrease with increasing alkyl substitution in BDP-oCB-43 to BDP-oCB-45, due to increased energy loss to nonradiative deactivation processes.
The absorption spectra of BDP-oCB-53, BDP-oCB-54, BDP-mCB-55, BDP-mCB-56, BDP-monoCB-57 and BDP-monoCB-58 (Scheme 17B) in EtOH exhibited very similar characteristics. The absorption maxima for these compounds were in the range 513–515 nm with a noticeable shoulder in the region 480–490 nm. In the same solvent, these compounds displayed fluorescence spectra with emission maxima in the range 528–538 nm. They also exhibited very high fluorescence QYs ranging from 62% to 100% (Supplementary Table S7). The results indicated that methyl-substituted BODIPY derivatives exhibited higher fluorescence efficiency compare to un-substituted derivatives. This is attributed to the restricted rotation of the meso-tetrafluorophenyl moiety around the BODIPY core in the methyl-substituted BODIPYs, which decreases the internal conversion rate. In contrast, the meso-tetrafluorophenyl group in unsubstituted derivatives rotates more freely, leading to increased non-radiative decay processes. Additionally, the presence of the carborane clusters did not affect the non-radiative decay pathway.
Compounds BDP-oCB-59, BDP-mCB-60 and BDP-monoCB-61 (Scheme 18), where the carboranyl substitution was at 3 and 6 positions of the BODIPY core instead of the pentafluorophenyl group, showed a red-shift of the absorption maxima in acetone solutions compared to previous dyes (Supplementary Table S7). BDP-oCB-59 and BDP-mCB-60 showed absorption maxima at 596 and 593 nm respectively, whereas dianionic BDP-monoCB-61 displayed a maximum at 607 nm. Similar absorption maxima are observed for BDP-oCB-62, BDP-mCB-63, and BDP-monoCB-64 (Scheme 18), with molar extinction coefficients ranging from 75,500 to 85,600 M−1cm−1. All compounds in acetone showed similar emission spectra, with maxima in the range 612–632 nm, red-shifted compared to previous dyes, where the carborane cluster is attached to the pentafluophenyl group. Notably, BDP-monoCB-61 and BDP-monoCB-64 exhibited the longest emission maxima at 625 and 632 nm respectively. This suggests that anionic mono-carborane clusters cause a more significant bathochromic shift. Additionally, these compounds have lower fluorescence QYs of 89% and 91% (64% in water) compared to the neutral derivatives. Notably, linking carborane clusters through sulfur to the BODIPY core results in a red-shift in both absorption and emission spectra compared to compounds where the carborane is linked to the pentafluorophenyl group. In addition, the anionic monocarborane clusters produce larger red-shift than the neutral carborane, and lower quantum efficiencies.
The spectral properties of BODIPY dyes can be easily modulated due to the manipulability of their structural backbone. Historically, modifications at the 3,5- and 2,6-positions of BODIPY-based probes (Chart 1) have resulted in red-shifted emission wavelengths and enhanced water solubility (Loudet and Burgess, 2007). Building on these findings, recent research into meso-substituents (i.e., 8-position substituents) has significantly impacted spectral properties, including fluorescence wavelengths, quantum yields, and Stokes shifts (Yan et al., 2022). For example, the relative rotation of the meso-aryl groups to the BODIPY core plane can greatly affect the excited-state dynamics of the dyes. Thus, an increase of QY is usually observed when such rotation is restricted (Kee et al., 2005). The substituents nature introduced into the various positions of the BODIPYs are very diverse and have been previously reviewed. In this review section We have only considered the reported X-ray crystal structures of carborane-functionalized BODIPYs designed for biological applications. We have compared the molecular structures and conformations within each family of compounds and related them to the photophysical properties.
Structural analysis of all carboranylthio-meso-BODIPYs (Figure 1A) confirmed a restricted rotation through the C-S bond of the carboranylthio groups relative to the BODIPY rings (Supplementary Table S8). Torsion angles between 86° and 100° indicate that the carboranylthio groups are essentially perpendicular to the BODIPY planes. The presence of methyl groups at positions 1 and/or 7 of the BODIPY rings (Chart 1A; Figure 1A) appears to hinder this rotation. As previously discussed QYs increase with increased alkyl substitution on the BODIPY ring due to the reduced rotational freedom of the carboranylthio group. Nevertheless, the introduction of the meso-thiol provokes an important decrease of the fluorescence efficiency (Supplementary Table S8).
Figure 1. (A) Molecular structures for meso-substituted carboranylthio BODIPYs (Gibbs et al., 2015; Xuan et al., 2016; Wang et al., 2014). Color code: B, pink; C, grey; H, white; S, yellow; N, blue; and F, green. (B) Molecular structures for 3/5-substituted carboranylthio BODIPYs (Zaitsev et al., 2023; Zhao et al., 2015). Color code: B, pink; C, grey; H, white; S, yellow; N, blue; and F, green.
In contrast to the carboranylthio-meso-BODIPYs, the molecular structure of the corresponding derivatives with substitution at positions 3- and/or 5- of the BODIPY moiety reveals that the carboranylthio groups can freely rotate relative to the BODIPY plane (Figure 1B; Supplementary Table S9). The rotation angles in these structures range from 9° (nearly coplanar) to 90° (perpendicular). It is noticeable that the substitution of the thiolcarborane at these positions produces a significant increase of the QYs, that even reach 100% (Supplementary Table S9).
The introduction of carboranyl moieties at the aryl-meso fragment does not appear to significantly affect the BODIPY structures (Figure 2A). The angles between the aryl-meso and BODIPY planes range from 50° to 89°, indicating limited rotation (Supplementary Table S10). As anticipated, a more perpendicular orientation is observed when the carborane moieties are bulkier and closer to the aryl-meso fragment. Generally, the quantum yields for this family of BODIPYs are relatively low, with only slight increases observed when carboranes are introduced (BDP-ref vs. BDP-Me-oCB-35 and BDP-Ph-oCB-36).
Figure 2. (A) Molecular structures for long carboranyl-aryl-meso-BODIPYs (Labra-Vázquez et al., 2020; Shlyakhtina et al., 2015). Color code: B, pink; C, grey; H, white; S, yellow; N, blue; and F, green. (B) Crystal packing views for BDP-Ph-oCB-36 (left) and BDP-Ph-mCB-37 (right) showing the disordered n-hexane in deep blue color. Other color codes: B, pink; C, grey; and F, green. H atoms are omitted for clarity.
The supramolecular structures of the BODIPY-carboranyl dyads BDP-Ph-oCB-36 and BDP-Ph-mCB-37 (Figure 2A) revealed intriguing arrangements within the crystal lattice (Labra-Vázquez et al., 2020). In the case of BDP-Ph-oCB-36, the solvent molecules (n-hexane) were confined within supramolecular pores formed by an antiparallel head-to-tail arrangement of pairs of fluorophores, extending along the crystallographic a axis (Figure 2B). This arrangement created distinct channels (5.2% of the unit cell) where the solvent molecules were encapsulated. On the other hand, for BDP-Ph-mCB-37, the n-hexane molecules were closely surrounded by the fluorophore molecules, indicating a more intimate interaction between the solvent and the fluorophores (Figure 2B). Unlike in the ortho-isomer, where the solvent molecules moved freely through supramolecular channels, in the meta-isomer, the solvent molecules were densely trapped within the crystal lattice. This difference in supramolecular organization suggests varying degrees of solvent-fluorophore interactions and highlights the influence of molecular structure on the overall packing arrangement within the crystals. Such compounds are, therefore, crystalline inclusion compounds. The awkward shape of the BODIPY-carboranyl dyads prevents efficient packing in the solid state, leading to the entrapment of molecular guests in their crystal lattice (Tsang et al., 2017).
R. Núñez and coworkers (Chaari et al., 2018a) reported a series of anionic boron cluster-BODIPY conjugated bearing closo-dodecaborate (BDP-DCB-1), cobaltabisdicarbollie (BDP-CoMCB-1) and ferrabisdicarbollide (BDP-FeMCB-1). These compounds exhibited excellent biological properties as fluorescent markers in cells and potential applications in BNCT (vide infra). They were synthesized by nucleophilic oxonium ring-opening reaction of [Bu4N][B12H11(C4H8O2)] (43), [3,3′-Co(8-C4H8O2-1,2-C2B9H10)(1′,2′-C2B9H11)] (44) and [3,3′-Fe (8-C4H8O2-1,2-C2B9H10)(1′,2′-C2B9H11)] (45) (Scheme 19). The first step was the deprotonation of the phenol group in BDP-38 with K2CO3 in CH3CN followed by the nucleophilic attack to the oxonium ring. BODIPYs BDP-DCB-1, BDP-CoMCB-1 and BDP-FeMCB-1 were precipitated as [NBu4]+ or [NMe4]+salts in good yields, ranged from 68% to 88%. The corresponding sodium salts were obtained by cation exchange by using cation-exchange resin.
Absorption and emission properties were analyzed in THF solutions and for BDP-DCB-2 also in water at room temperature. The UV-vis spectra for all of them showed a characteristic absorption maxima at around 497 nm (Supplementary Table S11), corresponding to the S0-S1 (π-π*) transitions in the BODIPY core. A shoulder at higher energy attributed to vibrational transitions was also present. The emission maxima were in the range from 504 to 520 nm due to the BODIPY with low QYs (3%–6%) and Stokes shift values from 7 to 23 nm. The link of these anionic boron clusters to the BODIPY did not significantly affect the absorption and emission properties of the BODIPY dye except for a slight decrease in the quantum efficiency.
BNCT is a promise cancer therapy for the treatment of high-grade brain tumors, such as glioblastoma multiforme (GBM). For this purpose, one of the main challenges for the application of BNCT in brain tumors arises from the existence of the blood–brain barrier (BBB) that prevents most drugs from penetrating into the brain and from reaching the targeted tumor cells. Carborane-BODIPY derivatives were designed as potential boron delivery agents for BNCT. For this purpose, M.G. Vicente et al. (Gibbs et al., 2015) studied the BBB permeability of BDP-Me-oCB-20 (Pe = 0.05 × 10−6 cm/s) and BDP-Me-oCB-21 (Pe = 0.13 × 10−6 cm/s) in human brain endothelial cell line hCMEC/D3 to demonstrated that these BODIPYs were poorly internalized probably due to their high hydrophobicity (log P of 1.41 and 1.35, respectively).
The BODIPY derivatives BDP-oCB-41 to BDP-oCB-48 were also designed for potential use for BNCT. Examination of their BBB permeability in the human brain endothelial cell line hCMEC/D3 showed that they were effectively internalized. BDP-oCB-41 showed higher BBB permeability (Pe = 39 × 10−6 cm/s) than Lucifer yellow (Pe = 25.23 × 10−6), probably as a result of its lower molecular weight and lower hydrophobic character (log p = 0.85) (Supplementary Table S12). These BODIPYs showed low dark toxicity and phototoxicity (IC50 > 80 μM) in human glioma T98G cells, with the exception of BDP-oCB-45 (IC50 = 40 μM), probably as a result of its remarkably high uptake into cells. On the other hand, BDP-oCB-42 showed the largest permeability (Pe = 164 × 10−6 cm/s) across the BBB model consisting of hCMEC/D3 cells. Results indicated that those BODIPYs with MW < 400 Da and log p < 1.7 are the most efficient at crossing the BBB model. The most hydrophobic compound BDP-oCB-48 (log p = 2.70) was poorly soluble in aqueous solutions, showed very low uptake into T98G cells, as it was precipitated in buffer precluded determination of its BBB permeability. In general, BODIPYs tested showed higher BBB permeability compared with Lucifer yellow and lower dark cytoxicity, suggesting that they may be effective boron delivery agents for BNCT of brain tumors. Among this series, BDP-oCB-42 and BDP-oCB-43 showed the highest BBB permeability, while BDP-oCB-45 and BDP-oCB-46 were more accumulated within tumor cells; therefore, these are the most promising BNCT agents.
Compounds BDP-oCB-53, BDP-mCB-54, BDP-oCB-55, BDP-mCB-26, BDP-monoCB-57 and BDP-monoCB-58 exhibited single oxygen (1O2) production upon irradiation, being BDP-oCB-53 the one with the highest ability to generate 1O2. Studies reported by A. Ol’shevskaya and coworkers indicated that non-methylated derivatives showed an increased non-radiative deactivation due to enhanced rotational freedom of meso-tetrafluorophenyl group. These compounds have lower fluorescence efficiency whereas the rate of intersystem crossing is increased. None of the compounds showed cytotoxic in HCT116 colon adenocarcinoma cells in the dark, whereas they potently induced lipid peroxidation and rapid (within minutes) cell death upon photoactivation. Compounds BDP-oCB-53, BDP-monoCB-57 and BDP-monoCB-58 formed stable complexes with Bovine Serum Albumin (BSA) as it has been demonstrated by the binding constants (Kb) that range from 1.1 to 3.5 × 10−5 M−1, property that can help the delivery of these dyes in the body. Compound BDP-oCB-53 was localized in lysosomes and partially in mitochondria of HCT116 colon adenocarcinoma cells, whereas BDP-monoCB-58 was visualized in cytoplasm, membrane and mitochondria, but not in lysosomes after incubation for 24 h. Data on lipid peroxidation in cells incubated with BDP-oCB-53 or BDP-monoCB-58 clearly demonstrated that the increased fluorescence of the oxidized form upon light excitation indicates massive lipid peroxidation that precedes the plasma membrane damage. The peroxidation of membrane lipids was higher for BDP-monoCB-58 than for BDP-oCB-53 and BDP-monoCB-58 was a more potent inducer of necrosis than BDP-oCB-53. Carboranyl-BODIPY conjugates represent a new chemotype perspective for binary therapeutic modalities.
Careborane-BODIPYs BDP-Me-oCB-23 to BDP-Me-mCB-26 and carboranes-aza-BODIPY aza-BDP-Me-mCB-38, aza-BDP-Me-mCB-39 and aza-BDP-Ph-mCB-40 were studied as potential fluorescence probes for biological systems (Bellomo et al., 2018). All of them, except aza-BDP-Me-oCB-38, were detected by confocal microscopy indicating that they were internalized and accumulated in the cytoplasm (Figure 3A), suggesting that Me-o-carborane caused a negative effect on the permeability of the BODIPY. BDP-Me-oCB-23 to BDP-Me-mCB-26 were in the range of green fluorescence, aza-BDP-Me-mCB-39 and aza-BDP-Ph-mCB-40 were observed in the range of far-red (NIR region, which is outside the visible spectra of human eye), whereas compound BDP-Me-mCB-26 showed a wide emission range, from red to far-red of the spectral region. Compounds BDP-Me-o-CB-23, BDP-Ph-mCB-25 and BDP-Me-mCB-26 showed exceptional fluorescence characteristics for cell imaging purposes. BDP-Me-oCB-23, BDP-Ph-mCB-25 (and BDP-Me-oCB-26 when detected in the visible red wavelength) would be the first choice when a simple or double labelling is necessary. In addition, compound BDP-Me-oCB-26 with NIR emission, could be very useful to avoid overlapping emission, mainly when multiple fluorochromes are used in the same sample. Compounds BDP-Me-oCB-35, BDP-Ph-oCB-36 and BDP-Ph-mCB-37 showed different behavior in HeLa cells internalization and subcellular distribution that were related to their unlike static dipole moments and partition coefficients (Labra-Vázquez et al., 2020). The Ph-m-carborane derivative BDP-Ph-mCB-37 showed the highest lipophilicity with a log p = 0.677 being the best internalized and localized in the plasma membrane (Figure 3B). This evidence provides a molecular design strategy for improving the prospective applications of BODIPY–carboranyl dyads as potential fluorescence imaging agents and boron carriers for BNCT.
Figure 3. (A) Orthogonal projections of HeLa cells incubated with compounds aza-BDP-Me-oCB-39 (grey), aza-BDP-Ph-oCB-40 (grey) and BDP-Me-mCB-24 (green) (scale bar: 50 µm). (B) Confocal images of 10 μM BDP-mPh-CB-37 in live HeLa cells after 30 min incubation. Panels from left to right show the confocal green and red channel, merged and bright channels, respectively (scale bars:13 μm. (C) Cellular uptake of BDP-DCB-1, BDP-CoMCB-1 and BDP-FeMCB-1 after 2 h of incubation by confocal microscopy (scale bar: 50 μm).
Cellular uptake of BODIPYs BDP-DCB-1, BODB-DCB-2, BDP-CoMCB-1, BDP-CoMCB’ and BDP-FeMCB-1 in HeLa cells at different concentration confirmed the excellent internalization of them by cells, especially for those containing the cobaltabisdicarbollide, showing a notable cytoplasmic staining (Figure 3C). The cellular uptake was analyzed by flow cytometry and confocal microscopy, and both techniques indicated better internalization of the target boron cluster-BODIPY conjugates compared to the starting BDP-38. These results suggested that linking the boron cluster clearly produces a synergistic effect, aiding the BODIPY in crossing the cell membrane. Noticeably, these dyes, especially compounds BDP-CoMCB-1 and BDP-CoMCB-2, could be considered excellent candidates as theranostic agents for fluorescence cell imaging (diagnosis) and boron delivery systems for BNCT.
In the last decade, using electricity as a redox agent has made it possible to conduct chemical reactions efficiently and environmentally friendly. Therefore, electrocatalysis has become a useful tool for the regioselective activation of CH. However, there are a few examples of B-H functionalization.
In a groundbreaking development in 2021, Ackermann and his coworkers unveiled a novel strategy for the electrochemical regioselective cage B-H nitrogenation of nido-carborane 45 in a dehydrogenative manner, assembling a variety of N-heterocycles compounds, between them the BODIPY-labeled-pyridine 46 and 47 to obtain N-substituted nido-Carborane such as BDP-nidoCB-1 and BDP-nidoCB-2 (Scheme 20). (Yang et al., 2021) The electrochemical-catalyzed B-N reactions were carried out at room temperature in a simple undivided cell setup equipped with a GF (Graphite Felt) anode and a Pt-plate cathode. Two equivalents of NMe4Cl were used as an additive, and a mixture of 1,2-dimethoxyethane (DME)/H2O served as a solvent mixture.
The optical properties of BDP-nidoCB-1 and BDP-nidoCB-2 were studied by absorption and emission spectroscopy in five different solvents (Supplementary Table S13). Both compounds exhibited intense absorption. BDP-nidoCB-1 presented an absorption maxima band between 507–513 nm, while BDP-nidoCB-2 was between 570–582 nm. Both bands can be attributed to the S1←S0 (π-π*) transition of the BODIPY core. Additionally, both compounds displayed high Stokes shifts and molar extinction coefficients.
In conclusion, the integration of carborane structures into BODIPY dyes has led to significant advancements in the fields of photonics and materials science. The unique properties of carboranes, including their exceptional thermal stability, chemical robustness, and ability to enhance photophysical characteristics, have been effectively harnessed to create novel dye systems with superior performance. The studies presented herein highlight the efficient electronic energy transfer (EET) mechanisms facilitated by varying linker lengths, underscoring the delicate balance between structural design and functional efficiency. Notably, the findings indicate that while shorter linkers promote higher EET efficiencies, the introduction of longer spacers can lead to a decrease in energy transfer rates, thereby providing critical insights for future design strategies.
Looking ahead, the potential applications of carborane-based BODIPY dyes are vast and varied. Their enhanced photostability and tunable emission properties position them as promising candidates for use in advanced optoelectronic devices, including organic light-emitting diodes (OLEDs), solar cells, and sensors. Furthermore, the biocompatibility and selective targeting capabilities of these dyes incorporation carboranes open new avenues for their application in bioimaging and therapeutic modalities, particularly in the realm of cancer treatment through BNCT.
However, it is important to note that studies of the photophysical properties of carborane-BODIPY dyes in the solid state have been scarcely explored. This area presents a significant opportunity for future research, as understanding the behavior of these dyes in solid matrices could lead to the development of more efficient materials for various applications. Moreover, the ability of certain carborane-BODIPY systems to exhibit AIE could enhance their utility in responsive materials and solid-state luminescent devices, further broadening their application spectrum. Additionally, the potential of carborane-BODIPY dyes in antimicrobial and anticancer photodynamic therapy (PDT) remains largely untapped. Investigating their efficacy in these domains could yield valuable insights and innovative therapeutic strategies.
Future research endeavors should focus on the development of new synthetic methodologies to streamline the production of carborane-BODIPY derivatives, as well as the exploration of their mechanisms of action at the molecular level. Investigating the interactions between these dyes and biological systems will be crucial for optimizing their performance in medical applications. Additionally, the incorporation of other functional groups and materials could lead to the discovery of hybrid systems that leverage the strengths of both organic and inorganic components.
In summary, the ongoing exploration of carborane-based BODIPY dyes holds great promise for unlocking innovative applications across multiple fields. As research progresses, we anticipate that these compounds will not only enhance our understanding of photophysical processes but also contribute to the development of next-generation technologies that harness light in novel and impactful ways. The journey of carborane-BODIPY research is just beginning, and we are excited to witness the unfolding of its potential in the coming years.
JO-H: Conceptualization, Formal Analysis, Resources, Validation, Visualization, Writing–original draft, Writing–review and editing. JP: Conceptualization, Data curation, Validation, Writing–original draft, Writing–review and editing. RN: Conceptualization, Funding acquisition, Investigation, Methodology, Resources, Supervision, Validation, Writing–original draft, Writing–review and editing.
The author(s) declare that financial support was received for the research, authorship, and/or publication of this article. This work was financially supported by MCIU (PID 2022-136892NB-I00) and Generalitat de Catalunya (AGAUR 2021-SGR-00442) projects. Authors acknowledge financial support from the State Investigation Agency, through the Severo Ochoa Programme for Centres of Excellence (CEX 2023-001263-S). JO-H and RN want to thanks European Union´s Horizon 2022 for the Marie Sklodowska-Curie Action (MSCA) Post-doctoral Fellowship No. 101109856.
The authors declare that the research was conducted in the absence of any commercial or financial relationships that could be construed as a potential conflict of interest.
All claims expressed in this article are solely those of the authors and do not necessarily represent those of their affiliated organizations, or those of the publisher, the editors and the reviewers. Any product that may be evaluated in this article, or claim that may be made by its manufacturer, is not guaranteed or endorsed by the publisher.
The Supplementary Material for this article can be found online at: https://www.frontiersin.org/articles/10.3389/fchem.2024.1485301/full#supplementary-material
Alamiry, M. A. H., Benniston, A. C., Hagon, J., Winstanley, T. P. L., Lemmetyinen, H., and Tkachenko, N. V. (2012). The fluorine effect: photophysical properties of borondipyrromethene (Bodipy) dyes appended at the meso position with fluorinated aryl groups. RSC Adv. 2, 4944–4950. doi:10.1039/c2ra20219k
Avellanal-Zaballa, E., Gartzia-Rivero, L., Arbeloa, T., and Bañuelos, J. (2022). Fundamental photophysical concepts and key structural factors for the design of BODIPY-based tunable lasers. Int. Rev. Phys. Chem. 41, 177–203. doi:10.1080/0144235X.2022.2096772
Bellier, Q., Pégaz, S., Aronica, C., Guennic, B.Le, Andraud, C., and Maury, O. (2011). Near-infrared nitrofluorene substituted aza-Boron-dipyrromethenes dyes. Org. Lett. 13, 22–25. doi:10.1021/ol102701v
Bellomo, C., Chaari, M., Cabrera-González, J., Blangetti, M., Lombardi, C., Deagostino, A., et al. (2018). Carborane-BODIPY dyads: new photoluminescent materials through an efficient heck coupling. Chem. - A Eur. J. 24, 15622–15630. doi:10.1002/chem.201802901
Bellomo, C., Zanetti, D., Cardano, F., Sinha, S., Chaari, M., Fin, A., et al. (2021). Red light-emitting Carborane-BODIPY dyes: synthesis and properties of visible-light tuned fluorophores with enhanced boron content. Dye. Pigment. 194, 109644. doi:10.1016/j.dyepig.2021.109644
Berksun, E., Nar, I., Atsay, A., Özçeşmeci, I., Gelir, A., and Hamuryudan, E. (2018). Synthesis and photophysical properties of a porphyrin-BODIPY dyad and a porphyrin-: O -carborane-BODIPY triad. Inorg. Chem. Front. 5, 200–207. doi:10.1039/c7qi00608j
Bhosle, A. A., Banerjee, M., and Chatterjee, A. (2024). Aggregation-induced emission-active azines for chemosensing applications: a five-year update. Sensors Diagnostics 3, 745–782. doi:10.1039/d3sd00348e
Bumagina, N. A., and Antina, E. V. (2024). Review of advances in development of fluorescent BODIPY probes (chemosensors and chemodosimeters) for cation recognition. Coord. Chem. Rev. 505, 215688. doi:10.1016/j.ccr.2024.215688
Burghart, A., Kim, H., Welch, M. B., Thoresen, L. H., Reibenspies, J., and Burgess, K. (1999). Dyes: synthesis, spectroscopic, electrochemical, and structural properties, 7813–7819.
Cabrera-Gonzalez, J., Bhattacharyya, S., Milian-Medina, B., Teixidor, F., Farfan, N., Arcos-Ramos, R., et al. (2017). Tetrakis{[(p-dodecacarboranyl)methyl]stilbenyl}ethylene: a Luminescent Tetraphenylethylene (TPE) Core System. Eur. J. Inorg. Chem. 2017, 4575–4580. doi:10.1002/ejic.201700453
Cabrera-González, J., Chaari, M., Teixidor, F., Viñas, C., and Núñez, R. (2020). Blue emitting star-shaped and octasilsesquioxane-based polyanions bearing boron clusters. Photophysical and thermal properties. Molecules 25, 1210. doi:10.3390/molecules25051210
Cabrera-Gonzalez, J., Vinas, C., Haukka, M., Bhattacharyya, S., Gierschner, J., and Nunez, R. (2016). Photoluminescence in carborane-stilbene triads: a structural, spectroscopic, and computational study. Chem. Eur. J. 22, 13588–13598. doi:10.1002/chem.201601177
Chaari, M., Gaztelumendi, N., Cabrera-González, J., Peixoto-Moledo, P., Viñas, C., Xochitiotzi-Flores, E., et al. (2018a). Fluorescent BODIPY-anionic boron cluster conjugates as potential agents for cell tracking. Bioconjug. Chem. 29, 1763–1773. doi:10.1021/acs.bioconjchem.8b00204
Chaari, M., Kelemen, Z., Choquesillo-Lazarte, D., Gaztelumendi, N., Teixidor, F., Viñas, C., et al. (2019). Efficient blue light emitting materials based on: M -carborane-anthracene dyads. Structure, photophysics and bioimaging studies. Biomater. Sci. 7, 5324–5337. doi:10.1039/c9bm00903e
Chaari, M., Kelemen, Z., Choquesillo-Lazarte, D., Teixidor, F., Viñas, C., and Núñez, R. (2020). Anthracene–styrene-substituted m-carborane derivatives: insights into the electronic and structural effects of substituents on photoluminescence. Inorg. Chem. Front. 7, 2370–2380. doi:10.1039/D0QI00127A
Chaari, M., Kelemen, Z., Planas, J. G., Teixidor, F., Choquesillo-Lazarte, D., Ben Salah, A., et al. (2018b). Photoluminescence in: M -carborane-anthracene triads: a combined experimental and computational study. J. Mater. Chem. C 6, 11336–11347. doi:10.1039/c8tc03741h
Claytor, K., Khatua, S., Guerrero, J. M., Tcherniak, A., Tour, J. M., and Link, S. (2009). Accurately determining single molecule trajectories of molecular motion on surfaces. J. Chem. Phys. 130, 164710. doi:10.1063/1.3118982
Das, S., Dey, S., Patra, S., Bera, A., Ghosh, T., Prasad, B., et al. (2023). BODIPY-based molecules for biomedical applications. Biomolecules 13, 1723. doi:10.3390/biom13121723
Feng, F., Wang, P., Li, Y., and Bao, X. (2023). Versatile π-bridges in nonfullerene electron acceptors of organic solar cells. Mater. Chem. Front. 7, 3855–3878. doi:10.1039/d3qm00321c
Ferrer-Ugalde, A., Cabrera-González, J., Juárez-Pérez, E. J., Teixidor, F., Pérez-Inestrosa, E., Montenegro, J. M., et al. (2017). Carborane-stilbene dyads: the influence of substituents and cluster isomers on photoluminescence properties. Dalt. Trans. 46, 2091–2104. doi:10.1039/C6DT04003A
Ferrer-Ugalde, A., González-Campo, A., Viñas, C., Rodríguez-Romero, J., Santillan, R., Farfán, N., et al. (2014). Fluorescence of new o-carborane compounds with different fluorophores: can it be tuned? Chem. - A Eur. J. 20, 9940–9951. doi:10.1002/chem.201402396
Ferrer-Ugalde, A., Juárez-Pérez, E. J., Teixidor, F., Viñas, C., Sillanpää, R., Pérez-Inestrosa, E., et al. (2012). Synthesis and characterization of new fluorescent styrene-containing carborane derivatives: the singular quenching role of a phenyl substituent. Chem. - A Eur. J. 18, 544–553. doi:10.1002/chem.201101881
Fisher, S. P., Tomich, A. W., Lovera, S. O., Kleinsasser, J. F., Guo, J., Asay, M. J., et al. (2019). Nonclassical applications of closo-carborane anions: from main group chemistry and catalysis to energy storage. Chem. Rev. 119, 8262–8290. doi:10.1021/acs.chemrev.8b00551
Gibbs, J. H., Robins, L. T., Zhou, Z., Bobadova-Parvanova, P., Cottam, M., McCandless, G. T., et al. (2013). Spectroscopic, computational modeling and cytotoxicity of a series of meso-phenyl and meso-thienyl-BODIPYs. Bioorg. Med. Chem. 21, 5770–5781. doi:10.1016/j.bmc.2013.07.017
Gibbs, J. H., Wang, H., Bhupathiraju, N. V. S. D. K., Fronczek, F. R., Smith, K. M., and Vicente, M. G. H. (2015). Synthesis and properties of a series of carboranyl-BODIPYs. J. Organomet. Chem. 798, 209–213. doi:10.1016/j.jorganchem.2015.05.009
Godoy, J., Vives, G., and Tour, J. M. (2010). Synthesis of highly fluorescent BODIPY-based nanocars. Org. Lett. 12, 1464–1467. doi:10.1021/ol100108r
Grimes, R. N. (2016). Carboranes. 3rd Edn. Available at: https://shop.elsevier.com/books/carboranes/grimes/978-0-12-801894-1.
Hablot, D., Sutter, A., Retailleau, P., and Ziessel, R. (2012). Unsymmetrical p-carborane backbone as a linker for donor-acceptor dyads. Chem. - A Eur. J. 18, 1890–1895. doi:10.1002/chem.201103307
Hablot, D., Ziessel, R., Alamiry, M. A. H., Bahraidah, E., and Harriman, A. (2013). Nanomechanical properties of molecular-scale bridges as visualised by intramolecular electronic energy transfer. Chem. Sci. 4, 444–453. doi:10.1039/c2sc21505e
Hao, E., Fabre, B., Fronczek, F. R., and Vicente, M. G. H. (2007). Syntheses and electropolymerization of carboranyl-functionalized pyrroles and thiophenes. Chem. Mater. 19, 6195–6205. doi:10.1021/cm701935n
Harriman, A., Alamiry, M. A. H., Hagon, J. P., Hablot, D., and Ziessel, R. (2013). Through-space electronic energy transfer across proximal molecular dyads. Angew. Chem. - Int. Ed. 52, 6611–6615. doi:10.1002/anie.201302081
Housecroft, C. E. (2011). Boron: metallacarbaboranes. New York: Encyclopedia of Inorganic and Bioinorganic Chemistry.
Imahori, H., and Akiyama, M. (2024). Porphyrins as key components for photoinduced charge separation, solar cells, and optogenetics. J. Porphyr. Phthalocyanines 1088424624 28, 319–337. doi:10.1142/S1088424624300015
Issa, F., Kassiou, M., and Rendina, L. M. (2011). Boron in drug discovery: carboranes as unique pharmacophores in biologically active compounds. Chem. Rev. 111, 5701–5722. doi:10.1021/cr2000866
Jelínek, T., Plesek, J., Hermanek, S. S. B., and Štíbr, B. (1986). Chemistry of compounds with the 1-carba-closo-dodecaborane(12) framework. Collect Czech Chem. Commun. 51, 819–829. doi:10.1135/cccc19860819
Jenekhe, S. A., and Osaheni, J. A. (1994). Excimers and exciplexes of conjugated polymers. Sci. (80-. ) 265, 765–768. doi:10.1126/science.265.5173.765
Jiao, L., Pang, W., Zhou, J., Wei, Y., Mu, X., Bai, G., et al. (2011). Regioselective stepwise bromination of boron dipyrromethene (BODIPY) dyes. J. Org. Chem. 76, 9988–9996. doi:10.1021/jo201754m
Jin, G. F., Cho, Y. J., Wee, K. R., Hong, S. A., Suh, I. H., Son, H. J., et al. (2015). BODIPY functionalized o-carborane dyads for low-energy photosensitization. Dalt. Trans. 44, 2780–2787. doi:10.1039/c4dt03123g
Karatay, A., Miser, M. C., Cui, X., Küçüköz, B., Yilmaz, H., Sevinç, G., et al. (2015). The effect of heavy atom to two photon absorption properties and intersystem crossing mechanism in aza-boron-dipyrromethene compounds. Dye. Pigment. 122, 286–294. doi:10.1016/j.dyepig.2015.07.002
Kee, H. L., Kirmaiery, C., Yu, L., Thamyongkit, P., Youngblood, W. J., Calder, M. E., et al. (2005). Structural control of the photodynamics of boron-dipyrrin complexes. J. Phys. Chem. B 109, 20433–20443. doi:10.1021/jp0525078
Khatua, S., Guerrero, J. M., Claytor, K., Vives, G., Kolomeisky, A. B., Tour, J. M., et al. (2009). Micrometer-scale translation and monitoring of individual nanocars on glass, Microm. Transl. 3, 351–356. doi:10.1021/nn800798a
Kim, S. Y., Cho, Y. J., Son, H. J., Cho, D. W., and Kang, S. O. (2018). Photoinduced electron transfer in a BODIPY- ortho -carborane dyad investigated by time-resolved transient absorption spectroscopy. J. Phys. Chem. A 122, 3391–3397. doi:10.1021/acs.jpca.8b01539
Kulinich, A. V., and Ishchenko, A. A. (2024). Design and photonics of merocyanine dyes. Chem. Rec. 24, e202300262. doi:10.1002/tcr.202300262
Labra-Vázquez, P., Flores-Cruz, R., Galindo-Hernández, A., Cabrera-González, J., Guzmán-Cedillo, C., Jiménez-Sánchez, A., et al. (2020). Tuning the cell uptake and subcellular distribution in BODIPY–carboranyl dyads: an experimental and theoretical study. Chem. - A Eur. J. 26, 16530–16540. doi:10.1002/chem.202002600
Leśnikowski, Z. J. (2016). Challenges and opportunities for the application of boron clusters in drug design. J. Med. Chem. 59, 7738–7758. doi:10.1021/acs.jmedchem.5b01932
Li, H., Wang, J., Jiao, L., and Hao, E. (2024). BODIPY-based photocages: rational design and their biomedical application. Chem. Commun. 60, 5770–5789. doi:10.1039/d4cc01412j
Littke, A. F., and Fu, G. C. (2001). A versatile catalyst for heck reactions of aryl chlorides and aryl bromides under mild conditions. J. Am. Chem. Soc. 123, 6989–7000. doi:10.1021/ja010988c
Loudet, A., and Burgess, K. (2007). BODIPY dyes and their derivatives: syntheses and spectroscopic properties. Chem. Rev. 107, 4891–4932. doi:10.1021/cr078381n
Morin, J. F., Sasaki, T., Shirai, Y., Guerrero, J. M., and Tour, J. M. (2007). Synthetic routes toward carborane-wheeled nanocars. J. Org. Chem. 72, 9481–9490. doi:10.1021/jo701400t
Mukherjee, S., and Thilagar, P. (2016). Boron clusters in luminescent materials. Chem. Commun. 52, 1070–1093. doi:10.1039/c5cc08213g
Naito, H., Nishino, K., Morisaki, Y., Tanaka, K., and Chujo, Y. (2017). Solid-state emission of the anthracene-o-carborane dyad from the twisted-intramolecular charge transfer in the crystalline state. Angew. Chem. Int. Ed. 56, 254–259. doi:10.1002/anie.201609656
Nar, I., Atsay, A., Buyruk, A., Pekbelgin Karaoğlu, H., Burat, A. K., and Hamuryudan, E. (2019). BODIPY-ortho-carborane-tetraphenylethylene triad: synthesis, characterization, and properties. New J. Chem. 43, 4471–4476. doi:10.1039/C9NJ00177H
Núñez, R., Farràs, P., Teixidor, F., Viñas, C., Sillanpää, R., and Kivekäs, R. (2006). A discrete PIIP assembly: the large influence of weak interactions on the 31P NMR spectra of phosphane–diiodine complexes. Angew. Chem. - Int. Ed. 45, 1270–1272. doi:10.1002/anie.200503007
Núñez, R., Romero, I., Teixidor, F., and Viñas, C. (2016a). Icosahedral boron clusters: a perfect tool for the enhancement of polymer features. Chem. Soc. Rev. 45, 5147–5173. doi:10.1039/c6cs00159a
Núñez, R., Tarrés, M., Ferrer-Ugalde, A., De Biani, F. F., and Teixidor, F. (2016b). Electrochemistry and photoluminescence of icosahedral carboranes, boranes, metallacarboranes, and their derivatives. Chem. Rev. 116, 14307–14378. doi:10.1021/acs.chemrev.6b00198
Ochi, J., Tanaka, K., and Chujo, Y. (2020). Recent progress in the development of solid-state luminescent o-carboranes with stimuli responsivity. Angew. Chem. Int. Ed. 59, 9841–9855. doi:10.1002/anie.201916666
Oliva, J. M., Allan, N. L., Schleyer, P. V. R., Viñas, C., and Teixidor, F. (2005). Strikingly long C···C distances in 1,2-disubstitutedortho-carboranes and their dianions. J. Am. Chem. Soc. 127, 13538–13547. doi:10.1021/ja052091b
Parejo, L., Chaari, M., Santiago, S., Guirado, G., Teixidor, F., Núñez, R., et al. (2021). Reversibly switchable fluorescent molecular systems based on metallacarborane–perylenediimide conjugates. Chem. - A Eur. J. 27, 270–280. doi:10.1002/chem.202002419
Parisotto, S., Lace, B., Artuso, E., Lombardi, C., Deagostino, A., Scudu, R., et al. (2017). Heck functionalization of an asymmetric aza-BODIPY core: synthesis of far-red infrared probes for bioimaging applications. Org. Biomol. Chem. 15, 884–893. doi:10.1039/C6OB02602H
Plesek, J., Janousek, Z. H. S., and Heřmánek, S. (1978). Chemistry of 9-mercapto-1,7-dicarba-closo-dodecaborane. Collect Czech Chem. Commun. 43, 1332–1338. doi:10.1135/cccc19781332
Poater, J., Solà, M., Viñas, C., and Teixidor, F. (2014). π aromaticity and three-dimensional aromaticity: two sides of the same coin. Angew. Chem. - Int. Ed. 53, 12191–12195. doi:10.1002/anie.201407359
Poater, J., Viñas, C., Bennour, I., Escayola, S., Solà, M., and Teixidor, F. (2020). Too persistent to give up: aromaticity in boron clusters survives radical structural changes. J. Am. Chem. Soc. 142, 9396–9407. doi:10.1021/jacs.0c02228
Rana, S., Nayak, S. R., Patel, S., and Vaidyanathan, S. (2023). Efficiency boost in non-doped blue organic light-emitting diodes: harnessing aggregation-induced emission - a comprehensive review. J. Mater. Chem. C 12, 765–818. doi:10.1039/d3tc03449f
Scholz, M., and Hey-Hawkins, E. (2011). Carbaboranes as pharmacophores: properties, synthesis, and application strategies. Chem. Rev. 111, 7035–7062. doi:10.1021/cr200038x
Sekhar, A. R., Kaloo, M. A., and Sankar, J. (2014). Aliphatic amine discrimination by pentafluorophenyl dibromo BODIPY. Chem. - Asian J. 9, 2422–2426. doi:10.1002/asia.201402389
Shlyakhtina, N. I., Safronov, A. V., Sevryugina, Y. V., Jalisatgi, S. S., and Hawthorne, M. F. (2015). Synthesis, characterization, and preliminary fluorescence study of a mixed-ligand bis(dicarbollyl)nickel complex bearing a tryptophan-BODIPY FRET couple. J. Organomet. Chem. 798, 234–244. doi:10.1016/j.jorganchem.2015.04.035
Sivaev, I. B., and Bregadze, V. I. (2019). “Borane, carborane and metallacarborane anions for stabilization of transient and highly reactive intermediates,” in Boron in organometallic chemistry, 147–203.
Sivaev, I. B., and Bregadze, V. V. (2009). Polyhedral boranes for medical applications: current status and perspectives. Eur. J. Inorg. Chem. 2009, 1433–1450. doi:10.1002/ejic.200900003
Stockmann, P., Gozzi, M., Kuhnert, R., Sárosi, M. B., and Hey-Hawkins, E. (2019). New keys for old locks: carborane-containing drugs as platforms for mechanism-based therapies. Chem. Soc. Rev. 48, 3497–3512. doi:10.1039/c9cs00197b
Tan, Y. X., Zhang, X., Lin, J., and Wang, Y. (2023). A perspective on photoelectrochemical storage materials for coupled solar batteries. Energy Environ. Sci. 16, 2432–2447. doi:10.1039/d3ee00461a
Teixidor, F., Nñez, R., Viñas, C., Sillanpää, R., and Kivekäs, R. (2000). The distinct effect of the o-carboranyl fragment: its influence on the I-I distance in R3PI2 complexes. Angew. Chem. - Int. Ed. 39, 4290–4292. doi:10.1002/1521-3773(20001201)39:23<4290::AID-ANIE4290>3.0.CO;2-3
Tominaga, M., Morisaki, Y., and Chujo, Y. (2013). Luminescent polymer consisting of 9,12-linked o-carborane. Macromol. Rapid Commun. 34, 1357–1362. doi:10.1002/marc.201300368
Tominaga, M., Naito, H., Morisaki, Y., and Chujo, Y. (2014). Control of the emission behaviors of trifunctional o-carborane dyes. Asian J. Org. Chem. 3, 624–631. doi:10.1002/ajoc.201300280
Tsang, M. Y., Viñas, C., Teixidor, F., Choquesillo-Lazarte, D., and Giner Planas, J. (2017). Crystalline inclusion compounds of a palladacyclic tetraol host featuring o-carborane units. Eur. J. Inorg. Chem. 2017, 4589–4598. doi:10.1002/ejic.201700535
Tu, D., Cai, S., Fernandez, C., Ma, H., Wang, X., Wang, H., et al. (2019). Boron-cluster-enhanced ultralong organic phosphorescence. Angew. Chem. Int. Ed. 58, 9129–9133. doi:10.1002/anie.201903920
Ulrich, G., and Ziessel, R. (2004). Convenient and efficient synthesis of functionalized oligopyridine ligands bearing accessory pyrromethene-BF2 fluorophores. J. Org. Chem. 69, 2070–2083. doi:10.1021/jo035825g
Valliant, J. F., Guenther, K. J., King, A. S., Morel, P., Schaffer, P., Sogbein, O. O., et al. (2002). The medicinal chemistry of carboranes. Coord. Chem. Rev. 232, 173–230. doi:10.1016/S0010-8545(02)00087-5
Vinas, C. (2013). No TitleThe uniqueness of boron as a novel challenging element for drugs in pharmacology, medicine and for smart biomaterials. Futur. Med. Chem. 5, 617–619. doi:10.4155/fmc.13.41
Vives, G., and Tour, J. M. (2009). Synthesis of single-molecule nanocars. Acc. Chem. Res. 42, 473–487. doi:10.1021/ar8002317
Wang, H., Vicente, M. G. H., Fronczek, F. R., and Smith, K. M. (2014). Synthesis and transformations of 5-chloro-2,2′-dipyrrins and their boron complexes, 8-chloro-BODIPYs. Chem. - A Eur. J. 20, 5064–5074. doi:10.1002/chem.201304310
Wei, X., Zhu, M. J., Cheng, Z., Lee, M., Yan, H., Lu, C., et al. (2019). Aggregation-induced electrochemiluminescence of carboranyl carbazoles in aqueous media. Angew. Chem. - Int. Ed. 58, 3162–3166. doi:10.1002/anie.201900283
Xie, Y., Li, Z., Zhao, C., Lv, R., Li, Y., Zhang, Z., et al. (2024). Recent advances in aggregation-induced emission–active type I photosensitizers with near-infrared fluorescence: from materials design to therapeutic platform fabrication. Luminescence 39, e4621. doi:10.1002/bio.4621
Xuan, S., Zhao, N., Zhou, Z., Fronczek, F. R., and Vicente, M. G. H. (2016). Synthesis and in vitro studies of a series of carborane-containing boron dipyrromethenes (BODIPYs). J. Med. Chem. 59, 2109–2117. doi:10.1021/acs.jmedchem.5b01783
Yadav, I. S., and Misra, R. (2023). Design, synthesis and functionalization of BODIPY dyes: applications in dye-sensitized solar cells (DSSCs) and photodynamic therapy (PDT). J. Mater. Chem. C 11, 8688–8723. doi:10.1039/d3tc00171g
Yan, M., He, D., Zhang, L., Sun, P., Sun, Y., Qu, L., et al. (2022). Explorations into the meso-substituted BODIPY-based fluorescent probes for biomedical sensing and imaging. Trac. - Trends Anal. Chem. 157, 116771. doi:10.1016/j.trac.2022.116771
Yang, L., Bongsuiru Jei, B., Scheremetjew, A., Kuniyil, R., and Ackermann, L. (2021). Electrochemical B−H nitrogenation: access to amino acid and BODIPY-labeled nido-carboranes. Angew. Chem. - Int. Ed. 60, 1482–1487. doi:10.1002/anie.202012105
Zaitsev, A. V., Kiselev, S. S., Smol’yakov, A. F., Fedorov, Y. V., Kononova, E. G., Borisov, Y. A., et al. (2023). BODIPY derivatives modified with carborane clusters: synthesis, characterization and DFT studies. Org. Biomol. Chem. 21, 4084–4094. doi:10.1039/D3OB00255A
Zaitsev, A. V., Kononova, E. G., Markova, A. A., Shibaeva, A. V., Kostyukov, A. A., Egorov, A. E., et al. (2022a). A straightforward approach to carborane-substituted BODIPY derivatives via nucleophilic aromatic substitution: synthesis and photodynamic properties. Dye. Pigment. 207, 110711. doi:10.1016/j.dyepig.2022.110711
Zaitsev, A. V., Morozyuk, A. S., Kononova, E. G., Smol’yakov, A. F., and Ol’shevskaya, V. A. (2022b). Maleimide-functionalized BODIPY dyes: synthesis, structural determination and conjugation with carborane thiols. J. Porphyr. Phthalocyanines 26, 741–747. doi:10.1142/S1088424622500596
Zhao, N., Vicente, M. G. H., Fronczek, F. R., and Smith, K. M. (2015). Synthesis of 3,8-Dichloro-6-ethyl-1,2,5,7-tetramethyl-BODIPY from an asymmetric dipyrroketone and reactivity studies at the 3,5,8-positions. Chem. - A Eur. J. 21, 6181–6192. doi:10.1002/chem.201406550
Keywords: BODIPY, carboranes, boron clusters, luminescence, bioimaging, fluorescence, FRET, BNCT
Citation: Ordóñez-Hernández J, Planas JG and Núñez R (2024) Carborane-based BODIPY dyes: synthesis, structural analysis, photophysics and applications. Front. Chem. 12:1485301. doi: 10.3389/fchem.2024.1485301
Received: 23 August 2024; Accepted: 02 October 2024;
Published: 05 November 2024.
Edited by:
Eusebio Juaristi, Center for Research and Advanced Studies, National Polytechnic Institute of Mexico (CINVESTAV), MexicoReviewed by:
Norberto Farfan, Universidad Nacional Autónoma de México, MexicoCopyright © 2024 Ordóñez-Hernández, Planas and Núñez. This is an open-access article distributed under the terms of the Creative Commons Attribution License (CC BY). The use, distribution or reproduction in other forums is permitted, provided the original author(s) and the copyright owner(s) are credited and that the original publication in this journal is cited, in accordance with accepted academic practice. No use, distribution or reproduction is permitted which does not comply with these terms.
*Correspondence: Rosario Núñez, cm9zYXJpb0BpY21hYi5lcw==
Disclaimer: All claims expressed in this article are solely those of the authors and do not necessarily represent those of their affiliated organizations, or those of the publisher, the editors and the reviewers. Any product that may be evaluated in this article or claim that may be made by its manufacturer is not guaranteed or endorsed by the publisher.
Research integrity at Frontiers
Learn more about the work of our research integrity team to safeguard the quality of each article we publish.