- 1Bio-Organic Division, Bhabha Atomic Research Centre, Mumbai, India
- 2Homi Bhabha National Institute, Mumbai, India
- 3National Centre for Nanoscience and Nanotechnology, University of Mumbai, Mumbai, India
- 4Chemistry Division, Bhabha Atomic Research Centre, Mumbai, India
With the aim to develop a FRET-based viscosity sensor, two dyad molecules, 4 and 5, comprising tetraarylpyrrolo[3,2-b]pyrrole (TAPP) (donor) and naked boron-dipyrromethene (BODIPY) dyes (acceptor), were designed. Dyads were synthesized via acid-catalyzed multicomponent reactions followed by Sonogashira coupling. In both dyads, the BODIPY and TAPP moieties are linked through phenylethynyl groups, which allow free rotation of the BODIPY dyes; that is, they can act as molecular rotors. This was supported by X-ray crystallographic and DFT-optimized structures. Spectroscopic studies also confirmed the presence of both TAPP and BODIPY dyes in dyads with no electronic interactions that are suitable for fluorescence resonance energy transfer (FRET). Very high energy transfer efficiency (ETE >99%) from the donor TAPP moiety to the acceptor BODIPY moiety on excitation at the TAPP part was observed. However, due to the non-fluorescent nature of naked BODIPY dyes, no fluorescence emission was observed from the BODIPY moiety in both dyads. With increasing solvent viscosities, emission from the BODIPY moieties increases due to the restricted rotation of the BODIPY moieties. Plotting the logarithms of the fluorescent intensity of dyad 5 and the viscosity of the solution showed a good linear correlation obeying a Förster–Hoffmann equation. Non-fluorescent dyad 5 in methanol became greenish-yellow fluorescent in a methanol/glycerol (1:1) solvent. Furthermore, with an increase in the temperature of the methanol/glycerol (1:1) system, as the viscosity decreases, the fluorescence also starts decreasing. Thus, dyad 5 is capable of sensing the viscosity of the medium via a FRET-based “Off-On” mechanism. This type of viscosity sensor with a very large pseudo-Stokes shift and increased sensitivity will be useful for advancing chemo-bio sensing and imaging applications.
Introduction
Molecular dyads with a combination of a donor chromophore (absorbs at lower wavelengths) and an acceptor molecule (absorbs at higher wavelengths) have diverse applications in different fields ranging from artificial light-harvesting to advanced biotechnology (Altan Bozdemir et al., 2011; Odobel et al., 2013). A dyad with suitably matched optical properties of the donor and acceptor can act as a fluorescence resonance energy transfer (FRET) system. In this type of system, photoexcitation of the donor will lead to the transfer of its excitation energy to the acceptor, showing emission from the acceptor molecule at a higher wavelength (Lin et al., 2010). The difference between the wavelengths of emission maximum of the acceptor and the absorption maximum of the donor is a pseudo-Stokes shift, which is higher than a Stokes shift of the acceptor. These kinds of systems are important for energy tunneling in artificial light-harvesting antennas. In addition, the enhanced pseudo-Stokes shifts of these dyads are highly useful for developing chemical sensors (Guliyev et al., 2009; Qu et al., 2012) and advanced bio-imaging agents (Ulrich et al., 2005; Wu et al., 2009; Wu et al., 2020).
Viscosity is an important cellular parameter used to control the intracellular chemical signaling interactions of biomolecules and the diffusion of active metabolites within cellular systems. Intracellular viscosity changes are indicative of different diseases, such as hypertension, diabetes (Nadiv et al., 1994), atherosclerosis (Deliconstantinos et al., 1995), and Alzheimer’s disease (Zubenko et al., 1999). Consequently, finding novel techniques that could image subcellular viscosity in order to recognize problems linked to viscosity is immensely important. Thus, various viscosity sensors have been developed over the years (Su et al., 2017; Lee et al., 2018; Miao et al., 2019; Ma et al., 2020). The majority of these are fluorescence “Off-On” or “On-Off” types, and the accuracy of these sensors is limited by the self-absorption of the fluorophores. In that respect, the FRET-based system has a potential advantage because its large pseudo-Stokes shift will nullify the effect of self-absorption, and viscosity measurement depends on the relative orientation of the two independent chromophores where one (acceptor) molecule is sensitive and other (donor) is less sensitive towards viscosity response. Designing this type of FRET-based viscosity sensor requires a molecular pair as a dyad where the energy donor and acceptor are connected via a linker with an optimal distance.
Boron-dipyrromethene (BODIPY) dyes have emerged as a highly important class of dyes for numerous applications (Loudet and Burgess, 2007; Mula et al., 2008; Choudhary et al., 2024; Mula, 2024). This is because of their exceptional properties, like high molar absorptivity, high fluorescence, high photostability, and relatively easy customization (Loudet and Burgess, 2007; Ulrich et al., 2008). However, small Stokes shifts restrict their wide applicability in different applications, including chemo/biosensing and imaging. The majority of the chemo/bio-sensors and imaging agents developed using BODIPY dyes are “Off-On” or “On-Off” types (Boens et al., 2012; Gorai et al., 2022; Choudhary and Mula, 2023). A small Stokes shift makes the detection problematic in this kind of sensor mainly due to the self-absorption of the fluorescence light. Suitably designed dyads with large pseudo-Stokes shifts can be used for sensing purposes with enhanced sensitivity. Thus, the development of dyads with enhanced pseudo-Stokes shift along with high energy transfer efficiency (ETE) will be ideal for advanced sensing and imaging applications.
In the past, various BODIPY-based dyads were synthesized for solar energy harvesting purposes. In all these cases, BODIPYs have been extensively used both as energy donors and energy acceptors (Harriman et al., 2009; Bozdemir et al., 2010; Mula et al., 2010). Typically, BODIPYs have absorption in the green region (∼500 nm), and tuning it to the blue region is hardly possible (Hee Kim and Kim, 2019). Thus, very often, polycyclic aromatic hydrocarbons (PAHs) like pyrene, perylene, anthracene, triptycene, fluorene, etc., are attached to the BODIPY core as blue energy donors. These donors absorb in the blue region (200–400 nm) and efficiently transfer their excitation energy to the BODIPY core, enhancing the pseudo-Stokes shifts of BODIPY dyes (Ulrich et al., 2005; Iehl et al., 2012; Porcu et al., 2022). These types of dyads could be highly useful as sensing/imaging agents. From a synthetic point of view, these PAHs are difficult to synthesize or functionalize. The development of custom-made dyads based on a PAH-BODIPY frame is always challenging. Thus, introducing new fluorophores with absorption, emission in the blue region along with easy synthesis and functionalization procedures will be a real game changer.
A new class of fluorescent dye, namely, tetraarylpyrrolo[3,2-b]pyrrole (TAPP) (Chart 1), has been known since 2013 (Janiga et al., 2013; Krzeszewski et al., 2017). Recent developments showed that synthesizing these dyes is relatively easy compared to other PAHs, and importantly, there are ample scopes for post-functionalization of the TAPP core to synthesize advanced chromophores for diverse applications (Janiga et al., 2014; Liu et al., 2017; Krzeszewski et al., 2018). These dyes are being used for photochromic analysis of halocarbons, direct solvent probing via H-bonding interactions, two-photon absorption application, aggregation-induced emission, and development of resistive memory devices, MOFs, organic opto-electronics, and organic light-emitting diodes (OLEDs) (Wu et al., 2016; Dereka and Vauthey, 2017; Ji et al., 2017; Krzeszewski et al., 2017; Li et al., 2017; Hawes et al., 2018; Wang et al., 2018). TAPP has strong absorption at ∼400 nm and high blue emission (∼450 nm) (Tasior et al., 2020). Superior photophysical properties and easy synthesis prompted us to use these dyes as blue energy donors in molecular dyads. As the TAPP molecules are blue energy donors, we have taken the naked BODIPY 1 (Chart 1) dye as an acceptor because (i) its absorption profile has reasonable overlap with the emission profile of TAPP and (ii) it shows a molecular rotor property (Chakraborty et al., 2021). Due to the molecular rotor property, naked BODIPY dyes are being used as viscosity sensors, where these dyes showed “Off-On” fluorescence sensing with an increase in viscosity of the medium (Sunahara et al., 2007). A narrow Stokes shift remains a problem for these sensors in accurately detecting analytes. In this respect, viscosity detection is anticipated to be much easier with enhanced sensitivity if the naked BODIPY dye can be used in a dyad-based molecular rotor system. Thus, we have designed two TAPP-BODIPY-based dyads where TAPP and naked BODIPY dyes are linked through the C2/C5 of the TAPP moiety using a phenylethynyl linker. This linker will allow these two chromophores to remain electronically separated, allowing the free rotations of the chromophores (Chart 1). These dyads are expected to be non-fluorescent and to show enhanced fluorescence with increasing viscosity. In this report, the synthesis and characterization of two TAPP-BODIPY dyads are reported. Their photophysical properties and resonance energy transfer were investigated, and they showed highly efficient energy transfer from the TAPP to the BODIPY moiety. Finally, the potential application of these newly synthesized dyads as viscosity sensors is discussed.
Results and discussion
Synthesis
Initially, the acceptor, BODIPY 9, was synthesized. For the synthesis of BODIPY 9, pyrrole (6) was condensed with p-iodo benzaldehyde (7) in the presence of a catalytic amount of acid to form corresponding dipyrromethane (8). DDQ was used to oxidize 8 followed by its complexation with BF3. OEt2 furnished the acceptor BODIPY 9 (Scheme 1).
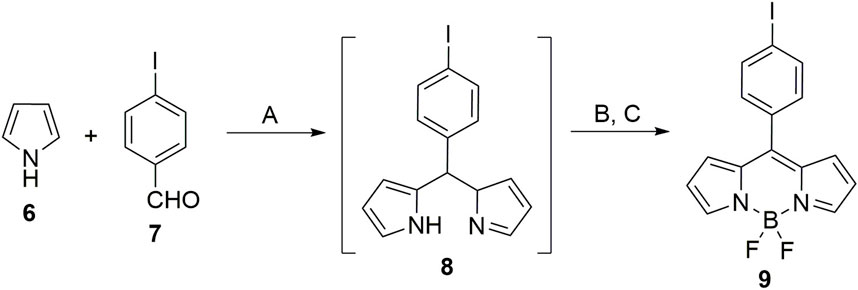
Scheme 1. Synthesis of acceptor BODIPY 9: Reaction conditions: (A) TFA (cat. amount), dry DCM, 25°C, 12 h; (B) DDQ, 25°C, 4 h; (C) NEt3, BF3. Et2O, 25°C, 12 h.
Next, the donor molecules TAPP 2 and 3 were synthesized via multicomponent condensation reactions. The acid-catalyzed reaction of p-methoxyaniline (10) and 4-((trimethylsilyl)ethynyl)benzaldehyde (12) formed the corresponding Schiff base, which was simultaneously condensed with 2,3-butanedione using Fe(ClO4)3.H2O as the catalyst to furnish TAPP 13. In another effort, p-methylaniline (11) and 4-((trimethylsilyl)ethynyl)benzaldehyde (12) were condensed in an acid-catalyzed reaction to generate the Schiff base, which was further condensed with 2,3-butanedione to furnish TAPP 14 in good yield (Tasior et al., 2020). Then, both TAPP 13 and 14 were desilylated to obtain the corresponding TAPP 2 and 3, respectively (Scheme 2). All the dyes were characterized by NMR spectroscopy and mass spectrometric analyses. For example, in 1H NMR spectrum of 13, phenyl protons were resonated as four doublets of four proton integration each in the aromatic region. Characteristic singlets for C-3/6 protons of the TAPP moiety and the methoxy and trimethyl silyl groups were observed at 6.34 ppm, 3.83 ppm, and 0.23 ppm, respectively, which confirmed the structure of 13 (Supplementary Figure S5). The disappearance of a singlet for the trimethylsilyl groups at 0.23 ppm and the appearance of a singlet at 3.08 ppm for the acetylenic protons confirm the formation of TAPP 3 (Supplementary Figure S11). Finally, the structures of TAPP 2 and 3 were confirmed by single-crystal X-ray crystallographic studies, as shown in Figure 1. Crystal structures of TAPP 2 and 3 showed that the pyrrolo[3,2-b]pyrrole units are planar, but the four aryl units remain out of plane, making them electronically non-conjugated with the pyrrolo[3,2-b]pyrrole units.
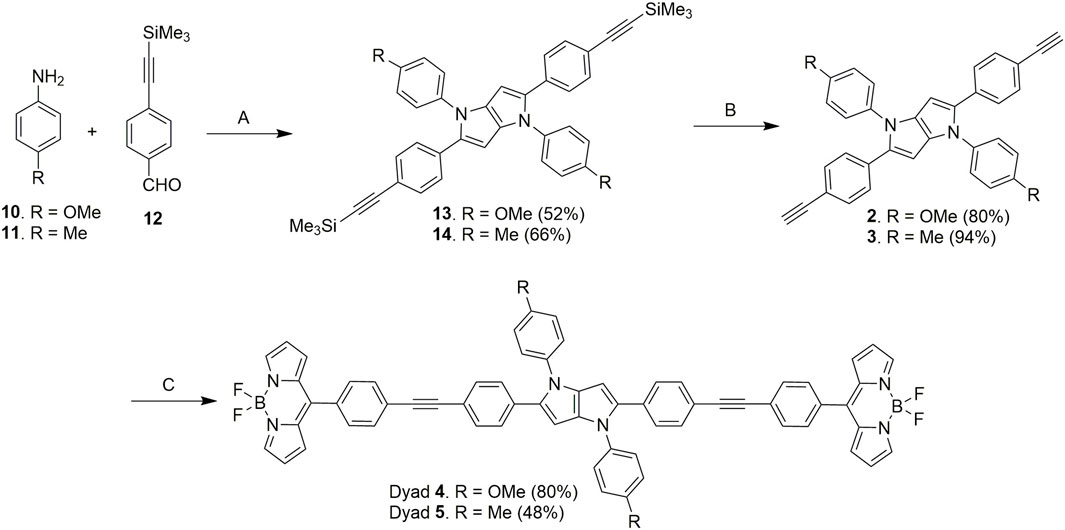
Scheme 2. Synthesis of TAPP 2, 3 and TAPP-BODIPY dyads 4, 5. Reaction conditions: (A) (i) AcOH: toluene, 50°C, 1 h; (ii) 2,3-butanedione, Fe(ClO4)3.H2O (6 mol%), 50°C, 12 h; (B) K2CO3, MeOH, 25°C, 6 h; (C) BODIPY 9, DIPA, trans-Pd(PPh3)2Cl2 (10 mol%), CuI (5 mol%), 25°C, dry THF, 12 h.
Finally, Sonogashira coupling of BODIPY 9 with TAPP 2 and 3 separately furnished dyads 4 and 5, respectively (Scheme 2). The structures of both dyads were confirmed by NMR spectroscopy and mass spectrometry analyses. In the 1H NMR spectrum of dyad 4, all the characteristic peaks for both the TAPP 2 and BODIPY 9 moieties were present (Figure 2; Supplementary Figure S15). The acetylenic proton signal of the TAPP 2 moiety was absent, which confirmed the coupling of the two moieties through the acetylenic bond.
DFT calculations
Orientations of the donors and acceptors in the dyads are important for efficient energy transfer between them. Thus, DFT studies were done to optimize the ground state geometries of the dyads 4 and 5 using the B3LYP/6-31G level of theory. These are shown in Table 1; Supplementary Table S4. In both dyads, the pyrrolo-pyrrole units are planar as expected, and the four attached aryl rings remain twisted with respect to the pyrrolo-pyrrole plane. Similar observations were found from the X-ray crystallographic structures discussed vide supra. Furthermore, BODIPY moieties are planar, but the attached 8-phenyl groups are twisted with respect to the BODIPY core. Thus, as a whole, in both dyads, the donor TAPP moieties and the acceptor BODIPYs are twisted with respect to each other; that is, they are not electronically conjugated. This suggests that energy transfer is possible in both dyads 4 and 5. The HOMO-LUMO structures and energies of both dyads are also calculated and tabulated in Table 1; Supplementary Table S4. None of the FMO’s electron densities are distributed over both chromophores. The electron densities in the HOMOs of both dyads are located on the pyrrolo-pyrrole unit and shifted towards the BODIPY core in LUMOs. These also indicate that the TAPP and BODIPY moieties are not conjugated; thus, energy transfer is feasible in both dyads, as discussed below.
Steady-state photophysical study
Steady-state absorption and fluorescence properties of dyads 4 and 5 were recorded in methanol and compared with that of acceptor BODIPY 1 and donors TAPP 2 and 3. Different photophysical parameters are tabulated in Table 2, and their absorption and fluorescence spectra are shown in Figures 3, 4, respectively.
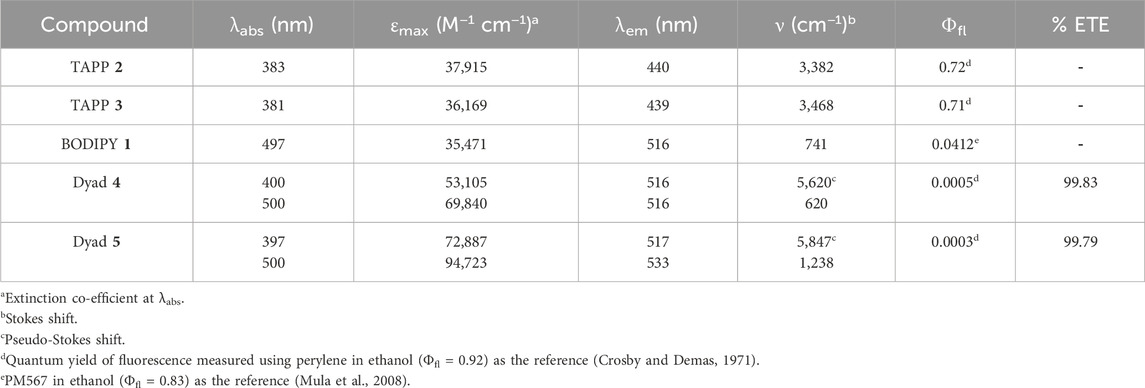
Table 2. Absorption and emission of acceptor BODIPY 1, donors TAPP 2, 3, and dyads 4, 5 in methanol.
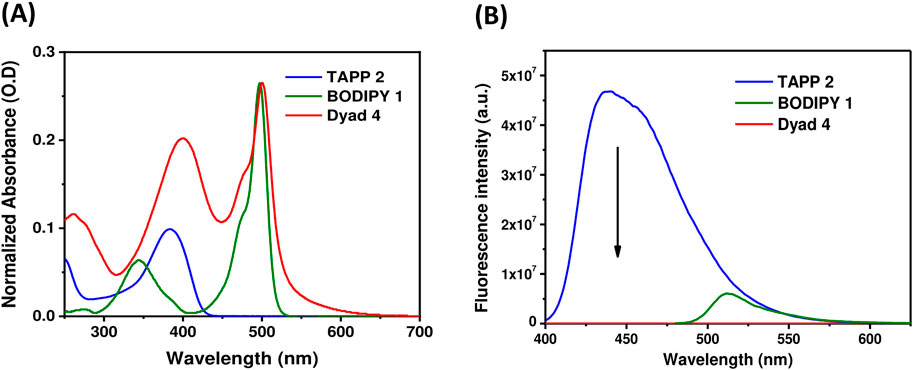
Figure 3. (A) UV-vis absorption spectra of TAPP 2, BODIPY 1, and dyad 4 in methanol (1.4–3.4 × 10−6 M); (B) Fluorescence spectra (O.D. corrected) of TAPP 2, dyad 4 (λex = 395 nm), and BODIPY 1 (λex = 475 nm) in methanol.
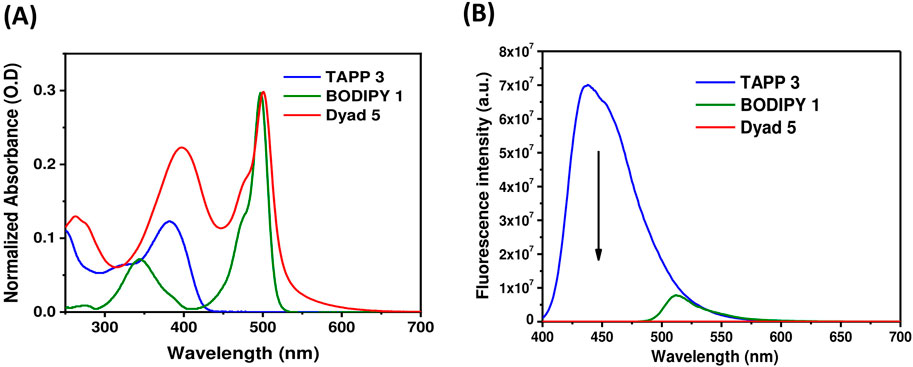
Figure 4. (A) UV-vis absorption spectra of TAPP 3, BODIPY 1, and dyad 5 in methanol (1.4–3.4 × 10−6 M); (B) Fluorescence spectra (O.D. corrected) of TAPP 3, dyad 5 (λex = 395 nm), and BODIPY 1 (λex = 475 nm) in methanol.
Donors TAPP 2 and 3 showed the longest absorption maximum (λabs) at ∼382 nm and high blue fluorescence (Φfl = 0.72), with a fluorescence maximum (λfl) at ∼440 nm; that is, the Stokes shifts of both the TAPPs are large (∼3,400 cm−1). On the other hand, the absorption and fluorescence spectra of acceptor BODIPY 1 are red-shifted compared to TAPP 2 and 3. The λabs of BODIPY 1 is at 497 nm, and it showed greenish fluorescence with λem at 516 nm. Importantly, the fluorescence of BODIPY 1 is quenched (Φfl = 0.04) because of high non-radiative decay due to the free rotation of the C-8 phenyl ring (Sunahara et al., 2007).
Photophysical property studies of dyad 4 showed that absorption spectra contain λabs peaks of both donor and acceptor moieties at 400 nm and 500 nm, respectively (Figure 3; Table 2). This indicates no/less electronic conjugation between the TAPP (donor) and the BODIPY (acceptor) moieties as seen in the DFT optimized structure discussed vide supra. In fluorescence studies, when the TAPP moiety was excited at 395 nm, no characteristic blue fluorescence of the TAPP moiety or greenish-yellow fluorescence of BODIPY was observed. Complete quenching of TAPP fluorescence clearly indicates an efficient transfer of TAPP excitation energy to the acceptor BODIPY dyes. Due to the non-fluorescent nature of the acceptor BODIPY as discussed vide supra, emission of the BODIPY dyes was also not observed. The energy transfer efficiency was calculated from the decrease in the donor TAPP fluorescence, which showed extremely high energy transfer (99.83%) from TAPP to the BODIPY moiety.
Similar to dyad 4, dyad 5 also showed absorption spectra containing λabs peaks of both donor and acceptor moieties at 397 nm and 500 nm, respectively (Figure 4; Table 2). These prove that in dyad 5, the donor and acceptor moieties are not electronically conjugated as also predicted from DFT optimized structures discussed vide supra. While exciting dyad 5 at the donor part (395 nm), no fluorescence was observed either from the donor part or from the acceptor part. The drastic decrease in TAPP fluorescence clearly indicates an efficient transfer of TAPP excitation energy to the acceptor BODIPY dyes, as also seen in the case of dyad 4. As the acceptor BODIPY is non-fluorescent in nature, emission of the BODIPY dyes was also not observed. The energy transfer efficiency was calculated from the decrease in the donor fluorescence, which showed extremely high energy transfer (99.79%) from TAPP to the BODIPY moiety.
Viscosity sensing study of dyad 5
Next, the viscosity-sensing ability of these TAPP-BODIPY dyads was investigated. For this, the absorbance and fluorescence of dyad 5 were checked in solvents with higher viscosity, that is, in ethanol, 1-propanol, 1-butanol, 1-nonanol, 1-decanol, and methanol/glycerol mixtures, and these properties were compared with those determined in low polar methanol as discussed before. The absorption spectra of dyad 5 in different n-alcohols and glycerol-methanol (1:1) mixtures were similar to that of methanol (Supplementary Figure S1). However, remarkable changes were observed in fluorescence studies (Figures 5A, B). With excitation at the TAPP moiety (λex = 395 nm), the fluorescence emission from the BODIPY moiety was increased sharply in both 1-decanol and the methanol/glycerol (1:1) mixture, showing greenish-yellow fluorescence (Figures 5A, B, D) compared to its non-fluorescent nature in methanol. In more viscous solvents, intramolecular rotation along C-C bonds connecting TAPP and BODIPY dyes decreases; thus, emission from the BODIPY moiety is enhanced. Importantly, fluorescence enhancement in both the solvents was similar when excited at TAPP as well as the BODIPY moiety in dyad 5. This also confirmed the efficient energy transfer from the TAPP moiety to the BODIPY moiety. In different alcohols as well as in methanol/glycerol mixtures, the fluorescence intensities of dyad 5 increased with an increase in their viscosities. Interestingly, in both studies, the logarithm of fluorescent intensity and the logarithm of viscosity of solution obeyed a linear relationship as per the Förster–Hoffmann equation (Förster and Hoffmann, 1971; Koenig et al., 2016). Furthermore, the temperature-dependent fluorescence of dyad 5 was measured in methanol and a glycerol-methanol (1:1) mixture. It was observed that with increasing temperature of the glycerol-methanol (1:1) mixture, emission from the BODIPY moiety decreases (Figure 5C). (Sen et al., 2022) Now, with increasing temperature, the viscosity of the glycerol-methanol (1:1) mixture decreases; thus, the emission from the BODIPY moiety also decreases (Figure 5C). On the other hand, no/very little change in the fluorescence of dyad 5 is observed with increasing temperature in pure methanol, as the temperature-dependent viscosity change in methanol is negligible (Supplementary Figure S4). This clearly indicates that the fluorescence properties of dyad 5 depend on the viscosity of the medium. This showed the ability of dyad 5 to sense the viscosity as anticipated.
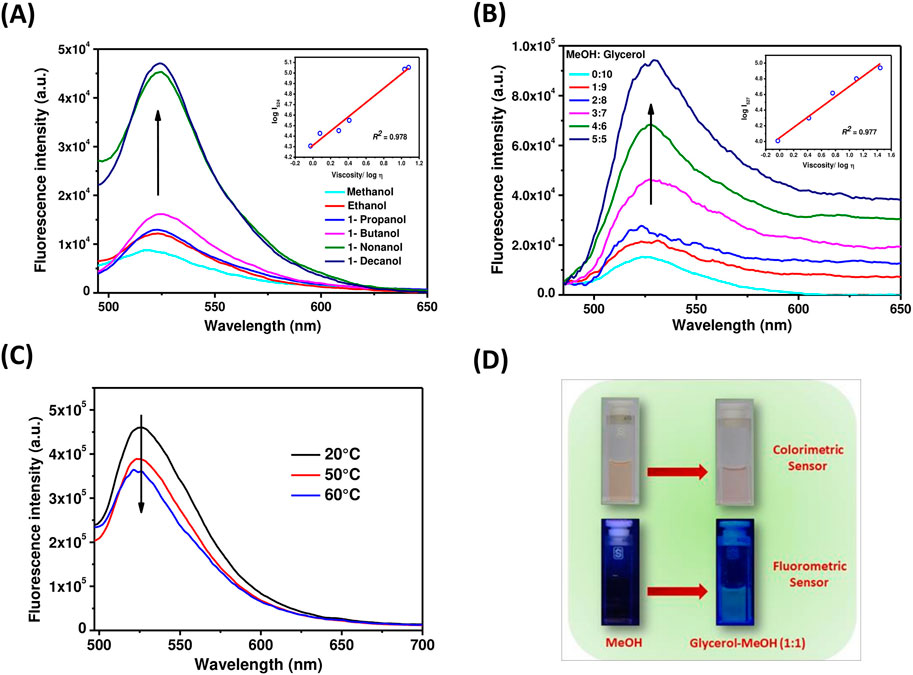
Figure 5. (A) Emission spectra of dyad 5 in methanol, ethanol, 1-propanol, 1-butanol, 1-nonanol, and 1-decanol (λex = 395 nm) at 25°C; inset: The linear response between log I524 and log η in different n-alcohols. (B) Emission spectra of dyad 5 in different ratios of a glycerol-methanol mixture (λex = 395 nm) at 25°C; inset: The linear response between log I527 and log η in different glycerol–methanol mixtures. (C) Temperature-dependent fluorescence of dyad 5 in a glycerol–methanol mixture (1:1) (λex = 395 nm). (D) Color change of dyad 5 solutions in a methanol and glycerol–methanol mixture (1:1) under visible and UV light.
Conclusion
Two TAPP-BODIPY dyads, 4 and 5, were developed as molecular rotors in which two naked BODIPY dyes (acceptors) are linked with TAPP moieties (donors) through the C2 and C5 positions via phenylethynyl linkers. X-ray crystallographic and theoretical studies showed that both the TAPP and BODIPY moieties are twisted with respect to each other; that is, they are not electronically conjugated. This is also confirmed by spectroscopic studies. Fluorescence studies showed highly efficient energy transfer from the donor TAPP moiety to the acceptor BODIPY moiety on excitation at the TAPP part. Due to the non-fluorescent characteristics of the naked BODIPY dyes, no fluorescence emission was observed from the BODIPY moiety. With the increase in solvent viscosity, the free rotations of the BODIPY dyes were restricted, and high emissions from the BODIPY moieties were observed. For example, the absorbance and fluorescence of dyad 5 were checked in solvents with higher viscosity, that is, in n-alcohols and methanol/glycerol (1:1) systems. On excitation at the TAPP moiety (λex = 395 nm), a remarkable greenish-yellow fluorescence was observed from the BODIPY moiety. Furthermore, with an increase in the temperature of the methanol/glycerol (1:1) system, the fluorescence started decreasing due to the lowering of the viscosity. All these observations confirmed that the dyad 5 is capable of sensing the viscosity of the medium via the FRET-based Off-On mechanism. This type of viscosity sensor with a very large pseudo-Stokes shift will be useful for advanced chemo-bio sensing and imaging applications.
Experimental section
General methods and materials
The detailed experimental methods and the data for the characterization of synthesized compounds (1H and 13C NMR spectrum) are given in the Supplementary Material.
General procedure for the synthesis of TAPP 13 and 14
p-Methoxy/methyl aniline (1 mmol) and 4-((trimethylsilyl)ethynyl) benzaldehyde (1 mmol) were mixed in glacial acetic acid/toluene (1:10, 4 mL), and the mixture was heated at 50°C for 1 h in a 50 mL Schlenk tube. Then, Fe(ClO4)3·xH2O (0.03 mmol) and 2,3-butadione (0.5 mmol) were added, and the mixture was heated again at 90°C for 12 h. Next, the crude product was dried under reduced pressure and subjected to column chromatography (silica gel, DCM/petroleum ether, 30:70) to furnish the pure products TAPP 13/14, respectively.
1,4-Bis(4-methoxyphenyl)-2,5-bis(4((trimethylsilyl)ethynyl)phenyl)-1,4-dihydropyrrolo[3,2-b]pyrrole (TAPP 13)
TAPP 13 was synthesized by following the general procedure using p-methoxyaniline (123 mg, 1.0 mmol), 4-((trimethylsilyl)ethynyl)benzaldehyde (202 mg, 1.0 mmol), 2,3-butadione (43 μL, 0.5 mmol), and Fe(ClO4)3 .H2O (11 mg, 0.03 mmol). Pure TAPP 13 was obtained as a yellow solid. Yield: 344 mg, (52%), Rf = 0.75 (DCM/hexane, 30:70, v/v); 1H NMR (500 MHz, CDCl3): δ 0.23 (s, 18H), 3.83 (s, 6H), 6.35 (s, 2H), 6.89 (d, J = 8.0 Hz, 4H), 7.12 (d, J = 7.0 Hz, 4H), 7.18 (d, J = 8.0 Hz, 4H), 7.30 (d, J = 8.5 Hz, 4H) ppm; 13C NMR (125 MHz, CDCl3): δ -0.03, 55.5, 94.1, 94.6, 105.3, 114.4, 120.2, 126.6, 127.5, 131.8, 132.5, 132.9, 133.6, 135.6, 157.7 ppm; HRMS (ESI/Q-TOF) m/z: [M + H]+ Calcd for C42H43N2O2Si2 663.2857; Found 663.2821.
1,4-Bis(4-methylphenyl)-2,5-bis(4((trimethylsilyl)ethynyl)phenyl)-1,4-dihydropyrrolo[3,2-b]pyrrole (TAPP 14)
TAPP 14 was synthesized by following the general procedure using p-methylaniline (107 mg, 1.0 mmol), 4-((trimethylsilyl)ethynyl) benzaldehyde (202 mg, 1.0 mmol), 2,3-butanedione (43 μL, 0.5 mmol), and Fe(ClO4)3.H2O (11 mg, 0.03 mmol). Pure TAPP 14 was obtained as a yellow solid. Yield: 208 mg (66%); Rf = 0.45 (DCM/hexane, 30:70, v/v); 1H NMR (500 MHz, CDCl3): δ 0.23 (s, 18H), 2.37 (s, 6H), 6.38 (s, 2H), 7.12–7.15 (m, 12H), 7.30 (d, J = 8.1 Hz, 4H) ppm; 13C NMR (125 MHz, CDCl3): δ -0.03, 20.9, 94.5, 94.8, 105.3, 120.3, 125.1, 127.6, 129.8, 131.7, 132.4, 133.7, 135.5, 135.7, 137.3 ppm; HRMS (ESI/Q-TOF) m/z: [M + H]+ Calcd for C42H43N2Si2 631.2959; Found 631.2964.
General procedure for the synthesis of TAPP 2 and 3
TAPP 13/14 (0.16 mmol) and K2CO3 (0.5 mmol) were mixed in dry MeOH:DCM (1:1, 10 mL), and the mixture was stirred at room temperature for 24 h. The reaction mixture turned yellow, was extracted with dichloromethane (3 × 30 mL), and washed with saturated aq. NaHCO3 (30 mL), brine (30 mL) and water (30 mL). The organic layer was dried over anhydrous Na2SO4 and evaporated under reduced pressure. The crude product was purified by column chromatography (silica gel, DCM/petroleum ether, 50:50) to furnish the pure products TAPP 2/3, respectively.
2,5-Bis(4-ethynylphenyl)-1,4-bis(4-methoxyphenyl)-1,4-dihydropyrrolo[3,2-b]pyrrole (TAPP 2)
TAPP 2 was synthesized by following the general procedure using TAPP 13 (105 mg, 0.16 mmol) and K2CO3 (68 mg, 0.5 mmol). Pure TAPP 2 was obtained as a yellow solid. Yield: 67 mg (80%); Rf = 0.64 (DCM/hexane, 40:60, v/v); 1H NMR (500 MHz, CDCl3): δ 3.08 (s, 2H), 3.84 (s, 6H), 6.36 (s, 2H), 6.91 (d, J = 9.0 Hz, 4H), 7.15 (d, J = 8.5 Hz, 4H), 7.20 (d, J = 9.0 Hz, 4H), 7.33 (d, J = 8.5 Hz, 4H) ppm; 13C NMR (125 MHz, CDCl3): δ 55.5, 77.5, 83.8, 94.3, 114.5, 119.3, 126.6, 127.6, 131.9, 132.6, 132.9, 134.0, 135.6, 157.8 ppm; HRMS (ESI/Q-TOF) m/z: [M + H]+ Calcd for C36H27N2O2 519.2067; Found 519.2076.
2,5-Bis(4-ethynylphenyl)-1,4-di-(4-methylphenyl)-1,4-dihydropyrrolo[3,2-b]pyrrole (TAPP 3)
TAPP 3 was synthesized by following the general procedure using TAPP 14 (101 mg, 0.16 mmol) and K2CO3 (69 mg, 0.5 mmol). Pure TAPP 3 was obtained as a yellow solid. Yield: 73 mg (94%); Rf = 0.46 (DCM/hexane, 30:70, v/v); 1H NMR (500 MHz, CDCl3): δ 2.38 (s, 6H), 3.07 (s, 2H)s, 6.39 (s, 2H), 7.15–7.17 (m, 12H), 7.33 (d, J = 8.3 Hz, 4H) ppm; 13C NMR (125 MHz, CDCl3): δ 21.0, 77.5, 83.8, 94.9, 119.3, 125.1, 127.6, 129.8, 131.9, 132.4, 134.0, 135.4, 135.8, 137.3 ppm; HRMS (ESI/Q-TOF) m/z: [M + H]+ Calcd for C36H27N2 487.2168; Found 487.2163.
4,4-Difluoro-8-(4′-iodophenyl)-4-bora-3a,4a-diaza-s-indecene (9)
A mixture of 4-iodobenzaldehyde (7) (700 mg, 3.0 mmol), an excess of pyrrole (6) (9 mL, 120.6 mmol), and trifluoroacetic acid (5 drops) was stirred at 25°C for 1 day. Excess pyrrole was distilled off, and the residue was purified by flash column chromatography (silica gel, ethyl acetate/petroleum ether, 20:80) to obtain dipyrromethane 8 (770 mg, 73%) as a cream color solid. Then, 8 (770 mg, 2.2 mmol) was dissolved in dry DCM, DDQ (753 mg, 3.3 mmol) was added into it, and the resulting mixture was stirred at 25°C for 4 h. Next, NEt3 (1.8 mL, 13 mmol) and BF3.OEt2 (1.6 mL, 13 mmol) were added, and stirring was continued for another 12 h. The reaction mixture was quenched with sat. NaHCO3 solution (50 mL), extracted with dichloromethane (100 mL), washed with water (3 × 25 mL), and dried with Na2SO4. The organic layer was concentrated in vacuo, and the crude product was purified by flash column chromatography (silica gel, ethyl acetate/petroleum ether, 5:95) to obtain BODIPY 9 as a dark orange solid (Betancourt-Mendiola et al., 2015). Yield: 159 mg (18%); Rf = 0.65 (ethyl acetate/hexane, 25: 75, v/v); 1H NMR (500 MHz, CDCl3): δ 6.56 (d, J = 4.2 Hz, 2H), 6.90 (d, J = 4.3 Hz, 2H), 7.30 (d, J = 8.3 Hz, 2H), 7.89 (d, J = 8.3 Hz, 2H), 7.95 (s, 2H) ppm; 13C NMR (125 MHz, CDCl3): δ 97.5, 118.8, 131.3, 131.9, 133.1, 134.6, 137.7, 144.5, 145.9 ppm; HRMS (ESI/Q-TOF) m/z: [M + H]+ Calcd for C15H11BF2IN2 395.0022; Found 395.0021.
General procedure for the synthesis of dyads 4 and 5
TAPP 2/3 (0.05 mmol), BODIPY 9 (0.10 mmol), and di-isopropyl amine (0.5 mL) were dissolved in dry THF (6 mL), and the solution was degassed properly. Pd(PPh3)2Cl2 (3.5 mg, 0.005 mmol) and CuI (1 mg, 0.005 mmol) were added, and the reaction was stirred at 25°C for 24 h. Removal of the solvent in vacuo followed by column chromatography of the residue (silica gel, DCM/petroleum ether, 70:30) furnished dyads 4/5, respectively.
Dyad 4
Dyad 4 was synthesized by following the general procedure using TAPP 2 (26 mg, 0.05 mmol) and BODIPY 1 (42 mg, 0.1 mmol). Pure dyad 4 was obtained as a dark red solid. Yield: 40 mg (80%). Rf = 0.35 (DCM/hexane, 70:30, v/v); 1H NMR (500 MHz, CDCl3): δ 3.86 (s, 6H), 6.42 (s, 2H), 6.57 (d, J = 7.4 Hz, 4H), 6.93–6.96 (m, 8H), 7.23–7.24 (m, 8H), 7.42 (d, J = 7.4 Hz, 4H), 7.56 (d, J = 7.7 Hz, 4H), 7.65 (d, J = 7.7 Hz, 4H), 7.96 (s, 4H) ppm; 13C NMR (125 MHz, CDCl3): δ 55.5, 88.9, 92.6, 94.4, 114.5, 118.7, 119.8, 126.3, 126.7, 127.7, 130.6, 131.4, 131.5, 131.6, 132.9, 133.3, 134.7, 144.3, 146.5, 157.9 ppm; MS (MALDI-TOF): m/z [M]+ Calcd for C66H44B2F4N6O2: 1,050.4; Found 1,050.1.
Dyad 5
Dyad 5 was synthesized by following the general procedure using TAPP 3 (25 mg, 0.05 mmol) and BODIPY 1 (42 mg, 0.10 mmol). Pure dyad 5 was obtained as a dark red solid. Yield: 24 mg (48%); Rf = 0.45 (DCM/hexane, 70:30, v/v); 1H NMR (500 MHz, CDCl3): δ 2.41 (s, 6H), 6.45 (s, 2H), 6.56 (d, J = 7.4 Hz, 4H), 6.95 (d, J = 7.6 Hz, 4H), 7.19–7.21 (m, 8H), 7.23 (d, J = 8.1 Hz, 4H), 7.42 (d, J = 8.1 Hz, 4H), 7.56 (d, J = 7.9 Hz, 4H), 7.65 (d, J = 7.9 Hz, 4H), 7.95 (s, 4H) ppm; 13C NMR (125 MHz, CDCl3): δ 21.0, 88.9, 92.6, 94.9, 118.7, 119.8, 125.1, 126.3, 127.7, 129.9, 130.5, 131.3, 131.4, 131.5, 132.6, 133.3, 133.9, 134.7, 135.5, 135.8, 137.3, 144.2, 146.4 ppm. HRMS (ESI/Q-TOF) m/z: [M + H]+ Calcd for C66H45B2F4N6 1,019.3822; Found 1,019.3820.
Data availability statement
The original contributions presented in the study are included in the article/Supplementary Material; further inquiries can be directed to the corresponding author.
Author contributions
RA: formal analysis, writing–original draft, data curation, investigation, and methodology. SG: formal analysis, investigation, methodology, and writing–review and editing. SY: formal analysis, investigation, and writing–review and editing. AW: investigation, writing–review and editing, and formal analysis. SM: writing–review and editing, conceptualization, supervision, and methodology.
Funding
The author(s) declare that financial support was received for the research, authorship, and/or publication of this article. This work is supported by the Department of Atomic Energy, Govt. of India (Project no. R&D-040-2018).
Acknowledgments
The authors gratefully acknowledge Dr. Rajib Ghosh and Dr. Goutam Chakraborty of BARC for their helpful assistance in photophysical studies.
Conflict of interest
The authors declare that the research was conducted in the absence of any commercial or financial relationships that could be construed as a potential conflict of interest.
Publisher’s note
All claims expressed in this article are solely those of the authors and do not necessarily represent those of their affiliated organizations, or those of the publisher, the editors, and the reviewers. Any product that may be evaluated in this article, or claim that may be made by its manufacturer, is not guaranteed or endorsed by the publisher.
Supplementary material
The Supplementary Material for this article can be found online at: https://www.frontiersin.org/articles/10.3389/fchem.2024.1473769/full#supplementary-material
References
Altan Bozdemir, O., Erbas-Cakmak, S., Ekiz, O. O., Dana, A., and Akkaya, E. U. (2011). Towards unimolecular luminescent solar concentrators: bodipy-based dendritic energy-transfer cascade with panchromatic absorption and monochromatized emission. Angew. Chem. Int. Ed. 50, 10907–10912. doi:10.1002/anie.201104846
Betancourt-Mendiola, L., Valois-Escamilla, I., Arbeloa, T., Bañuelos, J., López Arbeloa, I., Flores-Rizo, J. O., et al. (2015). Scope and limitations of the liebeskind–srogl cross-coupling reactions involving the biellmann BODIPY. J. Org. Chem. 80, 5771–5782. doi:10.1021/acs.joc.5b00731
Boens, N., Leen, V., and Dehaen, W. (2012). Fluorescent indicators based on BODIPY. Chem. Soc. Rev. 41, 1130–1172. doi:10.1039/c1cs15132k
Bozdemir, O. A., Cakmak, Y., Sozmen, F., Ozdemir, T., Siemiarczuk, A., and Akkaya, E. U. (2010). Synthesis of symmetrical multichromophoric bodipy dyes and their facile transformation into energy transfer cassettes. Chem. Eur. J. 16, 6346–6351. doi:10.1002/chem.200903449
Chakraborty, G., Choudhary, M. K., Sundararajan, M., Ray, A. K., Mula, S., and Pal, H. (2021). Stimuli responsive confinement of a molecular rotor based BODIPY dye inside a cucurbit[7]uril nanocavity. J. Phys. Chem. B 125, 7946–7957. doi:10.1021/acs.jpcb.1c02443
Choudhary, M. K., Gorai, S., Patro, B. S., and Mula, S. (2024). Imino-BODIPY-based, highly sensitive, fluorescent dosimeter for low-dose gamma radiation. ChemPhotoChem 8, e202300245. doi:10.1002/cptc.202300341
Choudhary, M. K., and Mula, S. (2023). Development of a BODIPY-based ratiometric fluorescence off-on dosimeter for gamma radiation. New J. Chem. 47, 9045–9049. doi:10.1039/d3nj01036h
Crosby, G. A., and Demas, J. N. (1971). Measurement of photoluminescence quantum yields. Review. J. Phys. Chem. 75, 991–1024. doi:10.1021/j100678a001
Deliconstantinos, G., Villiotou, V., and Stavrides, J. C. (1995). Modulation of particulate nitric oxide synthase activity and peroxynitrite synthesis in cholesterol enriched endothelial cell membranes. Biochem. Pharmacol. 49, 1589–1600. doi:10.1016/0006-2952(95)00094-g
Dereka, B., and Vauthey, E. (2017). Direct local solvent probing by transient infrared spectroscopy reveals the mechanism of hydrogen-bond induced nonradiative deactivation. Chem. Sci. 8, 5057–5066. doi:10.1039/c7sc00437k
Förster, Th., and Hoffmann, G. (1971). Die Viskositätsabhängigkeit der Fluoreszenzquantenausbeuten einiger Farbstoffsysteme. Z. Phys. Chem. 75, 63–76. doi:10.1524/zpch.1971.75.1_2.063
Gorai, S., Ghosh, A., Chakraborty, S., Retailleau, P., Ghanty, T. K., Patro, B. S., et al. (2022). Fluorescent Cu2+ sensor based on phenanthroline-BODIPY conjugate: a mechanistic study. Dyes Pigm 203, 110343. doi:10.1016/j.dyepig.2022.110343
Guliyev, R., Coskun, A., and Akkaya, E. U. (2009). Design strategies for ratiometric chemosensors: modulation of excitation energy transfer at the energy donor site. J. Am. Chem. Soc. 131, 9007–9013. doi:10.1021/ja902584a
Harriman, A., Mallon, L. J., Elliot, K. J., Haefele, A., Ulrich, G., and Ziessel, R. (2009). Length dependence for intramolecular energy transfer in three- and four-color Donor−Spacer−Acceptor arrays. J. Am. Chem. Soc. 131, 13375–13386. doi:10.1021/ja9038856
Hawes, C. S., Ó Máille, G. M., Byrne, K., Schmitt, W., and Gunnlaugsson, T. (2018). Tetraarylpyrrolo[3,2-b]pyrroles as versatile and responsive fluorescent linkers in metal–organic frameworks. Dalton Trans. 47, 10080–10092. doi:10.1039/c8dt01784k
Hee Kim, N., and Kim, D. (2019). “Blue-emitting BODIPY dyes,” in BODIPY dyes - a privilege molecular scaffold with tunable properties. Editors J. Bañuelos-Prieto, and R. Sola Llano (Rijeka: IntechOpen).
Iehl, J., Nierengarten, J.-F., Harriman, A., Bura, T., and Ziessel, R. (2012). Artificial light-harvesting arrays: electronic energy migration and trapping on a sphere and between spheres. J. Am. Chem. Soc. 134, 988–998. doi:10.1021/ja206894z
Janiga, A., Bednarska, D., Thorsted, B., Brewer, J., and Gryko, D. T. (2014). Quadrupolar, emission-tunable π-expanded 1,4-dihydropyrrolo[3,2-b]pyrroles – synthesis and optical properties. Org. Biomol. Chem. 12, 2874–2881. doi:10.1039/c4ob00143e
Janiga, A., Glodkowska-Mrowka, E., Stoklosa, T., and Gryko, D. T. (2013). Synthesis and optical properties of tetraaryl-1,4-dihydropyrrolo[3,2-b]pyrroles. Asian J. Org. Chem. 2, 411–415. doi:10.1002/ajoc.201200201
Ji, Y., Peng, Z., Tong, B., Shi, J., Zhi, J., and Dong, Y. (2017). Polymorphism-dependent aggregation-induced emission of pyrrolopyrrole-based derivative and its multi-stimuli response behaviors. Dyes Pigm 139, 664–671. doi:10.1016/j.dyepig.2016.12.061
Koenig, M., Storti, B., Bizzarri, R., Guldi, D. M., Brancato, G., and Bottari, G. (2016). A fluorescent molecular rotor showing vapochromism, aggregation-induced emission, and environmental sensing in living cells. J. Mater. Chem. C 4, 3018–3027. doi:10.1039/c5tc03541d
Krzeszewski, M., Gryko, D., and Gryko, D. T. (2017). The tetraarylpyrrolo[3,2-b]pyrroles—from serendipitous discovery to promising heterocyclic optoelectronic materials. Acc. Chem. Res. 50, 2334–2345. doi:10.1021/acs.accounts.7b00275
Krzeszewski, M., Sahara, K., Poronik, Y. M., Kubo, T., and Gryko, D. T. (2018). Unforeseen 1,2-aryl shift in tetraarylpyrrolo[3,2-b]pyrroles triggered by oxidative aromatic coupling. Org. Lett. 20, 1517–1520. doi:10.1021/acs.orglett.8b00223
Lee, S. C., Heo, J., Woo, H. C., Lee, J. A., Seo, Y. H., Lee, C. L., et al. (2018). Fluorescent molecular rotors for viscosity sensors. Chem. Eur. J. 24, 13706–13718. doi:10.1002/chem.201801389
Li, K., Liu, Y., Li, Y., Feng, Q., Hou, H., and Tang, B. Z. (2017). 2,5-bis(4-alkoxycarbonylphenyl)-1,4-diaryl-1,4-dihydropyrrolo[3,2-b]pyrrole (AAPP) AIEgens: tunable RIR and TICT characteristics and their multifunctional applications. Chem. Sci. 8, 7258–7267. doi:10.1039/c7sc03076b
Lin, W., Yuan, L., Cao, Z., Feng, Y., and Song, J. (2010). Through-bond energy transfer cassettes with minimal spectral overlap between the donor emission and acceptor absorption: coumarin–rhodamine dyads with large pseudo-Stokes shifts and emission shifts. Angew. Chem. Ed. 49, 375–379. doi:10.1002/anie.200904515
Liu, H., Ye, J., Zhou, Y., Fu, L., Lu, Q., and Zhang, C. (2017). New pyrrolo[3,2-b]pyrrole derivatives with multiple-acceptor substitution: efficient fluorescent emission and near-infrared two-photon absorption. Tetrahedron Lett. 58, 4841–4844. doi:10.1016/j.tetlet.2017.11.028
Loudet, A., and Burgess, K. (2007). BODIPY dyes and their derivatives: syntheses and spectroscopic properties. Chem. Rev. 107, 4891–4932. doi:10.1021/cr078381n
Ma, C., Sun, W., Xu, L., Qian, Y., Dai, J., Zhong, G., et al. (2020). A minireview of viscosity-sensitive fluorescent probes: design and biological applications. J. Mater. Chem. B 8, 9642–9651. doi:10.1039/d0tb01146k
Miao, W., Yu, C., Hao, E., and Jiao, L. (2019). Functionalized BODIPYs as fluorescent molecular rotors for viscosity detection. Front. Chem. 7, 825. doi:10.3389/fchem.2019.00825
Mula, S. (2024). BODIPY: a unique dye for versatile optical applications. Singapore: Springer Nature Singapore, 369–399.
Mula, S., Elliott, K., Harriman, A., and Ziessel, R. (2010). Energy transfer by way of an exciplex intermediate in flexible boron dipyrromethene-based allosteric architectures. J. Phys. Chem. A 114, 10515–10522. doi:10.1021/jp106626v
Mula, S., Ray, A. K., Banerjee, M., Chaudhuri, T., Dasgupta, K., and Chattopadhyay, S. (2008). Design and development of a new pyrromethene dye with improved photostability and lasing efficiency: theoretical rationalization of photophysical and photochemical properties. J. Org. Chem. 73, 2146–2154. doi:10.1021/jo702346s
Nadiv, O., Shinitzky, M., Manu, H., Hecht, D., Roberts, C. T., Leroith, D., et al. (1994). Elevated protein tyrosine phosphatase activity and increased membrane viscosity are associated with impaired activation of the insulin receptor kinase in old rats. Biochem. J. 298, 443–450. doi:10.1042/bj2980443
Odobel, F., Pellegrin, Y., and Warnan, J. (2013). Bio-inspired artificial light-harvesting antennas for enhancement of solar energy capture in dye-sensitized solar cells. Energy Environ. Sci. 6, 2041. doi:10.1039/c3ee24229c
Porcu, P., González-Méndez, I., Sorroza-Martínez, K., Estrada-Montaño, A. S., Cuétara-Guadarrama, F., Vonlanthen, M., et al. (2022). Pyrene-bodipy dyads: optical properties and applications. Dyes Pigm 207, 110713. doi:10.1016/j.dyepig.2022.110713
Qu, X., Liu, Q., Ji, X., Chen, H., Zhou, Z., and Shen, Z. (2012). Enhancing the Stokes' shift of BODIPY dyes via through-bond energy transfer and its application for Fe3+-detection in live cell imaging. Chem. Commun. 48, 4600. doi:10.1039/c2cc31011b
Sen, A., Mora, A. K., Koli, M., Mula, S., Kundu, S., and Nath, S. (2022). Sensing lysozyme fibrils by salicylaldimine substituted BODIPY dyes - a correlation with molecular structure. Int. J. Biol. Macromol. 220, 901–909. doi:10.1016/j.ijbiomac.2022.08.112
Su, D., Teoh, C. L., Wang, L., Liu, X., and Chang, Y.-T. (2017). Motion-induced change in emission (MICE) for developing fluorescent probes. Chem. Soc. Rev. 46, 4833–4844. doi:10.1039/c7cs00018a
Sunahara, H., Urano, Y., Kojima, H., and Nagano, T. (2007). Design and synthesis of a library of BODIPY-based environmental polarity sensors utilizing photoinduced electron-transfer-controlled fluorescence ON/OFF switching. J. Am. Chem. Soc. 129, 5597–5604. doi:10.1021/ja068551y
Tasior, M., Vakuliuk, O., Koga, D., Koszarna, B., Górski, K., Grzybowski, M., et al. (2020). Method for the large-scale synthesis of multifunctional 1,4-Dihydro-pyrrolo[3,2-b]pyrroles. J. Org. Chem. 85, 13529–13543. doi:10.1021/acs.joc.0c01665
Ulrich, G., Goze, C., Guardigli, M., Roda, A., and Ziessel, R. (2005). Pyrromethene dialkynyl borane complexes for “cascatelle” energy transfer and protein labeling. Angew. Chem. Ed. 44, 3694–3698. doi:10.1002/anie.200500808
Ulrich, G., Ziessel, R., and Harriman, A. (2008). The chemistry of fluorescent bodipy dyes: versatility unsurpassed. Angew. Chem. Int. Ed. 47, 1184–1201. doi:10.1002/anie.200702070
Wang, J., Chai, Z., Liu, S., Fang, M., Chang, K., Han, M., et al. (2018). Organic dyes based on tetraaryl-1,4-dihydropyrrolo-[3,2-b]pyrroles for photovoltaic and photocatalysis applications with the suppressed electron recombination. Chem. Eur. J. 24, 18032–18042. doi:10.1002/chem.201803688
Wu, J.-Y., Yu, C.-H., Wen, J.-J., Chang, C.-L., and Leung, M.-K. (2016). Pyrrolo-[3,2-b]pyrroles for photochromic analysis of halocarbons. Anal. Chem. 88, 1195–1201. doi:10.1021/acs.analchem.5b03374
Wu, L., Huang, C., Emery, B. P., Sedgwick, A. C., Bull, S. D., He, X.-P., et al. (2020). Förster resonance energy transfer (FRET)-based small-molecule sensors and imaging agents. Chem. Soc. Rev. 49, 5110–5139. doi:10.1039/c9cs00318e
Wu, L., Loudet, A., Barhoumi, R., Burghardt, R. C., and Burgess, K. (2009). Fluorescent cassettes for monitoring three-component interactions in vitro and in living cells. J. Am. Chem. Soc. 131, 9156–9157. doi:10.1021/ja9029413
Keywords: tetraarylpyrrolo[3,2-b]pyrrole, BODIPY, dyad, viscosity sensor, molecular rotor, FRET
Citation: Agrawal R, Gorai S, Yadav SS, Wadawale AP and Mula S (2024) Tetraarylpyrrolo[3,2-b]pyrrole-BODIPY dyad: a molecular rotor for FRET-based viscosity sensing. Front. Chem. 12:1473769. doi: 10.3389/fchem.2024.1473769
Received: 31 July 2024; Accepted: 11 September 2024;
Published: 10 October 2024.
Edited by:
Eusebio Juaristi, Center for Research and Advanced Studies, National Polytechnic Institute of Mexico (CINVESTAV), MexicoReviewed by:
Arturo Jiménez Sánchez, National Autonomous University of Mexico, MexicoChathura S. Abeywickrama, University of Connecticut, United States
Copyright © 2024 Agrawal, Gorai, Yadav, Wadawale and Mula. This is an open-access article distributed under the terms of the Creative Commons Attribution License (CC BY). The use, distribution or reproduction in other forums is permitted, provided the original author(s) and the copyright owner(s) are credited and that the original publication in this journal is cited, in accordance with accepted academic practice. No use, distribution or reproduction is permitted which does not comply with these terms.
*Correspondence: Soumyaditya Mula, c211bGFAYmFyYy5nb3YuaW4=