- 1Department of Interventional Medical Center, Affiliated Hospital of Qingdao University, Qingdao, Shandong, China
- 2Qingdao Cancer Institute, Qingdao University, Qingdao, China
In contemporary biomedical research, the development of nanotechnology has brought forth numerous possibilities for brain tumor imaging and therapy. Among these, π-conjugated materials have garnered significant attention as a special class of nanomaterials in brain tumor-related studies. With their excellent optical and electronic properties, π-conjugated materials can be tailored in structure and nature to facilitate applications in multimodal imaging, nano-drug delivery, photothermal therapy, and other related fields. This review focuses on presenting the cutting-edge advances and application prospects of π-conjugated materials in brain tumor imaging and therapeutic nanotechnology.
1 Introduction
π-Conjugated materials, a class of organic molecules or polymers with conjugated structures, possess extensive potential applications in the field of biomedicine (Pimachev et al., 2019). Their unique electronic structure and optical properties make them ideal candidates for biomedical imaging and therapy (Ma et al., 2021; Deng et al., 2022). By altering their conjugated structure and side-chain functional groups, π-conjugated materials can modulate absorption and fluorescence emission peaks, enabling high-selectivity imaging of biological tissues. Additionally, due to their excellent photothermal conversion performance, they can be utilized in photothermal therapy, generating localized temperature elevation through light energy conversion to deactivate tumor cells (Zhou and Liang, 2014; Parida et al., 2022). Consequently, the application of π-conjugated materials in biomedicine has become a focal point of research.
While π-conjugated materials have made significant strides in brain tumor imaging and therapy, their design, preparation, and clinical application still face certain limitations and challenges. To delineate the primary issues and unresolved matters concerning π-conjugated materials in these aspects, it is imperative to systematically review the existing research frontier to guide the direction of future studies. This paper aims to provide a thorough analysis and comparison of relevant research findings to clearly delineate the major knowledge gaps and limitations of π-conjugated materials in the following areas, with the intention of steering future research efforts:
Firstly, it will introduce the applications of π-conjugated materials in brain tumor imaging, including the design and preparation methods of various π-conjugated nanoprobes and their usage in multimodal imaging such as magnetic resonance imaging (MRI), fluorescence imaging, and photoacoustic imaging. By conducting comparative analyses of different imaging modalities, the advantages and challenges of π-conjugated materials in brain tumor imaging will be explored.
Secondly, this review will delve into the applications of π-conjugated materials in brain tumor therapy, with a particular focus on research progress in nanodrug delivery systems, photothermal therapy, and photodynamic therapy. Through a comprehensive review of the advantages and disadvantages of different therapeutic strategies, the potential value of π-conjugated materials in brain tumor treatment will be discussed. Moreover, the review will also discuss the application prospects of π-conjugated materials in combination therapy, exploring the synergistic effects of various treatment strategies and providing new insights and directions for precision treatment of brain tumors.
Lastly, this review will critically analyze the biological safety and toxicity assessment of π-conjugated materials in brain tumor therapy. By reviewing relevant literature, the metabolic pathways and biodistribution of π-conjugated materials in vivo, as well as their interactions with normal tissues and organs, will be comprehensively evaluated. A thorough exploration of the biocompatibility and potential toxicity of π-conjugated materials will be conducted, providing important references for further clinical applications.
Through a comprehensive review of the applications of π-conjugated materials in brain tumor imaging and therapeutic nanotechnology, we aim to deepen our understanding of this field, promote the translational application of π-conjugated materials in clinical brain tumor treatment, and provide patients with more precise and effective therapeutic options (Figure 1).
2 Application of π-conjugated materials in brain tumor imaging
2.1 Design and preparation of π-conjugated nanoprobes
π-Conjugated materials, as a unique class of nanoprobes, hold broad prospects for brain tumor imaging (Hiroto and Wu, 2019; Li et al., 2021a). In this section, we will focus on the design and preparation methods of π-conjugated nanoprobes, emphasizing their advantages and application value in brain tumor imaging. Firstly, material selection is crucial in the design of π-conjugated nanoprobes (Li et al., 2023). Generally, the materials for π-conjugated nanoprobes should possess excellent optical properties and biocompatibility (Hiroto and Wu, 2019). Commonly used materials include metallic nanoparticles, carbon nanotubes, and quantum dots. Metallic nanoparticles exhibit outstanding surface plasmon resonance effects, enhancing fluorescence signals (Haque et al., 2020); carbon nanotubes possess excellent optical properties and mechanical strength for biomedical imaging and drug delivery; quantum dots offer tunable size and narrow emission spectra, suitable for multi-channel imaging (Miki and Ohe, 2020; Lao et al., 2022). Secondly, the synthesis method is a critical step in the preparation of π-conjugated nanoprobes. Common synthesis methods include solution-based, gas-phase, and solid-phase approaches (Yoon and Dong, 2021). The solution-based method is one of the most commonly used methods, achieving controlled synthesis of nanomaterials through adjusting reaction conditions and adding surfactants (Hicks et al., 2021; M et al., 2022). The gas-phase method involves converting gaseous precursors into nanoparticles through thermal evaporation or pyrolysis (Figure 2). The solid-phase method converts solid precursors into nanomaterials through thermal treatment (Oubaha et al., 2019).
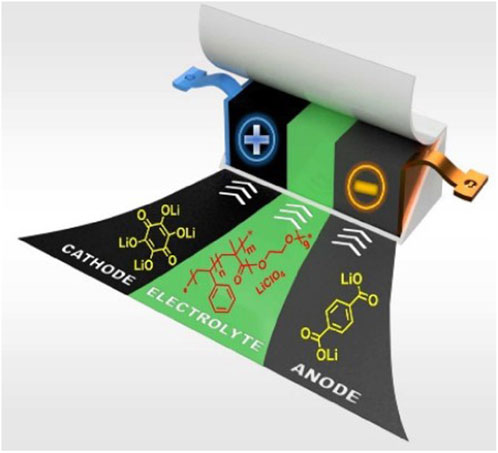
FIGURE 2. π-conjugated materials: from synthesis to applications (Oubaha et al., 2019).
Common characterization techniques include transmission electron microscopy (TEM), scanning electron microscopy (SEM), UV-Vis absorption spectroscopy, and fluorescence spectroscopy (Figure 3). TEM and SEM are used to observe the morphology and size distribution of nanoprobes; UV-Vis absorption spectroscopy characterizes their optical properties; fluorescence spectroscopy evaluates the fluorescence intensity and emission spectra of nanoprobes (Naciri et al., 2020; Zhao, 2020; Haque et al., 2023). By selecting appropriate materials, optimizing synthesis methods, and accurately characterizing performance, π-conjugated nanoprobes with excellent properties can be fabricated, providing strong support for research in areas such as biomedical imaging and drug delivery (Xu et al., 2015; Li and Pu, 2019; Song et al., 2022).
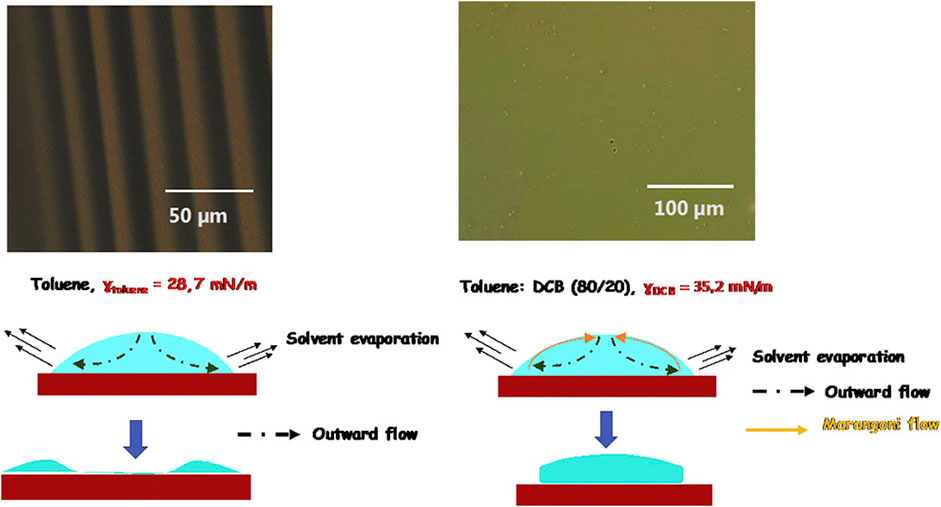
FIGURE 3. Large-scale patterning of π-conjugated materials (Richard et al., 2020).
In the practical application of brain tumors, the key to designing and preparing π-conjugated nanoprobes lies in the modulation of their structure and properties to achieve highly selective imaging of brain tumors (Stahl et al., 2017; Yin et al., 2017). Firstly, researchers typically design suitable targeting ligands, such as antibodies, oligonucleotides, and peptides, based on the specific surface biomarkers of brain tumor cells, and then modify them on the surface of π-conjugated materials (Guo et al., 2018). Such targeting modification can improve the nanoprobes’ cell recognition and affinity, achieving more accurate imaging of brain tumors (Jiang et al., 2019; Li and Pu, 2019; Neumann et al., 2021). Secondly, adjusting the physical properties of π-conjugated materials, such as tuning the fluorescence emission peak and altering the absorption spectra, enables the selection of different imaging modes (Kubo, 2019; Ong et al., 2022). For instance, fluorescence emission peaks in the near-infrared region can reduce interference from tissue autofluorescence, enhancing imaging depth and signal-to-noise ratio. By designing the size and surface modification of nanoprobes rationally, penetration through the blood-brain barrier can be achieved, enhancing the targeting of brain tumors (Samori et al., 2019). Furthermore, the stability and biocompatibility of nanoprobes are also important factors to consider in the design process (Zhang et al., 2016). Through appropriate surface modification and coating materials, the stability of nanoprobes can be enhanced, extending their circulation time in the body. Simultaneously, studying the metabolic pathways and biodistribution of nanoprobes in the body contributes to assessing their biocompatibility and safety (Montalti et al., 2015).
In conclusion, the design and preparation of π-conjugated nanoprobes involve interdisciplinary collaboration, requiring in-depth research in nanomaterials, biomedical sciences, and chemistry (Xu et al., 2015). Through careful design and rational preparation, π-conjugated nanoprobes possess high targeting specificity and biocompatibility in brain tumor imaging, providing new means and tools for early detection and quantitative analysis of brain tumors (Kaeser and Schenning, 2010; Lin et al., 2022).
2.2 Application of π-conjugated materials in multimodal imaging
The application of π-conjugated materials in multimodal imaging is currently a hot topic in brain tumor research (Ge et al., 2023). Multimodal imaging techniques integrate different imaging modalities organically, providing more comprehensive and accurate information on brain tumors, thereby offering strong support for clinical diagnosis and treatment decisions (Gu et al., 2015; Li S. et al., 2022). In this section, we will explore the application of π-conjugated materials in multimodal imaging, as well as their advantages and limitations in brain tumor imaging (Figure 4).
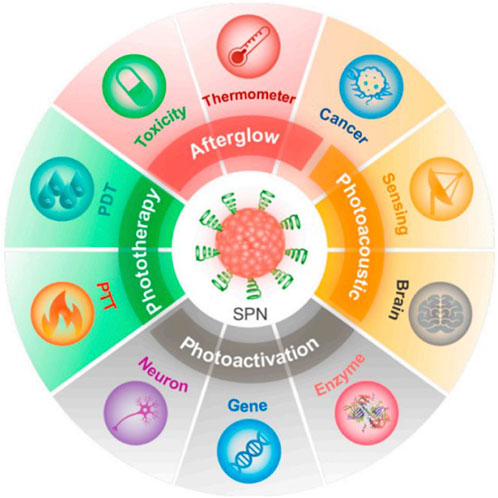
FIGURE 4. Schematic representation molecules developed for conjugated materials and photophysical processes.
π-Conjugated materials are a class of organic materials with unique electronic structures, and their electrons can freely move within the molecular backbone, forming a π-electron cloud (Mirzaei et al., 2021). This characteristic confers excellent optical properties on π-conjugated materials, including broad absorption and emission spectra and high-efficiency light conversion (Yin et al., 2021). These advantages endow π-conjugated materials with extensive applications in multimodal imaging (Zhan and Liu, 2016).
2.2.1 Magnetic resonance imaging (MRI)
The utilization of π-conjugated materials in MRI plays a pivotal role in multimodal imaging. Through surface modifications of π-conjugated materials on magnetic nanoparticles, a substantial enhancement in MRI contrast for brain tumors has been achieved, enabling high-resolution imaging. Furthermore, the tuning of their electronic structure and magnetic properties has demonstrated the potential to modulate their magnetic resonance signals, contributing to improved contrast in MRI (Zhu et al., 2018). Additionally, the targeted modification of π-conjugated nanoprobes has displayed the capacity to enhance their specificity for brain tumors, thereby augmenting the sensitivity of MRI imaging (Schmitt et al., 2018).
2.2.2 Fluorescence imaging
The outstanding fluorescence properties of π-conjugated materials have garnered significant attention in brain tumor fluorescence imaging (Doan et al., 2022). Structural modulation enables the adjustment of the fluorescence emission peak of π-conjugated materials, extending the emission into the near-infrared region to enhance imaging depth and signal-to-noise ratio (Chatterjee et al., 2020; Irshad et al., 2023). Moreover, in conjunction with targeted modifications, π-conjugated nanoprobes have achieved highly selective brain tumor imaging, offering pivotal insights for precise diagnosis. Several π-conjugated polymers and small molecules have been effectively employed in in vivo imaging, demonstrating remarkable biocompatibility and efficient fluorescence emission (Liu et al., 2013; Chen et al., 2022).
2.2.3 Photoacoustic imaging
An emerging biological imaging technique, photoacoustic imaging, has demonstrated the ability to achieve high-contrast imaging of brain tumors through photothermal effects. The exceptional photothermal conversion performance of π-conjugated materials positions them as ideal probes for photoacoustic imaging (Yao et al., 2022). In this context, π-conjugated nanoprobes can generate acoustic signals through laser-induced photothermal effects, enabling three-dimensional brain tumor imaging and presenting a novel avenue for non-invasive detection of brain tumors (Neumann et al., 2021). Some π-conjugated polymers have already proven to be efficient photoacoustic contrast agents for imaging tumors and blood vessels (Stahl et al., 2017).
2.2.4 Photothermal imaging
The photothermal conversion efficiency of π-conjugated materials has garnered extensive attention (Figure 5), and certain π-conjugated materials have demonstrated their efficiency as photothermal conversion agents for photothermal imaging and therapy (Chen et al., 2022; Yao et al., 2022).
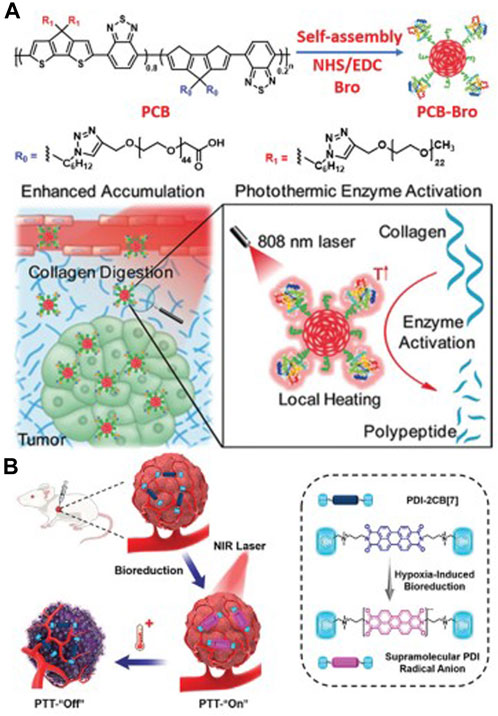
FIGURE 5. (A) The preparation process of PCB-Bro and its role in facilitating collagen digestion to enhance its tumor accumulation are depicted herein. (B) A schematic representation elucidates the generation of supramolecular PDI radical anions within tumors, serving as a specific mechanism for photothermal therapy (PTT). Adapted with permission from Lin et al. (2022).
2.3 Performance optimization and future development of imaging techniques
As the application of π-conjugated materials in brain tumor imaging becomes increasingly widespread, the optimization and development of imaging techniques become particularly important. The performance of imaging techniques directly affects the accuracy of brain tumor detection and quantitative analysis (Chen et al., 2004). In this section, we will discuss key technical optimization measures and future development directions to improve the application effectiveness of π-conjugated materials in brain tumor imaging.
2.3.1 Magnetic resonance imaging (MRI) optimization
MRI is a powerful non-invasive imaging technique widely used for diagnosing and monitoring various diseases, including brain tumors (Villanueva-Meyer et al., 2017). While MRI enables precise diagnosis without invasive procedures, there is still room for significant improvement in the quality and accuracy of the images produced. To enhance MRI contrast and sensitivity for brain tumor imaging using π-conjugated materials modified magnetic nanoparticles, further optimization of their magnetic properties is necessary (Soffietti et al., 2020; Ranjbarzadeh et al., 2023). The size, shape, and surface modifications of magnetic nanoparticles play a crucial role in their imaging performance in MRI. Therefore, optimizing the physical properties of the nanoprobes is essential to achieve high-resolution imaging of brain tumors.
The research “Application of π-Conjugated Materials in MRI-Guided Brain Tumor Diagnosis and Treatment” discusses the impact of π-conjugated materials as MRI contrast agents on brain tumor diagnosis and treatment (Smits, 2021). The study shows that due to the excellent optical and electronic properties of π-conjugated materials, they can generate exceptional contrast in MRI, allowing physicians to clearly visualize the size, shape, and location of brain tumors. Moreover, their characteristics enable them to maintain stability under high magnetic field strength, optimizing the accuracy of MRI scans (Brindle et al., 2017). These factors help doctors develop more precise treatment strategies, thus improving patient outcomes. Another research paper titled “Optimizing MRI Scans: Enhancing Brain Tumor Imaging Quality Using π-Conjugated Materials” aims to improve brain tumor imaging quality by optimizing MRI protocols and techniques and using specific types and quantities of π-conjugated materials (van der Voort et al., 2023). The researchers explore various methods and parameter adjustments, such as magnetic field strength, scanning time, and the type and amount of π-conjugated materials used, to determine which factors significantly enhance imaging quality (Ellingson et al., 2015). The results indicate that using specific types and quantities of π-conjugated materials during MRI scanning can substantially improve image clarity and resolution, allowing physicians to more accurately identify the tumor’s location and size (Kim et al., 2019). The article titled “The Importance of Using π-Conjugated Materials in MRI for Early Detection of Brain Tumors” discusses the value of π-conjugated materials in early brain tumor detection using MRI. This article extensively explains how the high contrast provided by π-conjugated materials in MRI imaging allows doctors to detect tumors even in their early stages (Bangalore Yogananda et al., 2020). The exceptional contrast enables physicians to observe subtle structural changes, providing higher sensitivity and specificity than traditional MRI techniques (Peng et al., 2022). Early detection allows doctors to begin treatment before the tumor progresses to more severe stages, significantly improving patient survival rates.
In summary, by using π-conjugated materials and optimizing MRI techniques and protocols, we can greatly enhance the effectiveness and accuracy of brain tumor imaging, providing physicians with more precise information to develop better treatment strategies. Through these advancements, medical imaging capabilities in diagnosing and treating brain tumors have significantly improved. However, further research is needed to fully understand all potential applications of π-conjugated materials in MRI optimization and determine their optimal use.
2.3.2 Fluorescence imaging optimization
In π-conjugated materials’ fluorescence imaging, optimizing the optical properties is essential. Adjusting the structure and functional groups of π-conjugated materials to tune the fluorescence emission peak to the near-infrared range can reduce interference from tissue autofluorescence, improving the imaging signal-to-noise ratio. Additionally, enhancing the fluorescence quantum yield and stability of the nanoprobes can enable long-term real-time monitoring.
The research titled “Optimization of Brain Tumor Imaging using Self-Assembled Nano Fluorescent Probes” utilizes self-assembled nano fluorescent probes for imaging brain tumors in mice (Wen et al., 2019). By optimizing the probes’ fluorescence characteristics and optical properties, the research team achieved highly sensitive brain tumor imaging. The results showed that the self-assembled nano fluorescent probes exhibited a comparative advantage in brain tumor imaging while minimally affecting surrounding normal tissues (Li et al., 2019a; Wen et al., 2019). Another research paper, “Optimization of Brain Tumor Imaging using Multi-Modal Fluorescent Probes,” employed a multi-modal fluorescent probe that combined different fluorescence imaging modes, such as fluorescence resonance energy transfer and fluorescence excitation spectroscopy, to enhance the accuracy and resolution of brain tumor imaging (Tomitaka et al., 2019). The results demonstrated that the multi-modal fluorescent probe offered high sensitivity and specificity in brain tumor imaging, effectively locating and identifying brain tumor tissue (Tomitaka et al., 2019). Additionally, a study titled “Enhancing the Application of Fluorescent Conjugated Materials in Brain Tumor Imaging” focused on optimizing the application of fluorescent conjugated materials in brain tumor imaging (Sheikh Mohamed et al., 2016). The research team designed and synthesized a series of fluorescent conjugated materials with different structures and properties, validating their performance in brain tumor imaging through animal experiments (Sheikh Mohamed et al., 2016). The results showed that the optimized fluorescent conjugated materials had higher fluorescence brightness, stability, and biocompatibility, enabling high-resolution and high-contrast brain tumor imaging (Sheikh Mohamed et al., 2016).
In conclusion, optimizing the application of π-conjugated materials in fluorescence imaging for brain tumors is a promising research field. These studies provide crucial support for early detection and accurate diagnosis of brain tumors, laying the foundation for subsequent research and clinical applications. With ongoing technological development and innovation, more breakthroughs are expected in enhancing the application of π-conjugated materials in brain tumor imaging.
2.3.3 Photoacoustic imaging optimization
In photoacoustic imaging, it is essential to optimize the photoacoustic conversion efficiency of π-conjugated nanoprobes and the sensitivity of acoustic signal detection (Wu et al., 2022). By carefully selecting the composition and morphology of π-conjugated materials, optimizing photoacoustic effects, and improving the efficiency of acoustic signal detection, researchers can achieve more accurate brain tumor imaging (Guo et al., 2023).
The study “Multi-Modal Photoacoustic and MRI Imaging Detection and Monitoring of Brain Tumors using π-Conjugated Polymer Nanoparticles” combines π-conjugated polymer nanoparticles with photoacoustic and MRI imaging techniques to enhance the sensitivity and resolution of brain tumor imaging (Stahl et al., 2017). The experimental results demonstrate that this multi-modal imaging approach allows high-resolution image reconstruction in deep tissues, facilitating early diagnosis and treatment monitoring. Additionally, the research “Transparency Imaging of Brain Tumors using Photoacoustic Microscopy and π-Conjugated Polymer Nanoparticles” combines photoacoustic microscopy with π-conjugated polymer nanoparticles to achieve transparent imaging of brain tumors (Guo et al., 2018). Through this method, researchers can observe fine internal structures and vascular distribution within brain tumors, furthering the understanding of tumor growth and metastasis mechanisms. This research provides essential groundwork for brain tumor microsurgery and treatment (Guo et al., 2018). Lastly, “Application of π-Conjugated Polymer Nanoprobes in Photoacoustic/MRI Multi-Modal Imaging for Brain Tumor Detection” explores the use of a novel π-conjugated polymer nanoscale probe for multi-modal photoacoustic/MRI imaging in brain tumor detection (Wang et al., 2021). The research team optimized the application of this multi-modal imaging method for tumor localization and edge identification, showing high accuracy, and effectively enhancing treatment planning precision and surgical success rates (Wang et al., 2021).
In summary, optimizing the application of π-conjugated materials through photoacoustic imaging is a cutting-edge research direction. By appropriately designing and improving the properties of π-conjugated materials and combining them with photoacoustic imaging techniques, researchers can enhance brain tumor imaging’s sensitivity, resolution, and accuracy, providing crucial support for early detection and precise treatment of brain tumors. Future research can further explore the combined application of photoacoustic imaging techniques with other medical imaging modalities, offering more possibilities for comprehensive evaluation and accurate treatment of brain tumors.
2.3.4 Multimodal imaging technology integration
The future development will focus on the integration of multimodal imaging technology. The application of π-conjugated materials in various imaging modes provides a strong foundation for achieving multimodal imaging (Lewis et al., 2021). By organically combining different imaging modes, a more comprehensive and accurate depiction of brain tumors can be achieved, enhancing the reliability of diagnosis. The fusion of multimodal imaging technology also allows for complementary information acquisition about brain tumors, aiding in a deeper understanding of their biological characteristics and treatment responses (Cheng et al., 2020).
In conclusion, the application of π-conjugated materials in brain tumor imaging faces numerous opportunities for optimization and development. By appropriately designing the structure and properties of nanoprobes, optimizing the performance of imaging techniques, and achieving the integration of multimodal imaging technology, the application of π-conjugated materials in brain tumor imaging can be enhanced, providing new opportunities and possibilities for early detection and treatment of brain tumors. Future research efforts will promote the extensive application of π-conjugated materials in clinical brain tumor diagnosis and treatment, leading to better clinical outcomes and quality of life for patients (Chen et al., 2014; Neumann et al., 2020).
Wang et al. (2016) reviews the recent progress in the application of multimodal imaging technology using π-conjugated materials for brain tumor imaging. The research finds that by integrating MRI, PET, and optical imaging technologies, the sensitivity and specificity of brain tumor imaging can be improved, providing more accurate information for clinical diagnosis and treatment. Lin et al. (2022) used MRI, PET, and optical imaging technologies to inject π-conjugated materials into a mouse brain tumor model, obtaining accurate brain tumor images through the fusion of multimodal imaging technology. The results indicate that π-conjugated materials have potential application value in brain tumor imaging. Liu et al. (2019) used MRI, PET, and ultrasound imaging technologies to introduce π-conjugated materials into a mouse brain tumor model, obtaining high-resolution brain tumor images through the fusion of multimodal imaging technology. The results show that multimodal imaging technology fusion can improve the accuracy and visualization of brain tumor imaging. A study reviews the prospects of the application of π-conjugated materials in brain tumor imaging and explores the development trends of multimodal imaging technology in this field. The research finds that by integrating MRI, PET, and optical imaging technologies, the accuracy and visualization of brain tumor imaging can be improved, offering new ideas for individualized treatment of brain tumors (Calhoun and Sui, 2016).
In summary, the improvement of the application of multimodal imaging technology in brain tumor imaging is a highly researched area. By integrating MRI, PET, optical imaging, and ultrasound imaging technologies, more accurate and comprehensive brain tumor images can be obtained, providing more information for clinical diagnosis and treatment (Yoon et al., 2014). Future research should further explore the application of multimodal imaging technology using π-conjugated materials to enhance early detection and personalized treatment of brain tumors.
3 Application of π-conjugated materials in brain tumor therapy
3.1 Advances in nanomedicine delivery systems
Nanomedicine delivery systems involve the nanoscale formulation of drug carriers to achieve precise drug delivery and release, making it a significant research direction in brain tumor therapy (Chen et al., 2022; Narmani et al., 2023). In the context of π-conjugated materials, they serve as excellent nanocarriers, offering new ideas and means for the research of nanomedicine delivery systems.
The advantages of π-conjugated materials lie in their large surface area, tunable optical properties, and good biocompatibility (Li et al., 2022b). These characteristics make them effective drug carriers that can achieve targeted drug delivery through surface modification. By encapsulating drugs within π-conjugated material nanoparticles, the biological distribution of drugs can be improved, effectively reducing toxicity and side effects in normal tissues (Haque et al., 2020). Furthermore, nanomedicine delivery systems can achieve high selective delivery to brain tumor tissues through passive or active targeting strategies, enhancing the local therapeutic effect of drugs (Zhu et al., 2018; Hiroto and Wu, 2019; Haque et al., 2023).
In nanomedicine delivery systems, π-conjugated materials can also enable light-triggered drug release through their optical properties (Xu et al., 2015; Tang et al., 2021). By utilizing the absorption characteristics of π-conjugated materials, drug-loaded nanoparticles can be selectively stimulated to release drugs in specific tumor areas upon exposure to light. This light-triggered drug release approach can significantly reduce nonspecific drug release in the body, enhancing drug targeting and therapeutic efficacy (Qiao et al., 2022).
However, the application of nanomedicine delivery systems also faces some challenges. For instance, the blood-brain barrier restricts drug delivery to brain tissues (Patra et al., 2018). Thus, further optimization and improvement of π-conjugated materials as nanocarriers are needed to overcome this issue. Additionally, comprehensive studies on the biocompatibility and toxicity assessment of π-conjugated materials are necessary to ensure their safety in clinical applications (Haque et al., 2023).
3.2 Photothermal therapy and photodynamic therapy
Photothermal therapy and photodynamic therapy are therapeutic approaches that utilize optical properties, enabled by the photothermal conversion performance of π-conjugated materials, for brain tumor treatment. In both therapies, the local photothermal effect of π-conjugated materials plays a central role in the treatment mechanism (Chen et al., 2020).
Photothermal therapy involves exciting the optical properties of π-conjugated materials to convert light energy into heat, generating high temperatures in localized areas with nanoparticles (Guo et al., 2018). This local hyperthermia can lead to the coagulative necrosis of brain tumor cells, achieving tumor ablation. Photothermal therapy offers advantages such as non-invasiveness, excellent local efficacy, and repeatability (Figure 6). By selecting appropriate light parameters and characteristics of π-conjugated materials, efficient brain tumor treatment can be achieved (Xu et al., 2015).
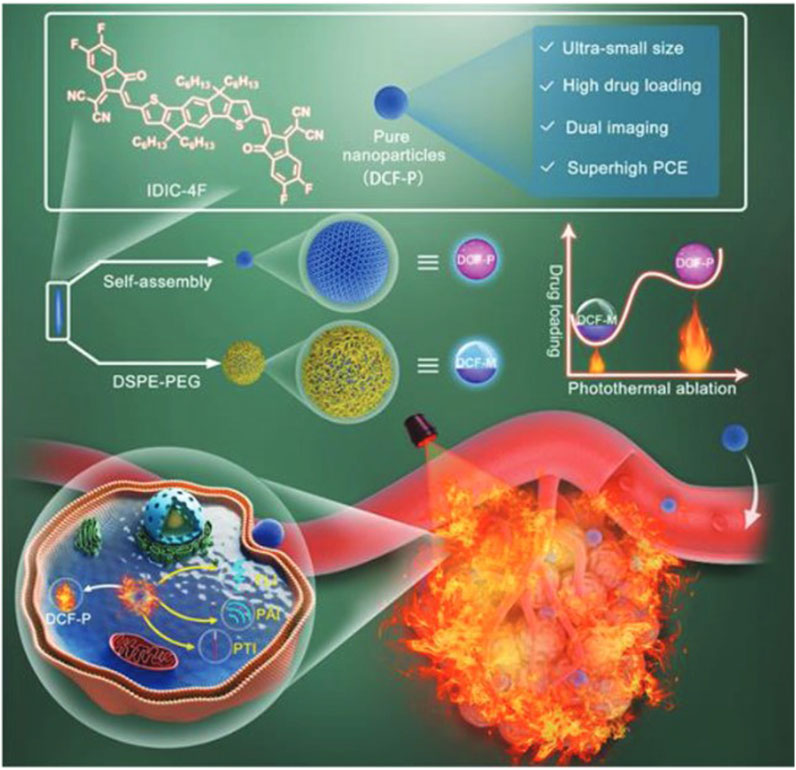
FIGURE 6. Schematic illustration for carrier-free and carrier-assistant PTT (Zheng et al., 2022).
Researchers have synthesized a novel π-conjugated polymer nanoparticle with excellent optical properties, which was used in photothermal therapy for glioma. Experimental results showed that these nanoparticles could generate high temperatures under near-infrared light irradiation, leading to the destruction of brain tumor tissue. This study provides a new method for photothermal therapy of brain tumors. Additionally, π-conjugated carbon dots as optical absorption materials for photothermal therapy and applied them to brain tumor treatment. The experimental results demonstrated that these carbon dots could generate high temperatures under near-infrared light irradiation and accurately locate brain tumor tissue. This research offers a new strategy for photothermal therapy of brain tumors (He et al., 2023). Moreover, a near-infrared phosphorescent π-conjugated metal-organic framework and utilized it in photothermal therapy for brain tumors. The results indicated that this metal-organic framework could generate high temperatures under near-infrared light irradiation and effectively kill brain tumor cells. This study provides a new option for photothermal therapy of brain tumors (Jouaiti et al., 2023). Additionally, a photothermal π-conjugated nanomaterial with excellent optical properties and investigated its application in brain tumor treatment. Experimental results showed that this nanomaterial could generate high heat under light irradiation and effectively kill brain tumor cells. This research offers new perspectives for photothermal therapy of brain tumors (Li et al., 2019b).
In summary, the application of π-conjugated materials in photothermal therapy for brain tumors holds tremendous potential. These studies provide new methods and strategies for photothermal therapy of brain tumors, with the potential to further improve cancer treatment outcomes. Future research can further explore the potential of these π-conjugated materials in clinical applications and accelerate their translation into clinical use (Zheng et al., 2022).
Photodynamic therapy involves using π-conjugated materials as photosensitizers, exciting their photosensitive properties to generate harmful substances like reactive oxygen or reactive nitrogen species, achieving destruction of brain tumor cells (Lin et al., 2022). Photodynamic therapy offers high selectivity and local efficacy, minimizing damage to surrounding normal brain tissues (Figure 7). Additionally, through targeted modifications, photodynamic therapy can achieve high selective destruction of brain tumors, enhancing treatment precision (Luo et al., 2023).
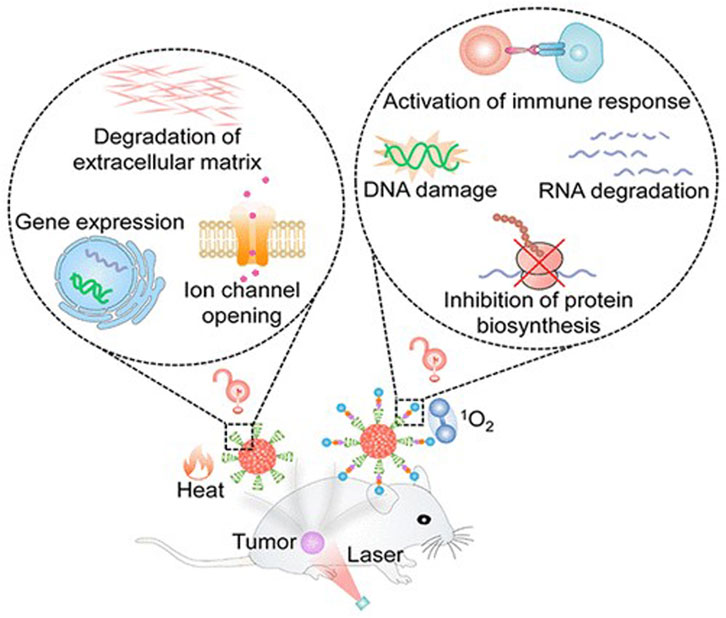
FIGURE 7. π-conjugated nanomaterials as near-infrared photoactivatable pro-therapeutics for cancer (Cevik et al., 2019).
Researchers proposed a novel π-conjugated polymer nanoparticle guided by nucleic acid conjugates for loading fluorophores. This nanoparticle exhibits infrared emission absorption capacity and can accurately release the fluorophore inside the cell, achieving effective treatment of gliomas that are challenging to treat directly (Cevik et al., 2019). This nanomaterial demonstrates exceptionally high biocompatibility and can be targeted to tumor cells. The application of π-conjugated materials in photodynamic therapy for brain tumors has broad and significant implications. This research is expected to provide a new and more effective treatment strategy for brain cancer (Tia et al., 2010).
However, the application of photothermal therapy and photodynamic therapy also faces some challenges (Cao et al., 2022). For instance, the depth of light penetration limits the therapeutic efficacy for deep-seated brain tumors. Therefore, further optimization of light parameters and the selection of suitable light sources to enhance light penetration depth are necessary. Additionally, research on the metabolic pathways and biodistribution of π-conjugated materials in the body, along with the assessment of the biocompatibility and safety of photothermal therapy and photodynamic therapy, are crucial (Li and Pu, 2020).
3.3 Application of π-conjugated materials in combination therapy
Combination therapy is an approach that integrates different treatment strategies to enhance treatment effectiveness. In brain tumor therapy, π-conjugated materials, as multifunctional carriers, offer new possibilities for combination therapy (Jiang et al., 2019).
Firstly, π-conjugated materials can be used as nanomedicine carriers to achieve combined drug delivery (Han et al., 2021). By modifying different anti-tumor drugs on the surface of π-conjugated materials, they can be simultaneously delivered to brain tumor tissues, enabling synergistic combination therapy. This approach overcomes the limitations of single-drug treatment and enhances therapeutic efficacy and anti-tumor effects. Additionally, combination therapy can reduce the occurrence of drug resistance and prolong the duration of treatment effectiveness. Research teams have prepared a conjugated material capable of carrying both chemotherapeutic drugs and photosensitizers, introducing them into brain tumor cells (Chowdhury et al., 2022). In vitro experiments showed that the conjugated material effectively released drugs into tumor cells and released photosensitizers under light conditions, further destroying tumor cells. Furthermore, experimental results indicated that the conjugated material, after surface modification, can achieve active targeting of tumor cells, enhancing treatment effectiveness. Secondly, the application of π-conjugated materials in photothermal therapy and photodynamic therapy also provides new options for combination therapy. By modifying photosensitizers on the surface of π-conjugated materials, photosensitized induction therapy for brain tumors can be achieved (Lin et al., 2022). Simultaneously, photothermal therapy or photodynamic therapy can be combined with chemotherapy to achieve multi-dimensional attacks on brain tumors. This combination therapy strategy can improve treatment efficacy and minimize patient damage during the treatment process (Guo et al., 2018). Additionally, π-conjugated materials can be used in combination with radiation therapy. By modifying π-conjugated materials in radiation therapy organs, the radiation dose can be enhanced (Li et al., 2020). The optical properties of π-conjugated materials allow them to absorb and scatter radiation, thereby increasing the radiation dose to the tumor. This combination therapy strategy can improve the effectiveness of radiation therapy and reduce radiation damage to surrounding normal tissues (Grimland et al., 2011; Xie et al., 2019).
However, the application of π-conjugated materials in combination therapy also faces some challenges. Firstly, in-depth research on the interaction between different treatment strategies is required (Chowdhury et al., 2022). There may be interactions between different treatment strategies that require careful adjustment of treatment parameters to achieve the best combination therapy effect. Secondly, the safety and interactions of drugs used in combination therapy must be thoroughly considered to ensure the safety and reliability of the treatment process (Du et al., 2016).
In conclusion, the application of π-conjugated materials in combination therapy for brain tumors provides new options and possibilities for brain tumor treatment. By fully utilizing the multifunctional advantages of π-conjugated materials, nanomedicine delivery, photothermal therapy, photodynamic therapy, and radiation therapy can be combined to improve treatment effectiveness, leading to better treatment outcomes and quality of life for brain tumor patients (Zhao et al., 2020). In the future, further research on the interactions and biocompatibility of π-conjugated materials in combination therapy is needed, continuously optimizing treatment strategies to achieve personalized and precise brain tumor treatment.
4 Biocompatibility and toxicity assessment of π-conjugated materials in brain tumor therapy
The potential application of π-conjugated materials in brain tumor therapy is promising, but their biocompatibility and toxicity assessment are critical research directions (Xu et al., 2015; Wang et al., 2020). In this section, we will focus on the biocompatibility and toxicity assessment of π-conjugated materials in brain tumor therapy, covering in vivo metabolism and distribution, biocompatibility assessment, and considerations for potential toxicity and safety.
4.1 In Vivo metabolism and distribution
Understanding the in vivo metabolism and distribution of π-conjugated materials is crucial for evaluating their biocompatibility (Bai et al., 2020). The in vivo metabolism of π-conjugated materials is typically determined by their physical and chemical properties. Nanoscale π-conjugated materials may be cleared through phagocytic cells in the liver and spleen. Additionally, the unique structure of brain vessels and the blood-brain barrier may affect the distribution of π-conjugated materials in brain tissue (Liu et al., 2020).
Research has shown that factors such as the size, shape, surface modification, and encapsulating materials of π-conjugated materials significantly influence their in vivo metabolism and distribution. By rationally designing and improving the physical properties of nanoprobes, optimization of in vivo metabolism and distribution can be achieved, enhancing the stability and circulation time of nanoprobes (Yoshizawa, 2012; Xu et al., 2020).
4.2 Biocompatibility assessment
Comprehensive assessment of the biocompatibility of π-conjugated materials is essential for their application in brain tumor therapy. Biocompatibility assessment aims to understand the interactions and influences between nanoprobes and organisms, predicting their in vivo behavior (Zhou et al., 2020). Common biocompatibility assessments include cytotoxicity experiments, in vitro blood stability, in vivo tissue stimulation, and immunogenicity testing (Xu et al., 2015).
Through in vitro and in vivo experiments, researchers can assess the toxicity and damage of π-conjugated materials to cells, further understanding their tolerance to organisms. Additionally, evaluations of blood stability can reveal the behavior of nanoprobes in the blood, such as platelet and plasma protein adsorption. These assessments help predict the in vivo circulation stability and biodegradability of nanoprobes (Wang et al., 2020).
4.3 Considerations for potential toxicity and safety
In the application of π-conjugated materials, potential toxicity and safety issues must be fully considered. Although π-conjugated materials show many potential advantages in brain tumor therapy, their toxicity may lead to adverse effects on normal tissues. Comprehensive evaluation of the potential toxicity and safety of π-conjugated materials requires considering various factors such as dose-dependent toxicity, potential long-term cumulative effects, biocompatibility, and biodegradability (Xie et al., 2019). Additionally, when combining nanoprobes with other treatment modalities, the comprehensive impact on treatment efficacy and toxicity needs to be evaluated (Xu et al., 2015). To ensure the safety and reliability of π-conjugated materials in brain tumor therapy, researchers need to thoroughly understand their biological behavior and interaction mechanisms (Xiang et al., 2020). Furthermore, strict adherence to relevant biosafety evaluation standards and regulations is essential to ensure the safety of nanoprobes in clinical applications (Coelho et al., 2017).
In conclusion, the biocompatibility and toxicity assessment of π-conjugated materials in brain tumor therapy are necessary steps for their clinical application. By thoroughly understanding the in vivo metabolism and distribution of nanoprobes, conducting comprehensive biocompatibility evaluations, and considering potential toxicity and safety issues, important references and guarantees can be provided for the rational application of π-conjugated materials in brain tumor therapy. Additionally, data from preclinical experiments and clinical trials will further verify their safety and effectiveness (de Deus et al., 2021).
5 Future development and prospects of π-conjugated materials
5.1 Development trends and prospective applications
Significant progress has been made in the research of π-conjugated materials in brain tumor therapy, but their future development trends and prospects are still full of challenges and potential (Zhou and Zhan, 2018). With the continuous advancement of nanotechnology and a deeper understanding of the properties of π-conjugated materials, it is expected that more novel nanoprobes will emerge, leading to breakthroughs in brain tumor therapy (Richard et al., 2020).
1. Development of Multifunctional Nanoprobes: One of the future development trends of π-conjugated materials is the construction of more functional nanoprobes. For example, integrating diagnostic and therapeutic functions into one nanoprobe to achieve multimodal imaging and combination therapy, thereby enhancing the accuracy and effectiveness of brain tumor therapy. Multifunctional nanoprobes can also realize multiple-targeted therapy for brain tumors, becoming a crucial strategy for personalized treatment (Yin et al., 2017).
2. Optimization of Targeting Strategies: Future research will focus on optimizing targeting strategies to address the unique microenvironment of brain tumors, such as the blood-brain barrier and high surface expression of tumor cells. By designing appropriate targeting ligands, high-selectivity recognition and treatment of brain tumor tissues can be achieved. Optimizing targeting strategies will help improve the therapeutic efficacy and biocompatibility of nanoprobes (Bu et al., 2022).
3. Achieving Personalized Treatment: Future development will pay more attention to personalized brain tumor therapy. By combining patients’ genomic information and pathological characteristics, customized π-conjugated nanoprobes can be designed for individualized brain tumor treatment. Personalized treatment will maximize treatment effectiveness and reduce patient side effects (Torres et al., 2020; Li X. et al., 2022).
4. Novel Strategies for Combination Therapy: As multifunctional carriers, π-conjugated materials are expected to become a new strategy for combination therapy. Future research will explore the combination of π-conjugated materials with photothermal therapy, immunotherapy, chemotherapy, and other treatment modalities to achieve synergistic enhancement of treatment effects. This comprehensive approach is likely to become the future direction of brain tumor therapy (Chowdhury et al., 2022).
5.2 Challenges and solutions in clinical applications
The clinical application of π-conjugated materials in brain tumor therapy faces challenges that warrant closer examination and innovative solutions (Tian et al., 2022).
1. Conversion Efficiency and Stability: In the application of nanoprobes, conversion efficiency and stability are of paramount importance. While we acknowledge the significance of these aspects, it is essential to delve deeper into the associated challenges. Future research should concentrate on enhancing the synthesis methods of π-conjugated materials, optimizing their optical and photothermal properties to boost conversion efficiency and stability. Additionally, a rational design of surface modification of nanoprobes to increase their circulation time is crucial for improving their in vivo stability, and this area requires further exploration (Rakstys et al., 2019; Deng et al., 2021).
2. Biocompatibility and Toxicity Assessment: Comprehensive assessment of the biocompatibility and toxicity of π-conjugated materials is pivotal before contemplating clinical applications. This issue merits further attention. Future research should intensify the study of the biocompatibility and toxicity of nanoprobes to ensure their safety and reliability in brain tumor therapy. Furthermore, rigorous adherence to pertinent biosafety evaluation standards and regulations, alongside thorough preclinical evaluations of nanoprobes, is indispensable for mitigating potential risks (Narayanan et al., 2020; Wang et al., 2020).
3. Overcoming the Blood-Brain Barrier: The blood-brain barrier presents a critical challenge in drug delivery to brain tissue. The overcoming of this obstacle requires deeper investigation. Future research should delve into the utilization of nanotechnology and targeting strategies to surmount the blood-brain barrier, enabling efficient delivery of nanoprobes to brain tumor tissues (Fu et al., 2019; Li et al., 2021b).
4. Clinical Validation and Regulation: Achieving the clinical application of π-conjugated materials in brain tumor therapy demands more comprehensive attention to the challenges associated with large-scale clinical studies and regulatory approvals. Future research should emphasize the feasibility and effectiveness of clinical validation, ensuring that the application of π-conjugated materials meets stringent safety and efficacy requirements (Xu et al., 2020; Wu et al., 2021).
In conclusion, π-conjugated materials have vast future development and application prospects in brain tumor therapy. Through the development of multifunctional nanoprobes, optimization of targeting strategies, realization of personalized treatment, exploration of novel strategies for combination therapy, and addressing challenges in clinical applications, π-conjugated materials are expected to bring revolutionary advancements to brain tumor therapy.
In the future, with the continuous advancement of nanotechnology, the synthesis methods of π-conjugated materials will become more refined and controllable. Novel π-conjugated nanoprobes may possess superior optical and photothermal properties, enabling precise imaging and efficient treatment of brain tumors (Barman et al., 2020). The development of multifunctional nanoprobes will make brain tumor therapy more comprehensive and personalized, improving treatment efficacy and reducing patient discomfort and side effects (Takahashi, 2021).
Optimization of targeting strategies is the key to achieving precise treatment of brain tumors. Future research will further study the unique microenvironment of brain tumors, design appropriate targeting ligands, and achieve high-selectivity recognition and treatment of brain tumor tissues (Shiraki et al., 2020). Additionally, the combined application of nanotechnology and targeting strategies will help overcome the limitations of the blood-brain barrier and achieve efficient delivery of nanoprobes to brain tumor tissues.
With the deepening of preclinical experiments and clinical validation, the safety and efficacy of π-conjugated materials in brain tumor therapy will be comprehensively evaluated (Guo et al., 2016). Researchers will strengthen the study of the biocompatibility and toxicity of nanoprobes to ensure their safety and reliability in brain tumor therapy. Moreover, adhering to relevant biosafety evaluation standards and regulations and conducting rigorous preclinical evaluations of nanoprobes will help reduce potential risks (Lai et al., 2021).
However, in the clinical application of π-conjugated materials in brain tumor therapy, there are still some challenges. The design and execution of clinical trials are complex and time-consuming processes, requiring careful consideration of factors such as sample size, treatment dosage, and patient selection (Hiroto and Wu, 2019). Additionally, nanotechnology may face cost and industrialization issues in large-scale production and application. To achieve the clinical application of π-conjugated materials in brain tumor therapy, further strengthening multidisciplinary cooperation and promoting the close integration of basic research and clinical practice are needed (Haque et al., 2020).
Overall, π-conjugated materials have vast potential for application in brain tumor imaging and therapy (Oubaha et al., 2019). Through continuous research and innovation, nanotechnology is expected to bring revolutionary changes to brain tumor therapy. In the future, we can expect π-conjugated materials to play a greater role in brain tumor therapy, providing more effective and personalized treatment options for patients.
6 Conclusion
6.1 Contributions of π-conjugated materials in brain tumor imaging and treatment
π-Conjugated materials have demonstrated substantial potential and made noteworthy contributions to brain tumor imaging and treatment. These multifunctional nanoprobes offer several advantages in brain tumor imaging. Through meticulous design and modifications, nanoprobes can achieve highly selective brain tumor imaging, providing high-resolution and high-contrast images. The realization of multimodal imaging facilitates the acquisition of multiple types of imaging data on a single platform, delivering comprehensive brain tumor information to clinical practitioners, thereby assisting in more precise diagnosis and treatment decisions.
In terms of brain tumor treatment, π-conjugated materials, when employed as drug carriers, offer unique advantages. Nanoscale π-conjugated materials can selectively target brain tumors using targeting strategies, thereby enhancing the precise delivery of therapeutic drugs and reducing toxicity to normal tissues. Additionally, the combined use of optical techniques, such as photothermal therapy and photodynamic therapy, enables the precise treatment of brain tumors, consequently improving treatment efficacy.
6.2 Future development prospects
The future development prospects of π-conjugated materials in brain tumor imaging and treatment are highly promising. As nanotechnology and biomedical fields continue to advance, we can anticipate the following developments:
1. Design and Synthesis of Novel Nanoprobes: Future research endeavors will persist in exploring novel methods for designing and synthesizing π-conjugated materials. By enhancing the material’s structure and physicochemical properties, nanoprobes can achieve multifunctionality and heightened efficiency, providing an enhanced platform for brain tumor imaging and treatment.
2. Optimization of Biocompatibility and Safety: Future research will focus on optimizing the biocompatibility and safety evaluation of π-conjugated materials. Enhancing the biocompatibility of nanoprobes and diminishing their toxicity to normal tissues will augment their reliability and safety in clinical applications.
3. Implementation of Personalized Treatment: The future will see the design of personalized π-conjugated nanoprobes that consider patients’ genomic information and pathological characteristics for individualized brain tumor treatment. Personalized treatment will better cater to patients’ specific needs, thereby improving treatment efficacy.
4. Application of Multimodal Imaging and Combination Therapy:*Subsequent research will delve into new strategies for multimodal imaging and combination therapy. By integrating multiple imaging and therapeutic functions into a single nanoprobe, comprehensive brain tumor monitoring and treatment can be achieved, augmenting the efficacy of brain tumor therapy.
5. Promotion of Clinical Application: Following advancements in the application of π-conjugated materials in brain tumor therapy, their clinical utilization will gradually expand. Large-scale clinical studies and monitoring will further validate the safety and efficacy of π-conjugated materials, promoting their clinical application.
In summation, π-conjugated materials exhibit extensive potential and offer promising application prospects in brain tumor imaging and treatment. Future research will concentrate on optimizing nanoprobe design and synthesis, improving biocompatibility and safety, implementing personalized treatment and multimodal combination therapy, and advancing the clinical application of π-conjugated materials. With the continuous advancement of science and technology, π-conjugated materials are poised to provide more precise and effective treatment approaches for brain tumors, ultimately enhancing treatment efficacy and the quality of life for individuals affected by brain tumors.
Author contributions
WS: Resources, Writing–original draft. CW: Conceptualization, Writing–review and editing. CT: Investigation, Methodology, Writing–review and editing. XL: Investigation, Writing–review and editing. XH: Conceptualization, Methodology, Supervision, Writing–review and editing. SL: Conceptualization, Supervision, Writing–review and editing.
Funding
The author(s) declare financial support was received for the research, authorship, and/or publication of this article. This work was funded by the following projects: Shandong Provincial Natural Science Foundation General Project Title: Mechanisms of ALDH9A1 in Hepatocellular Carcinoma and its Potential Correlation with Molecular Imaging Project Number: ZR2021MH052 National Key Research and Development Program Project Title: Research and Promotion of a Precise Treatment System and Key Technologies for Iodine-125 Particle Therapy in Glioblastoma Project Number: 2019YFE0120100 Clinical Real-World Study on Iodine-125 Particle Combined with Bevacizumab Monoclonal Antibody for the Treatment of High-Grade Glioma Project Number: BJHA-CRP-094 Funded by: Qijiang District Life Oasis Public Service Center, Quzhou City.
Conflict of interest
The authors declare that the research was conducted in the absence of any commercial or financial relationships that could be construed as a potential conflict of interest.
Publisher’s note
All claims expressed in this article are solely those of the authors and do not necessarily represent those of their affiliated organizations, or those of the publisher, the editors and the reviewers. Any product that may be evaluated in this article, or claim that may be made by its manufacturer, is not guaranteed or endorsed by the publisher.
References
Bai, Q., Zhang, C., Li, L., Zhu, Z., Wang, L., Jiang, F., et al. (2020). Subsequent monitoring of ferric ion and ascorbic acid using graphdiyne quantum dots-based optical sensors. Mikrochim. Acta 187 (12), 657. doi:10.1007/s00604-020-04624-w
Bangalore Yogananda, C. G., Shah, B. R., Vejdani-Jahromi, M., Nalawade, S. S., Murugesan, G. K., Yu, F. F., et al. (2020). Retracted: a novel fully automated MRI-based deep-learning method for classification of IDH mutation status in brain gliomas. Neuro Oncol. 22 (3), 402–411. doi:10.1093/neuonc/noz199
Barman, D., Gogoi, R., Narang, K., and Iyer, P. K. (2020). Recent developments on multi-functional metal-free mechanochromic luminescence and thermally activated delayed fluorescence organic materials. Front. Chem. 8, 483. doi:10.3389/fchem.2020.00483
Brindle, K. M., Izquierdo-Garcia, J. L., Lewis, D. Y., Mair, R. J., and Wright, A. J. (2017). Brain tumor imaging. J. Clin. Oncol. 35 (21), 2432–2438. doi:10.1200/jco.2017.72.7636
Bu, A., Zhao, Y., Xiao, H., Tung, C., Wu, L., and Cong, H. (2022). A conjugated covalent template strategy for all-benzene catenane synthesis. Angew. Chem. Int. Ed. Engl. 61 (39), e202209449. doi:10.1002/anie.202209449
Calhoun, V. D., and Sui, J. (2016). Multimodal fusion of brain imaging data: a key to finding the missing link(s) in complex mental illness. Biol. Psychiatry Cogn. Neurosci. Neuroimaging 1 (3), 230–244. doi:10.1016/j.bpsc.2015.12.005
Cao, W., Zhu, Y., Wu, F., Tian, Y., Chen, Z., Xu, W., et al. (2022). Three birds with one stone: acceptor engineering of hemicyanine dye with NIR-II emission for synergistic photodynamic and photothermal anticancer therapy. Small 18 (49), e2204851. doi:10.1002/smll.202204851
Cevik, E., Buyukharman, M., and Yildiz, H. B. (2019). Construction of efficient bioelectrochemical devices: improved electricity production from cyanobacteria (Leptolyngbia sp.) based on pi-conjugated conducting polymer/gold nanoparticle composite interfaces. Biotechnol. Bioeng. 116 (4), 757–768. doi:10.1002/bit.26885
Chatterjee, D. P., Pakhira, M., and Nandi, A. K. (2020). Fluorescence in "nonfluorescent" polymers. ACS Omega 5 (48), 30747–30766. doi:10.1021/acsomega.0c04700
Chen, J., Wen, K., Chen, H., Jiang, S., Wu, X., Lv, L., et al. (2020). Achieving high-performance photothermal and photodynamic effects upon combining D-A structure and nonplanar conformation. Small 16 (17), e2000909. doi:10.1002/smll.202000909
Chen, X., Hussain, S., Abbas, A., Hao, Y., Malik, A. H., Tian, X., et al. (2022). Conjugated polymer nanoparticles and their nanohybrids as smart photoluminescent and photoresponsive material for biosensing, imaging, and theranostics. Mikrochim. Acta 189 (3), 83. doi:10.1007/s00604-021-05153-w
Chen, X., Park, R., Hou, Y., Khankaldyyan, V., Gonzales-Gomez, I., Tohme, M., et al. (2004). MicroPET imaging of brain tumor angiogenesis with 18F-labeled PEGylated RGD peptide. Eur. J. Nucl. Med. Mol. Imaging 31 (8), 1081–1089. doi:10.1007/s00259-003-1452-2
Chen, Z. Y., Wang, Y. X., Lin, Y., Zhang, J. S., Yang, F., Zhou, Q. L., et al. (2014). Advance of molecular imaging technology and targeted imaging agent in imaging and therapy. Biomed. Res. Int. 2014, 1–12. doi:10.1155/2014/819324
Cheng, L., Wang, X., Gong, F., Liu, T., and Liu, Z. (2020). 2D nanomaterials for cancer theranostic applications. Adv. Mater 32 (13), e1902333. doi:10.1002/adma.201902333
Chowdhury, P., Banerjee, A., Saha, B., Bauri, K., and De, P. (2022). Stimuli-responsive aggregation-induced emission (AIE)-Active polymers for biomedical applications. ACS Biomater. Sci. Eng. 8 (10), 4207–4229. doi:10.1021/acsbiomaterials.2c00656
Coelho, J. P., Mayoral, M. J., Camacho, L., Martín-Romero, M. T., Tardajos, G., López-Montero, I., et al. (2017). Mechanosensitive gold colloidal membranes mediated by supramolecular interfacial self-assembly. J. Am. Chem. Soc. 139 (3), 1120–1128. doi:10.1021/jacs.6b09485
de Deus, W. F., de Franca, B. M., Forero, J. S. B., Granato, A. E. C., Ulrich, H., Dória, A. C. O. C., et al. (2021). Curcuminoid-tailored interfacial free energy of hydrophobic fibers for enhanced biological properties. ACS Appl. Mater Interfaces 13 (21), 24493–24504. doi:10.1021/acsami.1c05034
Deng, Y., Wang, Y., Xiao, X., Saucedo, B. J., Zhu, Z., Xie, M., et al. (2022). Progress in hybridization of covalent organic frameworks and metal-organic frameworks. Small 18 (38), e2202928. doi:10.1002/smll.202202928
Deng, Z., Cui, S., Kou, K., Liang, D., Shi, X., and Liu, J. (2021). Dopant-free pi-conjugated hole transport materials for highly stable and efficient perovskite solar cells. Front. Chem. 9, 664504. doi:10.3389/fchem.2021.664504
Doan, V. H. M., Mondal, S., Vo, T. M. T., Ly, C. D., Vu, D. D., Nguyen, V. T., et al. (2022). Fluorescence conjugated nanostructured cobalt-doped hydroxyapatite platform for imaging-guided drug delivery application. Colloids Surf. B Biointerfaces 214, 112458. doi:10.1016/j.colsurfb.2022.112458
Du, D., Wang, K., Wen, Y., Li, Y., and Li, Y. Y. (2016). Photodynamic graphene quantum dot: reduction condition regulated photoactivity and size dependent efficacy. ACS Appl. Mater Interfaces 8 (5), 3287–3294. doi:10.1021/acsami.5b11154
Ellingson, B. M., Bendszus, M., Boxerman, J., Barboriak, D., Erickson, B. J., Smits, M., et al. (2015). Consensus recommendations for a standardized brain tumor imaging protocol in clinical trials. Neuro Oncol. 17 (9), 1188–1198. doi:10.1093/neuonc/nov095
Fu, W., Yan, C., Guo, Z., Zhang, J., Zhang, H., Tian, H., et al. (2019). Rational design of near-infrared aggregation-induced-emission-active probes: in situ mapping of amyloid-beta plaques with ultrasensitivity and high-fidelity. J. Am. Chem. Soc. 141 (7), 3171–3177. doi:10.1021/jacs.8b12820
Ge, J., Koutarapu, S., Jha, D., Dulewicz, M., Zetterberg, H., Blennow, K., et al. (2023). Tetramodal chemical imaging delineates the lipid-amyloid peptide interplay at single plaques in transgenic alzheimer's disease models. Anal. Chem. 95 (10), 4692–4702. doi:10.1021/acs.analchem.2c05302
Grimland, J. L., Wu, C., Ramoutar, R. R., Brumaghim, J. L., and McNeill, J. (2011). Photosensitizer-doped conjugated polymer nanoparticles with high cross-sections for one- and two-photon excitation. Nanoscale 3 (4), 1451–1455. doi:10.1039/c0nr00834f
Gu, C., Huang, N., Chen, Y., Qin, L., Xu, H., Zhang, S., et al. (2015). π-Conjugated microporous polymer films: designed synthesis, conducting properties, and photoenergy conversions. Angew. Chem. Int. Ed. Engl. 54 (46), 13594–13598. doi:10.1002/anie.201506570
Guo, L., Ge, J., and Wang, P. (2018). Polymer dots as effective phototheranostic agents. Photochem Photobiol. 94 (5), 916–934. doi:10.1111/php.12956
Guo, L., Li, K. F., Zhang, X., Cheah, K. W., and Wong, M. S. (2016). Highly efficient multiphoton-pumped frequency-upconversion stimulated blue emission with ultralow threshold from highly extended ladder-type oligo(p-phenylene)s. Angew. Chem. Int. Ed. Engl. 55 (36), 10797–10802. doi:10.1002/ange.201604064
Guo, Y., Li, B., and Yin, X. (2023). Dual-compressed photoacoustic single-pixel imaging. Natl. Sci. Rev. 10 (1), nwac058. doi:10.1093/nsr/nwac058
Han, W., Xiang, W., Li, Q., Zhang, H., Yang, Y., Shi, J., et al. (2021). Water compatible supramolecular polymers: recent progress. Chem. Soc. Rev. 50 (18), 10025–10043. doi:10.1039/d1cs00187f
Haque, A., Al-Balushi, R. A., Raithby, P. R., and Khan, M. S. (2020). Recent advances in π-conjugated N^C-chelate organoboron materials. Molecules 25 (11), 2645. doi:10.3390/molecules25112645
Haque, A., Alenezi, K. M., Khan, M. S., Wong, W. Y., and Raithby, P. R. (2023). Non-covalent interactions (NCIs) in pi-conjugated functional materials: advances and perspectives. Chem. Soc. Rev. 52 (2), 454–472. doi:10.1039/d2cs00262k
He, F., Yuan, H., Hu, Y., Huang, J., Wang, Z., Peng, S., et al. (2023). Construction of pi-conjugated crystalline carbon dots with carbon nitride nanofragments for efficient photocatalytic H(2) evolution. Chem. Commun. (Camb) 59 (66), 10016–10019. doi:10.1039/d3cc02859c
Hicks, G. E. J., Li, S., Obhi, N. K., Jarrett-Wilkins, C. N., and Seferos, D. S. (2021). Programmable assembly of pi-conjugated polymers. Adv. Mater 33 (46), e2006287. doi:10.1002/adma.202006287
Hiroto, S., and Wu, J. (2019). π-Conjugated compounds for molecular materials. Chem. Asian J. 14 (10), 1600–1601. doi:10.1002/asia.201900517
Irshad, R., Asim, S., Mansha, A., and Arooj, Y. (2023). Naphthalene and its derivatives: efficient fluorescence probes for detecting and imaging purposes. J. Fluoresc. 33 (4), 1273–1303. doi:10.1007/s10895-023-03153-y
Jiang, Y., Upputuri, P. K., Xie, C., Zeng, Z., Sharma, A., Zhen, X., et al. (2019). Metabolizable semiconducting polymer nanoparticles for second near-infrared photoacoustic imaging. Adv. Mater 31 (11), e1808166. doi:10.1002/adma.201808166
Jouaiti, A., Ballerini, L., Shen, H. L., Viel, R., Polo, F., Kyritsakas, N., et al. (2023). Binuclear copper(I) complexes for near-infrared light-emitting electrochemical cells. Angew. Chem. Int. Ed. Engl. 62, e202305569. doi:10.1002/anie.202305569
Kaeser, A., and Schenning, A. P. (2010). Fluorescent nanoparticles based on self-assembled pi-conjugated systems. Adv. Mater 22 (28), 2985–2997. doi:10.1002/adma.201000427
Kim, J. Y., Park, J. E., Jo, Y., Shim, W. H., Nam, S. J., Kim, J. H., et al. (2019). Incorporating diffusion- and perfusion-weighted MRI into a radiomics model improves diagnostic performance for pseudoprogression in glioblastoma patients. Neuro Oncol. 21 (3), 404–414. doi:10.1093/neuonc/noy133
Kubo, T. (2019). Synthesis, physical properties, and reactivity of stable, pi-conjugated, carbon-centered radicals. Molecules 24 (4), 665. doi:10.3390/molecules24040665
Lai, W. F., Deng, R., He, T., and Wong, W. (2021). A bioinspired, sustained-release material in response to internal signals for biphasic chemical sensing in wound therapy. Adv. Healthc. Mater 10 (2), e2001267. doi:10.1002/adhm.202001267
Lao, Y., Yang, S., Yu, W., Guo, H., Zou, Y., Chen, Z., et al. (2022). Multifunctional pi-conjugated additives for halide perovskite. Adv. Sci. (Weinh) 9 (17), e2105307. doi:10.1002/advs.202105307
Lewis, S. M., Asselin-Labat, M. L., Nguyen, Q., Berthelet, J., Tan, X., Wimmer, V. C., et al. (2021). Spatial omics and multiplexed imaging to explore cancer biology. Nat. Methods 18 (9), 997–1012. doi:10.1038/s41592-021-01203-6
Li, J., and Pu, K. (2019). Development of organic semiconducting materials for deep-tissue optical imaging, phototherapy and photoactivation. Chem. Soc. Rev. 48 (1), 38–71. doi:10.1039/c8cs00001h
Li, J., and Pu, K. (2020). Semiconducting polymer nanomaterials as near-infrared photoactivatable protherapeutics for cancer. Acc. Chem. Res. 53 (4), 752–762. doi:10.1021/acs.accounts.9b00569
Li, J., Tao, L., Wang, Y., Yao, Y., and Guo, Q. (2021a). Heptazine-based pi-conjugated materials for light-emitting. Front. Chem. 9, 717569. doi:10.3389/fchem.2021.717569
Li, L., Lv, Z., Man, Z., Xu, Z., Wei, Y., Geng, H., et al. (2021b). Polarity-active NIR probes with strong two-photon absorption and ultrahigh binding affinity of insulin amyloid fibrils. Chem. Sci. 12 (9), 3308–3313. doi:10.1039/d0sc03907a
Li, L., Shao, C., Liu, T., Chao, Z., Chen, H., Xiao, F., et al. (2020). An NIR-II-emissive photosensitizer for hypoxia-tolerant photodynamic theranostics. Adv. Mater 32 (45), e2003471. doi:10.1002/adma.202003471
Li, S., Deng, X., Cheng, H., Li, X., Wan, Y., Cao, C., et al. (2022a). Bright near-infrared pi-conjugated oligomer nanoparticles for deep-brain three-photon microscopy excited at the 1700 nm window in vivo. ACS Nano 16 (8), 12480–12487. doi:10.1021/acsnano.2c03813
Li, X., Jiang, H., He, N., Yuan, W. E., Qian, Y., and Ouyang, Y. (2022c). Graphdiyne-related materials in biomedical applications and their potential in peripheral nerve tissue engineering. Cyborg Bionic Syst. 2022 (9892526), 9892526. doi:10.34133/2022/9892526
Li, X., Liu, L., Li, S., Wan, Y., Chen, J. X., Tian, S., et al. (2019b). Biodegradable pi-conjugated oligomer nanoparticles with high photothermal conversion efficiency for cancer theranostics. ACS Nano 13 (11), 12901–12911. doi:10.1021/acsnano.9b05383
Li, X., Yu, S., Lee, Y., Guo, T., Kwon, N., Lee, D., et al. (2019a). In vivo albumin traps photosensitizer monomers from self-assembled phthalocyanine nanovesicles: a facile and switchable theranostic approach. J. Am. Chem. Soc. 141 (3), 1366–1372. doi:10.1021/jacs.8b12167
Li, Y., Tang, Y., Hu, W., Wang, Z., Li, X., Lu, X., et al. (2023). Incorporation of robust NIR-II fluorescence brightness and photothermal performance in a single large pi-conjugated molecule for phototheranostics. Adv. Sci. (Weinh) 10 (3), e2204695. doi:10.1002/advs.202204695
Li, Y., Zhu, B., Han, W., Tang, W., and Duan, X. (2022b). A bright chemiluminescence conjugated polymer-mesoporous silica nanoprobe for imaging of colonic tumors in vivo. Analyst 147 (10), 2060–2067. doi:10.1039/d2an00294a
Lin, H., Bai, H., Yang, Z., Shen, Q., Li, M., Huang, Y., et al. (2022). Conjugated polymers for biomedical applications. Chem. Commun. (Camb) 58 (52), 7232–7244. doi:10.1039/d2cc02177c
Liu, H., Hao, X., Duan, C., Yang, H., Lv, Y., Xu, H., et al. (2013). Al3+-induced far-red fluorescence enhancement of conjugated polymer nanoparticles and its application in live cell imaging. Nanoscale 5 (19), 9340–9347. doi:10.1039/c3nr02522e
Liu, J., Geng, Y., Li, D., Yao, H., Huo, Z., Li, Y., et al. (2020). Deep red emissive carbonized polymer dots with unprecedented narrow full width at half maximum. Adv. Mater 32 (17), e1906641. doi:10.1002/adma.201906641
Liu, W., Li, B., Gao, H., Wang, D., Wang, L., Yang, Z., et al. (2019). The application of small organic pi-conjugated discotic derivatives in photoacoustic imaging and photothermal conversion. Nanotechnology 30 (3), 035705. doi:10.1088/1361-6528/aaea25
Luo, M., Chen, D., Li, Q., and Xia, H. (2023). Unique properties and emerging applications of carbolong metallaaromatics. Acc. Chem. Res. 56 (8), 924–937. doi:10.1021/acs.accounts.2c00750
Ma, J., Lu, G., Huang, X., and Feng, C. (2021). π-Conjugated-polymer-based nanofibers through living crystallization-driven self-assembly: preparation, properties and applications. Chem. Commun. (Camb) 57 (98), 13259–13274. doi:10.1039/d1cc04825b
Mdluli, S. B., Ramoroka, M. E., Yussuf, S. T., Modibane, K. D., John-Denk, V. S., and Iwuoha, E. I. (2022). π-Conjugated polymers and their application in organic and hybrid organic-silicon solar cells. Polym. (Basel) 14 (4), 716. doi:10.3390/polym14040716
Miki, K., and Ohe, K. (2020). π-Conjugated macrocycles bearing angle-strained alkynes. Chemistry 26 (12), 2529–2575. doi:10.1002/chem.201904114
Mirzaei, S., Castro, E., and Hernandez Sanchez, R. (2021). Conjugated molecular nanotubes. Chemistry 27 (34), 8642–8655. doi:10.1002/chem.202005408
Montalti, M., Cantelli, A., and Battistelli, G. (2015). Nanodiamonds and silicon quantum dots: ultrastable and biocompatible luminescent nanoprobes for long-term bioimaging. Chem. Soc. Rev. 44 (14), 4853–4921. doi:10.1039/c4cs00486h
Naciri, Y., Hsini, A., Ajmal, Z., Navío, J., Bakiz, B., Albourine, A., et al. (2020). Recent progress on the enhancement of photocatalytic properties of BiPO(4) using pi-conjugated materials. Adv. Colloid Interface Sci. 280, 102160, 102160. doi:10.1016/j.cis.2020.102160
Narayanan, K. B., Kim, H. D., and Han, S. S. (2020). Biocompatibility and hemocompatibility of hydrothermally derived reduced graphene oxide using soluble starch as a reducing agent. Colloids Surf. B Biointerfaces 185, 110579, 110579. doi:10.1016/j.colsurfb.2019.110579
Narmani, A., Jahedi, R., Bakhshian-Dehkordi, E., Ganji, S., Nemati, M., Ghahramani-Asl, R., et al. (2023). Biomedical applications of PLGA nanoparticles in nanomedicine: advances in drug delivery systems and cancer therapy. Expert Opin. Drug Deliv. 20 (7), 937–954. doi:10.1080/17425247.2023.2223941
Neumann, E. K., Djambazova, K. V., Caprioli, R. M., and Spraggins, J. M. (2020). Multimodal imaging mass spectrometry: next generation molecular mapping in biology and medicine. J. Am. Soc. Mass Spectrom. 31 (12), 2401–2415. doi:10.1021/jasms.0c00232
Neumann, P. R., Erdmann, F., Holthof, J., Hädrich, G., Green, M., Rao, J., et al. (2021). Different PEG-PLGA matrices influence in vivo optical/photoacoustic imaging performance and biodistribution of NIR-emitting pi-conjugated polymer contrast agents. Adv. Healthc. Mater 10 (4), e2001089. doi:10.1002/adhm.202001089
Ong, A., Tao, T., Jiang, Q., Han, Y., Ou, Y., Huang, K., et al. (2022). Azulene-fused acenes. Angew. Chem. Int. Ed. Engl. 61 (44), e202209286. doi:10.1002/anie.202209286
Oubaha, H., Gohy, J. F., and Melinte, S. (2019). Carbonyl-based pi-conjugated materials: from synthesis to applications in lithium-ion batteries. Chempluschem 84 (9), 1179–1214. doi:10.1002/cplu.201800652
Parida, S., Patra, S. K., and Mishra, S. (2022). Self-assembling behaviour of perylene, perylene diimide, and thionated perylene diimide deciphered through non-covalent interactions. Chemphyschem 23 (23), e202200361. doi:10.1002/cphc.202200361
Patra, J. K., Das, G., Fraceto, L. F., Campos, E. V. R., Rodriguez-Torres, M. d. P., Acosta-Torres, L. S., et al. (2018). Nano based drug delivery systems: recent developments and future prospects. J. Nanobiotechnology 16 (1), 71. doi:10.1186/s12951-018-0392-8
Peng, J., Kim, D. D., Patel, J. B., Zeng, X., Huang, J., Chang, K., et al. (2022). Deep learning-based automatic tumor burden assessment of pediatric high-grade gliomas, medulloblastomas, and other leptomeningeal seeding tumors. Neuro Oncol. 24 (2), 289–299. doi:10.1093/neuonc/noab151
Pimachev, A., Nielsen, R. D., Karanovich, A., and Dahnovsky, Y. (2019). Ferromagnetism in 2D organic iron hemoglobin crystals based on nitrogenated conjugated micropore materials. Phys. Chem. Chem. Phys. 21 (46), 25820–25825. doi:10.1039/c9cp04509k
Qiao, K., Xu, L., Tang, J., Wang, Q., Lim, K. S., Hooper, G., et al. (2022). The advances in nanomedicine for bone and cartilage repair. J. Nanobiotechnology 20 (1), 141. doi:10.1186/s12951-022-01342-8
Rakstys, K., Igci, C., and Nazeeruddin, M. K. (2019). Efficiency vs. stability: dopant-free hole transporting materials towards stabilized perovskite solar cells. Chem. Sci. 10 (28), 6748–6769. doi:10.1039/c9sc01184f
Ranjbarzadeh, R., Caputo, A., Tirkolaee, E. B., Jafarzadeh Ghoushchi, S., and Bendechache, M. (2023). Brain tumor segmentation of MRI images: a comprehensive review on the application of artificial intelligence tools. Comput. Biol. Med. 152, 106405, 106405. doi:10.1016/j.compbiomed.2022.106405
Richard, M., Al-Ajaji, A., Ren, S., Foti, A., Tran, J., Frigoli, M., et al. (2020). Large-scale patterning of pi-conjugated materials by meniscus guided coating methods. Adv. Colloid Interface Sci. 275, 102080, 102080. doi:10.1016/j.cis.2019.102080
Samori, P., Feng, X., and Bonifazi, D. (2019). π-Conjugated molecules: from structure to function. Chempluschem 84 (9), 1177–1178. doi:10.1002/cplu.201900442
Schmitt, J., Jenni, S., Sour, A., Heitz, V., Bolze, F., Pallier, A., et al. (2018). A porphyrin dimer-GdDOTA conjugate as a theranostic agent for one- and two-photon photodynamic therapy and MRI. Bioconjug Chem. 29 (11), 3726–3738. doi:10.1021/acs.bioconjchem.8b00634
Sheikh Mohamed, M., Poulose, A. C., Veeranarayanan, S., Romero Aburto, R., Mitcham, T., Suzuki, Y., et al. (2016). Plasmonic fluorescent CdSe/Cu2S hybrid nanocrystals for multichannel imaging and cancer directed photo-thermal therapy. Nanoscale 8 (15), 7876–7888. doi:10.1039/c5nr05225d
Shiraki, T., Miyauchi, Y., Matsuda, K., and Nakashima, N. (2020). Carbon nanotube photoluminescence modulation by local chemical and supramolecular chemical functionalization. Acc. Chem. Res. 53 (9), 1846–1859. doi:10.1021/acs.accounts.0c00294
Smits, M. (2021). MRI biomarkers in neuro-oncology. Nat. Rev. Neurol. 17 (8), 486–500. doi:10.1038/s41582-021-00510-y
Soffietti, R., Ahluwalia, M., Lin, N., and Rudà, R. (2020). Management of brain metastases according to molecular subtypes. Nat. Rev. Neurol. 16 (10), 557–574. doi:10.1038/s41582-020-0391-x
Song, G., Lv, F., Huang, Y., Bai, H., and Wang, S. (2022). Conjugated polymers for gene delivery and photothermal gene expression. Chempluschem 87 (5), e202200073. doi:10.1002/cplu.202200073
Stahl, T., Bofinger, R., Lam, I., Fallon, K. J., Johnson, P., Ogunlade, O., et al. (2017). Tunable semiconducting polymer nanoparticles with INDT-based conjugated polymers for photoacoustic molecular imaging. Bioconjug Chem. 28 (6), 1734–1740. doi:10.1021/acs.bioconjchem.7b00185
Takahashi, M. (2021). Flat zigzag silicene nanoribbon with Be bridge. ACS Omega 6 (18), 12099–12104. doi:10.1021/acsomega.1c00794
Tang, J., Xie, T., Geng, J., Hua, J., and Wang, Z. (2021). Rearrangement strategy for the preparation of polymers with pi-conjugated structures. Front. Chem. 9, 665877. doi:10.3389/fchem.2021.665877
Tian, R., Feng, X., Wei, L., Dai, D., Ma, Y., Pan, H., et al. (2022). A genetic engineering strategy for editing near-infrared-II fluorophores. Nat. Commun. 13 (1), 2853. doi:10.1038/s41467-022-30304-9
Tian, Z., Yu, J., Wu, C., Szymanski, C., and McNeill, J. (2010). Amplified energy transfer in conjugated polymer nanoparticle tags and sensors. Nanoscale 2 (10), 1999–2011. doi:10.1039/c0nr00322k
Tomitaka, A., Ota, S., Nishimoto, K., Arami, H., Takemura, Y., and Nair, M. (2019). Dynamic magnetic characterization and magnetic particle imaging enhancement of magnetic-gold core-shell nanoparticles. Nanoscale 11 (13), 6489–6496. doi:10.1039/c9nr00242a
Torres, Y. A., Suarez, M., Caicedo, C., Valencia, H., and Flórez-López, E. (2020). Experimental Data on design, theoretical and correlation of the electronic and optical properties of diethynylphenylthiophene as photovoltaic materials. Data Brief. 30, 105579. doi:10.1016/j.dib.2020.105579
van der Voort, S. R., Incekara, F., Wijnenga, M. M. J., Kapsas, G., Gahrmann, R., Schouten, J. W., et al. (2023). Combined molecular subtyping, grading, and segmentation of glioma using multi-task deep learning. Neuro Oncol. 25 (2), 279–289. doi:10.1093/neuonc/noac166
Villanueva-Meyer, J. E., Mabray, M. C., and Cha, S. (2017). Current clinical brain tumor imaging. Neurosurgery 81 (3), 397–415. doi:10.1093/neuros/nyx103
Wang, S., Xiong, Y., Lalevee, J., Xiao, P., Liu, J., and Xing, F. (2020). Biocompatibility and cytotoxicity of novel photoinitiator pi-conjugated dithienophosphole derivatives and their triggered polymers. Toxicol Vitro 63, 104720. doi:10.1016/j.tiv.2019.104720
Wang, S. Y., Chen, X. X., Li, Y., and Zhang, Y. Y. (2016). Application of multimodality imaging fusion technology in diagnosis and treatment of malignant tumors under the precision medicine plan. Chin. Med. J. Engl. 129 (24), 2991–2997. doi:10.4103/0366-6999.195467
Wang, Z., Li, Y., Hao, A., and Xing, P. (2021). Multi-modal chiral superstructures in self-assembled anthracene-terminal amino acids with predictable and adjustable chiroptical activities and color evolution. Angew. Chem. Int. Ed. Engl. 60 (6), 3175–3184. doi:10.1002/ange.202011907
Wen, Q., Zhang, Y., Li, C., Ling, S., Yang, X., Chen, G., et al. (2019). NIR-II fluorescent self-assembled peptide nanochain for ultrasensitive detection of peritoneal metastasis. Angew. Chem. Int. Ed. Engl. 58 (32), 11117–11122. doi:10.1002/ange.201905643
Wu, D., Liu, S., Zhou, J., Chen, R., Wang, Y., Feng, Z., et al. (2021). Organic dots with large pi-conjugated planar for cholangiography beyond 1500 nm in rabbits: a non-radioactive strategy. ACS Nano 15 (3), 5011–5022. doi:10.1021/acsnano.0c09981
Wu, Y., Kang, J., Lesniak, W. G., Lisok, A., Zhang, H. K., Taylor, R. H., et al. (2022). System-level optimization in spectroscopic photoacoustic imaging of prostate cancer. Photoacoustics 27, 100378. doi:10.1016/j.pacs.2022.100378
Xiang, L., Zhang, J., Wang, W., Gong, L., Zhang, L., Yan, B., et al. (2020). Nanomechanics of pi-cation-pi interaction with implications for bio-inspired wet adhesion. Acta Biomater. 117, 294–301. doi:10.1016/j.actbio.2020.09.043
Xie, J., Wang, N., Dong, X., Wang, C., Du, Z., Mei, L., et al. (2019). Graphdiyne nanoparticles with high free radical scavenging activity for radiation protection. ACS Appl. Mater Interfaces 11 (3), 2579–2590. doi:10.1021/acsami.8b00949
Xu, X., Liu, R., and Li, L. (2015). Nanoparticles made of pi-conjugated compounds targeted for chemical and biological applications. Chem. Commun. (Camb) 51 (94), 16733–16749. doi:10.1039/c5cc06439b
Xu, Z., Liu, F., Zhang, T., Gu, Y., Lu, N., Xu, H., et al. (2020). Density functional theory-assisted electrochemical assay manipulated by a donor-acceptor structure toward pharmaceutical diagnostic. Anal. Chem. 92 (23), 15297–15305. doi:10.1021/acs.analchem.0c01272
Yao, Y., Ran, G., Hou, C. L., Zhang, R., Mangel, D. N., Yang, Z. S., et al. (2022). Nonaromatic organonickel(II) phototheranostics. J. Am. Chem. Soc. 144 (16), 7346–7356. doi:10.1021/jacs.2c00710
Yin, C., Zhen, X., Fan, Q., Huang, W., and Pu, K. (2017). Degradable semiconducting oligomer amphiphile for ratiometric photoacoustic imaging of hypochlorite. ACS Nano 11 (4), 4174–4182. doi:10.1021/acsnano.7b01092
Yin, X., Liu, J., and Jakle, F. (2021). Electron-deficient conjugated materials via p–π* conjugation with boron: extending monomers to oligomers, macrocycles, and polymers. Chemistry 27 (9), 2973–2986. doi:10.1002/chem.202003481
Yoon, J., Kwag, J., Shin, T. J., Park, J., Lee, Y. M., Lee, Y., et al. (2014). Nanoparticles of conjugated polymers prepared from phase-separated films of phospholipids and polymers for biomedical applications. Adv. Mater 26 (26), 4559–4564. doi:10.1002/adma.201400906
Yoon, K. Y., and Dong, G. (2021). Multicomponent polymerization for pi-conjugated polymers. Macromol. Rapid Commun. 42 (6), e2000646. doi:10.1002/marc.202000646
Yoshizawa, K. (2012). An orbital rule for electron transport in molecules. Acc. Chem. Res. 45 (9), 1612–1621. doi:10.1021/ar300075f
Zhan, R., and Liu, B. (2016). Functionalized conjugated polyelectrolytes for biological sensing and imaging. Chem. Rec. 16 (3), 1715–1740. doi:10.1002/tcr.201500308
Zhang, C., Zhou, L., Zhang, J., Fu, Y. Y., Zhang, X., Yu, C., et al. (2016). Green and facile synthesis of a theranostic nanoprobe with intrinsic biosafety and targeting abilities. Nanoscale 8 (36), 16204–16211. doi:10.1039/c6nr01845a
Zhao, Y. (2020). Special issue "new studies of conjugated compounds. Molecules 25 (14), 3220. doi:10.3390/molecules25143220
Zhao, Y., Dai, W., Peng, Y., Niu, Z., Sun, Q., Shan, C., et al. (2020). A corrole-based covalent organic framework featuring desymmetrized topology. Angew. Chem. Int. Ed. Engl. 59 (11), 4354–4359. doi:10.1002/anie.201915569
Zheng, R., Zhao, Q., Qing, W., Li, S., Liu, Z., Li, Q., et al. (2022). Carrier-free delivery of ultrasmall pi-conjugated oligomer nanoparticles with photothermal conversion over 80% for cancer theranostics. Small 18 (4), e2104521. doi:10.1002/smll.202104521
Zhou, X., and Liang, F. (2014). Application of graphene/graphene oxide in biomedicine and biotechnology. Curr. Med. Chem. 21 (7), 855–869. doi:10.2174/0929867320666131119124325
Zhou, X., Lv, F., Huang, Y., Liu, L., and Wang, S. (2020). Biohybrid conjugated polymer materials for augmenting energy conversion of bioelectrochemical systems. Chemistry 26 (66), 15065–15073. doi:10.1002/chem.202002041
Zhou, Y. B., and Zhan, Z. P. (2018). Conjugated microporous polymers for heterogeneous catalysis. Chem. Asian J. 13 (1), 9–19. doi:10.1002/asia.201701107
Keywords: π-conjugated materials, brain tumor, molecular design, fluorescence imaging, photothermal therapy, photodynamic therapy
Citation: Sun W, Wang C, Tian C, Li X, Hu X and Liu S (2023) Nanotechnology for brain tumor imaging and therapy based on π-conjugated materials: state-of-the-art advances and prospects. Front. Chem. 11:1301496. doi: 10.3389/fchem.2023.1301496
Received: 25 September 2023; Accepted: 26 October 2023;
Published: 08 November 2023.
Edited by:
Haichang Zhang, Qingdao University of Science and Technology, ChinaReviewed by:
Xing Li, Shaanxi Normal University, ChinaLimin Liu, Northwest University, China
Guangyao Kong, Xi’an Jiaotong University, China
Copyright © 2023 Sun, Wang, Tian, Li, Hu and Liu. This is an open-access article distributed under the terms of the Creative Commons Attribution License (CC BY). The use, distribution or reproduction in other forums is permitted, provided the original author(s) and the copyright owner(s) are credited and that the original publication in this journal is cited, in accordance with accepted academic practice. No use, distribution or reproduction is permitted which does not comply with these terms.
*Correspondence: Shifeng Liu, bGl1c2hpZmVuZzA5MDFAMTI2LmNvbQ==
†These authors have contributed equally to this work