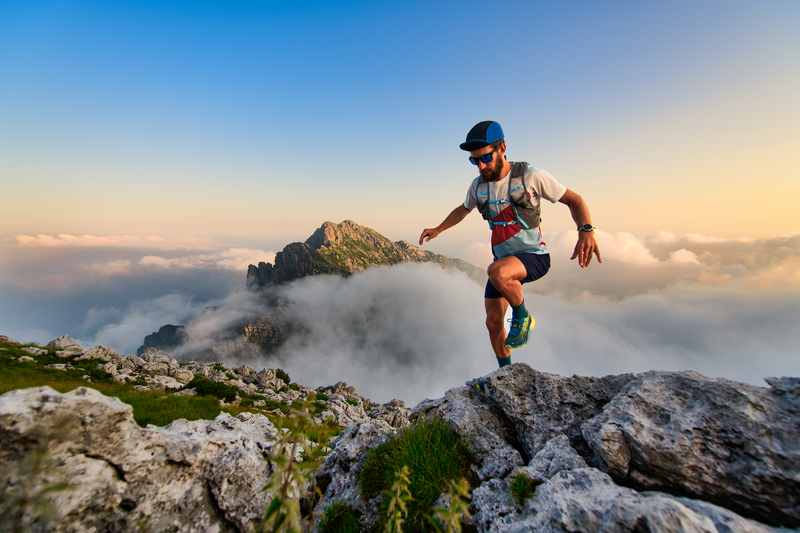
95% of researchers rate our articles as excellent or good
Learn more about the work of our research integrity team to safeguard the quality of each article we publish.
Find out more
ORIGINAL RESEARCH article
Front. Chem. , 26 March 2024
Sec. Solid State Chemistry
Volume 12 - 2024 | https://doi.org/10.3389/fchem.2024.1354690
This article is part of the Research Topic Syntheses Under Extreme Conditions View all 9 articles
The multi-anionic compound with the composition Dy36O11F50[AsO3]12 ∙ H2O, which can be described in the non-centrosymmetric cubic space group F
Regardless of the oxidation state at the involved arsenic atoms, rare-earth metal oxoarsenates can serve well as either host materials or concentrated phosphors for lumogenious applications. This holds for the monazite-, xenotime-, and scheelite-type oxoarsenates(V) RE[AsO4] (Schäfer and Will, 1971; Lohmüller et al., 1973; Long and Stager, 1977; Schäfer et al., 1979; Brahim et al., 2002; Kang et al., 2005a; Kang, 2009; Kang and Schleid, 2005; Schmidt et al., 2005; Hartenbach et al., 2006; Golbs et al., 2009; Metzger, 2012; Metzger et al., 2016; Ledderboge et al., 2018; Goerigk, 2021; Adala et al., 2022) (RE = rare-earth metal: Sc, Y, La, Ce–Lu), where O2−-to-As5+ ligand-to-metal charge-transfer processes (LMCT) within the tetrahedral [AsO4]3− anions have a beneficial impact on the necessary energy transfer as well as for the oxoarsenates(III) RE[AsO3] and RE4[As2O5]2[As4O8] (Ben Hamida et al., 2005; Kang, 2009; Kang and Schleid, 2006; Ben Hamida, 2007; Metzger, 2012; Metzger et al., 2012; Ledderboge et al., 2014; Ledderboge, 2016; Locke et al., 2023), where the O2−-to-As3+ LMCT is supported by the lone-pair antenna at the trivalent arsenic centers. The latter occur as ψ1-tetrahedral [AsO3]3− groups either isolated in the first cases (Pb[SeO3]- or K[ClO3]-type RE[AsO3]) or vertex-condensed to di- and tetranuclear anions (pyro-[As2O5]4− and cyclo-[As4O8]4−) for the latter ones (RE4[As2O5]2[As4O8] ≡ 2 × RE2As4O9). Driven by the influence of fluxing halides during the corresponding preparation efforts, halide-derivatized rare-earth metal(III) oxoarsenates(III) were obtained for the first time, exhibiting the empirical formula RE5X3[AsO3]4 (X = F (Ledderboge and Schleid, 2014; Ledderboge, 2016; Goerigk, 2021), Cl (Kang, 2009; Hamida and Wickleder, 2006; Ben Hamida, 2007; Schander, 2009; Ledderboge, 2016; Goerigk et al., 2019), and Br (Ledderboge, 2016; Goerigk, 2021)). With X = Cl and Br as soft halide anions, they occur as layered structures, while the fluoride-derivatives represent three-dimensionally hard materials according to the Pearson HSAB concept of “hard and soft acids and bases“ (Pearson, 1963). For this reason, they are well-suited as host substrates, which secure energy transfer with minimal losses from the rigid lattice and its hard components (RE3+, F− and [AsO3]3−) to the Ln3+ activator cations, such as Eu3+ and Tb3+ as most prominent ones. The crystallization and single-phase preparation of most fluoride oxoarsenates(III) RE5F3[AsO3]4 was not an easy task in the past, so we tried different synthetic pathways to tackle this challenge. In the case of Dy5F3[AsO3]4, one of these attempts involved a droplet of water added to the appropriate mixture of the well-ground solid starting materials (Dy2O3, DyF3, and As2O3 in a 2 : 1: 2 molar ratio) in order to heat it in a sealed gold ampoule within the set-up of a Boyd and England-type piston-cylinder high-pressure apparatus. As a surprising result, big well-faceted single crystals of what turned out to be Dy36O11F50[AsO3]12 with one molecule of interstitial water per formula unit were obtained. We report here on its fascinating unique crystal structure and several analytic methods to confirm its true nature as dysprosium(III) oxide fluoride oxoarsenate(III) hydrate according to Dy36O11F50[AsO3]12 · H2O.
Since phase-pure and single-crystalline RE5F3[AsO3]4 representatives are relatively difficult to access and preparative attempts to obtain them with conventional techniques from fused silica ampoules often led to oxosilicates, a reaction under high-pressure conditions in an inert gold capsule was considered as an alternative. Dysprosium sesquioxide (Dy2O3), dysprosium trifluoride (DyF3), and arsenic sesquioxide (As2O3) served as reactants in a molar ratio of 2 (164 mg): 1 (48 mg): 1 (87 mg). A fine blend of the reactants was prepared and filled into a gold ampoule (4 mm diameter and 10 mm length), which already contained some demineralized water (30 µL). To prevent water loss while sealing the ampoule, the upper fold was closed by cold welding with a pressure of almost 10 tons at the fold. The ampoule produced this way was placed in a rock-salt pressure cell (Figure 1) and then inserted into the pressure tube of the end-loaded high-pressure piston-cylinder reactor (Boyd and England-type) (Boyd and England, 1960; Massonne and Schreyer, 1986). The operating pressure was set to just 8.5 kbar at a temperature of 500°C for 4 days, then lowered to 400°C and dwelled for a further 3 days. After the end of the experiment and opening of the ampoule, a few very large cube-shaped colorless transparent single crystals, some with an edge length of several 100 µm (Figure 2), were found. These did not cause any rotation of the linearly polarized light in transmitted light under crossed polarizers, which is why the presence of a crystal in the cubic crystal system was assumed (Nickel, 1971). Due to the size, the habit, and the apparently cubic symmetry, it was initially wrongly assumed that the crystals should be sodium chloride (NaCl) deriving from the pressure cell. However, as the crystals did not dissolve in water, one of them was isolated and examined using single-crystal X-ray diffraction. The measurement was carried out with a STADI-VARI single-crystal diffractometer (Stoe & Cie, Darmstadt, Germany). A face-centered cubic metric with a ≈ 2590 pm was found, which could not be assigned to any known class of rare-earth metal(III) compounds. The composition Dy36O11F50[AsO3]12 ∙ H2O in space group F
Figure 1. Schematic structure of the rock salt-type pressure cell of the Boyd and England piston-cylinder apparatus 1) sheathed thermocouple (NiCr-Ni), 2) upper seal (fired pryophyllite ring and steel plug), 3) gold capsule with sample material (4 mm outer diameter), 4) steel furnace, 5) lower piston ring, 6) rock-salt cell, and 7) copper paste (Boyd and England, 1960; Massonne and Schreyer, 1986).
The cubic compound Dy36O11F50[AsO3]12 ∙ H2O crystallizes in the non-centrosymmetric space group F4
Table 2. Fractional atomic coordinates, Wyckoff positions, and coefficients of the equivalent isotropic displacement parameters of Dy36O11F50[AsO3]12 ∙ H2O.
Figure 3. Coordination polyhedra of the compositions [(Dy1)O4.333F2.667]8.333– (left), [(Dy2)O3.667F4.333]8.667– (mid), and [(Dy3)O4.667F3.333]9.667– (right) as well as the As3+ cations covalently bonded to most oxygen atoms and their remaining oxygen atoms in ψ1-tetrahedral [AsO3]3– anions in the crystal structure of Dy36O11F50[AsO3]12 ∙ H2O.
In the binary dysprosium sesquioxide (Dy2O3, bixbyite type), the dysprosium-oxygen distances are in the range of 215–254 pm, while in dysprosium oxide fluoride (DyOF, YOF type), they occur in a narrower interval of 227–234 pm. Thus, the refined bond lengths in Dy36O11F50[AsO3]12 ∙ H2O are in good agreement with those of the simpler representatives Dy2O3 and DyOF (Rudenko and Boganov, 1970; Dutton et al., 2012). For the distances to the F− anions, a similar situation is observed, as they are 242–249 pm in DyOF and in binary DyF3 (YF3 type) in between 234 and 274 pm (Garashina and Sobolev, 1971; Dutton et al., 2012). Here, F− anions always show slightly longer bonds to the Dy3+ cations as compared to the O2– anions, while the O2– and F− anions as well as arsenic-bonded oxygen atoms in Dy36O11F50[AsO3]12 ∙ H2O have quite similar distances to the Dy3+ cations (Table 3).
The polyhedra around the Dy3+ cations are linked by both corners and edges with different patterns. For a better understanding, in the following, first the linkages of Dy3+-centered polyhedra to each other with crystallographically identical Dy3+ cations are described. The [(Dy1)O4.333F2.667]8.333– polyhedra are connected to each other by common edges, and always four of these polyhedra form a tetrahedral body in which the central oxide anion (O4)2– is tetrahedrally surrounded exclusively by four (Dy1)3+ cations (Figure 4, left). Two oxoarsenate(III) units [AsO3]3– are attached to each polyhedron, with only one common oxygen atom being present. In the case of the [(Dy2)O3.667F4.333]8.667– antiprisms, the situation is different: here, only three polyhedra are linked to each other exclusively by common edges. It is interesting to note that all three polyhedra share the (F1)- anion and the three oxygen atoms of an oxoarsenate(III) unit of the (As2)3+ cation provide the remaining edge links.
Figure 4. Linkage pattern of Dy3+-centered coordination polyhedra in the crystal structure of Dy36O11F50[AsO3]12 ∙ H2O with drawing of the linkage to polyhedra with the crystallographically identical central cations (Dy1)3+ (left), (Dy2)3+ (mid), and (Dy3)3+ (right).
The [(As2)O3]3– anion thus bridges all three (Dy2)3+-centered polyhedra. This creates a distorted tetrahedral gap at the center of an imaginary triangular surface through the three (Dy2)3+ cations (Figure 4, mid). In the case of the [(Dy3)O4.667F3.333]9.667– polyhedra, the situation is quite similar. Here, three antiprisms are connected by common edges, but the main difference to the case of the (Dy2)3+ cation lies in the bridging atoms. Here, the linked [(Dy3)O4.667F3.333]9.667– polyhedra are surrounded on both sides by ψ1-tetrahedra [(As3)O3]3–, forming an empty trigonal prism from the oxygen atoms of these oxoarsenate(III) units (Figure 4, right).
The other linkage patterns are quite similar and are described here, but not further shown graphically. The polyhedra around (Dy1)3+ are connected with three (Dy2)3+-centered polyhedra each, with one edge and two corner links present. There are also three contacts to the (Dy3)3+-centered polyhedra, with two edge and only one corner linkage occurring here. For the [(Dy2)O3.667F4.333]8.667– antiprism there are, in addition to the already mentioned, three contacts to the capped [(Dy1)O4.333F2.667]8.333– prisms (twice via edge and once via corner); there are also contacts to four (Dy3)3+-centered polyhedra, with two edge and two corner-connections. The [(Dy3)O4.667F3.333]9.667– antiprism is linked to three (Dy1)3+- and four (Dy2)3+- centered polyhedra, where it is linked to the [(Dy1)O4.333F2.667]8.333– polyhedra twice via edge and once over corner and to the [(Dy2)O3.667F4.333]8.667– antiprisms twice via edge and twice via corner.
The crystal structure exhibits three crystallographically different sites for the As3+ cations as well (Table 2). The first and second coordination spheres of these arsenic centers are identical in all three cases, only the bond lengths show a slight variance. In all cases, isolated ψ1-tetrahedra [AsO3]3– are formed, whose oxygen atoms each have contact with a terminal and two bridging Dy3+ cations (Figure 5). Thereby, the arsenic-oxygen distances with values of 177–179 pm are in a rather narrow range, but very close to the typical arsenic(III)-oxygen bonds in claudetite-I (172–181 pm) (Pertlik, 1978a), claudetite-II (177–182 pm) (Pertlik, 1975), and arsenolite (179 pm, 3×; Table 3) (Pertlik, 1978b), to name just those of the crystalline As2O3 modifications.
Figure 5. The first and second coordination sphere of the As3+ cations occurring in the crystal structure of Dy36O11F50[AsO3]12 ∙ H2O as ψ1-tetrahedral [AsO3]3– anions with their Dy3+ decoration.
In the crystal structure, there are also eight anion positions, which do not maintain any contacts to the As3+ cations (Figure 6). The corresponding elements were assigned to the sites in such a way that all oxygen atoms are tetrahedrally surrounded by Dy3+ cations (C.N. = 4), while angled and trigonal coordination spheres also occur for the F− anions (C.N. = 2 and 3). Two peculiarities stand out here: on the one hand, there is a mixed-occupied position with the (O5)2– and (F5)– anions, which is necessary for the charge neutrality of the compound; on the other hand, it is not possible to say with certainty whether only this site is actually mixed and all the other anion positions of Table 2 are occupied by only one kind of non-metallic element.
Figure 6. The anion sites occurring in the crystal structure of Dy36O11F50[AsO3]12 ∙ H2O without binding contacts to As3+ cations and their extended environments for (F1)− (A), (F2)− (B), (F3)− (C), (F4)− (D), (O4)2− (E), (O5/F5)1.667− (F) and (F6)− (G).
The (O4)2–-centered (Dy3+)4 tetrahedron could also be mixed with fluoride, since the coordination spheres hardly differ. However, since the counts (= multiplicity of Wyckoff positions) of the (O4)2– and (O5)2– sites and the possible variance of the negative charges due to the different Wyckoff positions (24d versus 96h) differ, charge neutrality would not be achievable by a pure mixed occupation of the (O4)2– site. From a merely mathematical point of view, it would also be possible for both positions to be mixed, but for the sake of simplicity, only a mixed occupation of the (O5)2– site was assumed as a structural model. Furthermore, oxygen as an O2– anion also strives for higher coordination numbers than F− anions, which is why a mixed occupation of the only two- and three-coordinated anion sites can be regarded as rather unlikely. The tendency of oxygen atoms, which are not covalently bonded to As3+ cations, to be coordinated by Ln3+ cations in quaternary lanthanoid(III) oxide halide oxoarsenates(III) in the form of [OLn4]10+ tetrahedra is already well-known from literature. In the compounds with the structured formula Ln3OX[AsO3]2 (Ln = La–Nd, Sm–Dy for X = Cl, Ln = La–Nd, Sm, Gd–Dy for X = Br, and Ln = Pr for X = I) such [OLn4]10+ tetrahedra are present, which share common edges according to
The point of highest residual electron density is located at the origin of the unit cell (8a: 0, 0, 0). In terms of the scattering power, the intensity corresponds to a position occupied by an oxygen atom. However, this hypothetical oxygen atom has no binding contacts with other particles present in the unit cell. The closest contact is at 368 pm to As3+ cations and at 371 pm to F− anions, well outside the range of plausible chemical bonding. However, based on the synthesis parameters, one explanation would be that this could be the oxygen atom of a crystal water molecule. Upon further search for a suitable hydrogen atom, residual electron density can also be found at a distance of about 96.4 pm (32e: x, x, x, with x = 0.0215) from this oxygen atom. However, due to the applicable symmetry operations, this hydrogen atom would be arranged tetrahedral around the central oxygen atom, a circumstance that does not seem to make sense from a structural-chemical point of view. An under-occupation of this hydrogen position by one half to achieve a neutral water molecule (H2O) would be possible and very likely with a H–O–H angle of 109.5°, but hardly be detected by X-ray diffraction. The hypothetical empirical formula of the title compound would then be Dy36O11F50[AsO3]12 ∙ H2O. The coordination environment of this interstitial crystal-water molecule is shown in Figure 7 with minimal H∙∙∙As distances of 272 pm and H∙∙∙F distances of 325 pm, both far too long for significant bridging hydrogen bonds. Figure 8 presents a section of the whole crystal structure including the cell edges.
Figure 7. Tetrahedral coordination environment of the oxygen atom Ow in the crystal structure of Dy36O11F50[AsO3]12 ∙ H2O, which can be explained by an intercalated crystal-water molecule in a rhomboid-dodecahedral cavity formed by (As1)3+, (As2)3+, and (F2)–.
Figure 8. Section of the crystal structure of Dy36O11F50[AsO3]12 ∙ H2O as viewed along the a-axis. The covalent bonds of the As3+ cations to their oxygen atoms within the ψ1-tetrahedral [AsO3]3– anions and the ones in the crystal-water molecules are emphasized.
In order to investigate the composition of the obtained material, powder X-ray diffraction techniques were applied (Figure 9). Since the available amount of the sample was rather low, the signal-to-noise-ratio of the data is challenging.
Figure 9. Measured (blue) and calculated (green) powder X-ray diffractogram of Dy36O11F50[AsO3]12 ∙ H2O. Unindexed reflections are marked with asterisks.
The target compound Dy36O11F50[AsO3]12 ∙ H2O could be identified as the main phase in the mixture. However, multiple reflections that do not belong to the target compound are also present (marked with “asterisks" *). Regarding the reaction conditions, the theoretical patterns of the starting materials and possible by-products were used to check if one or several of these phases are present in the reaction mixture. Nevertheless, none of the tested compounds (As2O3 in its claudetite- and arsenolite-type, respectively, Dy2O3 (A-, B- and C-type), DyOF, DyF3, Dy[AsO4] (xenotime- and scheelite-type), and even Dy5F3[AsO3]4) could be identified as possible side-phases.
Since no As2O3 and Dy2O3 seem to be residual, a complete reaction of the starting materials should have taken place that lead to multiple products of which the target compound Dy36O11F50[AsO3]12 ∙ H2O can be seen as the main phase.
To further investigate the mysterious crystal and to verify the interstitial crystal-water molecules, a single-crystal Raman spectrum of Dy36O11F50[AsO3]12 ∙ H2O was recorded with an excitation wavelength of λ = 638 nm (Figure 10). The peaks in the range from 100 to 500 cm–1 (107, 168, 206, 293, 353, 424 cm–1) can be assigned to the stretching vibrations ν(Dy(O,F)) and the deformation vibrations δ(AsO3) with the three strongest ones probably belonging to ν(Dy(O,F)) and lattice vibrations. The two peaks at 608 and 655 cm–1 with the shoulder at 700 cm–1 are caused by the antisymmetric stretching vibrations νas(AsO3) of the three crystallographically different [AsO3]3– anions, whereas the significantly stronger three peaks at 733, 763, and 792 cm–1 undoubtedly belong to the symmetric stretching vibrations νs(AsO3) of these units. The slight elevation at 1600 cm–1 can be assigned to the deformation vibrations δ(H2O) of the crystal-water molecules, while the very sharp peak at 3607 cm1 belongs to the symmetrical valence vibration νs(H2O) of them and the somewhat smaller one at 3637 cm–1 stems from the antisymmetrical one νas(H2O). The two weaker and broader peaks at 3520 and 3553 cm–1 can be interpreted as results from the stretching vibrations ν(H2O)n of other very few water species that are bound to the detected crystal-water molecule, but may also well be surface-bonded water of the investigated crystal. The very broad band from 3000 to 3400 cm–1 can be attributed to air humidity and is always observable in spectra from this kind of instrument (Weidlein et al., 1981; Weidlein et al., 1986).
Figure 10. Single-crystal Raman spectrum of Dy36O11F50[AsO3]12 ∙ H2O recorded at an excitation wavelength of λ = 638 nm.
Electron microscopy and X-ray spectroscopy methods were used to further characterize Dy36O11F50[AsO3]12 ∙ H2O. Figure 11 shows a backscattered electron image of a single crystal, which demonstrates the above-average crystal size and the almost isotropic crystal growth. Before the single-crystal X-ray diffraction, the likewise recognizable covering particles were rinsed off by washing them in paraffin oil.
Figure 11. Backscattered electron image of a single crystal of Dy36O11F50[AsO3]12 ∙ H2O recorded at an accelerating voltage of 15 kV and a current of 4 nA. The formed edges of the crystal are clearly visible.
Qualitative wavelength-dispersive X-ray spectra (WDXS) were recorded to verify the elements assumed in the single-crystal structure refinement. For the lighter elements, pseudo-crystal multilayer elements were used as diffraction crystals in the spectrometers in order to reach the low-energy regions. The relevant spectra of the measurements carried out on the single crystal are shown in Figure 12. The energy range for the heavy atoms dysprosium and arsenic corresponds to the expectations. In the energy ranges not shown here outside these characteristic lines, there are no extraneous bands from other types of atoms. It can also be seen that it would not be possible to detect arsenic using the (energy-dispersive) EDXS method, as interferences of Dy-Mα and Dy-Mβ with the As-Lα and As-Lβ lines occur, which can be resolved in the wavelength-dispersive system. The following findings can be obtained in the low-energy range of Figures 12C, D: it can be seen that both fluorine and oxygen are present and, furthermore, higher orders of the Dy-Mα and Dy-Mβ lines are also detectable in the fluorine region, but with sufficiently low interference, at least for qualitative detection.
Figure 12. Wavelength-dispersive X-ray spectra (WDXS) of Dy36O11F50[AsO3]12 ∙ H2O in different energy ranges with the relevant emission lines depicted. Given are the regions of Dy-Lα [LiF crystal, (A)], As-Lα and As-Lβ [TAP crystal, (B)], as well as O-Kα and F-Kα (on two different spectrometers with multilayer elements, (C, D), respectively).
An unexpected cubic dysprosium(III) oxide fluoride oxoarsenate(III) hydrate with the composition Dy36O11F50[AsO3]12 · H2O could be obtained by water-assisted high-pressure synthesis from cold-welded gold ampoules in an attempt to synthesize Dy5F3[AsO3]4. Its crystal structure features Dy3+ cations with coordination numbers of seven and eight with respect to the non-metal elements (O and F) along with discrete ψ1-tetrahedral [AsO3]3− anions. Interstitial crystal-water molecules are trapped within a large cavity confined by eight arsenic atoms and six fluoride anions. Upon heating some crystals up to 500 °C for several days, they were destroyed owing to decrepitation under water-release. From a distance, the “odd” composition Dy36O11F50[AsO3]12 · H2O with a = 2587.59(14) pm for Z = 8 could well be misinterpreted as “Dy3OF4[AsO3] · 1/12 H2O” with Z = 96 and even the low water-content might have been overlooked. Under these circumstances, the resulting formula “Dy36O12F48[AsO3]12 · H2O” for Z = 8 would have only 12 + 48 = 60 non-metal elements without bonds to arsenic instead of 11 + 50 = 61 as in the true composition. But after all, we have found no evidence for an under-occupation concerning any of these seven non-metal positions in Table 2.
The original contributions presented in the study are included in the article/Supplementary material, further inquiries can be directed to the corresponding author.
FG: Conceptualization, Data curation, Formal Analysis, Investigation, Methodology, Resources, Software, Validation, Visualization, Writing–original draft, Writing–review and editing. RL: Conceptualization, Data curation, Formal Analysis, Investigation, Methodology, Project administration, Resources, Software, Validation, Visualization, Writing–original draft, Writing–review and editing. TS: Conceptualization, Data curation, Formal Analysis, Investigation, Methodology, Project administration, Resources, Software, Supervision, Validation, Visualization, Writing–original draft, Writing–review and editing.
The author(s) declare that no financial support was received for the research, authorship, and/or publication of this article.
We would like to thank Dr. Falk Lissner (AOR, University of Stuttgart) for the single-crystal measurement and Dr. Klaus Locke (Robert Bosch GmbH, Reutlingen) for his support in analyzing the Raman spectrum.
The authors declare that the research was conducted in the absence of any commercial or financial relationships that could be construed as a potential conflict of interest.
All claims expressed in this article are solely those of the authors and do not necessarily represent those of their affiliated organizations, or those of the publisher, the editors and the reviewers. Any product that may be evaluated in this article, or claim that may be made by its manufacturer, is not guaranteed or endorsed by the publisher.
Adala, N., Marzougui, B., Ben Smida, Y., Marzouki, R., Ferhi, M., and Onwudiwe, D. C., (2022). An experimental and theoretical study of the structural, optical, electrical, and dielectric properties of PrAsO4. J. Alloys Compd. 910, 164894. doi:10.1016/j.jallcom.2022.164894
Ben Hamida, M. (2007). Oxo-Selenate(IV) und Oxo-Arsenate(III) der Selten-Erd-Metalle und ihre Derivate. Dissertation. Oldenburg: Carl von Ossietzky Universität Oldenburg.
Ben Hamida, M., Warns, C., and Wickleder, M. S. (2005). Syntheses and Crystal Structures of RE2As4O9 (RE = Nd, Sm): Oxo-Arsenates(III) according to RE4(As2O5)2(As4O8) Exhibiting the Cyclic As4O84– Anion, Z. Naturforsch. 60b, 1219–1223.
Ben Yahia, H., Pöttgen, R., and Rodewald, U. C. (2009). Crystal Structure of the New Phosphate AgMnPO4, Z. Naturforsch 64b, 896–900.
Ben Yahia, H., Pöttgen, R., and Rodewald, U. C. (2010). Condensed [OPr4]10+ and Discrete [AsO3]3−-Ψ1-Tetrahedra in Pr5O4Cl[AsO3]2, Z. Naturforsch 65b, 1289–1292.
Boyd, F., and England, J. (1960). Apparatus for phase-equilibrium measurements at pressures up to 50 kilobars and temperatures up to 1750°C. J. Geophys. Res. 65, 741–748. doi:10.1029/jz065i002p00741
Brahim, A., Ftini, M. M., and Amor, H. (2002). Cerium arsenate, CeAsO4, Acta Crystallogr. E58, i98–i99.
Dutton, S., Hirai, D., and Cava, R. (2012). Low temperature synthesis of LnOF rare-earth oxyfluorides through reaction of the oxides with PTFE. J. Mater. Res. Bull. 47, 714–718. doi:10.1016/j.materresbull.2011.12.014
Garashina, L., and Sobolev, D. (1971). X-ray and neutron diffraction study of the defect crystal structure of the as-grown nonstoichiometric phase Y0.715Ca0.285F2.715, Sov. Phys. Crystallogr. 16, 254–258.
Goerigk, F. C. (2021). Synthese und Charakterisierung von Seltenerdmetall-Oxidoarsenaten und -antimonaten sowie deren Anwendungsbezug. Dissertation. Stuttgart: Universität Stuttgart
Goerigk, F. C., Schander, S., Hamida, M. B., Kang, D.-H., Ledderboge, F., and Wickleder, M. S., (2019). Die monoklinen Seltenerdmetall(III)-Chlorid-Oxidoarsenate(III) mit der Zusammensetzung SE5Cl3[AsO3]4 (SE = La–Nd, Sm), Z. Naturforsch. 74b, 497–506.
Golbs, S., Cardoso-Gil, R., and Schmidt, M. Z. (2009). Crystal structure of europium arsenate, EuAsO4. Z. Kristallogr. 224, 169–170. doi:10.1524/ncrs.2009.0076
Hamida, B. M., and Wickleder, M. S. Z. (2006). Nd5(AsO3)4Cl3: the first oxo-arsenate(III)-chloride of the lanthanides. Z. Anorg. Allg. Chem. 632, 2195–2197. doi:10.1002/zaac.200600173
Hartenbach, I., Müller, A. C., and Schleid, Th. Z. (2006). CuGd3SiS7: ein Gadoliniumsulfid mit zwei isolierten komplexen Thioanionen gemäß Gd3[CuS3] [SiS4]. Z. Anorg. Allg. Chem. 632, 2147. doi:10.1002/zaac.200670141
Herrendorf, W., and Habitus, B. H., Program for the optimisation of the crystal shape for numerical absorption correction, Universities of Karlsruhe and Gießen: Karlsruhe, Gießen (Germany), 1993, 1997. In X-SHAPE (version 1.06), STOE & Cie.: Darmstadt (Germany), 1999.
Kang, D.-H. (2009). Oxoarsenate(III/V) und Thioarsenate(III) der Selten-Erd-Metalle. Dissertation. Stuttgart: Universität Stuttgart
Kang, D.-H., Höss, P., and Schleid, Th. (2005a). Xenotime-Type Ytterbium(III) Oxoarsenate(V), Yb[AsO4]. Acta Crystallogr. E61, i270–i272.
Kang, D.-H., Komm, T., and Schleid, Th. Z. (2005b). Gd3OCl[AsO3]2: Das erste Oxidchlorid-Oxoarsenat(III) der Lanthanoide. Kristallogr S22, 157.
Kang, D.-H., and Schleid, Th. Z. (2005). Einkristalle von La[AsO4] im Monazit- und Sm[AsO4] im Xenotim-Typ. Z. Anorg. Allg. Chem. 631, 1799–1802. doi:10.1002/zaac.200500209
Kang, D.-H., and Schleid, Th. Z. (2006). Sm2As4O9: Ein ungewöhnliches Samarium(III)-Oxoarsenat(III) gemäß Sm4[As2O5]2[As4O8]. Z. Anorg. Allg. Chem. 632, 91–96. doi:10.1002/zaac.200500333
Kang, D.-H., and Schleid, Th. Z. (2007). La3OCl[AsO3]2: Ein Lanthan-Oxidchlorid-Oxoarsenat(III) mit “lone-pair”-Kanalstruktur. Z. Anorg. Allg. Chem. 633, 1205–1210. doi:10.1002/zaac.200700099
Ledderboge, F. (2016). Synthese und Charakterisierung von Oxo- und Thioarsenaten(III/V) der Seltenerdmetalle und ihrer Derivate. Dissertation. Stuttgart: Universität Stuttgart.
Ledderboge, F., Metzger, S. J., Heymann, G., Huppertz, H., and Schleid, Th. (2014). Dimorphic cerium(III) oxoarsenate(III) Ce[AsO3]. Solid State Sci. 37, 164–169. doi:10.1016/j.solidstatesciences.2014.08.005
Ledderboge, F., Nowak, J., Massonne, H.-J., Förg, K., Höppe, H. A., and Schleid, Th. (2018). High-pressure investigations of yttrium(III) oxoarsenate(V): crystal structure and luminescence properties of Eu3+-doped scheelite-type Y[AsO4] from xenotime-type precursors. J. Sol. State Chem. 263, 65–71. doi:10.1016/j.jssc.2018.03.002
Ledderboge, F., and Schleid, Th. Z. (2014). The First Lanthanoid(III) Fluoride Oxoarsenate(III): Lu5F3[AsO3]4. Z. Anorg. Allg. Chem. 640, 2350.
Locke, R. J. C., Ledderboge, F., and Schleid, Th. (2023). Zur Kenntnis ternärer Oxoarsenate(III) dreiwertiger Lanthanoide: Synthese und Charakterisierung von LnAsO3− und Ln2As4O9−Vertretern mit Ln = La und Ce sowie Ln = Pr, Nd, Sm–Gd. Z. Naturforsch. 78b, 525–536.
Lohmüller, G., Schmidt, G., Deppisch, B., Gramlich, V., and Scheringer, C. (1973). Die Kristallstrukturen von Yttrium-Vanadat, Lutetium-Phosphat und Lutetium-Arsenat. Acta Crystallogr. B29, 141–142.
Long, F. G., and Stager, C. V. (1977). Low temperature crystal structure of TbAsO4 and DyAsO4. Can. J. Phys. 55, 1633–1640. doi:10.1139/p77-208
Massonne, H.-J., and Schreyer, W. N. (1986). High-pressure syntheses and X-ray properties of white micas in the system K2O-MgO-Al2O3-SiO2-H2O. Jahrb. Min. 153, 177–215.
Metzger, S. J. (2012). Hochdruckmodifikationen von Oxoarsenaten(V) und -arsenaten(III) der Selten-Erd-Metalle und Lithium-Mangan-Eisen-Oxophosphat(V) als Kathodenmaterial für Lithium-Akkumulatoren. Dissertation. Stuttgart: Universität Stuttgart
Metzger, S. J., Heymann, G., Huppertz, H., and Schleid, Th. (2012). La[AsO3]: Lanthanum Oxo-arsenate(III) with K[ClO3]− Type Crystal Structure. Z. Anorg. Allg. Chem. 637, 1119–1122.
Metzger, S. J., Ledderboge, F., Heymann, G., Huppertz, H., and Schleid, Th. Z. (2016). High-pressure investigations of lanthanoid oxoarsenates: I. Single crystals of scheelite-type Ln[AsO4] phases with Ln = La–Nd from monazite type precursors, Z. Naturforsch 71b, 439–445.
Pearson, R. G. (1963). Hard and soft acids and bases. J. Am. Chem. Soc. 85, 3533–3539. doi:10.1021/ja00905a001
Pertlik, F. (1975). Die Kristallstruktur der monoklinen Form von As2O3 (Claudetit II). Monatsh. Chem. / Chem. Mon. 106, 755–762. doi:10.1007/bf00902181
Pertlik, F. (1978a). Theoretical studies on arsenic oxide and hydroxide species in minerals and in aqueous solution, Monatsh. Chem. / Chem. Mon. 109, 277–282. doi:10.1007/bf00906344
Pertlik, F. (1978b). Strukturverfeinerung von kubischem As2O3 (Arsenolith) mit Einkristalldaten. Czech. J. Phys. 28, 170–176. doi:10.1007/bf01591036
Rudenko, V., and Boganov, A. (1970). Stoichiometry and phase transitions in rare earth oxides. Moscow: Techn Ber Inst of Silicate Chem,
Schäfer, W. P., and Will, G. (1971). Neutron diffraction study of antiferromagnetic DyAsO4. J. Phys. C4, 3224–3233.
Schäfer, W. P., Will, G., and Müller-Vogt, G. (1979). Refinement of the crystal structure of terbium arsenate TbAsO4 at 77 K and 5 K by profile analysis from neutron diffraction powder data. Acta Crystallogr. B35, 588–592.
Schander, S. (2009). Struktur und Eigenschaften übergangsmetallhaltiger Oxo-arsenate(III) der Selten-Erd-Elemente. Dissertation. Oldenburg: Carl von Ossietzky Universität Oldenburg.
Schmidt, M., Müller, U., Cardoso Gil, R., Milke, E., and Binnewies, M. Z. (2005). Zum chemischen Transport und zur Kristallstruktur von Seltenerdarsenaten(V). Z. Anorg. Allg. Chem. 631, 1154–1162. doi:10.1002/zaac.200400544
Sheldrick, G. M. (1997). Shelxs-97 and Shelxl-97. Programs for the solution and refinement of crystal structures from X-ray diffraction data. Göttingen (Germany): University of Göttingen.
Weidlein, J., Müller, U., and Dehnicke, K. (1981), Stuttgart, New York: Georg-Thieme-Verlag. Schwingungsfrequenzen I – Hauptgruppenelemente, 1. Auflage
Keywords: high-pressure synthesis, oxoarsenates(III), crystal structures, crystal water, dysprosium, oxide fluorides, X-ray diffraction, microprobe analysis
Citation: Goerigk FC, Locke RJC and Schleid T (2024) Synthesis under high pressure: crystal structure and properties of cubic Dy36O11F50[AsO3]12 ∙ H2O. Front. Chem. 12:1354690. doi: 10.3389/fchem.2024.1354690
Received: 12 December 2023; Accepted: 29 January 2024;
Published: 26 March 2024.
Edited by:
Jörn Bruns, University of Cologne, GermanyReviewed by:
Rosana Mariel Romano, CEQUINOR (CONICET-UNLP), ArgentinaCopyright © 2024 Goerigk, Locke and Schleid. This is an open-access article distributed under the terms of the Creative Commons Attribution License (CC BY). The use, distribution or reproduction in other forums is permitted, provided the original author(s) and the copyright owner(s) are credited and that the original publication in this journal is cited, in accordance with accepted academic practice. No use, distribution or reproduction is permitted which does not comply with these terms.
*Correspondence: Thomas Schleid, c2NobGVpZEBpYWMudW5pLXN0dXR0Z2FydC5kZQ==
Disclaimer: All claims expressed in this article are solely those of the authors and do not necessarily represent those of their affiliated organizations, or those of the publisher, the editors and the reviewers. Any product that may be evaluated in this article or claim that may be made by its manufacturer is not guaranteed or endorsed by the publisher.
Research integrity at Frontiers
Learn more about the work of our research integrity team to safeguard the quality of each article we publish.