Corrigendum: Evaluation of the viability of microencapsulated Trichoderma longibrachiatum conidia as a strategy to prolong the shelf life of the fungus as a biological control agent
- 1Escuela de Ciencias Agrarias, Universidad Nacional, Heredia, Costa Rica
- 2National Nanotechnology Laboratory, National Center for High Technology, San José, Costa Rica
- 3Laboratorio de Fitopatología, Escuela de Ciencias Agrarias, Universidad Nacional, Heredia, Costa Rica
- 4Center for Sustainable Development Studies, Universidad Técnica Nacional, Alajuela, Costa Rica
Trichoderma is an antagonistic fungus used commercially; however, the viability of these formulations is affected by biotic and abiotic factors. In this research, microcapsules of sodium alginate reinforced with nanocellulose and/or chitosan were developed to encapsulate T. longibrachiatum conidia and characterized by SEM, FTIR, and TGA. The viability of the microencapsulated conidia was evaluated through different temperatures (room temperature, 5°C and 37°C), as well as their in vitro antagonistic potential against Fusarium oxysporum. The formulations evaluated had encapsulation efficiencies above 92% and the microcapsules with alginate, chitosan, and nanocellulose maintained 100% viability at 37°C for 2 months. In addition, all formulations evaluated retained antagonistic ability against F. oxysporum. These findings support the use of alginate, nanocellulose and chitosan for the formulation of microcapsules to maintain the viability of T. longibrachiatum conidia over time and at different temperature conditions.
1 Introduction
The continuous use of agrochemicals for the management of plant diseases has generated several environmental and health problems, so biological control strategies have gained importance in recent years (Chandrika et al., 2019; Sehrawat et al., 2022). The use of beneficial microorganisms such as fungi and bacteria (Herrera et al., 2020; Asaturova et al., 2021; Elnahal et al., 2022), has an advantage that it allows the control of pests and diseases, and reduces the risk of pesticide resistance development (Saberi-Riseh et al., 2021). Fungi of the genus Trichoderma are used as biological control agents (BCAs) and plant growth promoters (PGPFs) (Saldaña-Mendoza et al., 2023; Martinez et al., 2023). T. longibrachiatum species have been evaluated in disease and pest control, the production of metabolites involved in these processes, as well as the compounds it stimulates in plants under stress conditions (Sornakili et al., 2020; Sridharan et al., 2021; TariqJaveed et al., 2021; Poveda, 2021).
Several strategies have been developed to create Trichoderma formulations from the fungal biomass (Martinez et al., 2023), however, these products have certain disadvantages, such as low viability and stability (Løvschall et al., 2024), which makes it difficult for these microorganisms to establish themselves and express their full antagonistic potential for the control of phytopathogens or pests (Braga et al., 2019). Formulation development must ensure the protection and maintenance of the viability of conidia or mycelium of antagonistic fungi (Mulatu et al., 2021; Saldaña-Mendoza et al., 2023). Several strategies have been employed for the preservation of Trichoderma spp. conidia. These include the use of solid carriers, such as vermiculite and biochar, liquid carriers like oils and hydrogels, and processing techniques such as freeze-drying, spray-drying, and fluidized bed drying (Martinez et al., 2023). In a study conducted by Kaewkhon et al. (2024), T. longibrachiatum conidia were preserved by developing pellets using spent mushroom substrate. This approach was shown to extend conidial shelf life and enhance their efficiency for biological control.
Moreover, encapsulation of conidia is a strategy that can be used to overcome environmental constraints and give the antagonist a competitive advantage over pathogens and another microflora (Locatelli et al., 2018; Saberi-Riseh et al., 2021). Microcapsules are usually produced from the formation of liquid droplets, either by dripping or by emulsifying and solidifying the liquid droplets to form particles (Bejarano and Puopolo, 2020). Various polymers have been used for the encapsulation of active compounds (Martínez et al., 2023; Lotfalinezhad et al., 2024) and biological control agents, among which are alginate, starch, pectin, cellulose, carrageenan, agar, and chitosan (Chaudhary and Shukla, 2020; Saberi-Riseh et al., 2021).
Alginate is a natural anionic polysaccharide derived from brown algae, composed of residues of β-d-mannuronate and α-l-guluronate, linked by 1,4 glycosidic bonds (Murugappan and Muthadhi, 2022; Cao et al., 2023). This has been used to microencapsulate Trichoderma conidia to maintain the viability of formulations (Locatelli et al., 2018; Mancera et al., 2019; Adzmi et al., 2021), as well as enhance their antagonistic characteristics (Løvschall et al.2024), as it has been found to exhibit synergism with Trichoderma for disease control (Singh and Chittenden, 2021). Moreover, chitosan has been used in agriculture in the encapsulation of agrochemicals and micronutrients (García et al., 2023). Otherwise, nanocellulose, obtained from plant cellulose or synthesized by some bacteria, has been utilized in the formulation of micro- and nanoparticles, owing to its ability to enhance the mechanical and physical properties of these materials (Gan et al., 2020).
To date, very little is known about the use of additives such as chitosan and nanocellulose to improve the characteristics of alginate microcapsules, particularly their mechanical and physical properties (Siqueira et al., 2019). Chitosan, in particular, helps enhance these properties by increasing the rigidity of the microcapsules (Qu and Luo, 2020). Some studies have shown that encapsulating Trichoderma spp. conidia in alginate microcapsules, compared to non-encapsulated conidia, significantly improves their viability and stability in field trials under various environmental stresses, such as heat and UV treatments, while also extending their activity during storage at different temperatures (Maruyama, et al., 2020; Qi et al., 2023). However, studies are lacking to understand how factors such as storage time, temperature, and humidity affect the viability of fungal formulations, mainly under more realistic conditions of Trichoderma exposure in the field (Locatelli et al., 2018). Due to the above, in the present study, the effect of microencapsulation of T. longibrachiatum was evaluated by measuring the viability of conidia when exposed to different temperature conditions, as a strategy to prolong the shelf life of the fungus as a biological control agent, founding that the microcapsules with alginate, chitosan, and nanocellulose maintained 100% viability at 37°C for 2 months. We hypothesize that adding chitosan and nanocellulose to the microcapsule formulation allows a better protection of the conidia while preserving their viability in adverse conditions.
2 Materials and methods
2.1 Microorganism
The fungus T. longibrachiatum was provided by the Phytopathology Laboratory of the School of Agricultural Sciences of the Universidad Nacional of Costa Rica, which had been previously recovered from the rhizosphere of soils destined to pineapple (Ananas comosus L. Merr) production in Puerto Viejo, Sarapiquí, with the purpose of carrying out tests for the control of Fusarium oxysporum, the second most important phytopathogen in this crop in Costa Rica. T. longibrachiatum was under storage conditions at 10°C in vials with sterile mineral oil.
2.2 Reactivation of Trichoderma longibrachiatum
The isolates stored in vials were transferred to Petri dishes containing potato-dextrose-agar (PDA) culture medium, which were incubated for 7 days at 28°C, until the colonies were densely populated and sporulated covering the dishes. Subsequently, for further recovery of the growth vigor of the isolates, 1 cm diameter discs were cut in the region between the center and the edge of the colonies and transferred to new dishes containing PDA culture medium. These were incubated for 7 days at room temperature.
2.3 Spore production of T. longibrachiatum
A plate with pure and sporulated culture of the T. longibrachiatum strain was taken, the mycelium was scraped with a sterile scalpel and placed in 20 mL of sterile distilled water. The concentration of the conidial suspension was determined with the aid of a hemocytometer, according to the methodology described by French and Hebert (1980). For this, a Neubauer chamber was used and a count of the number of propagules in five secondary squares was performed. Finally, the suspensions were adjusted to obtain a concentration (1 × 106 conidia mL−1) and to be able to use them for microencapsulation and conventional formulation.
2.4 Conventional formulation of Trichoderma longibrachiatum
In the conventional formulation (TL) of T. longibrachiatum, 100 g of rice was placed in a beaker and washed with water until the water was not cloudy. Subsequently, the antibiotic chloramphenicol was added, the mixture was shaken, and left to stand for 5 min. The water with the antibiotic was discarded and the rice was placed in hermetically sealed polystyrene bags. The rice was autoclaved for 40 min at 121°C, with 1.2 kg/cm2. Once sterilized, an aliquot of 5 mL of the conidia suspension (1 × 106 conidia mL−1), described above, was placed in the rice contained in the bag, sealed and incubated at room temperature until mycelial growth and spore production were observed. Once the substrate with the inoculum was ready, 25 g were placed in plastic vials for viability tests. This test was conducted by placing 50 mg of the TL in plastic containers, exposed to storage at different temperature conditions: room temperature (20°C), 5°C and 37°C with a relative humidity of 80% in bioclimatic chambers. Conidial viability assessments were performed at 3, 5, 7, 15, 30, and 60 days of exposure and viability was determined by direct counts of viable and non-viable conidia after 24 h, using an optical microscope at ×40 magnification.
2.5 Preparation of nanocellulose
A 1.2% solution of cotton cellulose (fibers (medium), Sigma-Aldrich, MO, United States) was prepared and placed in an ultrasonic bath for 10 min. Subsequently, the solution was sonicated for 1 hour at 700 W (QSONICA Q700, Newton, CT, United States), with an amplitude of 40% and cycles of 50 s ON and 10 s OFF, during the sonication the sample was kept cold. Subsequently, the solution was allowed to stand for 30 min to precipitate and the supernatant was extracted, which was centrifuged at 3,500 rpm for 5 min. The precipitate obtained was discarded and the supernatant was centrifuged twice at 10,000 rpm for 5 min, preserving the precipitate in both centrifugations. Finally, the precipitate was dried in the oven at 35°C and stored at 4°C for further SEM analysis.
2.6 Experimental design of microcapsules formulation
Microcapsules were developed following the methodology described by Lupo et al. (2014) with some modifications. To standardize the method, the type of surfactant (Span 80 and Tween 20) was first evaluated at 5% and then the concentration of the surfactant Tween 20 (5%, 7.5%, and 10%). Once the type and concentration of surfactant were determined, different agitation speeds were compared (600, 800 and 1,000 rpm), and with the best result obtained, three concentrations of chitosan (Acros Organics, CAS:9012-76-4) and nanocellulose (0.25%, 0.5%, and 1%) were evaluated as coating and reinforcement polymers, respectively.
For the formulation with alginate (Alg), a 10 mL solution of 2% (w/w) sodium alginate (Sigma-Aldrich, CAS:9005-38-3) was prepared to which 1 mL of a 0.5 M CaCO3 solution was added. Subsequently, it was mixed with a homogenizer (Ultra-Turrax, IKA, T25, Germany) for 3 min at 12,000 rpm and left under refrigeration for 1 h to hydrate and remove air bubbles. The above mixture (dispersed phase) was dispersed in 40 mL of soybean oil with 5% (w/w) Tween 20 (oil phase). To promote the formation of the water-in-oil (W/O) emulsion, the dispersed phase was delivered dropwise in a controlled manner using a syringe with constant agitation for 15 min at 1,000 rpm. Subsequently, gelation of the emulsion sodium alginate droplets was induced by the controlled addition of a mixture consisting of 10 mL soybean oil and 80 μL of glacial acetic acid. The microcapsules formed were separated from the oil phase with the addition of 100 mL of aqueous CaCl2 solution 0.05 M (Sigma-Aldrich, CAS:10043-52-4) containing 10% (w/w) Tween 20 under agitation for 15 min at 650 rpm.
For the formulations of alginate with chitosan (0.5%) (AlgCh), alginate and nanocellulose (0.5%) (AlgNc) and alginate, chitosan (0.5%) and nanocellulose (0.5%) (AlgChNc), the methodology described above was followed, with the addition of the polymers to the dispersed phase with the sodium alginate.
2.7 Microencapsulation of Trichoderma longibrachiatum
Microencapsulation of the fungal conidia was carried out following the methodology described in the previous point, with the addition of 10 mL of conidial suspension (1 × 106 conidia ml-1) to the dispersed phase, followed by agitation for 20 min with the aid of a magnetic stirrer, before preparing the emulsion by mixing with soybean oil. The microcapsules obtained were filtered using a coffee filter and three washes were performed with sterile distilled water. Drying was carried out at room temperature for 24 h and the dried microcapsules were packaged for further analysis.
2.8 Characterization of microcapsules
2.8.1 Fourier transform infrared spectroscopy (FTIR)
Microcapsules with and without conidia were analyzed in duplicate, using a Nicolet 6700 FT-IR infrared spectrometer (Thermo Fisher Scientific, United States). The samples were placed directly in the equipment without any pretreatment. A scan was performed in the range 500–4,000 cm−1. The spectra were analyzed with OMNIC 8.1 software (OMNIC Series 8.1.10, Thermo Fisher Scientific, United States).
2.8.2 Thermogravimetric analysis (TGA)
Microcapsules without conidia were analyzed in duplicate using a TGA-Q500 thermogravimetric analyzer (TA Instruments, United States), equipped with Universal Analysis 2000 software (version 4.5A, TA Instruments, United States). A nitrogen purge flow rate of 40 mL/min and a sample purge flow rate of 60 mL/min were used. Initially, the equipment was kept at equilibrium at 25°C for 5 min and subsequently, heating was performed at 10°C/min, in a temperature range from 25°C to 600°C.
2.8.3 Differential scanning calorimetry (DSC)
Microcapsules without conidia were analyzed in duplicate by differential scanning calorimetry (DSC) (Q200, TA Instruments, United States), using TA Universal Analysis software. Temperature ramps covering a cycle from 25°C to 200°C at a rate of 10°C/min were used for the analysis.
2.8.4 Scanning electron microscopy (SEM)
Microcapsules with and without conidia were analyzed in duplicate, taking images at different magnifications, using SEM equipment (JEOL, JSM-6390 LV, Japan), operating at a voltage acceleration between 5 and 10 kV, with secondary electrons (SEI) and a spot size between 30 and 50.
2.9 Encapsulation efficiency
To determine the encapsulation efficiency, 50 mg of the microcapsules were added in 10 mL of a solution of NaHCO3 (0.2 M) and Na2C6H5O7·2H2O (0.06 M) and shaken for 2 h to achieve complete dissolution of the microcapsules. A 10 µL aliquot was extracted and conidia were counted using a Neubauer chamber under an optical microscope (Olympus Optical, CH, Japan). Encapsulation efficiency was defined from the amount of conidia added and the amount of conidia released from the dried microcapsules.
2.10 Conidial release rate
An optical microscope (Olympus Optical, CH, Japan) was used to determine the release rate of encapsulated conidia. The dried microcapsules (50 mg) were placed in a solution of NaHCO3 (0.2 M) and Na2C6H5O7·2H2O (0.06 M). The duration of the immersion was 0, 1, 5, 10, 30, 30, 60, 120, and 240 min (Liu and Liu, 2009). For each time, two samples were taken and analyzed under an optical microscope, determining the conidial concentration with the aid of a Neubauer chamber.
2.11 Conidial temperature tolerance
To determine the conidial tolerance of encapsulated and non-encapsulated conidia, 50 mg of each formulation were placed in plastic containers, which were exposed to storage at different temperature conditions: room temperature (20°C), 5°C and 37°C with a relative humidity of 80% in bioclimatic chambers. Conidial viability assessments were performed at 3, 5, 7, 15, 30, and 60 days of exposure and viability was determined by direct counts of viable and non-viable conidia after 24 h, using an optical microscope at ×40 magnification. Conidia were considered viable when the germ tube length was greater than the conidial diameter. For this, 5 mg of the microcapsules were taken and placed in a 2 mL tube to which 0.5 mL of a solution of NaHCO3 (0.2 M) and Na2C6H5O7·2H2O (0.06 M) were added. Vortex agitation was performed for 1 min to dissolve the microcapsules and a 25 μL aliquot was placed on a sterile slide containing a thin layer of Agar-Water medium on the upper side, the slide was contained inside a Petri dish with wet paper towel. The aliquot of microcapsules was spread with a glass rod over the medium and incubated for 24 h at room temperature. After incubation time, 20 conidia were counted randomly along the entire slide, counting germinated and non-germinated conidia.
2.12 Biological activity – In vitro antagonism
An in vitro antagonism test was performed to determine the antagonistic capacity of T. longibrachiatum after the microencapsulation process for the four formulations exposed at room temperature, 5°C and 37°C for 60 days. For this, the microcapsules were dissolved in a buffer (NaHCO3 (0.2 M) and Na2C6H5O7·2H2O (0.06 M)) and inoculated in Petri dishes containing PDA medium for growth. Once the fungus had sporulated (12 days), a 5 mm disc was taken, using a punch, and placed 1 cm from the edge of a Petri dish containing PDA medium, and at the other end, a 5 mm disc of the pathogen F. oxysporum 1 cm from the edge. The dish was incubated and evaluated at 5, 9, and 14 days to determine the antagonistic capacity of the microencapsulated fungus. This test was performed in duplicate for each of the formulations and the results were evaluated according to the Bell et al. (1982) class scale (Table 1).
2.13 Experimental design
The experimental unit and the observational unit for the temperature and storage tests was a plastic container containing microcapsules for each formulation. The structure of the treatments for the tests was bifactorial, where the first factor corresponds to encapsulation and non-encapsulation, and the second factor was the temperature levels. In the case of storage, the encapsulation factor, and the time factor (days) were analyzed. Each treatment had three replicates and the structure of the trial was a completely randomized design (CRD).
2.14 Data analysis
For the variables evaluated in the research, the most important summary measures were estimated. For release rate and conidial tolerance to temperature, point and line plots were made by taking the means, using the ggplot2 package (Wickham, 2016) of the statistical software R version 4.1.2 (R Core Team, 2020).
To determine the mean encapsulation efficiency and release rate of microencapsulated conidia, a 95% confidence interval for the mean was estimated using the following formula:
Where:
To identify significant differences in particle size among the treatments without conidia (since the microparticles agglomerated, making it difficult to precisely determine particle size), the R software was used. The normality of the data was assessed using the Shapiro-Wilk test, and homoscedasticity was evaluated with Levene’s test. As the data did not follow a normal distribution, a Kruskal–Wallis H test was implemented to identify differences among the treatments (p < 0.05), followed by a Dunn’s post hoc test to determine pairwise differences between treatments.
3 Results
3.1 Standardization of microcapsule formulation
Tests were carried out to standardize the formulation of alginate microcapsules using the emulsion-internal gelation method. The surfactants Span 80, and Tween 20 were evaluated, which were used at 5% (w/w) in the oil phase of the emulsion and at 10% with CaCl2 for hardening and washing of the microcapsules. The oil phase enables the formation of the emulsion by incorporating sodium alginate (emulsion step), and the microcapsules are subsequently produced using a gelling agent (gelation step) (Lin et al., 2021).
The microcapsules formulated using Span 80 had an average size of 261.84 ± 61.68 µm and showed regular circumference and slightly rough surface (Figure 1A). However, as seen in Figure 1B, they tended to agglomerate, and the observed sizes were very heterogeneous.
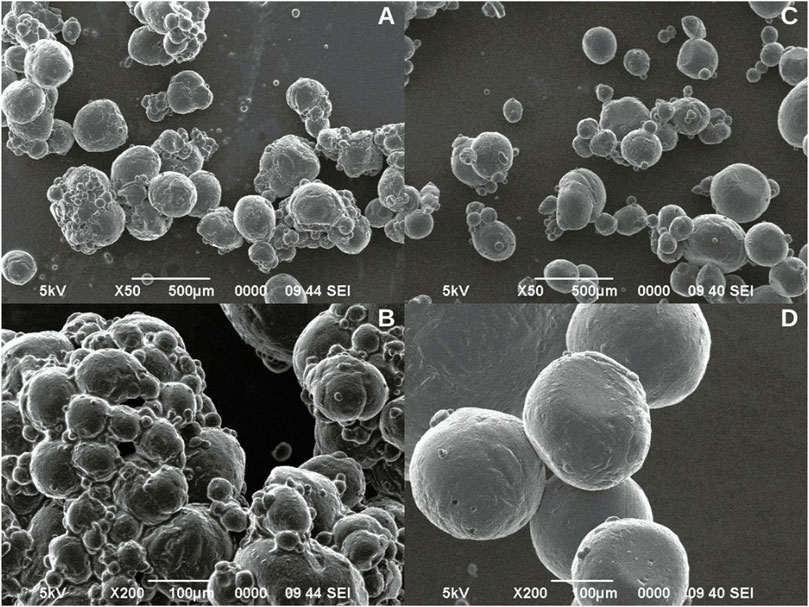
Figure 1. Microcapsules formulated with 5% Span 80 surfactant (A, B). Microcapsules formulated with 5% Tween 20 surfactant (C, D).
Otherwise, the microcapsules formulated with Tween 20 had an average size of 253.95 ± 96.85 µm and presented regular circumference, and smooth surface (Figure 1D). Moreover, the observed sizes were more homogeneous and no tendency to agglomeration was observed (Figure 1C).
Once the surfactant was selected, the effect of agitation speed on the formation of the emulsion and the gelation reaction was analyzed, for which three speeds (600, 800 and 1,000 rpm) were evaluated (Figure 2). The microcapsules obtained at 600 rpm exhibited size diversity, with an average size of 286.67 ± 75.28 µm, a regular circumference, and a smooth surface (Figure 2A), while those formulated at 800 rpm presented greater size diversity, with an average size of 249.17 ± 99.57 µm, and irregular circumference (Figure 2B). The best result was obtained when an agitation speed of 1000 rpm was used, since these showed greater size homogeneity, with two sizes predominating (100 and 300 µm), with an average size of 190.83 ± 91.75 µm, as well as regular circumference and smooth surface (Figure 2C).
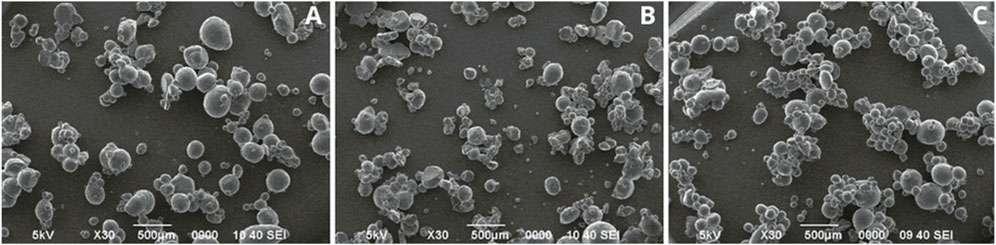
Figure 2. Microcapsules formulated at different agitation speeds with 5% Tween 20. (A) 600 rpm, (B) 800 rpm, (C) 1,000 rpm.
After identifying the agitation speed that presented the best results (1,000 rpm), the concentration of the surfactant Tween 20 (5%, 7.5%, and 10%) was evaluated (Figure 3). The microcapsules formulated at 7.5% had heterogeneity in size, with an average size of 100.99 ± 55.78 µm, irregular circumference, and rough surfaces (Figure 3B). Those formulated at 10% presented surfaces with less roughness than those formulated at 7.5%, with an average size of 136.91 ± 77.36 µm (Figure 3C). Microcapsules with Tween 20 at 5% presented regular circumference, smooth surface, and an average size of 124.81 ± 55.68 µm (Figure 3A).
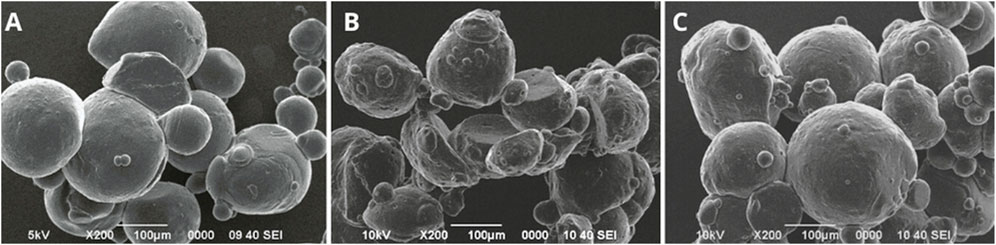
Figure 3. Evaluation of different concentrations of the surfactant Tween 20 at a stirring speed of 1,000 rpm. (A) 5%, (B) 7.5%, (C) 10%.
3.2 Nanocellulose synthesis
The results of the synthesis of nanocellulose from cotton cellulose are shown in Figure 4. It is observed that after treatment with sonication the cellulose fibers with sizes between 10 and 50 µm (Figure 4A), were reduced, obtaining nanofiber sizes between 100 and 300 nm (Figure 4B), which were used in the preparation of microcapsules.
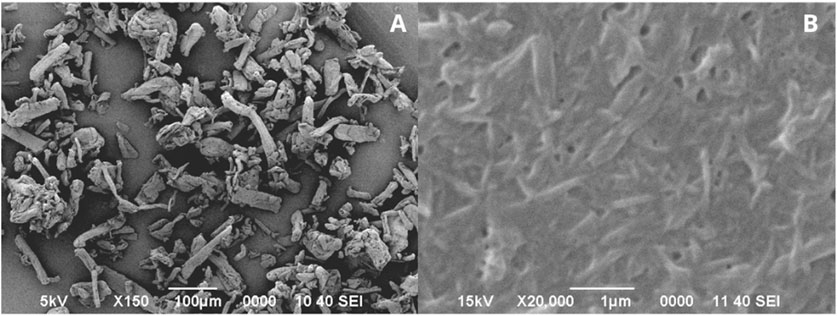
Figure 4. Cotton cellulose fibers (A). Cotton nanocellulose fibers obtained from sonication treatment (B).
3.3 Characterization of microcapsules
3.3.1 Scanning electron microscopy (SEM)
The results of the morphological characterization of the microcapsules with and without conidia are shown in Figure 5. The alginate microcapsules without conidia (Alg) had an average size of 153.67 ± 71.27 µm, with some smaller microcapsules around 50 µm. Additionally, a regular circumference and smooth surface were observed (Figure 5A). After the conidia encapsulation process, microcapsules smaller than 100 µm were observed; however, they were agglomerated, as shown in Figure 5E.
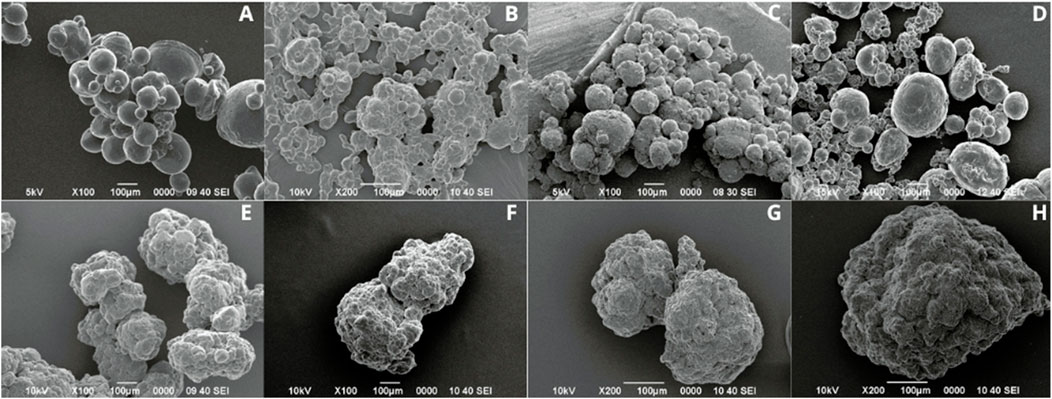
Figure 5. Microcapsules without conidia (A–D) and with conidia of T. longibrachiatum (E–H). Microcapsules Alg (A, E), AlgNc (B, F), AlgCh (C, G), AlgChNc (D, H).
Otherwise, the alginate with nanocellulose formulation without conidia (AlgNc) produced microcapsules with the smallest size, averaging 29.67 ± 7.77 µm, with regular circumference and smooth surface, although agglomeration was observed (Figure 5B). As shown in Figure 5F, microcapsules with alginate, nanocellulose and conidia agglomerated and showed irregular circumference. For the alginate and chitosan (AlgCh) formulation, the microcapsules had an average size of 105.67 ± 30.63 µm, with a regular circumference and surface roughness (Figure 5C). Additionally, in the presence of conidia, the particles exhibited agglomeration (Figure 5G). Moreover, the microcapsules formulated with chitosan, alginate, and nanocellulose (AlgChNc) had an average size of 198.67 ± 64.27 µm. They exhibited a regular circumference and a surface with some roughness (Figure 5D). However, similar to the previous formulations, they tended to agglomerate and display rough surfaces after encapsulation (Figure 5H).
The Kruskal–Wallis H test revealed a significant difference in particle size among the different treatments without conidia (χ2 (3) = 24.68, p < .001). Post-hoc Dunn’s test with Bonferroni correction indicated that the average size of the AlgNc treatment was significantly smaller than that of the other treatments.
3.3.2 Thermogravimetric analysis (TGA)
Figure 6 shows the thermogravimetric analysis of the formulated microcapsules without conidia. The results indicate that the microcapsules are relatively stable at temperatures below 220°C. The graph shows a first mass loss event between 25°C and 100°C. The second event occurs between 100°C and 350°C. Subsequently, a third event occurs from 350°C, with a mass loss of approximately 70% upon reaching 400°C, from which point the Alg, AlgCh and AlgChNc formulations remain constant. In the case of AlgNc, there is a mass loss of about 55% upon reaching 400°C, then another event with a 10% mass loss and remaining constant from 500°C onwards.
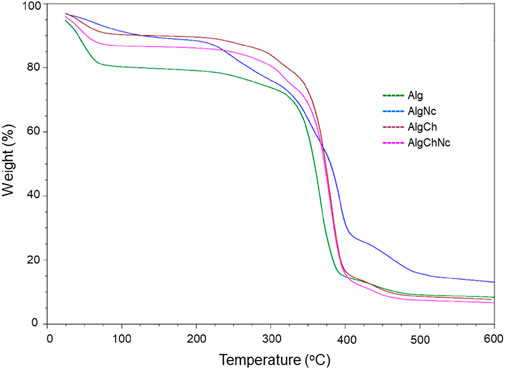
Figure 6. Thermogravimetric analysis (TGA) of microcapsules formulated with sodium alginate, chitosan and nanocellulose for the encapsulation of T. longibrachiatum conidia.
3.3.3 Differential scanning calorimetry (DSC)
The thermal transition curve of Alg, AlgNc, AlgCh and AlgChNc microcapsules was analyzed by DSC (Figure 7). The Alg formulation presented a first endothermic transition near 33°C and a subsequent endothermic peak at 75°C, also observed in AlgNc and AlgChNc microcapsules, whereas the AlgCh formulation showed a peak near 80°C.
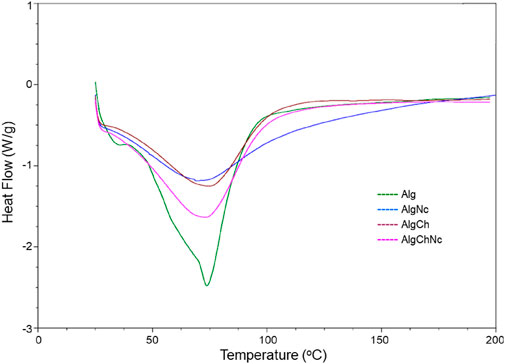
Figure 7. Differential Scanning Calorimetry (DSC) analysis of microcapsules formulated with sodium alginate, chitosan and nanocellulose for the encapsulation of T. longibrachiatum conidia.
3.3.4 Fourier transform infrared spectroscopy (FTIR)
The functional groups and intermolecular interactions of the microcapsules and the reagents used were analyzed by FTIR. The absorption bands of sodium alginate, chitosan, nanocellulose, calcium chloride and calcium carbonate, reagents used in the different microcapsule formulations, are shown in (Supplementary Table S1). Figures 8, 9 shows the FTIR spectra of the microcapsule formulations without conidia and with conidia, respectively. It can be noted that both spectra are very similar.
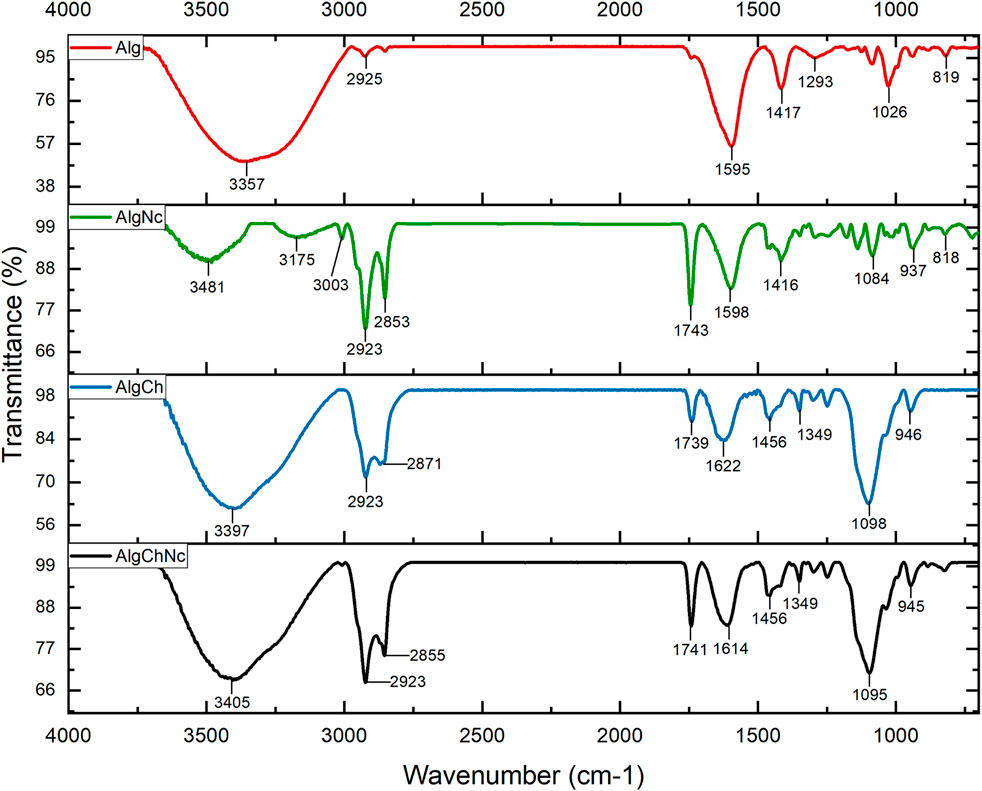
Figure 8. FTIR spectra of sodium alginate (Alg), sodium alginate and nanocellulose (AlgNc), sodium alginate and chitosan (AlgCh) and sodium alginate, chitosan and nanocellulose (AlgChNc) formulations.
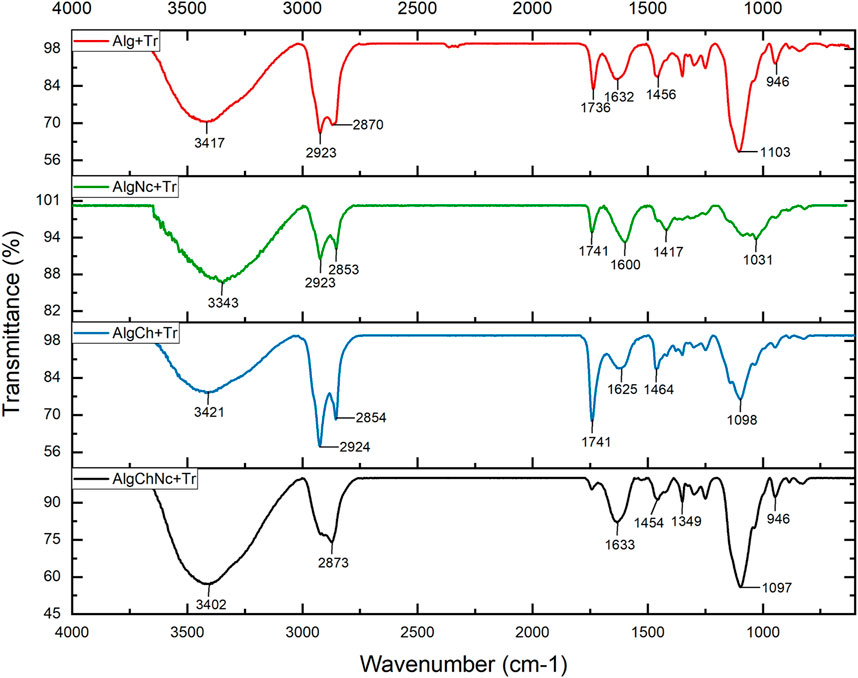
Figure 9. FTIR spectra of the microcapsule formulations with conidia of T. longibrachiatum. Sodium alginate (Alg + Tr), sodium alginate and nanocellulose (AlgNc + Tr), sodium alginate and chitosan (AlgCh + Tr) and sodium alginate, chitosan and nanocellulose (AlgChNc + Tr).
Figures 8, 9 show the FTIR spectra of the microcapsule formulations without conidia and with conidia, respectively. It is evident that both spectra exhibit striking similarities. Notably, both spectra show a prominent absorption band at 3357 cm−1, along with distinct peaks observed at 1595 cm−1, 1417 cm−1 and 2,925 cm−1. Furthermore, there was a peak at 1622 cm−1 in the AlgCh microcapsules. In the AlgCh and AlgChNc formulations, the peak at 1592 cm−1 disappeared, and a new peak was observed at 1739 cm−1.
3.4 Encapsulation efficiency and conidial release rate
Table 2 shows the encapsulation efficiency of the conidia in the microcapsules. A high encapsulation efficiency of more than 92% was found in all formulations.
The results of the release rate of conidia from the formulated microcapsules are shown in Figure 10. It was determined that the sodium alginate (Alg) microcapsules had the highest release rate, with about 66% of conidia released at 60 min and 100% at 100 min. Microcapsules with sodium alginate and chitosan (AlgCh) had similar behavior at 60 min, however, 100% of conidia were released near 110 min. Otherwise, microcapsules with sodium alginate, chitosan and nanocellulose (AlgChNc) showed a slower release rate, with about 65% of conidia released at 60 min and 93% at 120 min. Finally, microcapsules with sodium alginate and nanocellulose (AlgNc) had the slowest release rate, with 51% of conidia released at 60 min and about 85% at 120 min.
3.5 Conidial temperature tolerance
The results of the conidial viability of microencapsulated T. longibrachiatum exposed to three different storage temperatures (5, room temperature and 37°C) are shown in Figure 11. It was determined that at room temperature (20oC) and at 5°C the four microcapsule formulations (Alg, AlgNc, AlgCh and AlgChNc) and conventional formulation (TL) maintained 100% viability for 2 months (Figure 11). When conidial viability was evaluated at a temperature of 37oC (80% relative humidity), it was found that the TL formulation had a decrease in conidial viability from day 7 of exposure (65%), which reached 0% at 60 days. On the other hand, Alg and AlgCh microcapsules decreased their conidial viability to 0% at 60 days. The AlgNc microcapsules decreased their conidial viability from 15 days of exposure until reaching 0 at day 30. The best result was obtained with the AlgChNc formulation, which maintained 100% conidial viability during the 60 days of evaluation.
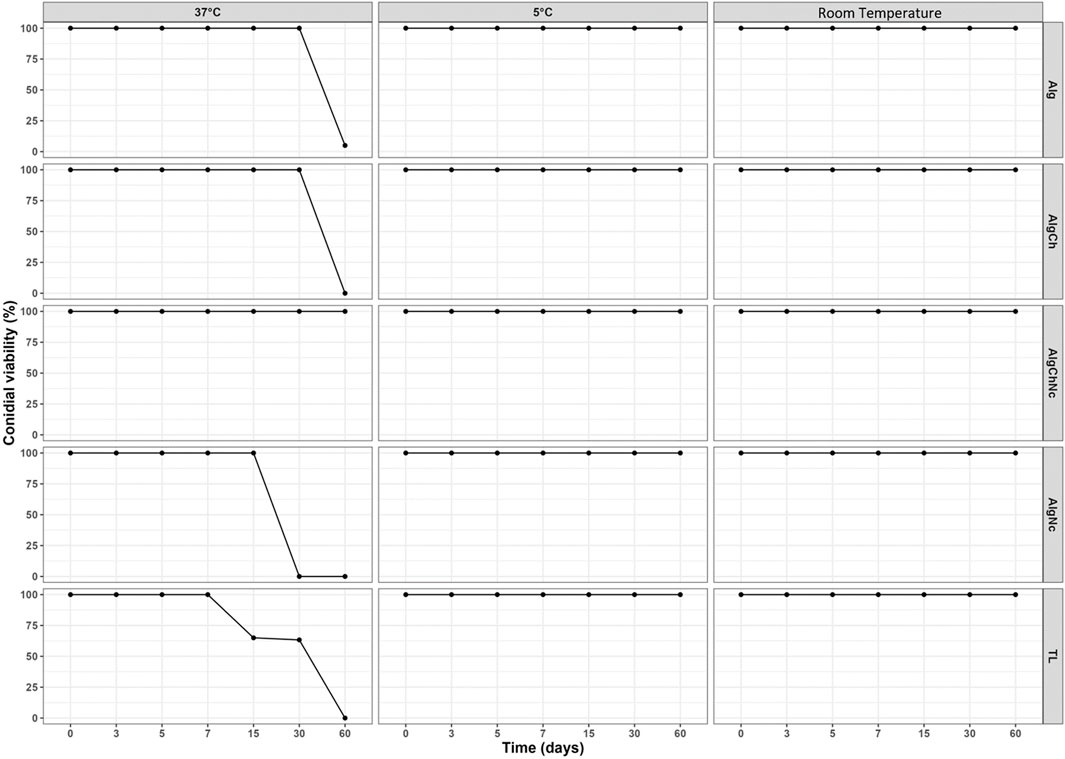
Figure 11. Conidial viability of 4 microencapsulated T. longibrachiatum in Alg, AlgCh, AlgChNc and AlgNC, and a conventional formulation (TL), exposed to three different storage temperatures (5, room temperature and 37°C).
3.6 Biological activity - antagonism in vitro
The results of the antagonism tests showed that T. longibrachiatum grew over at least two-thirds of the surface of the medium, finding a class 2 degree of antagonism (Figure 12). The four formulations evaluated behaved in the same way and the temperature to which the microcapsules were exposed prior to the antagonism tests did not influence them.
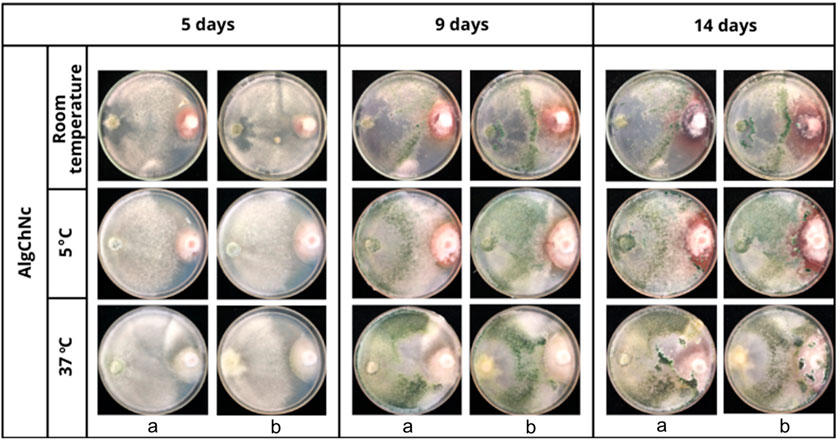
Figure 12. In vitro antagonism of AlgChNc + T. longibrachiatum (A) vs. F. oxysporum (B) following exposure of the formulation to three different temperatures.
4 Discussion
The findings of this research have significant implications for modern agriculture, particularly in the field of sustainable pest management. By providing an effective method for enhancing the stability and viability of T. longibrachiatum under various environmental stresses and reducing the dependence of chemical pesticides, this study contributes to the advancement of biopesticide applications. Moreover, by extending the shelf-life and improving the efficacy of biological agents, this research has the potential to transform pest management strategies, especially in regions with challenging environmental conditions.
4.1 Standardization of microcapsule formulation
Tests conducted to standardize the formulation of alginate microcapsules using Span 80 and Tween 20 surfactants determined that microcapsules formulated with Span 80 tended to agglomerate with highly heterogeneous sizes. Similarly, Yang et al. (2004) obtained irregularly shaped chitosan microcapsules using Span 80, however, the combination of Span 80 and Tween 60 produced spherical microcapsules with smooth surfaces. In contrast, the microcapsules formulated with Tween 20, presented regular circumference, and smooth surface, which agrees with what has been reported by other authors (Hwang et al., 2006; Martins et al., 2017). In addition, this formulation presented microcapsules more homogeneous and with no tendency to agglomeration. Similarly, Martins et al. (2017) found that the addition of Tween 20 (0.5% and 1.0% v/v) resulted in perfectly spherical microcapsules, because the surfactant reduced the surface tension of the alginate solution and thus the interfacial tension between the emulsion droplet and the alginate solution, which accelerated droplet detachment and the production of spherical capsules. This may be associated with the more condensed molecular structure of Tween 20, which allows for faster diffusion and reorganization on the droplet surface (Silva et al., 2019).
With respect to the effects of stirring speed on the morphology of the microparticles, it was determined that the higher speed, 1,000 rpm, decreased the size and increased the homogeneity. These results agree with the findings of other researchers, who determined that an increase in homogeneity and smaller size of the microparticles formed, could be due to the fact that a higher agitation speed provides a higher shear force, which allows better dispersing the droplets and avoiding agglomeration of the microcapsules (Li et al., 2021; Ma et al., 2020; Shi et al., 2019). Ma et al. (2020) obtained smaller size microcapsules by increasing the stirring speed to 1,000 rpm, like what was observed in this research. On the other hand, Shi et al. (2019) evaluated different agitation speeds (600, 800, 1,000, and 1,200 rpm) and obtained spherical and uniform nanocapsules, however, at 1,000 rpm they had a more homogeneous size.
Regarding the concentration of the surfactant Tween 20 (5%, 7.5%, and 10%), and in agreement with our results, other authors have determined that a higher concentration of Tween 20 increases the sizes of microcapsules (Teo et al., 2016). This could be because the increase in emulsion droplet size with increasing surfactant concentration may result in higher surfactant viscosity at the interface, preventing fluidity and easy movement of the organic phase into the aqueous phase (Barzegar et al., 2018). According to Baranauskaite et al. (2019) found that the optimal composition for their microcapsule formulation included the addition of Tween 20 at 4.7%, which is consistent with the percentage determined in this study.
4.2 Nanocellulose synthesis
Synthesis of nanocellulose from cotton cellulose using a sonication method, determined that this protocol is adequate. Sonication is a strategy based on an ultrasound mechanism that causes natural fibers to break down into nanofibers in water (Zhao et al., 2007), this method allows breaking micrometer aggregates and stabilizing suspensions (Gouveia et al., 2020). Soni and Mahmoud (2015) found that following treatment with ultrasonication, cotton stalk fibers were reduced to sizes of approximately 20 nm in length and 100–500 nm in diameter. When sonication is applied at a high intensity level, such as that used in this research (700 W), it results in the disruption of the strong glycosidic bonds of the crystalline portion of cellulose, which, together with the disruption of the interconnected network of hydrogen bridges, leads to the opening or defibrillation of the structure (Abd et al., 2016).
4.3 Characterization of microcapsules
4.3.1 Scanning electron microscopy (SEM)
The size of the alginate microcapsules decreased with the incorporation of the conidia, in accordance with the findings of De Oliveira et al. (2020). On the other hand, the change from a smooth to a rough surface, in the alginate and nanocellulose formulation, after encapsulation has been reported by Rungraung et al. (2022), who mention that the addition of nanocellulose to alginate microcapsules generates rough surfaces with irregularities. This roughness may be related to the drying process of the microcapsules and to the strong ionic crosslinking interactions (Brondi et al., 2022). In the case of the alginate and chitosan formulation, the roughness or scratched and fibrous surfaces may be due to the presence of chitosan on the microcapsule surface (Jurić et al., 2019). Moreover, the microcapsules formulated with chitosan, alginate and nanocellulose, presented regular circumference and roughness, and similar to what happened with the other formulations and after encapsulation they tended to agglomerate and show rough surfaces. Jurić et al. (2021) mention that agglomeration may be due to the size of the microcapsules and the presence of water molecules causing them to bind together upon drying. The rough surfaces observed on microcapsules with conidia may be since as conidia are introduced into the polymeric system, the uniform distribution of conidia throughout the matrix may make it possible to notice a roughness (Batista et al., 2017). Jurić et al. (2019) found that microcapsules prepared with T. viride conidia had numerous oval dimples and a germ tube was observed to cross the surface of the microcapsule within a few days. Near-surface sections revealed the presence of hyphae and mycelium formation within the matrix, which may account for the surface characteristics of microcapsules containing conidia, as the presence of Trichoderma and mycelial growth within the microcapsule change the structure of the gel network. It has been found that the drying process of microcapsules at room temperature causes a loss of sphericity and the surface becomes rough with irregular wrinkles, which is related to the relaxation of biopolymer tension due to water and moisture loss (Jurić et al., 2019; Rodrigues et al., 2020).
4.3.2 Thermogravimetric analysis (TGA)
The addition of chitosan to microcapsule formulations with alginate has been found to improve physicochemical properties, with enhanced stability in swelling media of different pH and improved structural strength (Qu and Luo, 2020). Hashim et al. (2019) mention that chitosan improves hydrogel stability due to the formation of polyelectrolyte complexes between the positively charged amino groups and the carboxylic residues of alginate. Siqueira et al. (2019) have determined that alginate formulations present higher stability when they contain nanocellulose in their composition. For the temperature range to which the commercial formulations could be exposed, it can be observed how the addition of polymers such as nanocellulose and chitosan, provide greater protection to the conidia, since the incorporation of both increases the stability to temperature degradation, decreasing the weight loss compared to the Alg formulation.
4.3.3 Differential scanning calorimetry (DSC)
The peak at 75°C observed in the Alg, AlgNc, and AlgChNc microcapsules, is attributed to the loss of water from the hydrophilic groups of alginate (Lim and Ahmad, 2017) and the moisture loss from nanocellulose (Mohamed et al., 2021). Additionally, the AlgCh formulation exhibits a peak near 80°C, which is similarly associated with the evaporation of absorbed water (Nikoo et al., 2018).
4.3.4 Fourier transform infrared spectroscopy (FTIR)
The FTIR spectra of the microcapsule formulations without conidia and with conidia, respectively. It can be noted that both spectra are very similar. A band at 3,357 cm−1 was observed. In addition, two wave peaks are observed at 1,595 cm−1 and 1,417 cm−1. The peak observed at 2,925 cm−1 refers to the C-H bending vibration in the methylene group (Nandiyanto et al., 2023). Meanwhile, the stretching of the N-H bond with the C=O (amide) group observed in the 1,622 cm−1 peak of sodium alginate and chitosan microcapsules suggests an interaction between the carboxyl and amino group of the polymers (Bulatao et al., 2017). The disappearance of the amino band of chitosan at 1,592 cm−1 (Table 1) may suggest the formation of a polyelectrolyte complex between sodium alginate and chitosan, furthermore, the new peak observed at 1,739 cm−1, belonging to the COOH group, is an indication of the acidic condition in which the microcapsules were prepared (Khorshidian et al., 2024). The sodium alginate, chitosan and nanocellulose (AlgChNc) formulation showed similar bands to the AlgCh formulation.
4.4 Encapsulation efficiency
A high encapsulation efficiency of more than 92% was found in all formulations. These encapsulation percentages are higher than those reported by other authors (Liu and Liu, 2009; Mancera et al., 2019). For example, Mancera et al. (2019) obtained an encapsulation efficiency of 80% in the formulation of calcium alginate microcapsules using the emulsion-internal gelation method. Meanwhile, Liu and Liu (2009) found that the sodium alginate formulation without any coating had the lowest encapsulation efficiency (45%). This indicates that the microcapsules developed in this research are a suitable method for encapsulation of T. longibrachiatum conidia. This high encapsulation efficiency could be related to that in the emulsion-internal gelation method, as CaCO3 is finely dispersed in the alginate solution, Ca2+ dissociated by acetic acid can gel in situ without disrupting the emulsion droplet to maintain the spherical shape (Huang et al., 2021).
4.5 Conidial release rate
The slower conidial release rate of the AlgCh in comparison to Alg microcapsules, have been reported previously. Belščak et al. (2015) found that caffeine release was slower when alginate microcapsules were reinforced with chitosan, this because this matrix was the least susceptible to rehydration, as the rate of water diffusion through the active ingredient coating is the limiting step for the rate of expansion and release. Otherwise, formulations containing nanocellulose had the slowest release of conidia, which may be attributed to the polysaccharide’s ability to restrict the movement of alginate chains (Lin et al., 2011), or high physical entanglement (Supramaniam et al., 2018) slowing release. Faster release of total conidia has been observed in another research (Liu and Liu, 2009; Aziz et al., 2015). Microcapsules that provide a more controlled release may require fewer applications, reducing costs and improving microbial viability (Adzmi et al., 2021). Cells that are gradually released from microcapsules, by degradation of the polymeric matrix, are more protected against destructive factors than uncoated microorganisms (Szczech and Maciorowski, 2016).
The results indicate that, with increasing time, the amount of released conidia increases, observing a positive correlation, like that reported by other authors (Adzmi et al., 2021; Vinceković et al., 2016). This could be due to various factors such as microcapsule surface moisture, water penetration into the microcapsule, transition from glassy to rubbery phase of polymers, swelling, diffusion of charged agents through the microcapsule matrix and surface layer, desorption from the surface, disintegration, dissolution, or erosion of the microcapsule structure (Vinceković et al., 2017).
In microencapsulation, the release of encapsulated cells is a crucial indicator of the sustained release effect and durability of the microcapsules (Qi et al., 2023). The cells can survive, and their metabolic activity can be maintained for extended periods, with controlled release occurring as they adapt to the surrounding environmental conditions. Encapsulated microorganisms can be significantly more efficient than conventional powder and liquid formulations (John et al., 2011). Vinceković et al. (2017) state that optimal release can be achieved through continuous release over an extended period, providing prolonged protection and nutritional effects for plants. It is important to note that the release rates observed in this study were determined under simulated in vitro conditions. However, under real field conditions, these results may vary, as several factors can influence release, such as climatic conditions, including temperature, humidity, rainfall, solar radiation, wind, and the presence of other microorganisms. For this reason, subsequent studies should be conducted under various real field conditions.
4.6 Conidial temperature tolerance
The AlgChNc formulation yielded the best results, maintaining 100% conidial viability throughout the entire 60-day evaluation period. This indicates that the combination of polymers such as alginate, chitosan and nanocellulose for the formulation of microcapsules allows the protection of T. longibrachiatum conidia, when exposed to temperatures between 5°C and 37°C. The maintenance of viability observed in AlgChNc microcapsules could be related to the fact that the combination of polymer matrices can retard oxygen diffusion in the microcapsules, which limits the amount of oxygen available to participate in the oxidation of macromolecules, as well as cause oxidative stress (Ishak et al., 2021; Cruz et al., 2022). The protection given by microcapsules leads to longer shelf life and maintenance of metabolic activity for long periods of time, both during storage and after application. On the other hand, higher temperatures can lead to greater reactivity and diffusion of reactive oxygen species (ROS), decreasing the viability of conidia (Ishak et al., 2021; Braga et al., 2022).
The maintenance of viability of encapsulated conidia in AlgChNc microcapsules is a promising result for commercial use and contrasts with the findings of other researchers who have determined that microencapsulated conidia maintain their viability at low storage temperatures (5°C–8°C), but that their viability decreases as temperature increases. For example, Przyklenk et al., 2017, determined that microencapsulated conidia of Metarhizium sp. remain viable at storage temperatures of 5°C for a period of 6 months, however, viability decreased at 25°C. De Oliveira et al. (2020) evaluated the exposure of microencapsulated Trichoderma conidia at 8oC, 25oC, and 35oC, finding that the best viability maintenance was obtained for lyophilized microcapsules stored at 8oC, however, there was decrease in viability at a temperature of 25oC. Adzmi et al. (2021) found that the best maintenance of viability of microencapsulated Trichoderma conidia was obtained at a temperature of 5oC. On the other hand, Szczech and Maciorowski (2016) managed to maintain the viability of Trichoderma conidia almost at the same level (104,5 cfu.g−1) in freeze-dried microcapsules, after 6 months of storage at 4oC, however the viability of conidia decreased in wet microcapsules, at storage temperatures of 30°C after 15 days, and after 90 days the spores were no longer viable.
4.7 Biological activity - antagonism in vitro
T. longibrachiatum showed a class 2 degree of antagonism by growing over two-thirds of the medium, and the four formulations evaluated exhibited consistent behavior without influence from the prior temperature exposure. Several investigations have determined that some species of the genus Trichoderma have a high antagonistic capacity with inhibition percentages of 50.68% (Redda et al., 2018), and 92.11% (Nofal et al., 2021) in the in vitro control of F. oxysporum, which coincides with what was observed in this research and demonstrates the conservation of the antagonistic capacity of the fungus after microencapsulation. Sundaramoorthy and Balabaskar (2013) obtained a growth inhibition of 27.22% when they used T. longibrachiatum for in vitro control of F. oxysporum. Sallam et al. (2019) on their part found that T. longibrachiatum reduced the severity of wilt disease by 24.8%.
Furthermore, it is important to highlight both the cost and effectiveness of the microencapsulation method. The cost of encapsulation depends on various factors, including production scale, the type and quantity of additives used, and the specific protocols employed, all of which influence energy consumption and the use of laboratory equipment. However, in this research, the protocol used involves simple equipment, as an ultra-turrax is employed for the generation of the microcapsules, in contrast to other protocols described in the literature. The use of natural biopolymers such as alginate, chitosan, and nanocellulose offers a cost-effective and environmentally sustainable alternative to synthetic polymers. Additionally, this approach enhances the shelf life and stability of Trichoderma conidia, providing a dual benefit: it ensures an eco-friendly solution while extending the efficacy and availability of these biocontrol agents over prolonged periods.
5 Conclusion
The results of this research demonstrate that the polymers sodium alginate, chitosan and nanocellulose allow the formulation of microcapsules that help to protect and maintain the viability of T. longibrachiatum conidia under different temperature conditions. An encapsulation efficiency above 92% was obtained for the three microcapsule formulations and the viability of the conidia was maintained when the microcapsules were stored at 5oC, 20oC, and 37oC, being the formulation with the three polymers (AlgChNc) the one that showed the best result when stored for 2 months at the temperature of 37°C. In vitro antagonism tests showed that microencapsulation of the fungus does not affect its antagonistic capacity, obtaining a category II in substrate competition against the phytopathogenic fungus F. oxysporum for the three microcapsule formulations; however, tests are required to determine whether this capacity is maintained under field conditions.
Data availability statement
The original contributions presented in the study are included in the article/Supplementary Material, further inquiries can be directed to the corresponding author.
Author contributions
LA-C: Data curation, Formal Analysis, Investigation, Software, Visualization, Writing–original draft, Writing–review and editing. DB-M: Conceptualization, Formal Analysis, Investigation, Methodology, Supervision, Writing–original draft, Writing–review and editing. SO-C: Conceptualization, Formal Analysis, Investigation, Methodology, Supervision, Writing–review and editing. AV-M: Conceptualization, Data curation, Formal Analysis, Methodology, Supervision, Visualization, Writing–review and editing. JV-B: Formal Analysis, Funding acquisition, Project administration, Resources, Writing–review and editing. GM-V: Conceptualization, Formal Analysis, Investigation, Methodology, Supervision, Writing–original draft, Writing–review and editing.
Funding
The author(s) declare that financial support was received for the research, authorship, and/or publication of this article. Funding for the research was provided by the scholarship awarded to Arias-Chavarría Luis Diego from the National Center for High Technology (CeNAT). The role of the funding source was to support the characterization analysis of the microcapsules. This work was also supported by the National Nanotechnology Laboratory (LANOTEC) and the School of Agricultural Sciences of the National University, Costa Rica.
Conflict of interest
The authors declare that the research was conducted in the absence of any commercial or financial relationships that could be construed as a potential conflict of interest.
Publisher’s note
All claims expressed in this article are solely those of the authors and do not necessarily represent those of their affiliated organizations, or those of the publisher, the editors and the reviewers. Any product that may be evaluated in this article, or claim that may be made by its manufacturer, is not guaranteed or endorsed by the publisher.
Supplementary material
The Supplementary Material for this article can be found online at: https://www.frontiersin.org/articles/10.3389/fchem.2024.1473217/full#supplementary-material
References
Abd, S., Zain, S., Das, R., and Centi, G. (2016). Synergic effect of tungstophosphoric acid and sonication for rapid synthesis of crystalline nanocellulose. Carbohydr. Polym. 138, 349–355. doi:10.1016/j.carbpol.2015.10.023
Adzmi, F., Musa, M., Siddiqui, Y., Yun, W., Hamid, H., Abdu, A., et al. (2021). Development of alginate-montmorillonite-starch with encapsulated Trichoderma harzianum and evaluation of conidia shelf life. Int. J. Agric. Biol. 26 (01), 87–96. doi:10.17957/IJAB/15.1812
Asaturova, A., Shternshis, M., Tsvetkova, V., Shpatova, T., Maslennikova, V., Zhevnova, N., et al. (2021). Biological control of important fungal diseases of potato and raspberry by two Bacillus velezensis strains. PeerJ 9, e11578. doi:10.7717/peerj.11578
Aziz, A., Vineela, V., and Vimala, P. (2015). Sodium humate as a promising coating material for microencapsulation of Beauveria bassiana conidia through spray drying. Dry. Technol. 33 (2), 162–168. doi:10.1080/07373937.2014.938814
Baranauskaite, J., Kopustinskiene, D., and Bernatoniene, J. (2019). Impact of gelatin supplemented with gum Arabic, tween 20, and β-cyclodextrin on the microencapsulation of Turkish oregano extract. Molecules 24 (1), 176. doi:10.3390/molecules24010176
Barzegar, H., Mehrnia, M., Nasehi, B., and Alipour, M. (2018). Fabrication of peppermint essential oil nanoemulsions by spontaneous method: effect of preparing conditions on droplet size. Flavour Fragr. J. 33 (5), 351–356. doi:10.1002/ffj.3455
Batista, D., De Oliveira, I., Ribeiro, A., Fonseca, E., Santos, N., de Sena-Filho, J., et al. (2017). Encapsulation and release of Beauveria bassiana from alginate–bentonite nanocomposite. RSC Adv. 7 (42), 26468–26477. doi:10.1039/C7RA02185B
Bejarano, A., and Puopolo, G. (2020). “Bioformulation of microbial biocontrol agents for a sustainable agriculture,” in How research can stimulate the development of commercial biological control against plant diseases. Editors A. De Cal, P. Melgarejo, and N. Magan (Cham, Switzerland: Springer), 275–293. doi:10.1007/978-3-030-53238-3_16
Bell, D., Wells, H., and Markham, C. (1982). In vitro antagonism of Trichoderma species against six fungal plant pathogens. Phytopathology 72 (4), 379–382. doi:10.1094/Phyto-72-379
Belščak, A., Komes, D., Karlović, S., Djaković, S., Špoljarić, I., Mršić, G., et al. (2015). Improving the controlled delivery formulations of caffeine in alginate hydrogel beads combined with pectin, carrageenan, chitosan, and psyllium. Food Chem. 167, 378–386. doi:10.1016/j.foodchem.2014.07.011
Braga, A., Costa, C., Pomella, A., Ribeiro, E., Santos, L., and Zotarelli, M. (2019). Evaluation of lethality temperature and use of different wall materials in the microencapsulation process of Trichoderma asperellum conidias by spray drying. Powder Technol. 347, 199–206. doi:10.1016/j.powtec.2019.02.037
Braga, A. B., Costa, C. J. M., Ribeiro, E. J., Zotarelli, M. F., and Santos, L. D. (2022). Evaluation of the microencapsulation process of conidia of Trichoderma asperellum by spray drying. Braz. J. Microbiol. 53 (4), 1871–1880. doi:10.1007/s42770-022-00832-z
Brondi, M., Florencio, C., Mattoso, L., Ribeiro, C., and Farinas, C. (2022). Encapsulation of Trichoderma harzianum with nanocellulose/carboxymethyl cellulose nanocomposite. Carbohydr. Polym. 295, 119876. doi:10.1016/j.carbpol.2022.119876
Bulatao, R., Samin, J., Salazar, J., and Monserate, J. (2017). Encapsulation of anthocyanins from black rice (Oryza sativa L.) bran extract using chitosan-alginate nanoparticles. J. Food Res. 6 (3), 40–47. doi:10.5539/jfr.v6n3p40
Cao, S., Li, L., Zhu, B., and Yao, Z. (2023). Alginate modifying enzymes: an updated comprehensive review of the mannuronan C5-epimerases. Algal Res. 69, 102952. doi:10.1016/j.algal.2022.102952
Chandrika, K., Prasad, R., and Godbole, V. (2019). Development of chitosan-PEG blended films using Trichoderma: enhancement of antimicrobial activity and seed quality. Int. J. Biol. Macromol. 126, 282–290. doi:10.1016/j.ijbiomac.2018.12.208
Chaudhary, T., and Shukla, P. (2020). “Commercial bioinoculant development: techniques and challenges,” in Microbial enzymes and biotechniques. Editor P. Shukla (Singapore: Springer), 57–70. doi:10.1007/978-981-15-6895-4_4
Cruz, M., Izquierdo, L., Gómez, M., Santos, A., Uribe, L., and Moreno, C. (2022). Hydrogel capsules as new delivery system for Trichoderma koningiopsis Th003 to control Rhizoctonia solani in rice (Oryza sativa). World J. Microbiol. Biotechnol. 40, 108. doi:10.1007/s11274-024-03897-0
De Oliveira, A., Locatelli, G., Barbosa, R., Lobo, M., Moura, G., and Luna, C. (2020). Preparation, characterisation and cell viability of encapsulated Trichoderma asperellum in alginate beads. J. Microencapsul. 37 (3), 270–282. doi:10.1080/02652048.2020.1729884
Elnahal, A., El-Saadony, M., Saad, A., Desoky, E., El-Tahan, A., Rady, M., et al. (2022). The use of microbial inoculants for biological control, plant growth promotion, and sustainable agriculture: a review. Eur. J. Plant Pathology 162, 759–792. doi:10.1007/s10658-021-02393-7
French, E., and Hebert, T. (1980). Métodos de Investigación Fitopatológica. San José, Costa Rica: Editorial IICA.
Gan, P., Sam, S., Abdullah, M., and Omar, M. (2020). Thermal properties of nanocellulose-reinforced composites: a review. J. Appl. Polym. Sci. 137 (11), 48544. doi:10.1002/app.48544
García, M., Valdez, O., Cabanillas, L. A., Bernal, M. J., Rivera, M. M., Gutiérrez, E. P., et al. (2023). Potential agricultural uses of micro/nano encapsulated chitosan: a review. Macromol 3 (3), 614–635. doi:10.3390/macromol3030034
Gouveia, A., Munk, M., Oliveira, V., Ferreira, A., Brandão, H., Gern, J., et al. (2020). Preparation, characterization and in vivo biocompatibility studies of cotton cellulose nanofibers. J. Nanosci. Nanotechnol. 20 (10), 6532–6541. doi:10.1166/jnn.2020.18571
Hashim, A., Hamed, S., Hamid, H., Abd-Elsalam, K., Golonka, I., Musiał, W., et al. (2019). Antioxidant and antibacterial activities of omega-3 rich oils/curcumin nanoemulsions loaded in chitosan and alginate-based microbeads. Int. J. Biol. Macromol. 140, 682–696. doi:10.1016/j.ijbiomac.2019.08.085
Herrera, W., Valbuena, O., and Pavone, D. (2020). Formulation of Trichoderma asperellum TV190 for biological control of Rhizoctonia solani on corn seedlings. Egypt. J. Biol. Pest Control 30 (1), 1–8. doi:10.1186/s41938-020-00246-9
Huang, L., Wu, K., He, X., Yang, Z., and Ji, H. (2021). One-step microfluidic synthesis of spherical and bullet-like alginate microcapsules with a core–shell structure. Colloids Surfaces A Physicochem. Eng. Aspects 608, 125612. doi:10.1016/j.colsurfa.2020.125612
Hwang, J., Kim, J., Wee, Y., Jang, H., Kim, S., and Ryu, H. (2006). Factors affecting the characteristics of melamine resin microcapsules containing fragrant oils. Biotechnol. Bioprocess Eng. 11 (5), 391–395. doi:10.1007/BF02932304
Ishak, A., Zulkepli, F., Hayin, N., Zain, N., and Sapak, Z. (2021). Effect of high inlet temperature of spray dryer on viability of microencapsulated Trichoderma asperellum conidia. IOP Conf. Ser. Earth Environ. Sci. 757 (1), 012023. doi:10.1088/1755-1315/757/1/012023
John, R., Tyagi, R., Brar, S., Surampalli, R., and Prévost, D. (2011). Bio-encapsulation of microbial cells for targeted agricultural delivery. Crit. Rev. Biotechnol. 31 (3), 211–226. doi:10.3109/07388551.2010.513327
Jurić, S., Šegota, S., and Vinceković, M. (2019). Influence of surface morphology and structure of alginate microparticles on the bioactive agents release behavior. Carbohydr. Polym. 218, 234–242. doi:10.1016/j.carbpol.2019.04.096
Jurić, S., Tanuwidjaja, I., Fuka, M., Kahlina, K., Marijan, M., Boras, A., et al. (2021). Encapsulation of two fermentation agents, Lactobacillus sakei and calcium ions in microspheres. Colloids Surfaces B Biointerfaces 197, 111387. doi:10.1016/j.colsurfb.2020.111387
Kaewkhon, W., Naksupan, N., Kamtaeja, S., Suwandee, P., Molamsa, S., and Inpan, W. (2024). Optimizing Trichoderma longibrachiatum pellets with spent mushroom substrate: a study on conidial viability and shelf life. J. Food Health Bioenvironmental Sci. 17 (3), li01.tci-thaijo.org/index.php/sdust/article/view/263700.
Khorshidian, N., Mahboubi, A., Kalantari, N., Hosseini, H., Yousefi, M., and Arab, M. (2019). Chitosan-coated alginate microcapsules loaded with herbal galactagogue extract: formulation optimization and characterization. Iran. J. Pharm. Res. 18 (3), 1180–1195. doi:10.22037/ijpr.2019.1100776
Li, W., Wei, Q., Chen, Q., and Jiang, Z. (2021). Microencapsulation and evaluation of styrene maleic anhydride/epoxy for mechanical triggering self-healing of cementitious materials. Cem. Concr. Compos. 124, 104247. doi:10.1016/j.cemconcomp.2021.104247
Lim, G., and Ahmad, M. (2017). Development of Ca-alginate-chitosan microcapsules for encapsulation and controlled release of imidacloprid to control dengue outbreaks. J. Industrial Eng. Chem. 56, 382–393. doi:10.1016/j.jiec.2017.07.035
Lin, D., Kelly, A., and Miao, S. (2021). Alginate-based emulsion micro-gel particles produced by an external/internal O/W/O emulsion-gelation method: formation, suspension rheology, digestion, and application to gel-in-gel beads. Food Hydrocoll. 120, 106926. doi:10.1016/j.foodhyd.2021.106926
Lin, N., Huang, J., Chang, P., Feng, L., and Yu, J. (2011). Effect of polysaccharide nanocrystals on structure, properties, and drug release kinetics of alginate-based microspheres. Colloids Surfaces B Biointerfaces 85 (2), 270–279. doi:10.1016/j.colsurfb.2011.02.039
Liu, C., and Liu, S. (2009). Formulation and characterization of the microencapsulated entomopathogenic fungus Metarhizium anisopliae MA126. J. Microencapsul. 26 (5), 377–384. doi:10.1080/02652040802365455
Locatelli, G., dos Santos, G., Botelho, P., Finkler, C., and Bueno, L. (2018). Development of Trichoderma sp. formulations in encapsulated granules (CG) and evaluation of conidia shelf-life. Biol. Control 117, 21–29. doi:10.1016/j.biocontrol.2017.08.020
Lotfalinezhad, E., Taheri, A., Razavi, S. E., and Sanei, S. J. (2024). Preparation and assessment of alginate-microencapsulated Trichoderma harzianum for controlling Sclerotinia sclerotiorum and Rhizoctonia solani on tomato. Int. J. Biol. Macromol. 259, 129278. doi:10.1016/j.ijbiomac.2024.129278
Løvschall, K. B., Velasquez, S. T., Kowalska, B., Ptaszek, M., Jarecka, A., Szczech, M., et al. (2024). Enhancing stability and efficacy of Trichoderma bio-control agents through layer-by-layer encapsulation for sustainable plant protection. Adv. Sustain. Syst. 8, 2300409. doi:10.1002/adsu.202300409
Lupo, B., Maestro, A., Porras, M., Gutiérrez, J., and González, C. (2014). Preparation of alginate microspheres by emulsification/internal gelation to encapsulate cocoa polyphenols. Food Hydrocoll. 38, 56–65. doi:10.1016/j.foodhyd.2013.11.003
Ma, L., Shang, Y., Zhu, Y., Zhang, X., Zhao, L., Wang, J., et al. (2020). Study on microencapsulation of Lactobacillus plantarum LIP-1 by emulsification method. J. Food Process Eng. 43 (8), e13437. doi:10.1111/jfpe.13437
Mancera, M., Izquierdo, W., Escalante, A., Ibarra, J., and Barrera, J. (2019). Encapsulation of Trichoderma harzianum conidia as a method of conidia preservation at room temperature and propagation in submerged culture. Biocontrol Sci. Technol. 29 (2), 107–130. doi:10.1080/09583157.2018.1535053
Martinez, Y., Ribera, J., Schwarze, F. W., and De France, K. (2023). Biotechnological development of Trichoderma-based formulations for biological control. Appl. Microbiol. Biotechnol. 107 (18), 5595–5612. doi:10.1007/s00253-023-12687-x
Martins, E., Poncelet, D., Marquis, M., Davy, J., and Renard, D. (2017). Monodisperse core-shell alginate (micro)-capsules with oil core generated from droplets millifluidic. Food Hydrocoll. 63, 447–456. doi:10.1016/j.foodhyd.2016.09.018
Maruyama, C. R., Bilesky-José, N., de Lima, R., and Fraceto, L. F. (2020). Encapsulation of Trichoderma harzianum preserves enzymatic activity and enhances the potential for biological control. Front. Bioeng. Biotechnol. 8, 225. doi:10.3389/fbioe.2020.00225
Mohamed, S., Hossain, M., Mohamad, M., Ahmad, M., Omar, F., Balakrishnan, V., et al. (2021). Recycling waste cotton cloths for the isolation of cellulose nanocrystals: a sustainable approach. Polymers 13 (4), 626. doi:10.3390/polym13040626
Mulatu, A., Alemu, T., Megersa, N., and Vetukuri, R. R. (2021). Optimization of culture conditions and production of bio-fungicides from Trichoderma species under solid-state fermentation using mathematical modeling. Microorganisms 9 (8), 1675. doi:10.3390/microorganisms9081675
Murugappan, V., and Muthadhi, A. (2022). Studies on the influence of alginate as a natural polymer in mechanical and long-lasting properties of concrete – a review. Mater. Today Proc. 65, 839–845. doi:10.1016/j.matpr.2022.03.424
Nandiyanto, A. B. D., Ragadhita, R., and Fiandini, M. (2023). Interpretation of Fourier Transform Infrared Spectra (FTIR): a practical approach in the polymer/plastic thermal decomposition. Indonesian J. Sci. Technol. 8 (1), 113–126. doi:10.17509/ijost.v8i1.53297
Nikoo, A., Kadkhodaee, R., Ghorani, B., Razzaq, H., and Tucker, N. (2018). Electrospray-assisted encapsulation of caffeine in alginate microhydrogels. Int. J. Biol. Macromol. 116, 208–216. doi:10.1016/j.ijbiomac.2018.04.167
Nofal, A. M., El-Rahman, M. A., Abdelghany, T. M., and Abd El-Mongy, M. (2021). Mycoparasitic nature of Egyptian Trichoderma isolates and their impact on suppression Fusarium wilt of tomato. Egypt. J. Biol. Pest Control 31, 103–108. doi:10.1186/s41938-021-00450-1
Poveda, J. (2021). Trichoderma as biocontrol agent against pests: new uses for a mycoparasite. Biol. Control 159, 104634. doi:10.1016/j.biocontrol.2021.104634
Przyklenk, M., Vemmer, M., Hanitzsch, M., and Patel, A. (2017). A bioencapsulation and drying method increases shelf life and efficacy of Metarhizium brunneum conidia. J. Microencapsul. 34 (5), 498–512. doi:10.1080/02652048.2017.1354941
Qi, Q., Fan, C., Wu, H., Sun, L., and Cao, C. (2023). Preparation of Trichoderma asperellum microcapsules and biocontrol of cucumber powdery mildew. Microbiol. Spectr. 11 (3), e0508422. doi:10.1128/spectrum.05084-22
Qu, B., and Luo, Y. (2020). Chitosan-based hydrogel beads: preparations, modifications and applications in food and agriculture sectors – a review. Int. J. Biol. Macromol. 152, 437–448. doi:10.1016/j.ijbiomac.2020.02.240
R Core Team (2020). R: a language and environment for statistical computing. Vienna, Austria: R Foundation for Statistical Computing. Available at: https://www.R-project.org/.
Redda, E., Ma, J., Mei, J., Li, M., Wu, B., and Jiang, X. (2018). Antagonistic potential of different isolates of Trichoderma against Fusarium oxysporum, Rhizoctonia solani, and Botrytis cinerea. Eur. J. Exp. Biol. 8 (2), 1–8. doi:10.21767/2248-9215.100053
Rodrigues, C., Bilesky, N., de Lima, R., and Fraceto, L. (2020). Encapsulation of Trichoderma harzianum preserves enzymatic activity and enhances the potential for biological control. Front. Bioeng. Biotechnol. 8, 225. doi:10.3389/fbioe.2020.00225
Rungraung, N., Jain, S., Mitbumrung, W., Khomein, P., Suphantharika, M., McClements, D., et al. (2022). Controlling the in vitro gastrointestinal digestion of emulsified lipids by encapsulation within nanocellulose-fortified alginate beads. Food Struct. 32, 100266. doi:10.1016/j.foostr.2022.100266
Saberi-Riseh, R., Moradi, M., Mohammadinejad, R., and Thakur, V. (2021). Biopolymers for biological control of plant pathogens: advances in microencapsulation of beneficial microorganisms. Polymers 13 (12), 1938. doi:10.3390/polym13121938
Saldaña-Mendoza, S. A., Pacios-Michelena, S., Palacios-Ponce, A. S., Chávez-González, M. L., and Aguilar, C. N. (2023). Trichoderma as a biological control agent: mechanisms of action, benefits for crops and development of formulations. World J. Microbiol. Biotechnol. 39 (10), 269. doi:10.1007/s11274-023-03695-0
Sallam, N., Eraky, A., and Sallam, A. (2019). Effect of Trichoderma spp. on Fusarium wilt disease of tomato. Mol. Biol. Rep. 46, 4463–4470. doi:10.1007/s11033-019-04901-9
Sehrawat, A., Sindhu, S., and Glick, B. (2022). Hydrogen cyanide production by soil bacteria: biological control of pests and promotion of plant growth in sustainable agriculture. Pedosphere 32 (1), 15–38. doi:10.1016/S1002-0160(21)60058-9
Shi, J., Wu, X., Sun, R., Ban, B., Li, J., and Chen, J. (2019). Nano-encapsulated phase change materials prepared by one-step interfacial polymerization for thermal energy storage. Mater. Chem. Phys. 231, 244–251. doi:10.1016/j.matchemphys.2019.04.032
Silva, K., Carvalho, A., Rabelo, R., and Hubinger, M. (2019). Sacha inchi oil encapsulation: emulsion and alginate beads characterization. Food Bioprod. Process. 116, 118–129. doi:10.1016/j.fbp.2019.05.001
Singh, T., and Chittenden, C. (2021). Synergistic ability of chitosan and Trichoderma harzianum to control the growth and discolouration of common sapstain fungi of Pinus radiata. Forests 12 (5), 542. doi:10.3390/f12050542
Siqueira, P., Siqueira, É., De Lima, A., Siqueira, G., Pinzón, A., Lopes, A., et al. (2019). Three-dimensional stable alginate-nanocellulose gels for biomedical applications: towards tunable mechanical properties and cell growing. Nanomaterials 9 (1), 78. doi:10.3390/nano9010078
Soni, B., and Mahmoud, B. (2015). Chemical isolation and characterization of different cellulose nanofibers from cotton stalks. Carbohydr. Polym. 134, 581–589. doi:10.1016/j.carbpol.2015.08.031
Sornakili, A., Thankappan, S., Sridharan, A., Nithya, P., and Uthandi, S. (2020). Antagonistic fungal endophytes and their metabolite-mediated interactions against phytopathogens in rice. Physiological Mol. Plant Pathology 112, 101525. doi:10.1016/j.pmpp.2020.101525
Sridharan, A., Sugitha, T., Karthikeyan, G., Nakkeeran, S., and Sivakumar, U. (2021). Metabolites of Trichoderma longibrachiatum EF5 inhibits soil borne pathogen, Macrophomina phaseolina by triggering amino sugar metabolism. Microb. Pathog. 150, 104714. doi:10.1016/j.micpath.2020.104714
Sundaramoorthy, S., and Balabaskar, P. (2013). Biocontrol efficacy of Trichoderma spp. against wilt of tomato caused by Fusarium oxysporum f. sp. lycopersici. J. Appl. Biol. Biotechnol. 1 (3), 036–040. doi:10.7324/JABB.2013.1306
Supramaniam, J., Adnan, R., Kaus, N. H. M., and Bushra, R. (2018). Magnetic nanocellulose alginate hydrogel beads as potential drug delivery system. Int. J. Biol. Macromol. 118, 640–648. doi:10.1016/j.ijbiomac.2018.06.043
Szczech, M., and Maciorowski, R. (2016). Microencapsulation technique with organic additives for biocontrol agents. J. Hortic. Res. 24 (1), 111–122. doi:10.1515/johr-2016-0013
TariqJaveed, M., Farooq, T., Al-Hazmi, A., Hussain, M., and Rehman, A. (2021). Role of Trichoderma as a biocontrol agent (BCA) of phytoparasitic nematodes and plant growth inducer. J. Invertebr. Pathology 183, 107626. doi:10.1016/j.jip.2021.107626
Teo, A., Goh, K., Wen, J., Oey, I., Ko, S., Kwak, H., et al. (2016). Physicochemical properties of whey protein, lactoferrin and Tween 20 stabilised nanoemulsions: effect of temperature, pH and salt. Food Chem. 197, 297–306. doi:10.1016/j.foodchem.2015.10.086
Vinceković, M., Jalsenjak, N., Topolovec-Pintaric, S., Đermić, E., Bujan, M., and Juric, S. (2016). Encapsulation of biological and chemical agents for plant nutrition and protection: chitosan/alginate microcapsules loaded with copper cations and Trichoderma viride. J. Agric. Food Chem. 64 (43), 8073–8083. doi:10.1021/acs.jafc.6b02879
Vinceković, M., Jurić, S., Đermić, E., and Topolovec-Pintarić, S. (2017). Kinetics and mechanisms of chemical and biological agents release from biopolymeric microcapsules. J. Agric. Food Chem. 65 (44), 9608–9617. doi:10.1021/acs.jafc.7b04075
Wickham, H. (2016). ggplot2: elegant graphics for data analysis. New York: Springer-Verlag. Available at: https://ggplot2.tidyverse.org.
Yang, Z., Song, B., Li, Q., Fan, H., and Ouyang, F. (2004). Effects of surfactant and acid type on preparation of chitosan microcapsules. China Particuology 2 (2), 70–75. doi:10.1016/S1672-2515(07)60026-8
Keywords: microcapsules, alginate, nanocellulose, chitosan, phytopathogenic controller
Citation: Arias-Chavarría LD, Batista-Menezes D, Orozco-Cayasso S, Vargas-Martínez A, Vega-Baudrit JR and Montes de Oca-Vásquez G (2025) Evaluation of the viability of microencapsulated Trichoderma longibrachiatum conidia as a strategy to prolong the shelf life of the fungus as a biological control agent. Front. Chem. 12:1473217. doi: 10.3389/fchem.2024.1473217
Received: 30 July 2024; Accepted: 30 December 2024;
Published: 15 January 2025.
Edited by:
Shahid Ul Islam, Jamia Millia Islamia, IndiaReviewed by:
Divya Koilparambil, Apple International School, United Arab EmiratesTengxiao Ji, University of British Columbia, Canada
Manju Nehra, Chaudhary Devi Lal University, India
Copyright © 2025 Arias-Chavarría, Batista-Menezes, Orozco-Cayasso, Vargas-Martínez, Vega-Baudrit and Montes de Oca-Vásquez. This is an open-access article distributed under the terms of the Creative Commons Attribution License (CC BY). The use, distribution or reproduction in other forums is permitted, provided the original author(s) and the copyright owner(s) are credited and that the original publication in this journal is cited, in accordance with accepted academic practice. No use, distribution or reproduction is permitted which does not comply with these terms.
*Correspondence: Gabriela Montes de Oca-Vásquez, bW1vbnRlc2Rlb2NhQHV0bi5hYy5jcg==