- Department of Chemistry, University of Bergen, Bergen, Norway
Polyoxometalates (POMs) are a class of compounds known for the vast range of tunable structures and properties available, leading to applications in areas such as catalysis, energy, and advanced medicine. The ability to covalently functionalize POMs with organic components has been investigated extensively to tune the physical and chemical properties of the resulting hybrid materials. These hybrids, where the organic entity is covalently attached to the POM-core (Class II hybrid POMs) result in a vast library of promising customizable catalytic systems, displaying tunable properties with a high level of synergy between the polyanion and the organic component. A number of Class II hybrids have been investigated for a wide range of catalytic applications, and here, we give a brief overview of Class II hybrids of the p-block elements and their applications in catalysis.
1 Introduction
Polyoxometalates (POMs) represent an exceptional group of metal-oxo nanoclusters, comprising metal-oxygen-metal linkages, typically constituted of early transition metals (group V or VI) in their highest oxidation states, with a large degree of structural and compositional versatility (Pope and Müller, 1991). POMs are obtained by condensation processes of simple anions in solution via careful tuning of the synthetic parameters i.e., concentration, pH, counter-cations, and temperature conditions (Dolbecq et al., 2010; Hutin et al., 2013). Due to the vast range of possible structures available and the possibility to control and fine-tune their properties, POMs are potential candidates for applications in life science (Bijelic et al., 2019), molecular magnetism (Clemente-Juan et al., 2012), and catalysis (Weinstock et al., 2018).
The presence of terminal and bridged oxygen atoms in POMs highlights their intrinsic potential to be applied as inorganic multidentate ligands with versatile topologies. This is particularly evident in the lacunary species, where one or more metal addenda are missing compared to the “plenary” or complete parent polyanion (Long et al., 2006). A variety of organic entities have been covalently grafted to POMs via these lacunary sites, by employing for example, organosilicon functionalization. Another approach is the use of organic alkoxy groups which bond to plenary polyanions like on the Anderson-POM via esterification or condensation reactions with protonated bridging oxo groups on the POMs (Chen and Zubieta, 1992). Both these examples are categorized as Class II hybrids, where the organic and inorganic moieties are linked via covalent or iono-covalent bonds (Dolbecq et al., 2010; Proust et al., 2012). Class II hybrids provide good chemical control of the bridging unit and hence the interaction between the units, as well as spatial control of the resulting compounds (Kibler and Newton, 2018). Although Class-II hybrids provide enhanced versatility of POM archetypes for tailoring the properties of resulting compounds for specific applications, the covalent or iono-covalent bonds between the POM core and organic ligands are more vulnerable to hydrolysis. Their hydrolytic stability is largely affected by temperature, nature of polyanion, type of organic ligand, and especially extreme pH conditions (Dolbecq et al., 2010). However, studies showed improved hydrolytic stability under certain physicochemical conditions (Hou and Hill, 1993; Al-Sayed et al., 2015; Blazevic et al., 2015; Salazar Marcano et al., 2021; Nowicka et al., 2024).
POMs have been intensely studied due to their versatile structures and tunability of respective properties, and have been investigated for a large number of catalytic applications, see for example, review by Yang (Wang and Yang, 2015). Class II hybrid POMs are promising firstly due to the excellent control and rational design of molecular assemblies, secondly, due to their versatility in liquid organic reactions arising from the wide variety of organic groups and the possibility to fine-adjustment the electronic surface states, and finally, for heterogenization purposes where a known catalyst is tethered to a POM-unit simply for heterogenization of the otherwise homogeneous catalyst. Interestingly, such tethering to POMs has also been reported to increase the catalytic activity, highlighting how the nature of the bridge between the two parts is essential for tuning the catalytic activity (Bar-Nahum et al., 2003; Mirkhani et al., 2008a). An overview of Class II hybrid POMs in catalysis is shown in Figure 1.
One of the key aspects for POMs in catalysis is the ability of polyoxoanions to be reversibly reduced without significant structural deformation, and due to the high number of metal centers present, POMs can undergo multi-electron redox processes. This has led to a large activity investigating POMs as photocatalysts. The visible light absorption of POMs is typically low (it is predominantly in the UV-region), but the visible light absorption can be increased by coupling POMs to suitable photosensitizers (PS), where continuous photo-excited electrons from a PS can be transported rapidly to the POM-core for e.g., photocatalytic hydrogen evolution reactions (HER). It is also reported that this can increase the lifetime of photo-generated carriers in the PS and that the electro-hole pair recombination will be suppressed (Li et al., 2020).
The coupling with photosensitizers in both Class I and Class II hybrids can allow for light-driven HER, where reports suggest that the effect is largest when utilizing Class II hybrids (Luo et al., 2020a). A much studied Class I hybrid is the tetrabutyl ammonium salt of decatungstate (N(C4H9)4[W10O32]), with its still-unparalleled reactivity, which is found to be active in light-driven direct hydrogen atom transfer reactions (Waele et al., 2016). Class I hybrid POMs can affect the catalytic properties of the system by steering for example, the catalyst solubility and even affect the catalysts formal reversible redox potentials by utilizing ionic liquids (Zhao and Bond, 2009). Despite the fact that Class II hybrid POMs often are more challenging to synthesize, Class II hybrids have the benefits of being able to tune (I) the electronic structure of the resulting catalyst system, with a reported stronger electronic coupling between the photosensitizer and the POM compared with Class I (Amthor et al., 2022), (II) the solubility, (III) the number of active sites, and (IV) the Brønsted acid activity (Lachkar et al., 2016). Several review papers have recently been published on hybrid POMs (Dolbecq et al., 2010; Proust et al., 2012; Kibler and Newton, 2018; Gouzerh and Proust, 1998; Proust et al., 2008; Long et al., 2010; Zhang et al., 2012; Ivanova, 2014; Liu et al., 2012; Mirzaei et al., 2014; Nisar and Wang, 2012; Nlate and Jahier, 2013; Ren et al., 2015; Santoni et al., 2014; Song and Tsunashima, 2012; Anyushin et al., 2020; Najafi, 2023). For details on the synthetic approaches and challenges, see for example, reviews by Proust (Proust et al., 2008), Dolbecq (Dolbecq et al., 2010), and Parac-Vogt (Anyushin et al., 2020). This review is aimed at giving a short overview of the Class II hybrids where the bridge between the polyanion and the organic unit is via p-group elements, and their applications in catalysis.
2 Class II hybrid POMs and their application in catalysis
To give a comprehensive overview of Class II hybrids in catalysis we focus on Class II hybrids of triol (Liu et al., 1987), imido (Du et al., 1992), organophosphorus (Kwak et al., 1975), organosilicon (Knoth, 1979), organotin (Sazani et al., 2000), and organoarsonic (Barkigia et al., 1981) functionalization. An overview is presented in Figure 2. There are several other examples of Class II hybrids employing other p-group elements such as organodiazenido (Hsieh and Zubieta, 1986), organogermanium (Sazani and Pope, 2004), and organoantimony (Piedra-Garza et al., 2009) functionalization, but to the best of our knowledge there are no reports on their catalytic activity.
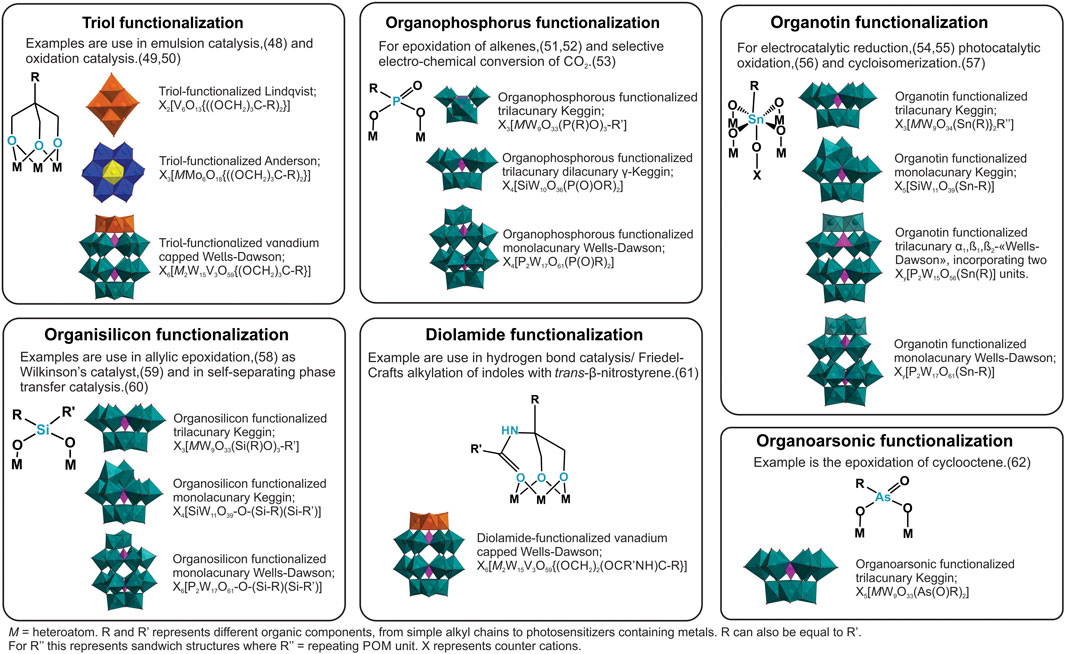
Figure 2. The different types of organo-functionalization discussed here, and relevant POM-archetypes with catalysis examples.
2.1 Triol functionalization
The first report on the synthesis of organic-alkoxy derivatives is credited to Knoth in 1981, where both the molybdenum and tungsten versions of the Keggin polyanion were successfully functionalized [PMo12O39(OMe)]2− and [PW12O39(OMe)]2− (Knoth and Harlow, 1981). The first report of tripodal-functionalized (triol-functionalized) Anderson was reported in 2002 (Hasenknopf et al., 2002), in 1990 for hexavanadate (Chen and Zubieta, 1990), and in 1993 for the Wells-Dawson (Hou and Hill, 1993). The major exploration of catalytic applications of alkoxy-functionalized covalent hybrids is focused on the Anderson POM, {MMo6O18((OCH2)3C-R)2}, in addition to some hexavanadate {V6O13((OCH2)3C-R)2}, and some Wells-Dawson {P2V3W15O59((OCH2)3CR)} examples.
2.1.1 Hybrid Anderson POMs
The electropolymerization of a Mn-Anderson POM bearing two pyridylgroups [MnMo6O18{(OCH2)3CNHCO(4-C5H4N)}2]3−, with zinc octaethylporphyrins, results in highly ordered hybrid copolymers. The hybrid polymers act as catalysts for the photocatalytic reduction of AgI2SO4 under visible light and aerobic conditions in the presence of propan-2-ol (Schaming et al., 2010). A tetrabromo-bifunctionalized Mn-Anderson POM has been successfully included in conjugated microporous polymers via Sonogashira–Hagihara cross-coupling with 1,3,5-triethynylbenzene. The polymers exhibited outstanding heterogeneous photocatalytic activities for degrading organic dyes in water, where hydrogen peroxide and singlet oxygen are the primary active catalytic species (Li et al., 2017).
A series of Anderson POM dyads [MMo6O18{(OCH2)3CNH2}2]3−, with M = Mn3+, Fe3+, Co3+, coupled to an established iridium photosensitizer have been investigated for light-driven hydrogen evolution reaction (HER), where the highest intermolecular excited states were observed for Mn (Schönweiz et al., 2017). The investigation of the photoinduced electron-transfer processes in the dyads found that it was the yield rather than the lifetime of the charge-separated states which determine the catalytic capacity (Luo et al., 2020a). Comparing the structural variation of linkages using an imine (Schiff-base) bond vs. a direct C-C bond it was found that the complex with the imine bond increases the HER activity, which might be (partially) attributed to the slightly higher charge-separation yield for the imine-complex (Luo et al., 2020b). The HER activity of a covalently linked Anderson/Pt-complex has also been reported, where the POM acts as a photosensitizer/catalyst-binding site and facilitates light-induced charge-transfer and catalytic turnover (Maloul et al., 2021).
The one-sided tris-functionalized Fe-Anderson POM [[NH2C(CH2O)3]Fe(OH)3Mo6O18]3-, has been employed as a molecular heterogenous catalyst for the selective and effective aerobic oxidation of a diverse range of aldehydes to the corresponding carboxylic acids in aqueous media (Yu et al., 2017). A Cr-Anderson POM {CrMo6O18(OH)3[(OCH2)3CCH2OH]}, has been reported as a catalyst for oxidative esterification of alcohols, using low catalyst loading and avoiding expensive ligands in the catalyst system (Wang et al., 2021). The Al-Anderson POM, {AlMo6O18(OH)3[(OCH2)3CCH3]}, has been reported as a catalyst for the oxidation of alcohols to aldehyde, where it was found that added halide ions blocked the catalytic activity by forming hydrogen bonding to the “non-functionalized” side of the POM, which can be reversed by the addition of water (She et al., 2019).
A bi-functionalization on one side of the β-Mn-Anderson POM, β-{[H3NC(CH2O)3]2MnMo6O18} is reported to behave as a highly active catalyst for selective oxidation of cyclohexanone and cyclohexanol, as well as their mixture, due to the presence of the Mn3+ atom site and the topology (Luo et al., 2018).
2.1.2 Hybrid hexavanadate POMs
Both one and two-sided functionalization of the hexavanadate, {V6O13((OCH2)3CCH2OH)((OCH2)3CCH2OOC(CH2)16CH3)} and {V6O13((OCH2)3CCH2OOC(CH2)16CH3)2}, have been found to be highly efficient emulsion catalysts in oxidation desulfurization processes (Yin et al., 2012). A derivative containing free carboxyl groups, {V6O13((OCH2)3CCOOH)2}, formed POM-based coordination polymers upon reaction with zinc ions (Bayaguud et al., 2016). The resulting 2D nanosheets demonstrated high catalytic activity for the aerobic oxidation of propanethiol to its corresponding disulfide under mild conditions. A Lindqvist POM attached to porphyrin units coordinated to Zn; {V6O13{C61H58N5O4Zn}2}, is reported to have an effective photocatalytic activity for degrading the water-soluble pollutant, Rhodamine B (RhB), under visible-light illumination. The activity is ascribed to the possible energy and electron transfer between the POM cluster and the porphyrin (Zhu et al., 2020).
2.1.3 Hybrid Wells-Dawson POMs
A norbornene monomer containing organofunctionalized {P2V3W15O62} POM shows that the catalytic activity of the hybrid for the oxidation of a sulfide was retained when the same hybrid-containing monomer was incorporated in a norbornene-type polymer (Miao et al., 2014). A Cu–dipyridylamine tethered to a Dawson-type {P2V3W15O62} POM shows photo-redox properties, where the complex exhibit the ability to store up to three reducing equivalents upon irradiation with visible light. Moreover, its ability to catalytically photogenerate CF3 radicals, in the presence of Togni’s reagent is demonstrated (Wang et al., 2023).
2.2 Organotin functionalization
Organotin functionalized derivatives of lacunary POMs were first reported by Knoth, with several tungsten-based Keggin-type POMs, such as {SiW11O39} functionalized with R2SnCl3 to give {SiW11O39(SnR)} (Knoth, 1979).
2.2.1 Hybrid Wells-Dawson POMs
A tetra (carboxyethyltin)-decorated POM, {K2Na2{Sn(CH2)2COO}4(H2O)2{WO5(H2O)}(P2W15O56)2}, was reported as an electrocatalyst for the reduction of H2O2. Electrochemical studies demonstrated that it is the reduced hybrid POM species that are electrocatalytically active in the reduction (Zhang et al., 2010). A sandwich-type carboxyethyltin functionalized Keggin POM [K3{Sn(CH2)2COO}2(A-α-PW9O34)2]11−, can function as an electrocatalyst for the reduction of H2O2, showing enhanced catalytic performance for the oxidation of cyclohexanol compared to its parent species, with good recyclability without the loss of catalytic activity (Yang et al., 2013). An estertin derivative of tungstoarsenate Keggin, {(W4O16){Sn(CH2)2COO}4(B-α-AsW9O33)2}, is reported to act as an efficient, eco-friendly, green catalyst for cyclohexanol oxidation and photocatalytic degradation of RhB, and for H2O2 detection (Ji et al., 2016).
Catalytically active gold complexes such as [AuPPh3]+ can be tethered to the Wells-Dawson POM, {P2W17O61{Sn(CH2)2CO}. The resulting catalysts extend the scope of the cycloisomerization of allenols to substrates that are extremely sensitive to dehydration, as well as stabilizing the cationic gold active sites, which lead to the recycling of the hybrid gold-POM catalysts (Dupré et al., 2012). The Keggin POM functionalized with a photosensitive organotin moiety {SiW11(Sn(CH2)2HCNC16H9)O39} has been reported as an eco-friendly, visible light photocatalyst for aerobic oxidation of sulfides to sulfoxides in good yield at low cost. The covalent tethering significantly reduced the band gap of POM, allowing it to absorb visible light and to conduct catalytic reactions under visible light (Karimian and Zangi, 2021).
2.3 Organosilicon functionalization
Organosilicon functionalization is reported for only certain polyoxotungstate versions of the Wells-Dawson and Keggin based structures; {XW9O33}, {XW10O38}, {XW11O39} and the {X2W17O61}. Knoth et al. first reported the reactions of RSiCl3 (R = C2H5, C6H5, NC(CH2)3, C3H5) with {SiW11O39} (Knoth, 1979).
2.3.1 Hybrid Keggin POMs
The first metallosalen-type ligands anchored on the Keggin POM {SiW11O39} were reported in 2003 (Bar-Nahum et al., 2003). Several {M(salen)-SiW11O39} hybrids (M = Co, Fe, Cu, Ni, etc.) followed (Mirkhani et al., 2008a; Mirkhani et al., 2007; Mirkhani et al., 2008b; Moghadam et al., 2010a; Moghadam et al., 2010b), which are reported to exhibit strong electronic effects attributed to the attachment of the metallosalen complex to the POM, leading to an intramolecular charge transfer. This effect was successfully employed in homogeneous (Mirkhani et al., 2007; Mirkhani et al., 2008b; Moghadam et al., 2010b; Karimian et al., 2014) and heterogeneous catalysis (Mirkhani et al., 2008a; Moghadam et al., 2010a) of alkenes with H2O2 where the hybrid POM shows higher catalytic activity than the metallosalen complexes alone.
A study of alkene epoxidation catalyzed by [α-B-SbW9O33(tBuSiO)3Ti(OiPr)]3– highlights how the allylic epoxidation occurred at the molecular level with aqueous solutions of H2O2. Two modified catalysts, i.e, [α-B-SbW9O33(RSiO)3Ti-(OiPr)]3– (R = iPr, and nPr), were able to epoxidize both functionalized and nonfunctionalized substrates (Solé-Daura et al., 2020).
The Wilkinson’s catalyst (Rh(I)Cl(PPh3)3) inspired POM, {SiW11O39[O(SiCH2CH2PPh2)2PPh3Rh(I)Cl]}, has been employed as an effective, recyclable catalyst for the hydrogenation of alkenes under monophasic and aqueous biphasic reaction conditions (Bar-Nahum and Neumann, 2003). The {Pd(salen)–SiW11O39} was investigated for the Suzuki cross-coupling reaction in EtOH/H2O under mild reaction conditions, where the hybrid derivative showed greatly improved catalytic activity. For the coupling of aryl bromides and iodides with both deactivated and sterically hindered aryl-boric acids, both high levels of reactivity and turnover numbers were obtained (Tong et al., 2014).
An anthracene functionalized {SiW11O39} showed good photocatalytic activity for the oxidation of alcohols to carbonyl compounds under aerobic conditions, with good yields (Karimian et al., 2014). TiIV complexes, i.e, [XW9O34–x (tBuSiO)3Ti(OiPr)]3− (X = P, x = 0; X = Sb, x = 1,2), are reported as relevant soluble models for heterogeneous titanium silicalite epoxidation catalysts, showing high selectivity in allylic alcohol epoxidation (Zhang et al., 2018a). A silanol-decorated POM, [SbW9O33(tBuSiOH)3]3−, can stabilize a low-valent d2-vanadiumIII ion in a trigonal coordination environment to aid its distribution on silica or anatase surfaces, resulting in the formation of a vanadiumIII POM i.e, [SbW9O33(tBuSiO)3V(tetrahydrofuran)]3-, where the VIII entity behaves as a putative intermediate in a Mars–van Krevelen type mechanism for partial oxidation of light alcohols (Zhang et al., 2018b). Amphiphilic {PW11O39-O-(Si(CH2)nCH3)2} (n = 2, 7, 11), has been reported, arguing that it is a suitable catalyst for the oxidation of benzyl alcohol at room temperature (Bai et al., 2020).
Sulfonic-acid functionalized POMs [SiW11O39–O(SiC3H6SO3H)2)]4– and [SiW11O39–(O(SiC8H8SO3H)2)]4–, alter the behavior of [SiW11O39]8– as an electron-absorbing group to promote H+ release and enhance acidity by decreasing the electron density of O–H on the surface of sulfonic acid groups. The π–π interactions of the benzene ring enervated the dissociation of H+ and displayed weak acid-catalyzed hydroxyalkylation and alkylation characteristics (Lian et al., 2022).
Nanoparticles based on alkyl-thiol functionalized Keggin POM [SiW11O40(SiCH2CH2CH2SH)2]4–, were employed as binary catalysts for the aerobic oxydehydrogenation of reactive allylic tertiary carbon-hydrogen bonds i.e., vinylcyclohexene and vinylcyclohexane to styrene, where the hybrid POM stabilized the Pd-nanoparticles (De Bruyn and Neumann, 2007). Organosilyl-POMs have also contributed to catalytic photooxygenation in water under heterogeneous conditions (Bonchio et al., 2004), in catalyzing C–C cross-coupling and aromatic dehalogenation (Berardi et al., 2010), in oxidative desulfurization of organic sulfides, (Carraro et al., 2012), and as oxidation catalysts with H2O2 under microwave irradiation for diverse substrates (Carraro et al., 2006). Finally, by covalent modification with graphitic carbon nitride the O2 adsorption of [SiW11O39]8− is enhanced, and exhibits excellent activity in photocatalytic H2O2 production, stabilizing the formed H2O2 under sunlight irradiation (Zhao et al., 2018).
2.3.2 Hybrid Wells-Dawson POMs
A catalytically active Wells-Dawson POM, the {α2-P2W17O61(SiC6H4CH2N3)2O}, has been covalently attached to a microporous resin via click chemistry, and the resulting resin was evaluated for the oxidation of tetrahydrothiophene, with good recyclability and reusability without any detectable loss of catalytic activity nor leakage of inorganic components (Xiao et al., 2013).
The covalent tethering of [P2W17O61]8− with sulfonic acid-based organosilane entities resulted in a catalyst with improved esterification efficacy, owing to effective interactions between substrates and the catalyst. The ingrained emulsification–precipitation cycle induces excellent self-recycling properties in the catalytic system, leading to facile and low-cost catalyst recovery at high yields (Lian et al., 2020).
2.4 Diolamide functionalization
The first report of a diolamide functionalized POM was on the Dawson type with 2-amino-2-ethyl-1,3-propanediol [P2V3W15O59{(OCH2)2C(Et)NHCOCH3}]5-, introducing a new family of acidic POMs, in which the acidity of an amide proton is exalted upon grafting to the vanadium-oxide crown (Li et al., 2009).
By using the diolamide functionalization approach to insert tertiary amides and carbamates it was found that these Dawson-based hybrids showed catalytic activity for the oxidation of sulfides with high selectivity (Oble et al., 2011), and because the electron-withdrawing character of the polyanion increases the acidity of the amide proton, the hybrid can be used as a Brønsted organo-catalyst and for the hydrogenation of quinolines (Lachkar et al., 2016). Picolinic amide derivative-functionalized Dawson POM, generating stable palladacycles, is reported as a catalyst for Heck coupling (Riflade et al., 2013). and as an electrophilic catalyst in the allylation of aldehydes and imines (Riflade et al., 2014). Later, it was found that the insertion of a urea-carbonyl into the POM activates the urea toward hydrogen-bond catalysis. The H-bond catalysis of the hybrid POM was tested for Friedel-Crafts arylation of trans-β-nitrostyrene, where it showed improved overall efficiency due to the electron-withdrawing nature of POM scaffold and the catalyst could be recycled several times (Vilona et al., 2021).
2.5 Organophosphorus functionalization
Organophosphorus derivatives were first synthesized by Hill et al. by reaction between the monovacant species α-[SiW11O39]8- or α-[PW11O39]7- with PhPOCl2, resulting in the formation of the hybrids α-[(PhPO)2SiW11O39]4- and α-[(PhPO)2PW11O39]3-, respectively (Kim et al., 1992).
2.5.1 Hybrid Keggin POMs
Several organophosphrous (and organosilicon) hybrids have been screened as oxidative catalysts with H2O2 under microwave (MW) irradiation, where it was found that [γ-SiW10O36(PhPO)2]4- was the best-performing catalyst for a broad scope of reactions; from the epoxidation of terminal and internal double bonds and sulfoxidation to oxygen transfer to electron-deficient substrates such as sulfoxides (Carraro et al., 2006). H2O2-based epoxidation of internal and terminal double bonds in hydrophobic ionic liquids was also reported for [γ-SiW10O36(PhPO)2]4-, with selectivity >99% and up to 200 turnovers per minute under MW irradiation (Berardi et al., 2007). The first example of a tri-phosphopohrous functionalized polyoxotungstate, K4H6[H4{(AsW9O33)Zn(H2O)W5O11(N(CH2PO3)3)}2(μ2-O)2], was evaluated as a catalyst for alkene epoxidation with H2O2 in acetonitrile (Huo et al., 2015). Organophosphorus derivatives of the trivacant {AsW9O33} POM have also been reported as an efficient catalyst for the epoxidation of cyclooctene and cyclohexene with H2O2 (see section 2.6) (Bentaleb et al., 2015; Makrygenni et al., 2020). Three sandwich-type organophosphorous-functionalized clusters {[Ni(H2O)5]x(AsW6O21)2[Ni(OOCCH2NCH2PO3)2]3} (x = 0, 1, 3), exhibited efficient catalytic performance for the oxidation of allylic alcohols to epoxides in water with H2O2 (Xu et al., 2018).
2.5.2 Hybrid polyoxomolybdats
A crown-shaped 36-molybdic-18-phosphorous POM, where copper was grafted into the polyanions via the amino trimethylene phosphonic acid-based unit, was tested as a heterogeneous catalyst for selective oxidation of sulfides to sulfoxides with impressive catalytic and selective performances after heat treatment (Xu et al., 2020a). Later, the (NH4)17Na7H12 [Co(H2O)TeMo6O21{N(CH2PO3)3}]6·42H2O, was reported as the first polyoxomolybdate-based heterogeneous catalyst for Knoevenagel condensation with efficient catalysis and good recyclability (Xu et al., 2020b). The hybrid polyoxomolybdate (NH4)18Na7H11[Zn(H2O)TeMo6O21{N(CH2PO3)3}]6·23H2O have been evaluated for the catalytic oxidation of thioanisole to sulfoxides (Kong et al., 2021a), whilst the (NH4)6Na3H13[TeMo10O37{CoMo2O6((O3P)2C(O)(CH2)3NH2)}4]·11H2O have been employed as heterogeneous catalysts for the Knoevenagel condensation reaction (Kong et al., 2021b).
2.5.3 Hybrid Wells-Dawson POMs
K6[P2W17O57(PO5H5C7)2]⋅6C4H9NO has been reported with enhanced redox behavior and photochemistry compared to its purely inorganic counterpart, where it was found that the hybridization of the POM with electron-withdrawing moieties reduced the HOMO–LUMO gap. This facilitated direct visible-light photoactivation, establishing a simple, cheap, and effective approach for the generation of visible-light-activated hybrid nanomaterials (Cameron et al., 2017). A range of organophosphorous-based assemblies of [P2W17O61]10− were explored for fine-tuning of the electron-withdrawing properties in covalently bonded phosphonate groups, providing an orbital engineering approach for tuning the visible-light photocatalytic activity of the hybrid POM system (Fujimoto et al., 2017).
A photochemical molecular dyad composed of a ruthenium-complex photosensitizer covalently linked to a Dawson POM, α-[P2W17O61]10−, has been reported as an electron-storage site and hydrogen-evolving catalyst, with on-demand hydrogen release made possible by the addition of a proton donor to the dyad solution (Amthor et al., 2022). A bis-Ru-polypyridyl substituted hybrid POM, {(Ru(dmbpy)(ppt)Cl)2P2W17O57}, where dmbpy = 4,4′-dimethyl-2,2′-dipyridyl, and ppt = 4′-(4-phosphonophenyl)-2,2′:6′,2″-terpyridine), enabled the selective electrochemical conversion of CO2 to H2/CO or formic acid in the presence of a proton donor in solution state. It was demonstrated that the choice of the obtained C1 feedstocks can be controlled by managing the acidity of reaction media (Kuramochi et al., 2023).
2.6 Organoarsonic functionalization
The first report of structurally characterized organoarsonic functionalized POM was disclosed by Pope’s group in 1976 (Kwak et al., 1976).
Organoarsonic functionalization of the tri-lacunary Keggin with free amine groups, {[PW9O34{As(O)(C6H4NH2)}2}, as well as the related organophosphorous functionalized {[AsW9O34{P(O)(CH2CH2CO2H)}2}, were shown to be efficient catalysts for cyclooctene epoxidation with aqueous H2O2 at room temperature in homogeneous conditions (Bentaleb et al., 2015). The hybrids were anchored to silica supports and again employed for the same catalytic reaction resulting in conversions in the range 19%–97% after 24 h. The difference is ascribed to the POM-organization on the silica-supports. {[PW9O34{As(O)(C6H4NH2)}2} was again reported on mesoporous silica supports but using phenylene diisothiocyanate as a cross-linking agent. The catalytic activity was evaluated in the epoxidation of cyclooctene with aqueous H2O2 in acetonitrile at 50 °C (cyclooctene conversion of 97% for amine-{PW9} and 43% for the diisothiocyanate-{PW9}), which was reduced when grafted on to the silica (Makrygenni et al., 2019).
The three POMs B,α-{NaHAsW9O33{P(O)R}2} with -tBu and -CH2CH2CO2H, and A,α-{NaHPW9O34{As(O)p-C6H4NH2}2} (Makrygenni et al., 2020), has been studied for their catalytic oxidation properties for the homogeneous epoxidation of limonene with H2O2 at room or low temperature (4 °C), as well as cyclooctene and cyclohexene. The organoarsonic functionalized POM showed a lower efficiency for the conversion of limonene, but it is not apparent if this is due to the swap of heteroatom, or the different functionalization.
3 Summary and future perspectives
Herein we have provided an overview of Class II hybrid POMs in catalysis, highlighting the different types of functionalization and types of POMs that have been investigated. Class II hybrid POMs show a wide range of catalytic applications, highlighting the feasibility and flexibility of this class of materials. It is apparent that hybrid POMs have a large potential for a wide range of catalytic applications, for example, in photocatalysis. One of the major benefits for using the Class II hybrids for photocatalysis is the increase in the absorption of visible light, and this outweighs the known challenges for synthesizing more complex Class II hybrids. There are some highly successful Class I hybrids used in photocatalysis, and in particular the decatungstate POM, which have the benefits of a simpler synthetic strategy, which show high stability and the ability to undergo reversible multi-electron processes. However, for developing more efficient photocatalysts with tuenability and flexibility under visible-light, developing catalysts based on Class II hybrid POMs is an exciting and promising field. A more systematic approach comparing types of POMs, the nature of the anchoring, and properties of the attached organic entity would be beneficial for the future development of hybrid POMs in catalysis. This, in parallel with theoretical studies holds promise for future application of hybrid POMs in catalysis.
Author contributions
TI: Conceptualization, Data curation, Investigation, Validation, Visualization, Writing–original draft, Writing–review and editing. MR: Conceptualization, Data curation, Funding acquisition, Investigation, Project administration, Resources, Supervision, Validation, Visualization, Writing–original draft, Writing–review and editing.
Funding
The author(s) declare that financial support was received for the research, authorship, and/or publication of this article. The authors gratefully acknowledge financial support by the Department of Chemistry and the Faculty of Natural Sciences at the University of Bergen, and the Trond Mohn Research Foundation, Grant no. TMS2022STG02.
Conflict of interest
The authors declare that the research was conducted in the absence of any commercial or financial relationships that could be construed as a potential conflict of interest.
Publisher’s note
All claims expressed in this article are solely those of the authors and do not necessarily represent those of their affiliated organizations, or those of the publisher, the editors and the reviewers. Any product that may be evaluated in this article, or claim that may be made by its manufacturer, is not guaranteed or endorsed by the publisher.
References
Al-Sayed, E., Blazevic, A., Roller, A., and Rompel, A. (2015). The synthesis and characterization of aromatic hybrid anderson–evans POMs and their serum albumin interactions: the shift from polar to hydrophobic interactions. Chem. Eur. J. 21 (49), 17800–17807. doi:10.1002/chem.201502458
Amthor, S., Knoll, S., Heiland, M., Zedler, L., Li, C., Nauroozi, D., et al. (2022). A photosensitizer–polyoxometalate dyad that enables the decoupling of light and dark reactions for delayed on-demand solar hydrogen production. Nat. Chem. 14 (3), 321–327. doi:10.1038/s41557-021-00850-8
Anyushin, A. V., Kondinski, A., and Parac-Vogt, T. N. (2020). Hybrid polyoxometalates as post-functionalization platforms: from fundamentals to emerging applications. Chem. Soc. Rev. 49 (2), 382–432. doi:10.1039/C8CS00854J
Bai, X., Song, N., Wen, L., Huang, X., Zhang, J., Zhang, Y., et al. (2020). Environmentally benign multiphase solid–liquid–gas catalysis. Green Chem. 22 (3), 895–902. doi:10.1039/C9GC04063C
Barkigia, K. M., Rajkovic-Blazer, L. M., Pope, M. T., and Quicksall, C. O. (1981). Dodecamolybdotetrakis(organoarsonates) and the structure of a neutral zwitterionic heteropoly complex. Inorg. Chem. 20 (10), 3318–3323. doi:10.1021/ic50224a035
Bar-Nahum, I., Cohen, H., and Neumann, R. (2003). Organometallic−Polyoxometalate hybrid compounds: metallosalen compounds modified by Keggin type polyoxometalates. Inorg. Chem. 42 (11), 3677–3684. doi:10.1021/ic034095s
Bar-Nahum, I., and Neumann, R. (2003). Synthesis, characterization and catalytic activity of a Wilkinson’s type metal-organic–polyoxometalate hybrid compound. Chem. Commun. (21), 2690–2691. doi:10.1039/B310236J
Bayaguud, A., Chen, K., and Wei, Y. (2016). Controllable synthesis of polyoxovanadate-based coordination polymer nanosheets with extended exposure of catalytic sites. Nano Res. 9 (12), 3858–3867. doi:10.1007/s12274-016-1255-y
Bentaleb, F., Makrygenni, O., Brouri, D., Coelho Diogo, C., Mehdi, A., Proust, A., et al. (2015). Efficiency of polyoxometalate-based mesoporous hybrids as covalently anchored catalysts. Inorg. Chem. 54 (15), 7607–7616. doi:10.1021/acs.inorgchem.5b01216
Berardi, S., Bonchio, M., Carraro, M., Conte, V., Sartorel, A., and Scorrano, G. (2007). Fast catalytic epoxidation with H2O2 and [γ-SiW10O36(PhPO)2]4- in ionic liquids under microwave irradiation. J. Org. Chem. 72 (23), 8954–8957. doi:10.1021/jo7016923
Berardi, S., Carraro, M., Iglesias, M., Sartorel, A., Scorrano, G., Albrecht, M., et al. (2010). Polyoxometalate-based N-heterocyclic carbene (NHC) complexes for palladium-mediated C-C coupling and chloroaryl dehalogenation catalysis. Chem. Eur. J. 16 (35), 10662–10666. doi:10.1002/chem.201001009
Bijelic, A., Aureliano, M., and Rompel, A. (2019). Polyoxometalates as potential next-generation metallodrugs in the combat against cancer. Angew. Chem. Int. Ed. 58 (10), 2980–2999. doi:10.1002/anie.201803868
Blazevic, A., Al-Sayed, E., Roller, A., Giester, G., and Rompel, A. (2015). Tris-functionalized hybrid Anderson polyoxometalates: synthesis, characterization, hydrolytic stability and inversion of protein surface charge. Chem. Eur. J. 21 (12), 4762–4771. doi:10.1002/chem.201405644
Bonchio, M., Carraro, M., Scorrano, G., and Bagno, A. (2004). Photooxidation in water by new hybrid molecular photocatalysts integrating an organic sensitizer with a polyoxometalate core. Adv. Synth. Catal. 346 (6), 648–654. doi:10.1002/adsc.200303189
Cameron, J. M., Fujimoto, S., Kastner, K., Wei, R.-J., Robinson, D., Sans, V., et al. (2017). Orbital engineering: photoactivation of an organofunctionalized polyoxotungstate. Chem. Eur. J. 23 (1), 47–50. doi:10.1002/chem.201605021
Carraro, M., Fiorani, G., Mognon, L., Caneva, F., Gardan, M., Maccato, C., et al. (2012). Hybrid polyoxotungstates as functional comonomers in new cross-linked catalytic polymers for sustainable oxidation with hydrogen peroxide. Chem. Eur. J. 18 (41), 13195–13202. doi:10.1002/chem.201201849
Carraro, M., Sandei, L., Sartorel, A., Scorrano, G., and Bonchio, M. (2006). Hybrid polyoxotungstates as second-generation POM-based catalysts for microwave-assisted H2O2 activation. Org. Lett. 8 (17), 3671–3674. doi:10.1021/ol061197o
Chen, Q., and Zubieta, J. (1990). Synthesis and structural characterization of a polyoxovanadate coordination complex with a hexametalate core: [(n-C4H9)4N]2[V6O13{O2NC(CH2O)3}2]. Inorg. Chem. 29 (8), 1456–1458. doi:10.1021/ic00333a002
Chen, Q., and Zubieta, J. (1992). Coordination chemistry of soluble metal oxides of molybdenum and vanadium. Coord. Chem. Rev. 114 (2), 107–167. doi:10.1016/0010-8545(92)85001-7
Clemente-Juan, J. M., Coronado, E., and Gaita-Ariño, A. (2012). Magnetic polyoxometalates: from molecular magnetism to molecular spintronics and quantum computing. Chem. Soc. Rev. 41 (22), 7464–7478. doi:10.1039/C2CS35205B
De Bruyn, M., and Neumann, R. (2007). Stabilization of palladium nanoparticles by polyoxometalates appended with alkylthiol tethers and their use as binary catalysts for liquid phase aerobic oxydehydrogenation. Adv. Synth. Catal. 349 (10), 1624–1628. doi:10.1002/adsc.200600613
Dolbecq, A., Dumas, E., Mayer, C. R., and Mialane, P. (2010). Hybrid Organic−Inorganic polyoxometalate compounds: from structural diversity to applications. Chem. Rev. 110 (10), 6009–6048. doi:10.1021/cr1000578
Du, Y., Rheingold, A. L., and Maatta, E. A. (1992). A polyoxometalate incorporating an organoimido ligand: preparation and structure of [Mo5O18(MoNC6H4CH3)]2-. J. Am. Chem. Soc. 114 (1), 345–346. doi:10.1021/ja00027a046
Dupré, N., Brazel, C., Fensterbank, L., Malacria, M., Thorimbert, S., Hasenknopf, B., et al. (2012). Self-buffering hybrid gold-polyoxometalate catalysts for the catalytic cyclization of acid-sensitive substrates. Chem. Eur. J. 18 (41), 12962–12965. doi:10.1002/chem.201201007
Fujimoto, S., Cameron, J. M., Wei, R.-J., Kastner, K., Robinson, D., Sans, V., et al. (2017). A simple approach to the visible-light photoactivation of molecular metal oxides. Inorg. Chem. 56 (20), 12169–12177. doi:10.1021/acs.inorgchem.7b01499
Gouzerh, P., and Proust, A. (1998). Main-group element, organic, and organometallic derivatives of polyoxometalates. Chem. Rev. 98 (1), 77–112. doi:10.1021/cr960393d
Hasenknopf, B., Delmont, R., Herson, P., and Gouzerh, P. (2002). Anderson-type heteropolymolybdates containing tris(alkoxo) ligands: synthesis and structural characterization. Eur. J. Inorg. Chem. 2002 (5), 1081–1087. doi:10.1002/1099-0682(200205)2002:5<1081::AID-EJIC1081>3.0.CO;2-W
Hou, Y., and Hill, C. L. (1993). Hydrolytically stable organic triester capped polyoxometalates with catalytic oxygenation activity of formula [RC(CH2O)3V3P2W15O59]6- (R = CH3, NO2, CH2OH). J. Am. Chem. Soc. 115 (25), 11823–11830. doi:10.1021/ja00078a022
Hsieh, T.-C., and Zubieta, J. A. (1986). Synthesis and characterization of oxomolybdate clusters containing coordinatively bound organo-diazenido units: the crystal and molecular structure of the hexanuclear diazenido-oxomolybdate, (NBun4)3[Mo6O18(N2C6H5)]. Polyhedron 5 (10), 1655–1657. doi:10.1016/S0277-5387(00)84576-3
Huo, Y., Huo, Z., Ma, P., Wang, J., and Niu, J. (2015). Polyoxotungstate incorporating organotriphosphonate ligands: synthesis, characterization, and catalytic for alkene epoxidation. Inorg. Chem. 54 (2), 406–408. doi:10.1021/ic502404m
Hutin, M., Rosnes, M. H., Long, D.-L., and Cronin, L. (2013). “Polyoxometalates: synthesis and structure – from building blocks to emergent materials,” in Comprehensive inorganic chemistry II. Editors J. Reedijk, and K. Poeppelmeier, 241–269.
Ivanova, S. (2014). Hybrid organic-inorganic materials based on polyoxometalates and ionic liquids and their application in catalysis. Int. Sch. Res. Not. 2014, 1–13. doi:10.1155/2014/963792
Ji, L.-P., Du, J., Li, J.-S., Zhang, L.-C., Sang, X.-J., Yang, H., et al. (2016). A new boat-like tungstoarsenate functionalized by carboxyethyltin and its catalytic properties. RSC Adv. 6 (34), 28956–28959. doi:10.1039/C5RA27781G
Karimian, D., Yadollahi, B., and Mirkhani, V. (2014). Harvesting visible light for aerobic oxidation of alcohols by a novel and efficient hybrid polyoxometalate. Dalton Trans. 44 (4), 1709–1715. doi:10.1039/C4DT03299C
Karimian, D., and Zangi, F. (2021). Aerobic photooxidation of sulfides using unique hybrid polyoxometalate under visible light. Catal. Commun. 152, 106283. doi:10.1016/j.catcom.2021.106283
Kibler, A. J., and Newton, G. N. (2018). Tuning the electronic structure of organic–inorganic hybrid polyoxometalates: the crucial role of the covalent linkage. Polyhedron 154, 1–20. doi:10.1016/j.poly.2018.06.027
Kim, G. S., Hagen, K. S., and Hill, C. L. (1992). Synthesis, structure, spectroscopic properties, and hydrolytic chemistry of organophosphonoyl polyoxotungstates of formula [C6H5P(O)]2Xn+W11O39(8-n)- (Xn+ = P5+, Si4+). Inorg. Chem. 31 (25), 5316–5324. doi:10.1021/ic00051a025
Knoth, W. H. (1979). Derivatives of heteropolyanions. 1. Organic derivatives of W12SiO404-, W12PO403-, and Mo12SiO404-. J. Am. Chem. Soc. 101 (3), 759–760. doi:10.1021/ja00497a057
Knoth, W. H., and Harlow, R. L. (1981). Derivatives of heteropolyanions. 3. O-alkylation of Mo12PO403- and W12PO403-. J. Am. Chem. Soc. 103 (14), 4265–4266. doi:10.1021/ja00404a056
Kong, H., Liu, S., Wang, Y., Xu, Q., Li, K., Ma, P., et al. (2021a). Assembly of a hexameric cluster of polyoxomolybdotriphosphonate builts from [Zn(H2O){TeMo6O21}{N(CH2PO3)3}]6– subunits and its optical and catalytic properties. Inorg. Chem. 60 (20), 15759–15767. doi:10.1021/acs.inorgchem.1c02417
Kong, H., Xu, B., Zhen, J., Liu, S., Li, K., Ma, P., et al. (2021b). Organophosphonate-functionalized telluromolybdate containing a [TeMo10O37]10– building block and its catalytic efficiency for Knoevenagel condensation. Inorg. Chem. 60 (19), 14872–14879. doi:10.1021/acs.inorgchem.1c02235
Kuramochi, S., Cameron, J. M., Fukui, T., Jones, K. D., Argent, S. P., Kusaka, S., et al. (2023). Selective electrochemical CO2 conversion with a hybrid polyoxometalate. Chem. Commun. 59 (72), 10801–10804. doi:10.1039/D3CC02138F
Kwak, W., Pope, M. T., and Scully, T. F. (1975). Stable organic derivatives of heteropoly anions, pentamolybdobisphosphonates. J. Am. Chem. Soc. 97 (20), 5735–5738. doi:10.1021/ja00853a017
Kwak, W., Rajkovic, L. M., Stalick, J. K., Pope, M. T., and Quicksall, C. O. (1976). Synthesis and structure of hexamolybdobis(organoarsonates). Inorg. Chem. 15 (11), 2778–2783. doi:10.1021/ic50165a042
Lachkar, D., Vilona, D., Dumont, E., Lelli, M., and Lacôte, E. (2016). Grafting of secondary diolamides onto [P2W15V3O62]9− generates hybrid heteropoly acids. Angew. Chem. 128 (20), 6065–6069. doi:10.1002/ange.201510954
Li, J., Huth, I., Chamoreau, L.-M., Hasenknopf, B., Lacôte, E., Thorimbert, S., et al. (2009). Insertion of amides into a polyoxometalate. Angew. Chem. Int. Ed. 48 (11), 2035–2038. doi:10.1002/anie.200805964
Li, N., Liu, J., Dong, B.-X., and Lan, Y.-Q. (2020). Polyoxometalate-based compounds for photo- and electrocatalytic applications. Angew. Chem. Int. Ed. 59 (47), 20779–20793. doi:10.1002/anie.202008054
Li, Y., Liu, M., and Chen, L. (2017). Polyoxometalate built-in conjugated microporous polymers for visible-light heterogeneous photocatalysis. J. Mater Chem. A 5 (26), 13757–13762. doi:10.1039/C7TA03776G
Lian, L., Chen, X., Yi, X., Liu, Y., Chen, W., Zheng, A., et al. (2020). Modulation of self-separating molecular catalysts for highly efficient biomass transformations. Chem. Eur. J. 26 (51), 11900–11908. doi:10.1002/chem.202001451
Lian, L., Liu, Y., Yi, X., Hu, H., Chen, X., Li, H., et al. (2022). Covalently tethering disulfonic acid moieties onto polyoxometalate boosts acid strength and catalytic performance for hydroxyalkylation/alkylation reaction. Sci. China Chem. 65 (4), 699–709. doi:10.1007/s11426-021-1181-7
Liu, S., Shaikh, S. N., and Zubieta, J. (1987). Coordination complexes of polyoxomolybdate anions. Characterization of a tetranuclear core from reactions in methanol: syntheses and structures of two polyoxomolybdate alcoholates, (MePPh3)2[Mo4O10(OCH3)6] and (n-Bu4N)2[Mo4O10(OCH3)2(OC6H4O)2], and their relationship to a general class of tetranuclear cluster types [Mo4Ox(OMe)2(L)y(LL)z]2-. Inorg. Chem. 26 (26), 4303–4305. doi:10.1021/ic00273a003
Liu, Y., Shrestha, S., and Mustain, W. E. (2012). Synthesis of nanosize tungsten oxide and its evaluation as an electrocatalyst support for oxygen reduction in acid media. ACS Catal. 2 (3), 456–463. doi:10.1021/cs200657w
Long, D.-L., Burkholder, E., and Cronin, L. (2006). Polyoxometalate clusters, nanostructures and materials: from self assembly to designer materials and devices. Chem. Soc. Rev. 36 (1), 105–121. doi:10.1039/B502666K
Long, D.-L., Tsunashima, R., and Cronin, L. (2010). Polyoxometalates: building blocks for functional nanoscale systems. Angew. Chem. Int. Ed. 49 (10), 1736–1758. doi:10.1002/anie.200902483
Luo, J., Huang, Y., Ding, B., Wang, P., Geng, X., Zhang, J., et al. (2018). Single-atom Mn active site in a triol-stabilized β-anderson manganohexamolybdate for enhanced catalytic activity towards adipic acid production. Catalysts 8 (3), 121. doi:10.3390/catal8030121
Luo, Y., Maloul, S., Endres, P., Schönweiz, S., Ritchie, C., Wächtler, M., et al. (2020b). Organic linkage controls the photophysical properties of covalent photosensitizer–polyoxometalate hydrogen evolution dyads. Sustain. Energy Fuels 4 (9), 4688–4693. doi:10.1039/D0SE00582G
Luo, Y., Maloul, S., Schönweiz, S., Wächtler, M., Streb, C., and Dietzek, B. (2020a). Yield—not only lifetime—of the photoinduced charge-separated state in iridium complex–polyoxometalate dyads impact their hydrogen evolution reactivity. Chem. Eur. J. 26 (36), 8045–8052. doi:10.1002/chem.202000982
Makrygenni, O., Brouri, D., Proust, A., Launay, F., and Villanneau, R. (2019). Immobilization of polyoxometalate hybrid catalysts onto mesoporous silica supports using phenylene diisothiocyanate as a cross-linking agent. Micropor Mesopor Mater. 278, 314–321. doi:10.1016/j.micromeso.2018.11.036
Makrygenni, O., Vanmairis, L., Taourit, S., Launay, F., Shum Cheong Sing, A., Proust, A., et al. (2020). Selective Formation of epoxylimonene catalyzed by phosphonyl/arsonyl derivatives of trivacant polyoxotungstates at low temperature. Eur. J. Inorg. Chem. 2020 (7), 605–612. doi:10.1002/ejic.201901152
Maloul, S., van den Borg, M., Müller, C., Zedler, L., Mengele, A. K., Gaissmaier, D., et al. (2021). Multifunctional polyoxometalate platforms for supramolecular light-driven hydrogen evolution. Chem. Eur. J. 27 (68), 16846–16852. doi:10.1002/chem.202103817
Miao, W.-K., Yan, Y.-K., Wang, X.-L., Xiao, Y., Ren, L.-J., Zheng, P., et al. (2014). Incorporation of polyoxometalates into polymers to create linear poly(polyoxometalate)s with catalytic function. ACS Macro Lett. 3 (2), 211–215. doi:10.1021/mz5000202
Mirkhani, V., Moghadam, M., Tangestaninejad, S., Mohammadpoor-Baltork, I., and Rasouli, N. (2007). Catalytic oxidation of olefins with hydrogen peroxide catalyzed by [Fe(III)(salen)Cl] complex covalently linked to polyoxometalate. Inorg. Chem. Commun. 10 (12), 1537–1540. doi:10.1016/j.inoche.2007.09.016
Mirkhani, V., Moghadam, M., Tangestaninejad, S., Mohammadpoor-Baltork, I., and Rasouli, N. (2008a). A comparative study of oxidation of alkanes and alkenes by hydrogen peroxide catalyzed by Cu(salen) complex covalently bound to a Keggin type polyoxometalate and its neat counterpart. Catal. Commun. 9 (14), 2411–2416. doi:10.1016/j.catcom.2008.05.040
Mirkhani, V., Moghadam, M., Tangestaninejad, S., Mohammadpoor-Baltork, I., Shams, E., and Rasouli, N. (2008b). Catalytic epoxidation of olefins with hydrogen peroxide by hybrid complex containing nickel(III) Schiff base complex covalently linked to polyoxometalate. Appl. Catal. A 334 (1), 106–111. doi:10.1016/j.apcata.2007.09.041
Mirzaei, M., Eshtiagh-Hosseini, H., Alipour, M., and Frontera, A. (2014). Recent developments in the crystal engineering of diverse coordination modes (0–12) for Keggin-type polyoxometalates in hybrid inorganic–organic architectures. Coord. Chem. Rev. 275, 1–18. doi:10.1016/j.ccr.2014.03.012
Moghadam, M., Mirkhani, V., Tangestaninejad, S., Mohammadpoor-Baltork, I., and Javadi, M. M. (2010a). Molybdenum Schiff base-polyoxometalate hybrid compound: a heterogeneous catalyst for alkene epoxidation with tert-BuOOH. Polyhedron 29 (1), 648–654. doi:10.1016/j.poly.2009.09.016
Moghadam, M., Mirkhani, V., Tangestaninejad, S., Mohammadpoor-Baltork, I., and Javadi, M. M. (2010b). Polyoxometalate–molybdenylacetylacetonate hybrid complex: a reusable and efficient catalyst for oxidation of alkenes with tert-butylhydroperoxide. Inorg. Chem. Commun. 13 (2), 244–249. doi:10.1016/j.inoche.2009.11.022
Najafi, M. (2023). Polyoxometalate-based inorganic-organic hybrids as heterogeneous catalysts for asymmetric and tandem reactions. ChemCatChem. 15 (1), e202201045. doi:10.1002/cctc.202201045
Nisar, A., and Wang, X. (2012). Surfactant-encapsulated polyoxometalate building blocks: controlled assembly and their catalytic properties. Dalton Trans. 41 (33), 9832–9845. doi:10.1039/C2DT30470H
Nlate, S., and Jahier, C. (2013). Dendritic polyoxometalate hybrids: efficient and recoverable catalysts for oxidation reactions. Eur. J. Inorg. Chem. 2013 (10–11), 1606–1619. doi:10.1002/ejic.201201129
Nowicka, D., Marcinkowski, D., Vadra, N., Szymańska, M., Kubicki, M., Consiglio, G., et al. (2024). The effect of ionic versus covalent functionalization of polyoxometalate hybrid materials with coordinating subunits on their stability and interaction with DNA. Dalton Trans. 53, 11678–11688. Advance Article. doi:10.1039/D4DT00965G
Oble, J., Riflade, B., Noël, A., Malacria, M., Thorimbert, S., Hasenknopf, B., et al. (2011). Carbonyl-inserted organo-hybrids of a dawson-type phosphovanadotungstate: scope and chemoselective oxidation catalysis. Org. Lett. 13 (22), 5990–5993. doi:10.1021/ol202430e
Piedra-Garza, L. F., Dickman, M. H., Moldovan, O., Breunig, H. J., and Kortz, U. (2009). Organoantimony-containing polyoxometalate: [{PhSbOH}3(A-α-PW9O34)2]9−. Inorg. Chem. 48 (2), 411–413. doi:10.1021/ic8021694
Pope, M. T., and Müller, A. (1991). Chemie der Polyoxometallate: Aktuelle Variationen über ein altes Thema mit interdisziplinären Bezügen. Angew. Chem. 103 (1), 56–70. doi:10.1002/ange.19911030107
Proust, A., Matt, B., Villanneau, R., Guillemot, G., Gouzerh, P., and Izzet, G. (2012). Functionalization and post-functionalization: a step towards polyoxometalate-based materials. Chem. Soc. Rev. 41 (22), 7605–7622. doi:10.1039/C2CS35119F
Proust, A., Thouvenot, R., and Gouzerh, P. (2008). Functionalization of polyoxometalates: towards advanced applications in catalysis and materials science. Chem. Commun. (16), 1837–1852. doi:10.1039/B715502F
Ren, Y., Wang, M., Chen, X., Yue, B., and He, H. (2015). Heterogeneous catalysis of polyoxometalate based organic–inorganic hybrids. Materials 8 (4), 1545–1567. doi:10.3390/ma8041545
Riflade, B., Lachkar, D., Oble, J., Li, J., Thorimbert, S., Hasenknopf, B., et al. (2014). Pd-containing organopolyoxometalates derived from Dawson polyoxometalate [P2W15V3O62]9–: lewis acidity and dual site catalysis. Org. Lett. 16 (15), 3860–3863. doi:10.1021/ol501644c
Riflade, B., Oble, J., Chenneberg, L., Derat, E., Hasenknopf, B., Lacôte, E., et al. (2013). Hybrid polyoxometalate palladacycles: DFT study and application to the Heck reaction. Tetrahedron 69 (27), 5772–5779. doi:10.1016/j.tet.2013.03.045
Salazar Marcano, D. E., Lentink, S., Moussawi, M. A., and Parac-Vogt, T. N. (2021). Solution dynamics of hybrid anderson–evans polyoxometalates. Inorg. Chem. 60 (14), 10215–10226. doi:10.1021/acs.inorgchem.1c00511
Santoni, M.-P., Hanan, G. S., and Hasenknopf, B. (2014). Covalent multi-component systems of polyoxometalates and metal complexes: toward multi-functional organic–inorganic hybrids in molecular and material sciences. Coord. Chem. Rev. 281, 64–85. doi:10.1016/j.ccr.2014.09.003
Sazani, G., Dickman, M. H., and Pope, M. T. (2000). Organotin derivatives of α-[XIIIW9O33]9- (X = as, Sb) heteropolytungstates. Solution- and solid-state characterization of [{(C6H5Sn)2O}2H(α-AsW9O33)2]9- and [(C6H5Sn)3Na3(α-SbW9O33)2]6-. Inorg. Chem. 39 (5), 939–943. doi:10.1021/ic991095c
Sazani, G., and Pope, M. T. (2004). Organotin and organogermanium linkers for simple, direct functionalization of polyoxotungstatesElectronic supplementary information (ESI) available: syntheses. Dalton Trans. (13), 1989–1994. doi:10.1039/B402421D
Schaming, D., Allain, C., Farha, R., Goldmann, M., Lobstein, S., Giraudeau, A., et al. (2010). Synthesis and photocatalytic properties of mixed Polyoxometalate−Porphyrin copolymers obtained from anderson-type polyoxomolybdates. Langmuir 26 (7), 5101–5109. doi:10.1021/la903564d
Schönweiz, S., Heiland, M., Anjass, M., Jacob, T., Rau, S., and Streb, C. (2017). Experimental and theoretical investigation of the light-driven hydrogen evolution by polyoxometalate–photosensitizer dyads. Chem. Eur. J. 23 (61), 15370–15376. doi:10.1002/chem.201702116
She, S., Li, M., Li, Q., Huang, Z., Wei, Y., and Yin, P. (2019). Unprecedented halide-ion binding and catalytic activity of nanoscale anionic metal oxide clusters. ChemPlusChem 84 (11), 1668–1672. doi:10.1002/cplu.201900307
Solé-Daura, A., Zhang, T., Fouilloux, H., Robert, C., Thomas, C. M., Chamoreau, L.-M., et al. (2020). Catalyst design for alkene epoxidation by molecular analogues of heterogeneous titanium-silicalite catalysts. ACS Catal. 10 (8), 4737–4750. doi:10.1021/acscatal.9b05147
Song, Y.-F., and Tsunashima, R. (2012). Recent advances on polyoxometalate-based molecular and composite materials. Chem. Soc. Rev. 41 (22), 7384–7402. doi:10.1039/C2CS35143A
Tong, J., Wang, H., Cai, X., Zhang, Q., Ma, H., and Lei, Z. (2014). Suzuki coupling reaction catalyzed heterogeneously by Pd(salen)/polyoxometalate compound: another example for synergistic effect of organic/inorganic hybrid. Appl. Organomet. Chem. 28 (2), 95–100. doi:10.1002/aoc.3086
Vilona, D., Lelli, M., Dumont, E., and Lacôte, E. (2021). Organo-Polyoxometalate-based hydrogen-bond catalysis. Chem. Eur. J. 27 (71), 17761–17764. doi:10.1002/chem.202102807
Waele, V. D., Poizat, O., Fagnoni, M., Bagno, A., and Ravelli, D. (2016). Unraveling the key features of the reactive state of decatungstate anion in hydrogen atom transfer (HAT) photocatalysis. ACS Catal. 6 (10), 7174–7182. doi:10.1021/acscatal.6b01984
Wang, J., Jiang, F., Tao, C., Yu, H., Ruhlmann, L., and Wei, Y. (2021). Oxidative esterification of alcohols by a single-side organically decorated Anderson-type chrome-based catalyst. Green Chem. 23 (7), 2652–2657. doi:10.1039/D1GC00161B
Wang, S.-S., and Yang, G.-Y. (2015). Recent advances in polyoxometalate-catalyzed reactions. Chem. Rev. 115 (11), 4893–4962. doi:10.1021/cr500390v
Wang, W., Chamoreau, L.-M., Izzet, G., Proust, A., Orio, M., and Blanchard, S. (2023). Multi-electron visible light photoaccumulation on a dipyridylamine copper(II)–Polyoxometalate conjugate applied to photocatalytic generation of CF3 radicals. J. Am. Chem. Soc. 145 (22), 12136–12147. doi:10.1021/jacs.3c01716
Weinstock, I. A., Schreiber, R. E., and Neumann, R. (2018). Dioxygen in polyoxometalate mediated reactions. Chem. Rev. 118 (5), 2680–2717. doi:10.1021/acs.chemrev.7b00444
Xiao, Y., Chen, D., Ma, N., Hou, Z., Hu, M., Wang, C., et al. (2013). Covalent immobilization of a polyoxometalate in a porous polymer matrix: a heterogeneous catalyst towards sustainability. RSC Adv. 3 (44), 21544–21551. doi:10.1039/C3RA43373K
Xu, Q., Li, Y., Ban, R., Li, Z., Han, X., Ma, P., et al. (2018). Polyoxotungstates incorporated organophosphonate and nickel: synthesis, characterization and efficient catalysis for epoxidation of allylic alcohols. Dalton Trans. 47 (38), 13479–13486. doi:10.1039/C8DT02590H
Xu, Q., Liang, X., Xu, B., Wang, J., He, P., Ma, P., et al. (2020a). 36-Nuclearity organophosphonate-functionalized polyoxomolybdates: synthesis, characterization and selective catalytic oxidation of sulfides. Chem. Eur. J. 26 (65), 14896–14902. doi:10.1002/chem.202001468
Xu, Q., Xu, B., Kong, H., He, P., Wang, J., Kannan, T., et al. (2020b). Synthesis and characterization of a crown-shaped 36-molybdate cluster and application in catalyzing Knoevenagel condensation. Inorg. Chem. 59 (15), 10665–10672. doi:10.1021/acs.inorgchem.0c01122
Yang, H., Zhang, L.-C., Yang, L., Zhang, X.-L., You, W.-S., and Zhu, Z.-M. (2013). Synthesis, characterization and catalytic activity of a new sandwich-type tungstophosphate functionalized by carboxyethyltin. Inor Chem. Commun. 29, 33–36. doi:10.1016/j.inoche.2012.12.011
Yin, P., Wang, J., Xiao, Z., Wu, P., Wei, Y., and Liu, T. (2012). Polyoxometalate–organic hybrid molecules as amphiphilic emulsion catalysts for deep desulfurization. Chem. Eur. J. 18 (30), 9174–9178. doi:10.1002/chem.201201551
Yu, H., Ru, S., Dai, G., Zhai, Y., Lin, H., Han, S., et al. (2017). An efficient iron(III)-Catalyzed aerobic oxidation of aldehydes in water for the green preparation of carboxylic acids. Angew. Chem. Int. Ed. 56 (14), 3867–3871. doi:10.1002/anie.201612225
Zhang, J., Xiao, F., Hao, J., and Wei, Y. (2012). The chemistry of organoimido derivatives of polyoxometalates. Dalton Trans. 41 (13), 3599–3615. doi:10.1039/C2DT11948J
Zhang, L.-C., Zheng, S.-L., Xue, H., Zhu, Z.-M., You, W.-S., Li, Y.-G., et al. (2010). New tetra(organotin)-decorated boat-like polyoxometalate. Dalton Trans. 39 (14), 3369–3371. doi:10.1039/B925822A
Zhang, T., Mazaud, L., Chamoreau, L.-M., Paris, C., Proust, A., and Guillemot, G. (2018a). Unveiling the active surface sites in heterogeneous titanium-based silicalite epoxidation catalysts: input of silanol-functionalized polyoxotungstates as soluble analogues. ACS Catal. 8 (3), 2330–2342. doi:10.1021/acscatal.8b00256
Zhang, T., Solé-Daura, A., Hostachy, S., Blanchard, S., Paris, C., Li, Y., et al. (2018b). Modeling the oxygen vacancy at a molecular vanadium(III) silica-supported catalyst. J. Am. Chem. Soc. 140 (44), 14903–14914. doi:10.1021/jacs.8b09048
Zhao, C., and Bond, A. M. (2009). Photoinduced oxidation of water to oxygen in the ionic liquid BMIMBF4 as the counter reaction in the fabrication of exceptionally Long semiconducting silver-tetracyanoquinodimethane nanowires. J. Am. Chem. Soc. 131 (12), 4279–4287. doi:10.1021/ja806893t
Zhao, S., Zhao, X., Ouyang, S., and Zhu, Y. (2018). Polyoxometalates covalently combined with graphitic carbon nitride for photocatalytic hydrogen peroxide production. Catal. Sci. Techno 8 (6), 1686–1695. doi:10.1039/C8CY00043C
Keywords: polyoxometalates, hybrid POMs, catalysis, organofunctionalization, class II poms
Citation: Iftikhar T and Rosnes MH (2024) Covalent organic-inorganic polyoxometalate hybrids in catalysis. Front. Chem. 12:1447623. doi: 10.3389/fchem.2024.1447623
Received: 11 June 2024; Accepted: 13 August 2024;
Published: 29 August 2024.
Edited by:
Soyeb Pathan, Parul University, IndiaReviewed by:
Laia Vilà Nadal, University of Glasgow, United KingdomMasahiro Sadakane, Hiroshima University, Japan
Graham Newton, University of Nottingham, United Kingdom
Copyright © 2024 Iftikhar and Rosnes. This is an open-access article distributed under the terms of the Creative Commons Attribution License (CC BY). The use, distribution or reproduction in other forums is permitted, provided the original author(s) and the copyright owner(s) are credited and that the original publication in this journal is cited, in accordance with accepted academic practice. No use, distribution or reproduction is permitted which does not comply with these terms.
*Correspondence: Mali H. Rosnes, TWFsaS5Sb3NuZXNAdWliLm5v