- Research Center for Applied Science and Technology, Tribhuvan University, Kathmandu, Nepal
The article discusses the promising synergy between MXenes and polymers in developing advanced nanocomposites with diverse applications in biomedicine domains. MXenes, possessing exceptional properties, are integrated into polymer matrices through various synthesis and fabrication methods. These nanocomposites find applications in drug delivery, imaging, diagnostics, and environmental remediation. They offer improved therapeutic efficacy and reduced side effects in drug delivery, enhanced sensitivity and specificity in imaging and diagnostics, and effectiveness in water purification and pollutant removal. The perspective also addresses challenges like biocompatibility and toxicity, while suggesting future research directions. In totality, it highlights the transformative potential of MXenes-polymer nanocomposites in addressing critical issues across various fields.
1 Introduction
MXenes are a family of two-dimensional (2D) transition metal carbides, nitrides, or carbonitrides that exhibit a unique combination of properties, making them highly versatile materials (Naguib et al., 2014). MXenes are derived from layered ternary carbide, nitride, or carbonitride precursors known as MAX phases. The structure involves selective etching (Ghidiu et al., 2014) of the “A” element layers (typically aluminum) from the MAX phase, leaving behind a 2D layered structure. The general formula for MXenes is Mn+1XnTx, where M represents a transition metal (e.g., titanium, tantalum, or niobium), X is carbon and/or nitrogen, T represents surface terminations (such as hydroxyl or fluorine), and n is the number of metal layers (Halim et al., 2014). Without the hydroxyl or oxygen termination, they are hydrophilic (Harris et al., 2015; Halim, Cook, et al., 2016). MXenes typically exhibit metallic conductivity, making them suitable for applications in electronics, sensors (Guo et al., 2019), and energy storage devices (Simon and Gogotsi, 2008; Meng et al., 2014; Kurra, Hota, and Alshareef, 2015). The presence of transition metal layers contributes to their excellent electronic conductivity. MXenes possess impressive mechanical properties, including high stiffness and strength, making them suitable for applications where mechanical integrity is crucial (Ling et al., 2014). MXenes are chemically stable in various environments, showing feasibility for oxidation and resistance to corrosion. MXenes and their oxides are promising topological materials that are experimentally and computationally studied (Parajuli and Samatha, 2022; 2019; Parajuli, Kaphle, and Samatha, 2019; Parajuli and Samatha, 2022; Parajuli, Uppugalla, et al., 2023) (S. Huang and Mochalin, 2019)(S. Huang and Mochalin, 2019). This stability contributes to their applicability in a wide range of conditions. The surface of MXenes is rich in functional groups, such as hydroxyl (OH-) and/or fluorine (F-) terminations, providing reactive sites for further functionalization. Surface functionalization enhances their compatibility with other materials and expands their application range (Harris et al., 2015; Halim, Cook, et al., 2016). MXenes typically have a high specific surface area due to their layered structure, which is advantageous for applications such as energy storage, catalysis, and sensing. MXenes exhibit interesting optical properties, including strong light absorption, which can be exploited in various optoelectronic and sensing applications. Their incorporation for energy storage were found more efficient that those we studied earlier (Parajuli, Murali, Samatha, and Veeraiah, 2022; Parajuli, Taddesse, Murali, Veeraiah, et al., 2022). MXenes demonstrate good thermal conductivity, making them promising candidates for applications in thermal management and electronic devices (Forrest, 2004; Y; Shi et al., 2015; Reese et al., 2004). The unique combination of electronic, mechanical, chemical, and optical properties makes MXenes a promising class of materials with diverse applications in fields ranging from electronics and energy storage to catalysis and biomedicine (Z. Liu et al., 2018; C; Dai, Chen, et al., 2017; Lin et al., 2018; X; Han et al., 2018) (C. Dai, Lin, et al., 2017).
The different types of MXenes derived from their precursors through their selective etching processes are shown in Figure 1A, it showcases three categories of mono-M MAX phases: M2AX, M3AX2, and M4AX3, along with the selective etching procedure targeting the A-group layers (depicted by red atoms). In Figure 1B, it follows the selective etching process, MXenes emerge, characterized by the formation of surface terminations (depicted by yellow atoms) denoted as T. Figure 1C presents the potential elements for M, A, X, and T in both MAX and MXene phases. Additionally, although MXenes with orders as high as M5X4 have been successfully synthesized, they are not included in this table. It’s noteworthy that while Mn occupies the M sites in MAX phases, MXenes containing Mn have not been synthesized to date, indicated by the horizontally patterned background of Mn.
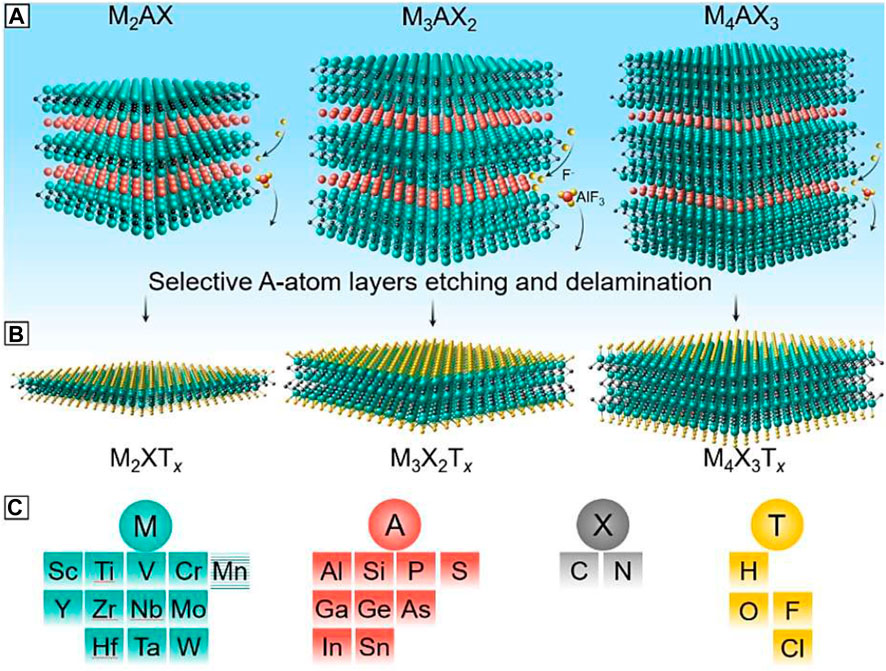
Figure 1. Different types of MXenes with different compositions with their precursor MAX phases. (A) MAX phases, (B) terminated MXenes after etching of MAX phases and (C) representation of M, A, X, and T. Adapted with the permission of (Hong et al., 2020)©Springer Nature 2020.
MXenes in addition to their exhibiting excellent electrical conductivity, mechanical strength, and chemical stability, often lack certain properties required for specific applications (Bilibana, 2023). By incorporating MXenes into different matrices such as polymers, ceramics, or metals, it can be exploited synergistic effects to achieve desired functionalities. For instance, MXene nanocomposites can offer improved mechanical strength, electrical conductivity, thermal stability, and even functionalities like electromagnetic interference shielding or energy storage (Gao et al., 2020). These nanocomposites find applications across various fields including energy storage devices like batteries and supercapacitors, electromagnetic shielding materials, sensors, catalysis, and biomedical devices. The versatility and tunability of MXene nanocomposites make them highly desirable for addressing specific needs in diverse technological domains (Perera et al., 2023). MXenes exhibit chemical stability, resisting oxidation and corrosion, contributing to the durability of nanocomposites in various environments (W. Wu et al., 2019a; M; Zhu et al., 2016b). MXenes can be synthesized with different transition metals, providing versatility in tailoring their properties for specific nanocomposite applications (Naguib et al., 2016).
In nanocomposite applications, MXenes are often combined with polymers, ceramics, or other materials to create hybrid materials with enhanced or multifunctional properties. The combination of these MXene properties allows for the development of advanced nanocomposites with diverse applications across various fields.
2 MXene, polymer, and their nanocomposites
MXene nanocomposites, combining the unique properties of MXenes with the versatility of polymers, offer enhanced mechanical, thermal, and electrical properties. These materials hold promise in applications such as flexible electronics and energy storage. Understanding fabrication techniques and interface engineering is crucial for tailoring MXene-polymer nanocomposites to specific needs. The MXene, Polymer, and their classification and nanocomposites are described briefly below.
2.1 MXenes
MXenes possess a distinctive set of properties that make them highly suitable for nanocomposite applications, particularly in the fields of biomedical science. MXenes have a unique 2D layered structure, providing a large surface area for interactions within nanocomposites. MXenes exhibit excellent electrical conductivity (Wang et al., 2019), making them advantageous for applications in electronics, sensors (Guo et al., 2019), and energy storage devices (George and Kandasubramanian, 2020). MXenes demonstrate impressive mechanical strength and stiffness, contributing to enhanced mechanical properties when incorporated into nanocomposites (Jimmy and Kandasubramanian, 2020). The surface of MXenes is rich in functional groups, such as hydroxyl and/or fluorine terminations, enabling easy surface modification and compatibility with other materials (Chen et al., 2020). MXenes typically possess a high specific surface area due to their layered structure, facilitating improved adsorption and reactivity in nanocomposite applications (Oyedotun et al., 2009). MXenes exhibit good thermal conductivity, making them suitable for applications in thermal management and devices requiring efficient heat dissipation. MXenes often demonstrate biocompatibility, a crucial property for their integration into nanocomposites for biomedical applications, such as drug delivery systems and medical implants. Some MXenes, like titanium carbide (Ti3C2Tx), possess excellent photothermal properties, making them useful in nanocomposites for applications like cancer therapy (Z. Liu et al., 2018; C; Dai, Chen, et al., 2017; Lin et al., 2018; X; Han et al., 2018; C; Dai, Lin, et al., 2017). MXenes are available in various compositions (carbides, nitrides, or carbonitrides), allowing for versatility in tailoring nanocomposites for specific applications. MXenes display interesting optical properties, including strong light absorption, which can be harnessed for applications in sensing and optoelectronics (McKeen, 2017).
2.1.1 Classification of MXenes on different basis
MXenes can be classified based on several different criteria, including composition, synthesis method, structural properties, and applications. The classification of MXenes based on various factors is listed in Table 1.
This classification scheme highlights the diverse nature of MXenes and their potential across multiple fields, from energy storage and electronics to catalysis, sensing, and biomedical applications depending also on the transitional metals.
2.2 Polymer
Polymers are large molecules composed of repeating structural units called monomers. These molecules are characterized by their long chains and versatile properties, making them essential in various industries and everyday products (Painter and Coleman, 2019). They can be natural, such as proteins and DNA, or synthetic, like plastics and rubbers. Synthetic polymers are typically created through polymerization processes, where monomers are chemically bonded together to form long chains or networks (McCrum, Buckley, and Bucknall, 1997). This process allows for the manipulation of properties like strength, flexibility, and durability, making polymers highly customizable for specific applications. Polymers play a vital role in modern society, being used in countless products ranging from packaging materials and textiles to medical devices and electronics. Their versatility, affordability, and ease of manufacturing have led to their widespread adoption across industries, driving innovation and technological advancement. However, concerns about environmental impact and sustainability have prompted efforts to develop biodegradable and eco-friendly alternatives to traditional polymers, reflecting the ongoing evolution of polymer science and engineering (Young and Lovell, 2008).
2.2.1 Classification of polymers
Polymers can be classified into several types based on their structure, origin, properties and applications. Some common types of polymers are listed in Table 2 (Young and Lovell, 2008).
2.3 MXene nanocomposites
MXene nanocomposites are a class of materials that have garnered significant attention due to their unique properties and potential applications. (Parajuli et al., 2022). MXene nanocomposites are formed by incorporating MXene nanosheets into a matrix material, such as polymers, ceramics, or metals, to enhance or impart specific properties. The addition of MXenes can improve mechanical strength, electrical conductivity, and thermal stability of the composite material. Research in MXene nanocomposites has demonstrated their potential in various fields (Aghamohammadi, Amousa, and Eslami-Farsani, 2021). For instance, MXene/polymer nanocomposites have shown promise in flexible electronics and sensors due to their excellent electrical conductivity and mechanical flexibility. In energy storage applications, MXene-based nanocomposites have been explored for supercapacitors and batteries, where they offer high specific capacitance and stability. One example of a study in MXene nanocomposites is the work by Liu et al. (2021), where they developed MXene/polymer nanocomposites for electromagnetic interference shielding applications. The study demonstrated that the incorporation of MXene nanosheets into the polymer matrix significantly enhanced the electromagnetic interference shielding effectiveness of the composite material (Zhang and Gu, 2022).
2.4 Polymer nanocomposites
Polymer nanocomposites are a class of materials composed of a polymer matrix reinforced with nanoscale fillers or additives. These nanofillers typically have at least one dimension in the nanometer range, providing unique properties and enhanced performance compared to traditional composite materials (Koo, 2019). The incorporation of nanofillers into polymers can result in significant improvements in mechanical, thermal, electrical, and barrier properties (Pinto et al., 2023). Common types of nanofillers used in polymer nanocomposites include nanoparticles such as metal oxides (e.g., silica, alumina), carbon-based materials (e.g., carbon nanotubes, graphene), and clay minerals (e.g., montmorillonite).
Polymer nanocomposites offer numerous advantages over conventional composites, including:
• Enhanced mechanical properties such as increased stiffness, strength, and toughness.
• Improved thermal stability and flame retardancy due to the barrier effect of nanofillers.
• Enhanced electrical conductivity or dielectric properties, making them suitable for electronic and electrical applications.
• Increased gas barrier properties, making them ideal for packaging materials.
• Reduced weight and improved fuel efficiency in transportation applications.
The fabrication of polymer nanocomposites involves various techniques such as melt blending, solution mixing, in situ polymerization, and electrospinning. The selection of nanofillers, their dispersion within the polymer matrix, and the processing conditions play crucial roles in determining the properties and performance of the nanocomposite material. Polymer nanocomposites find applications in diverse industries, including automotive, aerospace, electronics, packaging, biomedical, and energy storage. Ongoing research in this field aims to further optimize the properties of nanocomposites and explore novel applications, paving the way for the development of advanced materials with tailored properties and multifunctionality.
2.5 MXene-polymer nanocomposites
By integrating MXenes, renowned for their exceptional conductivity and mechanical properties, into polymer matrices, the resulting nanocomposites exhibit enhanced functionalities such as improved electrical conductivity, mechanical strength, and thermal stability (Gong et al., 2021). These tailored properties make MXene polymer nanocomposites highly promising for a wide array of applications, including flexible electronics, energy storage devices like batteries and supercapacitors, electromagnetic interference shielding materials, and even biomedical applications (Bilibana, 2023). Moreover, the versatility of polymers allows for easy processing and shaping, further expanding the potential applications and manufacturability of MXene polymer nanocomposites. The synthesis of MXene polymer nanocomposites represents a synergistic approach to harnessing the strengths of both materials, paving the way for advancements in numerous fields (Riazi et al., 2021).
From few years back, we have studied the MXenes and Ferrites (Parajuli, Raghavendra, et al., 2021; Parajuli, Murali, Rao, Ramakrishna, et al., 2022; Parajuli, Taddesse, Murali, and Samatha 2022; Parajuli, Murali, Raghavendra, et al., 2023; Parajuli, Murali, Samatha, et al., 2023) separately and their mixtures with the most exploited material in the society polymer in the form of their nanocomposites (Parajuli et al., 2022; Parajuli, Uppugalla, et al., 2023). We found them most efficient combination among the recently developed other nanocomposites which are far efficient than their pristine form.
Nanocomposites represent a cutting-edge technology with transformative potential in biomedical fields. Their ability to address specific challenges and provide tailored solutions underscores their significance in advancing healthcare and promoting environmental sustainability. The need for advanced materials like MXenes-polymer nanocomposites stems from their exceptional properties, versatility, and potential to address challenges in diverse applications, ranging from healthcare protection to energy storage and beyond. Their unique combination of attributes positions them as key players in advancing technological solutions for the future. In this article, the synthesis of MXene Polymer nanocomposites, their biomedical and environmental aspects along with the issues, challenges, and perspectives are discussed. MXenes properties for nanocomposite applications.
2.6 MXenes and polymers compatibility for nanocomposite
The compatibility of MXene with polymers is a crucial aspect in the formation of nanocomposites, determining the effectiveness and performance of the resulting hybrid materials (X. Chen et al., 2020). The compatibilities of MXene with polymers for nanocomposite formation are:
2.6.1 Surface functional groups
MXenes possess surface functional groups, such as hydroxyl (-OH) or fluorine (-F), which enhance their compatibility with polymers. These functional groups provide active sites for bonding and interactions with polymer chains.
2.6.2 Chemical affinity
The surface chemistry of MXene allows for strong interactions with various polymers. The presence of transition metal terminations and surface functional groups promotes chemical compatibility, ensuring a stable interface between MXene and polymer components.
2.6.3 Electrostatic interactions
The charged nature of MXene surfaces facilitates electrostatic interactions with polymers, especially those with opposite charges. This can contribute to improved dispersion and stability of MXene within the polymer matrix.
2.6.4 Enhanced mechanical properties
The mechanical strength of MXene, coupled with its compatibility with polymer matrices, results in nanocomposites with enhanced mechanical properties. This is particularly beneficial in applications requiring increased strength and durability.
2.6.5 Synergistic effects
The combination of MXene and polymers often leads to synergistic effects, where the unique properties of each component complement and enhance the overall performance of the nanocomposite. This synergy can result in improved conductivity, mechanical strength, or other desired characteristics.
2.6.6 Versatility of polymer selection
MXenes can be compatible with a wide range of polymers, including but not limited to, thermoplastics, thermosets, and elastomers. This versatility allows for the tailoring of nanocomposite properties to suit specific applications.
2.6.7 Improved processability
MXene’s compatibility with polymers facilitates the processing and fabrication of nanocomposites. Techniques such as solution mixing, melt blending, or in situ polymerization can be employed to achieve homogeneous dispersion and integration.
2.6.8 Biocompatibility
MXenes can be engineered to be biocompatible, making them suitable for integration into biopolymer matrices. This opens up opportunities for applications in biomedical nanocomposites, such as drug delivery systems and tissue engineering (K. Chen et al., 2017).
The compatibility of MXene with polymers is a critical factor in the successful formation of nanocomposites. The chemical affinity, surface functional groups, and synergistic effects contribute to creating hybrid materials with tailored properties, expanding the range of applications in various fields.
3 Synthesis of MXene-polymer nanocomposites
Synthesizing MXene-polymer nanocomposites involves various techniques, each with its advantages, limitations, and recent advancements. In most cases, the MXenes are synthesized from etching processes. The layered structures in colloidal solution form obtained from etching can be sonicated and delaminated to get a few layered MXenes (Carey and Barsoum, 2021) as shown in Figure 3. Their synthesis involves integrating MXene nanosheets into a polymer matrix to create materials with enhanced properties. The detail of the synthesis of MXene-Polymer nanocomposites is found in our recently published articles (Parajuli et al., 2022; Parajuli, Uppugalla, et al., 2023).
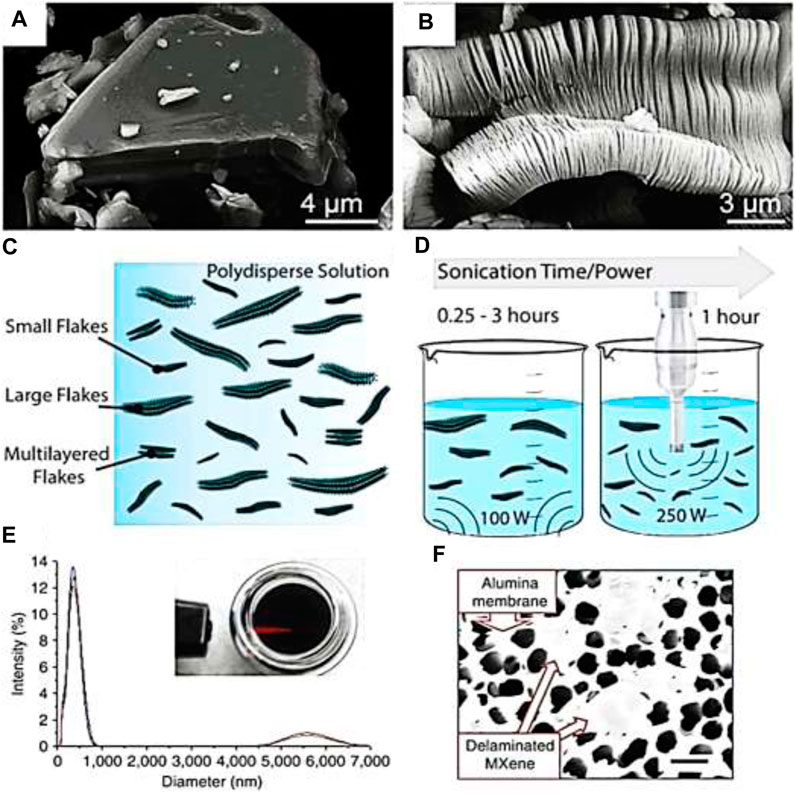
Figure 3. (A) Scanning electron microscopy (SEM) depiction of Ti3AlC2 particle (B) Formation of Ti3C2Tz after etching in hydrofluoric acid (HF). (C) Diagrammatic representation illustrating MXene suspension exhibiting various flake sizes. (D) Representation demonstrating the impact of sonication on dimensions of MXene flakes, with increasing sonication time and power. (E) Light scattering analysis of a typical colloidal MXene suspension post-sonication, accompanied by an inset image demonstrating the Tyndall effect. (F) SEM micrograph displaying drop-cast colloidal MXene on a porous alumina substrate, showcasing transparent delaminated MXene sheets. (A, B) are reproduced from Naguib et al., 2012. (C, D) are reproduced from Maleski et al., 2018. (E, F) are reproduced from Mashtalir et al., 2013.
3.1 Steps of synthesis methods
The synthesis typically involves the following steps.
1 Preparation of MXene Nanosheets: MXene nanosheets are synthesized through methods such as chemical etching of MAX phases or exfoliation of precursor materials. The resulting MXene nanosheets are typically functionalized to improve their dispersion and compatibility with the polymer matrix.
2 Preparation of Polymer Matrix: The polymer matrix is prepared separately, often by dissolving the polymer in a suitable solvent to form a polymer solution. The choice of polymer depends on the desired properties of the nanocomposite and the intended application.
3 Dispersion of MXene in Polymer solution: The MXene nanosheets are then dispersed in the polymer solution using techniques such as sonication or mechanical stirring. Proper dispersion is crucial to ensure uniform distribution of MXene within the polymer matrix and to prevent aggregation.
4 Fabrication of Nanocomposite: The dispersed MXene-polymer solution is then subjected to a suitable fabrication method to form the nanocomposite material. Common techniques include solution casting, spin-coating, or electrospinning, depending on the desired morphology and application of the nanocomposite.
5 Characterization and Optimization: The synthesized MXene-polymer nanocomposite is characterized to evaluate its structural, mechanical, electrical, and thermal properties. Optimization of synthesis parameters may be performed to achieve desired properties such as improved mechanical strength, conductivity, or thermal stability.
6 Application Testing: The MXene-polymer nanocomposite is then evaluated for its performance in specific applications such as energy storage, electromagnetic interference shielding, sensors, or biomedical devices. The nanocomposite’s properties are tailored to meet the requirements of the intended application.
The synthesis of MXene-polymer nanocomposites involves careful control of MXene dispersion, polymer compatibility, and fabrication techniques to achieve nanocomposites with enhanced properties suitable for various practical applications.
3.2 Types of synthesis methods
3.2.1 Selective etching
The synthesis of MXenes and hence the MXene Nanocomposites, involves a multistep process, primarily starting with the selective etching of layers from a precursor material known as a MAX phase. The etching of MXene typically refers to a process of selectively removing the “A” layer (typically aluminum) from the MXene structure, leaving behind a porous 2D material with various applications. The etching process is essential because it alters the surface chemistry and structure of MXene, allowing for tuning of its properties and enabling various applications. The etching of MXene can be used to control the surface chemistry, surface area, and interlayer spacing, which are crucial for applications such as energy storage devices (like batteries and supercapacitors), electromagnetic interference shielding, catalysis, sensors, and more. The steps for the synthesis of MXene Polymer nanocomposite by selective etching process are as below.
3.2.1.1 Selective etching
The primary step in MXene synthesis involves the selective etching of the A-element layers from the MAX phase. Common etchants include hydrofluoric acid (HF) or a combination of hydrochloric acid (HCl) and an oxidizing agent. The etching process exposes the MXene layers, creating a 2D structure (Naguib et al., 2012).
3.2.1.2 Washing and delamination
After etching, the resulting MXene flakes are thoroughly washed to remove residual etchants and by-products. This step is crucial for the removal of any remaining A-element residues. Delamination processes, such as intercalation of ions or molecules, may be employed to exfoliate MXene layers and enhance their dispersibility (Ling et al., 2014).
3.2.1.3 Surface functionalization
MXene surfaces often have terminations, such as hydroxyl (-OH) or fluorine (-F), which affect their properties. Surface functionalization can be performed to introduce desired functional groups, enhancing their compatibility with other materials or improving specific characteristics.
MXenes are frequently integrated into polymer matrices to form nanocomposites. This can be achieved through methods like solution mixing, melt blending, or in situ polymerization. The choice of polymer and the synthesis method can be tailored to achieve the desired properties for specific applications (Khazaei et al., 2013; Ashton et al., 2016; T; Hu et al., 2015).
3.2.1.4 Drying and powder formation
The resulting MXene dispersion is often dried to produce a powder form for ease of handling and storage. Techniques like freeze-drying or spray-drying are commonly employed to preserve the 2D structure.
3.2.1.5 MXene-polymer nanocomposite formation
The schematic representation of the synthesis cycle for the MXene-SP composite is illustrated in Figure 4. The bottom-up methodology can also be employed for MXene synthesis through techniques such as EVD (Manawi et al., 2018).
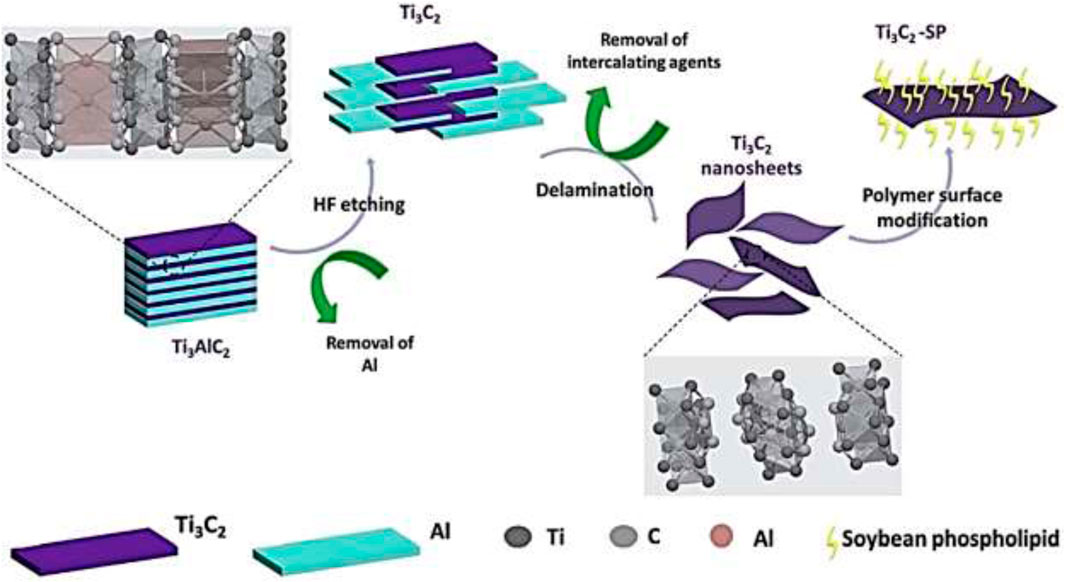
Figure 4. Synthesis cycle of Ti3C2-SP nanocomposite (Jain et al., 2013).
Figure 5 presents scanning transmission electron microscopy (STEM) images of Ti3AlC2 before and after etching, high-resolution transmission electron microscope (HRTEM) of Ti3C2 after etching, bright and dark selected area electron diffraction (SAED) of Nb2C, high angular annular dark field (HAADF) respectively, and showcasing hexagonally layered intercalated nanosheets with a space group of P63/mmc and atomic arrangement dependent on “n,” with lateral dimensions ranging from 0.5 to 200 nm and a thickness of a few nanometers (Naguib et al., 2014) (X. Ren et al., 2019).
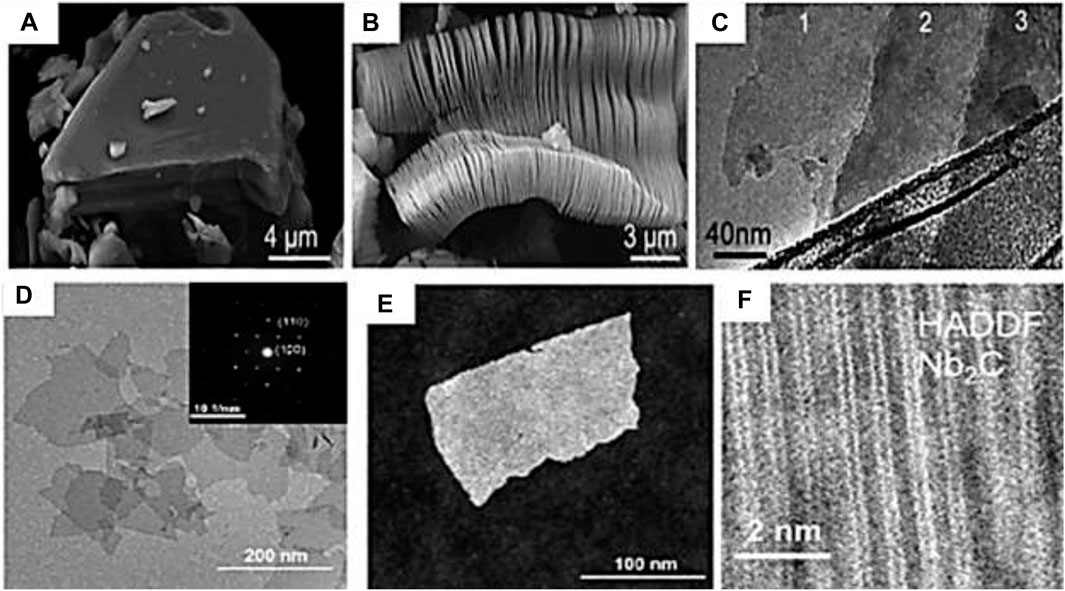
Figure 5. (A) SEM micrographs of Ti3AlC2 before and (B) after etching. (C) TEM images of Ti3C2 after etching (Naguib et al., 2012) © American Chemical Society (2012). (D, E) TEM images (Bright and dark field Nb2C). (F) High resolution HAADF-STEM image. © American Chemical Society (2019).
3.2.2 Solution mixing
They are simple and cost-effective, suitable for a wide range of polymers, and allow for precise control over the composition. However, they are limited to polymers soluble in the chosen solvent and may require additional steps for complete mixing and dispersion. Advanced mixing techniques, such as sonication or high-shear mixing, can be used to improve dispersion. Solution mixing is a common and straightforward method for preparing MXene-polymer nanocomposites (Maleski, Mochalin, and Gogotsi, 2017).
3.2.2.1 Material and method
The MXene of interest, such as titanium carbide (Ti3C2Tx) or others, is prepared through appropriate synthesis methods. The chosen polymer, compatible with the solvent is used for MXene dispersion. Common polymers include polyvinyl alcohol (PVA), polyethylene glycol (PEG), or others. A solvent in which both MXene and the polymer are soluble. Common solvents include water, N-methyl-2-pyrrolidone (NMP), or dimethyl sulfoxide (DMSO). A sonicator is needed to assist in dispersing MXene and polymer in the solvent. For continuous mixing during the preparation is done by magnetic stirrer or stirring rod. A suitable container is used for mixing and storing the nanocomposite solution.
The MXene is dispersed in the chosen solvent, with uniform dispersion often achieved through sonication. For example, a desired amount of MXene powder is added to the solvent, and the mixture is sonicated for a specific duration until a well-dispersed MXene solution is obtained. The polymer is dissolved in the same solvent used for MXene dispersion, with the solution stirred until the polymer is fully dissolved, forming a clear polymer solution. The MXene solution is slowly added into the polymer solution while continuously stirring, with this process carried out dropwise to ensure a gradual and homogeneous mixture. The combined solution is stirred for an extended period to ensure proper mixing and distribution of MXene within the polymer matrix. Depending on the desired concentration of MXene in the nanocomposite, the ratio of MXene to polymer solutions is adjusted, with this step potentially requiring optimization based on the specific application. The resulting nanocomposite solution is characterized to ensure the desired properties, with techniques such as UV-Vis spectroscopy, Fourier-transform infrared spectroscopy (FTIR), or other relevant methods employed. Depending on the application, the nanocomposite solution can be cast into films or coatings or dried to obtain the final nanocomposite material.
3.2.2.2 Considerations
In this process, some special considerations are needed. The concentration of MXene, polymer, and the ratio between them should be optimized based on the desired properties of the nanocomposite. The choice of solvent is critical, as it affects the solubility of both MXene and the polymer. Consideration should be given to environmental and safety aspects when selecting solvents. Proper sonication is essential to ensure a well-dispersed MXene solution, preventing agglomeration. Sufficient stirring time is crucial for achieving a homogeneous nanocomposite solution.
3.2.3 In-situ polymerization
They are a direct synthesis of nanocomposites during polymerization and have good control over the nanocomposite structure. They are suitable for a variety of polymer matrices. However, they are limited to polymers that can undergo in situ polymerization, and reaction conditions may affect MXene properties. The development of controlled polymerization methods for better control over nanocomposite structure. In situ polymerization is a method for preparing MXene-polymer nanocomposites in which the polymerization of monomers occurs directly in the presence of dispersed MXene sheets.
Due to limited polymerization energy, only certain types of monomers can undergo polymerization on the surface of MXenes through methods such as physical agitation, UV radiation, or electrochemical processes (C. Chen et al., 2017) (J. Chen et al., 2014; Boota et al., 2016). Chen et al. proposed two primary electron transfer mechanisms during the charge-transfer-induced polymerization of 3, 4-ethylenedioxythiophene (EDOT) under agitation. In one mechanism, electrons transition from the high-energy orbits (HOMOs) of the monomer to the lower-energy orbits (LUMOs) of the MXene, while in the other, electrons shift from the HOMOs of the MXene to the LUMOs of the monomer (Figure 6A) (C. Chen et al., 2017). Additionally, proximity between the monomer and MXene surface is crucial for efficient electron transfer during polymerization. Leveraging hydroxyl and fluorine terminations, 2-(dimethylamino) ethyl methacrylate (DMAEMA) was successfully polymerized on the surface of V2C (Figure 6B) (J. Chen et al., 2014), leading to the formation of PDMAEMA grafted V2C with demonstrated sensitivity to CO2 and temperature. Furthermore, MXene/polypyrrole (PPy) composites were synthesized via electrochemical polymerization by Zhu et al., involving the electrolysis of a pyrrole-containing solution with a Ti3C2-modified electrode. Although in situ polymerization ensures a uniform distribution of polymers on the MXene surface, it may alter the properties of MXene materials during the process.
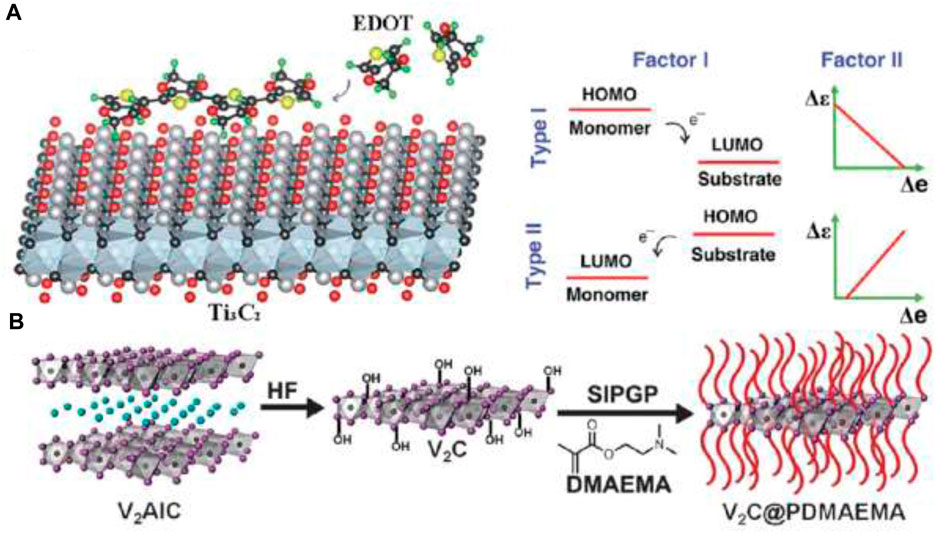
Figure 6. Synthesis of MXene/polymer composites through in situ polymerization: (A) Schematic depiction of the polymerization of EDOT on the surface of MXene and the process of charge transfer during polymerization, adapted from (C. Chen et al., 2017) ©2017 Royal Society of Chemistry and (B) Schematic illustration of the preparation of V2C@PDMAEMA composite. (A): Adapted with permission from reference (J. Chen et al., 2014) ©2015 Royal Society of Chemistry.
3.2.3.1 Material and method
In this method, the monomers are required for polymerization to make nanocomposite with the MXene of interest, such as titanium carbide (Ti3C2Tx). Common monomers include acrylic acid, acrylamide, or other suitable monomers compatible with the polymerization conditions. A suitable initiator to initiate the polymerization reaction. Common initiators include ammonium persulfate (APS), azobisisobutyronitrile (AIBN), or others, depending on the polymerization mechanism. A solvent in which both MXene and the monomers are dispersible. Common solvents include water, N-methyl-2-pyrrolidone (NMP), or dimethyl sulfoxide (DMSO). A suitable reaction vessel equipped with a stirring mechanism and temperature control is needed.
The MXene is dispersed in the chosen solvent to achieve a stable MXene dispersion, potentially involving sonication for uniform dispersion. The desired monomers are dissolved in the solvent to prepare the monomer solution, with the concentration adjusted based on the nanocomposite (NC) properties. e.g., epoxies (Sliozberg et al., 2020; H; Zhang et al., 2016; Zou et al., 2018) and polydimethylsiloxane (PDMS) like polymers are mixed from one side.
The most common reports of insitu polymerizations for MXene polymer NCs are of curing systems, such as epoxies (Hatter et al., 2020; Sliozberg et al., 2020) (A. Feng et al., 2020) and PDMS (D. Hu et al., 2020) (X. Wu et al., 2020)(D. Wang et al., 2020) aqueous solutions have been reported in the case of PPy (Zhu et al., 2016a; Boota et al., 2016; Zhang et al., 2020), polyaniline (Vahidmohammadi et al., 2018; Y; Ren et al., 2018; Wei et al., 2019; Vahidmohammadi et al., 2018; Xu et al., 2020), PAM(Zhang et al., 2019), PAM/PVA (Liao et al., 2019) and PEDOT (Chen et al., 2017; Qin et al., 2019), that can be polymerized with acetonitrile (Li et al., 2019).
The initiator is added to the monomer solution, its amount is determined by polymerization kinetics and reaction rate preferences. The MXene dispersion is slowly added into the monomer solution while continuously stirring to uniformly disperse MXene sheets. Polymerization is initiated by raising the temperature or other suitable methods activating the initiator. The polymerization reaction proceeds for the specified duration under controlled conditions. The resulting MXene-polymer nanocomposite is characterized using techniques such as FTIR, SEM, or other relevant methods. The nanocomposite material is isolated from the reaction mixture through filtration or other separation techniques and washed to remove unreacted monomers or by-products. Finally, the nanocomposite material is dried to obtain the final product.
3.2.3.2 Considerations
The polymerization conditions, including temperature, initiator concentration, and reaction time, to achieve the desired properties of the nanocomposite are optimized. Continuous stirring are ensured during the polymerization reaction to promote uniform dispersion of MXene sheets and facilitate homogeneous polymerization. Thorough characterization of the nanocomposite material are done to evaluate its structure, morphology, and properties.
In-situ polymerization offers precise control over the nanocomposite structure and properties, making it a valuable method for preparing MXene-polymer nanocomposites with tailored characteristics for specific applications. Adjustments can be made to this general procedure based on the choice of monomers, initiators, or MXene types.
3.2.4 Melt blending
Melt blending is a commonly used method for preparing MXene-polymer nanocomposites, particularly when working with thermoplastic polymers (Figure 7). They are scalable and applicable to various polymer matrices, suitable for industrial-scale production, and are simple with cost-effective. However, they are limited to thermoplastic polymers and may require high processing temperatures. The optimization of processing parameters improves dispersion and mechanical properties (Sheng et al., 2019).
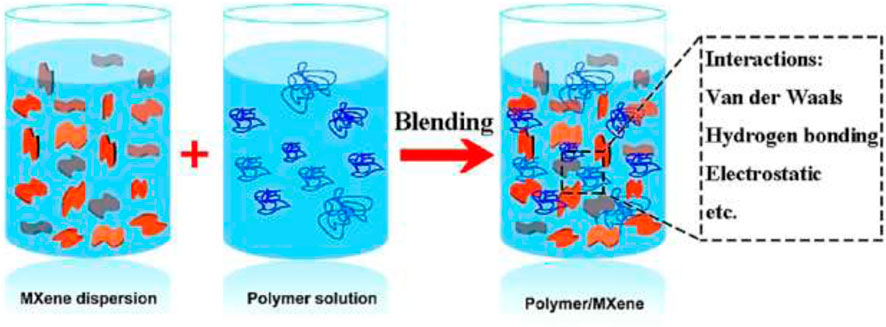
Figure 7. Creation of MXene/polymer composites through ex-situ solution blending. Reproduced with authorization from reference (Mirkhani et al., 2019). © 2019 American Chemical Society.
Ex-situ blending methods (Figure 7) are frequently utilized due to their ease in regulating the properties of polymer and MXene precursors. Leveraging their relatively modest interactions, such as van der Waals attraction, hydrogen bonding force, and electrostatic interaction, MXene/polymer composites are predominantly produced through solution mixing (Figure 7).
3.2.4.1 Material and method
The MXene of interest, such as titanium carbide (Ti3C2Tx) or others, is prepared through appropriate synthesis methods. The chosen thermoplastic polymer, is compatible with the processing temperature and MXene dispersion. A twin-screw extruder or internal mixer capable of high-temperature processing and efficient mixing. Additives such as compatibilizers, plasticizers, or stabilizers may be used to improve dispersion or enhance properties.
The MXene powder is properly dried to remove any residual moisture, as moisture can affect processing and properties. The thermoplastic polymer pellets are pre-dried to remove moisture and ensure uniform processing, with any desired additives optionally incorporated into the polymer pellets. The dried MXene powder and polymer pellets are loaded into the mixing equipment in the desired ratios, with the loading ratio adjusted based on the desired properties of the nanocomposite. The mixing equipment is heated to the appropriate processing temperature, typically above the melting point of the polymer. The mixing process is started, allowing the MXene powder and polymer pellets to melt and blend thoroughly, with high shear forces generated during mixing helping to disperse MXene within the polymer matrix. After thorough mixing, the molten mixture is extruded or discharged from the mixing equipment onto a cooling conveyor or casting surface. The mixture is allowed to cool and solidify, forming the MXene-polymer nanocomposite in the desired shape (e.g., pellets, sheets, or films). Further processing steps such as pelletizing, compression molding, or injection molding may be applied to the resulting nanocomposite material depending on the intended application. The nanocomposite material is characterized to evaluate its structure, morphology, and properties, with techniques such as SEM, XRD, or mechanical testing employed.
3.2.4.2 Consideration
The processing temperature should be carefully controlled to ensure proper melting of the polymer and dispersion of MXene while avoiding degradation of either component. Sufficient mixing time is essential to achieve uniform dispersion of MXene within the polymer matrix. Longer mixing times may be required for higher loading levels or less compatible polymer-MXene combinations. The incorporation of additives, such as compatibilizers or plasticizers, may improve the dispersion and compatibility of MXene within the polymer matrix. Melt blending involves high temperatures and mechanical shear forces. Proper safety precautions should be followed to prevent accidents or injuries.
Melt blending offers scalability and versatility, making it suitable for industrial-scale production of MXene-polymer nanocomposites. Adjustments to processing parameters can be made based on the specific polymer-MXene combination and intended application requirements.
3.2.5 Layer-by-layer assembly
This process has precise control over layer arrangement, suitable for a variety of polymers and MXenes. They can be used for thin films and coatings. However, the process is time-consuming and is limited to specific applications due to its layer-to-layer nature. Layer-by-layer (LbL) assembly (Figure 8) is a versatile technique for fabricating MXene-polymer nanocomposites with precise control over layer thickness and composition (Chen et al., 2020).
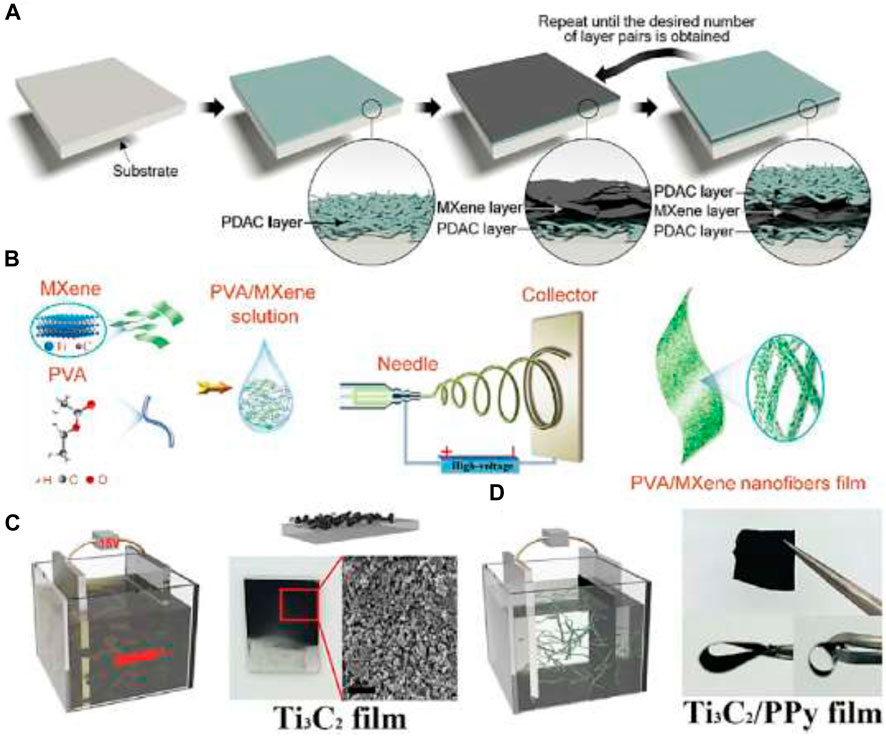
Figure 8. (A) Schematic illustration of the synthesis of MXene/PDAC membrane by LbL assembly process. (B) Schematic illustration of the fabrication process of MXene/PVA film by electrospinning approach. (C) Schematic illustration of the electrophoretic deposition of Ti3C2 flakes. (D) Schematic illustration of the synthesis of Ti3C2/PPy membrane by electrochemical polymerization of PPy on Ti3C2 film. Panel a: Reprinted from ref (An et al., 2018) ©2016, The Authors, (B): Reprinted with permission from ref (C. Jiang et al., 2019). ©2019 Elsevier. Panels c and d: Reprinted with permission from ref (M. Zhu et al., 2016a). © 2016 John Wiley and Sons. A step-by-step guide for the LbL assembly is as.
3.2.5.1 Material and method
The MXene of interest, such as titanium carbide (Ti3C2Tx) or others, is prepared through appropriate synthesis methods. The chosen polymer, typically in the form of a solution or dispersion compatible with the assembly process. Polyelectrolytes like poly (allylamine hydrochloride) (PAH) or poly (sodium 4-styrenesulfonate) (PSS) are commonly used. A solvent suitable for both MXene dispersion and polymer dissolution. Common solvents include water, ethanol, or other polar solvents. A solid substrate on which the LbL assembly will take place. Common substrates include silicon wafers, glass slides, or functionalized surfaces. Additives such as crosslinkers or stabilizers may be used to enhance film stability or modify properties.
The MXene is dispersed in the chosen solvent to create a stable MXene dispersion, with sonication or high-shear mixing possibly utilized to achieve uniform dispersion. The chosen polymer is dissolved in the solvent to generate a polymer solution or dispersion, with the polymer concentration adjustable based on desired film properties. The substrate is thoroughly cleaned to remove any contaminants or residues that may affect film formation, and optionally, the substrate surface is functionalized to promote adhesion or enhance film stability. The process is repeated by immersing the substrate in the polymer solution, allowing the polymer to adsorb onto the MXene-coated substrate surface. The substrate is rinsed with solvent to eliminate any unbound polymer molecules and stabilize the polymer layer. Deposition of MXene and polymer layers is continued alternately until the desired number of layers is achieved, with control over the sequence and thickness of layers by adjusting deposition parameters. Post-treatment steps, such as crosslinking or annealing, may be performed to enhance film stability or modify properties. The assembled MXene-polymer nanocomposite film is characterized to evaluate its structure, morphology, and properties, with techniques such as atomic force microscopy (AFM), ellipsometry, or spectroscopic methods employed.
3.2.5.2 Consideration
The pH and ionic strength of the deposition solutions can influence layer deposition and film properties, so these parameters should be controlled and optimized. Parameters such as immersion time, solution concentration, and drying conditions can affect film thickness and quality and should be carefully optimized. The substrate material and surface properties can influence film adhesion, stability, and performance, so substrate selection should be based on the desired application. LbL assembly allows for precise control over film composition, enabling the incorporation of multiple materials and functionalities into the nanocomposite film.
Layer-by-layer assembly offers fine control over film thickness, composition, and functionality, making it a versatile technique for fabricating MXene-polymer nanocomposites with tailored properties for various applications. Optimization of deposition parameters and thorough characterization are essential for achieving desired film properties and performance.
3.2.6 Electrospinning
They produce nanofibrous structures for enhanced properties, are suitable for a variety of polymers, and enable the fabrication of nanocomposite mats (Boys, 1887). However, they require specialized equipment and are limited to certain polymers and MXenes. Integration of electrospinning with other techniques for multifunctional nanocomposites may overcome this difficulty. Electrospinning is a versatile and widely used method for fabricating nanofibrous structures, including MXene-polymer nanocomposites (Ziabicki, 1976).
3.2.6.1 Material and method
After the preparation of the MXene of interest, such as titanium carbide (Ti3C2Tx), through an appropriate synthesis method, a polymer is chosen which is typically in the form of a solution or melt suitable for electrospinning. Common polymers include polyvinyl alcohol (PVA), polyethylene oxide (PEO), or others. A solvent suitable for both MXene dispersion and polymer dissolution. Common solvents include water, dimethylformamide (DMF), or other polar solvents. A grounded collector, typically in the form of a rotating drum or flat plate, collects the electrospun fibers. A high voltage power supply capable of generating electric fields in the range of 1–30 kV. A syringe pump or similar device for controlled delivery of the polymer solution during electrospinning. A spinneret or needle with a fine tip, through which the polymer solution is extruded during electrospinning (D. Li and Xia, 2004).
The MXene is dispersed in the chosen solvent to create a stable MXene dispersion, with sonication or high-shear mixing possibly employed to achieve uniform dispersion. The chosen polymer is dissolved in the solvent to form a polymer solution, with the polymer concentration optimized for electrospinning, typically in the range of 5%–20% w/v. The electrospinning apparatus is set up in a controlled environment to prevent airflow disturbances, and the grounded collector is positioned at an appropriate distance (usually 10–20 cm) from the spinneret. The polymer solution is loaded into a syringe or reservoir connected to the spinneret, ensuring it is free of air bubbles to prevent disruption during electrospinning. A high voltage (typically in the range of 1–30 kV) is applied between the spinneret and the collector to generate an electric field, initiating the electrospinning process by dispensing the polymer solution through the spinneret at a controlled flow rate (typically in the range of 0.1–10 mL/h). The electric field induces a charge on the surface of the polymer solution droplet, leading to the formation of a Taylor cone and subsequent elongation into nanofibers. Simultaneously, the MXene dispersion is introduced into the polymer solution or applied directly onto the collector to incorporate MXene into the electrospun fibers. The electrospun fibers are collected onto the grounded collector, forming a nonwoven mat or membrane, with the rotating drum or flat plate possibly used to facilitate continuous fiber collection. Post-treatment steps, such as drying, crosslinking, or annealing, may be performed to enhance fiber stability or modify properties. The electrospun MXene-polymer nanocomposite fibers are characterized to evaluate their structure, morphology, and properties, with techniques such as SEM, transmission electron microscopy (TEM), or mechanical testing employed (Merritt et al., 2012; Keirouz et al., 2020).
3.2.6.2 Consideration
The polymer concentration and solvent composition are optimized to achieve the desired solution viscosity for electrospinning. Control electrospinning parameters such as voltage, flow rate, and distance between the spinneret and collector to obtain uniform and well-aligned fibers. Uniform dispersion of MXene is ensured within the polymer solution to facilitate its incorporation into the electrospun fibers and enhance their properties. Cautions are exercised when working with high voltages and organic solvents, and follow appropriate safety protocols to prevent accidents or injuries.
Electrospinning offers precise control over fiber diameter, morphology, and composition, making it a valuable technique for fabricating MXene-polymer nanocomposite fibers with tailored properties for various applications such as filtration, tissue engineering, and sensors. Optimization of electrospinning parameters and thorough characterization are essential for achieving desired fiber properties and performance.
3.2.7 Chemical vapor deposition
They allow for direct synthesis on substrates and have uniform coatings on various surfaces along with precise control over film thickness. However, they require specialized equipment and are limited to specific substrates. These days, the scalability is improved, and have control over the CVD process. Chemical vapor deposition (CVD) is a method commonly used for the synthesis of thin films or coatings of MXene-polymer nanocomposites on solid substrates (Shareef et al., 1995; Schropp et al., 2000; Hamzan et al., 2021).
3.2.7.1 Material and method
The MXene of interest, such as titanium carbide (Ti3C2Tx) or others, is prepared through appropriate synthesis methods. The chosen polymer precursor, typically in the form of a vapor or gas, will react or deposit onto the substrate surface. A solid substrate onto which the MXene-polymer nanocomposite film will be deposited. Common substrates include silicon wafers, glass slides, or functionalized surfaces. A CVD reactor is equipped with the necessary components for gas flow control, temperature control, and vacuum pumping. An inert carrier gas such as nitrogen or argon, is used to transport the polymer precursor vapor to the substrate. Additives such as catalysts or dopants may be used to modify film properties or promote deposition. The substrate is thoroughly cleaned to remove any contaminants or residues that may affect film deposition, with the substrate surface optionally functionalized to promote adhesion or enhance film properties. The MXene powder is loaded onto a substrate holder inside the CVD reactor, ensuring uniform dispersion of MXene particles on the substrate surface. The polymer precursor is prepared in the desired form for CVD deposition, which may involve heating the precursor to its vaporization temperature or introducing it as a gas into the CVD reactor. The CVD reactor is purged with an inert gas (e.g., nitrogen or argon) to remove air and moisture. The polymer precursor vapor or gas is introduced into the CVD reactor at the desired flow rate, and the substrate is heated to the appropriate temperature for polymer deposition, typically in the range of 100°C–300°C. The polymer precursor reacts or deposits onto the substrate surface, forming a thin film or coating. Alternatively, MXene can be simultaneously deposited with the polymer precursor by introducing MXene vapor or gas into the CVD reactor alongside the polymer precursor. Deposition parameters such as temperature, precursor flow rate, and deposition time are controlled to achieve the desired film thickness and properties. Post-treatment steps, such as annealing or surface modification, may be performed to enhance film properties or stability. The deposited MXene-polymer nanocomposite film is characterized to evaluate its structure, morphology, and properties, with techniques such as SEM, X-ray diffraction (XRD), or spectroscopic methods employed.
A novel method utilizing chemical vapor deposition (CVD) has been uncovered for the direct production of ultrathin MXene materials, offering a new avenue for MXene-based material fabrication (Gogotsi, 2015) (C. Xu et al., 2015; Geng et al., 2017). By employing methane as a carbon source over a Cu/Mo alloyed surface at temperatures surpassing 1085°C (Figures 9A–C) (Gogotsi, 2015) (C. Xu et al., 2015) large-area high-quality 2D ultrathin α-Mo2C crystals (−3 nm) were successfully synthesized. Control over crystal size and thickness was achieved through manipulation of experimental conditions, where growth time influenced lateral size while growth temperature affected nucleation density. The method demonstrated versatility in generating various crystal shapes, including triangles, rectangles, hexagons, octagons, nonagons, and dodecagons, all exhibiting hexagonal packing of Mo atoms. Furthermore, enabling the fabrication of ultrathin β-Mo2C nanosheets using a rapid and scalable synthesis approach involving MoO2 nanosheets as templates and Mo sources. Unlike 2D materials obtained from alternative methods, CVD-fabricated MXenes showcased fewer defects and terminations, facilitating comprehensive investigations into their intrinsic properties and domain boundaries’ effects. The exploration of bottom-up synthetic approaches is encouraged to prepare other types of monolayered MXenes with diverse functionalities, thereby enabling further investigations into their inherent electronic and optical properties (Gogotsi, 2015; Jeon et al., 2018; Sang et al., 2018)(L. Wang et al., 2016).
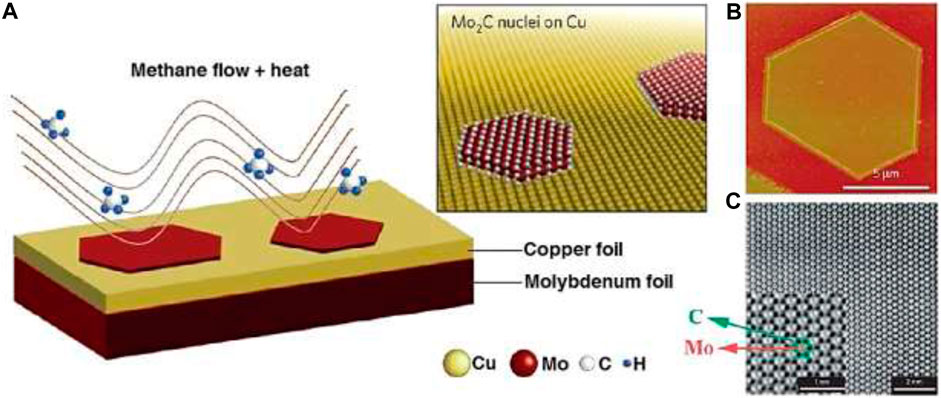
Figure 9. (A) The synthesis process of Mo2C. (B) Images depicting hexagonal ultra-thin Mo2C crystals. (C) STEM diagrams (P. Hu et al., 2022; Amu-Darko et al., 2024).
3.2.7.2 Consideration
A polymer precursor compatible with the CVD process and suitable for deposition onto the substrate surface is chosen and optimized the substrate temperature and deposition parameters to ensure uniform film growth and adhesion. The flow rates of carrier gas and precursor gas are controlled to achieve desired deposition rates and film properties. The deposition conditions are adjusted to control the composition and stoichiometry of the deposited MXene-polymer nanocomposite film.
CVD offers precise control over film thickness, composition, and properties, making it a valuable technique for fabricating MXene-polymer nanocomposites on solid substrates. Optimization of deposition parameters and thorough characterization are essential for achieving desired film properties and performance.
3.2.8 Hydrothermal/solvothermal methods
It is a method of producing a single crystal under high pressure using a hot mineral water solution that is hydrothermal and producing a chemical compound that is solvothermal (Demazeau, 2008). They have a low-temperature synthesis approach that is scalable applicable to various polymers and enables large-scale production. However, the reaction conditions may affect MXene properties and require careful optimization. They are advanced by using environmentally friendly solvents (like water, limonene, ethyl acetate, ethers, esters, ionic liquids, etc.) and improved reaction control (like catalysis, optimization techniques, etc.). Hydrothermal (Byrappa and Yoshimura, 2013) and solvothermal methods are commonly used for synthesizing MXene-polymer nanocomposites in a controlled environment (Rabenau, 1985).
3.2.8.1 Material and method
The MXene of interest, such as titanium carbide (Ti3C2Tx) or others, is prepared through appropriate synthesis methods. The chosen polymer precursor, is typically in the form of a solution or dispersion compatible with the hydrothermal/solvothermal conditions. Common polymers include polyvinyl alcohol (PVA), polyethylene glycol (PEG), or others. A solvent is suitable for both MXene dispersion and polymer dissolution under hydrothermal/solvothermal conditions. Common solvents include water, ethanol, or other polar solvents. A sealed autoclave or reaction vessel capable of withstanding high temperatures and pressures. A heating source capable of providing the required temperature for the hydrothermal/solvothermal reaction. Additives such as surfactants, catalysts, or stabilizers may be used to modify reaction kinetics or improve nanocomposite properties.
The MXene is dispersed in the chosen solvent to create a stable MXene dispersion, with sonication or high-shear mixing potentially employed to achieve uniform dispersion. The chosen polymer is dissolved in the solvent to form a polymer solution or dispersion, with the polymer concentration optimized for the hydrothermal/solvothermal conditions. The MXene dispersion and polymer solution are mixed in the desired ratio to obtain a homogeneous MXene-polymer mixture, ensuring thorough mixing to achieve uniform dispersion of MXene within the polymer matrix. The MXene-polymer mixture is transferred into a sealed autoclave or reaction vessel suitable for hydrothermal/solvothermal reactions, ensuring the vessel is clean and free of contaminants. The autoclave or reaction vessel is heated to the desired temperature for the hydrothermal/solvothermal reaction, typically ranging from 100°C to 250°C, depending on the polymer and MXene used. The reaction temperature is maintained for a specified duration to allow for the synthesis of the MXene-polymer nanocomposite. After the reaction is complete, the autoclave or reaction vessel is cooled to room temperature, and the MXene-polymer nanocomposite product is retrieved. The product may be in the form of a gel, powder, or precipitate, depending on the reaction conditions. Post-treatment steps, such as washing, drying, or annealing, may be performed to remove any unreacted precursors or by-products and to enhance the properties of the nanocomposite material. The synthesized MXene-polymer nanocomposite is characterized to evaluate its structure, morphology, and properties, with techniques such as SEM, XRD, or spectroscopic methods employed.
3.2.8.2 Consideration
The reaction temperature, pressure, and duration to achieve the desired properties of the MXene-polymer nanocomposite are optimized. A solvent suitable (like N-methyl-2-pyrrolidone (NMP) a polar aprotic solvent that has good solubility for a wide range of polymers, including many commonly used ones such as polyvinylidene fluoride (PVDF), polyvinyl alcohol (PVA), and polyethylene oxide (PEO)) for both MXene dispersion and polymer dissolution under hydrothermal/solvothermal conditions are chosen. The additives or surfactants are incorporated to control particle size, and morphology, or to enhance the dispersion of MXene within the polymer matrix. High temperatures and pressures are handled with caution and follow appropriate safety protocols during the hydrothermal/solvothermal reaction.
This method offer controlled conditions for synthesizing MXene-polymer nanocomposites with tailored properties. Optimization of reaction parameters and thorough characterization are essential for achieving desired nanocomposite properties and performance.
3.2.9 Emulsion polymerization
Production of hydrophobic polymers in industrial and academic scales (Hohenstein and Mark, 1946) They are suitable for water-soluble polymers and have good control over particle size and distribution. However, they are limited to specific polymer types and may require additional steps for nanocomposite formation. The novel emulsion systems are developed for improved stability. Emulsion polymerization is a versatile method for preparing polymer-MXene nanocomposites in the form of latex particles dispersed in an aqueous medium (Whitby and Katz, 1933).
3.2.9.1 Material and method
The MXene of interest, such as titanium carbide (Ti3C2Tx), is prepared through appropriate synthesis methods, and the monomers that are compatible with the emulsion polymerization process are selected for polymerization. Common monomers include styrene, methyl methacrylate, or butyl acrylate. An emulsifier or surfactant stabilizes the emulsion and prevents the coalescence of polymer particles. Common surfactants include sodium dodecyl sulfate (SDS), polyvinyl alcohol (PVA), or cetyltrimethylammonium bromide (CTAB). A water-soluble initiator to initiate the polymerization reaction in the aqueous phase. Common initiators include potassium persulfate (KPS), ammonium persulfate (APS), or hydrogen peroxide. Water is the continuous phase for emulsion polymerization. Optionally, a stabilizer such as polyvinyl alcohol (PVA) or polyethylene glycol (PEG) may be added to improve stability and control particle size.
The MXene is dispersed in water using mechanical agitation or sonication to obtain a stable aqueous dispersion, with the MXene concentration optimized based on the desired loading in the nanocomposite. The emulsion is prepared by mixing the MXene dispersion with the surfactant solution, with the surfactant concentration optimized to ensure the stabilization of the emulsion. The chosen monomer or monomer mixture is dissolved in water-soluble initiators to form the aqueous monomer phase, with the monomer concentration adjustable based on the desired properties of the polymer-MXene nanocomposite. The polymerization reaction is initiated by adding the monomer solution to the emulsion under stirring or agitation, with the addition performed gradually to control the particle size and nucleation rate. The reaction temperature is maintained, and stirring continues until the polymerization is complete, typically controlled below the boiling point of water. During polymerization, MXene particles become embedded within the growing polymer particles, forming a polymer-MXene nanocomposite. The surfactant molecules at the interface between water and monomer droplets stabilize the growing polymer particles, preventing coalescence. After polymerization, the nanocomposite latex can undergo post-polymerization treatments such as purification, drying, or chemical modification to remove unreacted monomers or improve their properties. The synthesized polymer-MXene nanocomposite is characterized to evaluate its structure, morphology, and properties, with techniques such as dynamic light scattering (DLS), TEM, or mechanical testing employed.
3.2.9.2 Consideration
An appropriate surfactant is selected to stabilize the emulsion and control particle size during polymerization. Select monomers compatible with water-based emulsion polymerization and capable of forming stable latex particles. Water-soluble initiators are used for polymerization in the aqueous phase, ensuring efficient initiation and control of the reaction. Maintain appropriate stirring and temperature conditions throughout the polymerization process to ensure uniform particle formation and polymerization kinetics.
Emulsion polymerization offers advantages such as easy scalability, control over particle size, and compatibility with aqueous systems, making it a promising method for preparing polymer-MXene nanocomposites in latex form. Optimization of parameters and thorough characterization are essential for tailoring the properties of the nanocomposite for specific applications.
Each synthesis method has its own set of considerations, and the choice depends on the specific requirements of the application. Recent advancements often focus on improving scalability, efficiency, and control over the nanocomposite’s structure and properties. Exploration is going on innovative approaches to enhance the performance and broaden the applicability of MXene-polymer nanocomposites in diverse fields.
3.2.10 Characterization
Techniques such as XRD and TEM are used to confirm the 2D structure and layer spacing of MXenes. X-ray photoelectron spectroscopy (XPS) and FTIR help analyze the chemical composition and functional groups on the MXene surface. Brunauer-Emmett-Teller (BET) surface area analysis is commonly used to determine the specific surface area of MXenes, providing insights into their porosity.
MXene synthesis methods are continuously evolving, with an exploration of new etchants, delamination techniques, and polymer integration strategies to enhance the properties and applications of these unique materials. The ability to customize MXene structures and incorporate them into nanocomposites opens up a wide range of possibilities for various technological advancements.
4 Biomedical application
There are several fields under the biomedical application of MXene Polymer nanocomposite (George, Tandon, and Kandasubramanian, 2020; Garg and Ahmad, 2022; Parajuli et al., 2022).
4.1 Role in drug delivery systems
MXene-polymer nanocomposites play a significant role in drug delivery systems due to their unique properties of higher photothermal conversion and pH sensitivity, which offer advantages for enhancing therapeutic efficacy and addressing challenges associated with conventional drug delivery methods. A sketch of Nb2C/PVP used for tumor ablation by In Vivo Photothermal irradiated with NIR-I and NIR-II is shown in Figure 10. Here are some key roles of MXene-polymer nanocomposites in drug delivery systems.
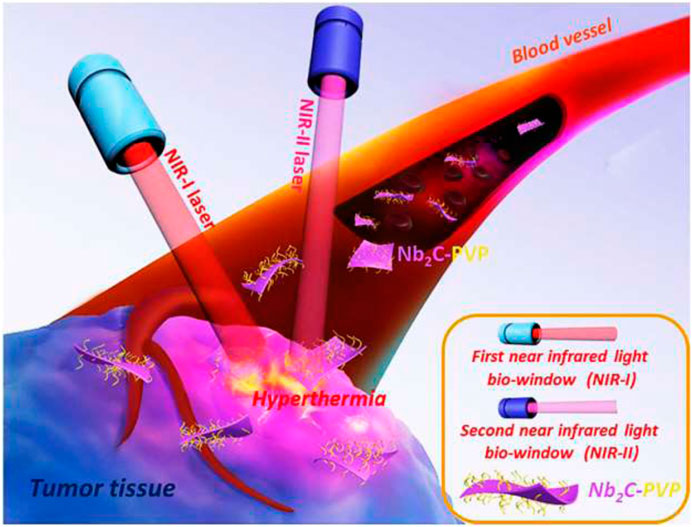
Figure 10. A sketch of Nb2C/PVP used for tumor ablation by In Vivo Photothermal irradiated with NIR-I and NIR-II (Reprinted with permission from ref (Lin, Gao, et al., 2017). Copyright 2017 American Chemical Society.
4.1.1 Improved drug loading and release
MXene nanosheets provide a high surface area and abundant functional groups, allowing for efficient loading of therapeutic agents such as drugs, proteins, or nucleic acids (Sur et al., 2019). The controlled release of these agents can be achieved by modulating the interaction between the drug molecules and the nanocomposite matrix, leading to sustained or triggered release profiles (P. Zhang et al., 2019). For example: the synergistic effect developed in SP surface modified with Ti3C2 destroys the cancerous cells with the help of NIR laser (X. Han et al., 2018) as shown in Figure 11.
4.1.2 Enhanced biocompatibility and stability
Incorporating MXenes into polymer matrices can improve the biocompatibility and stability of drug delivery systems. The polymer coating protects the MXene nanosheets from degradation and minimizes potential cytotoxicity, enhancing the overall biocompatibility of the nanocomposite (Zhao et al., 2021; Cui et al., 2023).
4.1.3 Targeted drug delivery
Surface functionalization of MXene-polymer nanocomposites allows for the introduction of targeting ligands such as antibodies, peptides, or aptamers. These ligands can selectively bind to specific receptors or biomarkers overexpressed on target cells, facilitating targeted drug delivery and minimizing off-target effects (Mohajer et al., 2022) (A. Liu et al., 2022). G. Liu et al. and Z. Li et al. studied MXene nanocomposites for the targeted drug delivery and found efficient for that. (Z. Li et al., 2018).
4.1.4 Responsive drug release
MXene-polymer nanocomposites can be engineered to respond to external stimuli such as pH, redox, temperature, light, electric, magnetic, etc. By incorporating responsive polymers or responsive MXene coatings, drug release from the nanocomposite can be triggered or modulated in a controlled manner, enabling on-demand drug delivery and localized therapy (He et al., 2024).
The pH of cancerous tissues is lower than normal (i.e. below 7.4) dealing with hydrophilic and hydrophilic interaction. (Lopes et al., 2013). In redox response, there is oxidation-reduction in extra and intracellular regions. For example: glutathione (GSH) can be the reducing agent as shown in Figure 12. (Hatakeyama, 2017). (Y. Dai, Chen, and Zhang, 2018). Some of the responsive polymers are Poly acrylic acid (PAA), polyethylene glycol (PEG), poly 2-dimethylamino ethyl methacrylate (PDMAEMA), polyethylene oxide (PEO), poly oligo ethylene methacrylate (POEGMA), etc. In electro-responsive drug release, the electrically conductive MXene by using the external electric field (Zavahir et al., 2020). Similarly, the external magnetic field plays the same role in magnetic-responsive drug release (Darroudi et al., 2023).The light-responsive molecules like photochromic compounds stimulated by light of a certain wavelength create structural changes in MXene or its surroundings for light-responsive drug release (Zavahir et al., 2020). In case of temperature-responsive drug release, MXene nanosheets into a polymer matrix with a lower critical solution temperature (LCST), the drug release can be triggered by changes in temperature above or below the LCST can be utilized (Karimi et al., 2016).
4.1.5 Theranostic application
MXene-polymer nanocomposites have shown promise for theranostic applications, combining therapeutic and diagnostic functionalities within a single platform. The efficient breast cancer theranostic was achieved by tantalum carbide MXene composite (Z. Liu et al., 2018; Lin et al., 2018). Polyoxometalate as functionalizing agent for Tantalum carbide was studied by L Zong et al., 2018 for theranostic application. MXene Functionalization with imaging agents or contrast agents enables real-time monitoring of drug distribution and therapeutic response, facilitating personalized medicine and treatment optimization (Sivasankarapillai et al., 2020; Aslam et al., 2023; Nikazar, Mofidi, and Mortazavi, 2023).
4.1.6 Synergistic therapeutic effect
The combination of MXenes with polymers can lead to synergistic therapeutic effects, where the unique properties of each component complement each other. For example, A. Liu et al., 2022, Yang et al., 2022, W Tang et al., 2019 found that MXene’s high conductivity and mechanical strength combined with the polymer’s biocompatibility and versatility can enhance the overall performance of drug delivery systems, enabling novel therapeutic strategies (A. Liu et al., 2022; Yang et al., 2022)(W. Tang et al., 2019).
4.1.7 Multifunctional platforms
MXene-polymer nanocomposites can serve as multifunctional platforms for combination therapy, allowing for the co-delivery of multiple drugs or therapeutic agents with different mechanisms of action. This approach can overcome drug resistance, improve treatment outcomes, and reduce side effects compared to single-agent therapy. Several studies and experiments have demonstrated the efficacy of MXene-polymer nanocomposites in Drug delivery systems. Wang et al. (2019) developed a pH-responsive drug delivery system based on MXene-polymer nanocomposites for targeted cancer therapy. The nanocomposites exhibited high drug loading capacity, controlled drug release behavior, and enhanced cytotoxicity against cancer cells compared to free drugs (Wang et al., 2019). In another study, Liu et al. (2021) synthesized MXene-polymer nanocomposites for the co-delivery of doxorubicin (DOX) and indocyanine green (ICG) for combined chemotherapy and photothermal therapy. The nanocomposites showed improved therapeutic efficacy and enhanced tumor inhibition in a mouse xenograft model compared to monotherapy (X. Liu et al., 2021).
4.1.8 Anticancer treatment
In cancer treatment, therapeutics such as PLGA/Ti3C2 (Lin, Wang, et al., 2017) are employed for photothermal ablation. Additionally, Ti3C2/Al has been utilized for cancer treatment under 808 nm laser radiation (Z. Liu et al., 2018). V2C nanosheets demonstrate efficacy as photothermal agents for photothermal treatment, with applications in photoacoustic (PA) and magnetic resonance imaging (MRI) (Zada et al., 2020). Moreover, AIPH@Nb2C@mSiO2 nanocomposites have been employed for thermodynamic therapy targeting cancer cells deficient in oxygen (Xiang et al., 2019). Ti3C2-DOX complexes have been demonstrated to generate reactive oxygen species (ROS) during photodynamic therapy, effectively targeting and killing cancerous cells (K. Huang et al., 2018). Additionally, they find utility in drug delivery applications. Nb2C/polymer nanocomposites have been utilized to ablate tumors via photothermal processes, particularly in the near-infrared region (Lin, Gao, et al., 2017). Furthermore, MnOx/Ti3C2-SP and MnOx/Ta4C3-SP MXene nanocomposites have been employed for the treatment of acidic tumors (C. Dai, Chen, et al., 2017; Lin, Chen, and Shi, 2018).
Thus, MXene-polymer nanocomposites hold great promise for revolutionizing drug delivery systems by offering tailored properties, enhanced functionality, and improved therapeutic outcomes. Continued research and development in this field are expected to lead to the translation of these nanocomposites into clinically relevant applications for the treatment of various diseases.
4.2 Imaging and diagnostics
MXene-polymer nanocomposites hold significant potential in imaging and diagnostics due to their unique properties and versatile functionalities. For example: They are used for the allocation of affected areas like tumor regions and monitoring the treatment effect by MRI. Here are some key aspects highlighting their potential in this field.
4.2.1 Enhanced contrast agents
MXene nanosheets can be functionalized with imaging agents such as fluorophores, magnetic nanoparticles, or radioisotopes to enhance contrast in various imaging modalities including optical imaging, MRI, and nuclear imaging techniques (e.g., positron emission tomography (PET) or single-photon emission computed tomography (SPECT)). The integration of MXenes into polymer matrices ensures stability and biocompatibility of the contrast agents, enabling their use in vivo for non-invasive imaging (Aslam et al., 2023). Ta4C3-IONP-SP nanocomposites represent a case employed in MRI applications (Z. Liu et al., 2018) as enhanced contrast agent.
4.2.2 Targeted imaging
Surface modification of MXene-polymer nanocomposites with targeting ligands (e.g., antibodies, peptides, or aptamers) allows for specific binding to molecular targets or biomarkers overexpressed in diseased tissues. This targeted approach improves imaging sensitivity and specificity, enabling early detection and precise localization of pathological lesions (Kumar, Barman, and Singh, 2021).
4.2.3 Multimodal imaging
MXene-polymer nanocomposites can be engineered to exhibit multimodal imaging capabilities by combining different imaging agents within a single platform. For example, integrating fluorescent dyes for optical imaging with magnetic nanoparticles for MRI or radionuclides for PET allows for complementary information acquisition and improved diagnostic accuracy (Z. Liu et al., 2018).
4.2.4 Responsive imaging
MXene-based nanocomposites can respond to external stimuli such as pH, temperature, or specific biomolecules, leading to changes in their imaging properties. C. Dai et al., 2017 used the nanocomposite in pH-guided MRI for hyperthermia treatment of tumors (C. Dai, Lin, et al., 2017). Responsive imaging agents enable dynamic monitoring of physiological processes or disease progression in real-time, providing valuable insights into treatment response and disease mechanisms (X. Han et al., 2018).
4.2.5 Theranostic application
MXene-polymer nanocomposites have the potential for theranostic applications, combining diagnostic and therapeutic functionalities within a single platform. By incorporating both imaging agents and therapeutic agents, these nanocomposites enable image-guided therapy, personalized treatment planning, and monitoring of therapeutic response in real-time (Lin et al., 2018).
4.2.6 Bioimaging
MXene-polymer nanocomposites exhibit excellent biocompatibility and low cytotoxicity, making them suitable for biomedical imaging applications. These nanocomposites can be used for in vitro cell imaging, in vivo molecular imaging, and bioimaging of tissues and organs, providing valuable information for disease diagnosis, prognosis, and treatment evaluation (Gomez et al., 2018).
4.2.7 Flexible and functional platforms
MXene-polymer nanocomposites offer flexibility in design and functionalization, allowing for the customization of imaging properties based on specific application requirements. Tailoring the composition, size, and surface chemistry of the nanocomposites enables fine-tuning of imaging performance and optimization for different imaging modalities (Ling et al., 2014).
Some additional prominent research was made in Imaging and diagnostics. Xu et al. (2020) developed MXene-polymer nanocomposites as dual-modal contrast agents for both photoacoustic imaging (PAI) and MRI. The nanocomposites exhibited excellent imaging performance with high signal intensity and superior contrast enhancement in tumor imaging (Xu et al., 2020). Kong and Chen in 2022 reported the synthesis of MXene-polymer nanocomposites as theranostic agents for combined photothermal therapy (PTT) and photodynamic therapy (PDT) of cancer (Kong and Chen, 2022). The nanocomposites showed efficient tumor ablation and simultaneous imaging capability for guiding therapy (H. Li et al., 2021).
4.3 Biosensors
Biosensors are employed to identify specific elements within the human body. These devices consist of several components: a sensing element, a transducer, and a data interpreter (Prajapati and Kandasubramanian, 2019). The sensing element, typically an immobilized biomolecule like an enzyme, can detect the concentration of a target analyte in its vicinity, generating a biochemical signal. This signal is then converted into an electrical signal by a transducer and subsequently interpreted by a data interpreter. In a study by Rakhi and colleagues, they developed an enzymatic biosensor (GOx/Au/Ti3C2/Nafion/GCE) for detecting glucose (Rakhi et al., 2016). Glucose is converted into gluconolactone and hydrogen peroxide (H2O2) through the action of the enzyme glucose oxidase (GOx). The presence of glucose leads to the production of peroxide, generating a high potential indicative of glucose levels. Nafion facilitates the enzyme’s adhesion to the glassy carbon electrode (GCE) (Harper and Anderson, 2010).
In Biosensing and detection, Li et al. (2020) synthesized MXene-polymer nanocomposites for glucose biosensing applications. The nanocomposites exhibited high sensitivity, excellent selectivity, and rapid response toward glucose detection, showing promise for diabetes management and point-of-care testing (X. Li, Zhao, and Wu, 2020). Jiang et al. (2021) developed MXene-polymer nanocomposites for ultrasensitive detection of circulating tumor DNA (ctDNA) in blood samples. The nanocomposites demonstrated high specificity and sensitivity for ctDNA detection, offering potential for early cancer diagnosis and prognosis monitoring (Y. Jiang et al., 2021). The GOx/Au/Ti3C2/Nafion/GCE configuration represents an enzymatic biosensor for glucose detection (Rakhi et al., 2016).
4.4 Tissue engineering
In Tissue engineering and regenerative medicine, Zhu et al. (2020) fabricated MXene-polymer nanocomposite scaffolds for bone tissue engineering applications. The nanocomposite scaffolds exhibited excellent biocompatibility, enhanced mechanical properties, and promoted osteogenic differentiation of mesenchymal stem cells (MSCs) in vitro (J. Zhu et al., 2020). In a study by Ma et al. (2021), MXene-polymer hydrogels were developed as injectable scaffolds for cartilage repair. The nanocomposite hydrogels exhibited excellent biocompatibility, controlled drug release, and promoted chondrogenic differentiation of MSCs, showing potential for cartilage regeneration (Ma et al., 2021)
The literature demonstrates the biocompatibility of MXene-polymer nanocomposites for biomedical applications, including tissue engineering, drug delivery, and implantable medical devices. Further research is needed to fully understand the biocompatibility mechanisms and optimize the design of MXene-polymer nanocomposites for safe and effective biomedical applications. The synthesis, application, and properties of MXene-polymer nanocomposites are listed in Table 3.
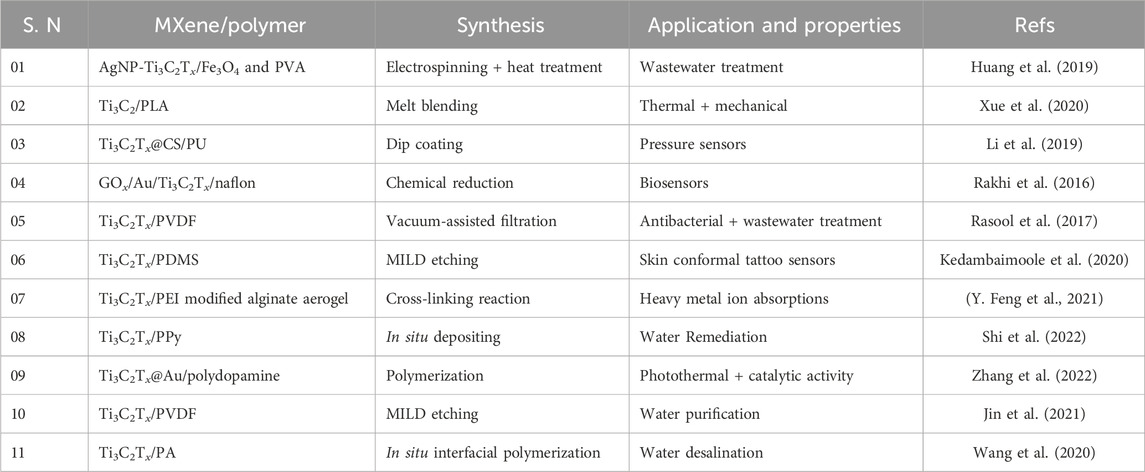
Table 3. MXene–polymer composite materials for Biocompatibility and environment remedies with synthesis, application, and properties (adopted from (Parajuli et al., 2022)).
Overall, MXene-polymer nanocomposites represent a promising platform for advancing imaging and diagnostics, offering enhanced contrast, targeted imaging, multimodal capabilities, responsiveness, and biocompatibility. Continued research and development in this area are expected to lead to the translation of these nanocomposites into clinically relevant imaging agents for early disease detection, precise diagnosis, and personalized medicine.
These studies highlight the diverse applications and promising outcomes of MXene-polymer nanocomposites in biomedical research, ranging from drug delivery systems and imaging diagnostics to tissue engineering and biosensing. Continued exploration and innovation in this field are expected to lead to the development of novel nanocomposite-based technologies for improved healthcare and disease management.
5 Biocompatibility and toxicity
MXene-polymer nanocomposites hold great promise for biomedical applications due to their unique properties and versatile functionalities. Biocompatibility refers to the ability of a material to perform its desired function without eliciting an adverse reaction in living organisms. In the context of MXene-polymer nanocomposites, assessing biocompatibility involves evaluating how these materials interact with biological systems, including cells, tissues, and organs. Several factors influence nanocomposites’ biocompatibility, including the materials’ physicochemical properties, surface characteristics, and degradation behavior (Parajuli et al., 2022). Toxicity assessment is another critical aspect, focusing on the potential adverse effects of MXene-polymer nanocomposites on biological systems. Toxicity can arise from various sources, such as the release of toxic ions, generation of reactive oxygen species (ROS), or physical damage to cells and tissues. Understanding the toxicity profile of nanocomposites is essential for ensuring their safety in biomedical applications, such as drug delivery, tissue engineering, and medical implants (Vasyukova et al., 2022).
Various techniques are being employed to evaluate the biocompatibility and toxicity of MXene-polymer nanocomposites, including in vitro assays using cell cultures, in vivo studies using animal models, and computational modeling approaches. These studies assess parameters such as cell viability, proliferation, inflammation, oxidative stress, genotoxicity, and organ function following exposure to nanocomposite materials (Khatri et al., 2013). Additionally, surface modification strategies can be employed to enhance the biocompatibility of MXene-polymer nanocomposites by reducing potential cytotoxicity and improving their interactions with biological systems. Surface functionalization with biocompatible molecules or polymers can mitigate adverse effects and promote desirable biological responses.
Ensuring the biocompatibility of these nanocomposites is crucial for their safe and effective use in biological systems. Ti3C2-SP, Ta4C3-SP, and MnOx/Ti3C2-SP are utilized in a photoacoustic signal approach, leveraging stress waves from tissues irradiated by NIR. Ta4C3-IONP-SPs and Ta4C3-SP nanocomposites possess the capability to attenuate X-rays, making them suitable for employment in computed tomography (CT) (Z. Liu et al., 2018; C; Dai, Chen, et al., 2017; Lin et al., 2018).
Several studies have investigated the biocompatibility of MXene-polymer nanocomposites, demonstrating their potential for various biomedical applications.
5.1 Biocompatibility assessment
Research by Li et al. (2021) conducted comprehensive biocompatibility assessments of MXene-polymer nanocomposites for biomedical applications. The study evaluated cytotoxicity, hemocompatibility, and in vivo biocompatibility, demonstrating minimal adverse effects and high biocompatibility of MXene-polymer nanocomposites (X. Li et al., 2021).
5.2 Cell viability and proliferation
Wang et al. (2020) investigated the effect of MXene-polymer nanocomposites on cell viability and proliferation for tissue engineering applications. The study showed that MXene-polymer nanocomposites supported cell attachment, proliferation, and differentiation, indicating their biocompatibility and potential for promoting tissue regeneration (Wang et al., 2020). The colloidal solution of Ti3C2 facilitates the proliferation of Gram-negative E. coli and Gram-positive Bacillus subtilis, demonstrating antibacterial properties (Han et al., 2017). Ag@Ti3C2@Cu2O nanocomposites serve as photocatalysts suitable for antibacterial applications (Feng et al., 2020).
5.3 Inflammatory response
Zhang et al. (2021) evaluated the inflammatory response of MXene-polymer nanocomposites in vitro and in vivo. The study demonstrated low levels of inflammatory cytokine production and minimal tissue inflammation upon exposure to MXene-polymer nanocomposites, highlighting their biocompatibility and suitability for biomedical applications (Zhang, Y., Wang, S., Liu, X., & Chen, 2021).
5.4 Tissue compatibility
Xu et al. (2019) investigated the tissue compatibility of MXene-polymer nanocomposites for implantable medical devices. The study showed favorable tissue responses, minimal fibrous encapsulation, and enhanced tissue integration with MXene-polymer nanocomposites, suggesting their potential for long-term implantation in vivo (Y. Xu et al., 2019). Ti3C2Tz combined with PLA and octyltriethoxysilane (OTES) exhibits favorable mechanical properties and biocompatibility, aiding in tissue engineering (Zhang et al., 2018; Zhou et al., 2018).
Conclusively, comprehensive evaluation of biocompatibility and toxicity is essential for advancing the development of MXene-polymer nanocomposites for biomedical applications, ensuring their safe and effective use in various healthcare settings.
6 Challenges, solutions, and outlooks
While MXene-polymer nanocomposites hold immense promise for various applications, several challenges still need to be addressed to fully exploit their potential. The challenges faced by MXenes and their future perspectives are sketched in Figure 13. The challenges are on the outer octagon and the future perspectives in the inner octagon of the Figure. Some of the current challenges in the development and application of MXene-polymer nanocomposites include.
6.1 Challenges and solutions
6.1.1 Scalability of synthesis
One major challenge is scaling up the synthesis of MXene-polymer nanocomposites to meet industrial production demands. Current synthesis methods often involve complex and time-consuming procedures, limiting their scalability and commercial viability.
6.1.1.1 Advanced synthesis techniques
Novel synthesis techniques are being employed that enable the scalable production of MXene-polymer nanocomposites with improved efficiency and reproducibility. Techniques such as aerosol-assisted synthesis, microwave-assisted synthesis, and template-assisted synthesis offer potential solutions for overcoming scalability issues.
6.1.2 Uniform dispersion
Achieving uniform dispersion of MXene nanosheets within polymer matrices is crucial for maintaining the desired properties and performance of nanocomposites. However, ensuring uniform dispersion remains a challenge due to the tendency of MXene nanosheets to agglomerate during processing.
6.1.2.1 Surface modification
Surface modification of MXene nanosheets with functional groups or surfactants can enhance their dispersibility within polymer matrices and improve interfacial adhesion. Surface modification techniques such as chemical functionalization, plasma treatment, and surfactant-assisted dispersion are being investigated to address agglomeration and achieve uniform dispersion.
6.1.3 Interfacial adhesion
Achieving strong interfacial adhesion between MXene nanosheets and polymer matrices is essential for enhancing mechanical properties and stability of nanocomposites. However, achieving optimal interfacial adhesion remains challenging due to the inherently different properties of MXenes and polymers.
6.1.3.1 Interfacial engineering
Strategies for optimizing interfacial adhesion between MXene nanosheets and polymer matrices are being explored to enhance the mechanical properties and stability of nanocomposites. Approaches such as compatibilizer incorporation, in-situ polymerization, and interfacial coupling agents aim to improve interfacial interactions and enhance nanocomposite performance.
6.1.4 Biocompatibility
While MXene-polymer nanocomposites show great potential for biomedical applications, ensuring their biocompatibility remains a challenge. Further research is needed to comprehensively evaluate the biocompatibility of these nanocomposites and mitigate potential cytotoxicity or immunogenicity issues.
6.1.4.1 Biocompatibility assessment
Ongoing research focuses on comprehensive biocompatibility assessment of MXene-polymer nanocomposites for biomedical applications. Studies involve evaluating cytotoxicity, immunogenicity, and long-term biocompatibility through in vitro and in vivo experiments, aiming to ensure the safety and efficacy of nanocomposites in biological environments.
6.1.5 Tunable properties
Tailoring the properties of MXene-polymer nanocomposites to meet specific application requirements poses a challenge. Achieving tunable properties such as electrical conductivity, mechanical strength, and thermal stability requires precise control over MXene loading, polymer composition, and processing conditions.
6.1.5.1 Property tuning
Investigations are going on for tuning the properties of MXene-polymer nanocomposites to meet specific application requirements. Strategies such as controlling MXene loading, varying polymer composition, and adjusting processing conditions enable the customization of nanocomposite properties such as electrical conductivity, mechanical strength, and thermal stability.
6.1.6 Longevity
Ensuring the long-term stability of MXene-polymer nanocomposites under various environmental conditions is essential for their practical applications. However, issues such as degradation, leaching, and agglomeration may affect the stability and performance of nanocomposites over time.
6.1.6.1 Stability enhancement
Ongoing research focuses on enhancing the long-term stability of MXene-polymer nanocomposites under various environmental conditions. Strategies such as surface passivation, encapsulation, and cross-linking aim to improve nanocomposite stability, preventing degradation, leaching, and agglomeration over time.
6.1.7 Cost-effectiveness
The cost-effectiveness of MXene-polymer nanocomposites remains a concern, especially for large-scale applications. The high cost of MXene precursors and complex synthesis processes may hinder their widespread adoption in commercial applications. Efforts are underway to develop cost-effective synthesis routes and utilize more affordable precursor materials to reduce the production cost of MXene-polymer nanocomposites. Additionally, process optimization and scale-up strategies aim to streamline production processes and lower overall manufacturing costs.
Since the cost is a major factor in the manufacture of the MXene-polymer nanocomposite on a large scale (Zaed et al., 2024), we have further gone in with the factors of impact as sketched in Figure 14. These factors are.
6.1.7.1 Raw material costs
The cost of raw materials, particularly the precursors needed to produce MXenes, significantly impacts the overall synthesis cost. Precursors may include transition metal carbides, nitrides, or carbonitrides, which can be expensive depending on their source and availability (Rasid et al., 2017).
• Transition metal carbides, nitrides, or carbonitrides: Prices can vary based on factors such as purity, synthesis method, and availability. For example, the cost of titanium carbide powder can range from $50 to $200 per kilogram depending on quality and quantity purchased.
• Precursor chemicals: Prices vary widely depending on the specific chemicals used in the synthesis process. For instance, hydrochloric acid (HCl), a common etchant used in MXene synthesis, costs approximately $0.30 to $1.00 per liter.
6.1.7.2 Synthesis methodology
The choice of synthesis method plays a crucial role in determining costs (Shuck et al., 2021). Some synthesis routes may require expensive equipment, specialized facilities, or energy-intensive processes, increasing production expenses. Optimizing synthesis methods to minimize energy consumption or utilizing simpler techniques can help reduce costs (Jolly, Paranthaman, and Naguib, 2021). High-temperature synthesis methods such as MAX phase etching and exfoliation may require energy-intensive processes, increasing operational costs. For example, the energy cost for heating furnaces or reactors can be significant, ranging from several hundred to thousands of dollars per batch depending on the scale and duration of the synthesis process. Alternatively, low-temperature synthesis methods, such as the use of ionic liquids or mild etchants, may reduce energy consumption but could require specialized equipment or reagents, impacting upfront capital costs (Jolly, Paranthaman, and Naguib, 2021).
6.1.7.3 Process efficiency
The efficiency of the synthesis process affects the yield of desired MXene-polymer nanocomposites per unit of input materials. Low efficiency may result in the wastage of raw materials, energy, and time, driving up production costs. Improving process efficiency through better reaction conditions, catalysts, or purification methods can lower overall costs (Tan et al., 2024).
• Yield of desired MXene product: Efficiency in converting precursor materials into high-quality MXene affects production costs. Higher yields reduce material wastage and associated costs.
• Time required for synthesis: Longer reaction times or complex purification processes can increase labor and energy costs. Optimizing reaction conditions and purification steps can improve process efficiency and reduce overall production time and costs.
6.1.7.4 Labor costs
Skilled labor is essential for synthesizing MXene-polymer nanocomposites, especially in research and development phases where experimentation and optimization are common. Labor costs can significantly impact the overall synthesis cost, particularly if extensive manual intervention is required. Automation and streamlining of processes can help mitigate labor expenses (Islam and Shazali, 2011).
• Skilled labor wages: Labor costs vary widely depending on location, expertise required, and labor market conditions. For example, researchers or technicians involved in MXene synthesis may earn salaries ranging from $40,000 to $100,000 or more annually, depending on experience and qualifications.
• Automation and process optimization: Investments in automation technologies and streamlined processes can reduce labor requirements and associated costs over time.
6.1.7.5 Scale of production
Economies of scale have a significant impact on synthesis costs, as larger production volumes often lead to reduced unit costs (Shuck et al., 2020a). However, expanding production while ensuring consistent quality can be challenging. To achieve cost-effective large-scale production, investments in infrastructure, equipment, and process optimization are essential. The advantages of economies of scale become apparent as production volumes increase, allowing fixed costs to be spread out over a larger output (Shuck et al., 2020). This typically results in lower unit costs. Nevertheless, the initial investments required to scale up production facilities and equipment can be considerable. For instance, transitioning from laboratory-scale to pilot-scale or industrial-scale production may necessitate investments ranging from thousands to millions of dollars. The magnitude of investment depends on factors such as the complexity of the process and the desired output capacity (Charitidis et al., 2014).
6.1.7.6 Purification and characterization
Purification steps to remove impurities and characterization techniques to assess product quality add to the synthesis cost. These processes require additional materials, equipment, and labor. Developing efficient purification methods and adopting cost-effective characterization techniques can help manage these expenses (H. Tang et al., 2021).
• Cost of purification agents and equipment: Purification processes, such as acid washing or filtration, require chemicals, solvents, and specialized equipment. For example, the cost of membrane filtration systems for removing impurities can range from a few hundred to several thousand dollars depending on capacity and efficiency.
• Analytical techniques: Characterization methods such as SEM, XRD, and atomic force microscopy (AFM) incur costs for instrument purchase, maintenance, and consumables. For example, an SEM instrument may cost anywhere from $100,000 to over $1 million, plus ongoing maintenance and operation expenses.
6.1.7.7 Regulatory compliance
This is to follow with environmental, health, and safety regulations adds to the cost of synthesis (Kumari et al., 2023). Implementing sustainable practices and ensuring compliance with regulations may require investments in waste management, safety measures, and compliance monitoring (Journal and Ondokuzmay 2020). One of the concerns is the environmental and safety regulations. It is compliance with regulations governing chemical handling, waste disposal, and workplace safety may require investments in training, protective equipment, and facility modifications (Foulkes et al., 2020). Costs associated with regulatory compliance can vary widely depending on the jurisdiction and specific requirements (Directorate et al., 2015).
6.1.7.8 Supply chain consideration
The availability and cost of ancillary materials, such as solvents, catalysts, and additives, can impact overall synthesis costs. Fluctuations in prices or supply chain disruptions can affect production economics. Diversifying supply sources or developing alternative materials can mitigate these risks (Lele, 2023).
• Ancillary materials and consumables: Prices of solvents, catalysts, and other consumables used in the synthesis process can fluctuate based on market conditions and availability. Bulk purchasing or negotiating long-term supply contracts may help stabilize costs.
• Supply chain disruptions: Events such as natural disasters, geopolitical tensions, or trade restrictions can disrupt the availability of raw materials or increase transportation costs. Diversifying supply sources and maintaining contingency plans can mitigate these risks.
Addressing these critical factors through research, innovation, and process optimization is essential for achieving cost-effective synthesis of MXene-polymer nanocomposites on a large scale. Collaboration between academia, industry, and government stakeholders can accelerate progress in this field and facilitate the commercialization of MXene-based materials.
6.1.8 Regulatory approval
Not only is the cost of manufacturing the nanocomposite, but it is also concerned with obtaining regulatory approval for MXene-polymer nanocomposites, particularly for biomedical and environmental applications, poses a challenge. Comprehensive safety assessments and standardized testing protocols are needed to ensure compliance with regulatory requirements.
6.1.8.1 Regulatory compliance
Investigation are going towards obtaining regulatory approval for MXene-polymer nanocomposites by conducting comprehensive safety assessments and adhering to standardized testing protocols. Collaboration with regulatory agencies and compliance with regulatory guidelines ensure the safe and ethical use of nanocomposites in commercial applications.
Addressing these challenges requires interdisciplinary research focusing on developing novel synthesis techniques, optimizing nanocomposite properties, improving scalability, enhancing biocompatibility, and conducting thorough performance evaluations. Collaborative efforts between academia, industry, and regulatory agencies are essential to overcome these challenges and unlock the full potential of MXene-polymer nanocomposites for diverse applications. Ongoing research in MXene-polymer nanocomposites aims to address the existing challenges through innovative solutions, interdisciplinary collaborations, and advancements in synthesis, characterization, and application techniques. These efforts pave the way for the widespread adoption of MXene-polymer nanocomposites across various industries, including electronics, energy, healthcare, and environmental remediation.
6.2 Outlooks
Future directions for the field of MXene-polymer nanocomposites encompass emerging trends and areas for exploration that hold promise for advancing research and applications. Some key future directions include.
6.2.1 Multifunctional nanocomposites
Future research will focus on developing MXene-polymer nanocomposites with multifunctional properties for diverse applications. They are composite materials composed of two or more distinct nanomaterials (such as nanoparticles, nanotubes, or nanosheets) dispersed within a matrix material (polymer, ceramic, or metal). These nanocomposites exhibit a combination of properties not present in the individual components alone. Various synthesis methods are employed to fabricate multifunctional nanocomposites, including solution mixing, in situ polymerization, melt blending, electrospinning, and layer-by-layer assembly. Each method offers advantages in terms of control over nanomaterial dispersion, interfacial interactions, and composite structure. These properties can be tailored by adjusting the composition, size, shape, and distribution of the nanomaterials within the matrix. These nanocomposites may integrate functionalities such as conductivity, mechanical strength, electrical conductivity, thermal stability, optical transparency, magnetic response, chemical reactivity, magnetism, biocompatibility, and stimuli-responsiveness, enabling their use in advanced electronics, sensors, actuators, biomedical devices electronics, aerospace, energy storage, sensing, catalysis, and environmental remediation (Yadav, 2023).
Despite their potential, multifunctional nanocomposites face challenges related to scalability, reproducibility, stability, and environmental impact. Future research aims to address these challenges by developing novel synthesis techniques, optimizing composite properties, and exploring new applications in emerging fields.
6.2.2 3D printing and additive manufacturing
The integration of MXene-polymer nanocomposites into additive manufacturing processes, such as 3D printing, will be explored for fabricating complex structures and functional devices with tailored properties. This approach offers opportunities for rapid prototyping, customization, and on-demand manufacturing of MXene-based products.
There are several techniques for 3D printing, including fused deposition modeling (FDM), stereolithography (SLA), selective laser sintering (SLS), binder jetting, and directed energy deposition (DED). Each technique has its advantages and is suitable for different materials and applications (Jiménez et al., 2019). 3D printing has diverse applications across industries such as aerospace, automotive, healthcare, architecture, consumer goods, and education. It is used for prototyping, rapid manufacturing, custom production, tooling, and even creating complex biological structures in biomedical engineering. Some key advantages of 3D printing include rapid prototyping, customization, reduced material waste, design freedom, and the ability to create intricate and lightweight structures. It also allows for on-demand production and decentralized manufacturing.
Despite its numerous advantages, 3D printing still faces challenges related to material limitations, surface quality, printing speed, scalability, and regulatory considerations, particularly in highly regulated industries such as healthcare.
6.2.3 Energy storage and conversion
Future research will focus on leveraging the unique properties of MXene-polymer nanocomposites for energy storage and conversion applications. These nanocomposites may be used as electrode materials in batteries, supercapacitors, and fuel cells, offering high energy density, fast charge-discharge rates, and long-term stability.
MXene-polymer nanocomposites offer several advantages that make them attractive for energy storage and conversion applications (Qiu et al., 2021). These include high surface area, excellent electrical conductivity, mechanical flexibility, and chemical stability. These properties enable them to serve as efficient electrode materials in various energy storage and conversion devices. Furthermore, MXene-polymer nanocomposites have shown promise in enhancing the performance of lithium-ion batteries, fuel cells, and other energy storage devices. Research in this area is ongoing, focusing on optimizing the composition, structure, and processing techniques of MXene-based materials to improve their energy storage and conversion properties (Parajuli et al., 2022).
By leveraging the unique properties of MXene-polymer nanocomposites, future research endeavors seek to develop next-generation energy storage and conversion technologies that offer high energy density, rapid charge-discharge rates, and long-term stability, contributing to the advancement of renewable energy integration and sustainable energy solutions.
6.2.4 Biomedical devices and therapeutics
The development of MXene-polymer nanocomposites for biomedical devices and therapeutics will be explored further. These nanocomposites may find applications in drug delivery systems, tissue engineering scaffolds, biosensors (Guo et al., 2019), and medical implants, offering biocompatibility, bioactivity, and controlled release properties.
The field of biomedical devices and therapeutics is rapidly evolving, driven by technological advancements and innovative research. Here are some future perspectives along.
6.2.4.1 Personalized medicine
Future biomedical devices and therapeutics will increasingly focus on personalized medicine approaches, tailoring treatments to individual patients based on their genetic makeup, lifestyle factors, and disease characteristics. This includes the development of biomarker-based diagnostics, targeted therapies, and precision drug delivery systems (Foroutan, 2015).
6.2.4.2 Implantable medical devices
Advances in materials science, microelectronics, and biocompatible coatings will lead to the development of smarter, more functional implantable devices for monitoring and treating various medical conditions. These devices may incorporate sensors, actuators, and wireless communication capabilities to provide real-time data and therapeutic interventions (Wodlinger, Dweiri, and Durand, 2015).
6.2.4.3 Biomedical devices
Nanomedicine will continue to revolutionize biomedical applications by enabling targeted drug delivery, enhanced imaging, and regenerative therapies. Nanoparticle-based drug carriers, nanofiber scaffolds for tissue engineering, and nanoscale imaging agents hold great potential for improving diagnosis and treatment outcomes (Bai et al., 2006).
6.2.4.4 Bioelectronics and wearable devices
The integration of biology with electronics will lead to the development of advanced wearable devices for continuous health monitoring, disease management, and drug delivery. Flexible and stretchable electronics, bio-compatible materials, and miniaturized sensors will enable seamless integration with the human body (Manjushree et al. 2022).
6.2.4.5 Gene editing and cell therapies
Advances in gene editing technologies such as CRISPR-Cas9 will revolutionize the development of gene therapies for treating genetic disorders and cancer (Jiang and Doudna, 2017). Cell-based therapies, including CAR-T cell immunotherapy and stem cell transplantation, hold promise for regenerative medicine and personalized cancer treatments (H. Wang, La Russa, and Qi, 2016).
In conclusion, the future of biomedical devices and therapeutics holds great promise, with ongoing research and innovation focusing on personalized medicine, implantable devices, nanotechnology, bioelectronics, and gene editing technologies, all aimed at revolutionizing healthcare delivery and improving patient outcomes.
6.2.5 Environmental remediation
Future research will focus on harnessing MXene-polymer nanocomposites for environmental remediation and pollution control. These nanocomposites may be used for water purification, air filtration, soil remediation, and wastewater treatment, offering high adsorption capacity, catalytic activity, and selectivity towards pollutants. One significant future direction involves the integration of emerging technologies such as artificial intelligence, machine learning, and remote sensing into environmental monitoring and remediation strategies. These technologies can improve the efficiency and effectiveness of pollution detection, site characterization, and remediation efforts by providing real-time data, predictive modeling, and automated decision-making capabilities (Shah, Rodriguez-Couto, and Sengor, 2020).
Furthermore, there is growing interest in the development of nature-based solutions and green infrastructure for environmental remediation. These approaches harness natural processes and ecosystems to restore habitats, purify water, and sequester carbon, offering cost-effective and sustainable alternatives to conventional remediation techniques.
6.2.6 Smart and responsive materials
The development of smart and responsive MXene-polymer nanocomposites that can sense and respond to external stimuli, such as light, temperature, pH, and mechanical stress, will be explored. These materials may find applications in smart textiles, adaptive coatings, drug delivery systems, and biomedical implants.
One significant future direction is the enhancement of the stimuli-responsive properties of MXene-polymer nanocomposites through precise control over their composition, structure, and interface interactions. This may involve the incorporation of stimuli-responsive polymers or functionalization of MXene nanosheets with responsive molecules to achieve tunable and reversible responses to environmental changes. Furthermore, research efforts are focused on integrating MXene-polymer nanocomposites into multifunctional devices and systems for diverse applications. These may include smart coatings for corrosion protection, stimuli-responsive drug delivery systems for targeted therapies, and adaptive materials for shape-shifting structures or wearable electronics (Parajuli et al., 2022).
6.2.7 Green synthesis and sustainability
Future research will focus on developing green synthesis routes and sustainable manufacturing processes for MXene-polymer nanocomposites. These efforts aim to reduce environmental impact, minimize resource consumption, and enhance the eco-friendliness of nanocomposite production. The future research in this direction involves the following.
6.2.7.1 Environmentally benign synthesis routes
One focal point of future research involves exploring synthesis pathways that minimize or eliminate hazardous chemicals, volatile organic compounds (VOCs), and other environmentally detrimental substances. Green synthesis methodologies, such as aqueous-based processes or solvent-free techniques, are gaining traction for their reduced environmental footprint and improved safety profiles.
6.2.7.2 Renewable feedstock utilization
Another pivotal aspect revolves around harnessing renewable feedstock and sustainable raw materials for MXene synthesis. By shifting away from fossil fuel-derived precursors towards bio-based or recycled materials, researchers aim to lessen dependence on finite resources and promote circular economy principles.
6.2.7.3 Energy-efficient production process
Efficiency improvements in energy consumption during the synthesis and processing stages represent a crucial avenue for sustainable manufacturing. Integration of energy-saving technologies, waste heat recovery systems, and renewable energy sources can contribute to lowering carbon emissions and enhancing overall energy sustainability.
6.2.7.4 Waste minimization and recycling strategies
Addressing waste generation throughout the production lifecycle is paramount. Implementation of waste minimization techniques, recycling initiatives, and closed-loop manufacturing systems can help reduce environmental burden and optimize resource utilization, thereby fostering a more sustainable nanocomposite manufacturing ecosystem.
6.2.7.5 Life cycle assessment and environmental impact analysis
Comprehensive life cycle assessments and environmental impact analyses play a pivotal role in quantifying the ecological footprint of MXene-polymer nanocomposites. By systematically evaluating the environmental consequences of different production pathways, researchers can identify areas for improvement and guide decision-making towards more sustainable practices (F. Zhang et al., 2022).
6.2.7.6 Regulatory compliance and green certification
Adherence to stringent environmental regulations and attainment of green certifications signify essential benchmarks for sustainable nanocomposite production. Compliance with eco-labeling schemes, such as the EU Ecolabel or Green Seal, can validate adherence to environmental standards and enhance market acceptance of green products.
6.2.7.7 Collaborative research and knowledge sharing
Collaboration among academia, industry, and regulatory bodies is instrumental in advancing green synthesis initiatives and fostering knowledge exchange. Interdisciplinary research consortia, public-private partnerships, and collaborative platforms facilitate innovation, accelerate technology transfer, and drive collective progress towards sustainability goals (Payumo et al., 2020).
By prioritizing green synthesis principles and sustainability-driven manufacturing paradigms, the research community aims to usher in a new era of environmentally responsible MXene-polymer nanocomposite production, thereby harmonizing technological progress with ecological stewardship.
6.2.8 Computational modeling and design
The integration of computational modeling and simulation techniques will play a significant role in the rational design and optimization of MXene-polymer nanocomposites. Computational approaches enable predictive modeling of nanocomposite properties, structure-property relationships, and performance under different conditions.
One significant aspect of computational modeling is its ability to explore the effects of different parameters, such as MXene concentration, polymer morphology, and processing conditions, on the final properties of nanocomposites. This predictive capability allows researchers to optimize material composition and processing parameters to achieve desired performance metrics, such as mechanical strength, electrical conductivity, and thermal stability. Moreover, computational modeling facilitates the exploration of novel MXene-polymer combinations and architectures, guiding experimental efforts toward the synthesis of materials with unprecedented properties or functionalities. By simulating the behavior of nanocomposites at the atomic or molecular level, researchers can uncover underlying mechanisms and design principles that govern their performance, leading to informed decision-making and accelerated materials development cycles (Ohno, Esfarjani, and Kawazoe, 2018).
Overall, future research in the field of MXene-polymer nanocomposites will be characterized by interdisciplinary collaborations, innovative approaches, and transformative applications across various industries. By addressing emerging challenges and exploring new frontiers, researchers aim to unlock the full potential of MXene-based materials for addressing societal needs and advancing technological innovation.
7 Conclusion
In conclusion, MXene-polymer nanocomposites hold significant promise in both biomedical fields. These advanced materials offer multifunctional properties, including biocompatibility, tunable surface chemistry, and high adsorption capacity, making them suitable for diverse applications. In biomedicine, MXene-polymer nanocomposites show potential for drug delivery, tissue engineering, biosensors, and medical implants. Ongoing research and development efforts focus on addressing challenges and exploring new frontiers, paving the way for the widespread adoption of MXene-polymer nanocomposites and their transformative impact on healthcare sustainability. In light of the promising potential of MXene-polymer nanocomposites in biomedical applications, it is imperative to emphasize the importance of continued research in this area. By addressing existing challenges, exploring emerging trends, and pushing the boundaries of innovation, researchers can unlock the full capabilities of MXene-polymer nanocomposites. The cost-effectiveness is studied deeply. Therefore, commitment is needed to enhance ongoing collaboration, investment, and exploration in MXene-polymer nanocomposites, as we strive to harness their transformative impact on our society and pave the way for a brighter, more sustainable future.
Data availability statement
The raw data supporting the conclusion of this article will be made available by the authors, without undue reservation.
Author contributions
DP: Writing–review and editing, Writing–original draft, Visualization, Validation, Supervision, Software, Resources, Project administration, Methodology, Investigation, Funding acquisition, Formal Analysis, Data curation, Conceptualization.
Funding
The author(s) declare that no financial support was received for the research, authorship, and/or publication of this article.
Conflict of interest
The author declares that the research was conducted in the absence of any commercial or financial relationships that could be construed as a potential conflict of interest.
Publisher’s note
All claims expressed in this article are solely those of the authors and do not necessarily represent those of their affiliated organizations, or those of the publisher, the editors and the reviewers. Any product that may be evaluated in this article, or claim that may be made by its manufacturer, is not guaranteed or endorsed by the publisher.
References
Aghamohammadi, H., Amousa, N., and Eslami-Farsani, R. (2021). Recent advances in developing the MXene/polymer nanocomposites with multiple properties: a review study. Synth. Met. 273 (March), 116695. doi:10.1016/J.SYNTHMET.2020.116695
Amu-Darko, Jesse Nii Okai Hussain, S., Wang, M., Lei, S., Alothman, A. A., Mohammad, S., Qiao, G., et al. (2024). Advanced 2D nanosheet-based gas sensor for sensitive detection of low concentration NO2 gas using In2O3/Ti3C2 layers. Sensors Actuators B Chem. 407 (May), 135464. doi:10.1016/J.SNB.2024.135464
An, H., Habib, T., Shah, S., Gao, H., Radovic, M., Green, M. J., et al. (2018). Surface-agnostic highly stretchable and bendable conductive MXene multilayers. Sci. Adv. 4 (3), eaaq0118. doi:10.1126/sciadv.aaq0118
Anasori, B., Lukatskaya, M. R., and Gogotsi, Y. (2017). 2D metal carbides and nitrides (MXenes) for energy storage. Nat. Rev. Mater. 2 (2), 16098–16117. doi:10.1038/natrevmats.2016.98
Ashton, M., Mathew, K., Hennig, R. G., and Sinnott, S. B. (2016). Predicted surface composition and thermodynamic stability of MXenes in solution. J. Phys. Chem. C 120 (6), 3550–3556. doi:10.1021/acs.jpcc.5b11887
Aslam, M., Ahmad, T., Husnain Manzoor, M., Verpoort, F., and Verpoort, F. (2023). MXenes as theranostics: diagnosis and therapy including in vitro and in vivo applications. Appl. Mater. Today 35 (December), 102002. doi:10.1016/J.APMT.2023.102002
Bilibana, M. P. (2023). Electrochemical properties of MXenes and applications. Adv. Sens. Energy Mater. 2 (4), 100080. doi:10.1016/J.ASEMS.2023.100080
Boota, M., Anasori, B., Voigt, C., Zhao, M. Q., Barsoum, M. W., and Gogotsi, Y. (2016). Pseudocapacitive electrodes produced by oxidant-free polymerization of pyrrole between the layers of 2D titanium carbide (MXene). Adv. Mater. 28 (7), 1517–1522. doi:10.1002/ADMA.201504705
Boys, C. V. (1887). On the production, properties, and some suggested uses of the finest threads. Proc. Phys. Soc. Lond. 9 (1), 8–19. doi:10.1088/1478-7814/9/1/303
Byrappa, K., and Yoshimura, M. (2013). Handbook of hydrothermal technology | 978-0-8155-1445-9. Elsevier, 779. Available at: https://books.google.com/books/about/Handbook_of_Hydrothermal_Technology.html?id=vA5tXzLsHioC.
Carey, M., and Barsoum, M. W. (2021). MXene polymer nanocomposites: a review. Mater. Today Adv. 9 (March), 100120. doi:10.1016/J.MTADV.2020.100120
Charitidis, C. A., Georgiou, P., Koklioti, M. A., Trompeta, A. F., and Markakis, V. (2014). Manufacturing nanomaterials: from research to industry. Manuf. Rev. 1, 11. doi:10.1051/MFREVIEW/2014009
Chen, C., Boota, M., Xie, X., Zhao, M., Anasori, B., Ren, C. E., et al. (2017). Charge transfer induced polymerization of EDOT confined between 2D titanium carbide layers. J. Mater. Chem. A 5 (11), 5260–5265. doi:10.1039/c7ta00149e
Chen, J., Chen, Ke, Tong, D., Huang, Y., Zhang, J., Xue, J., et al. (2014). CO2 and temperature dual responsive ‘smart’ MXene phases. Chem. Commun. 51 (2), 314–317. doi:10.1039/C4CC07220K
Chen, Ke, Qiu, N., Deng, Q., Kang, M.Ho, Yang, H., Baek, J.Uk, et al. (2017). Cytocompatibility of Ti3AlC2, Ti3SiC2, and Ti2AlN: in vitro tests and first-principles calculations. ACS Biomaterials Sci. Eng. 3 (10), 2293–2301. doi:10.1021/ACSBIOMATERIALS.7B00432
Chen, X., Zhao, Y., Li, L., Wang, Y., Wang, J., Xiong, J., et al. (2020). MXene/polymer nanocomposites: preparation, properties, and applications. Polym. Rev. 0 (0), 80–115. doi:10.1080/15583724.2020.1729179
Cui, T., Cai, W., Zheng, Y., Wang, J., Lin, B., Zhou, G., et al. (2023). Unlocking interfacial dynamics of biocompatible MXene/polyurethane-based nanocomposites for photothermal de-icing and thermal safety. Chem. Eng. J. 473 (October), 145357. doi:10.1016/J.CEJ.2023.145357
Dai, C., Chen, Yu, Jing, X., Xiang, L., Yang, D., Lin, H., et al. (2017). Two-dimensional tantalum carbide (MXenes) composite nanosheets for multiple imaging-guided photothermal tumor ablation. ACS Nano 11 (12), 12696–12712. doi:10.1021/acsnano.7b07241
Dai, C., Lin, H., Xu, G., Liu, Z., Wu, R., and Chen, Yu (2017). Biocompatible 2D titanium carbide (MXenes) composite nanosheets for PH-responsive MRI-guided tumor hyperthermia. Chem. Mater. 29 (20), 8637–8652. doi:10.1021/acs.chemmater.7b02441
Dai, Yu, Chen, X., and Zhang, X. (2018). Recent advances in stimuli-responsive polymeric micelles via click chemistry. Polym. Chem. 10 (1), 34–44. doi:10.1039/C8PY01174E
Darroudi, M., Elnaz Nazari, S., Karimzadeh, M., Asgharzadeh, F., Zahra Asghari, S., Khalili-Tanha, N., et al. (2023). Fabrication of magnetic nanocomposite as responsive drug delivery vehicle for cervical cancer therapy. Appl. Organomet. Chem. 37 (6), e7068. doi:10.1002/AOC.7068
Demazeau, G. (2008). Solvothermal reactions: an original route for the synthesis of novel materials. J. Mater. Sci. 43 (7), 2104–2114. doi:10.1007/s10853-007-2024-9
Directorate, E., Meeting, J., and The, O. F. (2015). Chemicals committee, T H E working, party on, current developments, I N delegations, O N the, and safety of 2015. 12. Oecd_–60. United States.
Feng, A., Hou, T., Jia, Z., Zhang, Yi, Zhang, F., and Wu, G. (2020). Preparation and characterization of epoxy resin filled with Ti3C2Tx MXene nanosheets with excellent electric conductivity. Nanomaterials 10 (1), 162. doi:10.3390/NANO10010162
Feng, H., Wang, W., Zhang, M., Zhu, S., and Wang, Q. (2020). 2D titanium carbide-based nanocomposites for photocatalytic bacteriostatic applications. in Applied catalysis B. Elsevier.
Feng, Y., Wang, H., Xu, J., Du, X., Cheng, Xu, Du, Z., et al. (2021). Fabrication of MXene/PEI functionalized sodium alginate aerogel and its excellent adsorption behavior for Cr(VI) and Congo red from aqueous solution. J. Hazard. Mater. 416 (August), 125777. doi:10.1016/J.JHAZMAT.2021.125777
Foroutan, B. (2015). Personalized medicine: a review with regard to biomarkers. J. Bioequivalence Bioavailab. 7 (6), 0. doi:10.4172/JBB.1000248
Forrest, S. R. (2004). The path to ubiquitous and low-cost organic electronic appliances on plastic.
Foulkes, R., Man, E., Thind, J., Yeung, S., Joy, A., and Hoskins, C. (2020). The regulation of nanomaterials and nanomedicines for clinical application: current and future perspectives. Biomaterials Sci. 8 (17), 4653–4664. doi:10.1039/D0BM00558D
Gao, L., Li, C., Huang, W., Mei, S., Lin, H., Qi, Ou, et al. (2020). MXene/polymer membranes: synthesis, properties, and emerging applications. Chem. Mater. 32 (5), 1703–1747. doi:10.1021/acs.chemmater.9b04408
Garg, N., and Ahmad, F. J. (2022). Biomedical applications of MXene/polymer nanocomposites. MXene-Filled Polym. Nanocomposites, 247–258. doi:10.1201/9781003164975-13
Geng, De C., Zhao, X.Xu, Chen, Z. X., Sun, W. W., Fu, W., Chen, J.Yi, et al. (2017). Direct synthesis of large-area 2D Mo2 C on in situ grown graphene. Adv. Mater. Deerf. Beach, Fla. 29 (35). doi:10.1002/ADMA.201700072
George, S. M., and Kandasubramanian, B. (2020). Advancements in MXene-polymer composites for various biomedical applications. Ceram. Int. 46, 8522–8535. doi:10.1016/j.ceramint.2019.12.257
George, S. M., Tandon, S., and Kandasubramanian, B. (2020). Advancements in hydrogel-functionalized immunosensing platforms. ACS Omega 5 (5), 2060–2068. doi:10.1021/acsomega.9b03816
Ghidiu, M., Lukatskaya, M. R., Zhao, M.-Q., Gogotsi, Y., and Barsoum, M. W. (2014). Conductive two-dimensional titanium carbide ‘clay’ with high volumetric capacitance. doi:10.1038/nature13970
Gogotsi, Y. (2015). Transition metal carbides go 2D. Nat. Mater. 14 (11), 1079–1080. doi:10.1038/nmat4386
Gomez, I. J., Arnaiz, B., Cacioppo, M., Arcudi, F., and Prato, M. (2018). Nitrogen-doped carbon nanodots for bioimaging and delivery of paclitaxel. J. Mater. Chem. B 6 (35), 5540–5548. doi:10.1039/c8tb01796d
Gong, K., Zhou, K., Qian, X., Shi, C., and Yu, B. (2021). MXene as emerging nanofillers for high-performance polymer composites: a review. Compos. Part B Eng. 217 (July), 108867. doi:10.1016/J.COMPOSITESB.2021.108867
Guo, Y., Zhong, M., Fang, Z., Wan, P., and Yu, G. (2019). A wearable transient pressure sensor made with MXene nanosheets for sensitive broad-range human-machine interfacing. Nano Lett. 19 (2), 1143–1150. doi:10.1021/ACS.NANOLETT.8B04514
Halim, J., Cook, K. M., Naguib, M., Gogotsi, Y., Rosén, J., Barsoum, M., et al. (2016). X-ray photoelectron spectroscopy of select multi-layered transition metal carbides (MXenes). Appl. Surf. Sci. 362, 406–417. doi:10.1016/j.apsusc.2015.11.089
Halim, J., Kota, S., Lukatskaya, M. R., Naguib, M., Zhao, M. Q., Ju Moon, E., et al. (2016). Synthesis and characterization of 2D molybdenum carbide (MXene). Adv. Funct. Mater. 26 (18), 3118–3127. doi:10.1002/adfm.201505328
Halim, J., Lukatskaya, M. R., Cook, K. M., Lu, J., Smith, C. R., Åke Näslund, L.-Å., et al. (2014). Transparent conductive two-dimensional titanium carbide epitaxial thin films. Chem. Mater. 7 (26), 2374–2381. doi:10.1021/cm500641a
Hamzan, N. binti, Yi Bin Ng, C., Rad, S., Lee, M. K., Chang, L. J., Tripathi, M., et al. (2021). Controlled physical properties and growth mechanism of manganese silicide nanorods. J. Alloys Compd. 851 (January), 156693. doi:10.1016/J.JALLCOM.2020.156693
Han, R., Ma, X., Xie, Y., Teng, Da, and Zhang, S. (2017). Preparation of a new 2D MXene/PES composite membrane with excellent hydrophilicity and high flux. RSC Adv. 7 (89), 56204–56210. doi:10.1039/C7RA10318B
Han, X., Huang, Ju, Lin, H., Wang, Z., Pan, Li, and Chen, Yu (2018). 2D ultrathin MXene-based drug-delivery nanoplatform for synergistic photothermal ablation and chemotherapy of cancer. Adv. Healthc. Mat. 7 (9), 1701394. doi:10.1002/adhm.201701394
Harper, A., and Anderson, M. R. (2010). Electrochemical glucose sensors—developments using electrostatic assembly and carbon nanotubes for biosensor construction. Sensors 10 (9), 8248–8274. doi:10.3390/s100908248
Harris, K. J., Bugnet, M., Naguib, M., Barsoum, M. W., and Goward, G. R. (2015). Direct measurement of surface termination groups and their connectivity in the 2D MXene V2CTx using NMR spectroscopy. J. Phys. Chem. C 119 (24), 13713–13720. doi:10.1021/acs.jpcc.5b03038
Hatakeyama, H. (2017). Recent advances in endogenous and exogenous stimuli-responsive nanocarriers for drug delivery and therapeutics. Chem. Pharm. Bull. 65 (7), 612–617. doi:10.1248/CPB.C17-00068
Hatter, C. B., Shah, J., Anasori, B., and Gogotsi, Y. (2020). Micromechanical response of two-dimensional transition metal carbonitride (MXene) reinforced epoxy composites. Compos. Part B Eng. 182 (February), 107603. doi:10.1016/J.COMPOSITESB.2019.107603
He, J., Zou, H., Zhou, J., and Deng, C. (2024). Thermoresponsive MXene-based hydrogel for controlled anticancer drug release. J. Drug Deliv. Sci. Technol. 91 (January), 105207. doi:10.1016/J.JDDST.2023.105207
Hohenstein, W. P., and Mark, H. (1946). Polymerization of olefins and diolefins in suspension and emulsion. Part I. J. Polym. Sci. 1 (2), 127–145. doi:10.1002/POL.1946.120010207
Hong, W., Wyatt, B. C., Nemani, S. K., and Anasori, B. (2020). Double transition-metal MXenes: atomistic design of two-dimensional carbides and nitrides. MRS Bull. 45 (10), 850–861. doi:10.1557/mrs.2020.251
Hu, D., Huang, X., Li, S., and Jiang, P. (2020). Flexible and durable cellulose/MXene nanocomposite paper for efficient electromagnetic interference shielding. Compos. Sci. Technol. 188 (March), 107995. doi:10.1016/J.COMPSCITECH.2020.107995
Hu, Po, Li, J., Jin, J., Lin, X., and Tan, X. (2022). Highly sensitive photopolymer for holographic data storage containing methacryl polyhedral oligomeric silsesquioxane. ACS Appl. Mater. Interfaces 14, 21544–21554. doi:10.1021/acsami.2c04011
Hu, T., Wang, J., Zhang, H., Li, Z., Hu, M., and Wang, X. (2015). Vibrational properties of Ti3C2 and Ti3C2T2 (T = O, F, OH) monosheets by first-principles calculations: a comparative study. Phys. Chem. Chem. Phys. 17 (15), 9997–10003. doi:10.1039/c4cp05666c
Huang, K., Li, Z., Lin, J., Han, G., and Huang, P. (2018). Two-dimensional transition metal carbides and nitrides (MXenes) for biomedical applications. Chem. Soc. Rev. 47, 5109–5124. doi:10.1039/c7cs00838d
Huang, S., and Mochalin, V. N. (2019). Hydrolysis of 2D transition-metal carbides (MXenes) in colloidal solutions. Inorg. Chem. 58 (3), 1958–1966. doi:10.1021/acs.inorgchem.8b02890
Huang, X., Wang, R., Jiao, T., Zou, G., Zhan, F., Yin, J., et al. (2019). Facile preparation of hierarchical AgNP-loaded MXene/Fe3O4 polymer nanocomposites by electrospinning with enhanced catalytic performance for wastewater treatment. ACS Omega 4 (1), 1897–1906. doi:10.1021/acsomega.8b03615
Islam, S., and Syed Shazali, S. T. (2011). Determinants of manufacturing productivity: pilot study on labor-intensive industries. Int. J. Prod. Perform. Manag. 60 (6), 567–582. doi:10.1108/17410401111150751
Jain, A., Ong, S. P., Hautier, G., Chen, W., Richards, W. D., Dacek, S., et al. (2013). Commentary: the materials Project: a materials genome approach to accelerating materials innovation. Apl. Mater. 1 (1). doi:10.1063/1.4812323
Jeon, J., Park, Y., Choi, S., Lee, J., Lim, S. S., Lee, B. H., et al. (2018). Epitaxial synthesis of molybdenum carbide and Formation of a Mo2C/MoS2 hybrid structure via chemical conversion of molybdenum disulfide. ACS Nano 12 (1), 338–346. doi:10.1021/acsnano.7b06417
Jiang, F., and Doudna, J. A. (2017). CRISPR-Cas9 structures and mechanisms. Annu. Rev. Biophys. 46, 505–529. doi:10.1146/annurev-biophys-062215-010822
Jiang, C., Wu, C., Li, X., Yao, Y., Lan, L., Zhao, F., et al. (2019). All-electrospun flexible triboelectric nanogenerator based on metallic MXene nanosheets. Nano Energy 59 (May), 268–276. doi:10.1016/J.NANOEN.2019.02.052
Jiang, Y., Zhang, L., Wang, S., and Chen, Y. (2021). MXene-polymer nanocomposites for ultrasensitive detection of circulating tumor DNA in blood samples. J. Nanobiotechnology 19 (1), 191. doi:10.1186/s12951-021-00955-2
Jiménez, M., Romero, L., Domínguez, I. A., Del Mar Espinosa, M., and Domínguez, M. (2019). Additive manufacturing technologies: an overview about 3D printing methods and future prospects. Complexity 2019, 1–30. doi:10.1155/2019/9656938
Jimmy, J., and Kandasubramanian, B. (2020). Mxene functionalized polymer composites: synthesis and applications. Eur. Polym. J. 122 (January), 109367. doi:10.1016/j.eurpolymj.2019.109367
Jin, Y., Fan, Y., Meng, X., Li, J., Li, C., Sunarso, J., et al. (2021). Modeling of hydrated cations transport through 2D MXene (Ti3C2Tx) membranes for water purification. J. Membr. Sci. 631 (August), 119346. doi:10.1016/J.MEMSCI.2021.119346
Jolly, S., Paranthaman, M. P., and Naguib, M. (2021). Synthesis of Ti3C2Tz MXene from low-cost and environmentally friendly precursors. Mater. Today Adv. 10, 100139. doi:10.1016/j.mtadv.2021.100139
Journal, O., and Ondokuzmay, M. E. (2020). Regulatory policies for safety of nanomaterials nanomalzemelerin guvenligi ile, 1–16.
Karimi, M., Zangabad, P. S., Ghasemi, A., Amiri, M., Bahrami, M., Malekzad, H., et al. (2016). Temperature-responsive smart nanocarriers for delivery of therapeutic agents: applications and recent advances. ACS Appl. Mater. Interfaces 8 (33), 21107–21133. doi:10.1021/acsami.6b00371
Kedambaimoole, V., Kumar, N., Shirhatti, V., Nuthalapati, S., Sen, P., Nayak, M. M., et al. (2020). Laser-induced direct patterning of free-standing Ti3C2-MXene films for skin conformal tattoo sensors. ACS Sensors 5 (7), 2086–2095. doi:10.1021/acssensors.0c00647
Keirouz, A., Radacsi, N., Ren, Q., Dommann, A., Beldi, G., Maniura-Weber, K., et al. (2020). Nylon-6/Chitosan core/shell antimicrobial nanofibers for the prevention of mesh-associated surgical site infection. J. Nanobiotechnology 18 (1), 51–17. doi:10.1186/s12951-020-00602-9
Khatri, M., Bello, D., Pal, A. K., Cohen, J. M., Woskie, S., Gassert, T., et al. (2013). Evaluation of cytotoxic, genotoxic and inflammatory responses of nanoparticles from photocopiers in three human cell lines. Part. Fibre Toxicol. 10 (1), 42–22. doi:10.1186/1743-8977-10-42
Khazaei, M., Arai, M., Sasaki, T., Chung, C. Y., Venkataramanan, N. S., Estili, M., et al. (2013). Novel electronic and magnetic properties of two-dimensional transition metal carbides and nitrides. Adv. Funct. Mater. 23 (17), 2185–2192. doi:10.1002/adfm.201202502
Khazaei, M., Mishra, A., Venkataramanan, N. S., Singh, A. K., and Yunoki, S. (2019). Recent advances in MXenes: from fundamentals to applications. Curr. Opin. Solid State Mater. Sci. 23 (3), 164–178. doi:10.1016/J.COSSMS.2019.01.002
Koc, R., and Kodambaka, S. K. (2000). Tungsten carbide (WC) synthesis from novel precursors. J. Eur. Ceram. Soc. 20 (11), 1859–1869. doi:10.1016/S0955-2219(00)00038-8
Kong, C., and Chen, X. (2022). Combined photodynamic and photothermal therapy and immunotherapy for cancer treatment: a review. Int. J. Nanomedicine 17, 6427–6446. doi:10.2147/IJN.S388996
Koo, J. H. (2019). Polymer nanocomposites: processing, characterization, and applications. Second Edi. Access Engineering - York University. United States: McGraw Hill.
Kumar, R., Barman, P. B., and Singh, R. R. (2021). An innovative direct non-aqueous method for the development of Co doped Ni-Zn ferrite nanoparticles. Mater. Today Commun. 27 (June), 102238. doi:10.1016/J.MTCOMM.2021.102238
Kumari, R., Suman, K., Karmakar, S., Mishra, V., Lakra, S. G., Saurav, G. K., et al. (2023). Regulation and safety measures for nanotechnology-based agri-products. Front. Genome Ed. 5 (June), 1–12. doi:10.3389/fgeed.2023.1200987
Kurra, N., Hota, M. K., and Alshareef, H. N. (2015). Conducting polymer micro-supercapacitors for flexible energy storage and Ac line-filtering. Nano Energy 13 (April), 500–508. doi:10.1016/j.nanoen.2015.03.018
Kurra, N., Jiang, Q., and Alshareef, H. N. (2015). A general strategy for the fabrication of high performance microsupercapacitors. Nano Energy 16 (September), 1–9. doi:10.1016/j.nanoen.2015.05.031
Lele, V. P. (2023). Influence of supply chain factors on material science and its developments. Int. J. Industrial Eng. 10 (2), 1–7. doi:10.14445/23499362/IJIE-V10I2P101
Li, D., and Xia, Y. (2004). Electrospinning of nanofibers: reinventing the wheel? Adv. Mater. 16 (14), 1151–1170. doi:10.1002/ADMA.200400719
Li, H., Li, T., Wu, Q., Wang, R., Hong, R., and Li, Y. (2021). Super stable water-based magnetic fluid as a dual-mode contrast agent. Nanotechnol. Rev. 10 (1), 1031–1045. doi:10.1515/ntrev-2021-0068
Li, J., Levitt, A., Kurra, N., Juan, K., Noriega, N., Xiao, Xu, et al. (2019). MXene-conducting polymer electrochromic microsupercapacitors. Energy Storage Mater. 20 (July), 455–461. doi:10.1016/J.ENSM.2019.04.028
Li, X., Wang, Y., Zhang, L., and Chen, Z. (2021). Comprehensive biocompatibility assessments of MXene-polymer nanocomposites for biomedical applications. ACS Appl. Mater. Interfaces 13 (17), 19607–19617. doi:10.1021/acsami.1c03192
Li, X., Zhao, Y., Wu, L., Li, G., Xu, L., and Ye, B. (2020). Highly ordered 3D electrochemical DNA biosensor based on dual orientation controlled rolling motor and graftable tetrahedron DNA. Biosens. Bioelectron. 147, 111759. doi:10.1016/j.bios.2019.111759
Li, X. P., Yue, Li, Li, X., Song, D., Peng, M., Hu, C., et al. (2019). Highly sensitive, reliable and flexible piezoresistive pressure sensors featuring polyurethane sponge coated with MXene sheets. J. Colloid Interface Sci. 542 (April), 54–62. doi:10.1016/J.JCIS.2019.01.123
Li, Z., Zhang, H., Han, J., Chen, Yu, Lin, H., and Yang, T. (2018). Surface nanopore engineering of 2D MXenes for targeted and synergistic multitherapies of hepatocellular carcinoma. Adv. Mater. 30 (25), 1706981. doi:10.1002/adma.201706981
Liao, H., Guo, X., Wan, P., and Yu, G. (2019). Conductive MXene nanocomposite organohydrogel for flexible, healable, low-temperature tolerant strain sensors. Adv. Funct. Mater. 29 (39), 1904507. doi:10.1002/ADFM.201904507
Lin, H., Chen, Yu, and Shi, J. (2018). Insights into 2D MXenes for versatile biomedical applications: current advances and challenges ahead. Adv. Sci. 5 (10), 1800518. doi:10.1002/advs.201800518
Lin, H., Gao, S., Chen, D., Chen, Yu, and Shi, J. (2017). A two-dimensional biodegradable niobium carbide (MXene) for photothermal tumor eradication in NIR-I and NIR-II biowindows. J. Am. Chem. Soc. 139 (45), 16235–16247. doi:10.1021/jacs.7b07818
Lin, H., Wang, X., Yu, L., Chen, Yu, and Shi, J. (2017). Two-dimensional ultrathin MXene ceramic nanosheets for photothermal conversion. Nano Lett. 17 (1), 384–391. doi:10.1021/acs.nanolett.6b04339
Lin, H., Wang, Y., Gao, S., Chen, Yu, and Shi, J. (2018). Theranostic 2D tantalum carbide (MXene). Adv. Mater. 30 (4), 1703284. doi:10.1002/adma.201703284
Ling, Z., Ren, C. E., Zhao, M. Q., Yang, J., Giammarco, J. M., Qiu, J., et al. (2014). Flexible and conductive MXene films and nanocomposites with high capacitance. Proc. Natl. Acad. Sci. U. S. A. 111 (47), 16676–16681. doi:10.1073/pnas.1414215111
Lipatov, A., Mohamed, A., Lukatskaya, M. R., Boson, A., Gogotsi, Y., and Sinitskii, A. (2016). Effect of synthesis on quality, electronic properties and environmental stability of individual monolayer Ti3C2 MXene flakes. Adv. Electron. Mater. 2 (12), 1600255. doi:10.1002/AELM.201600255
Liu, A., Liu, Y., Liu, G., Zhang, A., Cheng, Y., Li, Y., et al. (2022). Engineering of surface modified Ti3C2Tx MXene based dually controlled drug release system for synergistic multitherapies of cancer. Chem. Eng. J. 448 (November), 137691. doi:10.1016/J.CEJ.2022.137691
Liu, X., Feng, Y., Xu, J., Shi, Y., Yang, J., Zhang, R., et al. (2021). Combination of MAPK inhibition with photothermal therapy synergistically augments the anti-tumor efficacy of immune checkpoint blockade. J. Control. Release 332 (April), 194–209. doi:10.1016/J.JCONREL.2021.02.020
Liu, Z., Lin, H., Zhao, M., Chen, D., Zhang, S., Peng, W., et al. (2018). 2D superparamagnetic tantalum carbide composite MXenes for efficient breast-cancer theranostics. Theranostics 8 (6), 1648–1664. doi:10.7150/thno.23369
Lopes, J. R., Santos, G., Barata, P., Oliveira, R., and Lopes, C. M. (2013). Physical and chemical stimuli-responsive drug delivery systems: targeted delivery and main routes of administration. Curr. Pharm. Des. 19 (14): 7169–7184. doi:10.2174/13816128113199990698
Lukatskaya, M. R., Mashtalir, O., Ren, C. E., Dall’Agnese, Y., Rozier, P., Louis Taberna, P., et al. (2013). Cation intercalation and high volumetric capacitance of two-dimensional titanium carbide. Science 341 (6153), 1502–1505. doi:10.1126/science.1241488
Ma, L., Zhu, C., Chen, C., Meng, X., Chen, L., and Wang, H. (2021). MXene-polymer hydrogels as injectable scaffolds for cartilage repair. Biomaterials Sci. 9 (14): 4948–4958. doi:10.1039/D1BM00349G
Maleski, K., Mochalin, V. N., and Gogotsi, Y. (2017). Dispersions of two-dimensional titanium carbide MXene in organic solvents. Chem. Mater. 29 (4), 1632–1640. doi:10.1021/acs.chemmater.6b04830
Maleski, K., Ren, C. E., Zhao, M. Q., Anasori, B., and Gogotsi, Y. (2018). Size-dependent physical and electrochemical properties of two-dimensional MXene flakes. ACS Appl. Mater. Interfaces 10 (29), 24491–24498. doi:10.1021/acsami.8b04662
Manawi, Y., Ihsanullah, A. S., Al-Ansari, T., Atieh, M., and Atieh, M. (2018). A review of carbon nanomaterials’ synthesis via the chemical vapor deposition (CVD) method. Materials 11 (5), 822. doi:10.3390/ma11050822
Manjushree, S. G., Prashanth, S., Adarakatti, P. S., Almalki, A. S. A., and Alhadhrami, A. (2022). “MXenes-based polymer composites for bioelectronics,” in Bioelectronics, 237–250. doi:10.1201/9781003263265-15
Mashtalir, O., Naguib, M., Mochalin, V. N., Dall’Agnese, Y., Heon, M., Barsoum, M. W., et al. (2013). Intercalation and delamination of layered carbides and carbonitrides. Nat. Commun. 4, 1716–1717. doi:10.1038/ncomms2664
McCrum, N. G., Buckley, C. P., and Bucknall, C. B. (1997). The elastic properties of rubber. Princ. Polym. Eng., 84–116.
McKeen, L. W. (2017). “High-temperature/high-performance polymers,” in Film properties of plastics and elastomers (Elsevier), 389–417. doi:10.1016/b978-0-12-813292-0.00012-5
Meng, C., Maeng, J., John, S. W. M., and Irazoqui, P. P. (2014). Ultrasmall integrated 3D micro-supercapacitors solve energy storage for miniature devices. Adv. Energy Mater. 4 (7), 1301269. doi:10.1002/aenm.201301269
Merritt, S. R., Exner, A. A., Lee, Z., and Von Recum, H. A. (2012). Electrospinning and imaging. Adv. Eng. Mater. 14 (5), B266–B278. doi:10.1002/ADEM.201180010
Mirkhani, S. A., Zeraati, A. S., Aliabadian, E., Naguib, M., and Sundararaj, U. (2019). High dielectric constant and low dielectric loss via poly(vinyl alcohol)/Ti3C2Tx MXene nanocomposites. ACS Appl. Mater. Interfaces 11 (20), 18599–18608. doi:10.1021/acsami.9b00393
Mohajer, F., Mohammadi Ziarani, G., Badiei, A., Iravani, S., and Varma, R. S. (2022). Advanced MXene-based micro- and nanosystems for targeted drug delivery in cancer therapy. Micromachines 13 (10), 1773. doi:10.3390/MI13101773
Naguib, M., Kurtoglu, M., Presser, V., Lu, J., Niu, J., Heon, M., et al. (2011). Two-dimensional nanocrystals produced by exfoliation of Ti 3AlC 2. Adv. Mater. 23 (37), 4248–4253. doi:10.1002/adma.201102306
Naguib, M., Mashtalir, O., Carle, J., Presser, V., Lu, J., Hultman, L., et al. (2012). Two-dimensional transition metal carbides. ACS Nano 6 (2), 1322–1331. doi:10.1021/nn204153h
Naguib, M., Mochalin, V. N., Barsoum, M. W., and Gogotsi, Y. (2014). 25th anniversary article: MXenes: a new family of two-dimensional materials. Adv. Mater. 26 (7), 992–1005. doi:10.1002/adma.201304138
Naguib, M., Saito, T., Lai, S., Rager, M. S., Aytug, T., Parans Paranthaman, M., et al. (2016). Ti3C2Tx (MXene)–Polyacrylamide nanocomposite films. RSC Adv. 6 (76), 72069–72073. doi:10.1039/C6RA10384G
Nikazar, S., Mofidi, Z., and Mortazavi, M. (2023). Cancer theranostic applications of MXenes. ACS Symp. Ser. 1443 (September), 19–46. doi:10.1021/BK-2023-1443.CH002
Ohno, K., Esfarjani, K., and Kawazoe, Y. (2018). Computational materials science. Comput. Mater. Sci. doi:10.1007/978-3-662-56542-1
Oyedotun, K. O., Madito, M. J., Momodu, D. Y., Mirghni, A. A., Masikhwa, T. M., and Manyala, N. (2009). Synthesis of ternary NiCo-MnO2 nanocomposite and its application as a novel high energy supercapattery device. Chem. Eng. J. 335 (March), 416–433. doi:10.1016/J.CEJ.2017.10.169
Painter, P. C., and Coleman, M. M. (2019). Fundamentals of polymer science: an introductory text. Fundam. Polym. Sci. doi:10.1201/9780203755211
Parajuli, D., Devendra, K. C., Reda, T. G., Sravani, G. M., Murali, N., and Samatha, K. (2021). RHEED analysis of the oxidized M′2M″xXyene sheets by ablated plasma thrust method in pulsed laser deposition chamber. AIP Adv. 11 (11), 115019. doi:10.1063/5.0068659
Parajuli, D., Kaphle, G. C., and Samatha, K. (2019). First-principles study of electronic and magnetic properties of anatase and its role in anatase-mxene nanocomposite. J. Nepal Phys. Soc. 5 (1), 42–53. doi:10.3126/JNPHYSSOC.V5I1.26940
Parajuli, D., Murali, N., Devendra, K. C., Karki, B., Samatha, K., Kim, A. A., et al. (2022). Advancements in MXene-polymer nanocomposites in energy storage and biomedical applications. Polymers 14 (16), 3433. doi:10.3390/POLYM14163433
Parajuli, D., Murali, N., Raghavendra, V., Suryanarayana, B., Batoo, K. M., and Samatha, K. (2023). Investigation of structural, morphological and magnetic study of Ni–Cu-substituted Li0.5Fe2.5O4 Ferrites. Appl. Phys. A 129 (7), 502–512. doi:10.1007/S00339-023-06772-1
Parajuli, D., Murali, N., Samatha, K., Shah, N. L., and Sharma, B. R. (2023). Structural, morphological, and textural properties of coprecipitated CaTiO3 for anion exchange in the electrolyzer. J. Nepal Phys. Soc. 9 (1), 137–142. doi:10.3126/JNPHYSSOC.V9I1.57751
Parajuli, D., Murali, N., Samatha, K., and Veeraiah, V. (2022). Thermal, structural, morphological, functional group and first cycle charge/discharge study of Co substituted LiNi1−x-0.02Mg0.02CoxO2 (x = 0.00, 0.02, 0.04, 0.06, and 0.08) cathode material for LIBs. AIP Adv. 12 (8), 085010. doi:10.1063/5.0096297
Parajuli, D., Murali, N., Venkateswara Rao, A., Ramakrishna, A., Yonatan, M. S., and Samatha, K. (2022). Structural, dc electrical resistivity and magnetic investigation of Mg, Ni, and Zn substituted Co-Cu nano spinel Ferrites. South Afr. J. Chem. Eng. 42 (October), 106–114. doi:10.1016/J.SAJCE.2022.07.009
Parajuli, D., Raghavendra, V., Suryanarayana, B., Rao, P. A., Murali, N., Phanidhar Varma, P. V. S. K., et al. (2021). Corrigendum to ‘cadmium substitution effect on structural, electrical and magnetic properties of Ni-Zn nano Ferrites. Phys 19 (2020), 2211–2379. doi:10.1016/J.RINP.2021.103947
Parajuli, D., and Samatha, K. (2019). MXene as topological insulator. JETIR 6 (4), 689–706. doi:10.1729/Journal.23872
Parajuli, D., and Samatha, K. (2022). “Topological properties of MXenes,” in MXenes and their composites (Elsevier). doi:10.1016/B978-0-12-823361-0.00015-0
Parajuli, D., Taddesse, P., Murali, N., and Samatha, K. (2022). Correlation between the structural, magnetic, and dc resistivity properties of Co0.5m0.5-XCuxFe2O4 (M = Mg, and Zn) nano Ferrites. Appl. Phys. A Mater. Sci. Process. 128 (1), 58–59. doi:10.1007/s00339-021-05211-3
Parajuli, D., Taddesse, P., Murali, N., Veeraiah, V., and Samatha, K. (2022). Effect of Zn2+ doping on thermal, structural, morphological, functional group, and electrochemical properties of layered LiNi0.8Co0.1Mn0.1O2 cathode material. AIP Adv. 12 (12), 125012. doi:10.1063/5.0122976
Parajuli, D., Uppugalla, S., Murali, N., Ramakrishna, A., Suryanarayana, B., and Samatha, K. (2023). Synthesis and characterization MXene-ferrite nanocomposites and its application for dying and shielding. Inorg. Chem. Commun. 148 (February), 110319. doi:10.1016/J.INOCHE.2022.110319
Payumo, J., He, G., Chintamani Manjunatha, A., Higgins, D., and Calvert, S. (2020). Mapping collaborations and partnerships in SDG research. Front. Res. Metrics Anal. 5 (February), 612442. doi:10.3389/frma.2020.612442
Perera, A. A. P. R., Madhushani, K. A. U., Punchihewa, B. T., Kumar, A., and Gupta, R. K. (2023). MXene-based nanomaterials for multifunctional applications. Materials 16 (3), 1138. Page 1138 16. doi:10.3390/MA16031138
Pinto, G. M., Cremonezzi, J. M. O., Ribeiro, H., Andrade, R. J. E., Demarquette, N. R., and Fechine, G. J. M. (2023). From two-dimensional materials to polymer nanocomposites with emerging multifunctional applications: a critical review. Polym. Compos. 44 (3), 1438–1470. doi:10.1002/PC.27213
Prajapati, D. G., and Balasubramanian, K. (2019). Progress in the development of intrinsically conducting polymer composites as biosensors. Macromol. Chem. Phys. 220 (10), 1800561. doi:10.1002/macp.201800561
Qin, L., Tao, Q., Liu, X., Fahlman, M., Halim, J., Persson, P. O. Å., et al. (2019). Polymer-MXene composite films formed by MXene-facilitated electrochemical polymerization for flexible solid-state microsupercapacitors. Nano Energy 60 (April), 734–742. doi:10.1016/j.nanoen.2019.04.002
Qiu, Zi M., Yang, B., Gao, Yi D., Liu, C.Li, Yue, Ru, Pi, Ye C., et al. (2021). MXenes nanocomposites for energy storage and conversion. Rare Met. 41 (4), 1101–1128. doi:10.1007/S12598-021-01876-0
Rabenau, A. (1985). The role of hydrothermal synthesis in preparative chemistry. Angewandte Chemie Int. Ed. Engl. 24 (12), 1026–1040. doi:10.1002/ANIE.198510261
Rakhi, R. B., Nayuk, P., Xia, C., and Alshareef, H. N. (2016). Novel amperometric glucose biosensor based on MXene nanocomposite. Sci. Rep. 6 (1), 1–10. doi:10.1038/srep36422
Rasid, Z. A. M., Omar, M. F., Nazeri, M. F. M., A’Ziz, M. A. A., and Szota, M. (2017). Low cost synthesis method of two-dimensional titanium carbide MXene. IOP Conf. Ser. Mater. Sci. Eng. 209 (1), 012001. doi:10.1088/1757-899X/209/1/012001
Rasool, K., Mahmoud, K. A., Johnson, D. J., Helal, M., Berdiyorov, G. R., and Gogotsi, Y. (2017). Efficient antibacterial membrane based on two-dimensional Ti3C2Tx (MXene) nanosheets. Sci. Rep. 2017 7 (1), 1–11. doi:10.1038/s41598-017-01714-3
Reese, C., Roberts, M., Ling, M. M., and Bao, Z. (2004). Organic thin film transistors. Mater. Today 7 (9), 20–27. doi:10.1016/S1369-7021(04)00398-0
Ren, X., Huo, M., Wang, M., Lin, H., Zhang, X., Yin, J., et al. (2019). Highly catalytic niobium carbide (MXene) promotes hematopoietic recovery after radiation by free radical scavenging. ACS Nano 13, 6438–6454. doi:10.1021/acsnano.8b09327
Ren, Y., Zhu, J., Wang, L., Liu, H., Liu, Yi, Wu, W., et al. (2018). Synthesis of polyaniline nanoparticles deposited on two-dimensional titanium carbide for high-performance supercapacitors. Mater. Lett. 214 (March), 84–87. doi:10.1016/J.MATLET.2017.11.060
Riazi, H., Nemani, S. K., Grady, M. C., Anasori, B., and Soroush, M. (2021). Ti3C2 MXene–polymer nanocomposites and their applications. J. Mater. Chem. A 9 (13), 8051–8098. doi:10.1039/D0TA08023C
Sang, X., Xie, Yu, Yilmaz, D. E., Lotfi, R., Mohamed, A., Ostadhossein, A., et al. (2018). In situ atomistic insight into the growth mechanisms of single layer 2D transition metal carbides. Nat. Commun. 9 (1), 2266–2269. doi:10.1038/s41467-018-04610-0
Schropp, R. E. I., Stannowski, B., Brockhoff, A. M., van Veenendaal, P. A. T. T., and Rath, J. K. (2000). Hot wire CVD of heterogeneous and polycrystalline silicon semiconducting thin films for applications in thin film transistors and solar cells. Mater. Phys. Mech. 2 (2), 73–82.
Shah, M., Rodriguez-Couto, S., and Sevinc Sengor, S. (2020). Emerging technologies in environmental bioremediation. in Emerging technologies in environmental bioremediation. Elsevier. doi:10.1016/B978-0-12-819860-5.00021-3
Shahzad, F., Mohamed, A., Hatter, C. B., Anasori, B., Hong, S. M., Koo, C. M., et al. (2016). Electromagnetic interference shielding with 2D transition metal carbides (MXenes). Science 353 (6304), 1137–1140. doi:10.1126/science.aag2421
Shareef, I. A., Rubloff, G. W., Anderle, M., Gill, W. N., Cotte, J., and Kim, D. H. (1995). Subatmospheric chemical vapor deposition ozone/TEOS process for SiO2 trench filling. J. Vac. Sci. Technol. B Microelectron. Nanom. Struct. Process. Meas. Phenom. 13 (4), 1888–1892. doi:10.1116/1.587830
Sheng, X., Zhao, Y., Zhang, Li, and Xiang, Lu (2019). Properties of two-dimensional Ti3C2 MXene/thermoplastic polyurethane nanocomposites with effective reinforcement via melt blending. Compos. Sci. Technol. 181 (September), 107710. doi:10.1016/J.COMPSCITECH.2019.107710
Shi, X. ying, Gao, M. hang, Wen, wen Hu, Luo, D., Shao, zhong Hu, Huang, T., et al. (2022). Largely enhanced adsorption performance and stability of MXene through in-situ depositing polypyrrole nanoparticles. Sep. Purif. Technol. 287 (April), 120596. doi:10.1016/J.SEPPUR.2022.120596
Shi, Ye, Peng, L., Ding, Yu, Zhao, Yu, and Yu, G. (2015). Nanostructured conductive polymers for advanced energy storage. Chem. Soc. Rev. 44 (19), 6684–6696. doi:10.1039/c5cs00362h
Shuck, C. E., Sarycheva, A., Anayee, M., Levitt, A., Zhu, Y., Uzun, S., et al. (2020a). Scalable synthesis of Ti3C2Tx MXene. Adv. Eng. Mater. 22 (3), 1901241. doi:10.1002/ADEM.201901241
Shuck, C. E., Ventura-Martinez, K., Goad, A., Uzun, S., Shekhirev, M., and Gogotsi, Y. (2021). Safe synthesis of MAX and MXene: guidelines to reduce risk during synthesis. ACS Chem. Health Saf. 28 (5), 326–338. doi:10.1021/acs.chas.1c00051
Simon, P., and Gogotsi, Y. (2008). “Materials for electrochemical capacitors,” in Nature materials (United States, Germany, UK; Nature Publishing Group). doi:10.1038/nmat2297
Sivasankarapillai, V. S., Kumar Somakumar, A., Joseph, J., Nikazar, S., Rahdar, A., and Kyzas, G. Z. (2020). Cancer theranostic applications of MXene nanomaterials: recent updates. Nano-Structures Nano-Objects 22 (April), 100457. doi:10.1016/J.NANOSO.2020.100457
Sliozberg, Y., Jan, A., Hatter, C. B., Anasori, B., Gogotsi, Y., and Hall, A. (2020). Interface binding and mechanical properties of MXene-epoxy nanocomposites. Compos. Sci. Technol. 192 (May), 108124. doi:10.1016/J.COMPSCITECH.2020.108124
Sur, S., Rathore, A., Dave, V., Reddy, K. R., Singh Chouhan, R., and Sadhu, V. (2019). “Recent developments in functionalized polymer nanoparticles for efficient drug delivery system,” in Nano-structures and nano-objects (Elsevier B.V). doi:10.1016/j.nanoso.2019.100397
Tan, K. H., Abu Zaed, Md, Saidur, R., Abdullah, N., Ishak, N. A. I.Md, and Cherusseri, J. (2024). Strategic insights for bulk production of MXene: a review. E3S Web Conf. 488 (February), 01003. doi:10.1051/E3SCONF/202448801003
Tang, H., Wang, R., Shi, L., Sheremet, E., Rodriguez, R. D., and Sun, J. (2021). Post-processing strategies for improving the electrical and mechanical properties of MXenes. Chem. Eng. J. 425 (December), 131472. doi:10.1016/J.CEJ.2021.131472
Tang, W., Dong, Z., Zhang, R., Yi, X., Yang, K., Jin, M., et al. (2019). Multifunctional two-dimensional core-shell MXene@Gold nanocomposites for enhanced photo-radio combined therapy in the second biological window. ACS Nano 13 (1), 284–294. doi:10.1021/acsnano.8b05982
Vahidmohammadi, A., Moncada, J., Chen, H., Kayali, E., Orangi, J., Carrero, C. A., et al. (2018). Thick and freestanding MXene/PANI pseudocapacitive electrodes with ultrahigh specific capacitance. J. Mater. Chem. A 6 (44), 22123–22133. doi:10.1039/C8TA05807E
Vasyukova, I. A., Zakharova, O. V., Kuznetsov, D. V., and Gusev, A. A. (2022). Synthesis, toxicity assessment, environmental and biomedical applications of MXenes: a review. Nanomaterials 12 (11), 1797. doi:10.3390/NANO12111797
Wang, D., Lin, Y., Hu, D., Jiang, P., and Huang, X. (2020). Multifunctional 3D-MXene/PDMS nanocomposites for electrical, thermal and triboelectric applications. Compos. Part A Appl. Sci. Manuf. 130 (March), 105754. doi:10.1016/J.COMPOSITESA.2019.105754
Wang, H., Russa, M.La, and Qi, L. S. (2016). CRISPR/Cas9 in genome editing and beyond. Annu. Rev. Biochem. 85 (June), 227–264. doi:10.1146/ANNUREV-BIOCHEM-060815-014607
Wang, J., Liu, Y., Zhang, H., and Chen, W. (2020). Effect of MXene-polymer nanocomposites on cell viability and proliferation for tissue engineering applications. Biomaterials Sci. 8 (15), 4170–4181. doi:10.1039/D0BM00682H
Wang, L., Xu, C., Liu, Z., Chen, L., Ma, X., Cheng, H. M., et al. (2016). Magnetotransport properties in high-quality ultrathin two-dimensional superconducting Mo2C crystals. ACS Nano 10 (4), 4504–4510. doi:10.1021/acsnano.6b00270
Wang, Qi W., Zhang, H. B., Liu, Ji, Zhao, S., Xie, Xi, Liu, L., et al. (2019). Multifunctional and water-resistant MXene-decorated polyester textiles with outstanding electromagnetic interference shielding and joule heating performances. Adv. Funct. Mater. 29 (7), 1806819. doi:10.1002/ADFM.201806819
Wang, X., Li, Q., Zhang, J., Huang, H., Wu, S., and Yang, Y. (2020). Novel thin-film reverse osmosis membrane with MXene Ti3C2T embedded in polyamide to enhance the water flux, anti-fouling and chlorine resistance for water desalination. J. Membr. Sci. 603 (May), 118036. doi:10.1016/J.MEMSCI.2020.118036
Wang, Y., Bai, F., Luo, Q., Wu, M., Song, G., Zhang, H., et al. (2019). Lycium barbarum polysaccharides grafted with doxorubicin: an efficient PH-responsive anticancer drug delivery system. Int. J. Biol. Macromol. 121 (January), 964–970. doi:10.1016/J.IJBIOMAC.2018.10.103
Wei, H., Dong, J., Fang, X., Zheng, W., Sun, Y., Qian, Y., et al. (2019). Ti3C2Tx MXene/polyaniline (PANI) sandwich intercalation structure composites constructed for microwave absorption. Compos. Sci. Technol. 169 (January), 52–59. doi:10.1016/J.COMPSCITECH.2018.10.016
Whitby, G. S., and Katz, M. (1933). Synthetic rubber. Industrial Eng. Chem. 25 (12), 1338–1348. doi:10.1021/ie50288a012
Wodlinger, B., Dweiri, Y., and Durand, D. M. (2015). Chapter 9 - biochips. Implantable Biomedical Microsystems. Oxford: William Andrew Publishing, 203–214. doi:10.1016/B978-0-323-26208-8.00009-1
Wu, W., Wei, D., Zhu, J., Niu, D., Wang, F., Wang, L., et al. (2019a). Enhanced electrochemical performances of organ-like Ti 3 C 2 MXenes/polypyrrole composites as supercapacitors electrode materials. Ceram. Int. 45 (6), 7328–7337. doi:10.1016/J.CERAMINT.2019.01.016
Wu, X., Han, B., Zhang, H. B., Xie, Xi, Tu, T., Zhang, Yu, et al. (2020). Compressible, durable and conductive polydimethylsiloxane-coated MXene foams for high-performance electromagnetic interference shielding. Chem. Eng. J. 381 (February), 122622. doi:10.1016/J.CEJ.2019.122622
Xiang, H., Lin, H., Yu, L., and Chen, Yu (2019). Hypoxia-irrelevant photonic thermodynamic cancer nanomedicine. ACS Nano 13 (2), 2223–2235. doi:10.1021/ACSNANO.8B08910
Xu, C., Wang, L., Liu, Z., Chen, L., Guo, J., Kang, N., et al. (2015). Large-area high-quality 2D ultrathin Mo2C superconducting crystals. Nat. Mater. 14 (11), 1135–1141. doi:10.1038/nmat4374
Xu, H., Zheng, D., Liu, F., Li, W., and Lin, J. (2020). Synthesis of an MXene/polyaniline composite with excellent electrochemical properties. J. Mater. Chem. A 8 (12), 5853–5858. doi:10.1039/D0TA00572J
Xu, Yu, Sun, G., Middha, E., Liu, Yu H., Chan, K. C., Liu, B., et al. (2020). Organic nanoparticle-doped microdroplets as dual-modality contrast agents for ultrasound microvascular flow and photoacoustic imaging. Sci. Rep. 10 (1), 17009–17012. doi:10.1038/s41598-020-72795-w
Xu, Y., Zhang, H., Li, Y., and Wang, L. (2019). Tissue compatibility of MXene-polymer nanocomposites for implantable medical devices. Acta Biomater. 97, 602–612. doi:10.1016/j.actbio.2019.08.028
Xue, Y., Feng, J., Huo, S., Song, P., Yu, B., Liu, L., et al. (2020). Polyphosphoramide-intercalated MXene for simultaneously enhancing thermal stability, flame retardancy and mechanical properties of polylactide. Chem. Eng. J. 397 (October), 125336. doi:10.1016/J.CEJ.2020.125336
Yadav, R. S. (2023). Multifunctional nanomaterials: synthesis, properties, and applications 2.0. Int. J. Mol. Sci. 24 (8), 7619. doi:10.3390/IJMS24087619
Yang, L., Chen, S., Wei, H., Luo, Y., Cong, F., Li, W., et al. (2022). Low-temperature photothermal therapy based on borneol-containing polymer-modified MXene nanosheets. ACS Appl. Mater. Interfaces 14 (40), 45178–45188. doi:10.1021/acsami.2c12839
Young, R. J., and Lovell, P. A. (2008). “Introduction to polymers,” in Food materials science: principles and practice. 3rd Editio (United States: CRC Press, Taylor and Francis Group). doi:10.1007/978-0-387-71947-4_4
Zada, S., Dai, W., Kai, Z., Lu, H., Zhang, Y., Meng, X., et al. (2020). Algae extraction controllable delamination of vanadium carbide nanosheets with enhanced near-infrared photothermal performance. Angew. Chem. Wiley Online Libr. 59 (16), 6601–6606. doi:10.1002/anie.201916748
Zaed, Md A., Tan, K. H., Norulsamani, A., Saidur, R., Pandey, A. K., and Ahmed, M. S. (2024). Cost analysis of MXene for low-cost production, and pinpointing of its economic footprint. Open Ceram. 17 (March), 100526. doi:10.1016/J.OCERAM.2023.100526
Zavahir, S., Sobolčiak, P., Krupa, I., Han, D. S., Tkac, J., and Kasak, P. (2020). Ti3C2Tx MXene-based light-responsive hydrogel composite for bendable bilayer photoactuator. Nanomaterials 10 (7), 1419. doi:10.3390/NANO10071419
Zhang, C., Xu, S., Cai, D., Cao, J., Wang, L., and Han, W. (2020). Planar supercapacitor with high areal capacitance based on Ti3C2/polypyrrole composite film. Electrochimica Acta 330 (January), 135277. doi:10.1016/J.ELECTACTA.2019.135277
Zhang, F., Wang, F., Wei, X., Yang, Y., Xu, S., Deng, D., et al. (2022). From trash to treasure: chemical recycling and upcycling of commodity plastic waste to fuels, high-valued chemicals and advanced materials. J. Energy Chem. 69 (June), 369–388. doi:10.1016/J.JECHEM.2021.12.052
Zhang, H., Wang, L., Zhou, A., Shen, C., Dai, Y., Liu, F., et al. (2016). Effects of 2-D transition metal carbide Ti 2 CT x on properties of epoxy composites. RSC Adv. 6 (90), 87341–87352. doi:10.1039/C6RA14560D
Zhang, J., Zeng, B., Li, D., Cui, Y., Wang, J., Duan, X., et al. (2022). Boron nitride-Au (Ag) loaded eggshell membrane with enhanced photothermal property. Colloids Surfaces A Physicochem. Eng. Aspects 642 (June), 128726. doi:10.1016/J.COLSURFA.2022.128726
Zhang, P., Yang, X. J., Peng, Li, Zhao, Y., and Niu, Q. J. (2019). Fabrication of novel MXene (Ti 3 C 2)/Polyacrylamide nanocomposite hydrogels with enhanced mechanical and drug release properties. Soft Matter 16 (1), 162–169. doi:10.1039/C9SM01985E
Zhang, Y., and Gu, J. (2022). A perspective for developing polymer-based electromagnetic interference shielding composites. Nano-Micro Lett. 14 (1), 89–9. doi:10.1007/s40820-022-00843-3
Zhang, Y., Wang, L., Zhang, N., and Zhou, Z. (2018). Adsorptive environmental applications of MXene nanomaterials: a review. RSC Adv. 8 (36), 19895–19905. doi:10.1039/C8RA03077D
Zhang, Y., Wang, S., Liu, X., and Chen, Z. 2021. “Evaluation of the inflammatory response of MXene-polymer nanocomposites in vitro and in vivo.” J. Biomed. Mater. Res. Part A 109 (9): 1875–1886. doi:10.1002/jbm.a.37199
Zhao, L., Wang, L., Zheng, Y., Zhao, S., Wei, W., Zhang, D., et al. (2021). Highly-stable polymer-crosslinked 2D MXene-based flexible biocompatible electronic skins for in vivo biomonitoring. Nano Energy 84 (June), 105921. doi:10.1016/J.NANOEN.2021.105921
Zhou, Z., Panatdasirisuk, W., Mathis, T. S., Anasori, B., Lu, C., Zhang, X., et al. (2018). Layer-by-Layer assembly of MXene and carbon nanotubes on electrospun polymer films for flexible energy storage. Nanoscale 10 (13), 6005–6013. doi:10.1039/C8NR00313K
Zhu, J., Tang, Y., Zhu, X., Zhang, B., Wang, Y., Xu, W., et al. (2020). MXene-polymer nanocomposite scaffolds for bone tissue engineering applications. Tissue Eng. Part A 26 (5–6), 292–301. doi:10.1089/ten.TEA.2019.0301
Zhu, M., Huang, Y., Deng, Q., Zhou, J., Pei, Z., Qi, X., et al. (2016a). Highly flexible, freestanding supercapacitor electrode with enhanced performance obtained by hybridizing polypyrrole chains with MXene. Adv. Energy Mater. 6 (21), 1600969. doi:10.1002/AENM.201600969
Zhu, M., Huang, Y., Deng, Q., Zhou, J., Pei, Z., Qi, X., et al. (2016b). Highly flexible, freestanding supercapacitor electrode with enhanced performance obtained by hybridizing polypyrrole chains with MXene. Adv. Energy Mater. 6 (21), 1600969. doi:10.1002/aenm.201600969
Ziabicki, A. (1976). Fundamentals of fiber formation. W Książce. United States: Wiley, University of Minnesota.
Zong, L., Wu, H., Lin, H., and Chen, Yu (2018). A polyoxometalate-functionalized two-dimensional titanium carbide composite MXene for effective cancer theranostics. Cancer Theranostics 11 (8), 4149–4168. doi:10.1007/s12274-018-2002-3
Keywords: MXene-polymers nanocomposites, biomedical applications, biocompatibility and toxicity, drug delivery and targeting, cost effective
Citation: Parajuli D (2024) MXenes-polymer nanocomposites for biomedical applications: fundamentals and future perspectives. Front. Chem. 12:1400375. doi: 10.3389/fchem.2024.1400375
Received: 13 March 2024; Accepted: 04 April 2024;
Published: 28 May 2024.
Edited by:
Andreas Rosenkranz, University of Chile, ChileReviewed by:
Madhurya Chandel, Warsaw University of Technology, PolandChenhui Yang, Northwestern Polytechnical University, China
Max Marian, Pontificia Universidad Católica de Chile, Chile
Copyright © 2024 Parajuli. This is an open-access article distributed under the terms of the Creative Commons Attribution License (CC BY). The use, distribution or reproduction in other forums is permitted, provided the original author(s) and the copyright owner(s) are credited and that the original publication in this journal is cited, in accordance with accepted academic practice. No use, distribution or reproduction is permitted which does not comply with these terms.
*Correspondence: D. Parajuli, ZGVlcGVucGFyYWpAZ21haWwuY29t