- 1Associate Laboratory i4HB, Institute for Health and Bioeconomy, NOVA Faculty of Sciences and Technology, NOVA University of Lisbon, Lisbon, Portugal
- 2UCIBIO, Applied Molecular Biosciences Unit, Chemistry and Life Sciences Departments, NOVA Faculty of Sciences and Technology, NOVA University of Lisbon, Lisbon, Portugal
- 3Unidad de Química en Sisal, Facultad de Química, Universidad Nacional Autónoma de México, Yucatán, Mexico
- 4CIIMAR/CIMAR—Interdisciplinary Centre of Marine and Environmental Research, University of Porto, Terminal de Cruzeiros do Porto de Leixões, Matosinhos, Portugal
- 5Department of Chemical Engineering, Cyprus University of Technology, Limassol, Cyprus
- 6Biology Department, Faculty of Sciences, Porto University, Porto, Portugal
Introduction: Biofouling poses a significant economic threat to various marine industries, leading to financial losses that can reach billions of euros annually. This study highlights the urgent need for effective alternatives to traditional antifouling agents, particularly following the global ban on organotin compounds.
Material and methods: Streptomyces aculeolatus PTM-346 was isolated from sediment samples on the shores of the Madeira Archipelago, Portugal. The crude extract was fractionated using silica flash chromatography and preparative HPLC, resulting in two isolated marinone compounds: madeirone (1), a novel marinone derivative discovered in this study, and neomarinone (2). The antifouling activities of these compounds were tested against five marine bacterial species and the larvae of the mussel Mytilus galloprovincialis. Additionally, in silico and in vivo environmental toxicity evaluations of madeirone (1) and neomarinone (2) were conducted.
Results: Madeirone (1) demonstrated significant antibiofilm efficacy, inhibiting Phaeobacter inhibens by up to 66%, Marinobacter hydrocarbonoclasticus by up to 60%, and Cobetia marina by up to 40%. Neomarinone (2) also exhibited substantial antibiofilm activity, with inhibition rates of up to 41% against P. inhibens, 40% against Pseudo-oceanicola batsensis, 56% against M. hydrocarbonoclasticus, 46% against C. marina, and 40% against Micrococcus luteus. The growth inhibition activity at the same concentrations of these compounds remained below 20% for the respective bacteria, highlighting their effectiveness as potent antibiofilm agents without significantly affecting bacterial viability. Additionally, both compounds showed potent effects against the settlement of Mytilus galloprovincialis larvae, with EC50 values of 1.76 µg/mL and 0.12 µg/mL for compounds (1) and (2), respectively, without impairing the viability of the targeted macrofouling species. In silico toxicity predictions and in vivo toxicity assays both support their potential for further development as antifouling agents.
Conclusion: The newly discovered metabolite madeirone (1) and neomarinone (2) effectively inhibit both micro- and macrofouling. This distinct capability sets them apart from existing commercial antifouling agents and positions them as promising candidates for biofouling prevention. Consequently, these compounds represent a viable and environmentally friendly alternative for incorporation into paints, primers, varnishes, and sealants, offering significant advantages over traditional copper-based compounds.
1 Background
Biofouling is a common biological phenomenon involving the adhesion of micro and macroorganisms, such as barnacles and mussels, to aquatic submerged surfaces (Magin et al., 2010). This occurrence poses a serious threat to maritime industries, resulting in significant economic losses and adverse impacts on marine environments and the economy (Xu et al., 2010). It causes substantial financial burdens, amounting to billions of euros annually, affecting aquaculture, shipping, and other industries dependent on coastal and offshore infrastructures. As ocean warming increases, the task of managing marine biofilms and biofouling is becoming more challenging (Schultz et al., 2011; Conrad and Poling-Skutvik, 2018; Sushmitha et al., 2023). Tributyltin was utilized to prevent biofouling, yet it resulted in serious environmental issues due to its toxicity, being banned by the International Maritime Organization in 1990 (Sonak et al., 2009). The global prohibition of organotin compounds as antifouling agents has amplified the urgency for safe and effective alternatives. Therefore, the identification of environmentally friendly strategies is imperative. Currently, there is a lack of sustainable, cost-effective, and environmentally benign solutions to adequately address this challenge. The quest for an environmentally safe antifouling agent is particularly noteworthy due to the persistent impact of biofoulers on marine habitats and the detrimental effects of biocides on the environment. Recent research efforts have concentrated on isolating natural, eco-friendly antifouling agents to counteract the toxicities associated with synthetic counterparts (Callow and Callow, 2011).
The marine ecosystem has proven to be a fundamental reservoir of economically and biotechnologically significant secondary metabolites. When exploring novel bioresources for economically important products, the marine environment garners special attention owing to its extraordinary diversity and extreme conditions, acknowledged for generating metabolites of immense value. It stands as an untapped resource for uncovering innovative secondary metabolites with diverse potential, as well as a wide array of bioactive compounds suitable for various biotechnological applications (Kirschner and Brennan, 2012; Rotter et al., 2021).
Marine-derived actinomycetes have emerged as a valuable source for such secondary metabolites that have demonstrated their importance for industries, supported by research on their properties and versatile applications (Bérdy, 2005). Notably, these actinomycetes produce a diverse collection of active metabolites, some of which exhibit remarkable antifouling properties. To date, there are very few studies on actinomycetes reporting antibiofilm activity against both Gram-positive and -negative bacteria, especially coupled with effects inhibiting larval settlement and the acetylcholinesterase enzyme, which signifies robust anti-macrofouling activity (Gaudêncio and Pereira, 2022; Morgan et al., 2023).
A comprehensive bibliographic search was conducted on antibiofilm and antifouling natural products from actinomycetes (Xu et al., 2007; Xu et al., 2010; Selvin, 2009; Cho, 2012; Cho and Kim, 2012; Cho et al., 2012; Prakash et al., 2015; Gopikrishnan et al., 2016; Waturangi et al., 2017; Kavitha and Vimala, 2020; Pereira et al., 2020; She et al., 2022; Stalin et al., 2022). Surprisingly, the reported studies referred to Streptomyces, with only one exception. Among actinomycetes, the Streptomyces genus has proven particularly prolific in producing antifouling agents. The chemical groups of these compounds encompass terpenoids, polyketides, furanones, butenolides, glycoglycerolipids, and alkaloids (Xu et al., 2010; Cho, 2012; Morgan et al., 2023).
For instance, Streptomyces praecox 291-11, isolated from Undaria pinnatifida rhizosphere, produced diketopiperazines with antifouling activity against Ulva pertusa and Navicula annexa (Cho et al., 2012). Streptomyces coelescens PK206-15, associated with seaweed, produced glycoglycerolipids inhibiting various fouling organisms (Cho, 2012). Streptomyces cinnabarinus PK209, co-cultured with Alteromonas sp. KNS-16, produced lobocompactol with significant antifouling activity (Cho and Kim, 2012). Streptomyces chrestomyceticus BCC 24770I synthesized albofungins, exhibiting antibiofilm and anti-macrofouling activities (She et al., 2022). Moreover, Streptomyces thermolineatus VITKV6A produced oxycyclopentadien with antimicrofouling activity against biofilm-forming bacteria (Stalin et al., 2022). Streptomyces dendra sp. nov. MSI051, isolated from Dendrilla nigra, demonstrated antagonistic potential against biofilm bacteria (Selvin, 2009). A deep-sea Streptomyces strain inhibited Balanus amphitrite larval settlement (Xu et al., 2007). Additionally, Streptomyces fradiae PE7 from Vellar estuarine sediment reported antifouling activity with quercetin (Gopikrishnan et al., 2016). Streptomyces fradiae RMS-MSU, isolated from Manakkudy mangroves, displayed antagonistic activity against marine biofilm bacterial strains (Prakash et al., 2015).
Additionally, antibiofilm and antifouling activity of napyradiomycins isolated from Streptomyces aculeolatus (S. aculeolatus), was reported by Gaudêncio and co-workers (Pereira et al., 2020).
The discovery of molecules exhibiting antibiofilm properties without antimicrobial effects presents an important alternative for treating infections linked to microorganisms that form biofilms. These compounds impede biofilm formation, thereby exposing bacteria to the surrounding environment, all without applying the typical selective pressure that often triggers the emergence of resistance mechanisms (Ahmad et al., 2014; Bauermeister et al., 2019; Sabotič et al., 2024).
Based on the aforementioned considerations, our research focused on investigating the marine-derived S. aculeolatus PTM-346 to evaluate the produced secondary metabolites as inhibitors of both marine micro and macrofouling.
2 Methods
2.1 Marine-derived actinomycetes isolation from ocean sediments
In June 2012, sediment samples were gathered off the shores of the Madeira Archipelago, Portugal (Prieto-Davó et al., 2016). Strain PTM-346 was isolated from samples retrieved at a depth of 14 m through SCUBA diving in Madeira Island waters. The sediment processing involved a heat-shock method: approximately 0.5 g of wet sediments were mixed with 2 mL of sterile seawater (SSW), settled briefly, and then subjected to a 6 min heat treatment at 55°C. Subsequently, 50 µL of the upper layer was spread on an agar plate containing seawater-based medium SW (1.8% p/v agar), supplemented with the antifungal cycloheximide (100 μg/L). The plates were incubated at room temperature (approximately 25°C) and regularly monitored for actinomycete growth over a period of 6 months. PTM-346 was sequentially transferred to fresh seawater-based A1 medium (10 g starch, 4 g yeast extract, 2 g peptone per L) until obtaining a pure strain. Strain PTM-346 was cultivated in A1 liquid culture medium (without agar) and preserved by cryopreservation in 10% (v/v) glycerol at −80°C.
2.2 PTM-346 actinomycete strain phylogenetic characterization
The actinomycete PTM-346 used in this study is phylogenetically related to the species S. aculeolatus, previously isolated by our group from oceanic sediments from the Madeira Archipelago (Prieto-Davó et al., 2016). The phylogenetic analysis involved incubating the culture in 20 mL of A1 medium with agitation (200 rpm) at 25°C for a duration of 7 days. Genomic DNA extraction was carried out using the Wizard® Genomic DNA Purification Kit (Promega, Madison, WI, United States) according to the protocol adapted for Gram-positive bacteria. To ensure sufficient genomic DNA extraction, extended incubation periods with lysozyme and RNase solution were employed, aligning with the manufacturer’s recommendations. The 16S rRNA gene was then amplified using the universal primers 27F (5′-AGAGTTTGATCCTGGCTCAG-3′) and 1492R (5′-TACGGCTACCTTGTTACGACTT-3′) (Gontang et al., 2007; Prieto-Davó et al., 2016; Pinto-Almeida et al., 2022). Subsequently, the amplified products were purified using the SureClean PCR cleanup kit (BioLine, London, UK) following the provided protocol. The purified PCR products underwent cycle sequencing at STABVIDA, Lda (www.stabvida.net), utilizing the ABI BigDye® Terminator v3.1 Cycle Sequencing Kit (Needham, MA, United States). The resulting sequences were analyzed on an ABI PRISM® 3730xl Genetic Analyzer (Needham, MA, United States), and the sequence traces were edited using Sequencing Analysis 5.3.1 from Applied Biosystems™ (Needham, MA, United States). For sequence comparison with the GenBank database, the BLASTn algorithm was employed (Altschul et al., 1990). The PTM-346 sequence has been deposited in GenBank under the accession number KP869060.1 and is accessible at www.ncbi.nlm.nih.gov/genbank.
2.3 Culture conditions for actinomycete PTM-346 crude extract production
The actinomycete strain PTM-346 was cultured in 40 Erlenmeyer flasks of 2 L volume, each with 1 L of seawater-based A1 medium. The cultures were agitated at 200 rpm and incubated at a temperature of 30°C. After 15 days of incubation, the culture underwent three extractions using half the volume of ethyl acetate (EtOAc) each time. The resulting mixture was then evaporated under vacuum, resulting in the production of approximately 6.5 g of crude extract.
2.4 Actinomycete PTM-346 secondary metabolites isolation and structure elucidation
PTM-346 crude extract, approximately 6.5 g in quantity, underwent fractionation using silica flash chromatography. This process involved step gradients of isooctane/EtOAc, followed by EtOAc/MeOH. The secondary metabolites (1) and (2) from PTM-346 were successfully obtained in the 4:6 and 0:1 fraction of isooctane/EtOAc, respectively. Further isolation procedures were carried out through reversed-phase HPLC, utilizing a Phenomenex Luna column (250 mm × 4.6 mm, 5 μm, 100 Å) at a flow rate of 1.5 mL/min, with DAD (Diode Array Detector) 190–500 nm. A gradient solvent system, ranging from 10% to 100% H2O:ACN (0.1% trifluoroacetic acid) over a 40 min period, followed by 100% acetonitrile during additional 40 min enabled the isolation of compound (1) (3.2 mg, yellow powder). Wilts, a gradient solvent system, ranging from 10% to 100% H2O:ACN in (0.1% trifluoroacetic acid) over a 20 min period, followed by 100% acetonitrile during additional 31 min allowed the isolation of compound (2) (3.9 mg, yellow powder). Infrared (IR) spectra were obtained using a Perkin Elmer Spectrum Two FT-IR Spectrometer. High-resolution ESI-TOF mass spectra were acquired through services provided by the mass spectrometry facility at the Department of Chemistry and Biochemistry, University of California, San Diego, La Jolla, CA, using an Agilent 6,230 Accurate-Mass TOFMS spectrometer in negative mode. Low-resolution LC/MS data were measured at NOVA-FCT analysis Lab, Portugal, utilizing an Agilent 1,200 Series LC with Binary pump HPLC System coupled with a Mass Spectrometry Agilent 6130B Single Quadropole (API-ES source, 3000 V), in positive mode. The analysis employed a reversed-phase C18 column (Phenomenex Luna, 100 mm × 1.0 mm, 5 µm), utilizing an H2O:ACN 10%–100% gradient with 0.1% formic acid at a flow rate of 0.7 mL/min, during 30 min. Elemental analysis (EA) was performed using a analyzer Thermo Finnigan-CE Instruments Flash EA 1112 CHNS series, in duplicate at NOVA FCT, Chemistry Department Analytical Lab. 1H-, 13C- and 2D- NMR spectral data measurements were conducted at 400 or 100 MHz using Bruker Advance and Bruker BioSpin spectrometers, (Ettlingen, Germany. Tetramethylsilane (TMS) was used as an internal standard. CDCl3 and DMSO-d6 were used as solvents for compounds (1) and (2), respectively.
Compound (1): yellow solid (3.2 mg); Rt = 50.1 min; UV λmax (nm): 210, 250, 290, and 335; IR NaCl νmax (cm−1): 3273.34, 2962.53, 2926.36, 1618.95, 1574.85, 1440.33, 1242.54, 1048.52, 1085.93; EA: C, 73.61: H, 7.60: O, 18.86, empirical formula C25H28O6; MS m/z: 425.2 Da [M + H]+, 849.3 Da [2M + H]+, (424.22 calcd. for C26H32O5); 13C-NMR (100 MHz, CDCl3) δC, 183.8, 180.1, 161.0, 159.3, 156.8, 139.1, 132.8, 128.9, 124.6, 111.2, 109.5, 109.3, 88.0, 56.3, 46.7, 40.3, 33.3, 31.7, 31.1, 27.1, 25.6, 21.2, 20.0, 19.1, 15.9, 15.3 ppm; 1H-NMR (400 MHz, CDCl3) δH 7.14 (s, 1H), 5.96 (s, 1H), 5.33 (s, 1H), 4.78 (q, 6.6 Hz, 1H), 3.76 (s, 2H), 1.95–1.78 (m, 4H), 1.68 (m, 1H), 1.46 (s, 3H) 1.37 (d, 6.6 Hz, 3H), 1.32 (m, 2H), 1.24–1.14 (m, 5H), 0.74 (m, 3H), 0.72 (s, 3H) ppm. The obtained spectrometric and spectroscopic data suggest a novel marinone derivative.
Compound (2): yellow solid (3.9 mg); Rt = 34 min, UV λmax (nm): 218, 263, 312, 400 nm; IR NaCl νmax (cm−1): 2957.52, 2923.03, 2851.87, 1738.95, 1572.27, 1464.32, 1379.87, 1303.05, 1182.50, 1052.63 cm-1; HR-MS m/z: 424.225 calcd. for C26H32O5, measured 424.2249; MS: m/z 425.2 Da [M + H]+; 849.3 Da [2M + H]+; 13C-NMR (100 MHz, DMSO-d6): δC 183.2, 181.1, 159.7, 157.2, 152.6, 138.7, 130.6, 127.1, 123.3, 120.0, 107.9, 107.6, 86.1, 46.5, 40.3, 32.2, 30.5, 30.0, 26.1, 24.7, 20.3, 19.0, 18.2, 15.1, 14.4, 7.6 ppm; 1H-NMR (400 MHz, DMSO-d6): δH 7.03 (s, 1H), 5.34 (s, 1H), 4.79 (q, 6.6 Hz, 1H), 1.98 (s, 3H), 1.93–1.82 (m, 4H), 1.67 (m, 1H), 1.47 (m, 3H), 1.37 (d, 6.6 Hz, 3H), 1.34 (m, 2H), 1.19 (m, 5H), 0.75 (m, 3H), 0.73 (s, 3H) ppm. The obtained spectrometric and spectroscopic data is in accordance with the reported for metabolite neomarinone (Hardt et al., 2000; Kalaitzis et al., 2003).
2.5 Antibiofilm activity (microfouling) evaluation
2.5.1 Marine fouling bacteria culture conditions
To assess antimicrofouling activity, we selected five marine bacterial species as models: Marinobacter hydrocarbonoclasticus DSM 8798 (ATCC 49840), C. marina DSM 4741, Phaeobacter inhibens DSM 17,395, Pseusooceanicola batsensis DSM 15,984, and Micrococcus luteus DSM 20030 (ATCC 4698) (Michael et al., 2016). These strains, sourced from DSMZ (Leibniz Institute DSMZ—German Collection of Microorganisms and Cell Cultures), were cultured in liquid marine broth (Carl Roth GmbH, Karlsruhe, Germany) with agitation at 180 rpm or on agar-supplemented marine broth. Incubation temperatures were set at 28°C for M. hydrocarbonoclasticus and Cobetia marina, 30°C for P. inhibens and P. batsensis, while M. luteus was maintained at 37°C in Brain Heart Infusion broth (BHI, Becton Dickinson, GmbH, Heidelberg, Germany) under similar agitation conditions.
2.5.2 Antibacterial activity evaluation
The antibacterial efficacy of compounds (1) and (2) was assessed in 96-well polystyrene flat-bottom microplates (Nunclon Delta Surface, Thermo Scientific, Roskilde, Denmark) following established procedures (Bauermeister et al., 2019). In the initial screening, bacterial overnight cultures were diluted to an optical density (OD600nm) of 0.2 and incubated statically at appropriate temperatures. The cultures were treated with the following final concentrations per well: 31.25, 15.60, 7.81, 3.91, 1.95, and 0.98 μg/mL (2-fold serial dilutions) of the compounds, solubilized in DMSO, or left untreated. After 24 h (M. hydrocarbonoclasticus, C. marina) or 48 h (P. batsensis, P. inhibens, M. luteus) incubation, optical density at 600 nm (OD600) was measured using a Molecular Devices Spectra Max 190. Growth inhibition percentages were calculated relatively to untreated bacterial species, using DMSO as a negative control. CuSO4 (0.16 μg/mL), a recognized antifouling agent, was used as positive control. All assays were conducted in triplicate, and the results represent the mean and standard error of the mean (SEM). Statistical analysis was carried out using GraphPad Prism 8.0.2 (San Diego, CA, United States), employing one-way ANOVA followed by Dunnett’s multiple comparisons test against the negative control.
2.5.3 Antibiofilm activity evaluation
The antibiofilm potential of compounds (1) and (2) against the five marine bacterial species was investigated in 96-well polystyrene flat-bottom microplates (Nunclon Delta Surface, Thermo Scientific, Roskilde, Denmark), as described previously (Bauermeister et al., 2019; Pereira et al., 2020). In the initial screening, bacterial overnight cultures were diluted to an OD600 of 0.2 and incubated statically at appropriate temperatures. The cultures were tested at 31.25, 15.60, 7.81, 3.91, 1.95, 0.98 μg/mL concentrations (2-fold serial dilutions) of compounds (1) and (2), solubilized in DMSO, or left untreated. After 24 h (M. hydrocarbonoclasticus, C. marina) or 48 h (P. batsensis, P. inhibens, M. luteus) incubation, OD600 was measured. The planktonic cells and media were discarded, and the wells were washed twice with deionized water. Biofilms were fixed, stained, and quantified by measuring the OD600 after solubilization with acetic acid. Biofilm inhibition percentages were calculated relative to untreated bacterial species, using DMSO as a negative control and CuSO4 (0.16 μg/mL), as a positive control. All assays were performed in triplicate, and the results represent the mean and standard error of the mean (SEM). Statistical analysis was conducted using GraphPad Prism 8.0.2, employing one-way ANOVA followed by Dunnett’s multiple comparisons test against the negative control.
2.6 Antibiofouling activity (macrofouling) evaluation
2.6.1 Mussel larvae (Mytilus galloprovincialis) acute toxicity assay
The in vivo antimacrofouling activity of compounds (1) and (2) was evaluated against mussel M. galloprovincialis adhesive larvae (plantigrades) in an acute bioassay. Juvenile mussel aggregates were harvested from the intertidal rocky shore during low spring tides at Memória beach, Matosinhos, Portugal. In the laboratory, precisely before the bioassays, mussel plantigrade larvae were meticulously screened and isolated from the juvenile aggregates using a binocular microscope (Olympus SZX2-ILLT, Hamburg, Germany). The isolated larvae were then washed with filtered seawater to eliminate organic debris, and competent plantigrade larvae, characterized by foot exploratory behavior, were selected for the exposure bioassays, following established protocols (Almeida et al., 2017; Almeida et al., 2020; Antunes et al., 2019; Mabrouk et al., 2020). Plantigrades were exposed in 24-well polystyrene plates for 15 h in darkness at 18°C. DMSO served as the solvent for the testing compounds in stock and working solutions. The DMSO concentration in the tested solutions was consistently maintained at 0.1%. Each condition was replicated in four wells, with five larvae per well. Two negative controls, one with ultra-pure water and other with DMSO were included in all bioassays, along with a positive control using 0.16 μg/mL CuSO4, as a reference antifouling agent. The anti-settlement bioactivity was assessed based on the presence or absence of fixed byssal threads produced by each individual larva for all tested conditions. Compounds (1) was tested at successive concentrations 10, 5, 2.5, 1.2, and 0.6 μg/mL and compound (2) at 4, 2, 1, 0.5, 0.25, 0.12 μg/mL to determine the semi-maximum response concentrations (EC50) with an anti-settlement effect on mussel larvae. EC50 values for each compound were calculated using Probit regression analysis. Significance was considered at p < 0.01, and 95% lower and upper confidence limits (95% LCL; UCL). The software IBM SPSS Statistics 28 was used for statistical analysis. The therapeutic ratio (LC50/EC50) was employed to evaluate the effectiveness versus the toxicity of the compounds (Qian et al., 2009; Almeida and Vasconcelos, 2015).
2.7 In silico environmental toxicity evaluation
The evaluation of in silico toxicity was conducted using the Toxicity Estimation Software Tool (T.E.S.T.) version 5.1.2 (CCTE, EPA, 2022), available at https://www.epa.gov/chemical-research/toxicity-estimation-software-tool-test.
2.8 In vivo environmental toxicity evaluation
The in vivo evaluation of compounds (1) and (2) for acute toxicity was performed towards marine bacterium Aliivibrio fischeri, and planktonic crustacean Daphnia magna. The chronic ecotoxicity tests were evaluated using algae Pseudokirchneriella subcapitata and Phaeodactylum tricornutum.
The inhibition of bioluminescence of the marine bacterium A. fischeri, were studied following a micro adaptation of the standard procedure ISO 11348-3 (“Water Quality-Determination of the inhibitory effect of water samples on the light emission of Vibrio fischeri - Luminescent bacteria test–using freeze-dried bacteria method”). Briefly, frozen lyophilized bacterial cells (Abraxis, Warminster, PA, United States) were reconstituted in Reconstitution Solution, supplied by the manufacturer of the Abratox kit (Abraxis). Madeirone (1) and neomarinone (2) were dissolved in DMSO and tested at the concentrations of 100, 50, 25, 12.5, 6.25, 3.13, and 1.56 μg/mL. The emitted luminescence was measured using a luminometer at time 0 min and at different exposure times (5, 15 and 30 min) and the percentage of inhibition of bioluminescence was determined. Each experiment was performed in duplicate and the results of three independent experiments with <10% SD are presented. Phenol was used as a positive control.
The immobilization of D. magna at 24 h and 48 h was studied following a micro adaptation of the OECD Guideline 202 procedures (“Daphnia sp., Acute Immobilisation Test and Reproduction Test”), using the Daphtoxkit F (Microbiotests, Gent, Belgium). Firstly, the ISO matrix medium was prepared, aerated and adjusted to pH 7. Next, the neonate daphnids were incubated for 72 h at 20 ± 1°C with light and fed spirulina 2 h prior to use, according to the manufacturer’s instructions. Subsequently, the daphnids were exposed to madeirone (1) and neomarinone (2) dissolved in DMSO at the concentrations of 100, 50, 25, 12.5, 6.25, 3.13, and 1.56 μg/mL, and the immobilization of the daphnids was recorded by direct observation at 24 and 48 h. For the miniaturization of the procedure, one daphnid neonate was exposed to each compound in 10 mL sterile tubes containing 2 mL of ISO matrix medium, with the compounds at the determined concentrations. The assays were performed in triplicate. Potassium dichromate (K2Cr2O7) was used as a positive control.
Freshwater and marine algal growth inhibition was assessed using P. subcapitata and P. tricornutum according to OECD Guideline 201 procedures (“Alga, Growth Inhibition Test”), ISO-8692 (“Water quality–Fresh water algal growth inhibition test with unicellular green algae”) and ISO-10253. Briefly, the algae cells were de-immobilized from algal beads using Matrix dissolving medium (Microbiotests), according to the manufacturer’s instructions, and grown in flasks containing algal culturing medium which was prepared following the OECD Guideline 201 and ISO-8692. Flasks with algae were kept for 7 days under light with orbital agitation. When the algae culture had sufficient cells, as verified by the optical density at 670 nm, these were tested at an initial cell density of 1 × 106 cells/mL in algal culturing medium. Madeirone (1) and neomarinone (2) were dissolved in DMSO and tested at the concentrations of 100, 50, 25, 12.5, 6.25, 3.13, and 1.56 μg/mL. The algae were cultured in microplates in triplicate and were kept for 4 days under light with orbital agitation. The optical density was measured at 670 nm, at time zero and after 24, 48, 72 and 96 h incubation, determining the algal growth inhibition of the compounds. Each experiment was performed in triplicate and the results of two independent experiments with <10% SD are presented. Growth inhibition calculations were performed using cell numbers (biomass), as determined by a calibration line. Potassium dichromate (K2Cr2O7) was used as a positive control.
3 Results and discussion
3.1 Structural elucidation of marinones isolated from Streptomyces aculeolatus PTM-346
Marine-derived strain PTM-346 was taxonomically characterized as S. aculeolatus (Prieto-Davó et al., 2016). These species belong to MAR4 group, which are known to produce meroterpenoids (Murray et al., 2020) from the classes napyradiomycins, marinones, lavanducyanins, nitropyrrolines, novobiocins or chlorobiocins (Pathirana et al., 1992; Hardt et al., 2000; Kawasaki et al., 2006; Gallagher et al., 2010; Gallagher et al., 2013; Kirschner and Brennan, 2012; Gallagher and Jensen, 2015).
In this study, the ethyl acetate (EtOAc) extracts of S. aculeolatus PTM-346 (Prieto-Davó et al., 2016; Bauermeister et al., 2019) underwent micro and macro antifouling bioassay-directed fractionation and isolation. This process initially was performed using silica flash chromatography and subsequently employed C18 reversed-phase semi-prep HPLC, resulting in the isolation of two compounds (1, 2). The chemical structures of (1) and (2) were elucidated using MS spectrometry and the interpretation of UV-Vis, IR, 1D- and 2D-NMR spectroscopic data. Both isolated compounds were determined to belong to the class of marinones, which are hybrid isoprenoids, sesquiterpene naphthoquinones with a mixed origin of polyketides and terpenoids also designated meroterpenoids (Olano et al., 2008; Gallagher et al., 2013). To date, six compounds of the marinone class have been reported (Table 1).
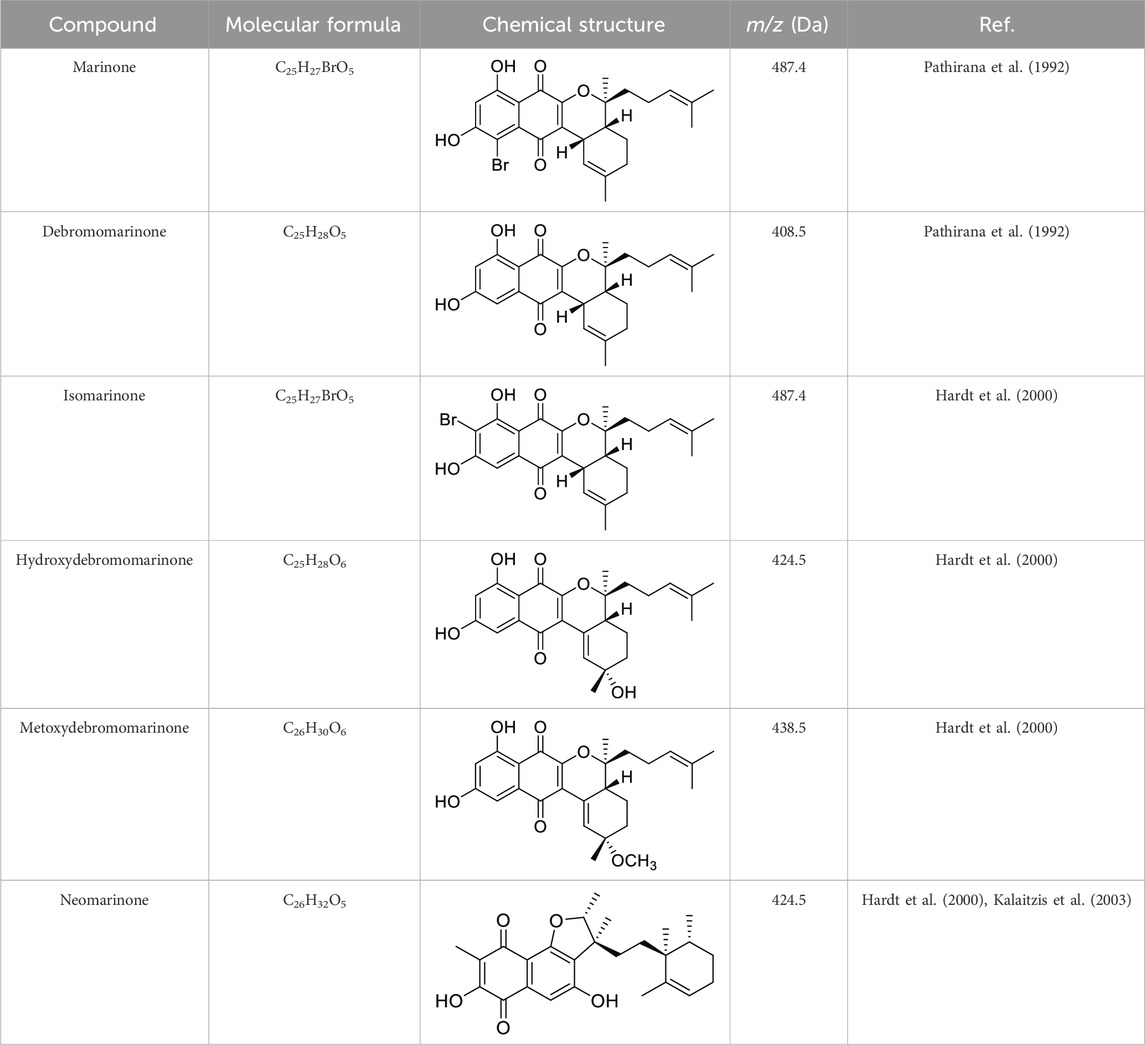
Table 1. List of all marinone metabolites described in the literature (Pathirana et al., 1992; Hardt et al., 2000; Kalaitzis et al., 2003).
The elucidation of compound (1) revealed a previously undescribed marinone derivative. This discovery expands the known class of marinones to seven, as shown in Table 2 and Figure 1. The novel compound (1) has been named madeirone, after the Madeira Archipelago, the origin of strain PTM-346.
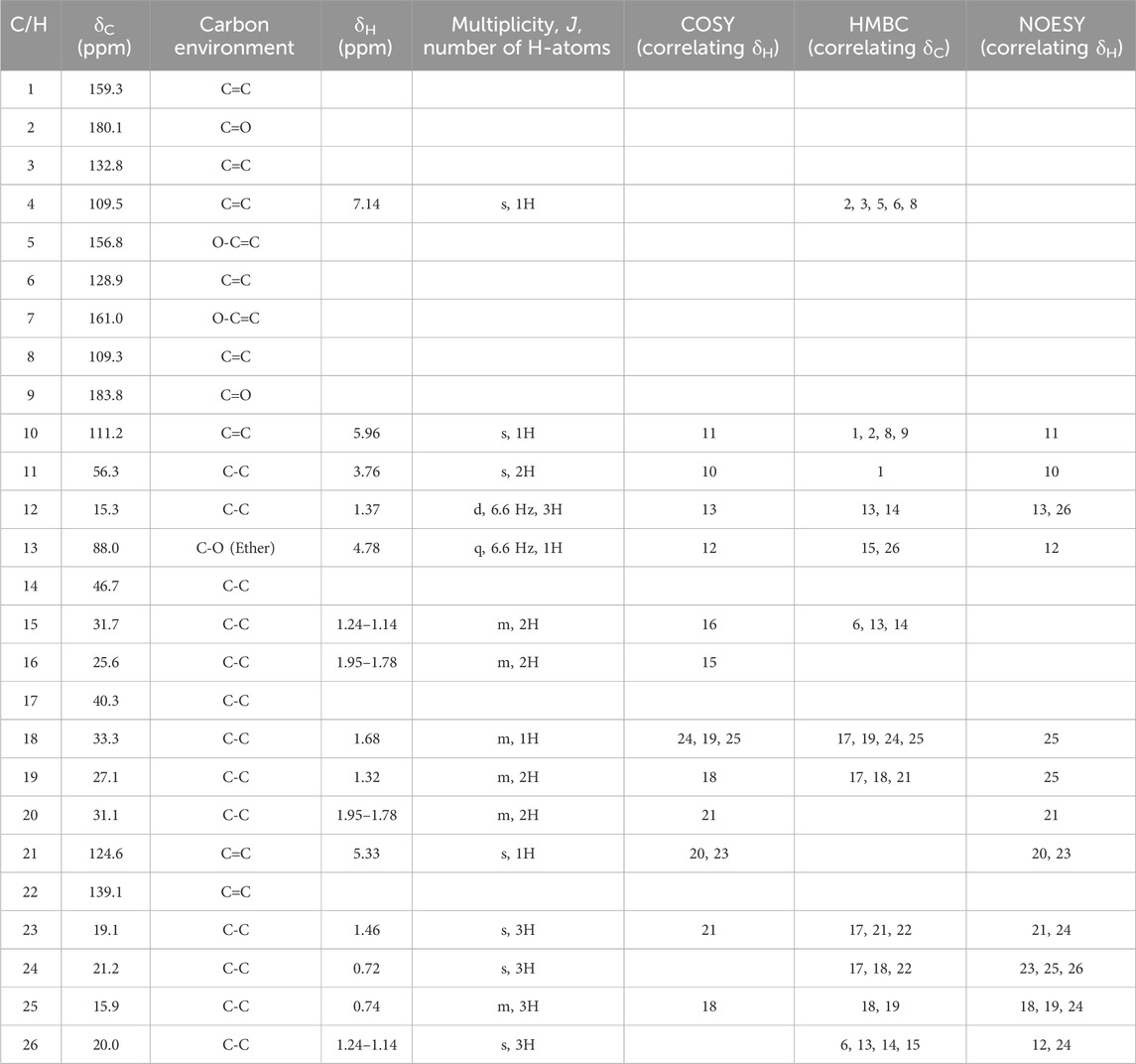
Table 2. Obtained NMR data for the novel compound madeirone (1) in CDCl3. Protons were assigned via HSQC to the corresponding carbon atoms. 1H-spectra were recorded at 400 MHz,13C-NMR spectra at 100 MHz.
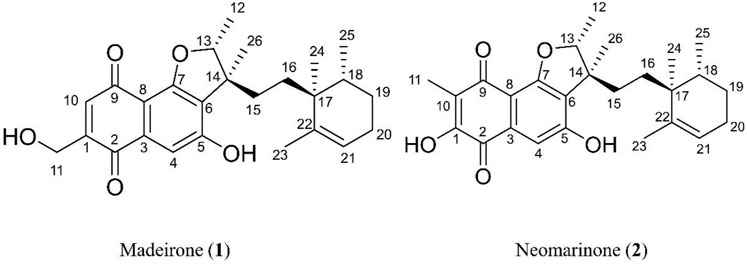
Figure 1. Chemical structures of madeirone (1) and neomarinone (2), isolated from marine-derived S. aculeolatus strain PTM-346.
The identification of (1) was aided by MS analysis, which revealed a molecular mass of 424.2 Da, corresponding to the molecular formula C26H32O5. The molecular formula was further confirmed by the hydrogen and carbon atom counts obtained from the 1H- and 13C-NMR spectra. IR spectroscopic data revealed absorption bands at 3273 and 1,633 cm−1, characteristic of marinone and its derivatives (Hardt et al., 2000). Compound (1) shares the same molecular mass and structural formula as neomarinone (Table 1), a known compound with previously described antibiotic activity and cytotoxicity against human colon carcinoma cells HCT-116 (Pathirana et al., 1992; Hardt et al., 2000; Peña-López et al., 2009). However, analysis of the 1D- and 2D-NMR spectra revealed that compound (1) is a derivative and molecular isomer of neomarinone.
Further evidence of the novelty of compound (1) was provided through the elucidation of compound (2) as neomarinone. Identification of compound (2) was facilitated by HR-MS, which revealed a molecular mass of 424.225 Da, corresponding to the molecular formula of C26H32O5. The spectrometric and spectroscopic data obtained for compound (2) confirmed its identity as neomarinone (Table 3; Figure 1) (Hardt et al., 2000; Kalaitzis et al., 2003). The distinct structures of compounds (1) and (2) were further supported by their differing retention times in analytical HPLC analysis, with compound (1) having a retention time of 20.9 min and compound (2) having a retention time of 22.0 min (data not shown).
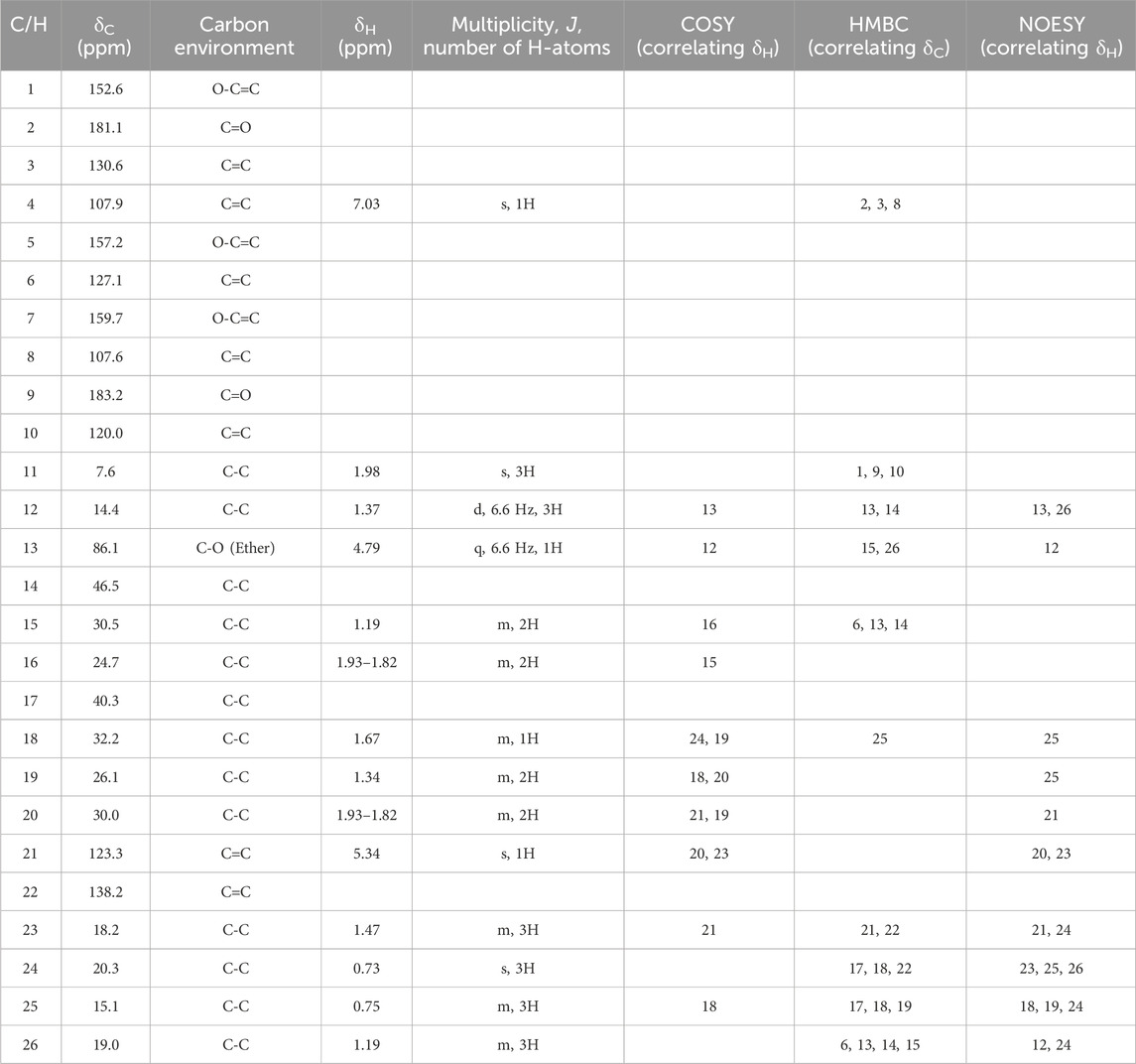
Table 3. Obtained NMR data for neomarinone (2) in DMSO-d6. Protons were assigned via HSQC to the corresponding carbon atoms. 1H-spectra were recorded at 400 MHz,13C-NMR spectra at 100 MHz.
The HSQC experiment for compound (1) enabled the assignment of all proton signals to their corresponding carbon atoms. A comparative analysis of the 1D- and 2D-NMR spectra (Table 2; Table 3) for compounds (1) and (2) revealed that most of the chemical shifts and coupling patterns were identical. The structural differences of compound (1) compared to compound (2) were localized to the 1,4-benzoquinone moiety, specifically at C-1, C10 and C-11. Minor chemical shift variations were attributed to the use of different NMR solvents (CDCl3 for compound (1) and DMSO-d6 for compound (2)).
HSQC data for compound (1) revealed the assignment of one proton to C-10 and two protons to C-11, with no proton assigned to C-1. HMBC spectrum for compound (1) showed correlations of H-11 to C-1, and H-10 to C-1, C-2, C-8 and C-9. Additionally, COSY data showed coupling of H-10 with H-11. The 1H-NMR data depicted both H-10 and H-11 as singlets, indicating that these protons are not located on neighboring C-atoms.
The stereochemistry of compounds (1) and (2) was determined via NOESY data. For both compounds, strong correlations were observed between the chiral methyl groups H-12 and H-26, H-24 and H-25, as well as H-24 and H-26. The correlations indicate cis orientations of these groups, as previously reported for compound (2) in the literature (Hardt et al., 2000; Kalaitzis et al., 2003). Thus, the chirality of compounds (1) and (2) was found to be identical. Consequently, the combined NMR data of compound (1) suggests the substituted 1,4-benzoquinone moiety presented in Figure 1.
Notably, S. aculeolatus PTM-346 exhibits a significant distinction, regarding secondary metabolite profile. Since the other five strains of the same species obtained from the same location, the Madeira Archipelago, Portugal, produced a different class of meroterpenoid compounds termed napyradiomycins (Gaudêncio et al., 2016; Bauermeister et al., 2019; Pereira et al., 2020).
3.2 Marinones micro and macrofouling inhibitory activity assessment
The antimicrofouling activity of marinones derivatives (1) and (2) was assessed by examining their inhibitory effects on bacterial growth and on the formation of bacterial biofilms. For the bioactivity assays, five species of marine bacteria were selected, based on their proficiency in biofilm production and recognized as influential contributors to fouling, serving as primary colonizers on submerged surfaces (Dang et al., 2008). These bacterial models include, P. inhibens (DSM 17395), P. batsensis (DSM 15984), M. hydrocarbonoclasticus (DSM 8798), C. marina (DSM 4741) and M. luteus (DSM 20030, ATCC 4698) (El-Masry et al., 1995; Ekblad et al., 2008; Akesso et al., 2009; Briand, 2009; D’Souza et al., 2010; Inbakandan et al., 2010; Michael et al., 2016; Majzoub et al., 2018).
The antimacrofouling activity of marinone derivatives (1) and (2) was assessed concerning the settlement of plantigrade larvae of M. galloprovincialis.
3.2.1 Antibacterial activity evaluation
The most promising compounds for antimicrofouling are those that inhibit biofilm formation without affecting the growth of the bacteria. The Minimum Biofilm Inhibitory Concentration (MBIC) is the value that inhibits biofilm formation by over 80% (upper threshold) and simultaneously inhibits bacterial growth by less than 40% (lower threshold) (Kwasny and Opperman, 2010; Bauermeister et al., 2019; Sabotič et al., 2024). To evaluate the anti-biofilm activity of the compounds, we first determined their antibacterial activity (Table 4).
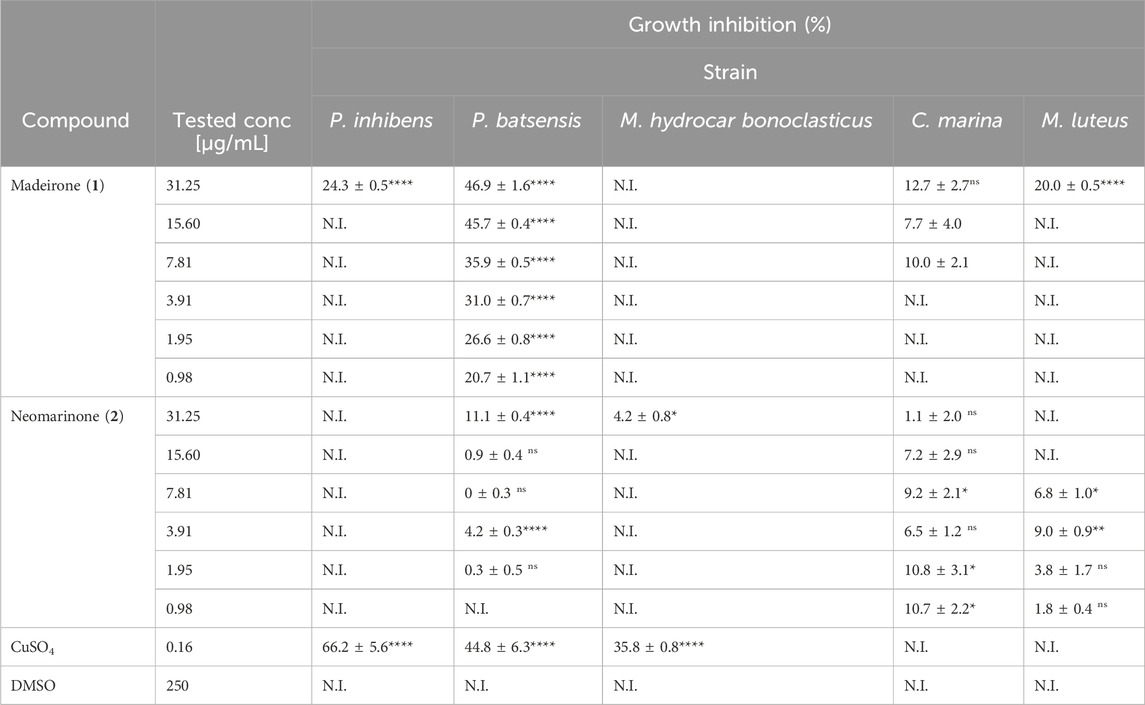
Table 4. Percentage of growth inhibition for several marine bacteria in the presence of different concentrations of madeirone (1) and neomarinone (2). Shown are the average values of the percentage of growth inhibition of three replicates with the standard error of the mean (SEM). N.I—not inhibited. Results were statistically significant (****p < 0.0001, ***p < 0.001, **p < 0.01, *p < 0.05, Dunnet’s test).
Madeirone (1) demonstrated a 24.3% ± 0.5% inhibition of P. inhibens growth for a concentration of 31.25 μg/mL and no inhibition for all the lower tested concentrations. It exhibited consistent inhibitory effects on P. batsensis across all tested concentrations, with inhibition values over 40% for concentrations 31.25 μg/mL and 15.60 μg/mL. No evident inhibition was observed for M. hydrocarbonoclasticus for any of the tested concentrations. Regarding the growth of C. marina, the compound showed inhibition percentages ranging from 12.7% ± 2.7% to 7.7% ± 4.0% at concentrations between 31.25 and 7.81 μg/mL. M. luteus growth was inhibited by 20.0% ± 0.5% and 9.2 %± 0.2% at a concentration of 31.25 μg/mL and 15.60 μg/mL, respectively.
Neomarinone (2) did not inhibit the growth of P. inhibens at any of the tested concentrations. It exhibited no to low inhibitory effects on P. batsensis, at concentrations ranging from 31.25 to 1.95 μg/mL, resulting in inhibition percentages ranging from 0.0% ± 0.3% to 11.1% ± 0.4% at the highest concentration. The growth of M. hydrocarbonoclasticus was only inhibited by 4.2% ± 0.8% at 31.25 μg/mL. C. marina was inhibited at tested concentrations from 1.1% ± 2.0% to 10.7% ± 2.2%, while compound (2) inhibited M. luteus at concentrations from 7.81 to 0.98 μg/mL, resulting in inhibition percentages ranging from 9.0% ± 0.9% to 1.8% ± 0.4%.
CuSO4, a highly effective antifouling agent utilized in antifouling paints, served as the reference in this study, at a concentration of 0.16 μg/mL. The application of CuSO4 resulted in significant inhibitions in the growth of P. inhibens, P. batsensis, and M. hydrocarbonoclasticus, with inhibitory percentages of 66.2% ± 5.6%, 44.8% ± 6.3%, and 35.8% ± 0.8%, respectively. No inhibition of growth was observed for M. luteus and C. marina under the influence of CuSO4. In DMSO no bacterial growth inhibition was observed for any of the tested strains in the presence of both madeirone (1) and neomarinone (2).
The results of growth inhibition of marine bacteria obtained for the metabolite madeirone (1) were <25% at the highest tested concentration for almost all tested bacteria (P. inhibens, M. hydrocarbonoclasticus, M. luteus and C. marina), except for P. batsensis where the growth inhibition was close to 50%. The growth inhibition for neomarinone (2) were around 10% or lower for P. batsensis, C. marina, M. hydrocarbonoclasticus, and M. luteus at the highest tested concentration.
Thus, both compounds showed growth inhibition activity mostly below the 40% threshold considered for antifouling activity (Kwasny and Opperman, 2010; Bauermeister et al., 2019; Sabotič et al., 2024), and below the values obtained for CuSO4, emerging as promising compounds for marine intervention, since they do not have a killing effect on marine bacteria.
3.2.2 Antibiofilm activity assessment
Madeirone (1) effectively hindered the biofilm formation of P. batsensis and C. marina across all tested concentrations, exhibiting inhibitory percentages ranging from 10.8% ± 1.7% to 59.2% ± 0.4%. The biofilm formation of P. inhibens was impeded at concentrations ranging from 31.25 μg/mL to 3.91 μg/mL, resulting in inhibition percentages ranging from 66.7% ± 0.3% to 10.2% ± 3.7%, respectively. Similarly, M. hydrocarbonoclasticus biofilm formation showed inhibition at concentrations ranging from 7.81 μg/mL to 31.25 μg/mL, with inhibitory percentages ranging from 14.2% ± 3.6% to 60.1% ± 0.5%, respectively. M. luteus reveals inhibition of 35.3% ± 0.2% only at the highest tested concentration of 31.25 μg/mL (Table 5).
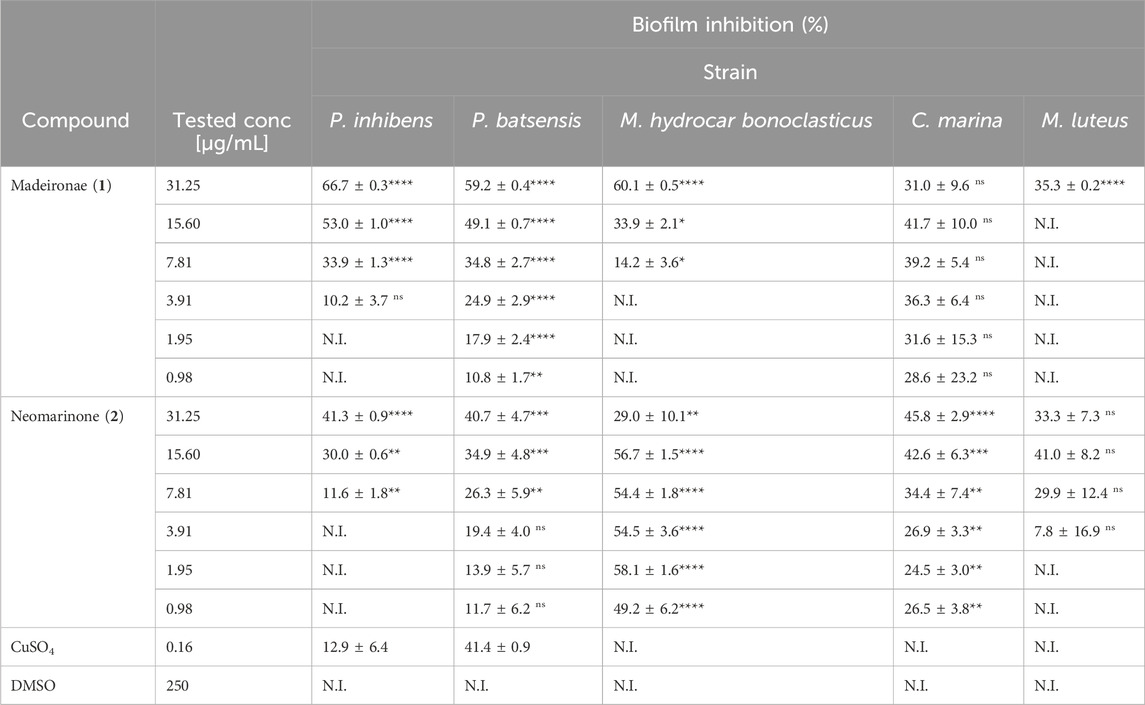
Table 5. Percentage of inhibition of biofilm formation for several marine bacteria in the presence of different concentrations of madeirone (1) and neomarinone (2). Shown are the average values of the percentage of biofilm inhibition of three replicates with the standard error of the mean (SEM). N.I—not inhibited. Results were statistically significant (****p < 0.0001, ***p < 0.001, **p < 0.01, *p < 0.05, Dunnet’s test).
In contrast, neomarinone (2) exhibited inhibitory effects on the biofilm formation of P. batsensis, M. hydrocarbonoclasticus, and C. marina at all tested concentrations, with the lowest inhibition at 11.7% ± 6.2% and the highest at 58.1% ± 1.6%. The biofilm formation of P. inhibens was hindered at concentrations ranging from 7.81 to 31.25 μg/mL, resulting in inhibition percentages ranging from 11.6% ± 1.8% to 41.3% ± 0.9%, respectively. Moreover, M. luteus inhibition occurred at concentrations ranging from 3.91 to 31.25 μg/mL, with inhibitory percentages ranging from 7.8% ± 16.9% to 41.0% ± 8.2% (Table 5).
Madeirone (1) showed biofilm inhibition results exceeding 50% for P. inhibens at 15.6 μg/mL, and M. hydrocarbonoclasticus at 31.35 μg/mL, and surpassing 30% for C. marina at all tested concentrations. At these concentration values, the growth inhibition was below 10%. The only exception was P. batsensis, for which the growth inhibition showed similar values than the ones for biofilm inhibition.
Neomarinone (2) demonstrated more than 40% inhibition of biofilm formation in all tested marine fouling bacteria. Specifically, at a concentration of 31.35 μg/mL, it exhibited inhibition for P. inhibens and P. batsensis, at 15.60 μg/mL for M. luteus, and at 15.60 and 31.25 μg/mL for C. marina. Notably, it displayed inhibition ranging from 40% to 60% for M. hydrocarbonoclasticus at all concentrations except 31.25 μg/mL. For neomarinone (2), the values of growth inhibition were below 10% or only slightly over this value.
CuSO4 only inhibited the biofilm formation of P. batsensis, with an inhibitory percentage of 41.4% ± 0.9%. There was no observable inhibition in the biofilm formation of M. luteus, M. hydrocarbonoclasticus and C. marina, and for P. inhibens the inhibition was only 12.9% ± 6.4%. No inhibition of biofilm formation was observed in DMSO for any of the tested strains in the presence of both madeirone (1) and neomarinone (2).
Although the values of percentage of biofilm inhibition were lower (between ∼40 and 66%) than the 80% threshold usually used to establish the MBIC value (Kwasny and Opperman, 2010; Bauermeister et al., 2019; Sabotič et al., 2024), we considered that the two compounds in study have promising antibiofilm activity, due to their very low effect on growth inhibition (0–∼20%).
Overall, madeirone (1) exhibited promising antibiofilm efficacy against P. inhibens, up to 66% inhibition, M. hydrocarbonoclasticus (up to 60% inhibition) and C. marina (up to 40% inhibition), along with growth inhibition activity below 10%, for the same respective concentrations of this compound.
Neomarinone (2) also displayed positive antibiofilm outcomes, with up to 41% inhibition against P. inhibens, 40% inhibition against P. batsensis, 56% inhibition against M. hydrocarbonoclasticus, 46% inhibition against C. marina, 40% inhibition against M. luteus, along with growth inhibition activity below 10%, for the same respective concentrations of this compound.
It is noteworthy that, except for madeirone (1) against P. batsensis, negligible growth inhibition was observed. This emphasizes the compound’s effectiveness as potent antibiofilm agents without compromising the viability of the targeted bacteria, a crucial point that is reinforced by this study. The antibiofilm activity of these marinone derivatives is independent of their antibacterial effects. This suggests that these compounds could serve as antibiofilm agents without contributing to antibiotic/biocide resistance.
Several reported studies involving Streptomyces compounds have highlighted their antibiofilm activity by referencing the Minimum Inhibitory Concentration (MIC) for growth inhibition. However, it is essential to clarify that the focus should be on the Minimum Biofilm Inhibitory Concentration (MBIC), which denotes the inhibition of biofilm growth without adversely affecting bacterial growth (Selvin, 2009; Cho and Kim, 2012; Gopikrishnan et al., 2016; Kavitha and Vimala, 2020; She et al., 2022; Stalin et al., 2022).
Our group previously reported the antifouling activity of napyradiomycins derivatives, meroterpenoides isolated from S. aculeolatus obtained from ocean sediments collected in the Madeira Archipelago. Napyradiomycins inhibited ≥80% of the marine biofilm formation for the same assayed bacteria. In comparison, marinones revealed lower antibiofilm inhibition than napyradiomycins, but also lower growth inhibition rates (Pereira et al., 2020). In comparison to napiradionycins, marinones hold a distinct edge as they inhibit antibiofilm-forming bacteria more effectively, and at lower concentrations. Both marinones and napyradiomycins surpass existing commercial biocides due to their non-toxic nature and their ability to inhibit microfouling.
3.2.3 Assessment of antifouling properties on Mytilus galloprovincialis larval settlement
The antimacrofouling activities of compounds (1) and (2) were assessed against the plantigrade larval settlement of M. galloprovincialis and exhibited EC50 values of 1.755 and 0.119 μg/mL, respectively (Table 6).

Table 6. Settlement response of M. galloprovincialis plantigrade larvae to marinone derivatives (1, 2) after a 15 h acute exposure assay. The therapeutic ratio (LC50/EC50) was used to assess the efficacy of each compound relative to its toxicity. Negative control: DMSO = 100% settlement; Positive control: 0.16 μg/mL CuSO4 = 0% settlement.
The marinones used in this study demonstrated notable effectiveness, with an EC50 value much lower than the advisable threshold for compounds consideration as effective AF agents, 25 μg/mL (Almeida and Vasconcelos, 2015). Remarkably, the EC50 of (2) was lower than that of ivermectin (EC50 = 0.4 μg/mL against M. edulis) (Davies et al., 1997) and lower than other promising reported compounds isolated from Streptomyces (Selvin, 2009; Cho, 2012; Cho et al., 2012; Cho and Kim, 2012; Prakash et al., 2015; Gopikrishnan et al., 2016), similar to the most promising napyradiomycins previously reported by our group from actinomycetes S. aculeolatus. This napyradiomycins displayed settlement results of M. galloprovincialis larvae with an EC50 of less than 5 μg/mL and a LC50/EC50 ratio greater than 15 (Pereira et al., 2020). Further, the EC50 of (2) was lower than that of other synthesized compounds or compounds from distinct bioresources (Neves et al., 2021; Pereira et al., 2021; Resende et al., 2021).
Regarding toxicity, none of the tested marinone derivatives caused mortality to M. galloprovincialis larvae at the maximum tested dose (10 μg/mL). Consequently, LC50 values exceeding 10 and 4 μg/mL, respectively were considered, and therapeutic ratios (LC50/EC50) were computed using the EC50 and LC50 values. To meet the standard requirement for the efficacy of natural antifouling agents, the US Navy program established (LC50/EC50) > 15 as a therapeutic ratio cut-off (Qian et al., 2009). Consequently, particularly neomarinone (2) (LC50/EC50 = 35.71) emerges as the most promising antifouling marinone-derivative agent for M. galloprovincialis larvae (Table 6).
3.3 In silico ecotoxicity analysis
The growing concern regarding the ecotoxicity of various pharmaceuticals, biocides, and chemical compounds has prompted regulatory authorities to advocate for the adoption of in silico risk assessment methodologies. Employing the Toxicity Estimation Software Tool (T.E.S.T.) (CCTE, EPA 2022), compounds (1) and (2) underwent a comprehensive evaluation to assess their potential ecotoxicity. For a comparative analysis, approved drugs such as paracetamol (3) and methicillin (4) were included, along with antifouling agents lobocompactol (5), invermictin B1b (6), and B1a (7) (commercialized ivermectin is a mixture of two molecular derivatives: 80% ivermectin B1a with an ethyl group at position C-26% and 20% ivermectin B1b with a methyl group at position C-26 (Pinori et al., 2011)), copper (8), and arsenic (9) (Table 7, 8). These approaches facilitate the anticipation of the fate of these molecules, their potential ecological impact, and potential indirect effects on human health.
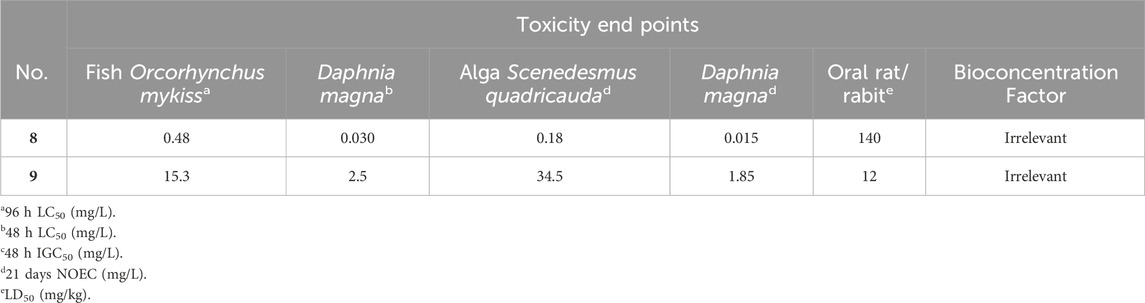
Table 8. Aquatic toxicity, environmental fate data and classification of copper and arsenic (Tišler and Zagorc-končan, 2003).
The Toxicity Estimation Software Tool (T.E.S.T.) does not predict the toxicity of salts, ions, or metals. Thus, for copper and arsenic the reported data of Tisler and Zagorc-Koncan is used (Tišler and Zagorc-končan, 2003) (Table 8).
In accordance with the European Union Directive 2001/59/EC and Regulation 1,272/2008 on the Classification, Labelling, and Packaging of Substances and Mixtures (CLP), the classification of a substance as “harmful,” “toxic,” or “very toxic” to aquatic organisms is determined based on various criteria. These criteria include the 96-h LC50 for fish (e.g., fathead minnow), 48 h LC50 for daphnids (e.g., D. magna), and other assays like the 72 h IC50 for algae or 40 h IGC50 for protozoans (e.g., Tetrahymena pyriformis). If the IC50, LC50, or IGC50 falls below 1 mg/L, the substance is labeled as “very toxic to aquatic organisms” (indicated by the danger symbol N and risk phrase R50). Values between 1 and 10 mg/L classify the substance as “toxic to aquatic organisms” (danger symbol N, risk phrase R51), while endpoints between 10 and 100 mg/L result in classification as “harmful to aquatic organisms” (risk phrase R52). Additionally, classification considers factors such as ready biodegradability and bioaccumulation potential, assessed through the bioconcentration factor (BCF). If the BCF is ≥ 100, the compound is categorized as “may cause long-term adverse effects in the aquatic environment” (risk phrase R53) (Tišler and Zagorc-končan, 2003; Schipper et al., 2010). Acute Toxicity Estimates (ATE) categories, as outlined by the CLP regulation, depend on the Oral rat LD50 (Diaza et al., 2015). The four ATE thresholds are as follows: 1) category 1, ATE ≤5 mg/kg, designating the substance as “Fatal if swallowed”; 2) category 2, 5 < ATE ≤50 mg/kg, also classified as “Fatal if swallowed”; 3) category 3, 50 < ATE ≤300 mg/kg, labeled as (“Toxic if swallowed”); 4) category 4, 300 < ATE ≤2000 mg/kg, categorized as “Harmful if swallowed”; and 5) category 5, ATE >2000 mg/kg, indicating that the substance “may be Harmful if swallowed”. For the evaluation of toxicity toward humans, mutagenicity, carcinogenicity, and reproductive toxicity are crucial endpoints. Mutagenic toxicity can be experimentally assessed through various test systems, with the Ames test being the most common, which uses genetically engineered Salmonella typhimurium and Escherichia coli bacterial strains (Gini et al., 2014).
Analysis of the in silico results from the Toxicity Estimation Software Tool (T.E.S.T.) (table 7 and 8) enabled the categorization of the toxicity endpoints of compounds (1)–(9) (Table 9).
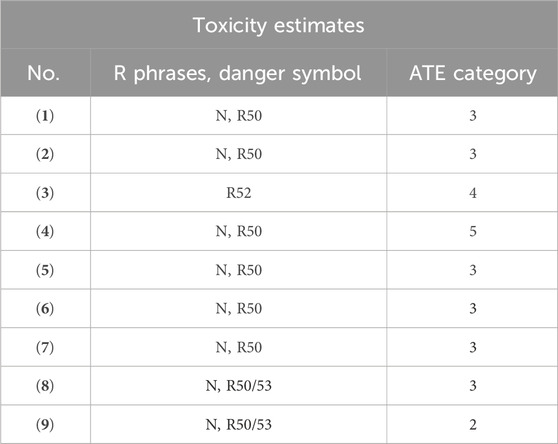
Table 9. Prediction of toxicity endpoint of madeirone (1), neomarinone (2) and approved drugs (3-9).
Madeirone (1) was assigned the danger symbol N (“dangerous for the environment”), risk phrase 50 (“very toxic to aquatic organisms”) and ATE 3 (“Toxic if swallowed”), neomarinone (2) was also categorized with symbol N (“dangerous for the environment”), risk phrase 50 (“very toxic to aquatic organisms”), but ATE 3 (“Toxic if swallowed”), meaning that compound (2) has higher Acute Toxicity Estimate category. Additionally, these marinone derivatives were identified as developmental toxicants with a low bioaccumulation factor and negative mutagenicity.
The approved drug paracetamol (3) has been classified as “harmful to aquatic organisms” (risk phrase R52) and ATE 4 (“Harmful if swallowed”). In contrast, the antibiotic methicillin (4) bears the danger symbol N (“dangerous for the environment”), risk phrase 50 (“very toxic to aquatic organisms”), and ATE 5 “may be Harmful if swallowed,” which does not differ significantly from the classifications of the studied marinone derivatives.
In silico values related to environmental toxicity for compounds (1, 2) (Table 7) showed similar orders of magnitude and classifications to those of the selected antibiofouling agents lobocompactol (5), invermictin B1b (6), and B1a (7), which all share the classification of symbol N (“dangerous for the environment”), risk phrase 50 (“very toxic to aquatic organisms”), and ATE 3 “Toxic if swallowed".
Copper (8) and arsenic (9) are also categorized with the symbol N (“dangerous for the environment”) and risk phrase 50 (“very toxic to aquatic organisms”). However, copper (8) has an ATE 3 “Toxic if swallowed,” while arsenic (9) has a higher ATE 2 “Fatal if swallowed.” Moreover, their BCF is ≥ 100, leading to their categorization with risk phrase R53, indicating that they “may cause long-term adverse effects in the aquatic environment” (Table 9) (Tišler and Zagorc-končan, 2003).
Globally, the in silico results suggest that madeirone (1) and neomarinone (2) are promising models for testing against Naval Sea Systems Command (NAVSEA) standards and advancing in the development roadmap for antifouling coatings (http://www.nstcenter.biz/navy-product-approval-process/navy-community-coatings-roadmap/, accessed on 26 January 2024).
3.4 In vivo ecotoxicity analysis
Madeirone (1) and neomarinone (2) were evaluated for acute toxicity towards A. fischeri and D. magna and chronic toxicity against P. subcapitata and P. tricornutum (Table 10).
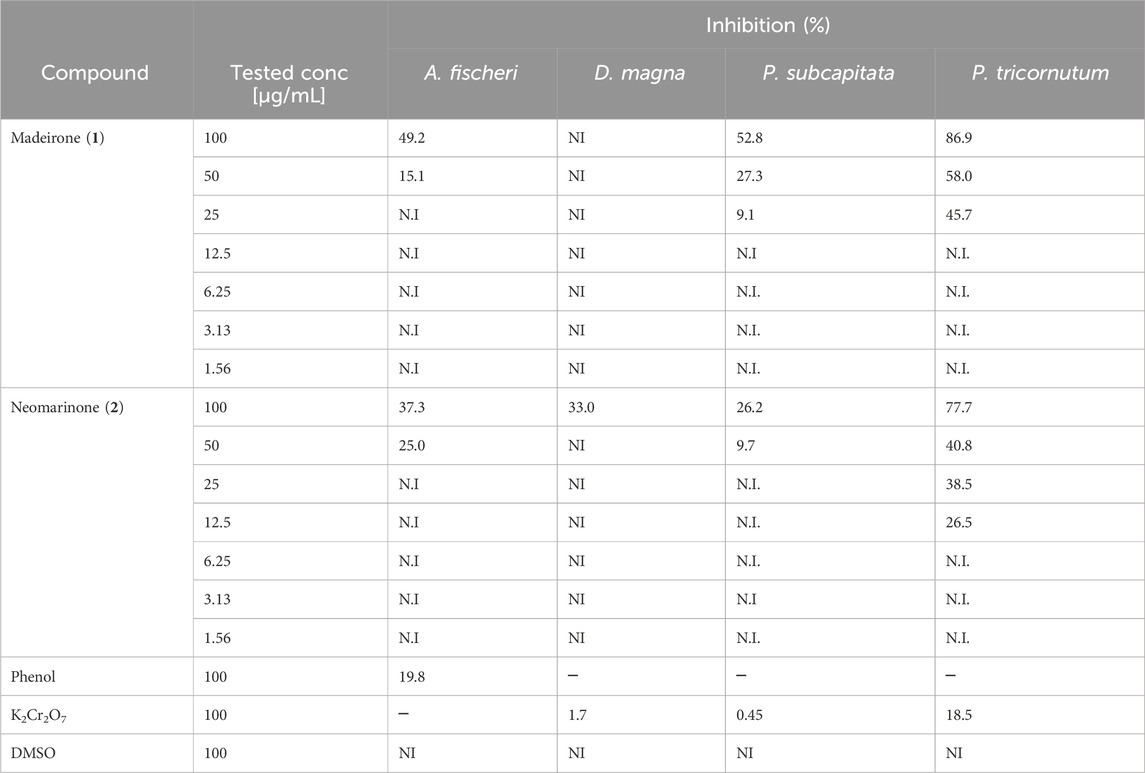
Table 10. Percentage of inhibition towards Aliivibrio fischeri, Daphnia magna, Pseudokirchneriella subcapitata and Phaeodactylum tricornutum at the tested concentrations of madeirone (1) and neomarinone (2). Shown are the average values of the percentage of three replicates. Only values with <10% SD are presented. N.I—not inhibited.
Madeirone (1) inhibited 49.2% the bioluminescence of A. fischeri at 100 μg/mL the highest tested concentration and 15.1% at 50 μg/mL. Neomarinone (2) demonstrated a similar toxicity of 37.3% and 25.0% at 100 μg/mL and 50 μg/mL against A. fischeri. D. magna was not immobilized at any of the concentrations tested for madeirone (1) and was immobilized by 33% at the highest tested concentration (100 μg/mL) for neomarinone (2) (Table 10).
The chronic ecotoxicity tests using algae P. subcapitata and P. tricornutum demonstrated that madeirone (1) was not toxic at the lowest tested concentrations (<12.5 μg/mL) (Table 10). Neomarinone (2) revealed no toxicity at concentrations ≤25 μg/mL against P. subcapitata and at concentrations ≤6.25 μg/mL for P. tricornutum (Table 10).
In vivo ecotoxicity studies highlight the compounds’ potential for further development as antifouling agents.
4 Conclusion
Novel metabolite madeirone (1) and neomarinone (2) exhibit a dual capability by effectively inhibiting both micro and macrofouling, distinguishing them from other commercial compounds and positioning them as agents for biofouling prevention. These possess antibiofilm and antifouling activities against species that contribute to fouling formation, without compromising their viability. These marinone derivatives not only impede the growth of various fouling organisms but also exhibit a minimal harmful impact on the marine ecosystem. Consequently, these compounds emerge as a promising alternative in the formulation of paints, varnishes, primers, and sealants, offering advantages over the use of copper compounds. In detail, madeirone (1) demonstrated significant antibiofilm efficacy against P. inhibens, achieving up to 66% inhibition, against M. hydrocarbonoclasticus (up to 60% inhibition) and C. marina (up to 40% inhibition). However, no biofilm inhibition was observed against M. luteus and similar antimicrobial and antibiofilm activity was observed against P. batsensis, with inhibition rates of up to 47% and 60%, respectively. Neomarinone (2) exhibited positive antibiofilm outcomes against all tested marine bacteria, with up to 41% inhibition against P. inhibens, 40% inhibition against P. batsensis, and 56% inhibition against M. hydrocarbonoclasticus. Furthermore, it demonstrated promising antibiofilm activity against C. marina, achieving up to 46% inhibition and against M. luteus, with 40% inhibition. In all cases, the growth inhibition activity was lower than 10%, for the same respective concentrations of the compounds. This underscores the compounds’ effectiveness as potent antibiofilm agents without compromising the viability of the targeted bacteria, a critical point that must be emphasized in this study. In fact, the antibiofilm activity of compounds (1) and (2) is independent of their antibacterial effects, suggesting that these could serve as antibiofilm agents without contributing to antibiotic/biocide resistance. Novel madeirone (1) and neomarinone (2), which was previously reported as having anticancer activity, are herein described as potent marine antibiofilm inhibitors and anti-macrofouling agents.
Madeirone (1) and neomarinone (2) also produced potent effects against the settlement of M. galloprovincialis larvae (EC50 = 1.76 and 0.12 μg/mL, respectively), along with no induced toxicity.
In silico environmental impact were predicted for madeirone (1) and neomarinone (2), with (1) assigned the danger symbol N, risk phrase 50, and ATE 4, while (2) shared the same symbol and risk phrase but had a higher ATE 3, indicating greater acute toxicity. These marinone derivatives were also identified as developmental toxicants with low bioaccumulation and as having negative mutagenicity. The compounds suggest not having higher toxicity than several approved drugs and antifouling agents and are less harmful than copper or arsenic. In vivo ecotoxicity studies emphasize the potential of compounds (1) and (2) as eco-friendly antibiofilm and antifouling agents.
Data availability statement
The datasets presented in this study can be found in online repositories. The names of the repository/repositories and accession number(s) can be found in the article/Supplementary Material.
Ethics statement
CIIMAR has a dedicated animal facility (Bioterium of Aquatic Organisms–BOGA) certified by “Direção Geral de Alimentação e Veterinária (DGAV)” issued under Article 21°, of Decree-Law N° 113/2013 of 7th August. All experimentation performed at BOGA is subject to an ethical review process by CIIMAR’s Ethical Committee along with the CIIMAR´S Animal Welfare Body (ORBEA) in compliance with the European Directive 2010/63/EU, on the protection of animals used for scientific purposes, and its transposition to the Portuguese law. A “Laboratory Animal Sciences” (LAS) Education and/or a DGAV certification is mandatory for researchers to perform animal experimentation if involving vertebrate and cephalopod species.
Author contributions
JW: Data curation, Formal Analysis, Investigation, Visualization, Writing–original draft, Writing–review and editing. JA: Data curation, Formal Analysis, Investigation, Visualization, Writing–review and editing. IG: Data curation, Formal Analysis, Investigation, Visualization, Writing–review and editing. JO: Formal Analysis, Investigation, Visualization, Writing–original draft. CB: Formal Analysis, Investigation, Visualization, Writing–original draft. WE-H: Data curation, Formal Analysis, Investigation, Visualization, Writing–review and editing. PP: Formal Analysis, Investigation, Visualization, Writing–original draft, Writing–review and editing. MV: Data curation, Methodology, Supervision, Formal Analysis, Validation, Visualization, Writing–original draft, Writing–review and editing. IC: Data curation, Formal Analysis, Investigation, Project administration, Supervision, Validation, Visualization, Writing–review and editing. RS: Data curation, Funding acquisition, Methodology, Project administration, Supervision, Validation, Visualization, Writing–review and editing. VV: Funding acquisition, Methodology, Project administration, Supervision, Validation, Visualization, Writing–review and editing. SG: Conceptualization, Data curation, Formal Analysis, Funding acquisition, Methodology, Project administration, Resources, Software, Supervision, Validation, Visualization, Writing–original draft, Writing–review and editing.
Funding
The author(s) declare that financial support was received for the research, authorship, and/or publication of this article. This work was financed by national funds from FCT—Fundação para a Ciência e a Tecnologia, IP, in the scope of the project UIDP/04378/2020 of the Research Unit on Applied Molecular Biosciences–UCIBIO and the project LA/P/0140/2020 of the Associate Laboratory Institute for Health and Bioeconomy. Funding from the 7th Framework Programme (FP7/2007–2013) under grant agreement PCOFUND-GA-2009-246542, DFRH/WIIA/102/2011 and SFRH/BI/52130/2013. Financial support provided by FCT/MCTES through grants IF/00700/2014 and SFRH/BPD/110020/2015. Funding also provided by the projects PTDC/QUI-QUI/119116/2010, PTDC/BIA-MIC/31645/2017, and PTDC/BTA-GES/32359/2017, financed by FCT/MCTES, COMPETE2020 and PORTUGAL 2020. The NMR spectrometers are part of The National NMR Facility, supported by FCT (RECI/BBB-BQB/0230/2012). We acknowledge the Laboratório de Análises/REQUIMTE/LAQV for the acquisition of the elemental analysis data. JW was supported in the form of a postdoctoral fellowship by UNAM-DGAPA, and JRA by the research contract under the Scientific Employment Stimulus Individual Call (2022.03876.CEECIND/CP1728/CT0005; https://doi.org/10.54499/2022.03876.CEECIND/CP1728/. This research was also supported by the Innovation Pact, Project No. C644915664-00000026 (WP2 Vertical Bivalves), under the “Blue Bioeconomy Pact”, resulting from the submission of the application to Notice No. 02/C05-i01/2022, within the scope of the Recovery and Resilience Plan (PRR), co-funded by the Portuguese Republic and the European Union.
Acknowledgments
SPG expresses gratitude to W. Fenical, P. R. Jensen, and C. A. Kauffman of Scripps Institution of Oceanography, San Diego, United States, for their generous support in facilitating the collection of sediment samples. Special thanks to P. Castilho of Madeira University and M. Freitas of Funchal Marine Biology Station for their warm hospitality and logistical assistance during the field expedition. CIIMAR acknowledges FCT (Foundation for Science and Technology) for the strategic funds within the scope of UIDB/04423/2020 and UIDP/04423/2020. CT0005). IG acknowledges the STSM grant funded by COST Action CA18238 (Ocean4Biotech).
Conflict of interest
The authors declare that the research was conducted in the absence of any commercial or financial relationships that could be construed as a potential conflict of interest.
The author(s) declared that they were an editorial board member of Frontiers, at the time of submission. This had no impact on the peer review process and the final decision.
The handling editor LP declared a past co-authorship with the author JA.
Publisher’s note
All claims expressed in this article are solely those of the authors and do not necessarily represent those of their affiliated organizations, or those of the publisher, the editors and the reviewers. Any product that may be evaluated in this article, or claim that may be made by its manufacturer, is not guaranteed or endorsed by the publisher.
Supplementary material
The Supplementary Material for this article can be found online at: https://www.frontiersin.org/articles/10.3389/fchem.2024.1425953/full#supplementary-material
References
Ahmad, I., Husain, F. M., Maheshwari, M., and Zahin, M. (2014). “Medicinal plants and phytocompounds: a potential source of novel antibiofilm agents,” in Antibiofilm Agents. Springer Science & Business Media, 205–232. doi:10.1007/978-3-642-53833-9_10
Akesso, L., Pettitt, M. E., Callow, J. A., Callow, M. E., Stallard, J., Teer, D., et al. (2009). The potential of nano-structured silicon oxide type coatings deposited by PACVD for control of aquatic biofouling. Biofouling 25, 55–67. doi:10.1080/08927010802444275
Almeida, J. R., Correia-Da-Silva, M., Sousa, E., Antunes, J., Pinto, M., Vasconcelos, V., et al. (2017). Antifouling potential of Nature-inspired sulfated compounds. Sci. Rep. 7, 42424. doi:10.1038/srep42424
Almeida, J. R., Palmeira, A., Campos, A., Cunha, I., Freitas, M., Felpeto, A. B., et al. (2020). Structure-antifouling activity relationship and molecular targets of bio-inspired (Thio)xanthones. Biomolecules 10, 1–17. doi:10.3390/biom10081126
Almeida, J. R., and Vasconcelos, V. (2015). Natural antifouling compounds: effectiveness in preventing invertebrate settlement and adhesion. Biotechnol. Adv. 33, 343–357. doi:10.1016/j.biotechadv.2015.01.013
Altschul, S. F., Gish, W., Miller, W., Myers, E. W., and Lipman, D. J. (1990). Basic local alignment search tool. J. Mol. Biol. 215, 403–410. doi:10.1016/S0022-2836(05)80360-2
Antunes, J., Pereira, S., Ribeiro, T., Plowman, J. E., Thomas, A., Clerens, S., et al. (2019). A multi-bioassay integrated approach to assess the antifouling potential of the cyanobacterial metabolites portoamides. Mar. Drugs 17, 111. doi:10.3390/md17020111
Bauermeister, A., Pereira, F., Grilo, I. R., Godinho, C. C., Paulino, M., Almeida, V., et al. (2019). Intra-clade metabolomic profiling of MAR4 Streptomyces from the Macaronesia Atlantic region reveals a source of anti-biofilm metabolites. Environ. Microbiol. 21, 1099–1112. doi:10.1111/1462-2920.14529
Bérdy, J. (2005). Bioactive microbial metabolites. J. Antibiot. (Tokyo) 58, 1–26. doi:10.1038/ja.2005.1
Briand, J. F. (2009). Marine antifouling laboratory bioassays: an overview of their diversity. Biofouling 25, 297–311. doi:10.1080/08927010902745316
Callow, J. A., and Callow, M. E. (2011). Trends in the development of environmentally friendly fouling-resistant marine coatings. Nat. Commun. 2, 244. doi:10.1038/ncomms1251
CCTE, EPA (2022). Toxicity Estimation Software Tool (TEST). The United States Environmental Protection Agency’s Center for Computational Toxicology and Exposure. Software. doi:10.23645/epacomptox.21379365.v3
Cho, J. Y. (2012). Glycoglycerolipids isolated from marine derived Streptomyces coelescens PK206-15. Biosci. Biotechnol. Biochem. 76, 1746–1751. doi:10.1271/bbb.120354
Cho, J. Y., Kang, J. Y., Hong, Y. K., Baek, H. H., Shin, H. W., and Kim, M. S. (2012). Isolation and structural determination of the antifouling diketopiperazines from marine-derived Streptomyces praecox 291-11. Biosci. Biotechnol. Biochem. 76, 1116–1121. doi:10.1271/bbb.110943
Cho, J. Y., and Kim, M. S. (2012). Induction of antifouling diterpene production by Streptomyces cinnabarinus PK209 in Co-culture with marine-derived Alteromonas sp. KNS-16. Biosci. Biotechnol. Biochem. 76, 1849–1854. doi:10.1271/bbb.120221
Conrad, J. C., and Poling-Skutvik, R. (2018). Confined flow: consequences and implications for bacteria and biofilms. Annu. Rev. Chem. Biomol. Eng. 9, 175–200. doi:10.1146/annurev-chembioeng-060817-084006
Dang, H., Li, T., Chen, M., and Huang, G. (2008). Cross-ocean distribution of Rhodobacterales bacteria as primary surface colonizers in temperate coastal marine waters. Appl. Environ. Microbiol. 74, 52–60. doi:10.1128/AEM.01400-07
Davies, I. M., McHenery, J. G., and Rae, G. H. (1997). Environmental risk from dissolved ivermectin to marine organisms. Aquaculture 158, 263–275. doi:10.1016/S0044-8486(97)00209-3
Diaza, R. G., Manganelli, S., Esposito, A., Roncaglioni, A., Manganaro, A., and Benfenati, E. (2015). Comparison of in silico tools for evaluating rat oral acute toxicity. Sar. QSAR Environ. Res. 26, 1–27. doi:10.1080/1062936X.2014.977819
D’Souza, F., Bruin, A., Biersteker, R., Donnelly, G., Klijnstra, J., Rentrop, C., et al. (2010). Bacterial assay for the rapid assessment of antifouling and fouling release properties of coatings and materials. J. Ind. Microbiol. Biotechnol. 37, 363–370. doi:10.1007/s10295-009-0681-1
Ekblad, T., Bergström, G., Ederth, T., Conlan, S. L., Mutton, R., Clare, A. S., et al. (2008). Poly(ethylene glycol)-containing hydrogel surfaces for antifouling applications in marine and freshwater environments. Biomacromolecules 9, 2775–2783. doi:10.1021/bm800547m
El-Masry, M. H., Hassona, M. S., El-Rakshy, N., and Mousa, I. E.-S. (1995). Bacterial populations in the biofilm and non-biofilm components of a sand filter used in water treatment. FEMS Microbiol. Lett. 131, 263–269. doi:10.1016/0378-1097(95)00266-8
Gallagher, K. A., Fenical, W., and Jensen, P. R. (2010). Hybrid isoprenoid secondary metabolite production in terrestrial and marine actinomycetes. Curr. Opin. Biotechnol. 21, 794–800. doi:10.1016/j.copbio.2010.09.010
Gallagher, K. A., and Jensen, P. R. (2015). Genomic insights into the evolution of hybrid isoprenoid biosynthetic gene clusters in the MAR4 marine streptomycete clade. BMC Genomics 16, 960. doi:10.1186/s12864-015-2110-3
Gallagher, K. A., Rauscher, K., Ioca, L. P., and Jensen, P. R. (2013). Phylogenetic and chemical diversity of a hybrid-isoprenoid- producing streptomycete lineage. Appl. Environ. Microbiol. 79, 6894–6902. doi:10.1128/AEM.01814-13
Gaudêncio, S. P., and Pereira, F. (2022). Predicting antifouling activity and acetylcholinesterase inhibition of marine-derived compounds using a computer-aided drug design approach. Mar. Drugs 20, 129. doi:10.3390/md20020129
Gaudêncio, S. P., Sobral, R. G., Pereira, F., Santos-Sanches, I., Gonçalves, S., Vasconcelos, V., et al. (2016). Use of marine natural products in antifouling paints and coatings.
Gini, G., Franchi, A. M., Manganaro, A., Golbamaki, A., and Benfenati, E. (2014). ToxRead: a tool to assist in read across and its use to assess mutagenicity of chemicals. Sar. QSAR Environ. Res. 25, 999–1011. doi:10.1080/1062936X.2014.976267
Gontang, E. A., Fenical, W., and Jensen, P. R. (2007). Phylogenetic diversity of gram-positive bacteria cultured from marine sediments. Appl. Environ. Microbiol. 73, 3272–3282. doi:10.1128/AEM.02811-06
Gopikrishnan, V., Radhakrishnan, M., Shanmugasundaram, T., Pazhanimurugan, R., and Balagurunathan, R. (2016). Antibiofouling potential of quercetin compound from marine-derived actinobacterium, Streptomyces fradiae PE7 and its characterization. Environ. Sci. Pollut. Res. 23, 13832–13842. doi:10.1007/s11356-016-6532-5
Hardt, I. H., Jensen, P. R., and Fenical, W. (2000). Neomarinone, and new cytotoxic marinone derivatives, produced by a marine filamentous bacterium (actinomycetales). Tetrahedron Lett. 41, 2073–2076. doi:10.1016/S0040-4039(00)00117-9
Inbakandan, D., Murthy, P. S., Venkatesan, R., and Khan, S. A. (2010). 16S rDNA sequence analysis of culturable marine biofilm forming bacteria from a ship’s hull. Biofouling 26, 893–899. doi:10.1080/08927014.2010.530347
Kalaitzis, J. A., Hamano, Y., Nilsen, G., and Moore, B. S. (2003). Biosynthesis and structural revision of neomarinone. Org. Lett. 5, 4449–4452. doi:10.1021/ol035748b
Kavitha, S., and Vimala, R. (2020). Screening of marine Actinomycetes for inhibitory activity against biofilm forming bacteria. J. Environ. Biol. 41, 995–1002. doi:10.22438/jeb/41/5/MRN-1215
Kawasaki, T., Hayashi, Y., Kuzuyama, T., Furihata, K., Itoh, N., Seto, H., et al. (2006). Biosynthesis of a natural polyketide-isoprenoid hybrid compound, furaquinocin A: identification and heterologous expression of the gene cluster. J. Bacteriol. 188, 1236–1244. doi:10.1128/JB.188.4.1236-1244.2006
Kirschner, C. M., and Brennan, A. B. (2012). Bio-Inspired antifouling strategies. Annu. Rev. Mater Res. 42, 211–229. doi:10.1146/annurev-matsci-070511-155012
Kwasny, S. M., and Opperman, T. J. (2010). Static biofilm cultures of Gram-positive pathogens grown in a microtiter format used for anti-biofilm drug discovery. Curr. Protoc. Pharmacol. 50, Unit 13A.8. doi:10.1002/0471141755.ph13a08s50
Mabrouk, S. B., Reis, M., Sousa, M. L., Ribeiro, T., Almeida, J. R., Pereira, S., et al. (2020). The marine seagrass Halophila stipulacea as a source of bioactive metabolites against obesity and biofouling. Mar. Drugs 18, 88. doi:10.3390/md18020088
Magin, C. M., Cooper, S. P., and Brennan, A. B. (2010). Non-toxic antifouling strategies. Mater. Today 13, 36–44. doi:10.1016/S1369-7021(10)70058-4
Majzoub, M. E., McElroy, K., Maczka, M., Thomas, T., and Egan, S. (2018). Causes and consequences of a variant strain of Phaeobacter inhibens with reduced competition. Front. Microbiol. 9, 2601. doi:10.3389/fmicb.2018.02601
Michael, V., Frank, O., Bartling, P., Scheuner, C., Göker, M., Brinkmann, H., et al. (2016). Biofilm plasmids with a rhamnose operon are widely distributed determinants of the “swim-or-stick” lifestyle in roseobacters. ISME J. 10, 2498–2513. doi:10.1038/ismej.2016.30
Morgan, R. N., Ali, A.Al, Alshahrani, M. Y., and Aboshanab, K. M. (2023). New insights on biological activities, chemical compositions, and classifications of marine actinomycetes antifouling agents. Microorganisms 11, 2444. doi:10.3390/microorganisms11102444
Murray, L. A. M., McKinnie, S. M. K., Moore, B. S., and George, J. H. (2020). Meroterpenoid natural products from: streptomyces bacteria-the evolution of chemoenzymatic syntheses. Nat. Prod. Rep. 37, 1334–1366. doi:10.1039/d0np00018c
Neves, A. R., Pereira, D., Gonçalves, C., Cardoso, J., Pinto, E., Vasconcelos, V., et al. (2021). Natural benzo/acetophenones as leads for new synthetic acetophenone hybrids containing a 1,2,3-triazole ring as potential antifouling agents. Mar. Drugs 19, 682. doi:10.3390/md19120682
Olano, C., Lombó, F., Méndez, C., and Salas, J. A. (2008). Improving production of bioactive secondary metabolites in actinomycetes by metabolic engineering. Metab. Eng. 10, 281–292. doi:10.1016/j.ymben.2008.07.001
Pathirana, C., Jensen, P. R., and Fenical, W. (1992). Marinone and debromomarinone: antibiotic sesquiterpenoid naphthoquinones of a new structure class from a marine bacterium. Tetrahedron Lett. 33, 7663–7666. doi:10.1016/0040-4039(93)88010-G
Peña-López, M., Martínez, M. M., Sarandeses, L. A., and Sestelo, J. P. (2009). Total synthesis of (+)-neomarinone. Chem. - A Eur. J. 15, 910–916. doi:10.1002/chem.200802021
Pereira, D., Gonçalves, C., Martins, B. T., Palmeira, A., Vasconcelos, V., Pinto, M., et al. (2021). Flavonoid glycosides with a triazole moiety for marine antifouling applications: synthesis and biological activity evaluation. Mar. Drugs 19, 5. doi:10.3390/md19010005
Pereira, F., Almeida, J. R., Paulino, M., Grilo, I. R., Macedo, H., Cunha, I., et al. (2020). Antifouling napyradiomycins from marine-derived actinomycetes Streptomyces aculeolatus. Mar. Drugs 18, 63. doi:10.3390/md18010063
Pinori, E., Berglin, M., Brive, L. M., Hulander, M., Dahlström, M., and Elwing, H. (2011). Multi-seasonal barnacle (Balanus improvisus) protection achieved by trace amounts of a macrocyclic lactone (ivermectin) included in rosin-based coatings. Biofouling 27, 941–953. doi:10.1080/08927014.2011.616636
Pinto-Almeida, A., Bauermeister, A., Luppino, L., Grilo, I. R., Oliveira, J., Sousa, J. R., et al. (2022). The diversity, metabolomics profiling, and the pharmacological potential of actinomycetes isolated from the estremadura spur pockmarks (Portugal). Mar. Drugs 20, 21. doi:10.3390/md20010021
Prakash, S., Ramasubburayan, R., Iyapparaj, P., Ramaswamy Arthi, A. P., Ahila, N. K., Ramkumar, V. S., et al. (2015). Environmentally benign antifouling potentials of triterpene-glycosides from Streptomyces fradiae: a mangrove isolate. RSC Adv. 5, 29524–29534. doi:10.1039/c4ra15335a
Prieto-Davó, A., Dias, T., Gomes, S. E., Rodrigues, S., Parera-Valadez, Y., Borralho, P. M., et al. (2016). The Madeira Archipelago as a significant source of marine-derived actinomycete diversity with anticancer and antimicrobial potential. Front. Microbiol. 7, 1594. doi:10.3389/fmicb.2016.01594
Qian, P. Y., Xu, Y., and Fusetani, N. (2009). Natural products as antifouling compounds: recent progress and future perspectives. Biofouling 26, 223–234. doi:10.1080/08927010903470815
Resende, D. I. S. P., Almeida, J. R., Pereira, S., Campos, A., Lemos, A., Plowman, J. E., et al. (2021). From natural xanthones to synthetic C-1 aminated 3,4-dioxygenated xanthones as optimized antifouling agents. Mar. Drugs 19, 638. doi:10.3390/md19110638
Rotter, A., Barbier, M., Bertoni, F., Bones, A. M., Cancela, M. L., Carlsson, J., et al. (2021). The essentials of marine biotechnology. Front. Mar. Sci. 8. doi:10.3389/fmars.2021.629629
Sabotič, J., Bayram, E., Ezra, D., Gaudêncio, S. P., Haznedaroğlu, B. Z., Janež, N., et al. (2024). A guide to the use of bioassays in exploration of natural resources. Biotechnol. Adv. 71, 108307. doi:10.1016/j.biotechadv.2024.108307
Schipper, C. A., Rietjens, I. M. C. M., Burgess, R. M., and Murk, A. J. (2010). Application of bioassays in toxicological hazard, risk and impact assessments of dredged sediments. Mar. Pollut. Bull. 60, 2026–2042. doi:10.1016/j.marpolbul.2010.07.018
Schultz, M. P., Bendick, J. A., Holm, E. R., and Hertel, W. M. (2011). Economic impact of biofouling on a naval surface ship. Biofouling 27, 87–98. doi:10.1080/08927014.2010.542809
Selvin, J. (2009). Exploring the antagonistic producer streptomyces MSI051: implications of polyketide synthase gene type II and a ubiquitous defense enzyme phospholipase A2 in the host sponge Dendrilla nigra. Curr. Microbiol. 58, 459–463. doi:10.1007/s00284-008-9343-1
She, W., Ye, W., Cheng, A., Ye, W., Ma, C., Wang, R., et al. (2022). Discovery, yield improvement, and application in marine coatings of potent antifouling compounds albofungins targeting multiple fouling organisms. Front. Microbiol. 13, 906345. doi:10.3389/fmicb.2022.906345
Sonak, S., Pangam, P., Giriyan, A., and Hawaldar, K. (2009). Implications of the ban on organotins for protection of global coastal and marine ecology. J. Environ. Manage 90, S96–S108. doi:10.1016/j.jenvman.2008.08.017
Stalin, K., Ravi, L., and Raghavan, V. (2022). Extraction, purification and structural elucidation of environmentally benign antifouling metabolite from Streptomyces thermolineatus VITKV6A. Environ. Technol. Innov. 25, 102096. doi:10.1016/j.eti.2021.102096
Sushmitha, T. J., Rajeev, M., Murthy, P. S., Rao, T. S., and Pandian, S. K. (2023). Planktonic and early-stage biofilm microbiota respond contrastingly to thermal discharge-created seawater warming. Ecotoxicol. Environ. Saf. 264, 115433. doi:10.1016/j.ecoenv.2023.115433
Tišler, T., and Zagorc-končan, J. (2003). Aquatic toxicity of selected chemicals as a basic criterion for environmental classification. Arh. Hig. Rada Toksikol. 54, 207–213.
Waturangi, D. E., Purwa Hariyanto, J., Lois, W., Hutagalung, R. A., and Hwang, J. K. (2017). Inhibition of marine biofouling by aquatic Actinobacteria and coral-associated marine bacteria. Malays J. Microbiol. 13, 92–99. doi:10.21161/mjm.86016
Xu, Y., He, H., Schulz, S., Liu, X., Fusetani, N., Xiong, H., et al. (2010). Potent antifouling compounds produced by marine Streptomyces. Bioresour. Technol. 101, 1331–1336. doi:10.1016/j.biortech.2009.09.046
Keywords: blue biotechnology, marine natural products, actinomycetes bioprospection, meroterpenoids, hybrid isoprenoids, marine biofilm and biofouling, antifouling. eco-friendly paints and coatings
Citation: Wissner JL, Almeida JR, Grilo IR, Oliveira JF, Brízida C, Escobedo-Hinojosa W, Pissaridou P, Vasquez MI, Cunha I, Sobral RG, Vasconcelos V and Gaudêncio SP (2024) Novel metabolite madeirone and neomarinone extracted from Streptomyces aculeoletus as marine antibiofilm and antifouling agents. Front. Chem. 12:1425953. doi: 10.3389/fchem.2024.1425953
Received: 30 April 2024; Accepted: 27 June 2024;
Published: 25 July 2024.
Edited by:
Lucia Panzella, University of Naples Federico II, ItalyReviewed by:
Lingli Liu, Northwest A&F University, ChinaValeria Costantino, University of Naples Federico II, Italy
Copyright © 2024 Wissner, Almeida, Grilo, Oliveira, Brízida, Escobedo-Hinojosa, Pissaridou, Vasquez, Cunha, Sobral, Vasconcelos and Gaudêncio. This is an open-access article distributed under the terms of the Creative Commons Attribution License (CC BY). The use, distribution or reproduction in other forums is permitted, provided the original author(s) and the copyright owner(s) are credited and that the original publication in this journal is cited, in accordance with accepted academic practice. No use, distribution or reproduction is permitted which does not comply with these terms.
*Correspondence: Susana P. Gaudêncio, cy5nYXVkZW5jaW9AZmN0LnVubC5wdA==