- 1Centro de Química Médica, Instituto de Ciencias e Innovación en Medicina, Facultad de Medicina, Clínica Alemana Universidad del Desarrollo, Santiago, Chile
- 2Facultad de Química y Biología, Universidad de Santiago de Chile, USACH, Santiago, Chile
The performance of Candida antarctica lipase B (CALB) has been evaluated in 1-butyl-3-methylimidazolium tetrafluoroborate (BMIMBF4)/water mixtures in a wide range of molar fractions (
1 Introduction
Natural resources facilitate reactions under gentle conditions. Enzymes, derived from readily available sources, serve as biodegradable, non-hazardous, and non-toxic catalysts. Typically, enzymatic reactions occur under mild conditions, such as physiological pH, room temperature, and atmospheric pressure. Leveraging enzymes in processes proves to be environmentally appealing, cost-effective, and sustainable.
In addition, biocatalysis considers at least 10 of the 12 principles of green chemistry (GC) (Sheldon and Woodley, 2018). GC, also known as sustainable chemistry, is not a particular set of technologies, but rather an area of study that emphasizes on the design of chemical products and processes with the aim of strongly reducing or eliminating chemicals that may become hazardous when transferred to the environment as waste (Sheldon, 2000). Catalysis is involved in i) highly selective and short synthesis and ii) products of high purity from a process that is efficient in energy with less waste compared to non-GC processes (Sheldon, 2016). Therefore, catalysts play a meaningful role in GC: i) decreasing energy requirements; ii) increasing selectivity; iii) diminishing hazardous conditions; and iv) minimizing side products (Sheldon, 1997; Sheldon et al., 2007; Vekariya, 2017).
Lipases are a sub-class of enzymes within the esterase family whose natural function is to hydrolyze long chains of oils and fats (Schomburg et al., 1991; Fojan et al., 2000). Hydrolytic enzymes have found widespread application in organic synthesis as ecofriendly catalysts with versatile substrate specificities. They exhibit high stereoselectivity, operate under mild reaction conditions, are readily available commercially, and do not require cofactors (Dolman et al., 1997; Ventura et al., 2012). Among these enzymes, Candida antarctica lipase B (CALB) stands out as one of the most effective catalysts, recognized for its exceptional stability compared to other lipases. CALB, a monomeric protein composed of 317 amino acids, belongs to the α/β-hydrolase fold family. Its active site comprises serine, asparagine/glutamate, and histamine. Notably, CALB distinguishes itself from most lipases by lacking a lid covering the entrance to its active site. Demonstrating efficiency, CALB is a catalyst for hydrolysis in water and esterification in certain organic solvents (Wu et al., 2013; Rabbani et al., 2015).
Water is considered the greenest solvent based on its chemical nature and quantity. However, some enzymatic reactions that contain hydrophobic substrates cannot take place in aqueous media (Xu et al., 2017). On the other hand, the removal of water from catalytic processes that proceed in aqueous media is extremely expensive due to its high boiling point (Sheldon and Woodley, 2018), which creates the need for water replacement toward conventional organic solvents (COSs). Hence, COSs have been used in biocatalysis to increase enzyme stability, improve the solubility of hydrophobic reagents, and to prevent unwanted side reactions (Zaks and Klibanov, 1984). However, COSs are highly volatile due to their significant vapor pressure, flammability, and toxicity. Moreover, the inhibitory activity rates related to the enzyme are much lower in COSs than in water (Zaks and Klibanov, 1984; Carrea and Riva, 2008; Sheldon, 2016; Xu et al., 2017). Khmelnitsky et al. (1994) reported that the enzymatic activity in COSs can be increased by lyophilization with large amounts of salt (KCl). An alternative to the COS are ionic liquids (ILs). Further studies of enzymatic catalysis in room-temperature ionic liquids (RTILs) have shown increases in their rate coefficients compared with COSs (Itoh, 2017). RTILs are molten salts composed entirely of cations and anions that melt below 100°C (Welton, 1999; Weingärtner, 2008) with remarkable physicochemical properties, i.e., being non-flammable, non-corrosive, and non-volatile and bulk physical constant, which can be tuned by combining different cations and anions (Freemantle, 1998; Chiappe et al., 2007). High combinatorial flexibility has converted these materials into “designer solvents” or “task-specific” solvents (Freemantle, 1998; Chiappe et al., 2007) whose properties can be specified to suit the requirements of a particular reaction (Reichardt and Welton, 2011). For these reasons, RTILs have gained importance in the biocatalysis field, being recognized as a very promising reaction medium. RTILs have shown that enzymes have the same catalytic behavior compared to water and COSs, improving enzyme selectivity, activity, and stability and preventing unwanted side reactions (Xanthakis et al., 2006; de Gonzalo et al., 2007; Sheldon, 2016). Previous studies have shown that RTILs with hydrophobic anions are less denaturing than COSs displaying high catalytic activities, while hydrophilic RTILs depend on the anion/cation moieties and alkyl chains, displaying harmful effects on enzyme activity/stability (Khmelnitsky et al., 1994; Sheldon et al., 2007; Van Rantwijk and Sheldon, 2007).
Since the 1990s, several types of enzymatic reactions in non-aqueous media have been studied, searching for alternative reaction media with an impact on GC (Gupta, 1992; Ballesteros et al., 1995; Cheong et al., 2022; Xue et al., 2022; Migowski et al., 2023). These have mainly considered proteases and lipases (Gupta, 1992; Ballesteros et al., 1995). The results based on the rate of the enzymatic reactions highlight the key role of hydrophobicity and polarity of the environment (Laszlo and Compton, 2001). Studies in solvent effects in enzymatic catalysis are a complex process as differences in enzyme hydration (Halling, 1994) and solvation of the enzyme and substrate must be considered. So, the key role of solvent effects focuses on the enzymatic activity for each solvent studied (Halling, 1994; Klibanov, 1997; Eckstein et al., 2002), and there is great scope within this field yet to be explored. Sheldon et al. (2002) published a second article on enzymes in RTILs and first on CALB in 1-butyl-3-methyl imidazolium hexafluorophosphate (BMIMPF6) and 1-butyl-3-methyl imidazolium tetrafluoroborate (BMIMBF4) comparing those RTILs with some COSs (Lau et al., 2000). Currently, a great number of publications show that RTILs based on hydrophobic anions, such as BF4−, PF6−, and bis(trifluoromethylsulfonyl)imide (NTF2-) are less denaturing than some COSs, and they are responsible for higher catalytic activities (Van Rantwijk and Sheldon, 2007; Abe et al., 2008). However, hydrophilic anions, such as nitrate, acetate, or lactate anions, have a deleterious effect on the enzyme activity/stability by the formation of a strong hydrogen bond (HB) or Coulombic interactions (Sheldon et al., 2002; Lau et al., 2004). Therefore, anion studies based on RTILs have suggested that employing less polar RTILs may maintain a protective water layer around the enzyme, thereby contributing to its stabilization (Micaêlo and Soares, 2008; Attri et al., 2011). Perhaps, this first shell of solvation might play a key role in enzyme activity through the HB established between the enzyme and anion(RTIL) (Sheldon et al., 2002). So, the anions should be able to accept the HB in order to maintain the structural conformation of the enzyme, discarding small and charged anions able to penetrate the protein matrix, reducing the flexibility or mobility of the enzyme active site (Anderson et al., 2002; Sheldon et al., 2002). On the other hand, increasing the alkyl chain in the cation leads to an increase in the hydrophobicity and van der Waals interactions responsible for the partial or total obstruction of the active site of the enzyme hindering the substrate–enzyme interaction and reducing the lipase activity (Fan et al., 2016).
Three approaches to working with non-conventional solvents in biocatalysis are i) pure solvent; ii) co-solvent in aqueous systems, and iii) biphasic systems (Kragl et al., 2002). In general, the solvent effect over the catalytic performance is described as i) stripping off the water layer around the enzyme interface; ii) penetrating the micro-aqueous phase to interact with the enzyme in order to change the conformation and/or active site; and iii) interacting directly with substrates and products or modifying their partitioning between hydrophilic and hydrophobic phases (Yang, 2009; Ventura et al., 2012). In summary, the influence of the reaction media over the enzymatic reaction is studied in terms of improving selectivity, activity, and stability. This influence depends on the catalyzed reaction and nature of the enzyme under study. Therefore, it is significant to elucidate under what circumstances and how the biocatalyst preserves its biological function and stability in these solvents. Currently, the research on relationships between solvents and enzyme functions is a large field to explore in order to identify suitable solvents that ensure enzyme stability/activity.
Despite the green features of the ILs, it is worth highlighting their potential damage to the environment (Pernak et al., 2001; Amde et al., 2015). Gonzalves et al. (2021) evidenced that the effect of these non-conventional solvents exerts an action over different organisms, suggesting that a critical role is centered over the cation based on their lipophilicity feature compared to the minor role of the anion (Gonzalves et al., 2021).
More recently, ionic liquids have been used as a surfactant in order to improve the lipase activity. In fact, surfactants immersed in ILs are a promising reaction medium because the interactions established (surfactant–water–IL) are minimized due to i) the nature of the surfactant headgroup and counterions (Calderón et al., 2019) and ii) solvation effects (Wijaya et al., 2016; Vicent-Luna et al., 2017). Then, this work uses BMIMBF4 as a reference solvent and its long chain derivative, i.e., 1-dodecyl-3-methylimidazolium tetrafluoroborate (C12-MIMBF4), and our main aim is to evaluate the influence of molar fractions of BMIMBF4/water mixtures over micellar aggregates in order to assess the activity of the enzymatic reaction. The investigated reaction corresponds to the hydrolysis of the substrate p-nitrophenyl laureate (p-NPL) in each reaction medium (see Scheme 1 below). This work shows a comparative study of the activity of CALB in pure and solvent mixtures at different molar fractions of BMIMBF4/potassium phosphate buffer solution (considered water) and the same molar fractions with C12-MIMBF4, respectively.
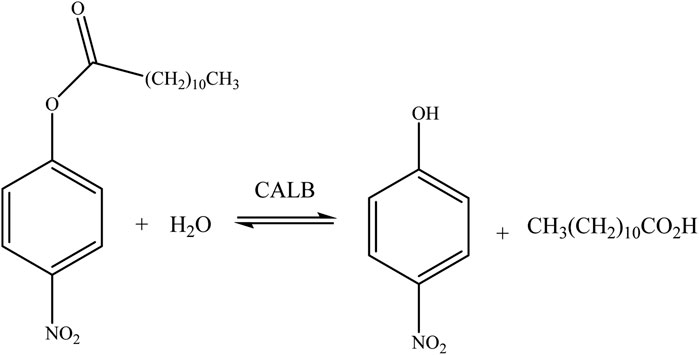
SCHEME 1. General picture of the hydrolysis reaction of the substrate p-nitrophenyl laureate (p-NPL) mediated by CALB.
2 Experimental section
2.1 Materials and methods
2.1.1 Materials
BMIMBF4 and C12-MIMBF4 were purchased from Merck and IoLiTec, respectively. The specifications for BMIMBF4 were purity (HPLC) >98% and water (KF) < 0.1%. C12-MIMBF4 was not fully tested based on regulation (EC) 1272/2008. CALB, p-NPL (purity ≥98% by gas chromatography [GC]) 1, p-nitrophenol (p-NP, purity (DSC assay) > 99.5%), and dimethyl sulfoxide (DMSO, purity (GC) > 99.9%) were acquired from Sigma. The salts KH2PO4 and K2HPO4 were acquired from Merck (purities (alkalimetric assay) > 99.5%). All reagents were used as soon as delivered. Ultrapure water was used for the preparation of the aqueous solutions used (Merck Millipore Simplicity™ UV water purification system).
2.1.2 Lipase activity assays
This study uses pure BMIMBF4 and phosphate buffer as the aqueous media, 50 mM and pH = 7.0, and BMIMBF4/buffer mixtures in a wide range of molar fractions (χ) with/without the presence of a surfactant derived from the same ionic liquid, C12-MIMBF4 (10 mM). Each mixture was prepared by weighting the proper amount of the IL and buffer in a screw-capped vial. To favor mixing, each mixture was shaken and sonicated for 1 min and then left to equilibrate overnight before use. In all cases, the mixtures appeared homogeneous after this treatment. In those mixtures with C12-BMIMBF4, it was added after to be shaken and sonicated.
The substrate solution (p-NPL) was prepared in DMSO at 58 mM, and it was directly injected (10 µL) in each reaction medium. Lipase activity was measured by UV–Vis spectrophotometry using an Agilent 8453 UV–Vis spectrometer. Aliquots from a stock solution (50 μL) of lipase were added to 2.5 mL of each reaction medium containing the p-NPL. The release of p-NP was recorded by following the increase in absorbance at 410 nm. The concentration of p-NP was determined from absorbance data using a calibration curve. The initial reaction rates were calculated during the first 150 s of the initial segment of the reaction profiles. The enzymatic solution was prepared by adding 10 mg by 1 mL of potassium phosphate buffer solution. From the plots of p-NP release vs. time obtained at different p-NLP concentrations while keeping the amount of CALB added to each kinetic experiment constant, the dependence of the initial reaction rates with p-NPL was established. The determination of the Michaelis–Menten kinetic parameters (
where
2.1.3 Critical micelle concentration determination
Conductivity measurements were used to evaluate the critical micelle concentration (CMC) of C12-MIMBF4 using an Adwa AD3000 conductometer provided with a 4-pole conductivity probe. The conductivity of water (buffer phosphate), BMIMBF4, and BMIMBF4/water mixtures was briefly measured upon adding some stock solution (c.a. 50–200 μL) of C12-MIMBF4 (100 mM), prepared in the corresponding solvent mixture. CMC values were determined at the breaking point observed in the plots of conductivity, expressed in mS/cm vs. [C12-MIMBF4] in all the ranges of molar fraction with respect to BMIMBF4 (see Supplementary Figures S1, S2 in electronic Supplementary Material) (Evans, 1956).
3 Results and discussion
In order to obtain useful kinetic information that can be compared with the data obtained in pure media and solvent mixtures, special emphasis was placed on the evaluation of the extent of the influence of the micellar aggregates at a fixed molar concentration of surfactant (10 mM). This concentration was used in order to ensure that the surfactant concentration is beyond the CMC, where the presence of micellar aggregates acquires relevance. Figure 1 shows the relationships between the variation in the CMC of C12-MIMBF4 and the molar fraction to respect to BMIMBF4 (
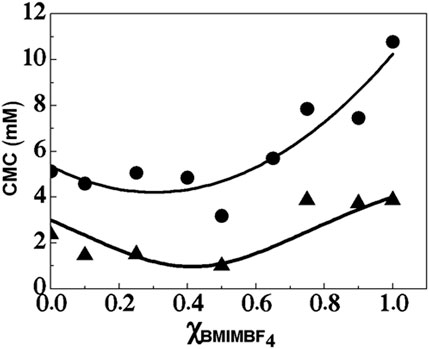
FIGURE 1. Variation in the critical micelle concentration (CMC) of C12-MIMBF4 with the molar fraction of BMIMBF4 in an aqueous solution, without p-NPL (•) and 0.58 mM p-NPL (▲).
Figure 1 shows two trends related to the variation in the CMC vs. IL content (in the presence and absence of the substrate), expressed as
Figure 2 shows the variation in the lipase-catalyzed reaction rate at different
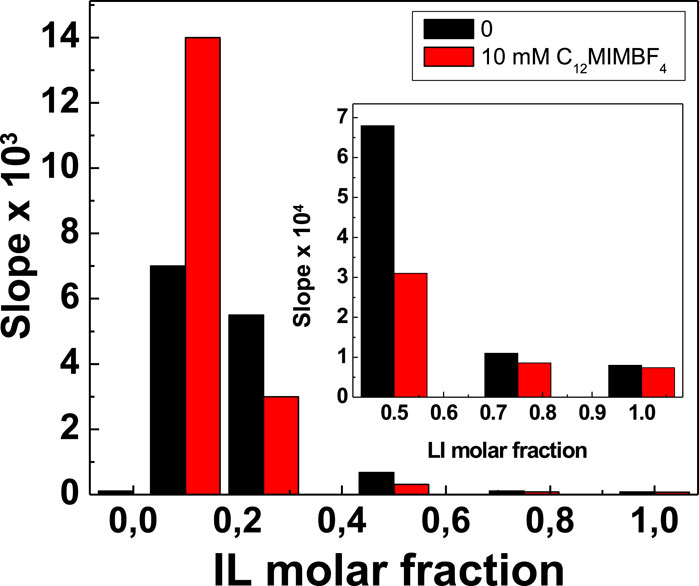
FIGURE 2. Reaction rate for the lipase-catalyzed decomposition of p-NPL as a function of the molar fraction of BMIMBF4 in an aqueous solution, without C12-MIMBF4 (▬) and 10 mM C12-MIMBF4 (▬). Inset: zoom of the data in the region of BMIMBF4 displaying lower CALB activity (region at
Figure 1 agrees with Figure 2 because the range of
Furthermore, Figure 2 shows two environments close to
Figure 3 shows the Michaelis–Menten kinetic parameters derived from the Lineweaver–Burk data analysis from the lipase activity assays (Viparelli et al., 1999; Biasutti et al., 2008; De Martino et al., 2018). The analyzed
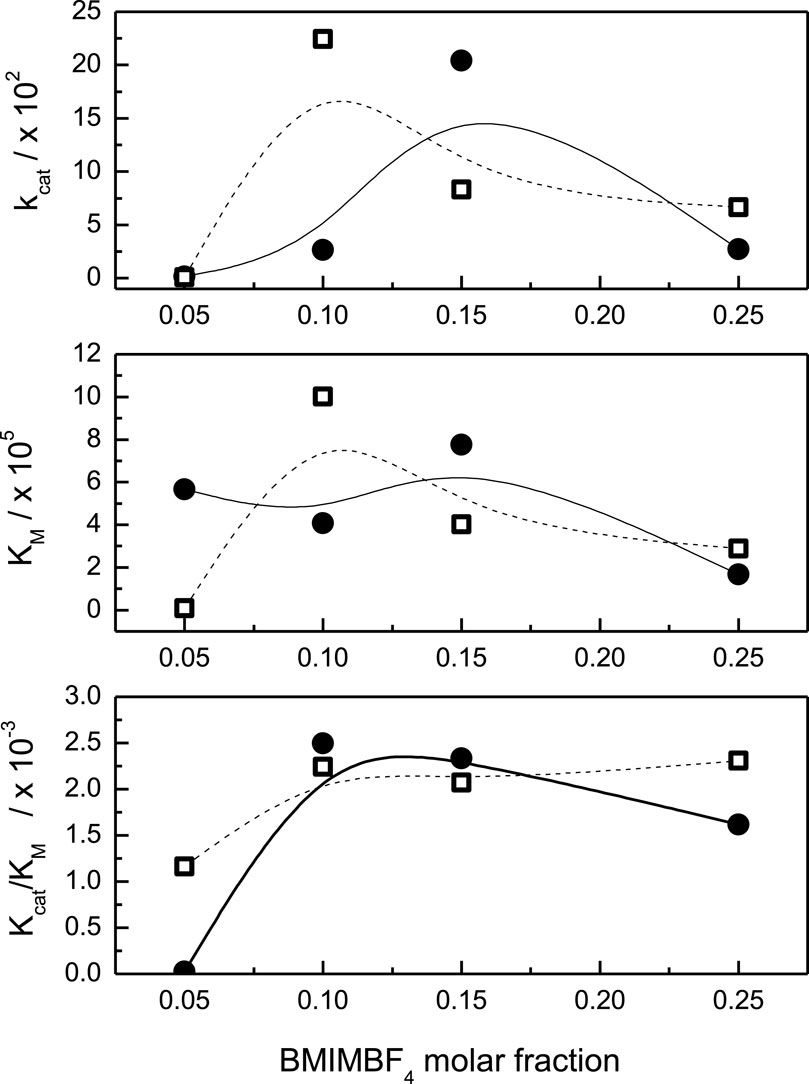
FIGURE 3. Michaelis–Menten parameters for the lipase-catalyzed solvolysis of p-NPL and its dependence on the molar fraction of BMIMBF4. Parameter calculated based on the data obtained in the presence and absence of the surfactant: no surfactant (•) and 10 mM C12-MIMBF4 (□).
Further inspection of the Michaelis–Menten catalytic parameters shows that there is a synergistic effect between BMIMBF4 and the imidazolium-based surfactant. All experimental conditions considered, a complete incorporation of the substrate into the micellar moiety can be expected, with the interfacial reaction taking place at the micelle/water interface, which appears to be enhanced by the presence of BMIMBF4. This is particularly interesting, considering that the influence of BMIMBF4 in the absence of the surfactant is regarded to have a moderate/high impact on the enzyme activity. Given that, in the presence of C12-MIMBF4, the studied lipase-catalyzed reaction requires the interaction of the enzyme with the micellar surface to have access to the substrate, there are at least three possible effects responsible for the observed phenomenon:
i) High substrate concentration and its incorporation into the micelles: The enzymatic reactions can take place in micellar environments; however, the occupation of the substrate and enzyme in the micelles is lower under the experimental condition considered. Moreover, BMIMBF4 can influence changes in the surfactant CMC values measured, which leads to changes in the concentration of micelles in the system (assuming a constant surfactant aggregation number), but these changes do not fully correlate with the observed catalytic behavior.
ii) Increased enzymatic activity due to enhanced micellar partition of the enzyme: Similar to i), an increase in the local concentration of the enzyme on the micellar surface might lead to increased activity. However, considering a low micellar occupancy of the substrate molecules, the impact of the increased enzyme concentration should be minimal.
iii) Enhanced lipase activity due to changes in the intrinsic nature of the micellar interface: According to the available data in the present work, particularly the determination of the counterion occupancy at the micellar interface, it can be proposed that the interaction between the enzyme and the micelles, taking place at the water/micelle interface, leads to a modification of the enzyme activity attributed to conformational changes of the enzyme. This is further enhanced by the presence of BMIMBF4, more specifically, by the BF4− anion, which is largely incorporated at the water/micelle interface.
In order to address these possible effects, particularly those related with interfacial changes (point iii) in the previous paragraph) taking place in the micellar moiety, i.e., the zone delimited by the interaction between the solvent and the headgroups of C12-MIMBF4, the counterion binding to the micellar surface was determined (Khalid et al., 2017). Figure 4 shows the counterion binding fraction (β) for micelles of C12-MIMBF4 as a function of
where α corresponds to the ratio between the slopes of the post- and pre-CMC segments of conductivity vs.
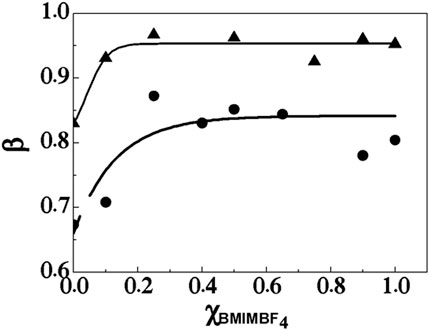
FIGURE 4. Counterion binding fraction (β) for micelles of C12-MIMBF4 as a function
Figure 4 shows differences in the micellar environment in the presence of the substrate (0.58 mM, full triangles). The β-values are at least 20% higher than without the presence of the substrate. This fact suggests that the substrate operates as a cosurfactant in the micellar environment. Then, the number of available adsorption sites for the incorporation of the surfactant counterions (BF4−) is improved. At
Some reports have associated the interfacial phenomenon influencing the interaction between the micelle-bound substrate and lipase with the potential distribution of substrate molecules among the population of micelles present under a given experimental condition (Huang et al., 2008; Wu et al., 2008). Figure 5 shows the estimated number of substrate molecules per micelles with respect to
where
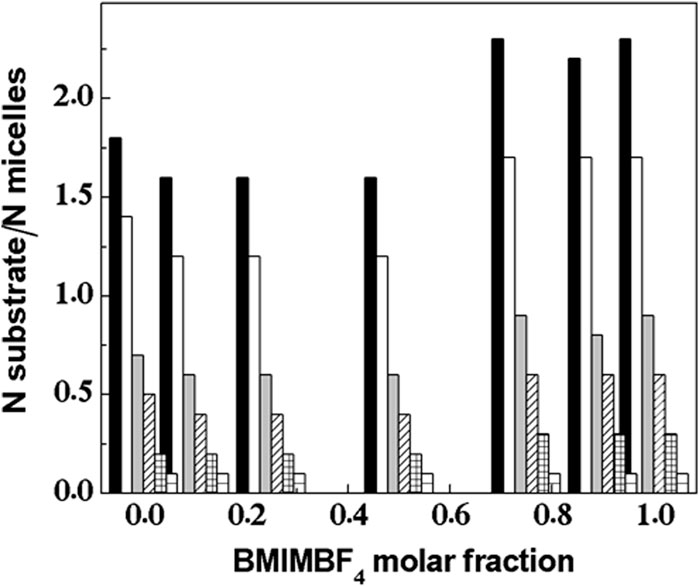
FIGURE 5. Estimated number of substrate molecules per micelle ratio as a function of BMIMBF4 content in the studied aqueous mixtures. Bars correspond to different substrate concentrations (left to right): 2.0 × 10−4 M; 1.5 × 10−4 M; 7.5 × 10−5 M; 5.0 × 10−5 M; 2.5 × 10−5 M; and 1.0 × 10−5 M (data are given in Supplementary Material).
Figure 5 shows an ideal scenario, where the substrate molecules are distributed as evenly as possible among the total number of micelles. Hence, the system is always dealing with low substrate occupancy in the micelles in the complete range of substrate concentrations considered. This indicates that the high efficiency achieved by the catalytic process takes place at the solvent/micelle interface, especially under the influence of BMIMBF4. On the other hand, the loss of activity observed with the increase in
As a plausible description of the aforementioned phenomena, Scheme 2 shows the effect of the micelles on the studied enzymatic reaction. Scheme 2 is a general picture that describes more easily the effect of the micelles on the enzymatic reaction. Scheme 2A shows the enzymatic reaction in water–BMIMBF4 mixtures. Schemes 2B shows the enzymatic reaction in micelles of C12-MIMBF4 in the presence of water–BMIMBF4 mixtures (low
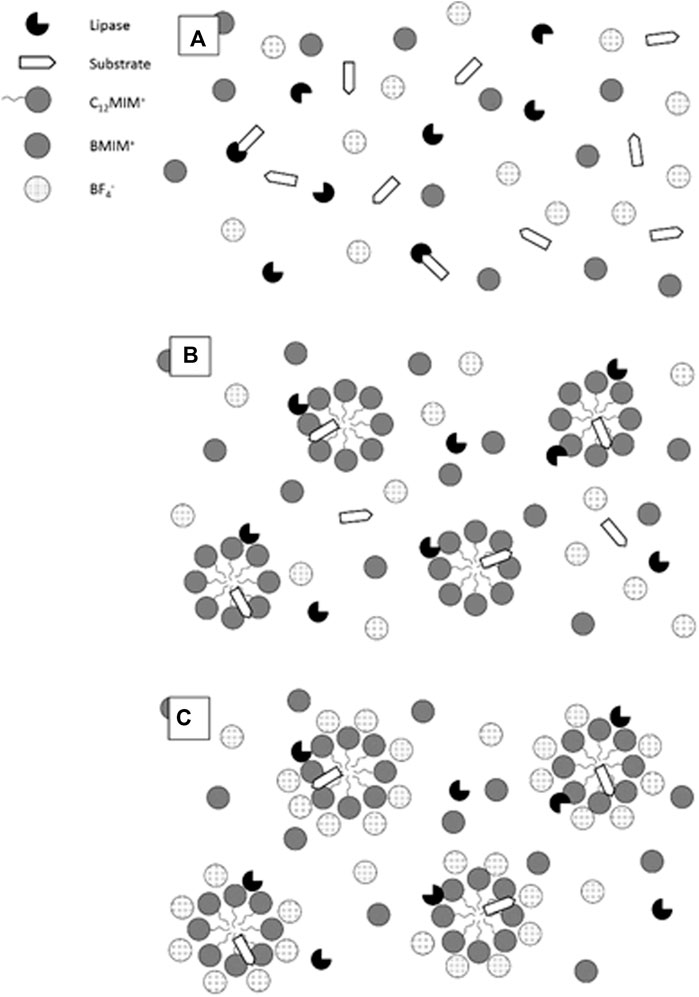
SCHEME 2. Depiction of the microenvironment for the lipase-catalyzed degradation of p-NPL. (A) Water/BMIMBF4 mixtures; (B) 10 mM C12-MIMBF4 in low-IL content water/BMIMBF4 mixtures; and (C) 10 mM C12-MIMBF4 in high-IL content water/BMIMBF4 mixtures.
Finally, one of the major limitations to the specific contributions made by the surfactant molecules in micellar aggregates lies in the fact that the concentration of micelles cannot be further increased without losing a significant amount of activity, attributable mainly to amounts of the enzyme that ends up adsorbed in micelles devoid of substrate molecules, hence lowering the effective concentration of the active enzyme in the system. Additionally, large micellar concentrations lead to changes in the aggregation number and geometry of the micelles, introducing further considerations to the overall phenomenon.
4 Conclusion
BMIMBF4 can increase the catalytic rate of CALB in the hydrolysis of the p-NPL reaction at low
Data availability statement
The original contributions presented in the study are included in the article/Supplementary Material; further inquiries can be directed to the corresponding author.
Author contributions
PC: conceptualization, data curation, formal analysis, funding acquisition, investigation, methodology, project administration, resources, supervision, validation, writing–original draft, and writing–review and editing. CC: conceptualization, data curation, formal analysis, investigation, methodology, validation, and writing–review and editing. JA: formal analysis, investigation, methodology, and writing–review and editing. BO: formal analysis, investigation, methodology, and writing–review and editing. LJ: formal analysis, investigation, methodology, and writing–review and editing. CS-R: formal analysis, investigation, methodology, and writing–review and editing.
Funding
The authors declare that no financial support was received for the research, authorship, and/or publication of this article.
Acknowledgments
PC acknowledges the Instituto de Ciencias e Innovación en Medicina (ICIM), Facultad de Medicina, Universidad del Desarrollo (UDD). LJ acknowledges the Doctorado en Ciencias e Innovación en Medicina, ICIM-UDD. JA acknowledges the Vicerectoría de Investigación y Doctorado (VRID) from ICIM-UDD.
Conflict of interest
The authors declare that the research was conducted in the absence of any commercial or financial relationships that could be construed as a potential conflict of interest.
Publisher’s note
All claims expressed in this article are solely those of the authors and do not necessarily represent those of their affiliated organizations, or those of the publisher, the editors, and the reviewers. Any product that may be evaluated in this article, or claim that may be made by its manufacturer, is not guaranteed or endorsed by the publisher.
Supplementary material
The Supplementary Material for this article can be found online at: https://www.frontiersin.org/articles/10.3389/fchem.2023.1289398/full#supplementary-material
References
Abe, Y., Kude, K., Hayase, S., Kawatsura, M., Tsunashima, K., and Itoh, T. (2008). Design of phosphonium ionic liquids for lipase-catalyzed transesterification. J. Mol. Catal. B Enzym. 51, 81–85. doi:10.1016/j.molcatb.2007.11.010
Alarcón-Espósito, J., Contreras, R., and Campodónico, P. R. (2017). Iso-solvation effects in mixtures of ionic liquids on the kinetics of a model SNAr reaction. New J. Chem. 41, 13435–13441. doi:10.1039/c7nj03246c
Alarcón-Espósito, J., Contreras, R., Tapia, R. A., and Campodónico, P. R. (2016). Gutmann's donor numbers correctly assess the effect of the solvent on the kinetics of SNAr reactions in ionic liquids. Chem. Eur. J. 22, 13347–13351. doi:10.1002/chem.201602237
Alarcón-Espósito, J., Tapia, R. A., Contreras, R., and Campodónico, P. R. (2015). Changes in the SN Ar reaction mechanism brought about by preferential solvation. RSC Adv. 5, 99322–99328. doi:10.1039/c5ra20779g
Amde, M., Liu, J.-F., and Pang, P. (2015). Environmental application, fate, effects, and concerns of ionic liquids. A review. Environ. Sci. Technol. 49, 12611–12627. doi:10.1021/acs.est.5b03123
Anderson, J. L., Ding, J., Welton, T., and Armstrong, D. W. (2002). Characterizing ionic liquids on the basis of multiple solvation interactions. J. Am. Chem. Soc. 124 (47), 14247–14254. doi:10.1021/ja028156h
Attri, P., Venkatesu, P., Kumar, A., and Byrne, N. (2011). A protic ionic liquid attenuates the deleterious actions of urea on α-chymotrypsin. Phys. Chem. Chem. Phys. 13, 17023–17026. doi:10.1039/c1cp22195g
Ballesteros, A., Bornscheuer, U., Capewell, A., Combes, D., Condoret, J.-S., Koenig, K., et al. (1995). Review article enzymes in non-conventional phases. Biocatal. Biotransformation 13, 1–42. doi:10.3109/10242429509040103
Bekhouche, M., Blum, L. J., and Doumèche, B. (2011). Ionic liquid-inspired cations covalently bound to formate dehydrogenase improve its stability and activity in ionic liquids. ChemCatChem 3, 875–882. doi:10.1002/cctc.201000390
Ben-Naim, A. (1990). Preferential solvation in two- and in three-component systems. Pure Appl. Chem. 62, 25–34. doi:10.1351/pac199062010025
Biasutti, M. A., Abuin, E. B., Silber, J. J., Correa, N. M., and Lissi, E. A. (2008). Kinetics of reactions catalyzed by enzymes in solutions of surfactants. Adv. Colloid Interface Sci. 136, 1–24. doi:10.1016/j.cis.2007.07.001
Calderón, C., Contreras, R., and Campodónico, R. (2019). Surfactant-mediated enzymatic superactivity in water/ionic liquid mixtures, evaluated on a model hydrolytic reaction catalyzed by α-chymotrypsin. J. Mol. Liq. 283, 522–531. doi:10.1016/j.molliq.2019.03.106
Cammarata, L., Kazarian, S. G., Salter, P. A., and Welton, T. (2001). Molecular states of water in room temperature ionic liquidsElectronic Supplementary Information available. Phys. Chem. Chem. Phys. 3, 5192–5200. doi:10.1039/B106900D
Carrea, G., and Riva, S. (2008). Organic synthesis with enzymes in non-aqueous media. Jhon Wiley&Sons.
Cheong, L.-Z., Mou, B., Wei, W., Hongli, Y., Hai, Z., Gege, Z., et al. (2022). “Ionic liquid as a green solvent for lipid processing,” in Recent advances in edible fats and oils Technology Y. Lee, T. K. Tang, Phuah, and O. M. Lai (Singapore: Springer). doi:10.1007/978-981-16-5113-7_7
Chiappe, C., Pieraccini, D., and Pomelli, C. S. (2007). The interactions affecting organic reactivity and selectivity in ionic liquids. ACS Symp. Ser. 1, 1–15. –15. doi:10.1021/bk-2007-0950.ch001
Constantinescu, D., Weingärtner, H., and Herrmann, C. (2007). Protein denaturation by ionic liquids and the Hofmeister series: a case study of aqueous solutions of ribonuclease A. Angew. Chem. Int. Ed. Engl. 46, 8887–8889. doi:10.1002/anie.200702295
Danna, F., and Harper, J. (2019). Ionic liquids: properties and applications. Frontiers Media SA. Available at: https://play.google.com/store/books/details?id=zxi3DwAAQBAJ.
de Gonzalo, G., Lavandera, I., Durchschein, K., Wurm, D., Faber, K., and Kroutil, W. (2007). Asymmetric biocatalytic reduction of ketones using hydroxy-functionalised water-miscible ionic liquids as solvents. Tetrahedron Asymmetry 18, 2541–2546. doi:10.1016/j.tetasy.2007.10.010
De Martino, M. T., Abdelmohsen, L. K. E. A., Rutjes, F. P. J. T., and van Hest, J. C. M. (2018). Nanoreactors for green catalysis. Beilstein J. Org. Chem. 14, 716–733. doi:10.3762/bjoc.14.61
Dolman, M., Halling, P. J., Moore, B. D., and Waldron, S. (1997). How dry are anhydrous enzymes? Measurement of residual and buried 18O-labeled water molecules using mass spectrometry. Biopolymers 41, 313–321. doi:10.1002/(sici)1097-0282(199703)41:3<313::aid-bip6>3.0.co;2-v
Eckstein, M., Sesing, M., Kragl, U., and Adlercreutz, P. (2002). At low water activity α-chymotrypsin is more active in an ionic liquid than in non-ionic organic solvents. Biotechnol. Lett. 24, 867–872. doi:10.1023/A:1015564608261
Elaiwi, A., Hitchcock, P. B., Seddon, K. R., Srinivasan, N., Tan, Y.-M., Welton, T., et al. (1995). Hydrogen bonding in imidazolium salts and its implications for ambient-temperature halogenoaluminate(III) ionic liquids. J. Chem. Soc. Dalton Trans. 3467, 3467. doi:10.1039/dt9950003467
Evans, H. C. (1956). 117. Alkyl sulphates. Part I. Critical micelle concentrations of the sodium salts. J. Chem. Soc., 579–586. doi:10.1039/JR9560000579
Fan, Y., Dong, X., Li, X., Zhong, Y., Kong, J., Hua, S., et al. (2016). Spectroscopic studies on the inhibitory effects of ionic liquids on lipase activity. Spectrochimica Acta Part A Mol. Biomol. Spectrosc. 159, 128–133. doi:10.1016/j.saa.2016.01.047
Fazio, B., Triolo, A., and Di Marco, G. (2008). Local organization of water and its effect on the structural heterogeneities in room-temperature ionic liquid/H2 O mixtures. J. Raman Spectrosc. 39, 233–237. doi:10.1002/jrs.1825
Fojan, P., Jonson, P. H., Petersen, M. T. N., and Petersen, S. B. (2000). What distinguishes an esterase from a lipase: a novel structural approach. Biochimie 82, 1033–1041. doi:10.1016/S0300-9084(00)01188-3
Freemantle, M. (1998). DESIGNER SOLVENTS: ionic liquids may boost clean technology development. Chem. Eng. News. 76 (13), 32–37. doi:10.1021/cen-v076n013.p032
Gonzalves, A. R. P., Paredes, X., Cristino, A. F., Santos, F. J. V., and Queirós, C. S. G. P. (2021). Ionic-liquids-a review of their toxicity to living organism. Int. J. Mol. Sci. 22, 5612–5662. doi:10.3390/ijms22115612
Gupta, M. N. (1992). Enzyme function in organic solvents. Eur. J. Biochem. 203, 25–32. doi:10.1111/j.1432-1033.1992.tb19823.x
Halling, P. J. (1994). Thermodynamic predictions for biocatalysis in nonconventional media: theory, tests, and recommendations for experimental design and analysis. Enzyme Microb. Technol. 16, 178–206. doi:10.1016/0141-0229(94)90043-4
Huang, H., Han, Y., Wang, Y., Cao, M., and Wang, Y. (2008). Aggregation properties of cationic gemini surfactants with dihydroxyethylamino headgroups in aqueous solution. Colloids ans Surfaces A Physicochem. Eng. Asp. 325, 26–32. doi:10.1016/j.colsurfa.2008.04.028
Itoh, T. (2017). Ionic Liquids as tool to improve enzymatic organic synthesis. Chem. Rev. 117, 10567–10607. doi:10.1021/acs.chemrev.7b00158
Kamlet, M. J., Abboud, J. L., and Taft, R. W. (1977). The solvatochromic comparison method. 6. The.pi.* scale of solvent polarities. J. Am. Chem. Soc. 99, 6027–6038. doi:10.1021/ja00460a031
Kamlet, M. J., Abboud, J. L. M., Abraham, M. H., and Taft, R. W. (1983). Linear solvation energy relationships. 23. A comprehensive collection of the solvatochromic parameters, pi.*, alpha., and.beta., and some methods for simplifying the generalized solvatochromic equation. J. Org. Chem. 48, 2877–2887. doi:10.1021/jo00165a018
Kamlet, M. J., and Taft, R. W. (1976). The solvatochromic comparison method. I. The.beta.-scale of solvent hydrogen-bond acceptor (HBA) basicities. J. Am. Chem. Soc. 98, 377–383. doi:10.1021/ja00418a009
Khalid, K., Noh, M. A. M., Zain, S. M., and Khan, M. N. (2017). Determination of relative counterion binding constant to cationic micelles. Top. Curr. Chem. (Z) 375, 45. doi:10.1007/s41061-017-0132-9
Khmelnitsky, Y. L., Welch, S. H., Clark, D. S., and Dordick, J. S. (1994). Salts dramatically enhance activity of enzymes suspended in organic solvents. J. Am. Chem. Soc. 116, 2647–2648. doi:10.1021/ja00085a066
Klahn, M., Lim, G. S., Seduraman, A., and Wu, P. (2011). On the different roles of anions and cations in the solvation of enzymes in ionic liquids. Phys. Chem. Chem. Phys. 13, 1649–1662. doi:10.1039/C0CP01509A
Klibanov, A. M. (1997). Why are enzymes less active in organic solvents than in water? TIBTECH 15, 97–101. doi:10.1016/S0167-7799(97)01013-5
Kragl, U., Eckstein, M., and Kaftzik, N. (2002). Enzyme catalysis in ionic liquids. Curr. Opin. Biotechnol. 13, 565–571. doi:10.1016/s0958-1669(02)00353-1
Laszlo, J. A., and Compton, D. L. (2001). α-Chymotrypsin catalysis in imidazolium-based ionic liquids. Biotechnol. Bioeng. 75, 181–186. doi:10.1002/bit.1177
Lau, R. M., Sorgedrager, M. J., Carrea, G., van Rantwijk, F., Secundo, F., and Sheldon, R. A. (2004). Dissolution of Candida Antarctica lipase B in ionic liquids: effects on structure and activity. Green Chem. 6, 483–487. doi:10.1039/B405693K
Lau, R. M., Van, R. F., Seddon, K. R., and Sheldon, R. A. (2000). Lipase-catalyzed reactions in ionic liquids. Org. Lett. 2 (26), 4189–4191. doi:10.1021/ol006732d
Łuczak, J., Latowska, A., and Hupka, J. (2015). Micelle formation of Tween 20 nonionic surfactant in imidazolium ionic liquids. Colloids Surfaces A Physicochem. Eng. Aspects 471, 26–37. doi:10.1016/j.colsurfa.2015.02.008
Matteis, L. D., De Matteis, L., Di Renzo, F., Germani, R., Goracci, L., Spreti, N., et al. (2016). α-Chymotrypsin superactivity in quaternary ammonium salt solution: kinetic and computational studies. RSC Adv. 6, 46202–46211. doi:10.1039/c6ra07425a
Micaêlo, N. M., and Soares, C. M. (2008). Protein structure and dynamics in ionic liquids. Insights from molecular dynamics simulation studies. J. Phys. Chem. B 112, 2566–2572. doi:10.1021/jp0766050
Migowski, P., Lozano, P., and Dupont, J. (2023). Imidazolium based ionic liquid-phase green catalytic reactions. Green Chem. 25, 1237–1260. doi:10.1039/D2GC04749G
Pernak, J., Rogoza, J., and Mirska, I. (2001). Synthesis and antimicrobial activities of new pyridinium and benzimidazolium chlorides. J. Med. Chem. 36, 313–320. doi:10.1016/s0223-5234(01)01226-0
Rabbani, G., Ahmad, E., Khan, M. V., Ashraf, M. T., Bhat, R., and Khan, R. H. (2015). Impact of structural stability of cold adapted Candida Antarctica lipase B (CaLB): in relation to pH, chemical and thermal denaturation. RSC Adv. 5, 20115–20131. doi:10.1039/C4RA17093H
Reichardt, C., and Welton, T. (2011). Solvents and solvent effects in organic chemistry. Wiley-VCH Verlang GmbH&Co. KGaA.
Sánchez, B., Calderón, C., Garrido, C., Contreras, R., and Campodónico, P. R. (2018a). Solvent effect on a model SNAr reaction in ionic liquid/water mixtures at different compositions. New J. Chem. 42, 9645–9650. doi:10.1039/c7nj04820c
Sánchez, B., Calderón, C., Garrido, C., Contreras, R., and Campodónico, P. R. (2018c). Solvent effect on a model SN Ar reaction in ionic liquid/water mixtures at different compositions. New J. Chem. 42, 9645–9650. doi:10.1039/C7NJ04820C
Sánchez, B., Calderón, C., Tapia, R. A., Contreras, R., and Campodónico, P. R. (2018b). Activation of electrophile/nucleophile pair by a nucleophilic and electrophilic solvation in a SNAr reaction. Front. Chem. 6, 509. doi:10.3389/fchem.2018.00509
Schomburg, D., and Salzmann, M. (1991). “Oligonucleotidase,” in Enzyme handbook 3: class 3: hydrolases. Editors D. Schomburg, and M. Salzmann (Berlin, Heidelberg: Springer Berlin Heidelberg), 765–768. doi:10.1007/978-3-642-76463-9_162
Seddon, K. R., Torres, M. J., and Annegret, S. (2000). Influence of chloride, water, and organic solvents on the physical properties of ionic liquids. Pure Appl. Chem. 72, 2275–2287. doi:10.1351/pac200072122275
Sheldon, R. A. (1997). Catalysis: the key to waste minimization. J. Chem. Technol. Biotechnol. 68, 381–388. doi:10.1002/(sici)1097-4660(199704)68:4<381::aid-jctb620>3.0.co;2-3
Sheldon, R. A. (2000). Atom utilisation, E factors and the catalytic solution. Comptes Rendus l’Académie Sci. - Series IIC - Chemistry 3, 541–551. doi:10.1016/S1387-1609(00)01174-9
Sheldon, R. A. (2016). Biocatalysis and biomass conversion in alternative reaction media. Chemistry 22, 12984–12999. doi:10.1002/chem.201601940
Sheldon, R. A., Arends, I., and Hanefeld, U. (2007). Green chemistry and catalysis. John Wiley&Sons. Verlog GmbH&Co. KGaA. doi:10.1002/9783527611003
Sheldon, R. A., Lau, R. M., Sorgedrager, M. J., van Rantwijk, F., and Seddon, K. R. (2002). Biocatalysis in ionic liquids. Green Chem. 4, 147–151. doi:10.1039/B110008B
Sheldon, R. A., and Woodley, J. M. (2018). Role of biocatalysis in sustainable chemistry. Chem. Rev. 118, 801–838. doi:10.1021/acs.chemrev.7b00203
Sintra, T. E., Ventura, S. P. M., and Coutinho, J. A. P. (2014). Superactivity induced by micellar systems as the key for boosting the yield of enzymatic reactions. J. Mol. Catal. B Enzym. 107, 140–151. doi:10.1016/j.molcatb.2014.06.001
Spreti, N., Alfani, F., Cantarella, M., D’Amico, F., Germani, R., and Savelli, G. (1999). α-Chymotrypsin superactivity in aqueous solutions of cationic surfactants. J. Mol. Catal. B Enzym. 6, 99–110. doi:10.1016/s1381-1177(98)00139-8
Stamatis, H., Xenakis, A., and Kolisis, F. N. (1999). Bioorganic reactions in microemulsions: the case of lipases. Biotechnol. Adv. 17, 293–318. doi:10.1016/s0734-9750(99)00007-5
Van Rantwijk, F., and Sheldon, R. A. (2007). Biocatalysis in ionic liquids. Chem. Rev. 107, 2757–2785. doi:10.1021/cr050946x
Vekariya, R. L. (2017). A review of ionic liquids: applications towards catalytic organic transformations. J. Mol. Liq. 227, 44–60. doi:10.1016/j.molliq.2016.11.123
Ventura, S. P. M., Santos, L. D. F., Saraiva, J. A., and Coutinho, J. A. P. (2012). Ionic liquids microemulsions: the key to Candida Antarctica lipase B superactivity. Green Chem. 14, 1620–1625. doi:10.1039/C2GC35197H
Vicent-Luna, J. M., Romero-Enrique, J. M., Calero, S., and Anta, J. A. (2017). Micelle Formation in aqueous solutions of room temperature ionic liquids: a molecular dynamics study. J. Phys. Chem. B 121, 8348–8358. doi:10.1021/acs.jpcb.7b05552
Viparelli, P., Alfani, F., and Cantarella, M. (1999). Models for enzyme superactivity in aqueous solutions of surfactants. Biochem. J. 344 (3), 765–773. doi:10.1042/bj3440765
Weingärtner, H. (2008). Understanding ionic liquids at the molecular level: facts, problems, and controversies. Angew. Chem. Int. Ed. Engl. 47, 654–670. doi:10.1002/anie.200604951
Welton, T. (1999). Room-temperature ionic liquids. Solvents for synthesis and catalysis. Chem. Rev. 99, 2071–2084. doi:10.1021/cr980032t
Wijaya, E. C., Separovic, F., Drummond, C. J., and Greaves, T. L. (2016). Micelle formation of a non-ionic surfactant in non-aqueous molecular solvents and protic ionic liquids (PILs). Phys. Chem. Chem. Phys. 18, 24377–24386. doi:10.1039/c6cp03332f
Wu, J., Li, N., Zheng, L., Li, X., Gao, Y., and Inoue, T. (2008). Aggregation behavior of polyoxyethylene (20) sorbitan monolaurate (tween 20) in imidazolium based ionic liquids. Langmuir 24, 9314–9322. doi:10.1021/la801358z
Wu, Q., Soni, P., and Reetz, M. T. (2013). Laboratory evolution of enantiocomplementary Candida Antarctica lipase B mutants with broad substrate scope. J. Am. Chem. Soc. 135, 1872–1881. doi:10.1021/ja310455t
Xanthakis, E., Zarevúcka, M., Šaman, D., Wimmerová, M., Kolisis, F. N., and Wimmer, Z. (2006). Application of ionic liquids in enzymic resolution by hydrolysis of cycloalkyl acetates. Tetrahedron Asymmetry 17, 2987–2992. doi:10.1016/j.tetasy.2006.10.045
Xu, P., Zheng, G.-W., Zong, M.-H., Li, N., and Lou, W.-Y. (2017). Recent progress on deep eutectic solvents in biocatalysis. Bioresour. Bioprocess 4, 34. doi:10.1186/s40643-017-0165-5
Xue, Y., Zhang, X.-G., Lu, Z.-P., Xu, C., Xu, H.-J., and Hu, Y. (2022). Enhancing the catalytic performance of Candida Antarctica lipase B by chemical modification with alkylated betaine ionic liquids. Front. Bioeng. Biotechnol. 10, 850890. doi:10.3389/fbioe.2022.850890
Yang, Z. (2009). Hofmeister effects: an explanation for the impact of ionic liquids on biocatalysis. J. Biotechnol. 144, 12–22. doi:10.1016/j.jbiotec.2009.04.011
Keywords: ionic liquids, enzyme, surfactant, catalysis, superactivity
Citation: Campodónico PR, Calderón C, Alcázar JJ, Olivares B, Jaldin L and Suárez-Rozas C (2024) Exploring the behavior of Candida antarctica lipase B in aqueous mixtures of an imidazolium ionic liquid and its surfactant analogue. Front. Chem. 11:1289398. doi: 10.3389/fchem.2023.1289398
Received: 05 September 2023; Accepted: 15 December 2023;
Published: 10 January 2024.
Edited by:
Maria Manuel Marques, Universidade Nova de Lisboa, PortugalReviewed by:
Abbul Bashar Khan, Jamia Millia Islamia, IndiaGiorgio Tofani, National Institute of Chemistry, Slovenia
Copyright © 2024 Campodónico, Calderón, Alcázar, Olivares, Jaldin and Suárez-Rozas. This is an open-access article distributed under the terms of the Creative Commons Attribution License (CC BY). The use, distribution or reproduction in other forums is permitted, provided the original author(s) and the copyright owner(s) are credited and that the original publication in this journal is cited, in accordance with accepted academic practice. No use, distribution or reproduction is permitted which does not comply with these terms.
*Correspondence: Paola R. Campodónico, cGNhbXBvZG9uaWNvQHVkZC5jbA==