- 1Bahir Dar Energy Center, Bahir Dar Institute of Technology, Bahir Dar University, Bahir Dar, Ethiopia
- 2Department of Chemistry, College of Science, Bahir Dar University, Bahir Dar, Ethiopia
Although cobalt oxides show great promise as supercapacitor electrode materials, their slow kinetics and low conductivity make them unsuitable for widespread application. We developed Ni and Cu-doped Co3O4 nanoparticles (NPs) via a simple chemical co-precipitation method without the aid of a surfactant. The samples were analyzed for their composition, function group, band gap, structure/morphology, thermal property, surface area and electrochemical property using X-ray diffraction (XRD), ICP-OES, Fourier transform infrared (FTIR) spectroscopy, Ultraviolet-visible (UV-Vis), Scanning electron microscopy (SEM), Thermogravimetric analysis (TGA) and/or Differential thermal analysis (DTA), Brunauer–Emmett–Teller (BET), and Impedance Spectroscopy (EIS), Cyclic voltammetry (CV), respectively. Notably, for the prepared sample, the addition of Cu to Co3O4 NPs results in a 11.5-fold increase in specific surface area (573.78 m2 g−1) and a decrease in charge transfer resistance. As a result, the Ni doped Co3O4 electrode exhibits a high specific capacitance of 749 F g−1, 1.75 times greater than the pristine Co3O4 electrode’s 426 F g−1. The electrode’s enhanced surface area and electronic conductivity are credited with the significant improvement in electrochemical performance. The produced Ni doped Co3O4 electrode has the potential to be employed in supercapacitor systems, as the obtained findings amply demonstrated.
1 Introduction
The development of sustainable and renewable energy devices depends on the efficient storage and recovery of electric energy (Cheng et al., 2019; Worku, 2022; Sun et al., 2023). The use of harmless, widely accessible materials for reduced manufacturing costs and improved operating safety has been the main focus of this topic’s research. With its capacity to offer high specific energy in a variety of electrical appliances, including medical equipment and communication implements, Li-ion battery technology stands out (Worku et al., 2022a; Worku et al., 2022b; Hossain and Sahajwalla, 2022; Ambissa Begaw et al., 2023). Yet, supercapacitors (SCs) with high specific power that can deliver significant amounts of electrical energy in a little length of time are needed for the creation of next-generation hybrid systems (Chen et al., 2017; Wang et al., 2023). SC operation depends heavily on fundamental electrochemical processes that take place at the electrodes of SCs (Baidya et al., 2017; Wang et al., 2024). Hence, SCs can be classified into two categories: pseudocapacitors with faradic charge storage and electrical double-layer capacitors (EDLC) with nonfaradic charge storage (Ambare et al., 2015; Huang et al., 2020). In general, SCs based on carbon nanomaterials including carbon nanotubes, graphene oxides, and activated carbon (AC) are EDLCs, which have huge surface areas and strong electrical conductivity (Chen et al., 2019; Iqbal et al., 2022). Contrarily, pseudocapacitive materials are made from transition metal oxides (TMOs), such as RuO2, Fe3O4, Mn3O4 (Chen et al., 2013), NiO, Co3O4 (Aadil et al., 2020a; Habtu et al., 2022), V2O5, and ZnO, which undergo reversible faradic reactions. Compared to carbon-based materials, these TMOs have a substantially higher energy density (Choi et al., 2012; Ketema Worku and Worku Ayele, 2023). Co3O4 material has received a lot of interest among transition metal oxides due to its greater theoretical capacitance (3,560 Fg−1), low cost, abundance, and environmental friendliness (Borenstein et al., 2017; Alem et al., 2023a). Moreover, Co3O4 electrode material has an outstanding electrochemical capacitive behavior due to its unique microstructure and shape (Ali and Khalid, 2020a). For use in supercapacitor applications, Co3O4 nanostructures with a variety of morphologies, including nanowires, nanorods, nano-cubes, thin films, nano porous, nanoplates, nanotubes, and hollow spheres structures, have been created (Farhadi et al., 2016; Joseph et al., 2022). Unfortunately, the weak electrical conductivity of Co3O4 NPs for supercapacitor application limits their performance (Galini et al., 2018). Moreover, Co3O4 NPs can be made using a variety of techniques that result in strong electrical conductivity and high ionic diffusion rates (Adhikari et al., 2020; Alemu et al., 2023). Doping or including impurities in the spinel Co3O4 NPs is one of many effective strategies (Ali et al., 2021a; Suganya et al., 2022). An innovative method for enhancing the material’s structural, electrical, and optical properties is the doping of metal oxide nanoparticles with a particular element (Ali and Khalid, 2020b). Several active metals, including Copper (Cu), Chromium (Cr) (Worku et al., 2021a), Iron (Fe) (Habtu et al., 2022), Zinc (Zn) (Al Boukhari et al., 2018), and Manganese (Mn) (Worku et al., 2021b), have been doped into Co3O4 NPs to increase supercapacitive activity (Gao et al., 2019; Aadil et al., 2021). Several synthesis techniques have been used to create Co3O4 NPs, including the co-precipitation, hydrothermal, sol-gel, spray pyrolysis, chemical deposition, and solvothermal technique (Das et al., 2019; Worku et al., 2021c). Unfortunately, such synthesis procedures are pricey, need for expensive equipment, and take more time to prepare (Ali et al., 2021b). Among these synthesis pathways, the co-precipitation method has the benefit of being quick and easy to use, inexpensive, and easy to regulate particle size throughout preparation (Arora et al., 2017; Begaw et al., 2023). The electrical, optical, structural, and electrochemical properties of Ni and Cu doped Co3O4 NPs have been the subject of several studies (Aadil et al., 2020b; Worku et al., 2021d). However, structural, functional, optical, morphological, thermal, and electrochemical properties of Ni and Cu doped Co3O4 NPs for supercapacitor applications have only been briefly documented in a few studies (Worku et al., 2021e; Maheshwaran et al., 2022). In the current study, Ni and Cu-doped Co3O4 NPs were developed using different dopant concentrations via co-precipitation method (Girirajan et al., 2022; Yayeh et al., 2024). The improved dimensional stability and decreased chance of particle aggregation during charge and discharge procedures are both benefits of the reduced Ni and Cu concentration on Co3O4 NPs (Ghaziani et al., 2022). Because of this, the novelty of the current study is that Ni and Cu-doped Co3O4 NPs with controlled size may be an alternative electrode material that improves electrochemical behavior with high specific capacitance coupled to its natural high conductivity and is also reasonably priced with low toxicity.
2 Experimental
2.1 Synthesis
Without further purification, all of the chemicals used in the studies were obtained from commercial sources and were of analytical quality (99.8%). A simple co-precipitation technique was used to develop Ni doped-Co3O4 NPs (Alem et al., 2023a). In this typical synthesis process, 100 mL of distilled water was used to dissolve 0.2 M of cobalt nitrate hexahydrate [Co(NO3)2. 6H2O] and the equivalent mole of nickel (II) nitrate hexahydrate [Ni(NO3)2. 6H2O]. The aforementioned solution of Co(NO3)2. 6H2O and Ni(NO3)2. 6H2O was agitated for 30 min before 0.02 mL (2.12 g) of Na2CO3 was added. To create a homogeneous solution, the mixture was agitated and heated at 60°C for 3 h. The as-prepared sample was cleaned with distilled water and dried in a hot air oven for 12 h at 110°C. Lastly, the resulting powder was calcined for 3 h in a muffle furnace at 500°C to produce Ni-doped Co3O4 NPs. The same method was used to make both pure Co3O4 and Co3O4 NPs doped with copper.
2.2 Characterization
Using powder X-ray diffraction (XRD-7000, SHIMADZU) in the (2
2.3 Electrodes fabrication
In order to fabricate electrodes for electrochemical investigations, a sample impregnated with CS (chitosan) was cast onto a 5 mm diameter glassy carbon electrode to create the supercapacitor electrode. The exposed glassy carbon working electrode (GCE) was cleaned extensively in ethanol and ultrapure water, respectively, after being polished with 0.3 and 0.05 m alumina slurry. To create a homogenous suspension, the electrode material (10 mg) was sonicated into 1 mL of 0.5 wt% CS. After that, the 30 L of dispersion was placed on the glassy carbon electrode and allowed to dry in the air. Prior to electrochemical testing, a second 30 L dispersion was applied to the dried electrode surface and allowed to dry naturally. Using electrochemical workstations (Shanghai Chenhua Instrument Co. Ltd. CHI660E) and a 1 M KOH electrolyte solution, all electrochemical tests were performed. Hence, Eq. 1 was used to determine an electrode’s specific capacitance based on CV curves.
where, m, I,
where, E, Cs,
3 Results and discussion
3.1 XRD analysis
By employing Cu-K radiation (=1.54061) and XRD at a doping level of 0.05 M of Ni and Cu in the (2
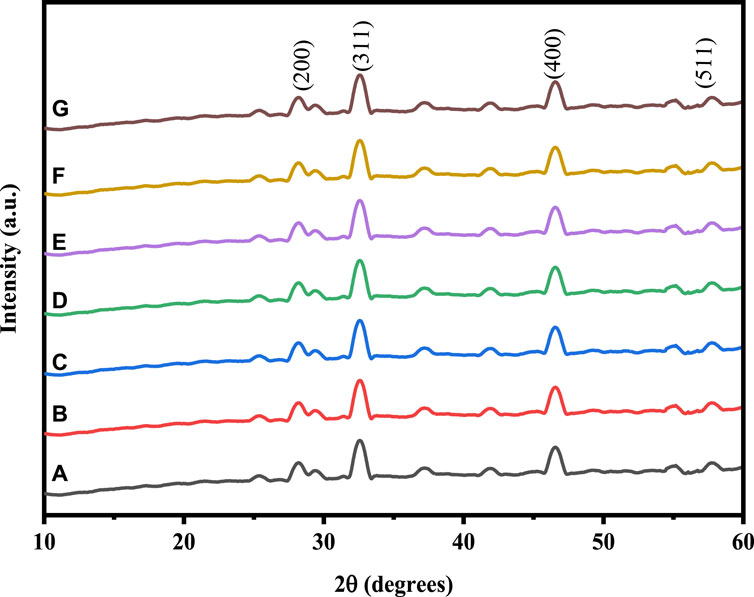
Figure 1. XRD pattern of: (A) Co3O4, (B) 0.01 M Cu-Co3O4, (C) 0.03 M Cu-Co3O4, (D) 0.05 M Cu- Co3O4, (E) 0.01M Ni-Co3O4, (F) 0.03 M Ni- Co3O4, (G) 0.05 M Ni-Co3O4 NPs.
The crystallite size (D) of the as-prepared NPs were estimated using the Scherer’s Eq. 4.
where, D, K,
where,
According to ICP-OES, the Cu content in 0.01 M Cu-Co3O4, 0.02 M Cu-Co3O4, 0.03 M Cu-Co3O4, 0.04 M Cu-Co3O4 and 0.05 M Cu-Co3O4 are 0.034, 0.052, 0.061, 0.073 and 0.085 at% (Table 2), correspondingly. Moreover, rendering to ICP-OES, the Cu content in 0.01 M Ni-Co3O4, 0.02 M Ni-Co3O4, 0.03 M Ni-Co3O4, 0.04 M Ni-Co3O4 and 0.05 M Ni-Co3O4 are 0.029, 0.036, 0.045, 0.058 and 0.067 at% (Table 3), correspondingly. The above results consist with the given content of Cu and Ni, indicating that the corresponding amount of Cu and Ni has been incorporated into cobalt oxide.
3.2 FTIR analysis
To confirm the functional groups that are present in the samples, the FTIR analysis of pure cobalt oxide and cobalt oxide nanoparticles that have been doped with Ni and Cu was conducted. Using an FTIR 6600 spectrometer, FTIR spectroscopy of pure and Ni and Cu-doped cobalt oxide nanoparticles was carried out in the absorption range of 4,000–400 cm−1 wave number. The FTIR spectra of Co3O4 and Cu-doped Co3O4 NPs at various doping levels are depicted in Figure 2. Due to the as-prepared samples collecting moisture from the air, the broad absorption bands around 3,496 and 1,623 cm−1 are attributed to the stretching and bending vibrations of water molecules (O-H). Furthermore, the stretching vibrations of NO3− caused by the precursor cobalt nitrate hexahydrate were identified as the absorption peaks at 1,383 cm−1 (Bai and Yang, 2021). Nanoparticles vibrate in the C-O stretching mode in the band at 1,114 cm−1 (Ali et al., 2021b). Hence, CO32−-anion may have a distinctive peak at 830 cm−1. It is possible to attribute the two absorption bands at 618 cm−1 were the stretching vibrations of metal-oxygen (Co-O or Cu-O) in spinel oxide Co3O4 NPs. The Co+2- O vibrations in the tetrahedral site of the Co3O4 NPs lattice are measured at 627 cm−1, while the Co+3-O vibrations are measured at 517 cm−1. The FTIR band of Co3O4 and Cu-doped Co3O4 NPs thus confirmed the production of M-O or M-O-M and O-H (Yang et al., 2020). The peak intensity and function groups for Ni and Cu doped Co3O4 increased as Ni and Cu concentration was added, improving the functionality of the nanoparticles. As a result, the FTIR bands of Co3O4 and Ni or Cu- Co3O4 NPs confirmed the formation of M-O and M-O-M (M= Ni, Cu or Co) and O-H (Worku et al., 2021f). The FTIR spectra seen in this investigation are compatible with the literature that has been previously reported (Jirátová et al., 2019).
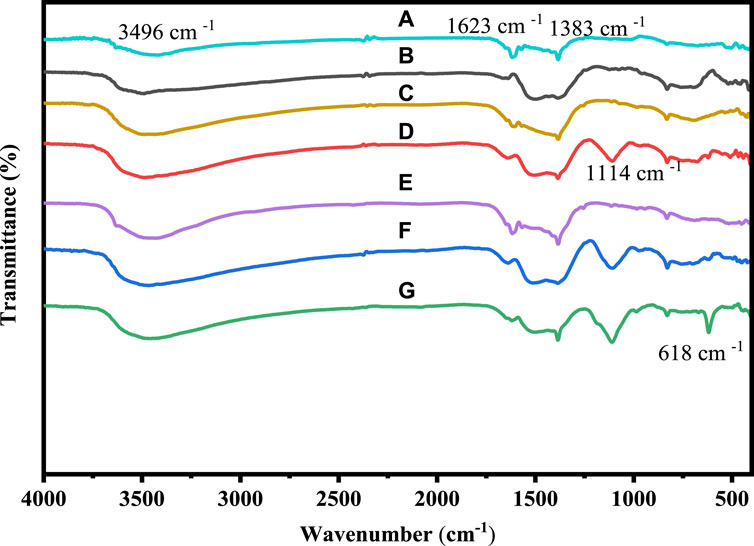
Figure 2. FTIR spectra of: (A) Co3O4, (B) 0.01 M Cu-Co3O4, (C) 0.03 M Cu-Co3O4, (D) 0.05 M Cu- Co3O4, (E) 0.01M Ni-Co3O4, (F) 0.03 M Ni- Co3O4, (G) 0.05 M Ni-Co3O4 NPs.
3.3 UV-Vis analysis
The optical characteristics of pure and Cu-doped Co3O4 NPs at various dopant concentrations was investigated via UV-Vis spectroscopy. The UV-Vis absorbance bands of Co3O4 and Cu-doped Co3O4 NPs are depicted in Figure 3. The various absorption bands in the wavelength range between 250 and 500 nm are visible in the optical characteristics of Co3O4 NPs. Co3O4 was found to exhibit absorption peaks at 273 nm (Figure 3A). The properties of the Co3O4 band at 273 nm are a sign that it was produced via the co-precipitation technique from cobalt nitrate hexahydrate. For Co3O4 with Cu doping the matching absorption peak was shifted to 327.40 nm and 235 nm for Figures 3B, C, respectively. According to absorption spectra, as Cu-dopant concentration rises, the peak changes to the positive wavelength. The presence of Cu-impurities may have caused many occupied localized states to be introduced, which in turn changed the absorption band characteristics of the nanoparticles as they were being created (Jirátová et al., 2019). Moreover, the absorption spectrum of Ni-doped Co3O4 were shown in Figures 3D–F. The absorption band shifted from 273 nm to 302 nm for 0.01M Ni-Co3O4, 353 nm for 0.03 M Ni- Co3O4, and 384 nm for 0.05 M Ni-Co3O4 NPs.
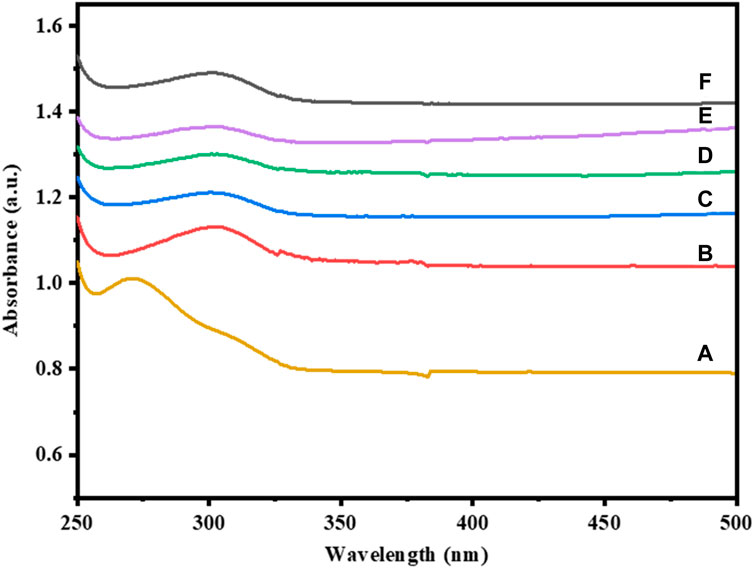
Figure 3. UV-Vis absorption spectra of: (A) Co3O4, (B) 0.01 M Cu-Co3O4, (C) 0.05 M Cu- Co3O4, (D) 0.01M Ni-Co3O4, (E) 0.03 M Ni- Co3O4, (F) 0.05 M Ni-Co3O4 NPs.
The energy band gap of pure Co3O4 and Cu-doped Co3O4 NPs can were estimated from plot (α
where,
Moreover, the estimated energy band gap values of Co3O4 and Ni-Co3O4 NPs were shown in Table 5.
3.4 SEM analysis
SEM was used to examine the morphologies of pure Co3O4 and Ni and Cu- Co3O4 NPs. Figure 4 displays the morphologies of Co3O4, Ni, and Cu- Co3O4 NPs at various magnifications. The Co3O4 NPs in the SEM pictures at 20 µm magnifications are aggregated and have porous, rocky-like features (Figure 4A). The Co3O4 Ni- Co3O4 SEM pictures demonstrate homogeneous particle distribution. As Ni concentration is added, the particles’ morphology changes to nano-structured particles with irregular shapes and small aggregated porous particles (Figure 4B). The SEM analysis of Cu-doped Co3O4 NPs at various magnifications is displayed in Figure 4C. The examination revealed small, uniform-sized, spherical, and aggregated nanoparticles that are suitable for use in supercapacitors and consistent with earlier studies (Chang et al., 2021).
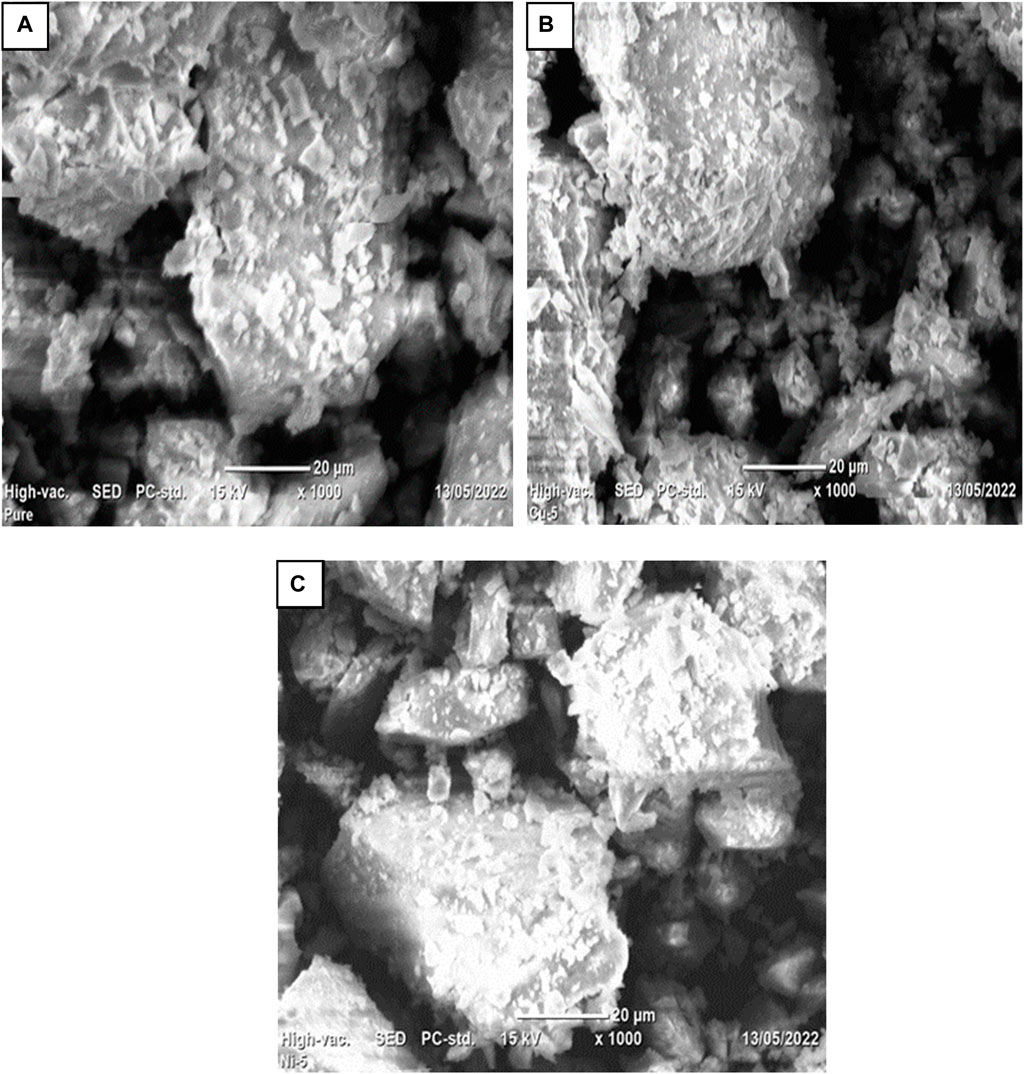
Figure 4. SEM images of Co3O4 NPs: (A) at 20 μm, (B) 0.05 Ni-Co3O4 at 20 μm, (C) 0.05 Cu-Co3O4 at 20 µm.
3.5 TGA/DTA analysis
TGA/DTA was used to examine the thermal characteristics of Co3O4 Ni and Cu-doped Co3O4 NPs. At the temperature range of 25°C–950°C at a heating rate of 20°C per minute in an environment of air, TGA and DTA spectra have been observed. The TGA/DTA curve of Co3O4 and Cu-doped Co3O4 NPs is shown in Figure 5. The first weight loss of 0.95 mg in the pure Co3O4 TGA curve happens between 25°C and 200°C, which indicates that water molecules begin to evaporate from the sample. In the temperature range of 200°C–300°C, the TGA-curve showed the second weight loss of 1.62 mg. By eliminating the organic components, it is showing that complete breakdown occurs, resulting in the creation of Co3O4 Np. Hence, the lack of weight loss over 300°C verifies the composite’s full breakdown and crystallization. The overall TGA data reveal that up to 300°C, a total loss of 32.12% occurs (Figure 5C). The endothermic peak at 90 °C that is seen from the DTA curve of pure Co3O4 may be the result of the sample losing absorbed water (dehydration). Corresponding to this, the full breakdown of the cobalt precursor and the crystallization of Co3O4 spinel correlate to an extreme exothermic peak of 285°C between temperature ranges of 200°C–300°C. The first weight loss of 1.55 mg in the Cu-doped Co3O4 TGA curve from room temperature to 150°C and its related endothermic peak at 100 °C may be caused by the loss of physically absorbed water (Figure 5B). At the temperature range of 150°C–200°C, the second weight loss of 1.05 mg was noted. It might be connected to the constant de-nitration of nitrates produced by cobalt nitrate precursor and melting of the precursor. Between 300°C and 400°C, a third weight loss of 0.18 mg was noticed, which might be attributed to the precursor’s complete disintegration as a result of the removal of its organic constituents. The graph is linear and straight after 400°C, showing that no more weight loss takes place. The elimination of organic molecules from the material, which indicates the creation of Co3O4 crystals, is likely the cause of the two exothermic peaks that arise at 298°C and 348°C. The average weight loss for this analysis from 25°C to 950°C was 32.12% for Co3O4 and 34.7% for Cu- Co3O4 NPs, respectively. As a result, the comparison of the TGA and DTA results with the earlier findings was successful. The TGA/DTA curve for 0.05 M Ni-doped Co3O4 was recorded between 25°C and 950°C. The TGA curve demonstrates that the material undergoes two stages of heat breakdown. The TGA/DTA analysis of 0.05 M Ni-doped Co3O4 is displayed in Figure 5A. The elimination of absorbed water causes the first weight loss at a temperature of between 25°C and 200°C (dehydration). The fact that the second weight loss of 1.19 mg occurred in the 200°C–300°C temperature range shows that complete thermal breakdown can occur, resulting in the creation of Co3O4. The endothermic peak at 76°C on the DTA curve can be the result of precursors losing water (water removal from sample). The loss of organic molecules from the sample results in an exothermic peak at 286°C, leaving only the Co3O4 spinel. Between 100°C and 300°C, the TGA displays a considerable weight loss. In the process of losing weight, important gaseous products like water and nitrogen dioxide are emitted. Weight loss at 350°C supports the reported results for the conversion of [Co(NO3)2. 6H2O] into Co3O4 (Geng et al., 2017).
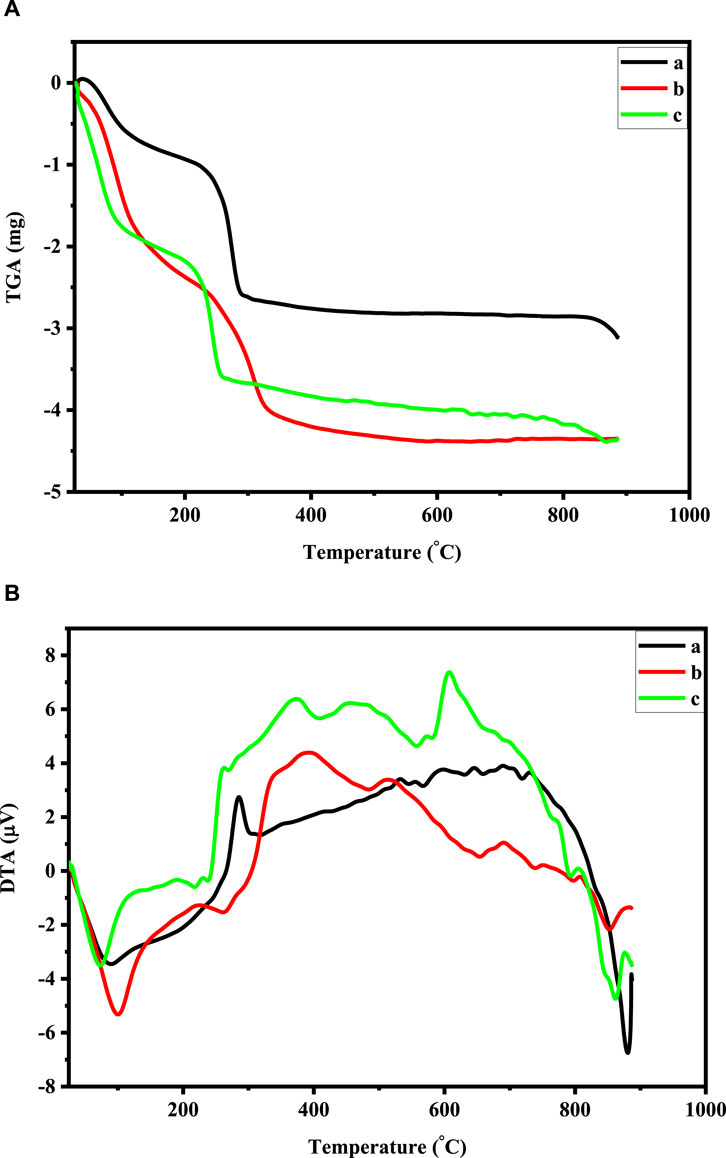
Figure 5. (A) TGA curve of: 0.05 M Ni-doped Co3O4, Co3O4, and 0.05 M Cu-doped Co3O4 NPs. (B) DTA curve of: 0.05 M Ni-doped Co3O4, Co3O4, and 0.05 M Cu-doped Co3O4 NPs.
3.6 BET analysis
To determine the total surface areas, pore diameters, and pore volumes of the materials as-prepared, BET was carried out in nitrogen gas at 77.35 K. Table 6 displays the overall surface area, pore volume, and pore diameter of Co3O4, Ni- Co3O4, and Cu-doped Co3O4 NPs. The dopant in Co3O4 caused a significant increase in surface area, as evidenced by the data. The increased contribution of the metal ions as extra nucleation sites during precipitation may be linked to the doped materials’ larger surface areas. Thus, the BET analysis revealed that the Co3O4 NPs with 0.05 M Ni and Cu doping had the highest surface areas, allowing for ion transfer and diffusion via the faradaic process (Molavi and Sheikhi, 2018). Doping impurities in Co3O4 NPs causes the BET surface to increase as a result. This shows that if dopants are added, with different atomic sizes than mother crystal, then the crystal will involve more imperfection. Such imperfections cause more surface roughness and might increase the specific surface area.
3.7 Electrochemical property measurement
3.7.1 CV study
CV analysis were conducted to examine electrochemical properties of the Co3O4, Ni and Cu-Co3O4 NPs. CV measurements were carried out in 1 M KOH electrolyte solution at a scan rates of 20, 30, 50, and 100 mV/s with a potential window of −0.2–1.6 V. Figure 6 shows the CV curves of Co3O4, Ni and Cu-Co3O4 NPs. Moreover, in the measurement glassy carbon electrode, Ag/AgCl and Pt were used as a working, reference and counter electrode, respectively (Yadav et al., 2017). Figure 6 displayed that Ni doped Co3O4 NPs exhibited higher current peak and potential compared to un-doped and Cu doped Co3O4 NPs at 100 mV/s. Moreover, when compared to un-doped Co3O4 NPs, the CV curve of Ni and Cu doped Co3O4 NPs has a greater potential and current. The formation of a bigger enclosed area for the supercapacitor’s electrodes is crucial for the transfer of charges and improves the specific capacitance values (Geng et al., 2017; Yue et al., 2022). The CV curves of 0.05 M Ni and Cu doped Co3O4 NPs with scan rates of 100 mV/s and a potential window of 0–1.5 V are shown in Figure 6. The data thus demonstrated that the anodic and cathodic peaks move to the higher and lower potential, respectively, as the doping material varies at 100 mV/s. Moreover, the peak currents noticeably rose as scan rates increased (Alem et al., 2023b). The Cs value of pure, 0.05 M Ni and 0.05 M Cu doped Co3O4 NPs is intended at different scan rates. The calculated specific capacitance values at 20 and 100 mV/s scan rate is 313 F/g and 307 F/g, 449 F/g and 408 F/g, 426 F/g and 403 F/g pure Co3O4, 0.05 M Ni, and 0.05 M Cu doped Co3O4 NPs, respectively. The specific capacitance value increase with scan rate and with the addition of Ni and Cu dopants (Chang et al., 2021).
3.7.2 Electrochemical impedance (EIS) analysis
In order to further examine the capacitive and resistive behaviour of pure and Ni and Cu doped Co3O4 NPs, EIS measurements were also carried. The Nyquist plots are displayed in Figure 7, where it is possible to see the occurrence of semicircles in the high frequency range. Their analogous circuit is depicted in the inset. The symbols R1, R2, CPE and W, represent in the equivalent circuit the internal resistance, the charge transfer resistance, constant phase element and the Warburg impedance, respectively. At higher frequencies, the point that coincides with the Z′ axis yields the value of R1, or internal resistance, which is a combination of contact resistance, the inherent resistance of the material that makes up the electrode’s surface, and the resistance of the electrolyte. In addition, the diameter of the semicircle provides the inter-facial resistance R2, also known as charge shifting resistance, and the vertical line at low-frequency values coupled with the diffusion resistance, also known as Warburg impedance. The R1 of 2.34, 1.81 and 1.53 Ω and R2 of 1.97, 1.53 and 0.79 Ω are recorded for Co3O4, 0.05M Cu-Co3O4 and 0.05 M Ni-Co3O4, respectively. The lower resistance of 0.05 M Ni-Co3O4 electrode might be due to the weak crystallinity, which facilitate the intercalation of protons during electrochemical reactions and efficient transport of electrons and ions via its expanded crystal structure. This demonstrated the material’s high electrical conductivity.
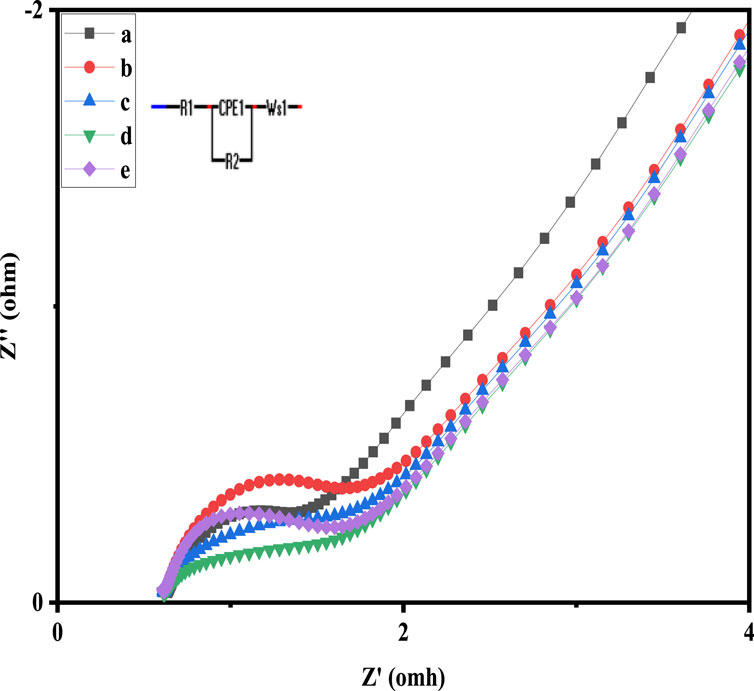
Figure 7. Nyquist graphs of: a) 0.05 M Ni-Co3O4, b) Co3O4, c) 0.01 M Cu-Co3O4, d) 0.01 M Ni-Co3O4, e) 0.05M Cu-Co3O4 NPs.
4 Conclusion
In conclusion, the co-precipitation approach was successfully used to create Ni and Cu-doped Co3O4 NPs in a range of doping concentrations. By varying the Ni and Cu content, the shape and crystal structure of the Co3O4 samples can be controlled. The sample’s morphology and the thickness of the materials as they were prepared are altered by the addition of Ni and Cu. The oxygen vacancies and surface morphology can be controlled by Ni and Cu doping. It increases the electrode material’s conductivity significantly and produces more electrochemically active spots. The addition of dopant Cu without the presence of any impurity phase resulted in a reduction in crystallite size, according to XRD measurements. The produced Ni and Cu-doped Co3O4 NPs’ M-O vibrations, active modes, and purity were validated by FTIR. The band gap reduced with increasing Ni and Cu doping concentration, and the obtained bandgap range supported the synthesized Ni and Cu-doped Co3O4 NPs’ semiconducting nature. Ni dopant also improved the electrochemical performance of Co3O4 NPs, which had a high specific capacitance of 449 F g−1 due to their higher surface area from the smaller particle size. Also, the produced samples preserve acceptable power and energy densities, showing that the synthesized materials have enormous promise as a future energy storage technology.
Data availability statement
The original contributions presented in the study are included in the article/supplementary material, further inquiries can be directed to the corresponding author.
Author contributions
AW: Methodology, Writing–original draft, Writing–review and editing. AA: Writing–original draft. DA: Supervision, Writing–review and editing.
Funding
The authors declare that no financial support was received for the research, authorship, and/or publication of this article.
Acknowledgments
The lab technicians from the Chemical and Food Engineering faculty of Bahir Dar Institute of Technology are gratefully acknowledged by the authors for their assistance with chemical analysis and characterization.
Conflict of interest
The authors declare that the research was conducted in the absence of any commercial or financial relationships that could be construed as a potential conflict of interest.
Publisher’s note
All claims expressed in this article are solely those of the authors and do not necessarily represent those of their affiliated organizations, or those of the publisher, the editors and the reviewers. Any product that may be evaluated in this article, or claim that may be made by its manufacturer, is not guaranteed or endorsed by the publisher.
References
Aadil, M., Zulfiqar, S., Sabeeh, H., Warsi, M. F., Shahid, M., Alsafari, I. A., et al. (2020b). Enhanced electrochemical energy storage properties of carbon coated Co3O4 nanoparticles-reduced graphene oxide ternary nano-hybrids. Ceram. Int. 46, 17836–17845. doi:10.1016/j.ceramint.2020.04.090
Aadil, M., Zulfiqar, S., Shahid, M., Haider, S., Shakir, I., and Warsi, M. F. (2020a). Binder free mesoporous Ag-doped Co3O4 nanosheets with outstanding cyclic stability and rate capability for advanced supercapacitor applications. J. Alloys Compd. 844, 156062. doi:10.1016/j.jallcom.2020.156062
Aadil, M., Zulfiqar, S., Warsi, M. F., Agboola, P. O., Shakir, I., Shahid, M., et al. (2021). Mesoporous and macroporous Ag-doped Co3O4 nanosheets and their superior photo-catalytic properties under solar light irradiation. Ceram. Int. 47, 9806–9817. doi:10.1016/j.ceramint.2020.12.121
Adhikari, S., Selvaraj, S., Ji, S. H., and Kim, D. H. (2020). Encapsulation of Co3O4 nanocone arrays via ultrathin NiO for superior performance asymmetric supercapacitors. Small 16, e2005414. doi:10.1002/SMLL.202005414
Al Boukhari, J., Zeidan, L., Khalaf, A., and Awad, R. (2018). Synthesis, characterization, optical and magnetic properties of pure and Mn, Fe and Zn doped NiO nanoparticles. Chem. Phys. 516, 116–124. doi:10.1016/j.chemphys.2018.07.046
Alem, A. F., Worku, A. K., Ayele, D. W., Habtu, N. G., Ambaw, M. D., and Yemata, T. A. (2023a). Enhancing pseudocapacitive properties of cobalt oxide hierarchical nanostructures via iron doping. Heliyon 9, e13817. doi:10.1016/J.HELIYON.2023.E13817
Alem, A. F., Worku, A. K., Ayele, D. W., Wubieneh, T. A., Abebaw Teshager, A., Mihret kndie, T., et al. (2023b). Ag doped Co3O4 nanoparticles for high-performance supercapacitor application. Heliyon 9, e13286. doi:10.1016/J.HELIYON.2023.E13286
Alemu, M. A., Getie, M. Z., and Worku, A. K. (2023). Advancement of electrically rechargeable multivalent metal-air batteries for future mobility. Ionics (Kiel). 29, 3421–3435. doi:10.1007/s11581-023-05131-7
Ali, F., and Khalid, N. R. (2020a). Facile synthesis and properties of chromium-doped cobalt oxide (Cr-doped Co3O4) nanostructures for supercapacitor applications. Appl. Nanosci. 10, 1481–1488. doi:10.1007/S13204-020-01266-5
Ali, F., and Khalid, N. R. (2020b). Effect of calcination temperature on structural, morphological and electrochemical properties of Sn doped Co3O4 nanorods. Ceram. Int. 46, 24137–24146. doi:10.1016/J.CERAMINT.2020.06.193
Ali, F., Khalid, N. R., Nabi, G., Ul-Hamid, A., and Ikram, M. (2021a). Hydrothermal synthesis of cerium-doped Co <sub>3</sub> O <sub>4</sub> nanoflakes as electrode for supercapacitor application. Int. J. Energy Res. 45, 1999–2010. doi:10.1002/ER.5893
Ali, F., Khalid, N. R., Niaz, N. A., Nabi, G., Tahir, M. B., and Rafique, M. (2021b). Novel Cr and Sn co-doped Co3O4 polygon-based electrode material for supercapacitor application. J. Mat. Sci. Mat. Electron. 32, 11467–11477. doi:10.1007/s10854-021-05657-4
Ambare, R. C., Bharadwaj, S. R., and Lokhande, B. J. (2015). Non-aqueous route spray pyrolyzed Ru:Co 3 O 4 thin electrodes for supercapacitor application. Appl. Surf. Sci. 349, 887–896. doi:10.1016/j.apsusc.2015.04.175
Ambissa Begaw, G., Worku Ayele, D., Ketema Worku, A., Alemneh Wubieneh, T., Atnafu Yemata, T., and Dagnew Ambaw, M. (2023). Recent advances and challenges of cobalt-based materials as air cathodes in rechargeable Zn–air batteries. Results Chem. 5, 100896. doi:10.1016/J.RECHEM.2023.100896
Arora, E., Chaudhary, R., Kumar, S., and Kumar, D. (2017). Synthesis and characterization of copper doped cobalt oxide (Co3O4) by co-precipitation method. Springer Proc. Phys. 178, 177–183. doi:10.1007/978-3-319-29096-6_23
Bai, X., and Yang, Z. (2021). Synthesis of Ag/Co 3 O 4 for high sensitive non-enzymatic glucose sensor through synergy of surface/interface engineering. J. Electrochem. Soc. 168, 107508. doi:10.1149/1945-7111/ac2d3d
Baidya, T., Murayama, T., Bera, P., Safonova, O. V., Steiger, P., Katiyar, N. K., et al. (2017). Low-temperature CO oxidation over combustion made Fe- and Cr-doped Co3O4 catalysts: role of dopant’s nature toward achieving superior catalytic activity and stability. J. Phys. Chem. C 121, 15256–15265. doi:10.1021/acs.jpcc.7b04348
Begaw, G. A., Ayele, D. W., Worku, A. K., Wubieneh, T. A., Yemata, T. A., and Ambaw, M. D. (2023). Recent advances and challenges of cobalt-based materials as air cathodes in rechargeable Zn–air batteries. Results Chem. 5, 100896. doi:10.1016/J.RECHEM.2023.100896
Borenstein, A., Hanna, O., Attias, R., Luski, S., Brousse, T., and Aurbach, D. (2017). Carbon-based composite materials for supercapacitor electrodes: a review. J. Mat. Chem. A Mat. Energy Sustain. 5, 12653–12672. doi:10.1039/C7TA00863E
Chang, A. S., Tahira, A., Chang, F., Memon, N. N., Nafady, A., Kasry, A., et al. (2021). Silky Co3O4nanostructures for the selective and sensitive enzyme free sensing of uric acid. RSC Adv. 11, 5156–5162. doi:10.1039/d0ra10462k
Chen, H., Wang, J., Liao, F., Han, X., Zhang, Y., Xu, C., et al. (2019). Uniform and porous Mn-doped Co 3 O 4 microspheres: solvothermal synthesis and their superior supercapacitor performances. Ceram. Int. 45, 11876–11882. doi:10.1016/j.ceramint.2019.03.070
Chen, L., Song, Z., Liu, G., Qiu, J., Yu, C., Qin, J., et al. (2013). Synthesis and electrochemical performance of polyaniline-MnO2 nanowire composites for supercapacitors. J. Phys. Chem. Solids. 74, 360–365. doi:10.1016/j.jpcs.2012.10.013
Chen, Y., Pang, W. K., Bai, H., Zhou, T., Liu, Y., Li, S., et al. (2017). Enhanced structural stability of nickel-cobalt hydroxide via intrinsic pillar effect of metaborate for high-power and long-life supercapacitor electrodes. Nano Lett. 17, 429–436. doi:10.1021/ACS.NANOLETT.6B04427
Cheng, L., Xu, M., Zhang, Q., Li, G., Chen, J., and Lou, Y. (2019). NH4F assisted and morphology-controlled fabrication of ZnCo2O4 nanostructures on Ni-foam for enhanced energy storage devices. J. Alloys Compd. 781, 245–254. doi:10.1016/j.jallcom.2018.11.402
Choi, H. J., Jung, S. M., Seo, J. M., Chang, D. W., Dai, L., and Baek, J. B. (2012). Graphene for energy conversion and storage in fuel cells and supercapacitors. Nano Energy 1, 534–551. doi:10.1016/j.nanoen.2012.05.001
Das, S., Manoharan, C., Venkateshwarlu, M., and Dhamodharan, P. (2019). Structural, optical, morphological and magnetic properties of nickel doped cobalt ferrite nanoparticles synthesized by hydrothermal method. J. Mat. Sci. Mat. Electron. 30, 19880–19893. doi:10.1007/S10854-019-02355-0
Farhadi, S., Javanmard, M., and Nadri, G. (2016). Characterization of cobalt oxide nanoparticles prepared by the thermal decomposition of [Co(NH3)5(H2O)](NO3)3 complex and study of their photocatalytic activity. Acta Chim. Slov. 63, 335–343. doi:10.17344/ACSI.2016.2305
Galini, M., Salehi, M., and Behzad, M. (2018). Structural, magnetic and dielectric properties of Dy-doped Co 3 O 4 nanostructures for the electrochemical evolution of oxygen in alkaline media. J. Nanostructures. 8, 391–403. doi:10.22052/JNS.2018.04.009
Gao, Y. P., Zhai, Z. B., Wang, Q. Q., Hou, Z. Q., and Huang, K. J. (2019). Cycling profile of layered MgAl2O4/reduced graphene oxide composite for asymmetrical supercapacitor. J. Colloid Interface Sci. 539, 38–44. doi:10.1016/j.jcis.2018.12.045
Geng, X., Zhang, Y., Han, Y., Li, J., Yang, L., Benamara, M., et al. (2017). Two-dimensional water-coupled metallic MoS2 with nanochannels for ultrafast supercapacitors. Nano Lett. 17, 1825–1832. doi:10.1021/ACS.NANOLETT.6B05134
Ghaziani, M. M., Mazloom, J., and Ghodsi, F. E. (2022). Effect of Mg substitution on the physical and electrochemical properties of Co3O4 thin films as electrode material with enhanced cycling stability for pseudocapacitors. J. Sol-Gel Sci. Technol. 103, 244–257. doi:10.1007/S10971-022-05832-X
Girirajan, M., Arumugam, V., Subramaniyan, S., Manimuthu, R. P., and Sakkarapani, S. (2022). Two-dimensional layered bismuthene/antimonene nanocomposite as a potential electrode material for the fabrication of high-energy density hybrid supercapacitors. Energy Fuels 36, 12299–12309. doi:10.1021/ACS.ENERGYFUELS.2C02321
Habtu, N. G., Worku, A. K., Ayele, D. W., Teshager, M. A., and Workineh, Z. G. (2022). Facile preparation and electrochemical investigations of copper-ion doped α-MnO2 nanoparticles. Lect. Notes Inst. Comput. Sci. Soc. Telecommun. Eng. LNICST. 412, 543–553. doi:10.1007/978-3-030-93712-6_36
Hossain, R., and Sahajwalla, V. (2022). Microrecycled Co3O4from waste lithium-ion battery: synthesis, characterisation and implication in environmental application. J. Environ. Chem. Eng. 10, 107858. doi:10.1016/J.JECE.2022.107858
Huang, Y., Bao, S., and Lu, J. (2020). Flower-like MnO2/polyaniline/hollow mesoporous silica as electrode for high-performance all-solid-state supercapacitors. J. Alloys Compd. 845, 156192. doi:10.1016/J.JALLCOM.2020.156192
Iqbal, H., Parveen, B., Kiran, S., Imran, M., Saddique, F., Hassan, M. U., et al. (2022). Fabrication of chromium sulfide nanoparticles and reduced graphene oxide based high power asymmetric supercapacitor. J. Mat. Sci. Mat. Electron. 33, 24845–24856. doi:10.1007/S10854-022-09195-5
Jirátová, K., Perekrestov, R., Dvořáková, M., Balabánová, J., Topka, P., Koštejn, M., et al. (2019). Cobalt oxide catalysts in the form of thin films prepared by magnetron sputtering on stainless-steel meshes: performance in ethanol oxidation. Catalysts 9, 806. doi:10.3390/catal9100806
Joseph, A., Sunny, J., Thomas, T., and Anantharaman, M. R. (2022). Amorphous Cr2O3 sheets: a novel supercapacitor electrode material. ChemistrySelect 7. doi:10.1002/SLCT.202203049
Ketema Worku, A., and Worku Ayele, D. (2023). Recent advances of graphene-based materials for emerging technologies. Results Chem. 5, 100971. doi:10.1016/J.RECHEM.2023.100971
Li, S., Wang, Y., Sun, J., Zhang, Y., Xu, C., and Chen, H. (2020). Hydrothermal synthesis of Fe-doped Co3O4 urchin-like microstructures with superior electrochemical performances. J. Alloys Compd. 821, 153507. doi:10.1016/j.jallcom.2019.153507
Maheshwaran, G, Seethalakshmi, G., Kousalya Devi, V., Venkata Krishna, L. M., Ramesh Prabhu, M., Krishna Kumar, M., et al. (2022). Synergistic effect of Cr2O3 and Co3O4 nanocomposite electrode for high performance supercapacitor applications. Curr. Appl. Phys. 36, 63–70. doi:10.1016/j.cap.2022.01.007
Molavi, R., and Sheikhi, M. H. (2018). Low temperature carbon monoxide gas sensor based on Ag-Co3O4 thick film nanocomposite. Mat. Lett. 233, 74–77. doi:10.1016/j.matlet.2018.08.087
Ramesh, S., Yadav, H. M., Lee, Y. J., Hong, G. W., Kathalingam, A., Sivasamy, A., et al. (2019). Porous materials of nitrogen doped graphene oxide@SnO2 electrode for capable supercapacitor application. Sci. Rep. 9, 12622. doi:10.1038/s41598-019-48951-2
Rani, B. J., Raj, S. P., Saravanakumar, B., Ravi, G., Ganesh, V., Ravichandran, S., et al. (2017). Controlled synthesis and electrochemical properties of Ag-doped Co3O4 nanorods. Int. J. Hydrogen Energy. 42, 29666–29671. doi:10.1016/j.ijhydene.2017.10.051
Suganya, S., Maheshwaran, G., Ramesh Prabhu, M., Devendran, P., Krishna Kumar, M., and Sudhahar, S. (2022). Enhanced electrochemical activity of ternary Co-Mn-Zn oxide for the fabrication of hybrid supercapacitor applications. J. Energy Storage. 56, 106057. doi:10.1016/j.est.2022.106057
Sun, Z., Han, X., and Wang, D. (2023). Zinc-iodine battery-capacitor hybrid device with excellent electrochemical performance enabled by a robust iodine host. J. Energy Storage. 62, 106857. doi:10.1016/J.EST.2023.106857
Wang, D., Han, X., and Zhang, X. (2024). Achieving high-capacity aqueous calcium-ion storage in amorphous manganese oxide nanospheres for calcium-ion asymmetric supercapacitors. J. Power Sources. 599, 234215. doi:10.1016/J.JPOWSOUR.2024.234215
Wang, D., Sun, J., and Chen, L. (2023). Structural reconstruction strategy enables CoFe LDHs for high-capacity NH4+ storage and application in high-energy density ammonium-ion hybrid supercapacitors. ChemSusChem 16, e202300207. doi:10.1002/cssc.202300207
Wei, F., Liu, W., Zhang, X., Liu, X., Sui, Y., and Qi, J. (2019). Controllable synthesis of polyhedral Au@Co3O4 electrode for high performance supercapacitors. Mat. Lett. 255, 126534. doi:10.1016/j.matlet.2019.126534
Worku, A. K. (2022). Engineering techniques to dendrite free Zinc-based rechargeable batteries. Front. Chem. 10, 1018461. doi:10.3389/fchem.2022.1018461
Worku, A. K., Ayele, D. W., and Habtu, N. G. (2021c). Recent advances and future perspectives in engineering of bifunctional electrocatalysts for rechargeable zinc–air batteries. Mat. Today Adv. 9, 100116. doi:10.1016/j.mtadv.2020.100116
Worku, A. K., Ayele, D. W., and Habtu, N. G. (2021d). Influence of nickel doping on MnO2 nanoflowers as electrocatalyst for oxygen reduction reaction. SN Appl. Sci. 3, 764. doi:10.1007/s42452-021-04746-7
Worku, A. K., Ayele, D. W., Habtu, N. G., Admasu, B. T., Alemayehu, G., Taye, B. Z., et al. (2022a). “Energy storage technologies; recent advances, challenges, and prospectives,” in Planning of hybrid renewable energy systems, electric vehicles and microgrid modeling, control and optimization. Editors A. K. Bohre, P. Chaturvedi, M. L. Kolhe, and S. N. Singh (Singapore: Springer Nature Singapore), 125–150. doi:10.1007/978-981-19-0979-5_7
Worku, A. K., Ayele, D. W., Habtu, N. G., and Ambaw, M. D. (2022b). Engineering nanostructured Ag doped α-MnO2 electrocatalyst for highly efficient rechargeable zinc-air batteries. Heliyon 8, e10960. doi:10.1016/J.HELIYON.2022.E10960
Worku, A. K., Ayele, D. W., Habtu, N. G., Melas, G. A., Yemata, T. A., Mekonnen, N. Y., et al. (2021a). Structural and thermal properties of pure and chromium doped zinc oxide nanoparticles. SN Appl. Sci. 3, 699. doi:10.1007/s42452-021-04682-6
Worku, A. K., Ayele, D. W., Habtu, N. G., Teshager, M. A., and Workineh, Z. G. (2021b). Recent progress in MnO2-based oxygen electrocatalysts for rechargeable zinc-air batteries. Mat. Today sustain. 13, 100072. doi:10.1016/j.mtsust.2021.100072
Worku, A. K., Ayele, D. W., Habtu, N. G., Teshager, M. A., and Workineh, Z. G. (2021e). Enhancing oxygen reduction reaction activity of ε-MnO2 nanoparticles via iron doping. J. Phys. Chem. Solids. 157, 110207. doi:10.1016/j.jpcs.2021.110207
Worku, A. K., Ayele, D. W., Habtu, N. G., and Yemata, T. A. (2021f). Engineering Co3O4/MnO2 nanocomposite materials for oxygen reduction electrocatalysis. Heliyon 7, e08076. doi:10.1016/j.heliyon.2021.e08076
Yadav, A. A., Lokhande, A. C., Kim, J. H., and Lokhande, C. D. (2017). High electrochemical performance asymmetric supercapacitor based on La2O3//Co3O4 electrodes. J. Ind. Eng. Chem. 56, 90–98. doi:10.1016/j.jiec.2017.06.051
Yang, X., Cai, C., Zou, Y., Xiang, C., Chu, H., Yan, E., et al. (2020). Co3O4-doped two-dimensional carbon nanosheet as an electrode material for high-performance asymmetric supercapacitors. Electrochim. Acta. 335, 135611. doi:10.1016/j.electacta.2020.135611
Yayeh, Z., Kahsay, G., Negussie, T., and Ketema, A. (2024). Dependence of critical temperature on doping and oxygen reduction in Nd2-xCexCuO4-δ superconductor. Nano Sel., e202300040. doi:10.1002/nano.202300040
Yue, X., Wang, G., Wang, J., Fan, L., Hao, J., Wang, S., et al. (2022). Nanosheets prepared by hydrothermal method. Coatings, 1–11. doi:10.3390/coatings12111682
Keywords: cubic spinel, supercapacitor, Ni and Cu doped Co3O4, specific capacitance, electrochemical
Citation: Worku AK, Asfaw A and Ayele DW (2024) Engineering of Co3O4 electrode via Ni and Cu-doping for supercapacitor application. Front. Chem. 12:1357127. doi: 10.3389/fchem.2024.1357127
Received: 17 December 2023; Accepted: 02 April 2024;
Published: 18 April 2024.
Edited by:
Pramod K. Kalambate, University of Waterloo, CanadaReviewed by:
Sriram Balasubramanian, National Taipei University of Technology, TaiwanDewei Wang, North Minzu University, China
Copyright © 2024 Worku, Asfaw and Ayele. This is an open-access article distributed under the terms of the Creative Commons Attribution License (CC BY). The use, distribution or reproduction in other forums is permitted, provided the original author(s) and the copyright owner(s) are credited and that the original publication in this journal is cited, in accordance with accepted academic practice. No use, distribution or reproduction is permitted which does not comply with these terms.
*Correspondence: Ababay Ketema Worku, d29ya3VrZXRlbWE5MUBnbWFpbC5jb20=