- 1GSI Helmholtzzentrum für Schwerionenforschung, Darmstadt, Germany
- 2Helmholtz-Institut Mainz, Mainz, Germany
- 3Department of Chemistry, Johannes Gutenberg-Universität Mainz, Mainz, Germany
- 4Department of Physics, Lund University, Lund, Sweden
- 5Hochschule Mannheim, Mannheim, Germany
- 6Łukasiewicz - Instytut Mikroelektroniki i Fotoniki, Warsaw, Poland
Chemical reactivity of the superheavy elements nihonium (Nh, element 113) and moscovium (Mc, element 115) has been studied by the gas-solid chromatography method using a new combined chromatography and detection setup. The Mc isotope, 288Mc, was produced in the nuclear fusion reaction of 48Ca ions with 243Am targets at the GSI Helmholtzzentrum Darmstadt, Germany. After isolating 288Mc ions in the gas-filled separator TASCA, adsorption of 288Mc and its decay product 284Nh on silicon oxide and gold surfaces was investigated. As a result of this work, the values of the adsorption enthalpy of Nh and Mc on the silicon oxide surface were determined for the first time,
1 Introduction
1.1 Relativistic effects and periodicity trends in the superheavy element chemistry
Significant progress in the synthesis of the heaviest man-made chemical elements was achieved in the last decades resulting in the completion of the 7th row in the Periodic Table of the Elements (PTE) (Oganessian and Utyonkov, 2015). Due to decreasing nuclear fusion- and survival probabilities with increasing atomic number Z, the production rates of the superheavy elements (SHE) decrease rapidly, reaching a level of a single atom per month for oganesson (Og, element 118) (Oganessian and Utyonkov, 2015). The commonly used technique for SHE isolation is the kinematic separation in a recoil separator. The time between the production of a nuclear fusion reaction product in the target and its implantation as a recoil ion in the detection system is about 1 microsecond, allowing studies of nuclear decay properties of very short-lived isotopes. Experimental methods for chemical studies of SHE are more demanding and time-consuming, and therefore less efficient, especially for short-lived SHE isotopes. This makes studies of chemical properties more challenging (Türler and Pershina, 2013). Among the chemical techniques applied for SHE, a fast and efficient chemical separation can be achieved by gas-solid chromatography studies of volatile species (Türler and Pershina, 2013; Türler et al., 2015).
Chemical properties of yet unstudied SHE can be extrapolated based on the general law of periodicity, which connects the electronic structure of the elements with their position in the PTE. However, with increasing nuclear charge of the SHE, the influence of increasingly important relativistic effects on chemical properties has to be taken into account (Schwerdtfeger et al., 2020; Pyper, 2020; Pershina, 2019; Pershina, 2015). Apparently, the strong contraction of the s and p1/2 atomic orbitals (AO) and a large spin-orbit (SO) splitting of the electron shells with the orbital angular momentum quantum number l ≥ 1, can lead to properties different from those of their lighter homologues. Thus, reliable predictions of chemical properties of SHE become of extreme importance for the heaviest members of groups 12–18 (Schwerdtfeger et al., 2020; Pershina, 2019; Smits et al., 2024). Since the reactivity of atoms is generally connected to the energy level of their valence AO, relativistic calculations of the AO energy levels and of the first ionization potentials (IP) allow assessing the validity of trends within the groups of the PTE (Eliav et al., 2015; Borschevsky et al., 2015; Dzuba and Flambaum, 2016; Trombach et al., 2019). A strong relativistic stabilization of the spherical atomic orbitals with closed-shell 7s2 and quasi closed-shell 7p1/22 electronic configurations, together with the large spin-orbit splitting within the 7p shell were shown to result in low AO energies and large IP values for Cn and Fl, respectively (Schwerdtfeger et al., 2020; Pershina, 2015; Eliav et al., 2015). Accordingly, these elements were predicted to be the least reactive members of groups 12 and 14 (Pitzer, 1975; Schwerdtfeger and Seth, 2002). These predictions were recently confirmed experimentally (Eichler et al., 2007; Eichler et al., 2010; Yakushev et al., 2014; Yakushev and Eichler, 2016; Yakushev et al., 2022).
The neighbouring odd-Z elements nihonium (Nh, element 113) and moscovium (Mc, element 115) are presently in the focus of theoretical studies. Since Nh and Mc possess one unpaired electron in the 7p1/2 or 7p3/2 subshells, respectively, higher AO energies and lower IP values than for Cn and Fl are predicted for Nh and Mc. This implies that the reactivity of Nh and Mc is higher compared with that of their closed-shell neighbours Cn and Fl. Therefore, chemical reactivity, i.e., the tendency to form chemical bonds, is predicted to be higher for Nh and Mc than for Cn and Fl (Schwerdtfeger et al., 2020; Pershina, 2015; Trombach et al., 2019). However, experimental data on the reactivity of Nh and Mc are required to validate the predicted local minimum at Fl. Indeed, Nh and Mc are expected to form chemical compounds, e.g., hydroxides, which should adsorb more strongly on surfaces than pure atoms (Pershina et al., 2009; Pershina and Iliaš, 2019). Recently, motivated by experimental developments (Yakushev et al., 2021), the interaction of Nh and Mc with Au and quartz surfaces was studied on the basis of periodic relativistic density functional theory (DFT) calculations (Pershina, 2016; Pershina et al., 2021). As a result, equal values of the adsorption energy/enthalpy (Eads = −ΔHads) on quartz, of 58 kJ/mol, were predicted for elemental Nh and Mc (Pershina et al., 2021). Significantly (by about 100 kJ/mol) higher Eads values were predicted for the adsorption of these elements on the Au (111) surface in several theoretical works (Trombach et al., 2019; Pershina, 2018; Rusakov et al., 2013; Fox-Beyer and van Wüllen, 2012). The obtained Eads values for Nh and Mc turned out to be significantly (by about 50–100 kJ/mol) lower than those of their lighter homologues, Tl and Bi, respectively, on both types of the surfaces, quartz and Au. This is due to the strong relativistic stabilization of the 7p1/2 AO with respect to the 6p1/2 AO (Pershina, 2018; Pershina, 2016; Pershina et al., 2021). Thus, all the available predictions agree that both Nh and Mc should be more reactive than Cn and Fl and should easily adsorb at room temperature on Au, and also adsorb on less reactive quartz surfaces (Ilias and Pershina, 2022).
1.2 Experimental challenges and methods
The gas chromatography method has proven being efficient for chemical studies of SHE compared to methods applied for chemical studies in the liquid phase (Türler and Pershina, 2013; Türler et al., 2015). However, the overall efficiency of this method is drastically dependent on the volatility and chemical reactivity of the species under study. In chemistry experiments with the lighter SHE rutherfordium (Rf, element 104) to bohrium (Bh, element 107), the chemical separation was achieved by the combination of the gas-jet transport with gas-solid chromatography (Türler and Pershina, 2013; Türler et al., 2015). A rather low overall efficiency of this multi-step technique was compensated by the relatively high production rates (Türler and Pershina, 2013). A significantly higher overall efficiency was achieved in the chemical study of hassium (Hs, element 108), which was separated in the form of the volatile compound HsO4 (Düllmann et al., 2002). This technique is based on the combination of gas-solid isothermal chromatography and thermochromatography with a detection system, i.e., by registration of the nuclear decay of a species adsorbed inside the chromatography column itself. The method was developed further during the past two decades, all along to the chemistry studies of the rather inert Cn and Fl (Eichler et al., 2007; Eichler et al., 2010; Yakushev et al., 2014; Yakushev and Eichler, 2016; Yakushev et al., 2022). The necessity of the spatial separation of the single volatile superheavy atoms from other volatile species that contain radionuclides with similar nuclear decay properties as the nuclide under study and that are created in vastly larger amounts in the form of unwanted side products of the nuclear reactions was recognised (Düllmann et al., 2005). This includes especially Rn isotopes and their daughters. This separation is nowadays routinely achieved by employing physical preseparation in an electromagnetic recoil separator (Yakushev et al., 2014; Yakushev et al., 2022; Wittwer et al., 2010).
Several offline and online studies of the adsorption of Tl species on quartz and gold surfaces were performed, in which a stronger interaction of Tl with Au than with quartz was found (Serov et al., 2013; Steinegger et al., 2016; Lens et al., 2018). In thermochromatography studies under dry and oxygen-free conditions, as well as in the presence of hydrogen, the deposition of Tl on gold was observed at a temperature of about 900°C and was assigned to elemental Tl (Serov et al., 2013). However, independent of the chemical composition in the gas phase, the deposition of Tl on quartz at a temperature of about 300°C was ascribed to be due to TlOH formation caused by a high reactivity of Tl towards hydroxyl groups expected to be present on the quartz surface (Serov et al., 2013). Whereas initial experimental studies with homologues are usually performed in chromatography columns made of bulk materials like quartz (fused silica) or Au metal foils, SHE experiments are often conducted in channels made of silicon radiation detectors with thin hetero-layers covering the detector surfaces. Thin silicon oxide (SiO2) or Au layers on silicon detectors are produced by oxidation or metal vapour deposition, respectively, and have poorly defined structures. These thin layers serve as reactive solid surface in the gas-solid chromatography process and are usually complex and differ from those used in most theoretical calculations on the adsorption of SHE.
For Cn and Fl, the focus of the chemical studies was on the interaction with Au surfaces (Eichler et al., 2007; Eichler et al., 2010; Yakushev et al., 2014; Yakushev and Eichler, 2016; Yakushev et al., 2022; Wittwer et al., 2010). In these experiments the adsorption enthalpy,
The longest-lived known Mc and Nh isotopes can be produced directly or as decay products in nuclear fusion reactions of 48Ca with 243Am and 249Bk targets, leading to the production of Mc and tennessine (Ts, element 117) isotopes, respectively (Oganessian and Utyonkov, 2015). The experimentally easiest access to Mc and Nh is via the nuclear fusion reaction 243Am (48Ca, 3n) 288Mc, which has a high cross section of approximately 10–20 pb (Oganessian et al., 2013; Rudolph et al., 2013; Gates et al., 2015; Oganessian et al., 2022; Oganessian et al., 2022b), similar to that for 288Fl produced in the reaction 244Pu (48Ca, 4n) 288Fl (Düllmann et al., 2010; Såmark-Roth et al., 2023), which was successfully used in chemical studies (Eichler et al., 2010; Yakushev et al., 2014; Yakushev and Eichler, 2016; Yakushev et al., 2022). The decay properties of the members of the 288Mc decay chain are rather well established based on more than two hundred observed decay chains (Oganessian et al., 2013; Rudolph et al., 2013; Gates et al., 2015; Oganessian et al., 2022; Oganessian et al., 2022b). The second member of the 288Mc decay chain, 284Nh, has a half-life of T1/2 =
2 Materials and methods
2.1 Experimental setup
The nuclear reaction 243Am (48Ca, 3n)288Mc, utilized for the discovery of element 115, Mc (Oganessian and Utyonkov, 2015), and for the detailed studies of the decay properties of the long α-decay chain starting from the isotope 288Mc (Oganessian et al., 2013; Rudolph et al., 2013; Gates et al., 2015; Oganessian et al., 2022; Oganessian et al., 2022b), was also used for the present chemical studies of Mc and Nh. The gas-filled recoil separator TASCA (Semchenkov et al., 2008) was used to separate products of the fusion-evaporation reaction from the intense primary beam and from the unwanted products of multi-nucleon transfer reactions. The chemical reactivity of Mc and Nh was investigated applying the gas-solid chromatography method in the isothermal regime at room temperature (20°C) (Türler and Pershina, 2013; Türler et al., 2015) via adsorption studies on two surface types, SiO2 and Au. The gas chromatography setup behind TASCA, as shown in Figure 1, was similar to that described in the first experiment on Nh chemistry at TASCA (Yakushev et al., 2021), except for the connecting tube between the Recoil Transfer Chamber (RTC) and the first detector array. The distance from the RTC gas volume to the first detector element was reduced to about 1 mm.
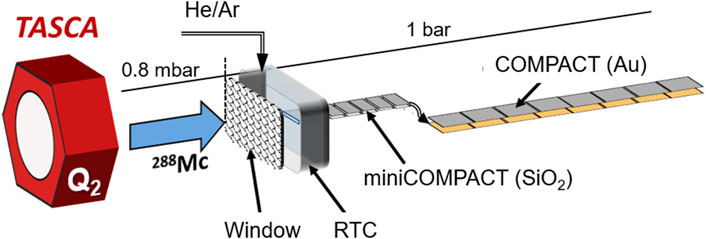
Figure 1. Experimental setup behind the second quadrupole (Q2) at the end of the TASCA separator. Recoiling 288Mc produced in the nuclear reaction were separated with TASCA and entered the Recoil Transfer Chamber (RTC) behind TASCA through a Mylar window. They were thermalized inside the RTC, the inner surface of which was covered with a Teflon™ layer and flushed out with a He/Ar gas mixture to the gas chromatography setup. The first gas chromatography and detection channel, miniCOMPACT, is connected directly to the RTC exit and covered with a SiO2 layer. The second detector array COMPACT has an Au layer on the detector surface. Both detector arrays were operated at room temperature.
A pulsed (5 ms beam-on, 15 ms beam-off) 48Ca10+ ion beam was provided by the UNIversal Linear ACcelerator (UNILAC) at GSI Darmstadt with a typical beam intensity of 5 1012 s−1. The target wheel contained four 243Am2O3 targets and rotated with a speed of 2000 revolutions per second, synchronized with beam pulses (Jäger et al., 2014). The 243Am targets with an average thickness of 0.83(1) mg/cm2 were electro-deposited on 2.2(1) μm Ti foils (Runke et al., 2014). The beam energy in the middle of the 243Am targets was 243.0(2) MeV. In total, 1.2(1) 1019 48Ca ions impinged on the targets during two experimental runs of 15 and 26 days duration, respectively. The evaporation residues were guided through the magnetic recoil separator TASCA with one dipole (D) and two quadrupole (Q) magnets in DQ1Q2 configuration, operated in the High-Transmission Mode (Semchenkov et al., 2008). TASCA was filled with He at a pressure of pHe = 0.8 mbar and was set to a magnetic rigidity of B·ρ = 2.21 Tm (Rudolph et al., 2013; Khuyagbaatar et al., 2012) to center 288Mc recoils in the TASCA focal plane. The nominal transmission efficiency in TASCA to focus the recoiling 288Mc ions into the RTC window area is about 40% (Yakushev et al., 2021). Products recoiling from the target and separated in TASCA according to their magnetic rigidities were thermalized in the RTC in a 1:1 gas mixture of He and Ar at 1 bar, similarly to the first Nh study at TASCA (Yakushev et al., 2021). Due to a large number of collisions with gas atoms, the isolated ions lose their kinetic energy, and their charge state is decreased accordingly to +1 or +2. The thermalized ions can be neutralized in gas by recombination or collisions with gas impurities or with any surface. The gas was circulated in a closed loop by a membrane pump at a flow rate of 2.9 L/min. The rare gases, He (99.9999%) and Ar (99.999%), were purified further by passing cartridges MC50-902FV and MC400-902FV (SAES™). These cartridges had internal particle filters, which efficiently remove aerosol particles with sizes down to 3 nm diameter and reduced the water and oxygen content to a level of about 1 ppm. The most critical impurity, water, was monitored by the dew point transmitter Pura (Michell Instruments). The isolated nuclear reaction products were flushed into the gas chromatography and detection setup. Two different detector channels were used, namely, a miniCOMPACT detector (Yakushev et al., 2021; Götz et al., 2021), followed by a COMPACT detector, used in the previous Fl chemistry studies at TASCA (Yakushev et al., 2014; Yakushev et al., 2022). The miniCOMPACT detector array was directly connected to the RTC via an exit slit with a cross section of (10.0 × 0.5) mm2, fitting to the cross section of the miniCOMPACT channel, and allowing for an effective transport and detection of less-volatile species. As Nh and Mc should interact more strongly with gold than with quartz (Pershina et al., 2021), the silicon photodiodes of the miniCOMPACT detector array were covered with a 30–50 nm-thick SiO2 layer. The photodiodes of the COMPACT detector array were covered with a 30 to 50 nm-thick Au layer. A 30-cm long capillary made of PTFE with an inner diameter of 2 mm connected the Au-covered COMPACT detector array to the miniCOMPACT array. Both detectors consisted of two panels, each divided into 32 detector elements. The opposite detector panels (top and bottom) were mounted with the active detector surfaces facing each other, and thus, forming a narrow gas channel with a distance between the opposite panels of 0.6 mm or 0.8 mm for the COMPACT array and the miniCOMPACT array, respectively. Each detector panel of the miniCOMPACT and COMPACT detectors consists of 32 Positive-Intrinsic-Negative (PIN) diodes mounted in a row (Wegrzecki et al., 2013). They registered α particles and fission fragments emitted by nuclear decays occurring inside the detector channel. Two different segmentation geometries of the miniCOMPACT detector arrays were used in the first and the second run. In the first run, a 15-cm long miniCOMPACT array had differently-sized detector elements mounted on a ceramic printed circuit board (PCB) as shown in Figure 2A. Each detector panel consisted of two silicon chips, which were 7 cm and 8 cm long. The first 7-cm long silicon chip was divided into three eight-element groups. They were 1, 2, and 4 cm long. The following 8-cm long chip had eight equal detector elements. In the second run, a 16-cm long miniCOMPACT detector array was used. The panels of the second detector consisted of two equal, 8-cm long silicon chips, each divided into sixteen detector elements, and were mounted on a multilayer FR4 PCB (flame retarding printed circuit board) as shown in Figure 2B.
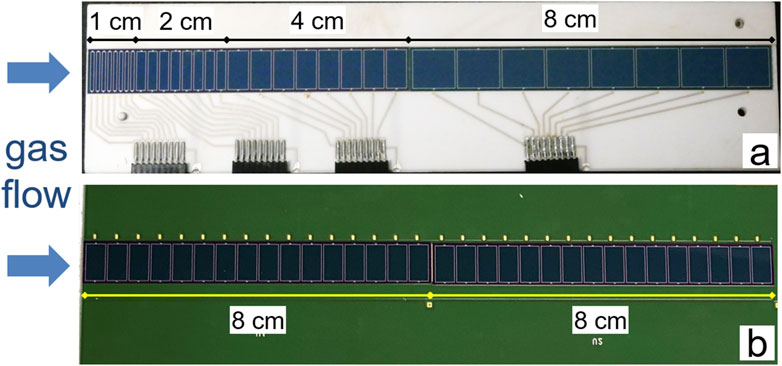
Figure 2. The open miniCOMPACT detector arrays. Only one of two detector panels forming the narrow channel is shown, as used in the first (A) and second experimental run (B). The direction of the gas flow through the detector channel is indicated with arrows.
2.2 Method of calculation
Theoretical calculations accompany our experimental study. We have calculated electronic energies of gas-phase reactions that single metal atoms (M, where M = Bi and Mc) and singly-charged metal ions (M+) could undergo in the recoil chamber. The aim was to investigate a potential reactivity of Bi and Mc species towards water and oxygen, which are present as gas impurities at the trace level.
Our considered systems are SHE, which can only be treated appropriately when relativistic effects are explicitly taken into account in the calculations. A well-proven method for such work is the Density Functional Theory (DFT) approach (Hohenberg and Kohn, 1964). The theory underlying is the DFT is the self-consistent Kohn–Sham approach (Kohn and Sham, 1965). Kohn-Sham DFT is a first-principles computational method to predict accurately chemical properties and to analyse them in simple chemical terms. Specifically, the Kohn-Sham approach in general delivers correct electron densities and related properties, as well as the total energy, but implies a one-electron representation of the many-electron systems. Although the precise exchange-correlation (XC) functional is unknown, by using appropriate XC functionals, binding energies may typically be determined with a precision of a few kJ/mol (Te Velde et al., 2001). DFT keeps at all levels of approximation the appealing one-electron molecular orbital (MO) view on chemical reactions and properties. The computed orbitals are suitable for typical MO-theoretical analyses and interpretations. The Kohn-Sham method also effectively incorporates all correlation effects. The calculations of electronic structures of the molecules of interest were performed using the Density Functional Theory approach implemented in the AMS ADF software (SCM, 2024). The AMS electronic structure approach uses the zeroth order regular approximation (ZORA) two-component Hamiltonian incorporating both scalar relativistic (SR) and SO relativistic effects. Although the regular approximation is not gauge invariant at any order, the scaled ZORA energy is exactly gauge invariant for hydrogen ions. The scaled ZORA approach, as it was used in our ADF calculations, is nearly gauge-invariant for many-electron systems, as explained in detail in Ref. (Van Lenthe et al., 1994). Briefly, the scaled ZORA equation differs from the original ZORA equation by the denominator to introduce a scaling of the ZORA energy. The calculated scaled-ZORA total energies prove to be very close to the fully relativistic energies for atoms. For all-electron calculations, the original ZORA total energy cannot be used as it suffers from gauge invariance problems that are particularly serious for deep-core states. The scaled ZORA total energy solved this problem, delivering results within “chemical” accuracy. The same holds for two-component calculations with the SO ZORA Hamiltonian. The spin-polarized non-collinear approach, also called as Kramers unrestricted approach, was applied. The basis sets are a combination of numerical AOs and Slater-type orbitals (STOs). The used basis sets are of the Triple Zeta plus Double Polarization (TZ2P) size. These basis sets are well saturated and describe the polarization of the electron density of an atom in molecules. Since correlation effects are also very important for SHE systems and can contribute up to the 50% of the binding energy, those are taken into account by using XC functionals. We utilized the BP86 (Becke, 1988) exchange-correlation functional. The geometry optimizations of studied molecules were carried out with the SO ZORA Hamiltonian, ensuring that both SR and SO relativistic effects were reflected in the optimized molecular structures.
3 Results
3.1 Observation of decay chains from 288Mc and 284Nh
A search for position- and time-correlated nuclear decay chains was performed, to identify multiple genetically-linked members of the well-known long 288Mc decay chain, decaying by emission of α-particles within the energy interval (8.0–10.5) MeV and within a 200-s time interval. This search resulted in the finding of eighteen decay chains, seven in the first run and eleven in the second run. The decay chains were assigned to 288Mc or to 284Nh in accordance with their known decay properties (Oganessian et al., 2022; Oganessian et al., 2022b; Forsberg et al., 2016; Rudolph, 2022). As usual for such an experiment, where an atom is adsorbed on a detector surface inside a gas chromatography channel, α particles or fission fragments have to penetrate several inactive layers. They deposit a fraction of their kinetic energy in a SiO2 or Au layer on top of the detector surface, and then in an inactive silicon layer on top of the diode. In addition, if the particles cross the detector channel, they experience further energy loss in the gas. These energy losses depend strongly on the angle to the surface, at which the particle is emitted. Thus, the energy distributions of registered α particles and spontaneous fission (SF) fragments have usually tailing towards low energies in contrast to the orresponding energy distributions registered in physics experiments, where the ions are implanted into a silicon detector. The energy calibration of the detectors was performed using a 227Ac source emanating 219Rn, and was linearly extrapolated up to the fission fragment energies. Independently, the search for high-energy events with an energy of ESF > 20 MeV was performed on a time scale of hours to days, to identify candidates for long-lived decay chain members terminating the decay chains by SF. In total, twenty-one candidate SF events were identified in the two runs. Sixteen SF events, twelve with two fragments and four with one fragment, occurred at the same detector positions, where chains with multiple α-decaying members were also detected, several hours before the SF events. These position-correlated SF events were assigned to the SF decay of 268Db or 264Lr (Oganessian et al., 2022). For two out of the eighteen registered decay chains, no terminating SF events were found, presumably due to a several-hour long problem with the data acquisition system in the period following the detection of these two chains. The decay parameters, energies and time intervals of the members of all observed decay chains are listed in Table 1. Figure 3 shows four representative examples (b–e) of observed chains, along with the reference decay chain (a).
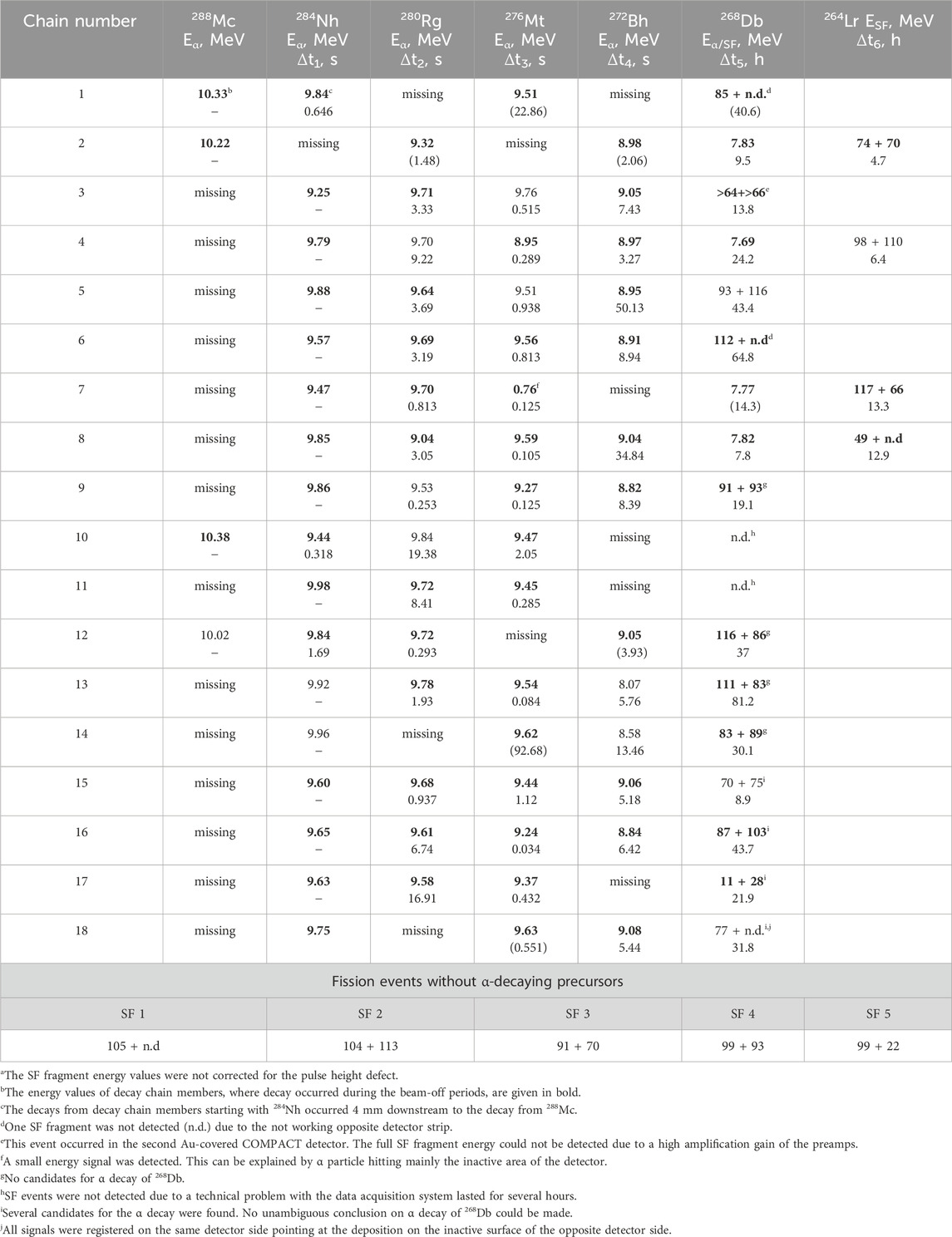
Table 1. Energies of α particles (Eα) and SF (ESF) fragmentsa and time intervals (Δt) of the observed decay chain members and fission events without α-decaying precursors. The total time difference for decay chain members following missing ones are given in parentheses.
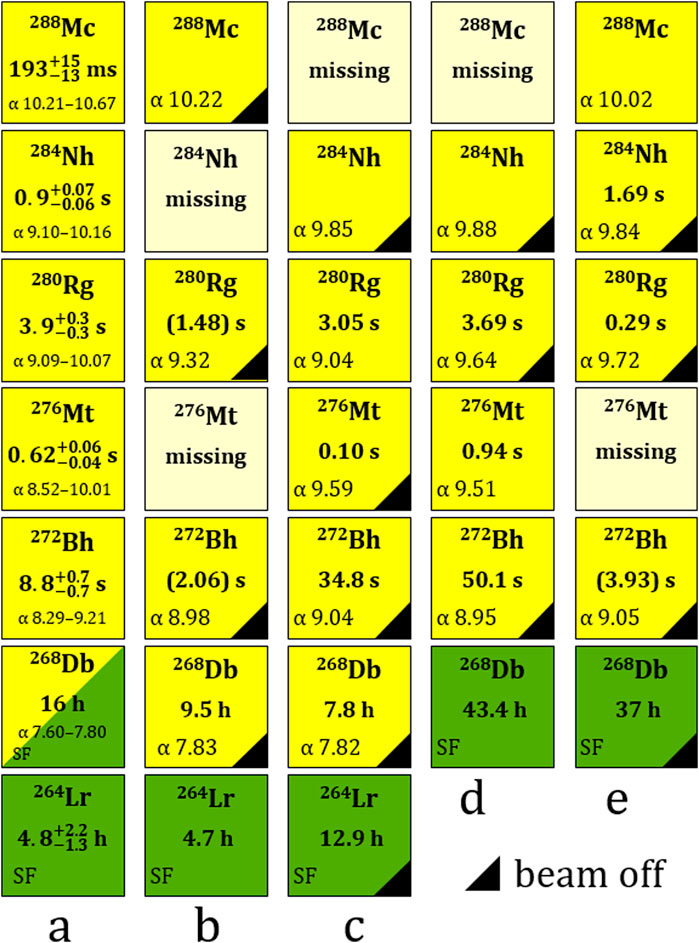
Figure 3. The decay properties of the 288Mc-decay chain members. (A) The presently known decay properties along the long decay chain from 288Mc are shown for comparison (Oganessian et al., 2022). (B–E) Four representative decay chains (#2, #8, #5, #12 in Table 1) out of eighteen observed in our experiments are shown. Color coding: α decay (yellow) and spontaneous fission (green). Half-lives (A) and correlation times (B–E) are indicated for decay chain members. The total time difference for decay chain members following missing ones are given in parentheses. The α-particle energies are given in mega electron volts (MeV). The black triangles represent the decays observed during beam-off periods.
Based on the decay properties along the long 288Mc decay chain (Figure 3A), four out of eighteen chains were attributed to originate from 288Mc (cf. Table 1). The missing observation of 288Mc in the other fourteen chains is attributed to the decay losses of the short-lived nucleus 288Mc (
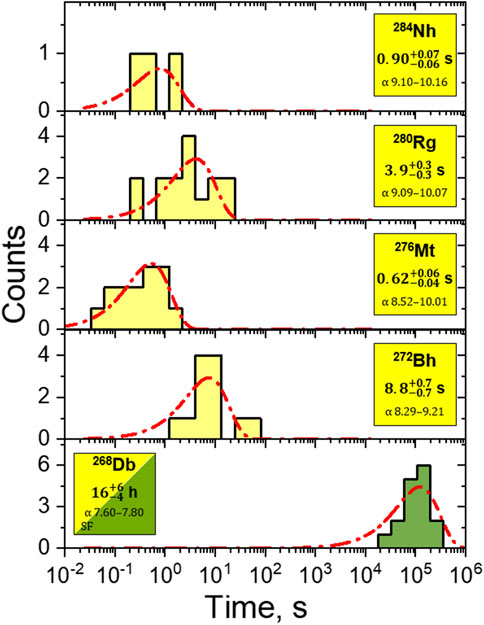
Figure 4. The lifetime distributions of the observed decay chain members 284Nh, 280Rg, 276Mt, 272Bh, and 268Db. The experimental lifetime distributions are shown for 284Nh, 280Rg, 276Mt, 272Bh, and 268Db (bars), in comparison with the lifetime probability curves (dash-dotted lines) calculated for the recently published half-lives of these isotopes (Oganessian et al., 2022; Oganessian et al., 2022b). Yellow denotes α-decay, green denotes spontaneous fission.
Five SF events, which were observed without position and time correlation to any α-decaying precursors within the energy range (9.0–10.5) MeV and a time interval of 1000 s. They could not be assigned to any registered long decay chain from 288Mc or 284Nh. Two such SF events were registered in the first centimeter of the miniCOMPACT array, and three at the 4th, 6th and 10th centimeter. In four cases, both fission fragments were detected. These SF-only events were considered as real ones and could be attributed to SF branches in early chain members (Oganessian et al., 2022; Forsberg et al., 2016; Rudolph, 2022). A detailed analysis on decay scenario aspects along the decay chain from 288Mc will be published elsewhere2. Even though the spatial distribution of these SF events in the detector channel resembles the distribution of decay chains from 284Nh, the ambiguity in the assignment of these SF-only events did not allow including them for the evaluation of the chemical behaviour.
A comparison of the number of events registered in the present experiment to the number of decay chains from the Mc decay spectroscopy experiment at TASCA (Rudolph et al., 2013) normalized to the number of produced 288Mc nuclei reveals that roughly 40% of the reaction products guided to the TASCA focal plane were extracted into the gas chromatography channel. The losses were primarily caused by adsorption of non-volatile species on the RTC surface before reaching the detection setup. In addition, decay losses also significantly contributed to the overall losses of the short-lived 288Mc.
3.2 Distribution of Mc and Nh in the detection setup
All observed decay chains originating from 288Mc or 284Nh were found in the miniCOMPACT detector, except for one decay chain from 284Nh, which was registered in the first centimeter of the Au-covered COMPACT. Thus, while all four Mc atoms adsorbed on the SiO2 surface, one of the 14 Nh atoms passed the miniCOMPACT detector and a 30-cm long connecting tube made of PTFE between miniCOMPACT and COMPACT, but adsorbed on the very first Au-covered detector. This observation may suggest a stronger interaction with the Au surface compared to interaction with SiO2 and PTFE surfaces, in line with the predictions (Pershina et al., 2021). This encourages future studies with higher statistics. The positions of the observed decay chains starting with 288Mc and starting with 284Nh in the detector channel are presented in Figure 5. All four events originating from 288Mc and about 85% of the 284Nh events were registered in the first half of the SiO2-covered miniCOMPACT detector.
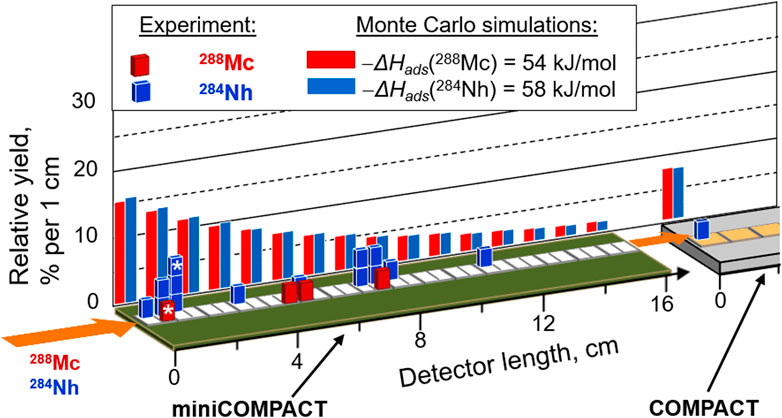
Figure 5. Event distribution in the detection setup. The positions of all observed decay chains in the detection setup assigned to 288Mc (red bars) and 284Nh (blue bars) are shown together with results of Monte Carlo simulations for the most probable values of the adsorption enthalpy on SiO2, −ΔHads (Mc) = 54 kJ/mol and −ΔHads (Nh) = 58 kJ/mol, for Mc and Nh, respectively. According to simulations, a fraction of about 8% of both, Mc and Nh atoms, entering the miniCOMPACT pass through. This fraction is indicated as deposition in the first centimeter of COMPACT. In one decay chain, the observed decays from 288Mc and 284Nh were distributed over two detectors; their positions are marked with an asterisk.
4 Discussion
Atoms without retention on the surface would pass miniCOMPACT within about 20 ms. Thus, almost all Nh and Mc nuclei would pass the miniCOMPACT detector array without decay. Clearly, the observation of the decay of Nh and Mc nuclei in the miniCOMPACT detector array demonstrated retention due to adsorption of Nh and Mc on SiO2 at room temperature. A key question is in which chemical form Mc and Nh deposited on the SiO2 detector surface. Despite the short lifetime of Mc and Nh, the possibility to form compounds through rare interactions with gas impurities cannot be excluded. The main gas impurities, O2 and H2O, were kept at a ppm level at most, similarly to the previous experiments at TASCA (Yakushev et al., 2014; Yakushev et al., 2022). To form compounds (oxides or hydroxides) in reactions with traces of O2 and H2O, Mc ions or atoms should be able to break rather strong chemical bonds in the O2 and H2O molecules after their thermalization in the gas. Energies of selected reactions of the M atoms and M+ ions with the O2 and H2O molecules, where M is a superheavy element Mc or Nh, or their nearest homologues Bi or Tl, were calculated with the ADF BAND code and are presented in Table 2. All values are positive, ranging from 2.07 to 11.77 eV. Because of the rather high positive values of the heat of reaction, these interactions are not energetically favored at room temperature for Mc and Nh and neither for their homologues Bi and Tl. Thus, the thermodynamical analysis of possible reactions with trace gas impurities clearly suggests that Mc and Nh should stay in the elemental form before they adsorb on the SiO2 surface. In order to obtain more quantitative information on the interaction strength between Mc and Nh atoms and the SiO2 surface, the Monte Carlo simulation method was applied to obtain numerical information on the interaction strength between SHE species and a surface. This approach is based on the model of mobile adsorption (Zvara, 1985) and allows to simulate individual histories of a large number of single atoms (e.g., 106) migrating through the chromatography column, using actual experimental parameters including the dimension of the column, the gas composition and flow rate, the radionuclide’s half-life etc. Single Mc or Nh atoms moving with the carrier gas along the detector arrays experience many collisions with the surface. In each surface collision, the adsorbed atom is immobilized at the surface for a time τa, which depends on the (experimentally known) oscillation period
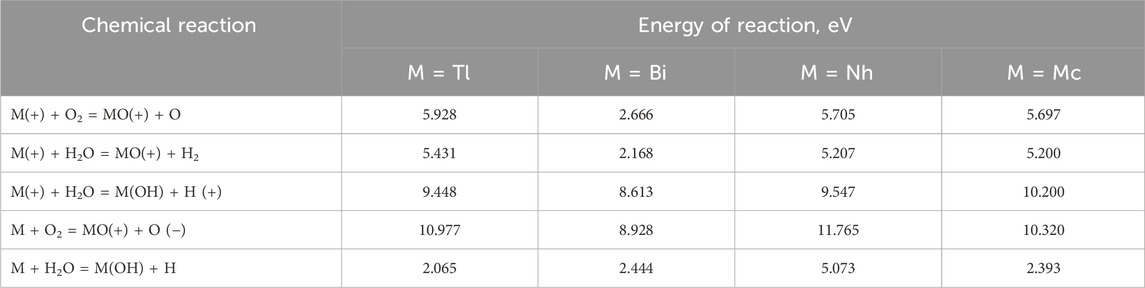
Table 2. Calculated values of the energy of reaction for selected chemical reactions of metal M (M = Bi, Mc) with oxygen and water. The initial metallic species are neutral atoms (M) or monovalent cations (M(+)). The initial oxygen and water are molecular O2 and H2O.
Supposed that the energy needed for the desorption is equal to the negative adsorption enthalpy,
Theoretical and experimental thermodynamic values for the reactivity and volatility of the superheavy elements in groups 12–15 and their nearest lighter homologues are summarized in Figure 6. For the heaviest members of the groups 12–15 of the PTE, trends in AO energies of the highest occupied orbitals show an irregular course with minima for groups 12 and 14. The spread is significantly more pronounced for SHE compared with their lighter homologues due to the strong spin-orbit splitting in the 7p shell and stabilisation of the 7s and 7p1/2 (sub) shells in Cn and Fl, respectively (Figure 6, upper panel). This trend also determines the chemical behaviour, e.g., the adsorption strength of these elements on the gold and quartz surfaces. The calculated and experimental values of −ΔHads on quartz for elements of the 6th row are shown in Figure 6 (middle panel) in comparison with their heat of vaporization (ΔHvap) values.
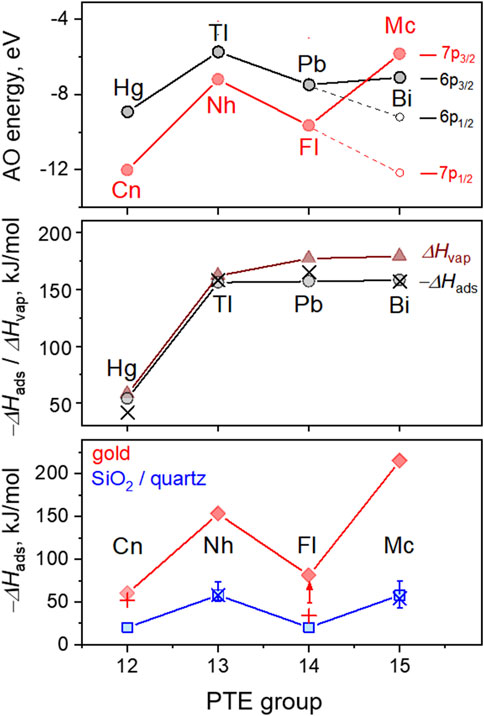
Figure 6. Calculated energies of the highest occupied atomic orbitals for elements of the groups 12–15 of the 6th and 7th period; adsorption enthalpies (−ΔHads) on quartz (theoretical values of 6p and 7p elements and experimental values of 6p elements); −ΔHads values on the SiO2 (experimental values of 7p elements) and Au surfaces (theoretical and experimental values of 7p elements); and vaporization enthalpies (ΔHvap). Upper panel: calculated Dirac-Fock energies of the highest occupied AO (in eV) are shown with red symbols for Cn to Mc and with black ones for their homologues, Hg to Bi (Desclaux, 1973). The black and red arrows show the values of the spin-orbit splitting for the 6p and 7p shells, respectively. Middle panel: The calculated and experimental values of −ΔHads on quartz for SHE homologues, Hg to Bi, are shown as grey circles and black crosses, respectively (Pershina, 2018; Trombach et al., 2019; Serov et al., 2013; Steinegger et al., 2016; Düllmann et al., 2022; Kraus, 2021). The experimental values of ΔHvap are shown as brown solid triangles (Zhang et al., 2011). Lower panel: calculated values of −ΔHads on quartz and Au for Cn to Mc are shown with blue square and red diamond symbols, respectively (Pershina, 2018; Pershina et al., 2021; Trombach et al., 2019; Düllmann et al., 2022). Experimental −ΔHads on Au for Cn and Fl are shown as red crosses and an arrow in the case of a limit (Eichler et al., 2010; Yakushev et al., 2014; Yakushev et al., 2022). Experimental values from this work,
The trend in −ΔHads values for the 6p elements is indicative of a strong increase in the interaction strength with the surface from Hg to Tl that remains almost unchanged for Tl, Pb, and Bi (Pershina, 2018; Trombach et al., 2019; Düllmann et al., 2022). The theoretical −ΔHads values show a good agreement with the experimental ones (Serov et al., 2013; Steinegger et al., 2016; Kraus, 2021). The experimental −ΔHads value for Bi was obtained in recent off-line gas chromatography studies with Po and At isotopes at the U-120 M cyclotron in Řež, Czech Republic, and will be published elsewhere. The ΔHvap values for these elements follow a similar trend (Zhang et al., 2011). For the heaviest members of groups 12–15, the calculated and experimental −ΔHads values on quartz and Au are shown in Figure 6 (lower panel). The predicted −ΔHads values for adsorption of Nh and Mc on quartz are significantly lower than those of their lighter homologues, Tl and Bi, respectively. Moreover, the trend of the −ΔHads values of the superheavy elements Cn to Mc has also an irregular course, similar to that of the energies of their highest occupied AOs. This difference in the trends between the 6p and 7p elements is attributed to the larger spin-orbital effects on the 7p AO (Figure 6, upper panel) and the relativistic stabilization of the 7p1/2 orbital, and is in line with the reduced chemical reactivity in Fl (Schwerdtfeger et al., 2020; Pershina, 2015; Eliav et al., 2015).
5 Conclusion
Eighteen decay chains originating from 288Mc and its decay products were identified upon adsorption on silicon oxide and gold surfaces (Table 1). The decay properties of the registered chain members are in excellent agreement with those obtained in earlier physics studies. Taking into account experimental conditions and results of state-of-the-art theoretical predictions, we conclude that Nh and Mc were deposited on the silicon oxide surface in the elemental state. The thermodynamic quantities for Nh and Mc, namely,
Data availability statement
The raw data supporting the conclusions of this article will be made available by the authors, without undue reservation.
Author contributions
AY: Conceptualization, Data curation, Formal Analysis, Investigation, Methodology, Project administration, Validation, Writing–original draft, Writing–review and editing. JKh: Data curation, Formal Analysis, Investigation, Software, Validation, Writing–review and editing. CD: Conceptualization, Funding acquisition, Methodology, Project administration, Resources, Supervision, Validation, Writing–review and editing. MB: Formal Analysis, Investigation, Validation, Writing–review and editing. RC: Data curation, Methodology, Resources, Software, Writing–review and editing. DC: Data curation, Formal Analysis, Investigation, Writing–review and editing. DD: Data curation, Formal Analysis, Investigation, Validation, Writing–review and editing. FG: Data curation, Investigation, Validation, Writing–review and editing. YH: Data curation, Formal Analysis, Writing–review and editing. MI: Methodology, Software, Validation, Writing–review and editing. EJ: Data curation, Resources, Software, Writing–review and editing. JKr: Data curation, Methodology, Resources, Writing–review and editing. DK: Data curation, Formal Analysis, Methodology, Writing–review and editing. NK: Data curation, Formal Analysis, Methodology, Resources, Software, Validation, Writing–review and editing. LL: Methodology, Writing–review and editing, Conceptualization, Data curation, Formal Analysis, Investigation. SL: Data curation, Formal Analysis, Resources, Software, Writing–review and editing. CM: Data curation, Methodology, Writing–review and editing. PM: Data curation, Formal Analysis, Investigation, Methodology, Software, Validation, Writing–review and editing. VP: Conceptualization, Formal Analysis, Investigation, Software, Validation, Writing–review and editing. SR: Data curation, Formal Analysis, Validation, Writing–review and editing. DR: Data curation, Formal Analysis, Investigation, Validation, Writing–review and editing. JR: Data curation, Methodology, Resources, Writing–review and editing. LS: Data curation, Formal Analysis, Methodology, Software, Validation, Writing–review and editing. BS: Data curation, Resources, Writing–review and editing. US: Data curation, Formal Analysis, Methodology, Validation, Writing–review and editing. PT-P: Data curation, Resources, Writing–review and editing. NT: Investigation, Methodology, Validation, Writing–review and editing. MW: Conceptualization, Methodology, Resources, Writing–review and editing. PW: Data curation, Formal Analysis, Methodology, Resources, Software, Writing–review and editing.
Funding
The author(s) declare that financial support was received for the research, authorship, and/or publication of this article. This work has been financially supported by the German BMBF (project 05P21UMFN2), the Swedish Research Council (Vetenskapsrådet, VR 2016-3969), and the Knut and Alice Wallenberg Foundation (KAW 2015.0021). The publication is funded by the Open Access Publishing Fund of GSI Helmholtzzentrum für Schwerionenforschung.
Acknowledgments
The results presented here are based on the experiments U308 and U327, which were performed at the beam line X8/TASCA at the GSI Helmholtzzentrum für Schwerionenforschung GmbH, Darmstadt (Germany) in the frame of FAIR Phase-0. The authors would like to thank the GSI staff of the ECR ion source group and the accelerator UNILAC for providing stable and intense ion beams, as well as the GSI department of experiment electronics, and the detector and target laboratories of GSI. The 243Am target material was provided by the U.S. DOE through ORNL.
Conflict of interest
The authors declare that the research was conducted in the absence of any commercial or financial relationships that could be construed as a potential conflict of interest.
Publisher’s note
All claims expressed in this article are solely those of the authors and do not necessarily represent those of their affiliated organizations, or those of the publisher, the editors and the reviewers. Any product that may be evaluated in this article, or claim that may be made by its manufacturer, is not guaranteed or endorsed by the publisher.
Footnotes
1Dietzel, D., Yakushev, A., Düllmann, Ch. E., Khuyagbaatar, J., Krier, J., Jäger, E., et al. (2024). Off-line single-atom gas chromatographic adsorption studies of 211Bi on SiO2 surfaces in the novel miniCOMPACT setup. Submitt. Radiochim. Acta. [under revision].
2Khuyagbaatar, J., Yakushev, A., Düllmann, Ch. E., and Mošať, P. (2024).
References
Aksenov, N. V., Steinegger, P., Abdullin, F.Sh., Albin, Yu. V., Bozhikov, G. A., Chepigin, V. I., et al. (2017). On the volatility of nihonium (Nh, Z = 113). Eur. Phys. J. A 53, 158. doi:10.1140/epja/i2017-12348-8
Becke, A. D. (1988). Density-functional exchange-energy approximation with correct asymptotic behavior. Phys. Rev. A 38, 3098–3100. doi:10.1103/physreva.38.3098
Borschevsky, A., Pašteka, L. F., Pershina, V., Eliav, E., and Kaldor, U. (2015). Ionization potentials and electron affinities of the superheavy elements 115–117 and their sixth-row homologues Bi, Po, and at. Phys. Rev. A 91, 020501. (R). doi:10.1103/physreva.91.020501
Brüchle, W. (2003). Confidence intervals for experiments with background and small numbers of events. Radiochim. Acta 91, 71–80. doi:10.1524/ract.91.2.71.19989
Desclaux, J. P. (1973). Relativistic Dirac-Fock expectation values for atoms with Z = 1 to Z = 120. Data Nucl. Data Tables 12, 311–406. doi:10.1016/0092-640x(73)90020-x
Dmitriev, S. N., Aksenov, N. V., Albin, Yu. V., Bozhikov, G. A., Chelnokov, M. L., Chepigin, V. I., et al. (2014). Pioneering experiments on the chemical properties of element 113. Mendeleev Commun. 24, 253–256. doi:10.1016/j.mencom.2014.09.001
Düllmann, Ch. E., Block, M., Heßberger, F. P., Khuyagbaatar, J., Kindler, B., Kratz, J. V., et al. (2022). Five decades of GSI superheavy element discoveries and chemical investigation. Radiochim. Acta 110 (6-9), 417–439. doi:10.1515/ract-2022-0015
Düllmann, Ch. E., Brüchle, W., Dressler, R., Eberhardt, K., Eichler, B., Eichler, R., et al. (2002). Chemical investigation of hassium (element 108). Nature 418, 859–862. doi:10.1038/nature00980
Düllmann, Ch. E., Folden, C. M., Gregorich, K. E., Hoffman, D. C., Leitner, D., Pang, G. K., et al. (2005). Heavy-ion-induced production and physical preseparation of short-lived isotopes for chemistry experiments. Nucl. Instrum. Meth. A 551, 528–539. doi:10.1016/j.nima.2005.05.077
Düllmann, Ch. E., Schädel, M., Yakushev, A., Türler, A., Eberhardt, K., Kratz, J. V., et al. (2010). Production and decay of element 114: high cross sections and the new nucleus 277Hs. Phys. Rev. Lett. 104, 252701. doi:10.1103/physrevlett.104.252701
Dzuba, V. A., and Flambaum, V. V. (2016). Electron structure of superheavy elements uut, Fl and uup (Z=113 to 115). Hyperfine Interact. 237, 160. doi:10.1007/s10751-016-1365-7
Eichler, R. (2013). First foot prints of chemistry on the shore of the Island of Superheavy Elements. J. Phys. Conf. Ser. 420, 012003. doi:10.1088/1742-6596/420/1/012003
Eichler, R., Aksenov, N. V., Albin, Yu. V., Belozerov, A. V., Bozhikov, G. A., Chepigin, V. I., et al. (2010). Indication for a volatile element 114. Radiochim. Acta 98, 133–139. doi:10.1524/ract.2010.1705
Eichler, R., Aksenov, N. V., Belozerov, A. V., Bozhikov, G. A., Chepigin, V. I., Dmitriev, S. N., et al. (2007). Chemical characterization of element 112. Nature 447, 72–75. doi:10.1038/nature05761
Eliav, E., Fritzsche, S., and Kaldor, U. (2015). Electronic structure theory of the superheavy elements. Nucl. Phys. A 944, 518–550. doi:10.1016/j.nuclphysa.2015.06.017
Forsberg, U., Rudolph, D., Andersson, L.-L., Di Nitto, A., Düllmann, Ch. E., Fahlander, C., et al. (2016). Recoil-α-fission and recoil-α–α-fission events observed in the reaction 48Ca + 243Am. Nucl. Phys. A 953, 117–138. doi:10.1016/j.nuclphysa.2016.04.025
Fox-Beyer, B. S., and van Wüllen, C. (2012). Theoretical modelling of the adsorption of thallium and element 113 atoms on gold using two-component density functional methods with effective core potentials. Chem. Phys. 395, 95–103. doi:10.1016/j.chemphys.2011.04.029
Frenkel, Ya. I. (1948). Statistical physics. Moscow-Leningrad: Izd. Akad. Nauk SSSR. [book in Russian].
Gates, J. M., Gregorich, K. E., Gothe, O. R., Uribe, E. C., Pang, G. K., Bleuel, D. L., et al. (2015). Decay spectroscopy of element 115 daughters: 280Rg → 276Mt and 276Mt → 272Bh. Phys. Rev. C 92, 021301. (R). doi:10.1103/physrevc.92.021301
Götz, S., Raeder, S., Block, M., Düllmann, Ch. E., Folden III, C. M., Glennon, K. J., et al. (2021). Rapid extraction of short-lived isotopes from a buffer gas cell for use in gas-phase chemistry experiments, Part II: on-line studies with short-lived accelerator-produced radionuclides. B 507, 27–35. doi:10.1016/j.nimb.2021.09.004
Hohenberg, P., and Kohn, W. (1964). Inhomogeneous electron gas. Phys. Rev. B 136, 864–B871. doi:10.1103/physrev.136.b864
Ilias, M., and Pershina, V. (2022). Reactivity of group 13 elements Tl and element 113, Nh, and of their hydroxides with respect to various quartz surfaces from periodic relativistic DFT calculations. Inorg. Chem. 61 (40), 15910–15920. doi:10.1021/acs.inorgchem.2c02103
Jäger, E., Brand, H., Düllmann, Ch.E., Khuyagbaatar, J., Krier, J., Schädel, M., et al. (2014). High intensity target wheel at TASCA: target wheel control system and target monitoring. J. Radioanal. Nucl. Chem. 299, 1073–1079. doi:10.1007/s10967-013-2645-1
Khuyagbaatar, J., Ackermann, D., Andersson, L.-L., Ballof, J., Brüchle, W., Düllmann, Ch. E., et al. (2012). Study of the average charge states of 188Pb and 252,254No ions at the gas-filled separator TASCA. Nucl. Instr. Meth. A 689, 40–46. doi:10.1016/j.nima.2012.06.007
Kohn, W., and Sham, L. J. (1965). Self-consistent equations including exchange and correlation effects. Phys. Rev. A 140, 1133–A1138. doi:10.1103/physrev.140.a1133
Kraus, B. (2021). Optimization of vacuum adsorption chromatography for superheavy element experiments [Doctoral dissertation]. Switzerland: University of Bern.
Lens, L., Yakushev, A., Düllmann, Ch. E., Asai, M., Ballof, J., Block, M., et al. (2018). Online chemical adsorption studies of Hg, Tl, and Pb on SiO2 and Au surfaces in preparation for chemical investigations on Cn, Nh, and Fl at TASCA. Radiochim. Acta 106, 949–962. doi:10.1515/ract-2017-2914
Oganessian, Yu Ts., Abdullin, F.Sh., Dmitriev, S. N., Gostic, J. M., Hamilton, J. H., Henderson, R. A., et al. (2013). Investigation of the 243Am + 48Ca reaction products previously observed in the experiments on elements 113, 115, and 117. Phys. Rev. C 87, 014302. doi:10.1103/physrevc.87.014302
Oganessian, Yu. Ts., and Utyonkov, V. K. (2015). Superheavy nuclei from 48Ca-induced reactions. Nucl. Phys. A 944, 62–98. doi:10.1016/j.nuclphysa.2015.07.003
Oganessian, Yu. Ts., Utyonkov, V. K., Kovrizhnykh, N. D., Abdullin, F.Sh., Dmitriev, S. N., Dzhioev, A. A., et al. (2022). New isotope 286Mc produced in the 243Am + 48Ca reaction. Phys. Rev. C 106, 064306. doi:10.1103/physrevc.106.064306
Oganessian, Yu. Ts., Utyonkov, V. K., Kovrizhnykh, N. D., Abdullin, F.Sh., Dmitriev, S. N., Ibadullayev, D., et al. (2022). First experiment at the Super Heavy Element Factory: high cross section of 288Mc in the 243Am + 48Ca reaction and identification of the new isotope 264Lr. Phys. Rev. C 106, L031301. doi:10.1103/PhysRevC.106.L031301
Pershina, V. (2015). Electronic structure and properties of superheavy elements. Nucl. Phys. A 944, 578–613. doi:10.1016/j.nuclphysa.2015.04.007
Pershina, V. (2016). A theoretical study on the adsorption behavior of element 113 and its homologue Tl on a quartz surface: relativistic periodic DFT calculations. J. Phys. Chem. C 120 (36), 20232–20238. doi:10.1021/acs.jpcc.6b07834
Pershina, V. (2018). Reactivity of superheavy elements Cn, Nh, and Fl and their lighter homologues Hg, Tl, and Pb, respectively, with a gold surface from periodic DFT calculations. Inorg. Chem. 57 (7), 3948–3955. doi:10.1021/acs.inorgchem.8b00101
Pershina, V. (2019). Relativity in the electronic structure of the heaviest elements and its influence on periodicities in properties. Radiochim. Acta 107, 833–863. doi:10.1515/ract-2018-3098
Pershina, V., Anton, J., and Jacob, T. (2009). Electronic structures and properties of M-Au and MOH, where M = Tl and element 113. Chem. Phys. Lett. 480, 157–160. doi:10.1016/j.cplett.2009.08.069
Pershina, V., and Iliaš, M. (2019). Properties and reactivity of hydroxides of group 13 elements in, Tl, and Nh from molecular and periodic DFT calculations. Inorg. Chem. 58, 9866–9873. doi:10.1021/acs.inorgchem.9b00949
Pershina, V., Ilias, M., and Yakushev, A. (2021). Reactivity of the superheavy element 115, Mc, and its lighter homologue, Bi, with respect to gold and hydroxylated quartz surfaces from periodic relativistic DFT calculations: a comparison with element 113. Nh. Inorg. Chem. 60 (13), 9848–9856.
Pitzer, K. S. (1975). Are elements 112, 114, and 118 relatively inert gases? J. Chem. Phys. 63, 1032–1033. doi:10.1063/1.431398
Pyper, N. C. (2020). Relativity and the periodic table. Phil. Trans. R. Soc. A 378, 20190305. doi:10.1098/rsta.2019.0305
Rudolph, D. (2022). Results and plans for nuclear spectroscopy of superheavy nuclei: the Lund perspective. Eur. Phys. J. A 58, 242. doi:10.1140/epja/s10050-022-00868-7
Rudolph, D., Forsberg, U., Golubev, P., Sarmiento, L. G., Yakushev, A., Andersson, L.-L., et al. (2013). Spectroscopy of element 115 decay chains. Phys. Rev. Lett. 111, 112502. doi:10.1103/physrevlett.111.112502
Runke, J., Düllmann, Ch. E., Eberhardt, K., Ellison, P. A., Gregorich, K. E., Hofmann, S., et al. (2014). Preparation of actinide targets for the synthesis of the heaviest elements. J. Radioanal. Nucl. Chem. 299, 1081–1084. doi:10.1007/s10967-013-2616-6
Rusakov, A. A., Demidov, Y. A., and Zaitsevskii, A. (2013). Estimating the adsorption energy of element 113 on a gold surface. Centr. Eur. J. Phys. 11, 1537–1540. doi:10.2478/s11534-013-0311-4
Såmark-Roth, A., Cox, D. M., Rudolph, D., Sarmiento, L. G., Albertsson, M., Carlsson, B. G., et al. (2023). Spectroscopy along flerovium decay chains. III. details on experiment, analysis, 282Cn, and spontaneous fission branches. Phys. Rev. C 107, 024301. doi:10.1103/PhysRevC.107.024301
Schwerdtfeger, P., and Seth, M. (2002). Relativistic quantum chemistry of the superheavy elements. Closed-shell element 114 as a case study. J. Nucl. Radiochem. Sci. 3 (1), 133–136. doi:10.14494/jnrs2000.3.133
Schwerdtfeger, P., Smits, O. R., and Pyykkö, P. (2020). The periodic table and the physics that drives it. Nat. Rev. Chem. 4, 359–380. doi:10.1038/s41570-020-0195-y
SCM (2024). Amsterdam modelling suite AMS ADF 2024.1, SCM, theoretical chemistry. Amsterdam, Netherlands: Vrije Universiteit. Available at: http://www.scm.com.
Semchenkov, A., Brüchle, W., Jäger, E., Schimpf, E., Schädel, M., Mühle, C., et al. (2008). The TransActinide separator and chemistry apparatus (TASCA) at GSI – optimization of ion-optical structures and magnet designs. Nucl. Instrum. Methods B 266, 4153–4161. doi:10.1016/j.nimb.2008.05.132
Serov, A., Eichler, R., Dressler, R., Piguet, D., Türler, A., Vögele, A., et al. (2013). Adsorption interaction of carrier-free thallium species with gold and quartz surfaces. Radiochim. Acta 101, 421–426. doi:10.1524/ract.2013.2045
Steinegger, P., Asai, M., Dressler, R., Eichler, R., Kaneya, Y., Mitsukai, A., et al. (2016). Vacuum chromatography of Tl on SiO2 at the single-atom level. J. Phys. Chem. C 120, 7122–7132. doi:10.1021/acs.jpcc.5b12033
Smits, O. R., Düllmann, Ch. E., Indelicato, P., Nazarewicz, W., and Schwerdtfeger, P. (2024). The quest for superheavy elements and the limit of the periodic table. Nat. Rev. Phys. 6, 86–98. doi:10.1038/s42254-023-00668-y
Te Velde, G., Bickelhaupt, F. M., Baerends, E. J., Fonseca Guerra, C., Van Gisbergen, S. J. A., Snijders, J. G., et al. (2001). Chemistry with ADF. J. Comput. Chem. 22 (9), 931–967. doi:10.1002/jcc.1056
Trombach, L., Ehlert, S., Grimme, S., Schwerdtfeger, P., and Mewes, J.-M. (2019). Exploring the chemical nature of super-heavy main-group elements by means of efficient plane-wave density-functional theory. Phys. Chem. Chem. Phys. 21 (33), 18048–18058. doi:10.1039/c9cp02455g
Türler, A., Eichler, R., and Yakushev, A. (2015). Chemical studies of elements with Z ≥ 104 in gas phase. Nucl. Phys. A 944, 640–689. doi:10.1016/j.nuclphysa.2015.09.012
Türler, A., and Pershina, V. (2013). Advances in the production and chemistry of the heaviest elements. Chem. Rev. 113 (2), 1237–1312. doi:10.1021/cr3002438
Van Lenthe, E., Baerends, E. J., and Snijders, J. G. (1994). Relativistic total energy using regular approximations. J. Chem. Phys. 101, 9783–9792. doi:10.1063/1.467943
Varentsov, V., and Yakushev, A. (2019). Concept of a new universal high-density gas stopping cell setup for study of gas-phase chemistry and nuclear properties of super heavy elements (UniCell). Nucl. Instrum. Methods A 940, 206–214. doi:10.1016/j.nima.2019.06.032
Węgrzecki, M., Bar, J., Budzyński, T., Cież, M., Grabiec, P., Kozłowski, R., et al. (2013). “Design and properties of silicon charged-particle detectors developed at the Institute of electron technology (ITE),” in Proc. SPIE 8902, Electron Technology Conference 2013, China, 25 July 2013. doi:10.1117/12.2031041
Wittwer, D., Abdullin, F.Sh., Aksenov, N. V., Albin, Yu. V., Bozhikov, G. A., Dmitriev, S. N., et al. (2010). Gas phase chemical studies of superheavy elements using the Dubna gas-filled recoil separator - stopping range determination. Nucl. Instr. Meth. B 268, 28–35. doi:10.1016/j.nimb.2009.09.062
Yakushev, A., and Eichler, R. (2016). Gas-phase chemistry of element 114, flerovium. EPJ Web Conf. 131, 07003. doi:10.1051/epjconf/201613107003
Yakushev, A., Gates, J. M., Türler, A., Schädel, M., Düllmann, Ch. E., Ackermann, D., et al. (2014). Superheavy element Flerovium (element 114) is a volatile metal. Inorg. Chem. 53, 1624–1629. doi:10.1021/ic4026766
Yakushev, A., Lens, L., Düllmann, Ch. E., Block, M., Brand, H., Dasgupta, M., et al. (2021). First study on nihonium (Nh, element 113) chemistry at TASCA. Front. Chem. 9, 753738. doi:10.3389/fchem.2021.753738
Yakushev, A., Lens, L., Düllmann, Ch. E., Khuyagbaatar, J., Jäger, E., Krier, J., et al. (2022). On the adsorption and reactivity of element 114, flerovium. Front. Chem. 10, 976635. doi:10.3389/fchem.2022.976635
Zhang, Y., Evans, J. R. G., and Yang, S. (2011). Corrected values for boiling points and enthalpies of vaporization of elements in handbooks. J. Chem. Eng. Data 56, 328–337. doi:10.1021/je1011086
Keywords: superheavy element chemistry, element 113–nihonium, element 115–moscovium, gas phase chromatography, recoil separators
Citation: Yakushev A, Khuyagbaatar J, Düllmann CE, Block M, Cantemir RA, Cox DM, Dietzel D, Giacoppo F, Hrabar Y, Iliaš M, Jäger E, Krier J, Krupp D, Kurz N, Lens L, Löchner S, Mokry C, Mošať P, Pershina V, Raeder S, Rudolph D, Runke J, Sarmiento LG, Schausten B, Scherer U, Thörle-Pospiech P, Trautmann N, Wegrzecki M and Wieczorek P (2024) Manifestation of relativistic effects in the chemical properties of nihonium and moscovium revealed by gas chromatography studies. Front. Chem. 12:1474820. doi: 10.3389/fchem.2024.1474820
Received: 02 August 2024; Accepted: 06 September 2024;
Published: 23 September 2024.
Edited by:
Xianglei Kong, Nankai University, ChinaReviewed by:
Andreas Türler, University of Bern, SwitzerlandChristian Tantardini, UiT The Arctic University of Norway, Norway
Susanta Lahiri, Saha Institute of Nuclear Physics (SINP), India
Copyright © 2024 Yakushev, Khuyagbaatar, Düllmann, Block, Cantemir, Cox, Dietzel, Giacoppo, Hrabar, Iliaš, Jäger, Krier, Krupp, Kurz, Lens, Löchner, Mokry, Mošať, Pershina, Raeder, Rudolph, Runke, Sarmiento, Schausten, Scherer, Thörle-Pospiech, Trautmann, Wegrzecki and Wieczorek. This is an open-access article distributed under the terms of the Creative Commons Attribution License (CC BY). The use, distribution or reproduction in other forums is permitted, provided the original author(s) and the copyright owner(s) are credited and that the original publication in this journal is cited, in accordance with accepted academic practice. No use, distribution or reproduction is permitted which does not comply with these terms.
*Correspondence: A. Yakushev, YS55YWt1c2hldkBnc2kuZGU=