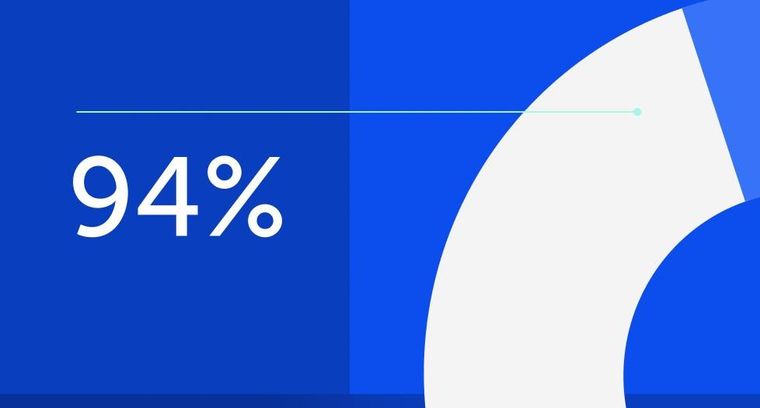
94% of researchers rate our articles as excellent or good
Learn more about the work of our research integrity team to safeguard the quality of each article we publish.
Find out more
ORIGINAL RESEARCH article
Front. Chem., 04 January 2024
Sec. Electrochemistry
Volume 11 - 2023 | https://doi.org/10.3389/fchem.2023.1298630
This article is part of the Research TopicInnovators in Chemistry: 2022View all 15 articles
Selecting the ideal anodic potential conditions and corresponding limiting current density to generate reactive oxygen species, especially the hydroxyl radical (•OH), becomes a major challenge when venturing into advanced electrochemical oxidation processes. In this work, a step-by-step guide for the electrochemical generation of •OH on boron-doped diamond (BDD) for beginners is shown, in which the following steps are discussed: i) BDD activation (assuming it is new), ii) the electrochemical response of BDD (in electrolyte and ferri/ferro-cyanide), iii) Tafel plots using sampled current voltammetry to evaluate the overpotential region where •OH is mainly generated, iv) a study of radical entrapment in the overpotential region where •OH generation is predominant according to the Tafel plots, and v) finally, the previously found ideal conditions are applied in the electrochemical degradation of amoxicillin, and the instantaneous current efficiency and relative cost of the process are reported.
When delving into the wide world of advanced oxidation processes (AOPs), we found an overwhelming amount of information that can be frustrating for a beginner. All these technologies mainly seek the generation of the hydroxyl radical (•OH) and other highly oxidizing reactive species (HORS) to carry out highly efficient oxidation processes. Advanced electrochemical oxidation (AEO) is one of the most studied AOPs for the oxidation/degradation of organic matter in aqueous media (Martínez-Huitle et al., 2015; Bessegato et al., 2021; Hand and Cusick, 2021; Pierpaoli et al., 2021; Espinoza-Montero et al., 2023). AEO consists of applying a polarization potential to a conductive substrate (metal or semiconductor) to produce HORS, mainly •OH if possible, on the electrode–solution interface (Ganiyu et al., 2021; Lee et al., 2022; Xu et al., 2023b). One of the most appreciated electrodes for AEO is the boron-doped diamond (BDD) electrode. BDD is a p-type semiconductor due to the chemical nature of boron, as they are impurity/dopant donors, so in thermal equilibrium, there is an imbalance of charge carriers in the valence and conduction bands, with the positive holes being the majority carriers (Yokoya et al., 2005; Yang et al., 2019). As an electrode, BDD is a very versatile material as it has unique electronic properties. It is a non-active electrode; i.e., its chemical structure is not compromised by the redox processes to which it may be subjected, although its reticular terminations are susceptible to slight changes during oxidation and reduction (Berenguer et al., 2019). As it has a very wide potential, this electrode has been used for multiple applications in electroanalytical chemistry (Wei et al., 2011b; Joshi et al., 2022), electrosynthesis (Ivandini and Einaga, 2017; Lips and Waldvogel, 2019), and AEO for water treatment (Cisneros-León et al., 2023).
For water treatment, electrochemical oxidation can take advantage of the complexity of mixtures of organic and inorganic species that can be found in typical contaminated water since, depending on the composition of the inorganic electrolyte in the system to be treated, the so-called HORS can be generated (Wen et al., 2017; Xu et al., 2021; Ahmed et al., 2022; Parvulescu et al., 2022; dos Santos et al., 2023; Mousset, 2023). Most HORS are “free” radicals and detrimental to most biological systems; the opposite is true for advanced oxidation processes, where they contribute to the oxidation of organic and inorganic matter (Murphy et al., 2022; Cisneros-León et al., 2023). HORS covers a wide spectrum of inorganic radicals, ions, and neutral molecules in aqueous media whose redox potentials are varied (Ross and Neta, 1982; Armstrong et al., 2015). Directly or indirectly, electrochemical oxidation can generate reactive oxygen species (ROS) such as the superoxide radical (O2•–), singlet oxygen (1O2), hydrogen peroxide (H2O2), and the strongest oxidant, the hydroxyl radical (•OH) (Xie et al., 2022). On the other hand, in the presence of nitrates and nitrites, through the reaction of the electrogenerated •OH, reactive nitrogen species (RNS) such as mono-, di-, and trioxide nitrogen radicals (NO•, NO2•, and NO3•) can be obtained (Wu et al., 2020; Rayaroth et al., 2022). Likewise, in the presence of sulfates, sulfites, and carbonates, sulfate (SO4•–) and sulfite (SO3•–) radicals, the persulfate anion (S2O82–), and the carbonate radical (CO3•) can be generated in situ, and in the presence of chlorides or chlorites, reactive chlorine species (RCS) are very common, such as the chlorine radical (Cl•) and the oxychloride radical (ClO•) (Ganiyu and Martínez-Huitle, 2019; Ganiyu et al., 2021; Zhou and Xiao, 2022). In addition, reactive phosphate species (RPS) are generated indirectly by reacting •OH with phosphates, producing phosphate radicals in three acidic forms, the H2PO4• radical being the most powerful oxidizing species of this type (Weiss et al., 2008). All these species can contribute greatly to the oxidation of organic and inorganic pollutants, achieving a predominant synergistic effect, or they can have the opposite effect since they all compete in coupled reactions in electrocatalysis.
This work intended to provide a step-by-step guide aimed primarily at beginners in the investigation of electrode properties in common electrolytic systems, with emphasis on the HORS that can be generated in the medium and that compete in catalysis. First, an approach from the existing theory in this field is addressed, and then, a real example is given using the BDD electrode in a known electrolytic environment.
To experimentally determine the optimal operating conditions at AEO, some fundamental electrochemical tests in known redox environments must be considered. In the following, we will carefully describe the suggested minimum steps that will allow us to develop our understanding of the optimal conditions for electrolysis at larger scales.
BDD is a “non-active” electrode when acting as an anode, and the HORS that are generated when a high oxidation overpotential is applied do not drastically change their chemical nature; this property makes it a unique material and is the basis for a large number of applications including in the area of environmental remediation (Sánchez-Montes et al., 2020; Carrera-Cevallos et al., 2021; Cisneros-León et al., 2023; Long et al., 2023). Therefore, studying the surface terminations of the crystal lattice of this material becomes key and is addressed in this section.
Diamond is a purely insulating material since its crystal lattice is composed of hybridized C-sp3. However, by introducing impurities of boron or nitrogen atoms during its synthesis using controlled methods at high temperatures and pressure, such as chemical vapor deposition (CVD) (Macpherson, 2015; Sun et al., 2021), a conducting, semiconducting, or superconducting material is obtained as a result of two factors; the first is that by introducing boron (or nitrogen) atoms, carriers that previously formed bonds are “released” and move freely through the lattice and participate in conduction in the BDD (Yokoya et al., 2005; Yang et al., 2019). On the other hand, when impurities are introduced to the diamond, it changes the hybridization of certain carbon atoms (from C-sp3 to Csp2), which extends to the surface terminations of the crystal lattice. The sp2 (C-sp2) hybridization (graphitic carbon) is the one that contributes the most to the conductive properties of BDD. Therefore, the surface termination of the BDD is a mixture of C-sp3 and C-sp2. When the surface termination is predominantly C-sp3, the BDD is said to have an H-termination, while when the surface termination is C-sp2, it is said that the surface termination is predominantly oxygen or oxygenated organic functional groups (Medeiros De Araújo et al., 2014; Garcia-Segura et al., 2015). Surface modifications of BDD from C-sp2 to C-sp3 or vice versa are possible through different controlled experimental methods. BDD electrode manufacturers often prefer methods that are as effective as their cost, e.g., oxygen plasma for oxygenated surfaces and hydrogen plasma for hydrogenated surfaces, but these are not practical for use in most laboratories (Kasahara et al., 2017; Einaga, 2022). On the other hand, surface modification of BDD electrochemically is simpler and cheaper; e.g., anodic polarization generates an oxygen surface termination, and cathodic polarization generates a hydrogen surface termination, for which a power source and a two-electrode cell are required (Figure 1A). This simple assay is commonly performed in strong acid media (H2SO4, HClO4, and HNO3) at moderate concentrations. The transitions from H to O or vice versa, in addition to the intrinsic equilibrium of adsorption–desorption of the gases produced on the surface—O2 at the anode and H2 at the cathode—are achieved as long as the redox potentials of the organic forms present in the lattice are reached; i.e., care must be taken to control the DC density applied to the cell as well as the time for which the electrode is exposed to oxidation or reduction.
FIGURE 1. (A) Two-electrode cell for electrolysis in an acidic medium performed in lab. (B) BDD surface transition by cathodic reduction, adapted from the work of Einaga (2022).
Einaga (2022) proposed a mechanism for the transition from oxygenated to hydrogenated BDD terminations. In essence, there is a homolytic contribution of hydrogen and the –O–H group from the BDD surface, yielding a good leaving group (water). In this way, the carbon formerly bonded to the R–CH2–O–H group leaves a hybrid orbital with an unpaired electron ripe to overlap with another atomic hydrogen and obtain surface groups with hydrogen-bonded carbons (R–CH2–H) (Figure 1B). In addition, electrolysis in a strong acidic medium serves to clean impurities from BDDs prior to use, if required. It is very common to adsorb carbon impurities generated during the synthesis of the BDD or by the adsorption of contaminants from the environment or from contact with other surfaces. Therefore, it is common that during the manufacture of BDDs, especially bipolar (double-sided modified) BDDs, a thin layer of hybrid material with major impurities remains, and electrochemical cleaning is useful to remove this layer, as recommended by the manufacturers themselves. Cleaning, which should be understood interchangeably as surface activation of the BDD, is a soft electrochemical method that does not compromise the crystalline integrity of the BDD, much less of the substrate on which the doped diamond layer has been deposited, which is commonly Nb or n-type Si.
By cyclic voltammetry (CV), the cleanliness and surface termination of the BDD can be evaluated, as shown in Figure 2A. The BDD has an impurity-free surface (Figure 2A top) when no peak appears in the potential range of the capacitive region, and only the extreme peaks of oxidation evolution (more positive potentials) and hydrogen evolution (more negative potentials) should be detected. However, additional signals characteristic of surface carbon impurities often appear when the electrode is not properly cleaned/activated (Figure 2A bottom). These CV profiles are typically recorded in the presence of the electrolyte only, commonly Na2SO4, H2SO4, and phosphate buffer solutions, in dilute concentration (0.1 M). Although it is also possible to use strong bases (NaOH or KOH) as the supporting electrolyte to generate these voltammograms, caution must be exercised when using a suitable reference electrode (Zoski, 2007).
FIGURE 2. (A) Voltammetric profiles of the full potential window of the BDD in the presence of an electrolyte. Without the presence of contaminants or adsorbed impurities, only the oxygen and hydrogen evolution peaks are observed (top), while on a soiled surface, peaks are present in the capacitive zone (bottom). (B) Typical BDD CVs in the ferri/ferro-cyanide redox couple for an oxygenated (left) and hydrogenated (right) surface.
CV, in addition to being useful for calculating the electrode area by applying the Randles–Sevcik equation, is also useful for evaluating the predominant surface termination of H or O on BDD after electrochemical treatment. For this purpose, one can use the [Fe(CN)6]3–/[Fe(CN)6]4– redox couple (or others) in dilute concentration and in a suitable electrolyte medium, typically KCl in a 1:100 ratio, although it is common to use more concentrated electrolyte solutions to avoid potential losses due to Ohmic drop (Suffredini et al., 2004; Actis et al., 2008; Zanin et al., 2014; Cheah and Chernev, 2021; Bard et al., 2022; Zheng, 2023). Figure 2B shows the typical CVs of BDD electrodes in the ferro/ferri-cyanide redox couple. At an electrode with oxygen surface terminations (Figure 2B left), the [Fe(CN)6]3–/[Fe(CN)6]4– redox process becomes very slow, and the separation of anodic potential (Ea) and cathodic potential (Ec) peaks is enormous; i.e., ΔEp (Ec- Ea) takes large values. On the contrary, in Figure 2B (right), a typical CV of a BDD electrode with H-terminations predominant on the surface is observed, where the redox couple response is fast, chemically and electrochemically reversible, ΔEp is close to 57 mV, and the width at half on the forward scan of the peak is close to 59 mV (Elgrishi et al., 2018). In practice, it is crucial to define which surface is of interest depending on the intended application of the BDD electrode (Oliveira et al., 2010; Kondo, 2022). For example, if a semiconductor-photocatalyst-modified electrode is required, it is ideal to have an oxidized surface as the physical and chemical interactions are stronger for oxygen with the semiconductor metal. On the other hand, if bare electrode electrocatalysis is what is sought, a predominantly hydrogenated surface is ideal since charge transfer at the solid–liquid interface is favored.
Once BDD is electrochemically characterized to “micro-scale,” the next step is the search for the “ideal” parameters to carry out electrochemical oxidation with the greatest efficiency, driven mainly by HORS, which will be discussed in depth later. AEO is a complex process involving the balance of several variables that must be carefully controlled in practice (Ganiyu et al., 2021; Bany Abdelnabi et al., 2022; Lee et al., 2022; Xu et al., 2023a; Zheng, 2023). In this section, we will focus mainly on the potential and/or current density to be applied to the system and how the electrochemical test should be carried out to find it. Our purpose is to systematically relate the Tafel analysis to the different electrolyte species that may be present and their consequent formation of HORS at the BDD electrode. For the construction of the Tafel curves, we will use voltammograms generated by sampled current voltammetry, a very effective and easy-to-apply technique.
To monitor an electrochemical process quantitatively and from the point of view of kinetics, the Tafel analysis is often very useful for certain processes (Bard et al., 2022; Shih et al., 2022). The equation that quantitatively relates the potential to the current of a specific system is called the Tafel equation (Eq. 1), and it was first designed to study the hydrogen evolution reaction (HER) (Tafel, 1905; Petrii et al., 2007; Fang and Liu, 2014; Jung et al., 2022).
where
To study the kinetics of redox processes in electrochemistry, the rotating disk electrode is employed since the speed of revolution is controlled and the diffusional and capacitive influences of the system are eliminated, thus allowing the kinetic parameters and charge transfer constants to be calculated with high reproducibility (Chen et al., 2020). However, not all laboratories have this sophisticated system at their disposal. Instead, sampled current voltammetry (SCV) is a useful technique that frees us from this experimental drawback, and the kinetics of reversible or irreversible reactions can be studied with the Tafel analysis without major problems (Soares et al., 2020; Rodríguez and Denuault, 2021).
An SCV assay consists of subjecting the working electrode to various polarization potentials—in a specific range and in the medium of interest—during a specific pulse time (chronoamperometry); in this sense, the so-called polarization curves are obtained (Figure 3A). The polarization curves are used to select the current density corresponding to each potential at a given time constant (
FIGURE 3. (A) Typical polarization curves at oxidation overpotentials in a given electrolyte. (B) j vs. η curve corresponding to OER. (C) Tafel plot for OER. (C) Adapted from the work of Kapałka et al. (2008).
Kapałka et al. (2008) pioneered the study of the kinetics of the oxygen evolution reaction (OER) at a BDD electrode (Figure 3C). They showed that in the Tafel analysis, there were two well-defined slopes corresponding to independent, one-to-one electron transfer processes. For our interest and depending on the electrolyte, the Tafel analysis gives us a hint of the optimal potential to produce HORS before oxygen evolution is favored (thermodynamically). Therefore, this kinetic study will allow us to select a wide range of anodic potentials and evaluate the production of HORS, as discussed below.
A further digression is necessary here to address the HORS generated on the BDD. Depending on the electrolyte in which the electrolysis is carried out, different reactive species capable of efficiently oxidizing organic molecules may coexist, some more than others. We cannot begin this discussion without first analyzing the ROS and, in essence, the hydroxyl radical (•OH). This radical is the first intermediate of the OER from water splitting (Reaction 1), and it is generated in a process favored by kinetics rather than thermodynamics; i.e., large overpotentials are required for its formation on the BDD surface (Siahrostami et al., 2017; Zhu, 2019; Xie et al., 2022).
BDD (OH*) refers to the •OH adsorbed on the surface of the BDD, specifically on the active site, which plays a crucial role (Moreira et al., 2017; Vogt and Weckhuysen, 2022). •OH is a highly reactive and unstable species whose lifetime is estimated to be a few nanoseconds (Moreira et al., 2017). The high reactivity of the •OH is explained by its unpaired electron in an antibonding orbital (π*) of an oxygen atom located at high energy (Figure 4). This instability (in energy) means that its electron easily pairs with an organic species to form covalent bonds in the electrooxidation pathway (Imlay, 2003; Krumova and Cosa, 2016). Its oxidizing power (∼2.73 V vs. NHE), second only to fluorine, is due to its high tendency to capture electrons and stabilize the antibonding orbital. Therefore, it is capable of non-selectively oxidizing a wide variety of organic pollutants as long as the redox potential of the target molecules is aligned with the redox potential of the radical at the interface (Armstrong et al., 2015).
FIGURE 4. Molecular orbital diagram for the •OH. Energy values were taken from the work of Mann et al. (2000).
On the other hand, molecular oxygen in its fundamental state is triplet (3O2) and is dissolved in the medium or generated by the electrolysis of water; it can trigger reduction reactions to generate other highly reactive species but with lower oxidizing power than the •OH (Krumova and Cosa, 2016). When O2 is reduced by the conduction band electrons of the BDD, on transfer of a first electron, it produces the superoxide anion radical O2•– (E0 = −0.18 V vs. NHE) (Armstrong et al., 2015; Hayyan et al., 2016). In brief, Figure 5 shows a schematic of the ROS generated by electron transfer and their redox potentials.
FIGURE 5. ROS formation by electron and energy transfer. Redox potential values are reported vs. NHE and were taken from the work of Armstrong et al. (2015).
In short, the •OH, due to its oxidizing power, is the species that is sought to be generated efficiently in AEO, and it will be seen from now on that this radical plays a crucial role in the generation of other highly reactive oxidizing species (Gligorovski et al., 2015). As for pH, it is also a parameter that greatly influences the generation of reactive species and electrocatalysis in general. We said that the •OH production process is kinetically favorable, where pH influences the rate of charge transfer (Tao et al., 2019; Zhang et al., 2020). At low pH, the concentration of H3O+ increases, which favors the generation of •OH. H3O+ can react with electrons from the electrode (depending on the electrode potential) and produce atomic hydrogen, which is highly reactive and reacts with water to produce •OH (Katsounaros et al., 2014). Very low pH can also be detrimental to the electrode as it can create a passivation layer on the electrode surface that limits charge transfer; in BDD, this is not a drawback as its high stability in strong acid environments frees it from this issue (Xie et al., 2022). Other considerations must be taken with respect to pH since the stability of the target molecule to be degraded is also a tremendously influential factor, as well as the concentration of the electrolyte species in the solution (Fornaciari et al., 2022).
The utility of ions in a solution is imperative for the vast majority of electrochemical processes (Bard et al., 2022). In the process at stake here, the supporting electrolyte plays a dual role since it is not only responsible for mitigating the migration of the electroactive species and serving as an ionic conductor in the medium but the ions can also react instantaneously with the electrogenerated •OH and produce other chemical species of high reactivity and, sometimes, high oxidizing power (Kim et al., 2018; Hand and Cusick, 2021). Many authors refer to this process as the “activation” of a certain species since in the absence of any perturbation, the ions are not a major threat to organic pollutants (Lin and Deng, 2021). In a medium containing sulfate ions, activation of the sulfate radical (
In the literature, the vast majority of works that seek to treat polluted water by electrochemical oxidation use sulfate-based electrolytes, typically sodium or potassium sulfate, or sulfuric acid. Why is this? This can be accounted for by the oxidizing power of this radical, which ranges from 2.5 to 3.1 V vs. NHE; these values compete with the oxidizing power of the •OH (Ganiyu and Martínez-Huitle, 2019; Ganiyu et al., 2021; Araújo et al., 2022). When comparing these two species, both radicals have an affinity for capturing electrons in redox processes since they have unpaired antibonding molecular orbitals in oxygen, while the electronegativity of S is greater than that of O, and the polarization of the bond is more efficient (Lin and Deng, 2021). Therefore, if these two species can coexist in a moderate “balance,” the treatment of contaminated water can be very efficient. Understanding the oxidation power of these species from theory can be a simple issue, but, in practice, it is not so since variables such as pH, applied potential and current density, temperature, and contaminants must be controlled.
Reactive chlorine species (RCS) are produced in an electrolytic chloride environment. Mainly, the chlorine radical (Cl•) is the outstanding species of this group with an oxidation power of approximately 2.43 V vs. NHE (Armstrong et al., 2015). It is produced by indirect oxidation, i.e., by the oxidation of Cl−at the electrode surface by the adsorbed •OH (Reaction 5) (Mostafa et al., 2018). On the other hand, the hypochlorite radical (ClO•), also a highly reactive species of chlorine, is produced by the same oxidation reaction by the •OH (Reaction 6) and has a relatively low oxidation power (1.39 V vs. NHE) (Armstrong et al., 2015). The •OH can trigger reactions with chloride in the medium to produce hypochlorite, chlorite, chlorate, and perchlorate anions, but they are not highlighted here since their oxidizing power is overshadowed by their toxicity, and reactions producing these species can be found anywhere (Ganiyu et al., 2021; Choo et al., 2022; Barnum and Coates, 2023).
Similarly, reactive phosphate species (RPS) are produced by hydroxyl radical chain reactions (Reactions 7–9). The phosphate radical (
Finally, it is not difficult to imagine that reactive nitrogen and carbon species are produced by the indirect oxidation of the hydroxyl or sulfate radical with nitrates, nitrites, and carbonates to generate nitrogen di- and trioxide radicals (
In this section, we left for a while the main aim of this work and tried not to digress in exposing all the species that can exist in an electrochemical reaction medium; instead, we emphasized the main highly reactive oxidizing species of each type depending on the electrolyte (Figure 6). In practice, it is obvious that after the ROS and, especially, •OH, the other species are the consequence of the redox reaction of the latter with the ions in solution; i.e., the other species can be regarded as trapping hydroxyl radicals. This is because •OH is not selective and reacts simultaneously with whoever stabilizes its unpaired electron most quickly and efficiently. All these species highlighted above compete in electrocatalysis, and attributing the major contribution to pollutant degradation to just one of them would be a mistake. Instead, emphasis should be placed on controlling the other variables that take place in electrochemical oxidation.
FIGURE 6. HORS generation pathway by reaction of the •OH in a one-to-one electron transfer. Redox potential values are reported vs. NHE and were taken from the work of Armstrong et al. (2015).
All the previous analyses can be carried out successfully with accuracies that slightly deviate from the theory, but when the results are transferred to a scale larger than that of the laboratory, it often involves complications that lead to results that deviate greatly from those predicted at the micro-scale, but are not wrong for the most part. These noticeable differences, which are often found at a real scale, are mainly due to the electrochemical cell and the intrinsic energy consumption. That is, when testing electrode behavior, electrolyte effects, and cell configuration in the laboratory, it is common to use simple systems on account of having known and small electrode areas, solution volumes no larger than 25 mL, and simple cell configurations, usually batch type (Bard et al., 2022). In the pre-pilot scale, all the dimensions of the upstream electrochemical components are increased. This increase has important implications for current and potential density. Thus, at scales larger than the laboratory, it is common to have control of the experimental limiting current, i.e., the current that maximizes the charge transfer efficiency of the redox reaction occurring at the electrode surface. Typically, the limiting current can be found in electrooxidation systems as a function of time in the chemical oxygen demand (COD) (Eq. 2) (Wei J. J. et al., 2011; Ochoa-Chavez et al., 2018).
where ilim is the limiting current, F is Faraday’s constant, and km is the mass transfer coefficient. For microelectrode or rotating disk arrays, the limiting current can be calculated by SCV in known redox systems, and its procedure can be found elsewhere (Soares et al., 2020; Bard et al., 2022).
Then, when scaling a system, it is not strange that current densities or overpotentials reach values higher than those predicted by Tafel curves or voltammetric profiles in various electrolytes (Moreira et al., 2017). This is because the diffusion flow of the species within the solution towards the electrode surface changes and, therefore, the energy barrier to be overcome also increases; on the other hand, the potential loss by Ohmic drop also increases significantly due to the resistance of the solution and the micro- and nano-bubbles that evolve on the surface, as well as the presence of the target molecule to be electrochemically oxidized (Petrii et al., 2007; Lee and Bazylak, 2021; Zheng, 2023). In practice and when disk electrodes are not available, the limiting potential and its limiting current are controlled in a non-analytical way (at least for now); i.e., when the electrooxidation test is carried out, the electrode potential is progressively increased, and the current density circulating in the reactor is controlled, and when the proportionality between the potential and current is lost, the limiting current has been exceeded; in other words, the maximum potential value that the system supports so that the charge transfer is also maximum corresponds to the current limit where the process is optimized.
Additionally, another parameter of interest when scaling certain processes is the determination of the instantaneous current efficiency (ICE), which gives us information regarding the optimum range of applicability of a process (Comninellis and Pulgarin, 1991; Xing et al., 2018); this can be calculated by the following equation:
where (COD)t and (COD)t+Δt are the chemical oxygen demand at times t and t + Δt (in g O2 dm-3), F is Faraday’s constant, V is the volume of electrolyte (dm3), and I is the applied current (A).
Furthermore, the energy consumption of the process is also relevant since it gives us a picture of the efficiency from the perspective of energy expenditure and also helps us to optimize not only the parameters but also the resources (Brillas et al., 2009). Energy consumption is calculated by the following equation:
where Ecell is the cell voltage (V), t is the electrolysis time (h), and I is the current passing through the cell (A). Therefore, the cost of the process can be obtained according to the following equation:
In summary, Scheme 1 presents a systematic representation of the steps that we aimed to introduce so far and serves as a guide for the user; we emphasize that this is a preliminary approach to get to know our working electrode in the presence of a suitable electrolyte. We encourage the authors to include new electrochemical and non-electrochemical techniques to gain a deeper understanding of the electrode materials to be investigated.
The following is a specific case study involving BDD in the search for the optimal potential range for the generation of HORS, applying the reasoning that has been shown so far in the search for the prime parameters by carrying out electrochemical tests that can be handled without major problems in any electrochemical laboratory.
Quite recently, Lu et al. (2023) highlighted the most recent advances in the effects of electrolytes in a solution for electrochemical processes and discussed their effects on different reactions of interest in electrochemistry, such as oxygen and hydrogen evolution reactions (HER and OER, respectively) and oxygen and CO2 reduction reactions (ORR and CO2RR, respectively). Nevertheless, to date, no review has been found in the literature that addresses, in a simplistic fashion and with an experimental approach, the investigation of ionic species in a solution and their participation in electrochemical oxidation as a guide for beginners. In this section, a real experimental example is presented as a case study that evaluates the behavior of BDD in Na2SO4 and other electrolytes of interest, emphasizing the steps that should be followed if one wishes to begin in this field.
Anhydrous sodium sulfate (N2SO4 99%) was purchased from J.T. Baker, N,N-Dimethyl-4-nitroso-aniline (for synthesis) was purchased from Merck, and amoxicillin was purchased from Sigma-Aldrich. All chemicals were used without further purification. BDD electrodes were purchased from CONDIAS and were cut into 25 mm × 15 mm rectangular plates, and an effective bipolar electrode area of 6.0 cm2 in a cell volume of 60.0 mL was used for all experiments. The BDD was previously electrochemically activated by anodic polarization for 10 min in 0.5 mol L-1 sulfuric acid (H2SO4 95%–97%, Merck) at 0.1 Acm-2 in a BK Precision AC/DC power supply. All electrochemical assays were carried out on a BioLogic potentiostat/galvanostat workstation in a typical three-electrode cell. The BDD served as the working electrode, Ag/AgCl served as the reference, and a graphite rod served as the counter electrode. For the degradation of amoxicillin (40 μM), a two-electrode system was used, with a BDD as the anode and a Pt mesh as the cathode.
Once the electrode or electrode material to be evaluated—BDD in this case—has been selected, the electrolyte medium in which the behavior of the electrode is to be investigated is chosen without any additional species in the solution. Therefore, the first step is to perform cyclic voltammetry at oxidative potentials—actually overpotentials—in the chosen medium; our interest is in Na2SO4 for reasons already discussed earlier. The CV gives us a first insight into the potential where oxygen evolution is kinetically and thermodynamically predominant. The inflection point of the oxidation branch of the CV indicates the potential where the transition from one electron transfer process to another is likely to occur and is likely to be the potential where most intermediate reactions compete, which is approximately 1.20 V vs. Ag/AgCl according to Figure 7A. Then, by chronoamperometry, we determine the polarization curves at the oxidation potentials determined in the previous CV profile (Figure 7B).
FIGURE 7. CV profile (A) and polarization curves (B) at oxidation overpotentials. The time constants were selected (C) for plotting the η vs. j curve by sampled current voltammetry (D). All assays were carried out on a typical three-electrode system (WE: BDD, CE: graphite rod, and RE: Ag/AgCl in Na2SO4 0.1 mol L-1).
After completing the polarization curves at the oxidation overpotentials, the next step is to choose a time interval, called time constant (τ), in which the process is controlled by charge transfer (Bard et al., 2022). For this purpose, the SCV is a useful tool that allows us to control this phenomenon when a rotating disk electrode is not available (Soares et al., 2020). Typically, it consists of choosing current values at small time constants and constructing η vs. j curves (Figures 7B, C). In this range, the process is kinetic-controlled, and the influence of diffusion, i.e., mass transfer, is limited, so the effect of the electrode capacitance does not affect our test, and all measurements are focused entirely on the Faradaic current (Soares et al., 2020; Rodríguez and Denuault, 2021; Bard et al., 2022). Finally, Figure 7D shows all the η vs. j curves corresponding to different time constants, and all of them fit the same trend.
As discussed in previous sections, the Tafel analysis is useful to evaluate the kinetics of an electrochemical process. In this sense, and summarized in the last step of our survey, the Tafel curve is constructed by plotting the logarithmic function of the current versus the overpotential (Figure 8). Three zones can be distinguished in this curve (highlighted by the straight lines). Each region of the curve has an associated slope, and this is the Tafel slope. The green line corresponding to the first zone has a slope value of 946 mV dec−1, and this region is purely capacitive (it can be checked with the CV profile). The overpotential values up to about 1.1 V vs. Ag/AgCl are potentials where the double layer of the electrode—at the solid–liquid interface—is charged, and all this charge is stored in this imaginary region of the electrode, the inner Helmholtz plane (Bard et al., 2022). The next region illustrated by the blue line has a Tafel slope of 458 mV dec−1, and this sharp change in slope indicates that another process(es) is taking place at the electrode surface. Finally, the last zone highlighted by the orange line has a slope of 370 mV dec−1. This region plotted at overpotentials above 1.3 V vs. Ag/AgCl refers to the last process. This zone is distinctive as oxygen evolution reaction is predominant (Kapałka et al., 2008). This is self-evident as the error bars in this last region are almost undistinguishable, i.e., the current and potential values do not oscillate to a large extent as long as the test is run again. The opposite is true for the previous zones. The intermediate zone is of interest to us as the former is easily discarded because of its capacitive contribution. The intermediate zone allows us to have an overview of the processes that can take place at these potential values. Since we know the chemical nature of the medium in which the test was carried out, we can say that this region is dominated by the generation of HORS that compete with the •OH, our main oxidant.
However, despite our efforts, we cannot be certain of the exact potential at which the most highly oxidizing species can be harnessed for any given process, and to do so would be a tremendous mistake. Instead, these steps allow us to determine a wide range of potentials and discriminate those that do not contribute to our process to save us errors, time, and costs of unnecessary laboratory testing. Quantitative assays such as the quantification of reactive species or degradation of a known contaminant should be added to this procedure to extend this assumption and have a more adequate approach to our purpose; with this, we will have approached our search for the optimal experimental conditions to achieve adequate efficiencies in our advanced electrochemical oxidation process. To experimentally verify the overpotential region where the highest amount of •OH is generated, a radical trapping study was performed, as shown below.
The •OH concentration was quantified by the N,N-Dimethyl-4-nitroso-aniline (RNO) method (Ochoa-Chavez et al., 2018). Anode overpotentials were chosen based on the Tafel plot at values above 1.26 V vs. Ag/AgCl, according to Figure 8. At an anodic overpotential value of 1.60 V vs. Ag/AgCl, the highest concentration of •OH is generated, while at 1.45 V vs. Ag/AgCl, the concentration is lower because the applied potential is not enough to generate the maximum concentration of •OH. On the other hand, at 1.70 V, the concentration decreases because OER is the predominant reaction (Figure 9).
FIGURE 9. Effect of anode overpotential in the production of •OH using a BDD anode, Pt cathode, and Ag/AgCl reference electrode.
vIn order to verify that the higher •OH radical production matches a maximum degradation efficiency, the electrooxidation of amoxicillin (AMX 40 μM) was tested. The electrochemical degradation of amoxicillin was evaluated under the best •OH production conditions, as discussed above. The degradation was followed by UV–Vis spectroscopy and chemical oxygen demand (COD). According to Figure 10A, at η = 1.40 V, the competition between the •OH electrogeneration (E° = 2.43 V vs. SHE) and formation of sulfate species
FIGURE 10. (A) 40 μM AMX degradation at different overpotentials. Inset: zero-order kinetic of amoxicillin removal. (B) COD of 40 μM AMX degradation at different overpotentials. Inset: pseudo-first-order kinetic of COD removal, using a BDD anode, Pt cathode, and Ag/AgCl reference electrode.
In summary, Table 1 shows the kinetic parameters, where rate constants for amoxicillin degradation were adapted to a zero-order kinetic model and a pseudo-first-order kinetic model to COD removal. The COD constant rate of 1.60 V was almost two times greater than others’ overpotentials; therefore, this is considered the optimal anode overpotential. In this case, this is the overpotential corresponding to the limit current, i.e., the current associated to the maximum capacity to degrade AMX.
Figure 11 shows the evolution of the instantaneous current efficiency (ICE) with respect to electrolysis time, where maximum values of ICE are reached in the first hours, after which it decays due to the oxidation process and the formation of organic compounds. In the case of 1.60 V, it can be observed that after 2 h, the ICE remains stable, so the generation of •OH is continuous and allows progressive degradation, as it was discussed previously. Nevertheless, the ICE of the other overpotentials decreases drastically.
FIGURE 11. Instantaneous current efficiency over time for the electrochemical degradation of amoxicillin using a BDD electrode.
On the other hand, Table 2 shows the energy consumption and operating cost for the degradation of amoxicillin at different overpotentials. The cost of operation was calculated based on the cost per kilowatt-hour in Ecuador, which is 0.096 USD until March 2023 (Global Petrol Prices, 2023).
The results showed that the energy used during the electrolysis under the optimal overpotential was 0.224 kWh m-3, and the operation cost was 0.022 USD m-3, which represents the lowest cost among the other potentials tested and is in mutual agreement with the most efficient potential of the degradation process.
Properly understanding the behavior of an electrode in a specific system can sometimes be a complicated endeavor, even more so when one does not know the charge transfer processes that may or may not take place in the medium under study and when this medium is a complicated mixture of organic and inorganic species. Electrochemical oxidation is a useful and efficient technology for many remediation applications if applied correctly, i.e., having adequate knowledge and control over the parameters that influence the process, both internal and external. In this sense, this work attempted to provide a step-by-step guide, particularly for beginners, on the tests to be considered in the investigation of electrodes and new electrode materials for advanced electrochemical oxidation processes, with emphasis on the range of anodic potentials where the generation of highly reactive oxidizing species predominates, which are priceless in these processes. It is not our intention that this guide should be followed rigorously, but rather that it should serve as a support for those who wish to get started in this field, adjusting these steps to their research objectives. The study of the kinetic behavior of BDD in different electrolytes by SCV, the Tafel plot, and trapping of radicals is essential to achieve results of this magnitude. A detailed study of the effect of the electrolyte is in progress in our research group.
Although this guide is particularly focused on the BDD, it is not limited to this electrode. This guide can be followed to investigate a new electrode material from an electrochemical point of view at first glance. In addition to the steps proposed here, the reader is free to implement other electrochemical techniques that suit his or her research. For example, electrochemical impedance spectroscopy is important when specific reaction mechanisms need to be studied and can provide valuable information about a particular electrode; however, this technique is beyond the scope of this work.
The original contributions presented in the study are included in the article/Supplementary Material; further inquiries can be directed to the corresponding author.
GC-C: formal analysis, investigation, methodology, and writing–original draft. CP-C: data curation, formal analysis, investigation, methodology, and writing–original draft. EM: funding acquisition, investigation, validation, and writing–review and editing. PE-M: conceptualization, data curation, funding acquisition, investigation, methodology, project administration, resources, supervision, validation, writing–original draft, and writing–review and editing.
The authors declare financial support was received for the research, authorship, and/or publication of this article. This work was funded by the Pontificia Universidad Católica del Ecuador through the project: Degradation of Microplastics by Photoelectrocatalysis Using a Titanium Dioxide-Modified Boron-Doped Diamond Photoanode (TiO2/BDD). Code: 030-UIO-2023.
The authors would like to thank the School of Chemical Sciences of the Pontificia Universidad Católica del Ecuador for lending them part of the equipment for the development of this work.
The authors declare that the research was conducted in the absence of any commercial or financial relationships that could be construed as a potential conflict of interest.
All claims expressed in this article are solely those of the authors and do not necessarily represent those of their affiliated organizations, or those of the publisher, the editors, and the reviewers. Any product that may be evaluated in this article, or claim that may be made by its manufacturer, is not guaranteed or endorsed by the publisher.
Actis, P., Denoyelle, A., Boukherroub, R., and Szunerits, S. (2008). Influence of the surface termination on the electrochemical properties of boron-doped diamond (BDD) interfaces. Electrochem. Commun. 10 (3), 402–406. doi:10.1016/j.elecom.2007.12.032
Ahmed, M., Mavukkandy, M. O., Giwa, A., Elektorowicz, M., Katsou, E., Khelifi, O., et al. (2022). Recent developments in hazardous pollutants removal from wastewater and water reuse within a circular economy. npj Clean. Water 5 (1), 12–25. doi:10.1038/s41545-022-00154-5
Araújo, K. C., dos Santos, E. V., Nidheesh, P. V., and Martínez-Huitle, C. A. (2022). Fundamentals and advances on the mechanisms of electrochemical generation of persulfate and sulfate radicals in aqueous medium. Curr. Opin. Chem. Eng. 38, 100870. doi:10.1016/j.coche.2022.100870
Armstrong, D. A., Huie, R. E., Koppenol, W. H., Lymar, S. V., Merényi, G., Neta, P., et al. (2015). Standard electrode potentials involving radicals in aqueous solution: inorganic radicals (IUPAC Technical Report). Pure Appl. Chem. 87 (11–12), 1139–1150. doi:10.1515/pac-2014-0502
Bany Abdelnabi, A. A., Al Theeb, N., Almomani, M. A., Ghanem, H., and Rosiwal, S. M. (2022). Effect of electrode parameters in the electro-production of reactive oxidizing species via boron-doped diamond under batch mode. Water Environ. Res. a Res. Publ. Water Environ. Fed. 94 (12), e10830. doi:10.1002/wer.10830
Bard, A. J., Faulkner, L. R., and White, H. S. (2022). Electrochemical methods fundamentals and applications. Hoboken, New Jersey: John Wiley and Sons, Ltd.
Barnum, T. P., and Coates, J. D. (2023). Chlorine redox chemistry is widespread in microbiology. ISME J. 17 (1), 70–83. doi:10.1038/s41396-022-01317-5
Berenguer, R., Quijada, C., La Rosa-Toro, A., and Morallón, E. (2019). Electro-oxidation of cyanide on active and non-active anodes: designing the electrocatalytic response of cobalt spinels. Sep. Purif. Technol. 208, 42–50. doi:10.1016/j.seppur.2018.05.024
Bessegato, G. G., Cooke, M., Christensen, P., Wood, D., and Zanoni, M. (2021). Synthesis and electrochemical characterization of Si/TiO2/Au composite anode: efficient oxygen evolution and hydroxyl radicals generation. Electrochimica Acta 370, 137742. doi:10.1016/J.ELECTACTA.2021.137742
Brillas, E., Sirés, I., and Oturan, M. A. (2009). Electro-fenton process and related electrochemical technologies based on fenton’s reaction chemistry. Chem. Rev. 109 (12), 6570–6631. doi:10.1021/cr900136g
Carrera-Cevallos, J. V., Prato-Garcia, D., Espinoza-Montero, P. J., and Vasquez-Medrano, R. (2021). Electro-oxidation of a commercial formulation of glyphosate on boron-doped diamond electrodes in a pre-pilot-scale single-compartment cell. Water, Air, Soil Pollut. 232 (2), 69. doi:10.1007/s11270-020-04941-z
Cheah, M. H., and Chernev, P. (2021). Electrochemical oxidation of ferricyanide. Sci. Rep. 11 (1), 23058–23067. doi:10.1038/s41598-021-02355-3
Chen, W., Xu, M. L., Li, M. F., Wei, Z., Cai, J., and Chen, Y. X. (2020). Quantifying intrinsic kinetics of electrochemical reaction controlled by mass transfer of multiple species under rotating disk electrode configuration. J. Electroanal. Chem. 872, 114042. doi:10.1016/j.jelechem.2020.114042
Choo, Z. S., Hsieh, M. C., Lin, H. H. H., Yang, J. S., and Lin, A. Y. C. (2022). Reactive chlorine species in the enhanced degradation of UV stabilizers during the sunlight/free chlorine process. Chemosphere 309, 136677. doi:10.1016/J.CHEMOSPHERE.2022.136677
Cisneros-León, D. G., Espinoza-Montero, P. J., Bolaños-Mendez, D., Alvarez-Paguay, J., Fernández, L., Saavedra-Alulema, P. F., et al. (2023). Electrochemical degradation of surfactants in domestic wastewater using a DiaClean® cell equipped with a boron-doped diamond electrode. Front. Chem. 11, 1–10. doi:10.3389/fchem.2023.900670
Comninellis, C., and Pulgarin, C. (1991). Anodic oxidation of phenol for waste water treatment. J. Appl. Electrochem. 21 (8), 703–708. doi:10.1007/BF01034049
dos Santos, A. J., Barazorda-Ccahuana, H. L., Caballero-Manrique, G., Chérémond, Y., Espinoza-Montero, P. J., González-Rodríguez, J. R., et al. (2023). Accelerating innovative water treatment in Latin America. Nat. Sustain. 2023, 349–351. doi:10.1038/s41893-022-01042-z
Einaga, Y. (2022). Boron-doped diamond electrodes: fundamentals for electrochemical applications. Accounts Chem. Res. 55, 3605–3615. doi:10.1021/acs.accounts.2c00597
Elgrishi, N., Rountree, K. J., McCarthy, B. D., Rountree, E. S., Eisenhart, T. T., and Dempsey, J. L. (2018). A practical beginner’s guide to cyclic voltammetry. J. Chem. Educ. 95 (2), 197–206. doi:10.1021/acs.jchemed.7b00361
Espinoza-Montero, P. J., Martínez-Huitle, C. A., and Loor-Urgilés, L. D. (2023). Technologies employed for carwash wastewater recovery. J. Clean. Prod. 401, 136722. doi:10.1016/J.JCLEPRO.2023.136722
Fang, J., Fu, Y., and Shang, C. (2014). The roles of reactive species in micropollutant degradation in the UV/free chlorine system. Environ. Sci. Technol. 48 (3), 1859–1868. doi:10.1021/es4036094
Fang, Y. H., and Liu, Z. P. (2014). Tafel kinetics of electrocatalytic reactions: from experiment to first-principles. ACS Catal. 4 (12), 4364–4376. doi:10.1021/cs501312v
Fornaciari, J. C., Weng, L. C., Alia, S. M., Zhan, C., Pham, T. A., Bell, A. T., et al. (2022). Mechanistic understanding of pH effects on the oxygen evolution reaction. Electrochimica Acta 405, 139810. doi:10.1016/J.ELECTACTA.2021.139810
Ganiyu, S. O., and Martínez-Huitle, C. A. (2019). Nature, mechanisms and reactivity of electrogenerated reactive species at thin-film boron-doped diamond (BDD) electrodes during electrochemical wastewater treatment. ChemElectroChem 6 (9), 2379–2392. doi:10.1002/celc.201900159
Ganiyu, S. O., Martínez-Huitle, C. A., and Oturan, M. A. (2021). Electrochemical advanced oxidation processes for wastewater treatment: advances in formation and detection of reactive species and mechanisms. Curr. Opin. Electrochem. 27, 100678. doi:10.1016/j.coelec.2020.100678
Garcia-Segura, S., Vieira Dos Santos, E., and Martínez-Huitle, C. A. (2015). Role of sp3/sp2 ratio on the electrocatalytic properties of boron-doped diamond electrodes: a mini review. Electrochem. Commun. 59, 52–55. doi:10.1016/j.elecom.2015.07.002
Gligorovski, S., Strekowski, R., Barbati, S., and Vione, D. (2015). Environmental implications of hydroxyl radicals (•OH). Chem. Rev. 115 (24), 13051–13092. doi:10.1021/cr500310b
Global Petrol Prices (2023). Ecuador electricity prices. Available at: https://www.globalpetrolprices.com/Ecuador/electricity_prices/.
Hand, S., and Cusick, R. D. (2021). Electrochemical disinfection in water and wastewater treatment: identifying impacts of water quality and operating conditions on performance. Environ. Sci. Technol. 55 (6), 3470–3482. doi:10.1021/acs.est.0c06254
Hayyan, M., Hashim, M. A., and Alnashef, I. M. (2016). Superoxide ion: generation and chemical implications. Chem. Rev. 116 (5), 3029–3085. doi:10.1021/acs.chemrev.5b00407
Imlay, J. A. (2003). Pathways of oxidative damage. Annu. Rev. Microbiol. 57, 395–418. doi:10.1146/annurev.micro.57.030502.090938
Ivandini, T. A., and Einaga, Y. (2017). Polycrystalline boron-doped diamond electrodes for electrocatalytic and electrosynthetic applications. Chem. Commun. 53 (8), 1338–1347. doi:10.1039/c6cc08681k
Joshi, P., Riley, P., Goud, K., Mishra, R. K., and Narayan, R. (2022). Recent advances of boron-doped diamond electrochemical sensors toward environmental applications. Curr. Opin. Electrochem. 32, 100920. doi:10.1016/j.coelec.2021.100920
Jung, O., Jackson, M. N., Bisbey, R. P., Kogan, N. E., and Surendranath, Y. (2022). Innocent buffers reveal the intrinsic pH- and coverage-dependent kinetics of the hydrogen evolution reaction on noble metals. Joule 6 (2), 476–493. doi:10.1016/j.joule.2022.01.007
Kapałka, A., Fóti, G., and Comninellis, C. (2008). Determination of the Tafel slope for oxygen evolution on boron-doped diamond electrodes. Electrochem. Commun. 10 (4), 607–610. doi:10.1016/j.elecom.2008.02.003
Kasahara, S., Natsui, K., Watanabe, T., Yokota, Y., Kim, Y., Iizuka, S., et al. (2017). Surface hydrogenation of boron-doped diamond electrodes by cathodic reduction. Anal. Chem. 89 (21), 11341–11347. doi:10.1021/acs.analchem.7b02129
Katsounaros, I., Cherevko, S., Zeradjanin, A. R., and Mayrhofer, K. J. J. (2014). Oxygen electrochemistry as a cornerstone for sustainable energy conversion. Angew. Chem. - Int. Ed. 53 (1), 102–121. doi:10.1002/anie.201306588
Kim, S., Kim, C., Lee, J., Kim, S., Lee, J., Kim, J., et al. (2018). Hybrid electrochemical desalination system combined with an oxidation process. ACS Sustain. Chem. Eng. 6 (2), 1620–1626. doi:10.1021/acssuschemeng.7b02789
Kondo, T. (2022). Recent electroanalytical applications of boron-doped diamond electrodes. Curr. Opin. Electrochem. 32, 100891. doi:10.1016/J.COELEC.2021.100891
Krumova, K., and Cosa, G. (2016). “Overview of reactive oxygen species,” in Singlet oxygen: applications in biosciences and nanosciences, 3–21. doi:10.1039/9781782620389-00001
Lee, J., Von Gunten, U., and Kim, J. H. (2020). Persulfate-based advanced oxidation: critical assessment of opportunities and roadblocks. Environ. Sci. Technol. 54 (6), 3064–3081. doi:10.1021/acs.est.9b07082
Lee, J. K., and Bazylak, A. (2021). Bubbles: the good, the bad, and the ugly. Joule 5 (1), 19–21. doi:10.1016/j.joule.2020.12.024
Lee, K. M., Lee, H. J., Seo, J., Lee, T., Yoon, J., Kim, C., et al. (2022). Electrochemical oxidation processes for the treatment of organic pollutants in water: performance evaluation using different figures of merit. ACS ES T Eng. 2 (10), 1797–1824. doi:10.1021/acsestengg.2c00228
Lin, Q., and Deng, Y. (2021). Is sulfate radical a ROS? Environ. Sci. Technol. 55 (22), 15010–15012. doi:10.1021/acs.est.1c06651
Lips, S., and Waldvogel, S. R. (2019). Use of boron-doped diamond electrodes in electro-organic synthesis. ChemElectroChem 6 (6), 1649–1660. doi:10.1002/CELC.201801620
Long, X., Huang, R., Li, Y., Wang, J., Zhang, M., and Zhang, I. Y. (2023). Understanding the electro-cocatalytic peroxymonosulfate-based systems with BDD versus DSA anodes: radical versus nonradical dominated degradation mechanisms. Sep. Purif. Technol. 309, 123120. doi:10.1016/j.seppur.2023.123120
Lu, X., Tu, W., Zhou, Y., and Zou, Z. (2023). Effects of electrolyte ionic species on electrocatalytic reactions: advances, challenges, and perspectives. Adv. Energy Mater. 13, 2300628. doi:10.1002/AENM.202300628
Macpherson, J. V. (2015). A practical guide to using boron doped diamond in electrochemical research. Phys. Chem. Chem. Phys. 17 (5), 2935–2949. doi:10.1039/c4cp04022h
Mann, J. B., Meek, T. L., and Allen, L. C. (2000). Configuration energies of the main group elements. J. Am. Chem. Soc. 122 (12), 2780–2783. doi:10.1021/ja992866e
Martínez-Huitle, C. A., Rodrigo, M. A., Sirés, I., and Scialdone, O. (2015). Single and coupled electrochemical processes and reactors for the abatement of organic water pollutants: a critical review. Chem. Rev. 115 (24), 13362–13407. doi:10.1021/acs.chemrev.5b00361
Medeiros De Araújo, D., Cañizares, P., Martínez-Huitle, C. A., and Rodrigo, M. A. (2014). Electrochemical conversion/combustion of a model organic pollutant on BDD anode: role of sp3/sp2 ratio. Electrochem. Commun. 47, 37–40. doi:10.1016/j.elecom.2014.07.017
Moreira, F. C., Boaventura, R. A., Brillas, E., and Vilar, V. J. (2017). Electrochemical advanced oxidation processes: a review on their application to synthetic and real wastewaters. Appl. Catal. B Environ. 202, 217–261. doi:10.1016/j.apcatb.2016.08.037
Mostafa, E., Reinsberg, P., Garcia-Segura, S., and Baltruschat, H. (2018). Chlorine species evolution during electrochlorination on boron-doped diamond anodes: in-situ electrogeneration of Cl2, Cl2O and ClO2. Electrochimica Acta 281, 831–840. doi:10.1016/j.electacta.2018.05.099
Mousset, E. (2023). Electrochemical hydrogenation for water purification made easy. Nat. Water 1 (1), 28–29. doi:10.1038/s44221-022-00011-2
Murphy, M. P., Bayir, H., Belousov, V., Chang, C. J., Davies, K. J. A., Davies, M. J., et al. (2022). Guidelines for measuring reactive oxygen species and oxidative damage in cells and in vivo. Nat. Metab. 4 (6), 651–662. doi:10.1038/s42255-022-00591-z
Ochoa-Chavez, A. S., Pieczyńska, A., Fiszka Borzyszkowska, A., Espinoza-Montero, P., and Siedlecka, E. (2018). Electrochemical degradation of 5-FU using a flow reactor with BDD electrode: comparison of two electrochemical systems. Chemosphere 201, 816–825. doi:10.1016/j.chemosphere.2018.03.050
Oh, W.Da, Dong, Z., and Lim, T. T. (2016). Generation of sulfate radical through heterogeneous catalysis for organic contaminants removal: current development, challenges and prospects. Appl. Catal. B Environ. 194, 169–201. doi:10.1016/j.apcatb.2016.04.003
Oliveira, B., and Oliveira-Brett, A. M. (2010). Voltammetric and electrochemical impedance spectroscopy characterization of a cathodic and anodic pre-treated boron doped diamond electrode. Electrochimica Acta 55 (15), 4599–4605. doi:10.1016/j.electacta.2010.03.016
Parvulescu, V. I., Epron, F., Garcia, H., and Granger, P. (2022). Recent progress and prospects in catalytic water treatment. Chem. Rev. 122 (3), 2981–3121. doi:10.1021/acs.chemrev.1c00527
Petrii, O. A., Nazmutdinov, R. R., Bronshtein, M. D., and Tsirlina, G. A. (2007). Life of the Tafel equation: current understanding and prospects for the second century. Electrochimica Acta 52 (11), 3493–3504. doi:10.1016/j.electacta.2006.10.014
Pierpaoli, M., Jakobczyk, P., Sawczak, M., Łuczkiewicz, A., Fudala-Książek, S., and Bogdanowicz, R. (2021). Carbon nanoarchitectures as high-performance electrodes for the electrochemical oxidation of landfill leachate. J. Hazard. Mater. 401, 123407. doi:10.1016/j.jhazmat.2020.123407
Rayaroth, M. P., Aravindakumar, C. T., Shah, N. S., and Boczkaj, G. (2022). Advanced oxidation processes (AOPs) based wastewater treatment - unexpected nitration side reactions - a serious environmental issue: a review. Chem. Eng. J. 430, 133002. doi:10.1016/j.cej.2021.133002
Rodríguez, O., and Denuault, G. (2021). The electron transfer kinetics of adsorbed species derived by sampled current voltammetry. J. Electroanal. Chem. 882, 115021. doi:10.1016/j.jelechem.2021.115021
Ross, A. B., and Neta, P. (1982). Rate constants for reactions of inorganic radicals in aqueous solution. Natl. Stand. Ref. Data Ser. 17 (3), 1027–1284. doi:10.1063/1.555808
Sánchez-Montes, I., Pérez, J. F., Sáez, C., Rodrigo, M. A., Cañizares, P., Aquino, J. M., et al. (2020). Assessing the performance of electrochemical oxidation using DSA® and BDD anodes in the presence of UVC light. Chemosphere 238, 124575. doi:10.1016/J.CHEMOSPHERE.2019.124575
Shih, A. J., Monteiro, M. C. O., Dattila, F., Pavesi, D., Philips, M., da Silva, A. H. M., et al. (2022). Water electrolysis. Nat. Rev. Methods Prim. 2 (1), 84–91. doi:10.1038/s43586-022-00164-0
Siahrostami, S., Li, G. L., Viswanathan, V., and Nørskov, J. K. (2017). One- or two-electron water oxidation, hydroxyl radical, or H2O2 evolution. J. Phys. Chem. Lett. 8 (6), 1157–1160. doi:10.1021/acs.jpclett.6b02924
Soares, C. O., Rodríguez, O., Buvat, G., Duca, M., Garbarino, S., Guay, D., et al. (2020). Sampled current voltammetry for kinetic studies on materials unsuitable for rotating discs or microelectrodes: application to the oxygen reduction reaction in acidic medium. Electrochimica Acta 362, 136946. doi:10.1016/j.electacta.2020.136946
Suffredini, H. B., Pedrosa, V. A., Codognoto, L., Machado, S. A., Rocha-Filho, R. C., and Avaca, L. A. (2004). Enhanced electrochemical response of boron-doped diamond electrodes brought on by a cathodic surface pre-treatment. Electrochimica Acta 49, 4021–4026. doi:10.1016/j.electacta.2004.01.082
Sun, L., Yuan, G., Gao, L., Yang, J., Chhowalla, M., Gharahcheshmeh, M. H., et al. (2021). Chemical vapour deposition. Nat. Rev. Methods Prim. 1 (1), 5. doi:10.1038/s43586-020-00005-y
Tafel, J. (1905). Über die Polarisation bei kathodischer Wasserstoffentwicklung. Z. für Phys. Chem. 50U (1), 641–712. doi:10.1515/zpch-1905-5043
Tao, H. B., Zhang, J., Chen, J., Zhang, L., Xu, Y., Chen, J. G., et al. (2019). Revealing energetics of surface oxygen redox from kinetic fingerprint in oxygen electrocatalysis. J. Am. Chem. Soc. 141 (35), 13803–13811. doi:10.1021/jacs.9b01834
Vogt, C., and Weckhuysen, B. M. (2022). The concept of active site in heterogeneous catalysis. Nat. Rev. Chem. 6 (2), 89–111. doi:10.1038/s41570-021-00340-y
Wei, J. J., Zhu, X. -P., Lü, F. -X., Ni, J. R., et al. (2011a). Comparative study of oxidation ability between boron-doped diamond (BDD) and lead oxide (PbO2) electrodes. Int. J. Minerals, Metallurgy Mater. 18 (5), 589–593. doi:10.1007/s12613-011-0482-1
Wei, M., Liu, Y., Gu, Z. -Z., and Liu, Z. -D. (2011b). Electrochemical detection of catechol on boron-doped diamond electrode modified with Au/TiO2 nanorod composite. J. Chin. Chem. Soc. 58 (4), 516–521. doi:10.1002/JCCS.201190015
Weiss, E., Sáez, C., Groenen-Serrano, K., Cañizares, P., Savall, A., and Rodrigo, M. A. (2008). Electrochemical synthesis of peroxomonophosphate using boron-doped diamond anodes. J. Appl. Electrochem. 38 (1), 93–100. doi:10.1007/s10800-007-9405-2
Wen, Y., Schoups, G., and Van De Giesen, N. (2017). Organic pollution of rivers: combined threats of urbanization, livestock farming and global climate change. Sci. Rep. 7 (1), 43289–9. doi:10.1038/srep43289
Wu, Y., Bu, L., Duan, X., Zhu, S., Kong, M., Zhu, N., et al. (2020). Mini review on the roles of nitrate/nitrite in advanced oxidation processes: radicals transformation and products formation. J. Clean. Prod. 273, 123065. doi:10.1016/j.jclepro.2020.123065
Xie, J., Zhang, C., and Waite, T. D. (2022). Hydroxyl radicals in anodic oxidation systems: generation, identification and quantification. Water Res. 217, 118425. doi:10.1016/j.watres.2022.118425
Xing, X., Ni, J., Zhu, X., Jiang, Y., and Xia, J. (2018). Maximization of current efficiency for organic pollutants oxidation at BDD, Ti/SnO2-Sb/PbO2, and Ti/SnO2-Sb anodes. Chemosphere 205, 361–368. doi:10.1016/j.chemosphere.2018.04.090
Xu, J., Zheng, X., Feng, Z., Lu, Z., Zhang, Z., Huang, W., et al. (2021). Organic wastewater treatment by a single-atom catalyst and electrolytically produced H2O2. Nat. Sustain. 4 (3), 233–241. doi:10.1038/s41893-020-00635-w
Xu, T., Fu, L., Zhang, M., Wang, W., Hu, B., Zhou, Y., et al. (2023a). Electrochemical oxidation degradation of rhodamine B dye on boron-doped diamond electrode: input mode of power attenuation. J. Clean. Prod., 116544. doi:10.1016/j.jclepro.2023.136794
Xu, Y., Mao, Z., Qu, R., Wang, J., Yu, J., Luo, X., et al. (2023b). Electrochemical hydrogenation of oxidized contaminants for water purification without supporting electrolyte. Nat. Water 1 (1), 95–103. doi:10.1038/s44221-022-00002-3
Yang, N., Yu, S., Macpherson, J. V., Einaga, Y., Zhao, H., Zhao, G., et al. (2019). Conductive diamond: synthesis, properties, and electrochemical applications. Chem. Soc. Rev. 48 (1), 157–204. doi:10.1039/c7cs00757d
Yokoya, T., Nakamura, T., Matsushita, T., Muro, T., Takano, Y., Nagao, M., et al. (2005). Origin of the metallic properties of heavily boron-doped superconducting diamond. Nature 438 (7068), 647–650. doi:10.1038/nature04278
Zanin, H., May, P. W., Fermin, D. J., Plana, D., Vieira, S. M. C., Milne, W. I., et al. (2014). Porous boron-doped diamond/carbon nanotube electrodes. ACS Appl. Mater. Interfaces 6 (2), 990–995. doi:10.1021/am4044344
Zhang, J., Tao, H. B., Kuang, M., Yang, H. B., Cai, W., Yan, Q., et al. (2020). Advances in thermodynamic-kinetic model for analyzing the oxygen evolution reaction. ACS Catal. 10 (15), 8597–8610. doi:10.1021/acscatal.0c01906
Zheng, W. (2023). iR compensation for electrocatalysis studies: considerations and recommendations. ACS Energy Lett. 8, 1952–1958. doi:10.1021/acsenergylett.3c00366
Zhou, H., and Xiao, D. (2022). Role of NOM in the photolysis of chlorine and the formation of reactive species in the solar/chlorine system. ACS Omega 7 (9), 7769–7776. doi:10.1021/acsomega.1c06616
Zhu, R. C. (2019). Fish the oxygen intermediates. Joule 3 (6), 1408–1409. doi:10.1016/j.joule.2019.05.024
Keywords: electrochemical oxidation, highly oxidizing reactive species, sampled current voltammetry, boron-doped diamond, Tafel plot, amoxicillin degradation
Citation: Castillo-Cabrera GX, Pliego-Cerdán CI, Méndez E and Espinoza-Montero PJ (2024) Step-by-step guide for electrochemical generation of highly oxidizing reactive species on BDD for beginners. Front. Chem. 11:1298630. doi: 10.3389/fchem.2023.1298630
Received: 22 September 2023; Accepted: 07 December 2023;
Published: 04 January 2024.
Edited by:
Citlalli Gaona-Tiburcio, Autonomous University of Nuevo León, MexicoReviewed by:
José Luis Tristancho Reyes, Technological University of Pereira, ColombiaCopyright © 2024 Castillo-Cabrera, Pliego-Cerdán, Méndez and Espinoza-Montero. This is an open-access article distributed under the terms of the Creative Commons Attribution License (CC BY). The use, distribution or reproduction in other forums is permitted, provided the original author(s) and the copyright owner(s) are credited and that the original publication in this journal is cited, in accordance with accepted academic practice. No use, distribution or reproduction is permitted which does not comply with these terms.
*Correspondence: Patricio J. Espinoza-Montero, pespinoza646@puce.edu.ec
†These authors have contributed equally to this work and share first authorship
Disclaimer: All claims expressed in this article are solely those of the authors and do not necessarily represent those of their affiliated organizations, or those of the publisher, the editors and the reviewers. Any product that may be evaluated in this article or claim that may be made by its manufacturer is not guaranteed or endorsed by the publisher.
Research integrity at Frontiers
Learn more about the work of our research integrity team to safeguard the quality of each article we publish.