- 1AGH University of Science and Technology, Krakow, Poland
- 2Department of Biomedical Engineering, Hebei University of Technology, Tianjin, China
- 3School of Engineering, Saveetha University, Chennai, India
- 4Program in Materials Science, UFPI, Teresina, Brazil
Cardiovascular diseases bear strong socioeconomic and ecological impact on the worldwide healthcare system. A large consumption of goods, use of polymer-based cardiovascular biomaterials, and long hospitalization times add up to an extensive carbon footprint on the environment often turning out to be ineffective at healing such cardiovascular diseases. On the other hand, cardiac cell toxicity is among the most severe but common side effect of drugs used to treat numerous diseases from COVID-19 to diabetes, often resulting in the withdrawal of such pharmaceuticals from the market. Currently, most patients that have suffered from cardiovascular disease will never fully recover. All of these factors further contribute to the extensive negative toll pharmaceutical, biotechnological, and biomedical companies have on the environment. Hence, there is a dire need to develop new environmentally-friendly strategies that on the one hand would promise cardiac tissue regeneration after damage and on the other hand would offer solutions for the fast screening of drugs to ensure that they do not cause cardiovascular toxicity. Importantly, both require one thing–a mature, functioning cardiac tissue that can be fabricated in a fast, reliable, and repeatable manner from environmentally friendly biomaterials in the lab. This is not an easy task to complete as numerous approaches have been undertaken, separately and combined, to achieve it. This review gathers such strategies and provides insights into which succeed or fail and what is needed for the field of environmentally-friendly cardiac tissue engineering to prosper.
1 Introduction
Cardiovascular diseases (CVDs) are some of the most common death-causing diseases, culminating into roughly 18 million deaths per year. 85% of these cases are due to stroke or myocardial infraction (World Health Organization, 2017). These conditions almost always require lengthy hospitalization stays, which is connected to the significant use of energy and resources, meaning that the healing process itself has a significant economic and ecological impact. As just one of many CVD examples, myocardial infraction survivors will suffer from mild to severe heart tissue damage within a few hours after the infraction and insufficient blood supply may kill up to 25% of cardiomyocytes with normal cardiac tissue irreversibly replaced by scar tissue because of the heart’s low tissue regenerative potential (Laflamme and Murry, 2011; Maher, 2013). This leads to changes in the heart’s mechanical properties–the tissue becomes stiffer and so the muscles need to perform more work to produce the same contraction, leading to reduced efficiency of this vital organ (Laflamme and Murry, 2011; Tzahor and Poss, 2017). As a result, a large share of patients who have suffered from myocardial infraction may never go back to functioning the way they used to before the incident. The overall deterioration of life quality is not the only downside of CVDs as such a state of matters also poses a large socioeconomic issue. Many patients will be permanently placed on drugs or have some sort of medical device implanted (such as pacemaker or stent derived from non-environmentally friendly materials), further increasing the environmental carbon footprint resulting from CVDs. Hence, although not discussed frequently enough, there is a large amount of socioeconomic and ecological pressure to design environmentally-friendly strategies for healthy cardiac tissue regeneration.
The negative environmental impact of developing and using biomaterials (including those for cardiac tissue regeneration) cannot be understated. For example, none of the polymers currently FDA approved as biomaterials are considered environmentally-friendly and all leave a large carbon footprint either during manufacturing or use. Further, with only 16% of all polymers recycled today, there is a growing concern over greenhouse gases emitted during polymer synthesis which is expected to increase from 850 metric tons in 2019 to 2.8 gigatons in 2050. Apart from this, there is also a dire need to develop fast and reliable screening strategies that could fast track drug approval and treatment strategies from the lab into the market, while reducing the environmental impact that comes from laboratory animal testing, in vitro screening, and associated costs. It is worth noting that toxicity towards the heart is one of the most serious side effects of numerus drugs from fighting COVID-19 to diabetes, which attributes to roughly 20% of pharmaceuticals being withdrawn from the market (Siramshetty et al., 2015; Onakpoya et al., 2016). Collectively, all of these issues point to a significant environmental crisis with current CVD research and products. To date, many researchers have focused on saving human health through the design of improved CVD biomaterials but have omitted the loss of human life that results from environmental damage.
Critical to future CVD biomaterial solutions involve developing green biomaterials as well as the use of cells. Because there is no stem cell niche in the heart that would allow recruitment of new cardiomyocytes (Karbassi et al., 2020), cardiac tissue does not self-regenerate (Laflamme and Murry, 2011). Various tissue-engineering strategies have been suggested to foster heart healing, and some of the most common assume that new cardiomyocytes (or their progenitors) need to be delivered to a scarred tissue site, resulting in its remuscularization. In this approach, it is envisioned that the newly delivered cells will become integrated into the target tissue, followed by new tissue formation, thus inducing the remuscularization process. Cells can be delivered by direct injection, inside the cell sheets, or growing within the 3D scaffolds. These 3 approaches are graphically depicted in Figure 1.
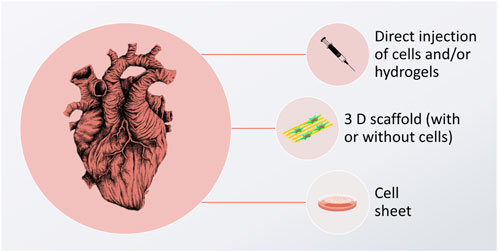
FIGURE 1. Graphic depiction of cardiomyocyte delivery approaches, aimed to induce cardiac tissue regeneration. The image of the heart is adapted from “ink heart” by Julia Bramer, CC BY-NC 4.0, graphical scheme designed by TinyPPT.com and the cell sheet is reprinted from Yamato et al. (2007) with permission form Elsevier, Copyright 2007.
While direct injection of cells is the simplest approach with a low impact on the environment, it has little chance of encouraging the cells to stay at the desired diseased place, resulting in low cellular retention (<1%), which often causes this strategy to be ineffective. Cell injections have also been reported to cause arrythmia (Zimmermann, 2020) and they do not come with the support from other cells, such as cardiac fibroblasts, which are known to play a crucial part in guaranteeing proper cardiomyocyte function (Fujita and Zimmermann, 2017; Zimmermann, 2020). One way to circumvent this is injecting cells with supportive gel-forming biomaterials. These biomaterials are liquid when mixed with cells and gel upon delivery to the target area. While this strategy forces the cells to stay in the designated damaged area of the heart, it is limited to treating small defects only; as such implantable biomaterials do not have sufficient mechanical strength, or complex architecture to temporarily substitute for the function of the damaged tissue.
Another approach is to fabricate a cell sheet construct–a cellular co-culture growing in an extra-cellular matrix (ECM) synthesized by the cells themselves (Yamato et al., 2007). Here, the main limitation lies in the fact that these constructs are hard to handle, and their implantation may be troublesome, especially when large defects are to be treated. It is also difficult to simulate the mechanical, physicochemical, and electrical properties of the heart itself in such constructs.
A third option involves using a scaffold that is tailored to mimic the native ECM, while still providing a satisfactory shape and size stability. Preferably, such scaffolds would be made of “green materials”, i.e., derived from natural, renewable sources, such as plants or animal tissues, synthesized by microorganisms (bacteria or fungi), or reconstituted from different industry by-products (such as the food industry). On such scaffolds, various cell types can be seeded concurrently. Additionally, various biofunctional cues can be introduced to enhance cellular proliferation and differentiation into cardiomyocytes. In this approach, cells are cultured on the scaffold for a given amount of time, until they reach the desired differentiation and maturation state, at which point epicardial implantation can be performed. Such 3D scaffolds are favorable in that they may not only be used for the creation of CVD implants, but they can also serve as in vitro drug screening platforms in the lab. By using such artificial tissue constructs, the length and cost of new drug development can be reduced. At the same time, the extensive environmental impact of conducting traditional in vitro and in vivo studies can be mitigated.
It is generally acknowledged that these man-made constructs should be able to replicate the cellular natural environment as closely as possible. Then, the cells behave as they would within the natural healthy heart, reconstituting healthy native tissue. Hence, the scaffold should bear characteristics similar to functional tissues. For this reason, a set of physicochemical properties of the healthy myocardium is presented in Table 1.
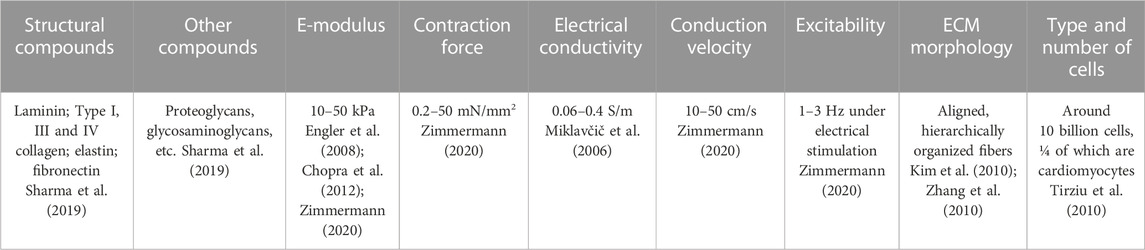
TABLE 1. Chemical composition and basic physicochemical properties of the native healthy cardiac tissue.
This review will provide some necessary background information needed to understand the subject, but the main focus will be the evaluation of some recent cardiac tissue engineering publications with special emphasis placed on using biopolymers and green-derived biomaterials, combined with specifically designed biofunctional cues. The most advanced strategies that can be combined to obtain multifunctional, biomimetic scaffolds are gathered, providing valid information about recent trends and directions into which the field is evolving to both save human health and the environment.
2 Cell sources
A successful green cardiac tissue engineering strategy consists of two, equally important components: a properly selected, biofunctional and biomimetic environmentally-friendly scaffold and an accurate cell source. Additionally, exogenous stimuli can be employed. Because the optimal goal is to obtain cardiomyocytes of an adult phenotype, preferably autologous, stem cells are the go-to candidates. A graph representing an evolution of cardiac tissue regeneration strategies from the cells’ perspective is given in Figure 2.
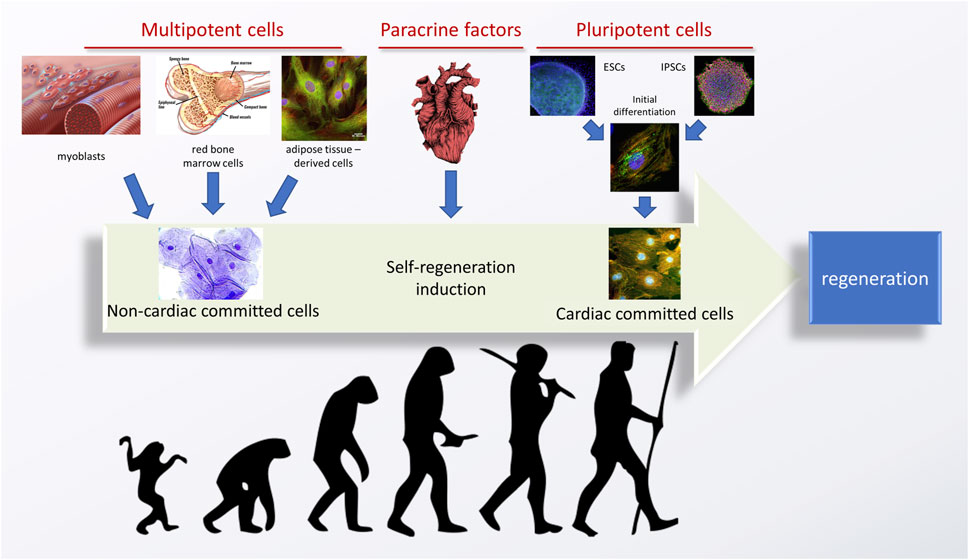
FIGURE 2. An evolution of stem cell-based strategies for tissue engineering of the heart. Adapted from (Silvestre and Menasché, 2015), using the images and graphs reproduced from an open source library—Flickr.com. Image of the heart is adapted from “ink heart” by Julia Bramer. All is reprinted under the CC BY-NC-ND 4.0 license.
Historically, the first attempts of inducing cardiac tissue regeneration revolved around multipotent (or progenitor) cells, obtained from the stem cell niches of an adult organism. These most often included bone marrow or muscle (satellite) cells, but can also include adipose tissue. While multipotent cells have a relatively high differentiation potential, they can only differentiate into closely related cells, from the same tissue type, and their proliferation potency is also lower than that of the pluripotent cells. Such therapies were able to induce a certain therapeutic effect–for example, the cells derived from bone marrow could differentiate into fibroblasts, which are cardiomyocyte supporting cells in the heart, leading to an improvement of some of the heart functions. Still, this effect was not permanent and complete regeneration of the heart was elusive because no new cardiomyocytes could be formed or recruited.
An obvious alternative was to use multipotent cardiac stem cells, obtained from the cardiac niche. In two different approaches, either the niche was identified based on cell harvesting and in vitro culturing, or paracrine factors were delivered into the heart with an aim to activate the already existing cells for in vivo regeneration. Despite the obvious logic behind this reasoning, good therapeutic effects were never obtained, and the cardiac stem cell niche was never found. Currently, it is believed that the cardiac stem cell niche is non-existent (Karbassi et al., 2020), with an extremely low regenerative potential of the heart being an obvious proof-of-concept.
A third possible source of cardiac cells are pluripotent cells. These can be obtained either from embryos (ESCs, which raises ethical concerns and is a very limited source) or induced by genetic reprogramming from somatic cells–these are called induced pluripotent stem cells (iPSCs) (Silvestre and Menasché, 2015; Zimmermann, 2020). Important advantages of using iPSCs over other cell sources are their unlimited source and ability to obtain patient-specific cardiomyocytes. The latter becomes favorable when considering tissue engineering strategies with a reduced rejection risk, as well as designing strategies for the in vitro evaluation of various genetic diseases (Martyniak et al., 2023). Pluripotent cells can be subjected to initial differentiation, which already has been proven to yield cardiomyocytes in vitro. However, these cells possess a juvenile phenotype, not able to perform as the adult cells would. Thus, their practical application is still elusive and efficient strategies to boost the cells’ maturation are needed. As various studies have proven, certain stimuli can aid this effect. One of the approaches suggests aggregating cells into specific organoids (Mills et al., 2019), but it has not been proven that this strategy significantly speeds up their maturation. Still, some increase in cardiomyocyte functionality has been reported when these cells are co-cultured with other cells (Varzideh et al., 2019).
Some very recent and promising approaches used to enhance the maturation of iPSCs-derived cardiomyocytes (iPSCs-CMs) are gathered in a review by Martyniak et al. (Martyniak et al., 2023). This excellent study provides a detailed description of the natural cardio genesis, lists important hallmarks of mature cardiomyocytes, as wells as gives validated protocols enhancing cell maturation efficacy in vitro. The readers are kindly referred to this review for a more extensive evaluation of various biochemical, electrical, and mechanical stimulants useful for promoting cardiomyocyte function. Instead, this article will focus mostly on strategies employed for the design, synthesis, and characterization of environmentally-friendly CVD biomaterials. Biomaterials serve as a critical support for cellular adhesion forcing the cells into specific desirable shape. Specific biomaterial morphology, chemical composition, and alignment can all enhance cellular maturation. These effects can be further boosted by selecting proper mechanical, electrical or biological cues. In the following sections, different types of available cues and/or biofunctional properties are listed, together with some exemplary cardiac tissue regeneration results, as reported in recent studies.
3 Co-cultures
Another important aspect of growing stem cells (such as derived CMs) in vitro is to understand what happens naturally during cardiac tissue development in vivo. The heart is composed of multiple cell types, fibroblasts being the second most abundant. Their main role is remodeling of the ECM and secreting signaling molecules that alter CM metabolism–from proliferation through maturation and growth (hypertrophy) (Li et al., 2017a; Karbassi et al., 2020; Martyniak et al., 2023). This clearly indicates that it might be very difficult, if not impossible, to obtain CMs of a mature phenotype in the absence of fibroblasts. But conducting such in vitro cultures is troublesome as fibroblasts proliferate much faster than CMs and can easily overtake a CM culture. Hence, different strategies have been formed to isolate the cells from one another, while still allowing the cells to exchange extracellular molecules. Some examples wherein two cell types are cultured in transwell inserts or where media is exchanged between the two separate cultures are reported throughout the literature, yielding positive outcomes (several examples are gathered in Table 2). Another possibility that has been reported in the literature is to fabricate a specific culture chamber/device, most often based on microfluidics, wherein two or more cell types are grown in separate, semi-permeable chambers, allowing for an exchange of nutrients. An example of such a solution can be found in a study by Veldhuizen et al., wherein a specifically designed co-culture yielded cells with improved cell maturation markers (Veldhuizen et al., 2020). A similar approach was also undertaken by Ronaldson-Bouchard (Ronaldson-Bouchard et al., 2018). These studies are further analyzed in Section 4 of this article.
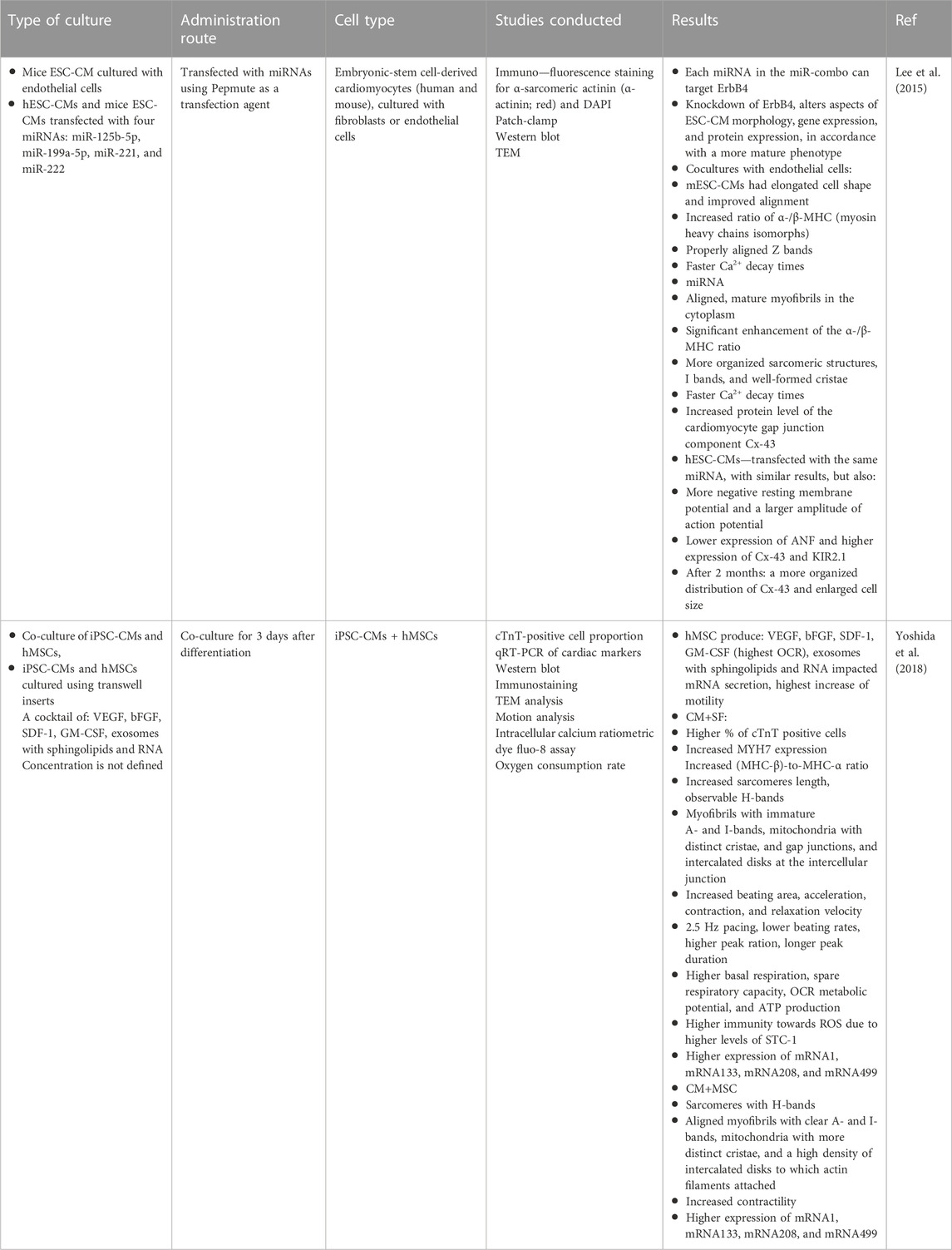
TABLE 2. Some strategies to conduct cardiomyocyte—fibroblast co-cultures and their positive outcomes.
4 Biofunctional cues
Constant progress in the field of biomaterials and other overlapping sciences (i.e., biology, chemistry, materials science, etc.) has led to an understanding that efficient regeneration of tissues cannot be completed without introducing proper biofunctional cues (Ratner et al., 2013a). For cardiac tissue engineering, examples of the most commonly reported biofunctional cues can be found in Figure 3.
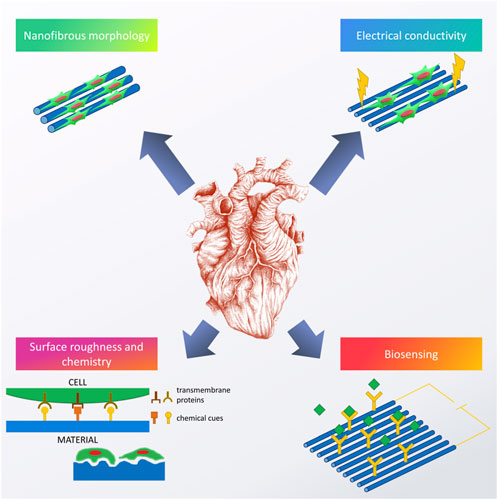
FIGURE 3. Examples of biofunctional cues used to enhance the performance of cardiac scaffolds. Image of the heart is adapted from “ink heart” by Julia Bramer, CC BY-NC 4.0.
Most of the current cardiac tissue engineering strategies are based on the idea that reproducing morphology and functionality of the natural cardiac ECM is the best way to guarantee successful regeneration (Dvir et al., 2010). As gathered in our recent chapter, cardiac ECM is mostly fibrous (Benko et al., 2022) and reproducing its morphology roots back to 2004, with recent articles proving a facility of doing so (Kitsara et al., 2017). However, the tricky part is to recreate not only the specific morphology, but also to mimic the chemical composition favorably, and include some additional functions.
Right now, it seems that the majority of scientific efforts in this field are focused on enhancing the as-obtained scaffolds with additional functions, thus creating highly biofunctional, smart biomaterials that would fit well into the category of third-generation biomaterials (Ratner et al., 2013a). Among others, fabricating materials with biomimetic mechanical properties (Young’s modulus of 10–50 kPa) and electrical conductivity (between 0.06 and 0.4 S/m (Miklavčič et al., 2006)), seems to be the most important. Thus, such scaffolds would enable cell to cell cross-talk, electrical stimulation in vitro, as well as the possibility of biosensing monitoring tissue regeneration and identifying any possible pathologies occurring at the implantation site. Furthermore, introducing specific roughness and especially nanoroughness, combined with proper chemical cues, enabling programmed cellular behavior, also seem to be very interesting research avenues. In the following chapters, a review on what has been done in these fields will be given.
5 Biomimetic composition
When designing biomaterials for tissue engineering, the first consideration is choosing the right material for the matrix. From the mechanical point of view, soft tissues like cardiac tissue are the most biomimetic to polymers than to any other material group. Among these, the easiest ones to work with are synthetic polymers, like polylactide (polylactic acid, PLA), polyglycolide (polyglycolic acid, PGA), polycaprolactone (PCL), polyurethanes (PU), or polyglycerol sebacate (PGS). Synthetic polymers have relatively good processability, i.e., can easily be tailored into specific shapes using standard and novel materials processing techniques such as: molding, various 3D printing techniques, freeze drying, and different spinning techniques. Apart from facile processing, some synthetic polymers are already approved by regulatory agencies for medical use as they guarantee high repeatability of the final product, and so, are relatively cost-effective to obtain a high-quality final product with desired properties. For this reason, these materials have dominated the field and there already have been some extensive reviews about their use in cardiac tissue engineering (McMahan et al., 2020; Trombino et al., 2021).
Still, it is important to keep in mind that the use of synthetic polymers has some important disadvantages. First off, there is low chemical biomimetism, reducing the chance of a positive reaction from cardiomyocytes, such as improved cell maturation or differentiation. Next, while some of them might be biodegradable (meaning they decompose into products that are safe for the environment and the living organisms), they are rarely bioresorbable (meaning that their products are incorporated into the natural metabolic cycle of cells). Further, these materials are far from green, i.e., they are rarely obtained from sustainable sources and their processing requires usage of toxic solvents (such as cholorform). All of these factors contribute to a relatively high environmental carbon footprint of synthetic biomaterials. A comparison of some of the most important pros and cons of synthetic and natural polymers are gathered in Figure 4.
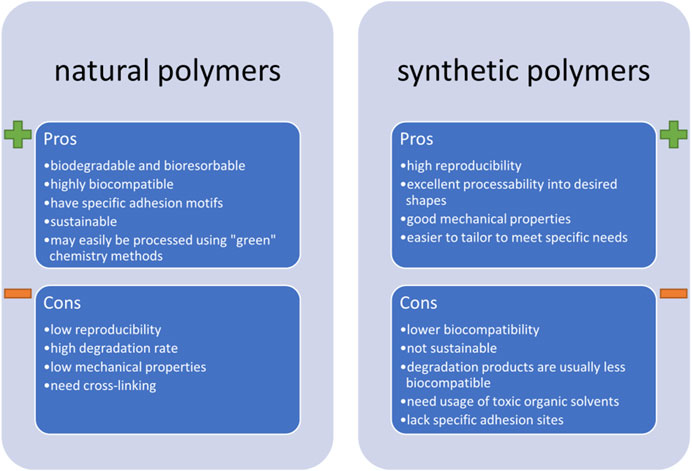
FIGURE 4. The most important pros and cons of using natural and synthetic biopolymers in fabricating scaffolds for cardiac tissue engineering.
All of the above-mentioned disadvantages of using synthetic polymers contribute to the rising trend of choosing those of natural origins. These can be grouped into three main categories: plant-derived materials, cell-produced materials, and animal-derived materials. Some recent examples of using natural polymers in the field of cardiac tissue engineering are gathered in our recent book chapter (Benko et al., 2022) and the readers are kindly referred to it if more details on the subject are sought. Instead, herein we will summarize some applications which we believe are the most promising. In fact, based on the cardiac ECM composition, it is our strong opinion that modern cardiac tissue engineering strategies should focus on fabricating scaffolds majorly based on collagen, especially type I. Collagen is a predominant component of numerous tissues. As such, it is a by-product of food, hide and skin industries–it is a common waste product that should be further utilized. This takes time and resources and instead, collagen could be incorporated into modern tissue engineering strategies. The easiest way of processing collagen involves its denaturation into gelatin and this is typically done through subjecting it to high temperature, acidic or basic hydrolysis (Van Vlierberghe et al., 2014). The outcome product has a gelling ability, but is also soluble in water, and is deprived of some of collagen’s biofunctional properties. To overcome the solubility problem, gelatin can be subjected to further processing, which often involves methacrylation (Yue et al., 2015), yielding photocrosslinkable materials. Apart from that, crosslinking of the functional groups by using amide bond activators, such as EDC (1-ethyl-3-(3Dimethylamino propyl)carbodiimide hydrochloride) can also be performed. This idea was investigated in a recent study by Kumar et al., wherein core-shell fibers were prepared by covering the PCL core with a gelatin shell (Kumar et al., 2020). But crosslinking does not recuperate the lack of specific adhesion motifs of collagen. Even worse, it can reduce the amount of functional groups and side chains, which are available to cells and responsible for their adhesion. To improve its biomimicry, recent studies focus on the use of natural collagen, and in some cases, even decellularized cardiac ECM. The latter was the subject of a 2016 review article by Wang and Kristman (Wang and Christman, 2016), followed by a newer one by Sakina et al. (Sakina et al., 2020) and the readers are kindly referred to these reviews for detailed background information and advances on the subject.
In a more recent study by Blazeski et al. (Blazeski et al., 2019), thin slices of adult myocardium from pigs were decellularized and then repopulated with iPSC-CM cells. The cells readily populated the as-obtained scaffold, revealing high levels of self-organization and alignment. Adult phenotype markers were revealed, and the slice had a highly synchronized contractile behavior. In a different approach, a hydrogel derived from cardiac ECM was mixed with chitosan and alginate to obtain a mechanically stable material. Sadly, its real performance and applicability are hard to evaluate as the authors seeded it with human mesenchymal stem cells, for which differentiation into cardiac cells is highly doubtful (Tamimi et al., 2020).
An example of superior performance of collagen type I in the CMs cultures can be found in a study by Veldhuizen et al. (Veldhuizen et al., 2020), which is described in detail in Section 4. Another example is MXene modified collagen scaffold, described in Section 5. Some older examples of the superior performance of collagen in cardiac tissue engineering can be found in a 2019 review by Wu (Wu et al., 2019).
Other examples of biopolymers used in cardiac tissue engineering are: fibrin (Tao et al., 2017; Ronaldson-Bouchard et al., 2018; Ronaldson-Bouchard et al., 2019; Pretorius et al., 2022), fibronectin (Amano et al., 2016), and albumin (Fleischer et al., 2017). All of these proteins were used to fabricate thick and functional patches, seeded with either iPSC-CMs or neonatal cardiomyocytes. In a study by Amano et al. (Amano et al., 2016), co-cultures with fibroblasts and endothelial cells resulted in vascularized tissue with high levels of troponin T recorded. While the results are very promising, further evaluation of the maturity of cardiomyocytes should be performed. Still, this is an interesting and important approach to obtain functional tissues in vitro which can be used for drug screening. A self-organizing fibrin hydrogel was suggested for a similar application by Tao et al. (Tao et al., 2017). Seeded with neonatal cardiac cells, the gel manifested synchronous and spontaneous beating as early as on the fourth day of culture. After 6 days of culture, the patches revealed adult heart beat-like contraction rates, with high levels of α-Actinin and Cx43 observed. Certainly, in order to move forward with the study, a more detailed evaluation of the maturation markers should be performed.
A multifunctional approach in the fabrication of the cardiac tissue engineering scaffold can be found in a study by Fleischer et al. (Fleischer et al., 2017). In order to replicate the complex architecture of the cardiac ECM, an electrospun albumin nanofibrous membrane was patterned with a laser to create aligned microgrooves for culturing neonatal cardiomyocytes. Alternatively, the same electrospun scaffold was patterned into microtunnels with side cages for culturing endothelial cells and releasing vascular endothelial growth factor (VEGF), respectively. Furthermore, another version of the same scaffold was processed to contain dexamethasone (DEX). The free layers were assembled into a thick scaffold, glued together with an ECM. This was used for the co-culture of two cell types and controllable release of factors known to speed up the CMs’ maturation (specifically, VEGF and DEX). It was revealed that CMs align with the fabricated patterns, are elongated, have a massive striation, and show upregulation of Cx43. An oriented propagation of electrical signal was observed. Sadly, the complex 3D architecture was not evaluated in vitro for its ability to induce cardiomyocyte maturation. Instead, it was implanted subcutaneously into a rat’s back to reveal its ability for neovascularization. Hence, further studies should be undertaken for evaluating the applicability of this system to in vitro cultures.
6 Chemical and biological modification
The idea of grafting the scaffolds for cardiac tissue engineering with various biological and chemical cues is often employed and as such, has been evaluated in quite a few reviews, both as a main topic (Tallawi et al., 2015; Jafarkhani et al., 2018), or as a part of a larger study (Kitsara et al., 2017). Generally, this strategy is employed to enhance the material’s functionality and thus, improve its biological performance. As with every other type of tissue engineering scaffold, this can be achieved either by surface or in-mass modifications, by physicochemical or biological methods. A graphical representation of some of the strategies used to modify a material’s functionality is found in Figure 5 (Bonzani et al., 2006). Surface modifications are most often designed to change the initial reaction of cells that come in contact with specific functional groups or peptide motifs, usually enhancing cellular adhesion, spreading and proliferation. Meanwhile, in-mass functionalization is aimed to cause more of a long-term, delayed effect, evoked by the release of active molecules: anti-inflammatory and anti-bacterial substances, specific growth factors, proteins, DNA or rDNA motifs, etc. (Tallawi et al., 2015). It is worth noting that in some cases, multi-step functionalization is justified to obtain desired results, for example, covering the material with a bioactive molecule can be preceded by surface modification via physicochemical techniques in order to introduce functional groups able to anchor the molecules (Boffito et al., 2018). In-mass and surface modifications can also be combined together to yield a material enriched with multiple biofunctional cues.
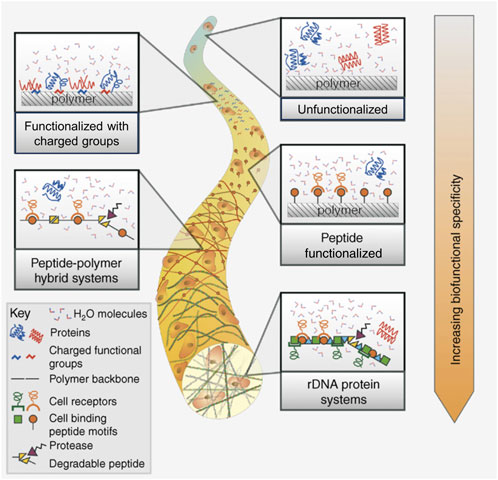
FIGURE 5. Strategies to enhance a biomaterial’s functionality by introducing chemical and biochemical cues (surface and in-mass modification). Reprinted with permission from I.C. Bonzani, J.H. George, M.M. Stevens, Novel materials for bone and cartilage regeneration, Current Opinion in Chemical Biology 10 (6) (2006) 568–575, Elsevier 2006 (Bonzani et al., 2006).
6.1 Physicochemical techniques
Physicochemical modification of the scaffold surface is typically performed to introduce functional groups to the surface of the material. Changing the surface free energy and altering the surface charge, can in turn have a direct effect on cell (Keselowsky et al., 2004; Thevenot et al., 2008; Nakaoka et al., 2010) and bacteria (Adolfsson et al., 2019; Duch et al., 2019) adhesion and proliferation, as well as cellular differentiation. Generally, the introduction of functional groups can be done via three alternative routes: 1) wet/dry chemistry, 2) plasma grafting/treatment, or 3) photo-induced grafting (Tallawi et al., 2015). While the first route is the cheapest, the most versatile and effective, making it possible to introduce virtually any kind of functional groups, it is also the one with the highest negative impact on the environment using aggressive, often toxic reactants. The process is hard to control and may also negatively affect the material’s bulk properties. Another disadvantage that is very troublesome is post-treatment purification, with the removal of unwanted substances requiring multiple washing steps. Still, it remains the most popular route to grant the material with a desirable type and amount of functional groups. Photo-induced grafting is safer than chemicals methods, but the availability of possible modifications is significantly slower and the efficiency of the process is relatively low. Meanwhile plasma modification, which is typically performed using oxygen or nitrogen plasma, can be used to obtain materials with a desired amount of functional groups but it is hard to control their type (for example, in O2 plasma, the oxidation level), and the depth of modification is typically limited to a very thin outer layer of the material, which can be insufficient for some applications (Tallawi et al., 2015; Kitsara et al., 2017; Jafarkhani et al., 2018).
In 2008, Natarajan et al. designed and performed a very important study aimed to investigate what types of functional groups are preferred by cardiomyocytes. To do so, the group performed wet chemistry modification with two types of amine-terminated chemical species ((3-aminopropyl)triethoxysilane (APTES) and trimethoxysilylpropyldiethylenetriamine (DETA)) and successive modification with self-assembled monolayers, terminated either with carboxyl or hydroxyl functional groups. It was found that the adhesion and proliferation of cells was enhanced on DETA modified surfaces, and retarded on surfaces with an abundance of hydroxyl species. Additionally, OH groups decreased the number of beating cardiomyocytes that generated longer action potentials. Thus, it had been suggested that OH groups impair cardiomyocyte viability, differentiation and maturation.
In 2014, Guex et al. modified the surface of an aligned PCL fibrous scaffold with a radio-frequency (RF) plasma process (Guex et al., 2014). The groups reported that the surface functionalization yielded biocompatible materials that supported mesenchymal stem cell adhesion. In vivo evaluation was completed using female Lewis rats with a surgical model of myocardial infarction and a cell-seeded and non-seeded scaffold. Non-seeded scaffolds did not alter the heart recovery process, but the cell-modified scaffold allowed for stem cell retention 4 weeks post-implantation which helped to stabilize heart function and reduce LV dilatation. The reported results are very promising and can serve as a stepping stone for further development of efficient cardiac tissue engineering scaffolds able to restore functional properties of the damaged heart. It seems that endowing the material with further biofunctional cues could yield even better results, and thus calls for further evaluation. However, it is critical to mention that PCL is not environmentally friendly, thus, this approach does harm the environment.
For more examples of surface modifications used to improve biomaterial biological performance for cardiac tissue engineering applications, the readers are kindly referred to Tallawi et al. (Tallawi et al., 2015).
6.2 Biological cues
As suggested by Tallawi et al., biological cues used to evoke a specific cellular reaction can be divided into four groups: short peptide sequences, ECM proteins, synthetic chemicals and growth factors (Figure 6). For a detailed description of what is used the most often and why, the readers are referred to the aforementioned article (Siramshetty et al., 2015). Here, we would like to list some of the most commonly used molecules and outline some of the more recent advances. Certainly, one of the biggest challenges in fabricating biomaterials embedded or covered with biological molecules is maintaining the biomolecule’s biological activity–this can be altered by physical and chemical interactions with polymers and dispersants or affected by processing techniques. In general, green processes are less susceptible to alter a biomolecule’s activity since they are often less aggressive, do not involved toxic chemicals, and are present in a natural form. This is most likely the main reason why recent advances in the use of more sophisticated biological modifications describe combining green materials with injectable hydrogels–in this form of administration, the risk of decreased biological activity is significantly reduced. Meanwhile, fibrous scaffolds are most often modified by covering them with natural ECM proteins and short peptide sequences, the latter giving a better chance to present receptor binding domains than the whole proteins which may become folded and denature more easily (Tallawi et al., 2015).
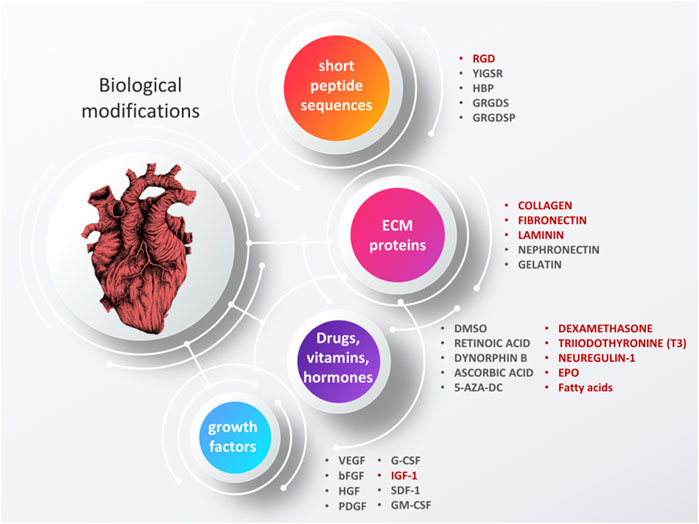
FIGURE 6. Biological modifications of cardiac tissue engineering scaffolds, aimed to grant materials with enhanced bioactive properties. Some of the additives that have shown the most promising results are highlighted in red. Based on (Tallawi et al., 2015). Image of the heart is adapted from “ink heart” by Julia Bramer, CC BY-NC 4.0, graphical scheme designed by TinyPPT.com.
6.2.1 ECM proteins
The ECM of the heart is built from multiple types of proteins, glycoproteins, proteoglycans, etc., but the most abundant and/or important ones are: collagen, laminin and fibronectin. At the embryonic stage of the heart, higher levels of fibronectin are responsible for cell proliferation and migration, while latter stages of heart development, growth, and functioning are associated with an increased ratio of collagen and laminin (Tallawi et al., 2015). Thus, when considering cardiac tissue engineering scaffold, it is important to plan which stage of cardiac growth one wants to simulate, and select the appropriate ECM proteins accordingly.
Fibronectin (FN) is a large molecular weight (500-kDa) glycoprotein which is known to be an ECM molecule essential in adhesion, survival and differentiation of stem cells (Kang et al., 2014). FN is secreted by mesenchymal cells and assembled into insoluble matrices which have significant biological functions in embryologic development (Limper and Roman, 1992). FN influences cellular migration and proliferation, both in vivo and in vitro, and also plays a critical role in cellular early development (Van Dijk et al., 2008). Fibronectin is organized into a fibrillar network through direct interactions with cell surface receptors (Mao and Schwarzbauer, 2005). This molecule is often used as a coating for various synthetically and naturally derived fibers to enhance cellular adhesion, spreading, growth, and proliferation (Kitsara et al., 2017; Liverani et al., 2019).
Layers of collagen can be deposited on a substrate of choice by a simple immersion technique. In cellular cultures, collagen is the main constituent of commercially available Geltrex and Matrigel (also rich in laminin) which are synthetic membrane matrices aimed to improve cellular adhesion and differentiation (Tallawi et al., 2015; Stepniewski et al., 2018). Its usage is a well-established procedure, guaranteeing good results and so, similarly, the modification of fibrous scaffolds also yields materials of improved cellular adhesion and growth. In a 2019 study by Kenar et al., collagen and hyaluronic acid were incorporated into a PLA/PCL fibrous scaffold. The collagen was harvested from human tissues, resulting in better biocompatibility and lower immune reaction. When cultured with adipose tissue-derived mesenchymal stem cells, a 3-fold adhesion increase was observed, while cultures with human umbilical vein endothelial cells revealed significantly improved vascularization. Although PLA and PCL are not environmentally friendly, if they can be avoided or a green polymer used as the scaffold, these results provide significant evidence that the obtained materials should be further studied for CVD tissue engineering applications (Kenar et al., 2019).
Laminin, which is crucial to cellular adhesion, spreading and migration is often used to modify fibrous and non-fibrous scaffolds (Tallawi et al., 2015). In 2014, Yu et al. compared the effects of modifying PLGA fibrous scaffolds with three different biomolecules: two types of short peptide sequences (RGD and YIGSR) and laminin. The RGD and YIGSR peptide sequences were added in-mass during electrospinning, while laminin was used as a covering layer. While all types of modifications improved cardiomyocyte adhesion, only laminin and YIGSR were able to enhance cellular maturation and contractile behavior. Laminin contains YIGSR motifs, so the results suggest that this short peptide sequence is essential for cardiomyocyte maturation. The authors advocate the use of YIGSR over laminin, as it can be more easily incorporated into the scaffold during fabrication with a reduced risk of denaturation, lack of necessity of additional modification steps, and higher efficiency of active molecule grafting (Yu et al., 2014). To overcome problems with the adhesion of active molecules in proper conformation, Boffito et al. subjected polyurethane scaffolds to plasma treatment, followed by laminin surface modification. Laminin modification improved cardiac primitive cell proliferation, inhibited their apoptosis and enhanced cellular maturation rate. Subcutaneous implantation in mice revealed excellent biocompatibility. Although polyurethane is not environmentally friendly, the obtained results indicated that well designed surface modification with laminin is a way to obtain highly biomimetic scaffolds, with good in vitro and in vivo performance (Boffito et al., 2018).
Other popular protein modifications include the use of nephronectin (Npnt) and gelatin. Npnt has been reported to enhance cell-to-cell communication, sarcomere maturation, alignment and synchronized contractions. Similarly, gelatin has been found to enhance cardiac cell adhesion, growth and differentiation (Tallawi et al., 2015; Jafarkhani et al., 2018). In 2011, Kai et al. had found that co-electrospinning of PCL and gelatin yielded cardiac tissue engineering scaffolds that promote cellular adhesion. When highly aligned, these fibers were able to enhance cellular attachment even furthermore and force cellular alignment–thus improving biofunctionality (Kai et al., 2011). Again, however, as with all of these studies above, the base polymer (here PCL) is not environmentally friendly leaving a large carbon footprint and should be replaced with a green polymer.
In 2017, Suhaeri et al. cultured fibroblasts on PLCL fibrous scaffolds to allow them to synthesize an ECM. After decellularization, a hybrid scaffold composed of synthetic and natural fibers was obtained. This interesting approach (although not environmentally friendly) resulted in excellent performance, with a significantly improved maturation of cardiomyocytes (Suhaeri et al., 2017).
6.2.2 Short peptide sequences
Among the many different short peptide sequences, the RGD motif (Arg–Gly–Asp) is the most abundant, responsible for cellular attachment via integrin binding. As such, it is very often used in tissue engineering scaffolds, including for the heart. However, as pointed out by Yu et al., better enhancement of cellular function can be achieved using YIGSR, with results similar to laminin, but with a higher ease of use and incorporation into scaffolds (Yu et al., 2014). Other modifications may include the use of longer RGD-containing sequences which were also found to stimulate integrins that are relevant in early cardiac development (Tallawi et al., 2015).
6.2.3 Growth factors (GFs)
Many types of GFs can be used to evoke specific cellular reactions, including directing stem cells along certain lineages (Sepantafar et al., 2016). A short reference of GFs used in cardiac tissue engineering is listed in Figure 6, with a description of the benefits and challenges in using those can be found in reviews by Tallawi et al. (Tallawi et al., 2015) and Hastings et al. (Hastings et al., 2015). Briefly, the most commonly employed are: VEGF (vascular endothelial growth factor), responsible for angiogenesis (and thus, survival of cells), G-CSF (granulocyte colony-stimulating factor), EPO (erythropoietin) and IGF-1 (insulin-like growth factor). G-CSF, EPS and IGF-1 have all been found to restore cardiac function, by the reduction of CM apoptosis and delay of CM ageing, respectively. In studies by Davis et al. (Davis et al., 2006) and Minato et al. (Minato et al., 2012), IGF-1 has been found to significantly enhance CM maturation. Alternatively, bFGF (basic fibroblast growth factor), HGF (hepatocyte growth factor) and PDGF (platelet-derived growth factor) have also been suggested, which are responsible for the proper growth and maturation of endothelial cells and development of an endothelium (Tallawi et al., 2015). Another growth factor recently reported to improve cardiac function in vivo is neuregulin-1 (NRG1). This epidermal growth factor has been found to improve cardiac function in a mouse model subjected to myocardial infraction. CM viability and proliferation were improved with increased angiogenesis. Meanwhile, fibrosis and apoptosis were significantly reduced, all indicating at least partial recovery (Chang et al., 2022). In Table 3, some examples of spectacular results obtained when using IGF-1 and NRG1 in CMs cultures are gathered, with more details regarding study methodology and outcomes.
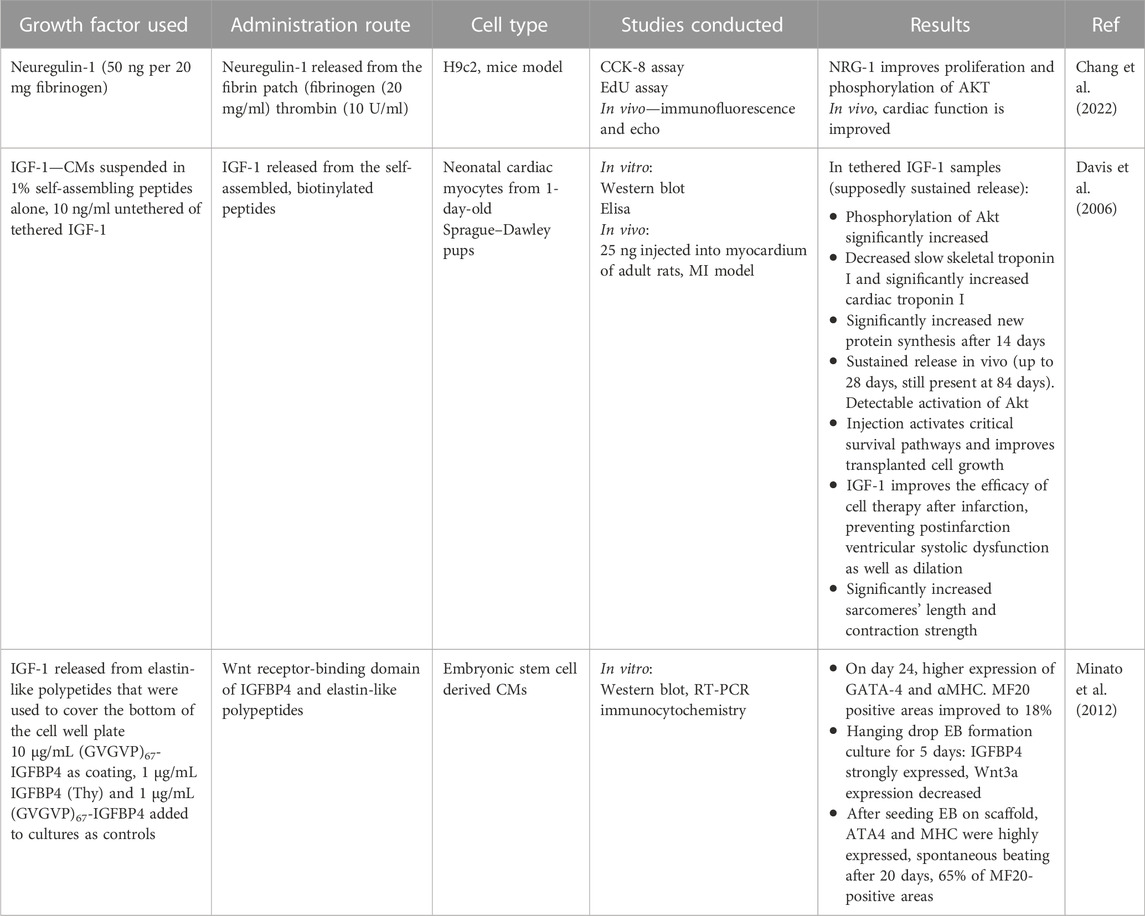
TABLE 3. Some examples of positive outcomes of using growth factors in cardiomyocyte in vitro cultures and in vivo mouse models.
6.2.4 Drugs
Several drugs have been reported to improve cardiomyocyte differentiation and maturation (Table 4). Among these, dexamethasone (DEX), triiodothyronine (T3) and IGF-1 seem to be the most interesting and promising. Recent articles suggest that the best positive outcomes can be obtained by combining these three (Gentillon et al., 2019; Huang et al., 2020). Different strategies suggest the use of fatty acids. In an interesting article by Yang et al. (Yang et al., 2019), fatty acids were modified with bovine serum albumin. In living organisms, albumin is responsible for binding fatty acids and transporting them inside cells. In vitro, unmodified fatty acids become toxic to cells as they are not able to penetrate the cell wall and instead, isolate the cells from extracellular stimulus. Hence, the hypothesis of the authors was that when fatty acids are modified with albumin, they can easily penetrate the cells. When cells recognize that their surrounding is rich in a new energy source (fatty acids instead of sugars), this might trigger alteration in their metabolism. Such alteration is characteristic of mature cardiomyocytes and hence, it was expected that this change would initiate a cascade of effects that in the end would yield a fully mature phenotype. This sound hypothesis has led to very positive outcomes after 2 weeks of culture. It can only be expected that longer cultures could guarantee successful obtainment of mature cardiomyocytes, in a relatively short period of time. More details on the positive outcomes of using different drugs are gathered in Table 4.
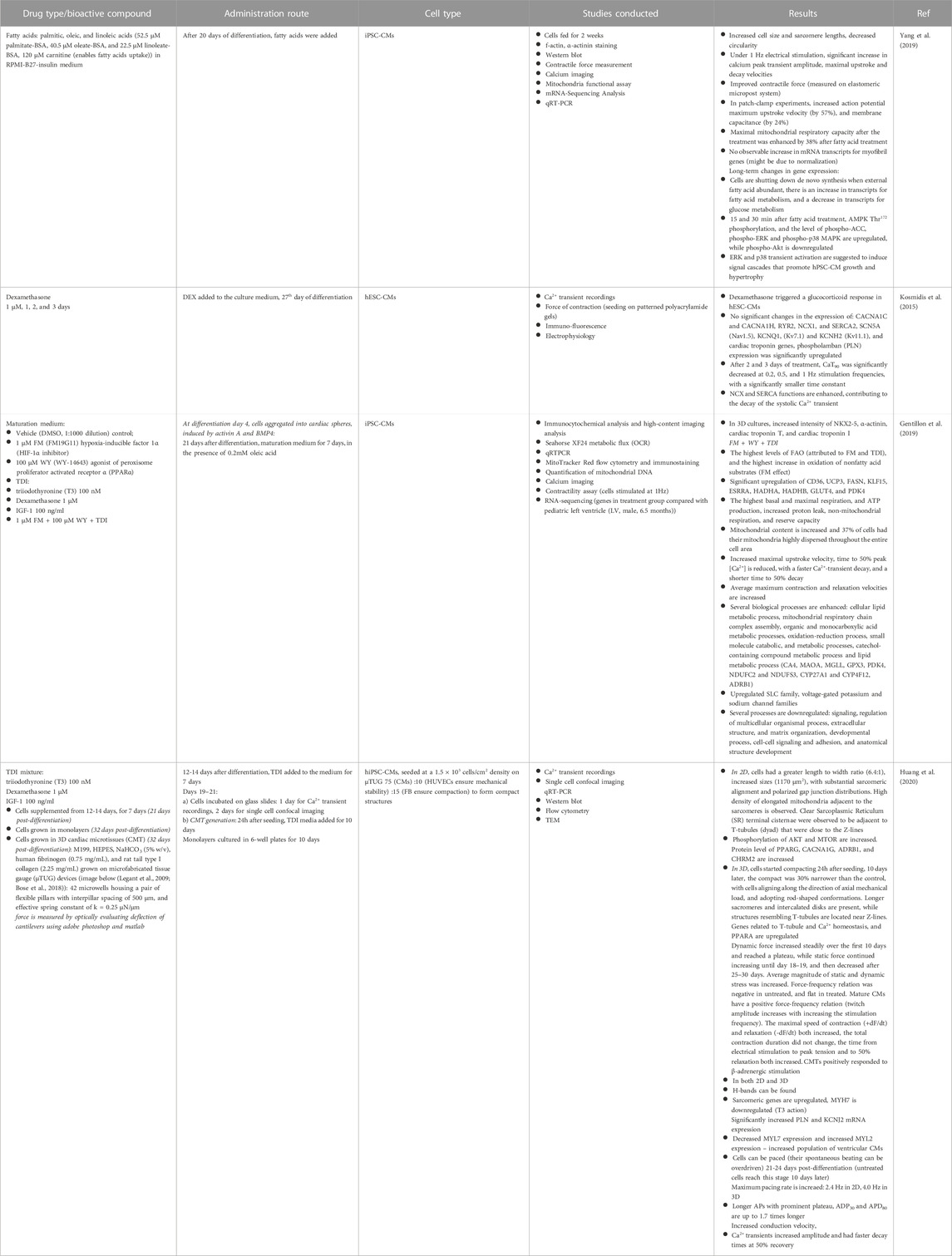
TABLE 4. Some examples of positive outcomes obtained through administration of different drugs and fatty acids into the CM cultures.
7 Biomimetic morphology
The ECM of the heart is fibrous, with an aligned topography (Benko et al., 2022), and some tissue engineering strategies for the heart aim to replicate that due to its importance in anisotropic mechanical, electrical, and biocompatibility properties. Further, it is documented that a typical ECM fibril diameter is between 30–100 nm (Kim et al., 2010). There are numerous literature reviews about using fibrous scaffolds, most notably, three done by: Zhao et al., in 2015 (Zhao et al., 2015), Capulli et al., in 2016 (Capulli et al., 2016) and Kitsara et al., in 2017 (Kitsara et al., 2017). In the 2017 article, M. Kitsara said that: “It is evident that more studies in the future should be performed using aligned structures which better mimic the native cardiac tissue anisotropy” and indeed, a lot more has been done on this subject from that time. In this chapter, we will focus on some important studies which have investigated the impact of aligned topography on cells.
The topography and morphology of a biomaterial surface has an important impact on cells. Size, shape, alignment, spacing, and depth of the features can all affect not only the cells’ alignment and clustering, but also, metabolism and differentiation. When the sizes of surface features are between 5 µm to tens of μm, cells are geometrically confined (a lower threshold depends on the cells’ dimensions and is bigger for larger cells) (Zinger et al., 2005). Between 70 nm and 5 μm, the sizes of the features approximate the cell’s sensorial organelles, i.e. focal complexes and focal adhesions, thus strongly contributing to cellular adhesion, morphology, migration, biosynthesis and differentiation. Below 70 nm, the size of features are smaller than the cell’s sensorial organelles and current research suggests that features smaller than 40 nm are not recognized by cells as adhesive and instead affect cells at different levels of performance (Ventre et al., 2012). When features are at the nanoscale, cells become more sensitive to their specific patterns or period of appearance. For example, in a 2010 study, Kim et al. found that when cultured on 800 nm wide grooves, separated 800 nm apart, important physiological parameters of neonatal rat ventricular CM improved, such as alignment, size, expression of the major gap junction protein, connexin 43, and directional electrophysiological properties (Kim et al., 2010). In a 2015 study by Morez et al. (Morez et al., 2015), similar grooves were found to enhance the efficiency of genetic reprogramming of iPSCs into CMs. Clearly, this bodes well for natural environmentally-friendly materials since such materials already have a natural built-in nanotexture. Synthetic polymers commonly used for CVD applications would need to be further modified for such promising nanotextures.
In recent years, different surface morphologies have been investigated with an aim to enhance CM maturation. For example, Kumar et al. (Kumar et al., 2020) suggested electrospun, aligned PCL fibers, covered with gelatin, with diameters of 578 ± 184 nm. It was observed that iPSCs–derived cardiomyocytes had significantly enhanced maturation markers, such as expression of cardiac troponin-T, α-sarcomeric actinin, connexin-43 (Cx-43), and synchronous calcium transients (Figure 7I). Of course, as mentioned, PCL is not environmentally-friendly, however, and selection of a green polymer would have decreased the environmental impact of such work. In a different approach (Figure 7II) (Bian et al., 2014), biomimetic molds of a human ventricle were obtained by reconstructing and replicating its ECM morphology, as visualized through magnetic resonance imaging (MRI). These molds were fabricated from polydimethylsiloxane (PDMS), via soft lithography, and the scaffolds for cell cultures were obtained by drop casting 10% Matrigel into the molds. After a 3-week culture, the as-obtained engineered tissue revealed a phenotype that was close to a mature tissue. Most notably, T-tubules with Z-disks were observed. The CMs were dense, aligned and electromechanically-coupled, and an action potential had a conduction velocity of near-adult cells. PDMS is also not environmentally friendly leaving a large carbon footprint, but it is anticipated that the same mold could be used to create green biopolymer tissue engineering materials.
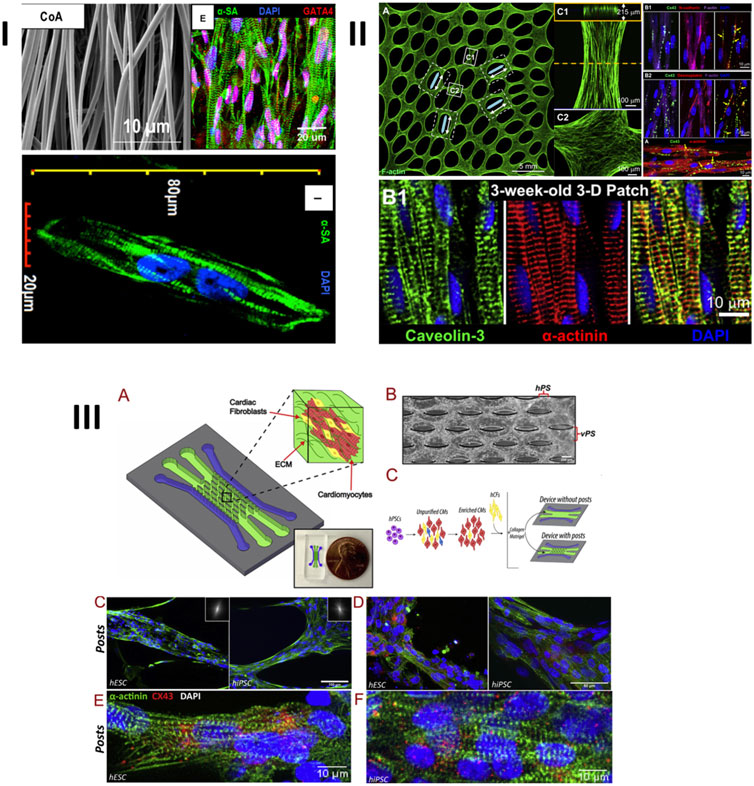
FIGURE 7. Some examples of enhanced expression of maturation markers of cardiomyocytes, cultured on different types of topographies/nanotopographies: 1) iPSCs–derived CMs cultured on electrospun aligned nanofibers; 2) neonatal rat ventricular cardiomyocytes grown on epicardial mimetics obtained by casting 10% Matrigel into biomimetic silicon molds, obtained via soft lithography, and 3) iPSCs–derived CMs and EPSc–derived CMs co-cultured with cardiac fibroblasts on a microfluidic chip. CMs are embedded inside a collagen-Matrigel hydrogel and cultured on top of microposts. I: Reprinted from Kumar et al. (2020) under the Creative Commons license (CC BY). II: Reprinted from Bian et al. (2014) with permission from Elsevier, Copyright 2014. III: Reprinted from Veldhuizen et al. (2020) with permission from Elsevier, copyright 2020.
In a more recent study (Veldhuizen et al., 2020), an effort to fabricate a heart-on-a chip based on microfluidics was undertaken. This more advanced study combined multiple cues: co-cultures, collagen-based scaffold, and microtopography in the form of microposts (Figure 7III). By using such a combinatorial approach, unparalleled results were obtained during a relatively short-timed culture. After 2 weeks, CMs revealed an upregulation of maturation markers (such as HCN1, KCNQ1, CAV1.2, CAV3.1, PLN, and RYR2 genes, and expression of Cx43 α-sarcomeric actinin). The cells had well-defined sarcomeric striations, were aligned and well organized, resulting in synchronized contractions. Even though the cells can’t yet be defined as fully adult, the obtained results are certainly very promising and could be further extrapolated.
8 Electrical conductivity
There are three major strategies to obtain electrically conductive (EC) biomaterials for CVD applications (Figure 8). The simplest, but the least reliable one is based on the use of hydrogels. In these materials, ionic type conductivity is obtained by the presence of free ions dissolved in water, which is a dispersion medium. The conductivity of hydrogels is often found to be insufficient for such advanced applications as sensors or electrically-stimulated materials. Furthermore, in hydrogels, the electrical conductivity might deteriorate over time due to crystallization of salts within their structure, defragmentation of material and negative impact of elevated temperatures. For these reasons, coatings or blending with other polymers and/or electrically conductive additives/nanoadditives are usually the methods of choice when one is to obtain CVD biomaterials. It is worth noting that some scientists suggest combining two or more methods: for example, it is not unusual to see articles regarding the fabrication of hydrogel/nanoadditive (Martins et al., 2014) composites or blending with conductive polymers (Guiseppi-Elie, 2010; Walker et al., 2019), which can be further modified by combining additives with electron-based conductivity (Qazi et al., 2014; Bao et al., 2017; Wu et al., 2017). Considering the goal of facilitating cell to cell cross-talk, the conductivity of the as-obtained CVD composite material should be similar to the native myocardium, i.e. between 0.06 and 0.4 S/m (Miklavčič et al., 2006). However, when one wants to achieve further functionalities, electrical stimulation and biosensing, the higher the conductivity, the better, as it increases the efficiency of the sensing/stimulation, thus improving the material’s sensitivity and specificity. However, remember, fibers in the myocardium are anisotropic and so are its electrical properties.
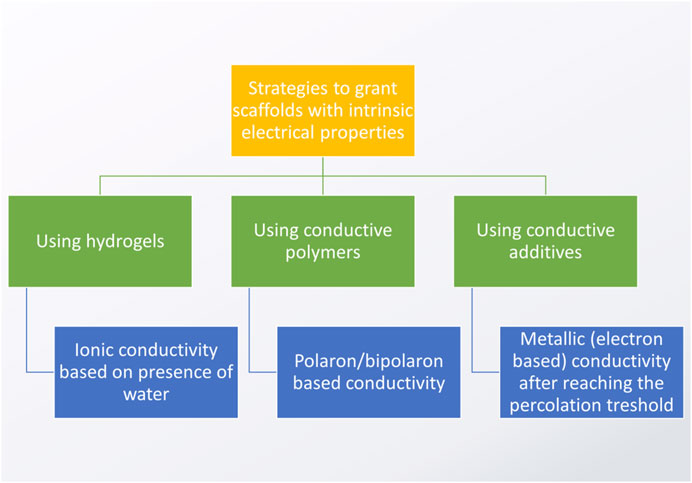
FIGURE 8. Major strategies to obtain electrically conductive scaffolds (based on the studies by: Pissis and Kyritsis, 1997; Hsu et al., 2004; Balint et al., 2014; Kaklamani et al., 2018).
The subject of fabricating electrically conductive scaffolds for cardiac tissue engineering, by using electrically conductive nanoadditives and conductive polymers, has been a focal point of a very recent and extensive review by Ashtari et al. (Ashtari et al., 2019), and the readers interested in more detailed information on the subject are kindly referred to it. In turn, the justification of using electrically conductive scaffolds for the regeneration of heart tissue, along with some important examples, are extensively elaborated on in a recent study done by Monteiro et al. (Monteiro et al., 2017). Here, we would like to provide a short guidance on what was accomplished with respect to fibrous materials recently, and what is the rationale for future perspectives of using electrically conductive environmentally-friendly cardiac scaffolds.
8.1 Electroconductive polymers (ECP)
The so-called conductive polymers are often polymeric materials “doped” with a dopant molecule that stabilizes their charge and is not friendly to the environment. When the conductive polymer is synthesized in its oxidized form, the dopant is positively charged, while in the reduced form, its charge is negative. If a polymer has a conjugated network, with overlapping p-orbitals, the charge can travel freely along the polymer chain and this is how electrical conductivity is achieved in this class of materials. For an elegant explanation of this phenomena, the readers are kindly referred to an excellent literature article by Balind, Cassidy and Cartmell (Balint et al., 2014).
Despite large discrepancies regarding the biocompatibility of conductive polymers and concerns regarding the environment and toxic fate of possible degradation products, there are many literature examples of their suggested biomedical applications, such as in biosensors, electrodes, drug delivery systems, bioactuators, and tissue engineering scaffolds. There are some interesting reviews on the matter and the readers are kindly referred to them for more extensive evaluation (Hardy et al., 2013; Balint et al., 2014; Yi et al., 2016; Gajendiran et al., 2017; Ning et al., 2018; Ashtari et al., 2019; Hosoyama et al., 2019). For electroconductive polymers, inherent biostability is one of the biggest challenges in their application as tissue engineering scaffolds. This quality can be modified to obtain a biodegradable polymer. The three possible routes are: 1) mixing with a degradable polymer, 2) modifying the polymer backbone and 3) synthesizing small chains that can be removed from the human body by renal clearance. Importantly, however, just because a polymer is biodegradable does not mean it is safe for use in the body or the environment. All of the above mentioned routes raise some questions regarding the fate and biocompatibility of the decomposition products which need further in vitro and in vivo studies to guarantee safe usage (Balint et al., 2014). Still, it seems that mixing conductive with biodegradable polymers may be a possible route towards the safe biomedical application of EPC-based fibrous scaffolds as it combines spinnability with degradability. This is the probable explanation why most of the recently published articles concern fabrication of such composite blends.
Currently, there are over 25 well-analyzed, electrically conductive polymers, but in cardiac tissue engineering, the ones that are the most commonly applied are polyaniline (PANi), polypyrrole (PPy) and poly (3,4-ethyl-enedioxythiophene) (PEDOT), due to their relatively low price and acceptable biocompatibility. None are considered safe to the environment and all leave a large carbon footprint. Even though PANi and PPy are electrospinnable, the conditions of electrospinning are very demanding and unsuitable for biomedical applications (for example, PANi needs to be dissolved in hot sulfuric acid). Additionally, these polymers also have unsuitable mechanical properties–they are stiff and brittle. Thus, to overcome these issues, they are most often used as coatings or blended with carrier materials and co-electrospun with other biocompatible and often biodegradable polymers, natural or synthetic: PLA, PLGA, PCL, gelatin, etc. (Balint et al., 2014). Positive results of ECP applications in vitro are generally attributed to their enhancement of cell to cell crosstalk (by the transmission of electrical impulses), manifested, among others, by increased expression of Cx43 protein. The addition of ECP reduces electrospun fiber diameters, most likely due to increased conductivity of the polymer solution during spinning. Cellular adhesion and proliferation are enhanced. At the same time, the synthesis of cardiac differentiation markers is improved and cellular maturation is boosted.
Still, in order to further improve the scaffold’s performance, additional cues are highly recommended. The benefits of ECP include an ability to apply electrical stimulation, which, unfortunately, has not been sufficiently exploited (less than 50% of the articles analyzed herein (Table 5, (Jeong et al., 2008; Jun et al., 2009; Fernandes et al., 2010; Yow et al., 2011; Hsiao et al., 2013; Gelmi et al., 2016; Mohammadi Amirabad et al., 2017))). It has been found that fiber alignment in CVD tissue engineering materials is also an important cue that guides cardiomyocyte growth, differentiation and maturation but only some of studies seem to be taking advantage of that fact (Ku et al., 2012; Chen et al., 2013; Hsiao et al., 2013; Mohammadi Amirabad et al., 2017), with only two exploiting both ES and fiber alignment (Hsiao et al., 2013; Mohammadi Amirabad et al., 2017). Based on the available literature, it is hard to point out which ECP would perform better as a cardiac tissue engineering scaffold–regardless of type, the presence of ECP seems beneficial but other physicochemical properties vary greatly between the studies and, thus, it is extremely difficult to ascertain which properties are enhancing cell function. Certainly, further studies are needed to be able to compare the impact of the conductive PANI, PPy and PEDOT polymers keeping all other properties the same (such as fiber morphology, diameter, alignment, mechanical properties, etc.). Furthermore, additional disadvantages regarding the usage of ECPs should be investigated, i.e. a conductivity decrease with time due to deprotonation (usually after 100 h (Hsiao et al., 2013), sometimes up to 2 weeks (Chen et al., 2013)) during which a dopant (usually acid) leaks from the sample, often causing a cytotoxic reaction. Also, environmentally-friendly materials and approaches must be investigated, even something as simple as using safer solvents–the majority of solvents used for conductive polymer synthesis are toxic. While investigating the beneficial properties of ECPs, one must also keep in mind that these polymers are not “green”—their processing requires a large carbon footprint and usage of toxic solvents. It is clear that such materials are not sustainable.
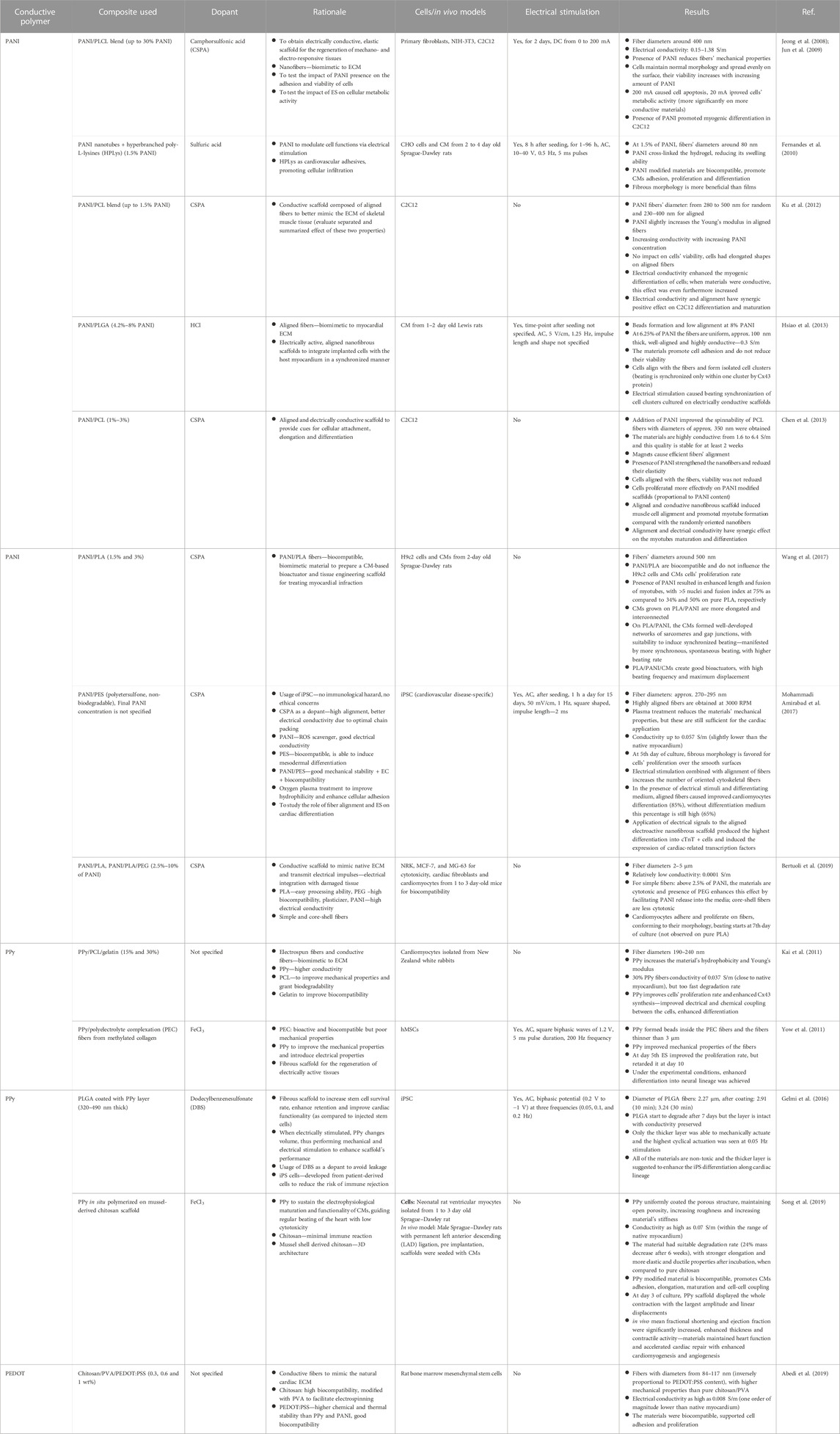
TABLE 5. An overview of electrically conductive polymers used to obtain fibrous scaffolds for applications in cardiac tissue engineering.
Due to some issues with long term stability and possible toxicity, it is hard to find in vivo studies testing the materials’ actual performance in treating damaged cardiac tissue. In fact, for this review, we were not able to find any in vivo studies done on conductive fibrous scaffolds as described herewithin. In 2018, Wang et al. (Wang et al., 2018) investigated the impact of injectable hydrogels based on tetraaniline, while a 2019 study by Song et al. concerned 3D porous scaffolds modified with PPy (Song et al., 2019). In both studies, the applied materials were able to maintain heart function and accelerate cardiac repair with enhanced cardiomyogenesis, angiogenesis and maturation. One can only imagine that endowing the materials with additional biofunctional cues (i.e. electrical, topographical, and/or mechanical stimulation with an aligned nanofibrous architecture) could yield even better results.
8.2 Nanoadditives
Blending polymers with electrically conductive additives is not a new subject. In fact, it dates back as far as the 1950s, when one of the first patents was filed (Cole, 1956). The invention assumed covering polymer particles used for molding with metallic films. In the 1990s, this idea was evolving and many researchers mixed polymer powders with conductive fillers, most notably, carbon black. Back then, it was already well understood that proper distribution is key to reaching an optimal conductivity with the lowest possible concentration of the additive (Ouyang and Chan, 1996). Most commonly, the electrical conductivity of composites related to the presence of the filler is described by the percolation theory and percolation threshold (pt). From the material scientist’s point of view, pt is the lowest possible concentration of the filler at which it is able to form a “connected component”—a network of connected particles through which electrical charge is able to travel freely. Generally, the higher the particle surface area (and, thus, smaller size), the easier it is to reach a percolation threshold–hence, smaller amounts of nanofillers are needed to yield electrically conductive materials, when compared to micro and macro-scaled additives. Higher amounts are uneconomical and could deteriorate a material’s mechanical properties. Additionally, some of the additives may evoke a toxic reaction at higher concentrations. For better understanding of the percolation threshold phenomenon, the reader is kindly referred to a recent study by Saberi (Saberi, 2015). To sum up, using nanoparticles is beneficial as lower amounts need to be added to the matrix to reach the certain conductivity, thus, representing a green approach. Additionally, when considering fabricating nano-sized morphologies, one is forced to also scale down the components’ size. For these reasons, the subject of fabricating electro conductive scaffolds is currently dominated with nanofillers, most notably carbon and gold. The main focus is to find a highly conductive filler that is biocompatible, easily dispersible in a polymer matrix and has a high surface area, reducing the percolation threshold (Mao et al., 2012). Morphology is also important–when the particles have elongated shapes (fibers, tubes), their alignment within the matrix can be forced during processing and may lead to a further increase in material properties (Ahadian et al., 2016). The two major approaches to introduce electrical conductivity to the scaffold by means of using conductive nanoparticles are: creation of a conductive layer (Zhao et al., 2018) or use of matrix modificators (Kitsara et al., 2017). While the former will generally grant higher surface conductivity, a risk of flaking resulting in an uncontrolled release of particles and electrical properties deterioration are some of the reasons why matrix modification is more common. Additionally, using a properly selected matrix modification may enhance not only the material’s electrical behavior but also its mechanical properties. For these reasons, the following paragraphs will focus mostly on matrix modification of scaffolds to enhance conductivity.
8.2.1 Questions of toxicity
When reducing the size of a conductive additive, a question of its potential toxicity becomes more and more burning. This is due to the fact that the nanoparticles can interact directly and indirectly with cells in numerous ways, damaging their structure, changing their metabolism and reducing their viability (Benko et al., 2021; Benko et al., 2023)—all of which can have negative consequences, starting at irritation and inflammation, and ending at necrosis and formation of malignant cancers (Shvedova et al., 2012; Vial et al., 2017). This is a very complicated matter as the occurrence of unwanted reactions depends not only on the nature of the nanoparticle, but also its chemistry and how it is introduced into the body and/or environment. While in classical biomaterial science, some substances are inherently toxic or non-toxic, in the case of the nanoparticles, there is a plethora of physicochemical properties that affect cellular reaction: such as size, shape, state of surface, dispersability, specific surface area, tissue specific behavior, administration route and overall an ability to create reactive oxygen species (ROS) (Figure 9). Specifically, surface related characteristics become more important as the surface-area-to-volume ratio becomes unproportionally higher than in micro and macro sized biomaterials. The complexity of the matter, together with an insufficient physicochemical evaluation of the nanoparticles used by different scientists, has led to large discrepancies concerning the safe application of nanomaterials, among which carbon nanotubes are likely the most controversial. However, careful evaluation of available data indicates that safe usage of nanoparticles may be achieved by using particles clean of metallic catalyst, with low aspect ratio, two dimensions exceeding 15 nm with small and polar functional groups covalently attached to the surface enabling good dispersion in body fluids, followed by facilitated clearance or even biodegradation. Additionally, target cells as well as form of application and administration route should always be carefully selected to be able to evoke specific reactions (Kostarelos et al., 2007; Kostarelos, 2008; Vittorio et al., 2009; Kotchey et al., 2013; Bhattacharya et al., 2016; Chatterjee et al., 2016; Zhang et al., 2016; Vial et al., 2017). Please do note that while some studies have been completed on in vivo toxicity to nanoparticles, much less research has been conducted on environmental toxicity of nanoparticles. This needs to be fully understood before nanoparticles can be viewed as environmentally-friendly as most likely such properties will depend on the chemistry of the nanoparticles, how they were synthesized, and how they were introduced into the environment.
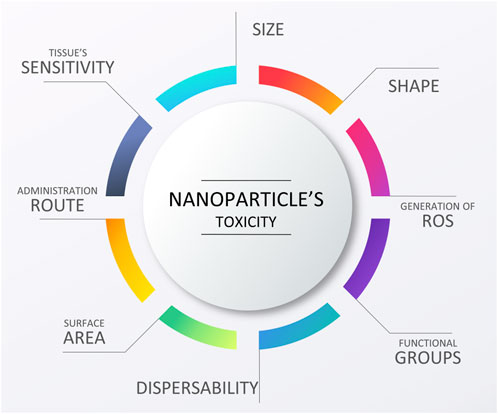
FIGURE 9. Factors affecting nanoparticle toxicity/biocompatibility, graphical scheme designed by TinyPPT.com.
8.2.2 Gold nanoparticles (GNPs or AuNPs)
Gold as a biomaterial has one of the oldest histories of use, with well-established biocompatibility manifested by at least a few thousand years of biomedical applications (Ratner et al., 2013b; Vial et al., 2017). From this point of view, gold nanoparticles are relatively young as their medical applications date back only to 1971, when they were applied in immunochemistry by Faulk and Taylor (Dykman and Khlebtsov, 2011). Progressing from the 70s, a plethora of possible medical applications have emerged: from cell therapies and drug delivery systems, through labeling tools, ending at scaffold modification. In tissue engineering applications, GNPs may be used to improve mechanical properties, enhance cellular adhesion or grant the material with electrical conductivity. GNPs can be synthesized in various shapes and sizes, tailored to meet specific applications (Dykman and Khlebtsov, 2011; Vial et al., 2017).
In cardiac tissue engineering, the idea to use gold nanoparticles started around 2011 with numerous scientists proving its excellent cytocompatibility and ability to promote desired cellular behaviors, such as improved cell adhesion, elongated morphology, faster proliferation and improved maturation of both adult and stem cells-derived cardiomyocytes. Most scientists suggest that the superior biological response of gold nanoparticles is due to its improved cell to cell cross-talk (due to electrical conductivity) and altered nanotopography (Vial et al., 2017; Ashtari et al., 2019).
In 2016, several scientific groups independently studied the beneficial impact of GNP modified polymers (both natural and synthetic) on cardiac lineage cells (Table 6). When combined with electrical stimulation, materials improved cellular adhesion, proliferation and differentiation. Cells were found to possess an elongated morphology. Forming well packed tissue-like constructs, cardiac cells revealed more synchronized beating with a reduced excitation threshold (Baei et al., 2016; Ganji et al., 2016; Navaei et al., 2016; Thrivikraman et al., 2016). Remarkably, Thrivikraman et al. induced stem cell differentiation along the cardiac lineage without using any exogenous biochemical factors but by combining GNPs inside cells and physiologically electrically stimulating them (Thrivikraman et al., 2016). However, it should be noted that the studies were conducted on human mesenchymal stem cells for which differentiation into CMs should be treated with great caution–their differentiation potential will depend on the cell source and it is generally acknowledged that these cells have the ability to form cardiomyocyte-like cells and not cardiomyocytes (Guo et al., 2020). This is due to elevation of some (but not all) CM-specific markers. Studies on hydrogel scaffolds revealed a beneficial impact of gold on the material’s mechanical properties (Li et al., 2016; Zhu et al., 2017). Interestingly, Li et al. (Li et al., 2016) found that GNP-modified scaffolds improved biofunctional properties, as compared to pure collagen, upon seeding with neonatal rat ventricular myocytes. The team hypothesized that improved maturation of cells and faster assembly of intercalated discs can be attributed to changes in nanolocal stiffness, emphasizing an importance of mechanical nanocues in cardiac tissue regeneration. However, in order to separate GNP presence (altering, among others, roughness and electrical conductivity) from their impact on mechanical properties, more studies should be performed.
Even though gold nanoparticles are quite often incorporated into scaffolds, benefits of their exclusive presence have not been carefully reported or investigated, i.e., electrical conductivity of gold-modified materials has not been well studied as electrical stimulation has not been applied, which is somehow surprising given the excellent results reported by Thrivikraman et al. and their successive review article on that matter (Thrivikraman et al., 2016; Thrivikraman et al., 2018). What is more, the morphology of the scaffolds is often not biomimetic to the native myocardial ECM–it is not fibrous. We strongly believe that progress in the treatment of myocardial infraction cannot be made without combining multiple biofunctional cues to induce a desired level of proliferation, differentiation and maturation into fully functional, adult tissues. What is more, the GNPs used in most of the studies are often non-dispersible in their un-modified state and their fate during uncontrolled or controlled release from the scaffold during its degradation tends to be neglected. It is our opinion that before the GNPs are to be used in clinics, their long term in vivo toxicity, with respect to their aspect ratio, shape and tendency to agglomerate, should be evaluated, similar to the multiple studies that were done on CNTs within this field (Kostarelos et al., 2007; Kostarelos, 2008). Moreover, protocols to grant the materials with long-term stability in polar fluids, as to facilitate the biological clearance, should be established. Further, although there are green chemistry methods to produce gold nanoparticles, most (if not all) of the gold nanopartilces used for CVD applications found in the literature are made through the use of toxic chemicals.
8.2.3 MXene
MXenes are a relatively new class of 2D materials, formed with an atomically thin layer of transitional metal carbides and nitrides. The first mention of MXenes date back to 2012, and recently, they have been attracting increasing attention in biomedical applications (Rafieerad et al., 2021; Bashandeh et al., 2022). This is because they are relatively easy to mix with various matrix materials, they are biocompatible, antibacterial, and can provide composites with electrical conductivity. In a recent study by Asaro et al. (Asaro et al., 2023), composites based on collagen modified with gelatin-modified MXene were fabricated. The product improved electrical conductivity and was found to facilitate CM adhesion, spreading and elongation. During in vitro cultures which were aided with electrical stimulation (biphasic, 2 ms long pulse, ±2.5 V amplitude, and a frequency of 2 Hz, 1 h/day for 4 days), iPSC-CMs cells enhanced expression of Cx43, indicative of a more mature phenotype. While these are preliminary results and more analyses is needed to confirm the findings, this is certainly an interesting an exciting new class of materials to be used in cardiac tissue engineering. It also remains to be seen how environmentally friendly or unfriendly MXenes are.
8.2.4 Carbon nanoparticles (CNPs)
Since Eiji Osawa’s study in 1971 that predicted the existence of a new carbon nanoform (fullerene) followed by its successful synthesis in 1985, nanoforms of carbon have been attracting significant attention in numerous fields of science, due to their incredible mechanical and electrical properties, high potency for chemical modification, and small size (Kratschmer et al., 1990; Smalley et al., 1998; Boyd and Slanina, 2001; Delgado et al., 2008; Aziz et al., 2011). For almost 40 years, studies have elucidated their unique physicochemical properties and have inspired multiple applications, including medical, upon which many extensive reviews have been written (Saito et al., 2011; Nunes et al., 2012; Fraczek-Szczypta, 2014; Saito et al., 2014; Gonçalves et al., 2016; Serpell et al., 2016). These point out that careful tailoring of carbon size, surface chemistry or structural properties is a route towards safe and effective biomedical applications, including those for CVD. By this means, tissue engineering materials with a unique impact on cells can be obtained: improving cellular adhesion, enabling cellular interactions, guiding and enhancing stem cell differentiation, and providing a possibility for electrical stimulation (Stout and Webster, 2012). In cardiac tissue engineering, the benefits of carbon nanoforms use has been analyzed in great details in the review by Dozois et al. (Dozois et al., 2017). In the following sections, we’d like to provide some more examples of such excellent results, dividing the carbon nanoforms by their structure. Importantly, while initial carbon structures were formulated through environmentally unfriendly methods (such as the use of methane and/or chemical vapor deposition), new efforts have revealed that carbon materials can be made from and found in numerous natural sources, such as microwaving honey to form carbon nanoparticles.
8.2.4.1 Carbon nanofibers
Carbon nanofibers (CNFs) are miniaturized forms of traditional carbon fibers (CFs) which have been analyzed for potential biomedical applications since the 1970s. Due to their excellent mechanical properties, low density and high inertness, traditional applications of CFs are in orthopedics (such as for tendon and ligament replacement, bone and cartilage tissue engineering, spinal implants, etc.) and other applications, including left-ventricular assist devices, tracheal prosthesis, or hernia meshes; all have reported excellent results (Saito et al., 2011). Thanks to progress in fabrication methods, CNFs can now be easily synthesized (most notably, via electrospinning (Stodolak-Zych et al., 2016; Benko et al., 2019)). They are regarded to be more interesting for biomedical applications than their micro-sized cousins, due to higher surface area, improved electrical conductivity and higher morphological biomimetism to natural tissue fibers (Elias et al., 2002; Price et al., 2003; Tran et al., 2009; Saito et al., 2011; Zhang et al., 2014). When it comes to cardiac tissue engineering, a series of studies in the Webster group by Stout et al. and Asiri et al. have proven that human cardiomyocytes prefer CNFs-modified composites over the unmodified polymers (manifested by improved cell adhesion, proliferation and metabolic activity), due to increased surface roughness and better electrical conductivity, with CNF alignment further enhancing these effects (Stout et al., 2011; Stout et al., 2012; Asiri et al., 2014). In another study done by Martins et al., the addition of CNFs into a chitosan scaffold resulted in materials with conductivity similar to the native myocardium, which was able to enhance cardiogenic properties of neonatal rat CMs (Martins et al., 2014) (Table 7). Despite these promising results, more recent reports on CNF applications in cardiac tissue engineering are hard to find. It seems that CNTs and graphene have taken over this field, most likely due to the higher availability of such materials with different physicochemical properties, including the ones that are more dispersible in polar liquids, and hence potentially safer in biomedical applications. CNTs and graphene are also more versatile and easier to work with than CNFs. None-the-less, they all show promise for CVD applications.
8.2.4.2 Carbon nanotubes
Carbon nanotubes are multiple (multi-walled CNTs, MWCNTs) or single (SWCNTs) rolled up sheets of graphene. Depending on the rolling axis (chiral vector), the chiral, arm-chair, or zig-zag configuration of the tube can be obtained. Different structural orientation yields varying mechanical and electrical properties, with arm-chair CNTs having lower critical tensile strain and metallic type conductivity, while zig-zag and chiral–higher critical tensile strain usually have a semi-conducting character (Mori et al., 2005; Sanchez-Valencia et al., 2014). It is estimated that the synthesis of SWCNTs yields a mixture of equal amounts of three types of configurations (not to mention possible imperfections in the structure)—this can cause issues in the reproducibility of the results, as with every usage, different amounts of each type of CNTs can be taken. Meanwhile, MWCNTs which are made of multiple layers of graphene sheets, forming tubes of varying chirality, are generally more uniform in their physicochemical properties, with metallic type conductivity and higher mechanical properties than SWCNTs. Another advantage of MWCNTs is their size–having diameters usually exceeding 15 nm, these CNTs have lower tendency to become tangled and agglomerated and are generally less toxic (Mori et al., 2005; Eres et al., 2010; Chatterjee et al., 2016). These are the main reasons why MWCNTs are more often used in biomedical applications than SWCNTs, and with better results (Dozois et al., 2017). For example, in 2011, Sirivisoot et al., found that myocytes preferred MWCNTs-modified polyurethanes over SWCNTs-modified ones, as manifested by increased proliferation rate, further improved by electrical stimulation (Sirivisoot and Harrison, 2011). Compared to other conductive additives, CNTs with their tubular shape have higher biomimetism to the natural ECM, have excellent mechanical and electrical properties, and are of high commercial availability (coming in different shapes, varying in surface chemistry, etc.). What is more, recent results suggest that highly functionalized CNTs may be degraded after internalization by immune cells, promising higher safety in applications in tissue engineering scaffolds (Kotchey et al., 2013; Bussy et al., 2016; Modugno et al., 2016). Further, recent research has shown that CNTs can be made without toxic catalysts and even with plants and natural materials representing an environmentally-friendly material.
Since the 2005 study by Garibaldi et al. which reported excellent CNT biocompatiblity properties with cardiomyocytes (Garibaldi et al., 2005), applications of CNTs in cardiac tissue engineering have gained increasing attention with very promising results (see Table 8 for a short selection, while more extensive information can be found in reviews by Ashtari et al. (Ashtari et al., 2019), Gorain et al. (Gorain et al., 2018) and Dozois et al. (Dozois et al., 2017)).
Overall, scientific data reports that cardiac cells grown on CNT-modified scaffolds exhibit improved proliferation rates, have spontaneous and synchronous beating, have elongated morphology, and synthesize increased levels of differentiation markers, with better results obtained when scaffolds are of fibrous morphology and even furthermore improved when electrical stimulation is applied (Table 8) (Ashtari et al., 2019). These indicate the importance of combining multiple biofunctional cues in the regeneration of cardiac tissue. Most often, the improved performance of cardiac cells grown on CNT modified scaffolds can be attributed to an increased electrical conductivity, changes in nanoroughness and altered mechanical properties. Gorain et al. pointed out that presence of CNTs significantly enhances the scaffolds’ performance: improving both the mechanical and electrical properties, augmenting CM growth, proliferation, differentiation, maturation and functioning, synchronous beating, and enhancing remodeling of intercalated discs (Gorain et al., 2018). It has been pointed out that the addition of CNTs leads to the creation of biomaterial composites with electrical conductivity similar to the native myocardium (i.e., between 0.06 and 0.4 S/m (Miklavčič et al., 2006)). However, it is important to point out that it is not uncommon for scientists to use CNTs as-received, without providing any physicochemical data, and sometimes even lacking information about the manufacturer, or whether the materials used were SWCNTs or MWCNTs. This practice leads to large discrepancies in the obtained data and makes it hard to analyze and compare CNT CVD results. It is our strong belief that further progress in the field of CNT-modified cardiac tissue scaffolds will not be made until one is able to know exactly what kind of material was used, how it was synthesized, and what are its properties, as CNT performance is strongly dependent on its structure, agglomeration state, chemistry, type, and amount of functional groups.
In a different approach, McCauley et al. (McCauley et al., 2019) were able to fabricate a completely new material–a carbon nanotube fiber (CNTf) and used this as sutures for transmitting electrical stimulation between regions of the heart and across epicardial scar in sheep. Without evident cytotoxicity, the material was able to restore the beating function of the heart. This solution was suggested to provide long-term restorative strategies to pathologies that interrupt the efficient electrical transduction in electrically excitable tissue, including the heart.
8.2.4.3 Graphene
The successful fabrication of a single-layer graphene in 2004 by Konstantin Novoselov, Andre Geim and their team (Novoselov et al., 2004) opened up the gate to a plethora of possible applications in various fields of science and industry, including biomedical applications. Significant scientific interest stems from the material’s outstanding properties–most notably, a single layer of graphene is a 2D material, with extremely high mechanical and electrical properties. Traditionally, graphene can be obtained as a deposited layer during chemical vapor deposition, chemical decomposition or direct chemical synthesis processes, or in the form of free-standing flakes by mechanical or chemical exfoliation. More recent methods report unzipping of CNTs to form so-called graphene nanoribbons, or a microwave synthesis (Bhuyan et al., 2016). The biggest issue in working with graphene is its high tendency to agglomerate via pi-stacking which spontaneously recreates the structure of graphite. Thus, instead of working with single-layered graphene, one is in fact using a few-layers of graphite. To circumvent this issue, various techniques reducing the pi-pi attraction by introducing additional repulsive forces are employed, most notably chemical oxidation (Johnson et al., 2015). Indeed, most commercially available graphene is in fact, graphene oxide (GO), obtained by chemical exfoliation of graphite oxide, with functional groups evading re-organization into graphite.
Most scientists work with the as-obtained materials, pointing out better dispersability as factors enhancing graphene biocompatibility (Table 9). However, the electrical conductivity of GO may be insufficient for some applications and thus, some researchers decided to reduce GO to obtain reduced GO (rGO), indicating that this type of material should possess better electrical properties, and its physicochemical properties may be tuned to meet specific cell type requirements (Shin et al., 2016). In this regard, an interesting approach was used by Jo et al., who was able to harvest the positive effect of GO’s functional groups on nanoparticle dispersion, while fabricating a material of fairly good electrical conductivity (still lower than that of the native myocardium, 0.014 S/m compared to between 0.06 and 0.4 S/m) (Miklavčič et al., 2006; Jo et al., 2017). He did it by first preparing the polyacrylamide/GO composites and then mildly reducing them in ascorbic acid, which caused the transition of GO to rGO without compromising the material’s integrity and mechanical properties. Probably, two of the most important studies completed on the application of GO in cardiac tissue engineering include those by Zhao et al. (Zhao et al., 2018) and Bao et al. (Bao et al., 2017). The first indicates the importance of combining multiple biofunctional cues–fibrous morphology, electrical conductivity (obtained by covering the fibers with a graphene nanolayer), and electrical stimulation to enhance the adhesion, proliferation, differentiation, and maturation of stem cell-derived cardiomyocytes. Even though the fabrication of the GO layers could still be improved (specifically, improving the layer’s adhesion), the obtained results are truly remarkable with a significantly up-regulated expression of cardiac markers. Meanwhile, Bao’s study is one of the rare ones to report in vivo results, indicating that GO modified hydrogels were able to rebuild cardiac function, restoring functionality close to a healthy heart, as it was before the myocardial infraction (Bao et al., 2017).
9 Designing mechanical properties
Stem cell differentiation and cardiomyocyte beating behavior are known to be sensitive to scaffold rigidity and elasticity. In their research article from 2008 (Engler et al., 2008), Engler et al. proved that cardiomyocyte beating is inhibited if the matrix is too stiff (>50 kPa, stiffness of scar tissue), because cells are forced to perform work that exceeds their native limit. Meanwhile, if the scaffold is too soft (<1 kPa), the cells preserve their beating behavior, but do less work than they should in a healthy tissue. As Chopra et al. suggested, this may lead to the formation of disorganized actin networks and sarcomeres, causing a decrease in tissue functionality (Chopra et al., 2012). It is hypothesized that the optimal stiffness of the scaffold for cardiac tissue engineering is between 10 kPa–50 Pa, which is close to that of the healthy tissue. In these conditions, cardiomyocytes are able to form well organized sarcomeres, exhibiting actomyosin striation and 1-Hz beating (Engler et al., 2008; Chopra et al., 2012). Again, this stresses the importance of biomimetism in designing a material that can be used to successfully regenerate the damaged heart. Designing scaffold stiffness for steering cellular behavior is the subject of recent review article by Rajendran et al. (Rajendran et al., 2023), as well as the studies by Zhong et al. (Zhong et al., 2020) and Irawan et al. (Irawan et al., 2018). The readers are kindly referred to these excellent articles if more details on how the substrate’s mechanical properties affect cellular functions are needed.
In a 2012 review by Sheehy et al., the authors list the importance of mechanical cues in dictating stem cell fate during early development of the heart. It can, thus, be postulated that by mimicking mechanical properties of the heart ECM in the early development stages of heart tissue formation, tissue engineering scaffolds can induce the differentiation and maturation of cells, guiding them along the cardiac lineage. This has been found to be true for embryonic cardiomyocytes (Sheehy et al., 2012). Similar are the conclusions of a 2014 study by Hazeltine et al., reporting that substrate stiffness is the determinant in the early stage of human pluripotent stem cell differentiation, pushing the differentiation trajectory towards mesendoderm. The two most favorable stiffness values were 50 and 20 kPa. Interestingly, at later stages of cellular differentiation, this impact was found to be less pronounced (Hazeltine et al., 2014).
Most biomimetic biopolymers and hydrogels are too soft to fully mimic the mechanical properties of the native myocardium. To aid this issue, various approaches have been undertaken. One idea concerns preparing blends with other stiffer polymers, and this subject has already been raised in Sections 3.1 and 5.1 of this review article. Preferably, a mixture of conductive and non-conductive polymers could be obtained to render a final product more mechanically suitable and electrically conductive. The introduction of various additives (micro and nano) has also been found to improve the polymers’ mechanical properties, as already raised in Section 5 of this review, in particular for CNTs (Sirivisoot and Harrison, 2011; Ostrovidov et al., 2014; Ahadian et al., 2016; Ahadian et al., 2017; Dozois et al., 2017; Gorain et al., 2018) and GNPs (Li et al., 2016; Navaei et al., 2016). In all of these materials, the presence of the additives resulted in obtaining electrically conductive and mechanically biomimetic scaffolds, which significantly enhanced cell maturation. Using modifications that have such a dual function is certainly the most favorable option.
A third strategy to improve the material’s mechanical properties is to induce chemical changes to its structure, which is typically done by crosslinking. This has been employed in various studies, for example by Fu et al. in PDMS substrates (Fu et al., 2017), by Choi et al. on gelatin-PEG-tyramine co-polymers (Choi et al., 2017), or by Shi et al. in hyaluronic acid-based hydrogels (Shi et al., 2023). It is worth noting that the latter study employed the use of numerous modifications to provide the composite with various biofunctional properties: CNTs to enhance the electrical conductivity (sadly, the reported CNTs were of unknown origin and their final concentration in the polymer was not defined in the report) and mixing of biopolymers with synthetic polymers to further improve the mechanical properties.
In our opinion, the most optimal solution for culturing stem cell-derived CMs would be to use a scaffold with steerable or adaptable mechanical properties. At first, it should mimic the stiffness of the developing cardiac tissue (4–11 kPa), but should then become stiffer and stiffer, training the cells and forcing them to perform an increasing amount work, until it reached the limit of that in the adult native tissue. By this means, mechanical properties of the scaffold can induce and maintain the progressing cellular maturation, which is guided by the cellular contractile work (Ulmer et al., 2018).
There is an analogy between electrical conductivity of the scaffold and the benefits of electrical stimulation with mechanical properties of the substrate (most importantly, stiffness), and use of mechanical stimulation. The scaffold should be designed so as to mimic the natural ECM, i.e. the scaffold should be able to transmit electrical signals from cell to cell, and be able to support them mechanically. At the same time, exogenous impulses, such as electrostimulation and mechanostimulation, may be used to mimic the natural occurrences in the living organism, with ECM fibers moving and contracting, and electrical fields naturally present in vivo. Such stimuli had been proven to enhance cellular adhesion, proliferation, differentiation and maturation (Godier-Furnémont et al., 2015). Further benefits of using electrostimulation and mechanostimulation can be found in a review by W. L. Stoppel, D. L. Kaplan and L. D. Black III (Stoppel et al., 2016).
10 Electrostimulation (ES) and mechanostimulation (MS)
For the in vitro tissue engineering approach, ES using a carefully selected stimulation regime (time, amplitude, frequency, stimulation onset in the cell culture, etc.), has proven to enhance cellular adhesion, guide migration, enhance viability and proliferation, and provide differentiation cues for stem cells, by affecting various signaling pathways (Mooney et al., 2012; Kwon et al., 2016; Thrivikraman et al., 2016; Li et al., 2017b; Thrivikraman et al., 2018) (Table 10). In in vitro studies, external ES is aimed to mimic endogenous electrical fields that naturally occur in vivo and are responsible for multiple phenomena including tissue regeneration. Considering mimicking the maturation of the heart in vitro, ES at first can be used to alter the cellular metabolism and then, mimic the signals from the pacemaker cells. By mimicking the signal from the pacemaker cells, exogenous ES can alter the cellular physiology, forcing them to switch from spontaneous, unsynchronized pacing, to synchronized pacing triggered only by external factors. In their extensive review from 2018, Thrivikraman, Boda and Basu identified the major effects of ES stimulation on stem cells, suggesting different mechanisms of interactions: alteration of biochemical signaling pathways, calcium diffusion transients, induced cytoskeletal reorganization, ATP synthesis, reactive oxygen species generation and synthesis of heat shock proteins (Thrivikraman et al., 2018).
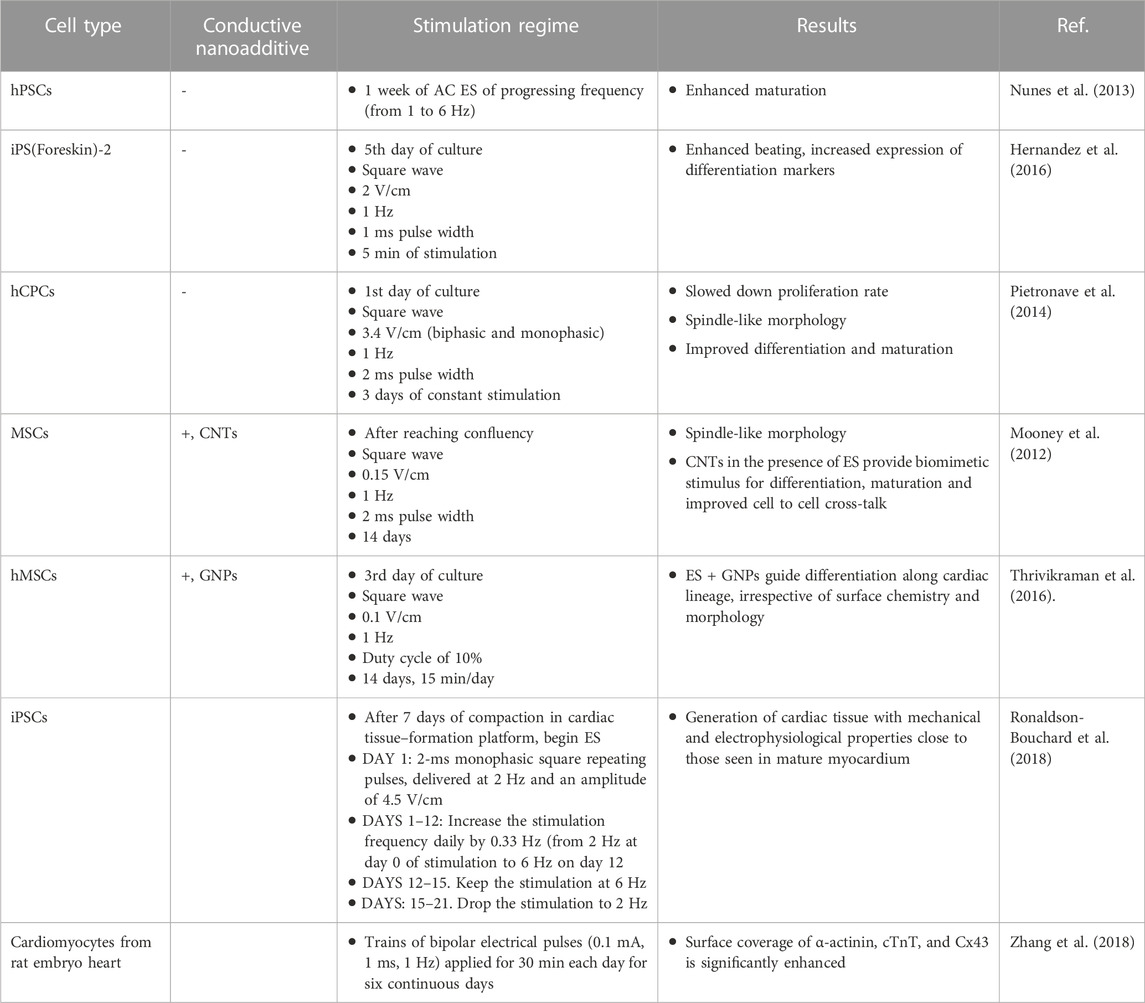
TABLE 10. The influence of electrical stimulation on cellular proliferation, maturation and differentiation along the cardiac cell lineage from different stem cells including mesenchymal stem cells.
In the heart, it is anticipated that direct current (DC) stimulation is able to mimic the electrical signals found in early tissue development stage, affecting cellular migration and morphology, while an attenuated current (AC) signal can mirror the reactions occurring in the adult heart, dictating the fate of the stem cell niche, affecting cardiomyocyte beating behavior, influencing assembly of sarcomeres and myotubes. Thus, in planning ES experiments and selecting a suitable stimulation regime, cell type and desired outcomes should be carefully considered. Currently, a majority of the studies report AC stimulation, most often, square-wave, with a frequency of 1–2 Hz, pulse duration of 1–2 ms and amplitude between 0.1–10 V/cm (Tandon et al., 2009; Thrivikraman et al., 2018). Nunes found that 1 week of ES stimulation of progressing frequency (from 1 to 6 Hz) is able to enhance cardiomyocyte differentiation that were different from human pluripotent stem cells (hPSCs) and cultured in a specially designed 3D construct, Biowire (Nunes et al., 2013). Hernandez and his team reported that cardiomyocytes differentiated from induced pluripotent stem cells (iPS(Foreskin)-2) as demonstrated by enhanced beating, increased expression of differentiation markers, etc. under AC ES (Hernandez et al., 2016). Meanwhile, Pietronave and his team researched human cardiac progenitor cells (hCPCs) and studied the impact of both biphasic and monophasic AC ES on cellular morphology, proliferation, viability and differentiation. While ES did not affect cellular viability, proliferation rate slowed down, which was accompanied with adopting of spindle-like morphology, improved differentiation and maturation (Pietronave et al., 2014). Thus, AC ES was found to improve differentiation and maturation efficiency of cardiomyocyte progenitor cells from different sources.
The synergistic effect of conductive nanoparticles and ES were studied with very promising results. In 2012, Mooney and co-workers studied effect of the presence of COOH–functionalized SWCNTs, both in the medium and in a PLA fibrous scaffold, on the differentiation and maturation of ES-stimulated mesenchymal stem cells (MSC). She had found that electrical stimulation alone was able to force cellular alignment, with a more pronounced effect observed in the presence of CNTs. Furthermore, the CNTs were found to provide a biomimetic stimulus for MSC differentiation, accelerate their maturation and improve cell to cell cross-talk (Mooney et al., 2012). Similar results were also reported by Thrivikraman and his co-workers, who worked with GNPs. The team found that AC electrical stimulation combined with GNPs internalized by cells was able to guide the differentiation of human mesenchymal stem cells (hMSCs) along a cardiac-like lineage without the use of any exogenous growth factor even on the surface of a glass coverslip (Thrivikraman et al., 2016). Because in these studies mesenchymal cells are used instead of actual stem cells, the obtained results should be treated as a guidance for cell culture conditions that may be favorable for cardiac-like cells. These studies point out the beneficial impact of synergy between conductive nanoparticles and electrical stimulation in promoting cellular differentiation into the cardiac lineage.
Some advantages of employing MS can be found in a recent study by Zhao et al. (2023). The benefits of combining MS with ES in vitro cultures of cardiac cells were analyzed in-depth in the 2016 review by Stoppel et al. (2016) and the readers are kindly referred to this excellent study for more details on the rationale, benefits and possible ways to employ ES and MS, both separately and in combination. It is worth noting that combining ES and MS is justified from the biological point of view as it mimics the natural environment of cardiac cells. Various approaches can be taken to induce both stimulants, both separately and concurrently, as presented in the two aforementioned reviews. One possibility, as suggested by Gouveia et al., is the use of piezoelectric materials that would spontaneously generate ES while under mechanical stress and vice versa. This simple idea yielded some promising results: cell attachment and alignment promoted, ratio of cell populations maintained, cell-cell communication and metabolic maturation enhanced, and cardiomyocyte (CM) contractility maintained for at least 12 days. Sadly, the study did not investigate whether or not such a system could induce or enhance the differentiation and/or maturation of juvenile cells and instead focused on maintaining the viability of mature cardiomyocytes. Hence, further studies are needed to verify any possible positive outcomes in these applications (Gouveia et al., 2017).
Apart from using the piezoelectric materials, methods of delivering MS into a system can be grouped into 3 major categories (Liaw and Zimmermann, 2016), as seen in Figure 10. The first one, also referred to as isotonic contractions, is achieved by physically stretching the cell culture. This can be done either by stretching the entire cell well (or only its bottom), or by seeding the cells on or around pillars which then can be moved inside the culture. Stretching of the cell well as a whole is employed in a commercially available C-Stretch system from IonOptix, wherein displacement of the cell well holders can cause cyclic stretch. The same idea can also be achieved by stretching of the elastic cell well bottom by changing pressure in the gas or liquid filled chambers located below it (Cortes et al., 2020; Correia Carreira et al., 2021; Zhao et al., 2023). Alternatively, specially designed poles can be placed inside the cell wells or at its bottom and the moved by some sort of physical force–mechanic or magnetic (Zhao et al., 2023).
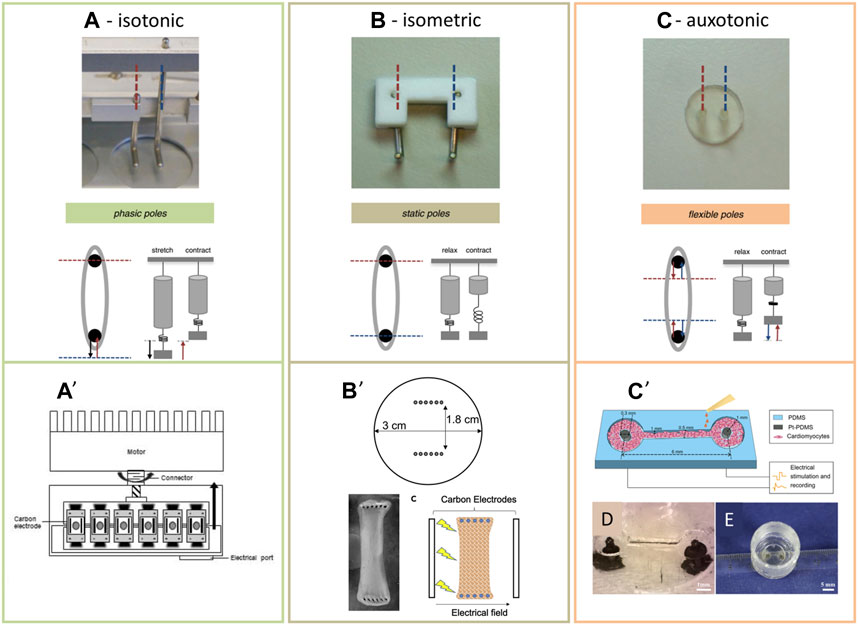
FIGURE 10. (A–C) different modes of mechanical stimulation, as presented by Liaw and Zimmermann (Liaw and Zimmermann, 2016), combined with their practical realizations, wherein MS is combined with ES: (A′): C-stretch system from IonOptix, as presented by Kroll et al. (Kroll et al., 2017); (B′): a layer-by-layer assembly of cells placed on top of two arrays of stiff needles. ES was employed by immersing two counter electrodes, perpendicular to the immersing two counter electrodes, perpendicular to the needle axes (C-pace system) (Pretorius et al., 2022); (C’—E): the cells are seeded inside the specifically shaped channels, which force their alignment around the elastic electrodes that can be used for electrical stimulation (Zhang et al., 2018). (A–C): N.Y. Liaw, W.-H. Zimmermann, Mechanical stimulation in the engineering of heart muscle, Advanced Drug Delivery Reviews 96 (2016) 156–160 https://doi.org/10.1016/j.addr.2015.09.001 with permission from Elsevier, Copyright 2016. (A′) Reprinted from Kroll et al. (2017) with permission from Elsevier, Copyright 2017. (B′) Reprinted from Pretorius et al. (2022), with permission form Elsevier, Copyright 2022 (CC BY-NC-ND). (C’—E) Reprinted from Zhang et al. (2018) with permission from Elsevier, Copyright 2018.
The second and third methods of introducing MS operate without any exogenous force that introduces the stretch and instead, the cells and the compacted scaffolds or ECM themselves are the source of the strain. These systems, referred to as isotonic and auxotonic contractions by Liaw and Zimmermann (Figure 10) (Liaw and Zimmermann, 2016), require arranging the cell and scaffolds into compact and organized tissues around specifically designed poles. When such poles are made of stiff materials, the arrangement and densification of the matrix introduces isometric strain into the culture. However, when such poles are fabricated from elastic materials, such as polydimethylsiloxane (PDMS), arrangement of a dense, tissue-like structure, combined with its self-pacing, introduce auxotonic contractions into the system. This is a very smart and elegant approach in which the cells generate the MS by themselves.
An example of employing the isotonic stretch along with ES can be found in a study by Pretorius et al. (Pretorius et al., 2022). A 2.1 mm thick cell sheet, fabricated by assembling 3 consecutive layers of juvenile cardiomyocytes inside the fibrin hydrogels, was stretched by being pierced between 2 sets of surgical needles (each composed of 6 needles, set 1 mm apart). The two sets created parallel lines, facing each other at a 18 mm distance. ES of two amplitudes (namely, 15 V and 22 V) was then employed (2 ms pulses at a 2 Hz frequency) perpendicularly to the needles, by using the C-pace system. It was observed that double stimulation (ES + MS) significantly enhances the maturation of the iPSC–derived CMs, which was further boosted by using a higher amplitude of voltage. After 10 days of culture, the CMs in this group had sarcomeres of physiologically relevant lengths (above 1.9 µm), with biochemical maturation markers significantly elevated. The authors suggested that positive outcomes could be further boosted by using a co-culture with cardiac fibroblasts, which we find probable. In our opinion, the observed effects could also be improved if the ES and MS were not perpendicular to one another and parallel instead. Moreover, we found that study lacks an important experimental detail, namely, in the ES protocol, the length of stimulation is not provided. Further, environmentally-friendly materials should be used.
Auxotonic systems are probably amongst the most popular solutions used to combine ES with MS. Some examples of these can be found in the studies done by the teams of Vunjak-Novakovic and Radisic (Nunes et al., 2013; Ronaldson-Bouchard et al., 2018; Ronaldson-Bouchard et al., 2019; Zhao et al., 2019), wherein protocols for enhancing the maturation of cardiomyocytes are developed. One version of the system, Biowire II, progressed into industry (originally as TARA biosystems, currently as a part of Valo Health) offering the first-of-its-kind cardiovascular screening platform (Nunes et al., 2013; Zhao et al., 2019). In what seems to be a new approach to the same problem (Ronaldson-Bouchard et al., 2018; Ronaldson-Bouchard et al., 2019), two elastic poles were used to induce auxotonic stretch to a CM–fibroblast co-culture in a fibrin hydrogel, and ES was induced parallel to the MS. The ES was conducted in the mode of “intensity training” (2 weeks at a frequency increasing from 2 Hz to 6 Hz by 0.33 Hz per day, followed by 1 week at 2 Hz, with voltage set to 4.5 V/cm). As a result, the speed of cellular maturation was enhanced three-fold, with sarcomere lengths exceeding 2.2 µm, the density of mitochondria was improved by 30%, and many important biochemical markers were also significantly elevated. These important studies suggest the need of employing a combinatorial approach which involves the use of different stimulants, biomaterials, and co-cultures in obtaining mature tissue fragments in vitro. Apart from the simplicity of using the auxotonic systems in introducing mechanical stretch, another advantage lies in the fact that the displacement of elastic poles can be used to monitor the pacing of the maturating cultures, as demonstrated in the studies by Legant et al. (Legant et al., 2009) and by Ma et al. (Ma et al., 2019). The simplicity and versatility of this strategy contributes to the fact that right now, auxotonic systems are the go-to methods for fabricating maturated tissue constructs out of iPSCs-derived cardiomyocytes.
A combination of auxotonic and isotonic stress applied to the system can be found in a study by Leonard et al. (Leonard et al., 2018). In what the authors refer to as the afterload simulator, a tissue construct made of iPSC-CMs embedded inside the fibrin gel was compacted around two posts. One post was made out of a stiff material, while the other had a varied elasticity. Pacing of the culture with ES would cause tissue contraction and displacement of the post which then resisted tissue relaxation–thus creating an “afterload” condition. It is worth noting that the authors tested different stiffnesses of their system to verify the optimal afterload for CM maturation. It was found that the contractile function was enhanced at moderate (0.45 μN/μm) afterload values and plateaued at higher values (1.2 μN/μm and 9.2 μN/μm). The structural maturation of cells improved on all of the “stressed” cultures, and to a similar extent regardless of the post stiffness. At the same time, markers of functional (calcium transient kinetic) and biochemical cellular maturation (increased expression of mature CM genes) were enhanced as a function of increasing afterload, but the same was also observed for pathological hypertrophy markers. Hence, it was suggested that an optimal value of the afterload (1.2 μN/μm) exists and that it can be used to speed up CM maturation.
11 Summary
Like any other tissue of a living organism, cardiac tissue is a complex structure, stemming from the different functions it performs. Unlike any other tissue, it has almost zero regenerative potential, due to the absence of the stem cell niche. This inability to self-regenerate, combined with frequency of CVDs, and absolute essentiality of this organ, create an absolute necessity to study the development of pathogenic diseases and engineer novel regeneration strategies. Even though it is now relatively facile to obtain cardiomyocytes from patient-specific iPSCs, there is still a long way to travel before these can be translated to medicine. The most important hurdle is an inability to create tissues of a mature phenotype in vitro, and in a relatively short time–i.e., without consuming excessive resources or increasing the risk of failure.
The current socioeconomic and ecological impact of CVDs stems from two factors: first is their prevalence in the modern society and second is the overall cost of research involving their pathogenesis and regeneration. Numerous strategies suggest creating mature tissue constructs in vitro, which could be used in treating damaged tissue in vivo, in studying the development of various diseases and their treatment, or in evaluating the cardiotoxicity of drugs. Such strategies could successfully reduce the current carbon footprint associated with treating CVDs. Moreover, we need to take a fresh look at the materials used for treating CVD as many involve polymers and/or other materials which use environmentally toxic agents. In vitro and in vivo testing also involves the use of tremendous amounts of polymers which are not environmentally-friendly. Designing solutions that would reduce the time needed for cell culture to develop a mature phenotype could significantly reduce the amount of resources and consumables–this is important, because current procedures often require culturing the cells for more than 1 year. Even though significant progress has been made on this subject in the recent years, a perfect solution has yet to be developed. Emphasis must be placed on using new, green-derived, sustainable, and biodegradable materials not just for CVD but across all of biomaterials. These include animal, plant and microorganism-derived materials, most notably, collagen.
Knowledge about the complexity of cardiac tissue, various interactions between different cell types, and between cells and the cardiac tissue ECM, point out directions in which the field should be evolving. The combination of biomimetic scaffolds with biological and physical cues has already yielded impressive results. For example, collagen scaffolds were successfully used for co-culturing CMs with fibroblasts, while introducing exogenous electrical stimulation (e.g. (Ronaldson-Bouchard et al., 2018; Ronaldson-Bouchard et al., 2019; Zhao et al., 2019)). Still, no single solution exists to mimic all the interactions from within living tissues. It can be suggested that a combination of a majority of factors is the only route to obtaining fully mature cardiac tissues in vitro, which could then be used for drug screening, various pathogeneses, and cardiac regeneration in vivo. Among different possibilities, we possibilities, we believe following approaches are the most promising to both fabricate improved CVD biomaterials and save the environment:
1) Use scaffolds that are biomimetic to the native cardiac tissue ECM (both in their chemistry, electrical and mechanical properties and morphology), preferably, fibrous scaffolds made of collagen type I, perhaps mixed in-mass, or surface-modified with laminin, elastin or fibrin;
2) In-mass modification with various additives that alter the scaffold’s mechanical and electrical properties, thus making it mimetic to the native cardiac tissue ECM. In our opinion, fibrous morphology and benign chemistry of environmentally-friendly carbon nanotubes make them perfect candidates in this matter. Furthermore, when these are short and highly functionalized, high biodegradability can be achieved;
3) In-mass modification of scaffolds with bioactive molecules for their controllable release. The most promising ones include: albumin-modified fatty acids (which could force a switch in the CM metabolic pathway), triiodothyronine (inducing a switch from fetal N2BA to an adult N2B titin isoform) and dexamethasone (both of which affect the CM electrophysiology, and IGF-1 (to induce the hyperplastic growth)) (Martyniak et al., 2023). Preferably, these should be released in a switchable manner, so as to mimic their natural occurrence within the developing and maturing heart;
4) A co-culture with cardiac fibroblasts which could aid in scaffold remodeling and secrete specific signaling molecules that further drive the CM maturation;
5) Introducing exogenous electrical and mechanical stimulation which could “train” the cardiac tissue, thus forcing CM maturation; and
6) Finally, in our opinion, CM culture should be dynamic–the mechanical and electrical properties of the growing tissue should be controllable. Tricking the cells that their surroundings are becoming mature is a known driving force of inducing faster cellular maturation.
Author contributions
AB: Conceptualization, Funding acquisition, Resources, Visualization, Writing–original draft, Writing–review and editing. TW: Writing–review and editing.
Funding
The author(s) declare financial support was received for the research, authorship, and/or publication of this article. Work by AB is supported by the National Centre for Research and Development under Grant No. LIDER/7/0020/L-11/19/NCBR/2020.
Acknowledgments
We thank Ebrahim Mostafavi for fruitful discussions on the scope of this review article.
Conflict of interest
The authors declare that the research was conducted in the absence of any commercial or financial relationships that could be construed as a potential conflict of interest.
Publisher’s note
All claims expressed in this article are solely those of the authors and do not necessarily represent those of their affiliated organizations, or those of the publisher, the editors and the reviewers. Any product that may be evaluated in this article, or claim that may be made by its manufacturer, is not guaranteed or endorsed by the publisher.
References
Abedi, A., Hasanzadeh, M., and Tayebi, L. (2019). Conductive nanofibrous Chitosan/PEDOT:PSS tissue engineering scaffolds. Mater. Chem. Phys. 237, 121882. doi:10.1016/j.matchemphys.2019.121882
Adolfsson, K. H., Golda-Cepa, M., Erdal, N. B., Duch, J., Kotarba, A., and Hakkarainen, M. (2019). Importance of surface functionalities for antibacterial properties of carbon spheres. Adv. Sustain. Syst. 3 (4), 1800148. doi:10.1002/adsu.201800148
Ahadian, S., Davenport Huyer, L., Estili, M., Yee, B., Smith, N., Xu, Z., et al. (2017). Moldable elastomeric polyester-carbon nanotube scaffolds for cardiac tissue engineering. Acta Biomater. 52, 81–91. doi:10.1016/j.actbio.2016.12.009
Ahadian, S., Yamada, S., Ramón-Azcón, J., Estili, M., Liang, X., Nakajima, K., et al. (2016). Hybrid hydrogel-aligned carbon nanotube scaffolds to enhance cardiac differentiation of embryoid bodies. Acta Biomater. 31, 134–143. doi:10.1016/j.actbio.2015.11.047
Amano, Y., Nishiguchi, A., Matsusaki, M., Iseoka, H., Miyagawa, S., Sawa, Y., et al. (2016). Development of vascularized iPSC derived 3D-cardiomyocyte tissues by filtration Layer-by-Layer technique and their application for pharmaceutical assays. Acta Biomater. 33, 110–121. doi:10.1016/j.actbio.2016.01.033
Asaro, G. A., Solazzo, M., Suku, M., Spurling, D., Genoud, K., Gonzalez, J. G., et al. (2023). MXene functionalized collagen biomaterials for cardiac tissue engineering driving iPSC-derived cardiomyocyte maturation. npj 2D Mater. Appl. 7 (1), 44. doi:10.1038/s41699-023-00409-w
Ashtari, K., Nazari, H., Ko, H., Tebon, P., Akhshik, M., Akbari, M., et al. (2019). Electrically conductive nanomaterials for cardiac tissue engineering. Adv. Drug Deliv. Rev. 144, 162–179. doi:10.1016/j.addr.2019.06.001
Asiri, A. M., Marwani, H. M., Khan, S. B., and Webster, T. J. (2014). Greater cardiomyocyte density on aligned compared with random carbon nanofibers in polymer composites. Int. J. Nanomedicine 9, 5533–5539. doi:10.2147/IJN.S71587
Aziz, A. A., Bakar, S. A., and Rusop, M. (2011). Carbon nanostructured materials, carbon and oxide nanostructures: Synthesis, characterisation and applications. Berlin, Heidelberg: Springer, 165–193.
Baei, P., Jalili-Firoozinezhad, S., Rajabi-Zeleti, S., Tafazzoli-Shadpour, M., Baharvand, H., and Aghdami, N. (2016). Electrically conductive gold nanoparticle-chitosan thermosensitive hydrogels for cardiac tissue engineering. Mater. Sci. Eng. C 63, 131–141. doi:10.1016/j.msec.2016.02.056
Balint, R., Cassidy, N. J., and Cartmell, S. H. (2014). Conductive polymers: Towards a smart biomaterial for tissue engineering. Acta Biomater. 10 (6), 2341–2353. doi:10.1016/j.actbio.2014.02.015
Bao, R., Tan, B., Liang, S., Zhang, N., Wang, W., and Liu, W. (2017). A π-π conjugation-containing soft and conductive injectable polymer hydrogel highly efficiently rebuilds cardiac function after myocardial infarction. Biomaterials 122, 63–71. doi:10.1016/j.biomaterials.2017.01.012
Bashandeh, K., Amiri, A., Rafieerad, A., Rahman, S., Yan, W., Dhingra, S., et al. (2022). MXene-aromatic thermosetting copolyester nanocomposite as an extremely wear-resistant biocompatible implant material for osteoarthritis applications. Appl. Surf. Sci. 600, 154124. doi:10.1016/j.apsusc.2022.154124
Benko, A., Medina-Cruz, D., Vernet-Crua, A., O’Connell, C. P., Świętek, M., Barabadi, H., et al. (2021). Nanocarrier drug resistant tumor interactions: novel approaches to fight drug resistance in cancer. Cancer Drug Resist. 4 (2), 264–297. doi:10.20517/cdr.2020.81
Benko, A., Nocuń, M., Gajewska, M., and Błażewicz, M. (2019). Addition of carbon nanotubes to electrospun polyacrylonitrile as a way to obtain carbon nanofibers with desired properties. Polym. Degrad. Stab. 161, 260–276. doi:10.1016/j.polymdegradstab.2019.01.033
Benko, A., Reczyńska-Kolman, K., Medina-Cruz, D., Cholula-Diaz, J. L., O’Connell, C., Truong, L. B., et al. (2023). “Chapter 2 - nanomaterials to aid wound healing and infection control,” in Antimicrobial activity of nanoparticles. Editor G. Guisbiers (Amsterdam, Netherlands: Elsevier), 19–67.
Benko, A., Truong, L. B., Medina-Cruz, D., Mostafavi, E., Cholula-Díaz, J. L., and Webster, T. J. (2022). “Chapter 11 - green nanotechnology in cardiovascular tissue engineering,” in Tissue engineering. Editors C. P. Sharma, T. Chandy, V. Thomas, and F. G. Thankam (United States: Academic Press), 237–281.
Bertuoli, P. T., Ordoño, J., Armelin, E., Pérez-Amodio, S., Baldissera, A. F., Ferreira, C. A., et al. (2019). Electrospun conducting and biocompatible uniaxial and core–shell fibers having poly(lactic acid), poly(ethylene glycol), and polyaniline for cardiac tissue engineering. ACS Omega 4 (2), 3660–3672. doi:10.1021/acsomega.8b03411
Bhattacharya, K., Mukherjee, S. P., Gallud, A., Burkert, S. C., Bistarelli, S., Bellucci, S., et al. (2016). Biological interactions of carbon-based nanomaterials: From coronation to degradation. Nanomedicine Nanotechnol. Biol. Med. 12 (2), 333–351. doi:10.1016/j.nano.2015.11.011
Bhuyan, M. S. A., Uddin, M. N., Islam, M. M., Bipasha, F. A., and Hossain, S. S. (2016). Synthesis of graphene. Int. Nano Lett. 6 (2), 65–83. doi:10.1007/s40089-015-0176-1
Bian, W., Badie, N., Himel, H. D., and Bursac, N. (2014). Robust T-tubulation and maturation of cardiomyocytes using tissue-engineered epicardial mimetics. Biomaterials 35 (12), 3819–3828. doi:10.1016/j.biomaterials.2014.01.045
Blazeski, A., Lowenthal, J., Zhu, R., Ewoldt, J., Boheler, K. R., and Tung, L. (2019). Functional properties of engineered heart slices incorporating human induced pluripotent stem cell-derived cardiomyocytes. Stem Cell Rep. 12 (5), 982–995. doi:10.1016/j.stemcr.2019.04.002
Boffito, M., Di Meglio, F., Mozetic, P., Giannitelli, S. M., Carmagnola, I., Castaldo, C., et al. (2018). Surface functionalization of polyurethane scaffolds mimicking the myocardial microenvironment to support cardiac primitive cells. PloS one 13 (7), e0199896. doi:10.1371/journal.pone.0199896
Bonzani, I. C., George, J. H., and Stevens, M. M. (2006). Novel materials for bone and cartilage regeneration. Curr. Opin. Chem. Biol. 10 (6), 568–575. doi:10.1016/j.cbpa.2006.09.009
Bose, P., Huang, C. Y., Eyckmans, J., Chen, C. S., and Reich, D. H. (2018). “Fabrication and mechanical properties measurements of 3D microtissues for the study of cell–matrix interactions,” in The surfaceome: Methods and protocols. Editors K. R. Boheler, and R. L. Gundry (New York, NY: Springer New York), 303–328.
Boyd, D. B., and Slanina, Z. (2001). Introduction and foreword to the special issue commemorating the thirtieth anniversary of Eiji Osawa’s C60 paper. J. Mol. Graph. Model. 19 (2), 181–184. doi:10.1016/s1093-3263(00)00106-6
Bussy, C., Hadad, C., Prato, M., Bianco, A., and Kostarelos, K. (2016). Intracellular degradation of chemically functionalized carbon nanotubes using a long-term primary microglial culture model. Nanoscale 8 (1), 590–601. doi:10.1039/c5nr06625e
Capulli, A. K., MacQueen, L. A., Sheehy, S. P., and Parker, K. K. (2016). Fibrous scaffolds for building hearts and heart parts. Adv. Drug Deliv. Rev. 96, 83–102. doi:10.1016/j.addr.2015.11.020
Chang, T., Liu, C., Yang, H., Lu, K., Han, Y., Zheng, Y., et al. (2022). Fibrin-based cardiac patch containing neuregulin-1 for heart repair after myocardial infarction. Colloids Surfaces B Biointerfaces 220, 112936. doi:10.1016/j.colsurfb.2022.112936
Chatterjee, N., Yang, J., Kim, S., Joo, S. W., and Choi, J. (2016). Diameter size and aspect ratio as critical determinants of uptake, stress response, global metabolomics and epigenetic alterations in multi-wall carbon nanotubes. Carbon 108, 529–540. doi:10.1016/j.carbon.2016.07.031
Chen, M.-C., Sun, Y.-C., and Chen, Y.-H. (2013). Electrically conductive nanofibers with highly oriented structures and their potential application in skeletal muscle tissue engineering. Acta Biomater. 9 (3), 5562–5572. doi:10.1016/j.actbio.2012.10.024
Choi, M.-Y., Kim, J.-T., Lee, W.-J., Lee, Y., Park, K. M., Yang, Y.-I., et al. (2017). Engineered extracellular microenvironment with a tunable mechanical property for controlling cell behavior and cardiomyogenic fate of cardiac stem cells. Acta Biomater. 50, 234–248. doi:10.1016/j.actbio.2017.01.002
Chopra, A., Lin, V., McCollough, A., Atzet, S., Prestwich, G. D., Wechsler, A. S., et al. (2012). Reprogramming cardiomyocyte mechanosensing by crosstalk between integrins and hyaluronic acid receptors. J. Biomechanics 45 (5), 824–831. doi:10.1016/j.jbiomech.2011.11.023
Cole, M. A. (1956). Manufacture of conductive plastics. Available at: https://patents.google.com/patent/US2761854A/en?oq=US2761854A.
Correia Carreira, S., Taghavi, M., Pavez Loriè, E., and Rossiter, J. (2021). FleXert: A soft, actuatable multiwell plate insert for cell culture under stretch. ACS Biomaterials Sci. Eng. 7 (6), 2225–2245. doi:10.1021/acsbiomaterials.0c01448
Cortes, D., McTiernan, C. D., Ruel, M., Franco, W., Chu, C., Liang, W., et al. (2020). BEaTS-α an open access 3D printed device for in vitro electromechanical stimulation of human induced pluripotent stem cells. Sci. Rep. 10 (1), 11274. doi:10.1038/s41598-020-67169-1
Davis, M. E., Hsieh, P. C., Takahashi, T., Song, Q., Zhang, S., Kamm, R. D., et al. (2006). Local myocardial insulin-like growth factor 1 (IGF-1) delivery with biotinylated peptide nanofibers improves cell therapy for myocardial infarction. Proc. Natl. Acad. Sci. U. S. A. 103 (21), 8155–8160. doi:10.1073/pnas.0602877103
Delgado, J. L., Herranz, M. a. A., and Martin, N. (2008). The nano-forms of carbon. J. Mater. Chem. 18 (13), 1417–1426. doi:10.1039/b717218d
Dozois, M. D., Bahlmann, L. C., Zilberman, Y., and Tang, X. (2017). Carbon nanomaterial-enhanced scaffolds for the creation of cardiac tissue constructs: A new frontier in cardiac tissue engineering. Carbon 120, 338–349. doi:10.1016/j.carbon.2017.05.050
Duch, J., Golda-Cepa, M., and Kotarba, A. (2019). Evaluating the effect of oxygen groups attached to the surface of graphenic sheets on bacteria adhesion: The role of the electronic factor. Appl. Surf. Sci. 463, 1134–1140. doi:10.1016/j.apsusc.2018.08.237
Dvir, T., Timko, B. P., Kohane, D. S., and Langer, R. (2010). Nanotechnological strategies for engineering complex tissues. Nat. Nanotechnol. 6, 13–22. doi:10.1038/nnano.2010.246
Dykman, L. A., and Khlebtsov, N. G. (2011). Gold nanoparticles in biology and medicine: Recent advances and prospects. Acta naturae 3 (2), 34–55. doi:10.32607/20758251-2011-3-2-34-56
Elias, K. L., Price, R. L., and Webster, T. J. (2002). Enhanced functions of osteoblasts on nanometer diameter carbon fibers. Biomaterials 23 (15), 3279–3287. doi:10.1016/s0142-9612(02)00087-x
Engler, A. J., Carag-Krieger, C., Johnson, C. P., Raab, M., Tang, H. Y., Speicher, D. W., et al. (2008). Embryonic cardiomyocytes beat best on a matrix with heart-like elasticity: scar-like rigidity inhibits beating. J. Cell Sci. 121 (22), 3794–3802. doi:10.1242/jcs.029678
Eres, G., Geohegan, D. B., Puretzky, A. A., and Rouleau, C. M. (2010). “All carbon nanotubes are not created equal,” in Nanotechnology for electronics, photonics, and renewable energy. Editors A. Korkin, S. P. Krstić, and C. J. Wells (New York: Springer), 131–152.
Fernandes, E. G. R., Zucolotto, V., and De Queiroz, A. A. A. (2010). Electrospinning of hyperbranched poly-L-lysine/polyaniline nanofibers for application in cardiac tissue engineering. J. Macromol. Sci. Part A 47 (12), 1203–1207. doi:10.1080/10601325.2010.518847
Fleischer, S., Shapira, A., Feiner, R., and Dvir, T. (2017). Modular assembly of thick multifunctional cardiac patches. Proc. Natl. Acad. Sci. 114 (8), 1898–1903. doi:10.1073/pnas.1615728114
Fraczek-Szczypta, A. (2014). Carbon nanomaterials for nerve tissue stimulation and regeneration. Mater. Sci. Eng. C 34, 35–49. doi:10.1016/j.msec.2013.09.038
Fu, J., Chuah, Y. J., Ang, W. T., Zheng, N., and Wang, D.-A. (2017). Optimization of a polydopamine (PD)-based coating method and polydimethylsiloxane (PDMS) substrates for improved mouse embryonic stem cell (ESC) pluripotency maintenance and cardiac differentiation. Biomaterials Sci. 5 (6), 1156–1173. doi:10.1039/c7bm00266a
Fujita, B., and Zimmermann, W.-H. (2017). Engineered heart repair. Clin. Pharmacol. Ther. 102 (2), 197–199. doi:10.1002/cpt.724
Gajendiran, M., Choi, J., Kim, S.-J., Kim, K., Shin, H., Koo, H.-J., et al. (2017). Conductive biomaterials for tissue engineering applications. J. Industrial Eng. Chem. 51, 12–26. doi:10.1016/j.jiec.2017.02.031
Ganji, Y., Li, Q., Quabius, E. S., Böttner, M., Selhuber-Unkel, C., and Kasra, M. (2016). Cardiomyocyte behavior on biodegradable polyurethane/gold nanocomposite scaffolds under electrical stimulation. Mater. Sci. Eng. C 59, 10–18. doi:10.1016/j.msec.2015.09.074
Garibaldi, S., Brunelli, C., Bavastrello, V., Ghigliotti, G., and Nicolini, C. (2005). Carbon nanotube biocompatibility with cardiac muscle cells. Nanotechnology 17 (2), 391–397. doi:10.1088/0957-4484/17/2/008
Gelmi, A., Cieslar-Pobuda, A., de Muinck, E., Los, M., Rafat, M., and Jager, E. W. (2016). Direct mechanical stimulation of stem cells: A beating electromechanically active scaffold for cardiac tissue engineering. Adv. Healthc. Mater 5 (12), 1471–1480. doi:10.1002/adhm.201600307
Gentillon, C., Li, D., Duan, M., Yu, W.-M., Preininger, M. K., Jha, R., et al. (2019). Targeting HIF-1α in combination with PPARα activation and postnatal factors promotes the metabolic maturation of human induced pluripotent stem cell-derived cardiomyocytes. J. Mol. Cell. Cardiol. 132, 120–135. doi:10.1016/j.yjmcc.2019.05.003
Godier-Furnémont, A. F. G., Tiburcy, M., Wagner, E., Dewenter, M., Lämmle, S., El-Armouche, A., et al. (2015). Physiologic force-frequency response in engineered heart muscle by electromechanical stimulation. Biomaterials 60, 82–91. doi:10.1016/j.biomaterials.2015.03.055
Gonçalves, G. A. B., Marques, P., and Vila, P. (2016). Graphene-based materials in health and environment. Berlin, Heidelberg: Springer International Publishing.
Gorain, B., Choudhury, H., Pandey, M., Kesharwani, P., Abeer, M. M., Tekade, R. K., et al. (2018). Carbon nanotube scaffolds as emerging nanoplatform for myocardial tissue regeneration: A review of recent developments and therapeutic implications. Biomed. Pharmacother. 104, 496–508. doi:10.1016/j.biopha.2018.05.066
Gouveia, P. J., Rosa, S., Ricotti, L., Abecasis, B., Almeida, H. V., Monteiro, L., et al. (2017). Flexible nanofilms coated with aligned piezoelectric microfibers preserve the contractility of cardiomyocytes. Biomaterials 139, 213–228. doi:10.1016/j.biomaterials.2017.05.048
Guex, A. G., Frobert, A., Valentin, J., Fortunato, G., Hegemann, D., Cook, S., et al. (2014). Plasma-functionalized electrospun matrix for biograft development and cardiac function stabilization. Acta Biomater. 10 (7), 2996–3006. doi:10.1016/j.actbio.2014.01.006
Guiseppi-Elie, A. (2010). Electroconductive hydrogels: Synthesis, characterization and biomedical applications. Biomaterials 31 (10), 2701–2716. doi:10.1016/j.biomaterials.2009.12.052
Guo, Y., Yu, Y., Hu, S., Chen, Y., and Shen, Z. (2020). The therapeutic potential of mesenchymal stem cells for cardiovascular diseases. Cell Death Dis. 11 (5), 349. doi:10.1038/s41419-020-2542-9
Hardy, J. G., Lee, J. Y., and Schmidt, C. E. (2013). Biomimetic conducting polymer-based tissue scaffolds. Curr. Opin. Biotechnol. 24 (5), 847–854. doi:10.1016/j.copbio.2013.03.011
Hastings, C. L., Roche, E. T., Ruiz-Hernandez, E., Schenke-Layland, K., Walsh, C. J., and Duffy, G. P. (2015). Drug and cell delivery for cardiac regeneration. Adv. Drug Deliv. Rev. 84, 85–106. doi:10.1016/j.addr.2014.08.006
Hazeltine, L. B., Badur, M. G., Lian, X., Das, A., Han, W., and Palecek, S. P. (2014). Temporal impact of substrate mechanics on differentiation of human embryonic stem cells to cardiomyocytes. Acta Biomater. 10 (2), 604–612. doi:10.1016/j.actbio.2013.10.033
Hernandez, D., Millard, R., Sivakumaran, P., Wong, R. C., Crombie, D. E., Hewitt, A. W., et al. (2016). Electrical stimulation promotes cardiac differentiation of human induced pluripotent stem cells. Stem cells Int. 2016, 1718041–1718112. doi:10.1155/2016/1718041
Hosoyama, K., Ahumada, M., Goel, K., Ruel, M., Suuronen, E. J., and Alarcon, E. I. (2019). Electroconductive materials as biomimetic platforms for tissue regeneration. Biotechnol. Adv. 37 (3), 444–458. doi:10.1016/j.biotechadv.2019.02.011
Hsiao, C.-W., Bai, M.-Y., Chang, Y., Chung, M.-F., Lee, T.-Y., Wu, C.-T., et al. (2013). Electrical coupling of isolated cardiomyocyte clusters grown on aligned conductive nanofibrous meshes for their synchronized beating. Biomaterials 34 (4), 1063–1072. doi:10.1016/j.biomaterials.2012.10.065
Hsu, W. K., Kotzeva, V., Watts, P. C. P., and Chen, G. Z. (2004). Circuit elements in carbon nanotube-polymer composites. Carbon 42 (8), 1707–1712. doi:10.1016/j.carbon.2004.02.037
Huang, C. Y., Peres Moreno Maia-Joca, R., Ong, C. S., Wilson, I., DiSilvestre, D., Tomaselli, G. F., et al. (2020). Enhancement of human iPSC-derived cardiomyocyte maturation by chemical conditioning in a 3D environment. J. Mol. Cell. Cardiol. 138, 1–11. doi:10.1016/j.yjmcc.2019.10.001
Irawan, V., Higuchi, A., and Ikoma, T. (2018). Physical cues of biomaterials guide stem cell fate of differentiation: The effect of elasticity of cell culture biomaterials. Open Phys. 16 (1), 943–955. doi:10.1515/phys-2018-0116
Jafarkhani, M., Salehi, Z., Kowsari-Esfahan, R., Shokrgozar, M. A., Rezaa Mohammadi, M., Rajadas, J., et al. (2018). Strategies for directing cells into building functional hearts and parts. Biomaterials Sci. 6 (7), 1664–1690. doi:10.1039/c7bm01176h
Jeong, S. I., Jun, I. D., Choi, M. J., Nho, Y. C., Lee, Y. M., and Shin, H. (2008). Development of electroactive and elastic nanofibers that contain polyaniline and poly(L-lactide-co-ε-caprolactone) for the control of cell adhesion. Macromol. Biosci. 8 (7), 627–637. doi:10.1002/mabi.200800005
Jing, X., Mi, H.-Y., Napiwocki, B. N., Peng, X.-F., and Turng, L.-S. (2017). Mussel-inspired electroactive chitosan/graphene oxide composite hydrogel with rapid self-healing and recovery behavior for tissue engineering. Carbon 125, 557–570. doi:10.1016/j.carbon.2017.09.071
Jo, H., Sim, M., Kim, S., Yang, S., Yoo, Y., Park, J.-H., et al. (2017). Electrically conductive graphene/polyacrylamide hydrogels produced by mild chemical reduction for enhanced myoblast growth and differentiation. Acta Biomater. 48, 100–109. doi:10.1016/j.actbio.2016.10.035
Johnson, D. W., Dobson, B. P., and Coleman, K. S. (2015). A manufacturing perspective on graphene dispersions. Curr. Opin. Colloid & Interface Sci. 20 (5), 367–382. doi:10.1016/j.cocis.2015.11.004
Jun, I., Jeong, S., and Shin, H. (2009). The stimulation of myoblast differentiation by electrically conductive sub-micron fibers. Biomaterials 30 (11), 2038–2047. doi:10.1016/j.biomaterials.2008.12.063
Kai, D., Prabhakaran, M. P., Jin, G., and Ramakrishna, S. (2011). Polypyrrole-contained electrospun conductive nanofibrous membranes for cardiac tissue engineering. J. Biomed. Mater. Res. Part A 99A (3), 376–385. doi:10.1002/jbm.a.33200
Kaklamani, G., Kazaryan, D., Bowen, J., Iacovella, F., Anastasiadis, S. H., and Deligeorgis, G. (2018). On the electrical conductivity of alginate hydrogels. Regen. Biomater. 5 (5), 293–301. doi:10.1093/rb/rby019
Kang, B.-J., Kim, H., Lee, S. K., Kim, J., Shen, Y., Jung, S., et al. (2014). Umbilical-cord-blood-derived mesenchymal stem cells seeded onto fibronectin-immobilized polycaprolactone nanofiber improve cardiac function. Acta biomater. 10 (7), 3007–3017. doi:10.1016/j.actbio.2014.03.013
Karbassi, E., Fenix, A., Marchiano, S., Muraoka, N., Nakamura, K., Yang, X., et al. (2020). Cardiomyocyte maturation: advances in knowledge and implications for regenerative medicine. Nat. Rev. Cardiol. 17 (6), 341–359. doi:10.1038/s41569-019-0331-x
Kenar, H., Ozdogan, C. Y., Dumlu, C., Doger, E., Kose, G. T., and Hasirci, V. (2019). Microfibrous scaffolds from poly(l-lactide-co-ε-caprolactone) blended with xeno-free collagen/hyaluronic acid for improvement of vascularization in tissue engineering applications. Mater. Sci. Eng. C 97, 31–44. doi:10.1016/j.msec.2018.12.011
Keselowsky, B. G., Collard, D. M., and Garcia, A. J. (2004). Surface chemistry modulates focal adhesion composition and signaling through changes in integrin binding. Biomaterials 25 (28), 5947–5954. doi:10.1016/j.biomaterials.2004.01.062
Kim, D.-H., Lipke, E. A., Kim, P., Cheong, R., Thompson, S., Delannoy, M., et al. (2010). Nanoscale cues regulate the structure and function of macroscopic cardiac tissue constructs. Proc. Natl. Acad. Sci. 107 (2), 565–570. doi:10.1073/pnas.0906504107
Kim, T. H., Kwon, C. H., Lee, C., An, J., Phuong, T. T. T., Park, S. H., et al. (2016). Bio-inspired hybrid carbon nanotube muscles. Sci. Rep. 6, 26687. doi:10.1038/srep26687
Kitsara, M., Agbulut, O., Kontziampasis, D., Chen, Y., and Menasché, P. (2017). Fibers for hearts: A critical review on electrospinning for cardiac tissue engineering. Acta biomater. 48, 20–40. doi:10.1016/j.actbio.2016.11.014
Kosmidis, G., Bellin, M., Ribeiro, M. C., van Meer, B., Ward-van Oostwaard, D., Passier, R., et al. (2015). Altered calcium handling and increased contraction force in human embryonic stem cell derived cardiomyocytes following short term dexamethasone exposure. Biochem. Biophysical Res. Commun. 467 (4), 998–1005. doi:10.1016/j.bbrc.2015.10.026
Kostarelos, K., Lacerda, L., Pastorin, G., Wu, W., Wieckowski, S., Luangsivilay, J., et al. (2007). Cellular uptake of functionalized carbon nanotubes is independent of functional group and cell type. Nat. Nano 2 (2), 108–113. doi:10.1038/nnano.2006.209
Kostarelos, K. (2008). The long and short of carbon nanotube toxicity. Nat. Biotech. 26 (7), 774–776. doi:10.1038/nbt0708-774
Kotchey, G. P., Zhao, Y., Kagan, V. E., and Star, A. (2013). Peroxidase-mediated biodegradation of carbon nanotubes in vitro and in vivo. Adv. Drug Deliv. Rev. 65 (15), 1921–1932. doi:10.1016/j.addr.2013.07.007
Kratschmer, W., Lamb, L. D., Fostiropoulos, K., and Huffman, D. R. (1990). Solid C60: a new form of carbon. Nature 347 (6291), 354–358. doi:10.1038/347354a0
Kroll, K., Chabria, M., Wang, K., Häusermann, F., Schuler, F., and Polonchuk, L. (2017). Electro-mechanical conditioning of human iPSC-derived cardiomyocytes for translational research. Prog. Biophysics Mol. Biol. 130, 212–222. doi:10.1016/j.pbiomolbio.2017.07.003
Ku, S. H., Lee, S. H., and Park, C. B. (2012). Synergic effects of nanofiber alignment and electroactivity on myoblast differentiation. Biomaterials 33 (26), 6098–6104. doi:10.1016/j.biomaterials.2012.05.018
Kumar, N., Sridharan, D., Palaniappan, A., Dougherty, J. A., Czirok, A., Isai, D. G., et al. (2020). Scalable biomimetic coaxial aligned nanofiber cardiac patch: A potential model for “clinical Trials in a dish”. Front. Bioeng. Biotechnol. 8, 567842. doi:10.3389/fbioe.2020.567842
Kwon, H. J., Lee, G. S., and Chun, H. (2016). Electrical stimulation drives chondrogenesis of mesenchymal stem cells in the absence of exogenous growth factors. Sci. Rep. 6, 39302. doi:10.1038/srep39302
Laflamme, M. A., and Murry, C. E. (2011). Heart regeneration. Nature 473 (7347), 326–335. doi:10.1038/nature10147
Lee, D. S., Chen, J. H., Lundy, D. J., Liu, C. H., Hwang, S. M., Pabon, L., et al. (2015). Defined MicroRNAs induce aspects of maturation in mouse and human embryonic-stem-cell-derived cardiomyocytes. Cell Rep. 12 (12), 1960–1967. doi:10.1016/j.celrep.2015.08.042
Legant, W. R., Pathak, A., Yang, M. T., Deshpande, V. S., McMeeking, R. M., and Chen, C. S. (2009). Microfabricated tissue gauges to measure and manipulate forces from 3D microtissues. Proc. Natl. Acad. Sci. 106 (25), 10097–10102. doi:10.1073/pnas.0900174106
Leonard, A., Bertero, A., Powers, J. D., Beussman, K. M., Bhandari, S., Regnier, M., et al. (2018). Afterload promotes maturation of human induced pluripotent stem cell derived cardiomyocytes in engineered heart tissues. J. Mol. Cell. Cardiol. 118, 147–158. doi:10.1016/j.yjmcc.2018.03.016
Li, Y., Asfour, H., and Bursac, N. (2017). Age-dependent functional crosstalk between cardiac fibroblasts and cardiomyocytes in a 3D engineered cardiac tissue. Acta Biomater. 55, 120–130. doi:10.1016/j.actbio.2017.04.027
Li, Y., Li, X., Zhao, R., Wang, C., Qiu, F., Sun, B., et al. (2017). Enhanced adhesion and proliferation of human umbilical vein endothelial cells on conductive PANI-PCL fiber scaffold by electrical stimulation. Mater. Sci. Eng. C 72, 106–112. doi:10.1016/j.msec.2016.11.052
Li, Y., Shi, X., Tian, L., Sun, H., Wu, Y., Li, X., et al. (2016). AuNP–Collagen matrix with localized stiffness for cardiac-tissue engineering: Enhancing the assembly of intercalated discs by β1-integrin-mediated signaling. Adv. Mater. 28 (46), 10230–10235. doi:10.1002/adma.201603027
Liaw, N. Y., and Zimmermann, W.-H. (2016). Mechanical stimulation in the engineering of heart muscle. Adv. Drug Deliv. Rev. 96, 156–160. doi:10.1016/j.addr.2015.09.001
Limper, A. H., and Roman, J. (1992). Fibronectin: a versatile matrix protein with roles in thoracic development, repair and infection. Chest 101 (6), 1663–1673. doi:10.1378/chest.101.6.1663
Liverani, L., Killian, M. S., and Boccaccini, A. R. (2019). Fibronectin functionalized electrospun fibers by using benign solvents: Best way to achieve effective functionalization. Front. Bioeng. Biotechnol. 7 (68), 68. doi:10.3389/fbioe.2019.00068
Ma, X., Dewan, S., Liu, J., Tang, M., Miller, K. L., Yu, C., et al. (2019). 3D printed micro-scale force gauge arrays to improve human cardiac tissue maturation and enable high throughput drug testing. Acta Biomater. 95, 319–327. doi:10.1016/j.actbio.2018.12.026
Maher, B. (2013). Tissue engineering: How to build a heart. Nature 499 (7456), 20–22. doi:10.1038/499020a
Mao, C., Zhu, Y., and Jiang, W. (2012). Design of electrical conductive composites: Tuning the morphology to improve the electrical properties of graphene filled immiscible polymer blends. ACS Appl. Mater. Interfaces 4 (10), 5281–5286. doi:10.1021/am301230q
Mao, Y., and Schwarzbauer, J. E. (2005). Fibronectin fibrillogenesis, a cell-mediated matrix assembly process. Matrix Biol. 24 (6), 389–399. doi:10.1016/j.matbio.2005.06.008
Martins, A. M., Eng, G., Caridade, S. G., Mano, J. F., Reis, R. L., and Vunjak-Novakovic, G. (2014). Electrically conductive chitosan/carbon scaffolds for cardiac tissue engineering. Biomacromolecules 15 (2), 635–643. doi:10.1021/bm401679q
Martyniak, A., Jeż, M., Dulak, J., and Stępniewski, J. (2023). Adaptation of cardiomyogenesis to the generation and maturation of cardiomyocytes from human pluripotent stem cells. IUBMB Life 75 (1), 8–29. doi:10.1002/iub.2685
McCauley, M. D., Vitale, F., Yan, J. S., Young, C. C., Greet, B., Orecchioni, M., et al. (2019). In vivo restoration of myocardial conduction with carbon nanotube fibers. Circulation Arrhythmia Electrophysiol. 12 (8), e007256. doi:10.1161/circep.119.007256
McMahan, S., Taylor, A., Copeland, K. M., Pan, Z., Liao, J., and Hong, Y. (2020). Current advances in biodegradable synthetic polymer based cardiac patches. J. Biomed. Mater. Res. Part A 108 (4), 972–983. doi:10.1002/jbm.a.36874
Miklavčič, D., Pavšelj, N., and Hart, F. X. (2006). in Electric properties of tissues. Editor M. Akay (New Jersey: Wiley Encyclopedia of Biomedical Engineering).
Mills, R. J., Parker, B. L., Quaife-Ryan, G. A., Voges, H. K., Needham, E. J., Bornot, A., et al. (2019). Drug screening in human PSC-cardiac organoids identifies pro-proliferative compounds acting via the mevalonate pathway. Cell Stem Cell 24 (6), 895–907.e6. doi:10.1016/j.stem.2019.03.009
Minato, A., Ise, H., Goto, M., and Akaike, T. (2012). Cardiac differentiation of embryonic stem cells by substrate immobilization of insulin-like growth factor binding protein 4 with elastin-like polypeptides. Biomaterials 33 (2), 515–523. doi:10.1016/j.biomaterials.2011.09.070
Modugno, G., Ksar, F., Battigelli, A., Russier, J., Lonchambon, P., Eleto da Silva, E., et al. (2016). A comparative study on the enzymatic biodegradability of covalently functionalized double- and multi-walled carbon nanotubes. Carbon 100, 367–374. doi:10.1016/j.carbon.2016.01.023
Mohammadi Amirabad, L., Massumi, M., Shamsara, M., Shabani, I., Amari, A., Mossahebi Mohammadi, M., et al. (2017). Enhanced cardiac differentiation of human cardiovascular disease patient-specific induced pluripotent stem cells by applying unidirectional electrical pulses using aligned electroactive nanofibrous scaffolds. ACS Appl. Mater. Interfaces 9 (8), 6849–6864. doi:10.1021/acsami.6b15271
Monteiro, L. M., Vasques-Nóvoa, F., Ferreira, L., Pinto-do-Ó, P., and Nascimento, D. S. (2017). Restoring heart function and electrical integrity: Closing the circuit. npj Regen. Med. 2 (1), 9. doi:10.1038/s41536-017-0015-2
Mooney, E., Mackle, J. N., Blond, D. J. P., O'Cearbhaill, E., Shaw, G., Blau, W. J., et al. (2012). The electrical stimulation of carbon nanotubes to provide a cardiomimetic cue to MSCs. Biomaterials 33 (26), 6132–6139. doi:10.1016/j.biomaterials.2012.05.032
Morez, C., Noseda, M., Paiva, M. A., Belian, E., Schneider, M. D., and Stevens, M. M. (2015). Enhanced efficiency of genetic programming toward cardiomyocyte creation through topographical cues. Biomaterials 70, 94–104. doi:10.1016/j.biomaterials.2015.07.063
Mori, H., Hirai, Y., Ogata, S., Akita, S., and Nakayama, Y. (2005). Chirality dependence of mechanical properties of single-walled carbon nanotubes under axial tensile strain. Jpn. J. Appl. Phys. 44 (42), L1307–L1309. doi:10.1143/jjap.44.l1307
Nair, R. S., Ameer, J. M., Alison, M. R., and Anilkumar, T. V. (2017). A gold nanoparticle coated porcine cholecyst-derived bioscaffold for cardiac tissue engineering. Colloids Surfaces B Biointerfaces 157, 130–137. doi:10.1016/j.colsurfb.2017.05.056
Nakaoka, R., Yamakoshi, Y., Isama, K., and Tsuchiya, T. (2010). Effects of surface chemistry prepared by self-assembled monolayers on osteoblast behavior. J. Biomed. Mater. Res. Part A 94A (2), 524–532. doi:10.1002/jbm.a.32714
Navaei, A., Saini, H., Christenson, W., Sullivan, R. T., Ros, R., and Nikkhah, M. (2016). Gold nanorod-incorporated gelatin-based conductive hydrogels for engineering cardiac tissue constructs. Acta Biomater. 41, 133–146. doi:10.1016/j.actbio.2016.05.027
Ning, C., Zhou, Z., Tan, G., Zhu, Y., and Mao, C. (2018). Electroactive polymers for tissue regeneration: Developments and perspectives. Prog. Polym. Sci. 81, 144–162. doi:10.1016/j.progpolymsci.2018.01.001
Novoselov, K. S., Geim, A. K., Morozov, S. V., Jiang, D., Zhang, Y., Dubonos, S. V., et al. (2004). Electric field effect in atomically thin carbon films. Science 306 (5696), 666–669. doi:10.1126/science.1102896
Nunes, A., Al-Jamal, K., Nakajima, T., Hariz, M., and Kostarelos, K. (2012). Application of carbon nanotubes in neurology: Clinical perspectives and toxicological risks. Arch. Toxicol. 86, 1009–1020. doi:10.1007/s00204-012-0860-0
Nunes, S. S., Miklas, J. W., Liu, J., Aschar-Sobbi, R., Xiao, Y., Zhang, B., et al. (2013). Biowire: a platform for maturation of human pluripotent stem cell–derived cardiomyocytes. Nat. Methods 10, 781–787. doi:10.1038/nmeth.2524
Onakpoya, I. J., Heneghan, C. J., and Aronson, J. K. (2016). Worldwide withdrawal of medicinal products because of adverse drug reactions: a systematic review and analysis. Crit. Rev. Toxicol. 46 (6), 477–489. doi:10.3109/10408444.2016.1149452
Ostrovidov, S., Shi, X., Zhang, L., Liang, X., Kim, S. B., Fujie, T., et al. (2014). Myotube formation on gelatin nanofibers – multi-walled carbon nanotubes hybrid scaffolds. Biomaterials 35 (24), 6268–6277. doi:10.1016/j.biomaterials.2014.04.021
Ouyang, M., and Chan, C. M. (1996). Electrical and mechanical properties of pre-localized polypyrrole/poly(vinyl chloride) conductive composites. Polym. Eng. Sci. 36 (21), 2676–2682. doi:10.1002/pen.10666
Patel, A., Mukundan, S., Wang, W., Karumuri, A., Sant, V., Mukhopadhyay, S. M., et al. (2016). Carbon-based hierarchical scaffolds for myoblast differentiation: Synergy between nano-functionalization and alignment. Acta Biomater. 32, 77–88. doi:10.1016/j.actbio.2016.01.004
Pietronave, S., Zamperone, A., Oltolina, F., Colangelo, D., Follenzi, A., Novelli, E., et al. (2014). Monophasic and biphasic electrical stimulation induces a precardiac differentiation in progenitor cells isolated from human heart. Stem cells Dev. 23 (8), 888–898. doi:10.1089/scd.2013.0375
Pissis, P., and Kyritsis, A. (1997). Electrical conductivity studies in hydrogels. Solid State Ionics 97 (1), 105–113. doi:10.1016/s0167-2738(97)00074-x
Pretorius, D., Kahn-Krell, A. M., LaBarge, W. C., Lou, X., and Zhang, J. (2022). Engineering of thick human functional myocardium via static stretching and electrical stimulation. iScience 25 (3), 103824. doi:10.1016/j.isci.2022.103824
Price, R. L., Waid, M. C., Haberstroh, K. M., and Webster, T. J. (2003). Selective bone cell adhesion on formulations containing carbon nanofibers. Biomaterials 24 (11), 1877–1887. doi:10.1016/s0142-9612(02)00609-9
Qazi, T. H., Rai, R., and Boccaccini, A. R. (2014). Tissue engineering of electrically responsive tissues using polyaniline based polymers: A review. Biomaterials 35 (33), 9068–9086. doi:10.1016/j.biomaterials.2014.07.020
Rafieerad, A., Amiri, A., Sequiera, G. L., Yan, W., Chen, Y., Polycarpou, A. A., et al. (2021). Development of fluorine-free tantalum carbide MXene hybrid structure as a biocompatible material for supercapacitor electrodes. Adv. Funct. Mater. 31 (30), 2100015. doi:10.1002/adfm.202100015
Rajendran, A. K., Sankar, D., Amirthalingam, S., Kim, H. D., Rangasamy, J., and Hwang, N. S. (2023). Trends in mechanobiology guided tissue engineering and tools to study cell-substrate interactions: a brief review. Biomaterials Res. 27 (1), 55. doi:10.1186/s40824-023-00393-8
Ratner, B. D. (2013). “A history of biomaterials,” in Biomaterials science. Editors B. D. Ratner, A. S. Hoffman, F. J. Schoen, and J. E. Lemons Third Edition (United States: Academic Press).
Ratner, B. D., Hoffman, A. S., Schoen, F. J., and Lemons, J. E. (2013). Introduction - biomaterials science: An evolving, multidisciplinary endeavor, biomaterials science Third Edition. United States: Academic Press. xxv-xxxix.
Ronaldson-Bouchard, K., Ma, S. P., Yeager, K., Chen, T., Song, L., Sirabella, D., et al. (2018). Advanced maturation of human cardiac tissue grown from pluripotent stem cells. Nature 556 (7700), 239–243. doi:10.1038/s41586-018-0016-3
Ronaldson-Bouchard, K., Yeager, K., Teles, D., Chen, T., Ma, S., Song, L., et al. (2019). Engineering of human cardiac muscle electromechanically matured to an adult-like phenotype. Nat. Protoc. 14 (10), 2781–2817. doi:10.1038/s41596-019-0189-8
Saberi, A. A. (2015). Recent advances in percolation theory and its applications. Phys. Rep. 578, 1–32. doi:10.1016/j.physrep.2015.03.003
Saito, N., Aoki, K., Usui, Y., Shimizu, M., Hara, K., Narita, N., et al. (2011). Application of carbon fibers to biomaterials: A new era of nano-level control of carbon fibers after 30-years of development. Chem. Soc. Rev. 40 (7), 3824–3834. doi:10.1039/c0cs00120a
Saito, N., Haniu, H., Usui, Y., Aoki, K., Hara, K., Takanashi, S., et al. (2014). Safe clinical use of carbon nanotubes as innovative biomaterials. Chem. Rev. 114 (11), 6040–6079. doi:10.1021/cr400341h
Sakina, R., Llucià-Valldeperas, A., Henriques Lourenço, A., Harichandan, A., Gelsomino, S., Wieringa, P., et al. (2020). “Chapter 1 - decellularization of porcine heart tissue to obtain extracellular matrix based hydrogels,” in Methods in cell biology. Editors D. Caballero, S. C. Kundu, and R. L. Reis (United States: Academic Press), 3–21.
Sanchez-Valencia, J. R., Dienel, T., Groning, O., Shorubalko, I., Mueller, A., Jansen, M., et al. (2014). Controlled synthesis of single-chirality carbon nanotubes. Nature 512 (7512), 61–64. doi:10.1038/nature13607
Sepantafar, M., Maheronnaghsh, R., Mohammadi, H., Rajabi-Zeleti, S., Annabi, N., Aghdami, N., et al. (2016). Stem cells and injectable hydrogels: Synergistic therapeutics in myocardial repair. Biotechnol. Adv. 34 (4), 362–379. doi:10.1016/j.biotechadv.2016.03.003
Serpell, C. J., Kostarelos, K., and Davis, B. G. (2016). Can carbon nanotubes deliver on their promise in biology? Harnessing unique properties for unparalleled applications. ACS Central Sci. 2 (4), 190–200. doi:10.1021/acscentsci.6b00005
Sharma, D., Ferguson, M., Kamp, T. J., and Zhao, F. (2019). Constructing biomimetic cardiac tissues: a review of scaffold materials for engineering cardiac patches. Emergent Mater. 2, 181–191. doi:10.1007/s42247-019-00046-4
Sheehy, S. P., Grosberg, A., and Parker, K. K. (2012). The contribution of cellular mechanotransduction to cardiomyocyte form and function. Biomechanics Model. Mechanobiol. 11 (8), 1227–1239. doi:10.1007/s10237-012-0419-2
Shi, T., Wang, P., Ren, Y., Zhang, W., Ma, J., Li, S., et al. (2023). Conductive hydrogel patches with high elasticity and fatigue resistance for cardiac microenvironment remodeling. ACS Appl. Mater. Interfaces 15 (11), 14005–14018. doi:10.1021/acsami.2c22673
Shin, S. R., Zihlmann, C., Akbari, M., Assawes, P., Cheung, L., Zhang, K., et al. (2016). Reduced graphene oxide-GelMA hybrid hydrogels as scaffolds for cardiac tissue engineering. Small 12 (27), 3677–3689. doi:10.1002/smll.201600178
Shvedova, A. A., Pietroiusti, A., Fadeel, B., and Kagan, V. E. (2012). Mechanisms of carbon nanotube-induced toxicity: Focus on oxidative stress. Toxicol. Appl. Pharmacol. 261 (2), 121–133. doi:10.1016/j.taap.2012.03.023
Silvestre, J.-S., and Menasché, P. (2015). The evolution of the stem cell theory for heart failure. EBioMedicine 2 (12), 1871–1879. doi:10.1016/j.ebiom.2015.11.010
Siramshetty, V. B., Nickel, J., Omieczynski, C., Gohlke, B.-O., Drwal, M. N., and Preissner, R. (2015). WITHDRAWN—A resource for withdrawn and discontinued drugs. Nucleic Acids Res. 44 (D1), D1080–D1086. doi:10.1093/nar/gkv1192
Sirivisoot, S., and Harrison, B. S. (2011). Skeletal myotube formation enhanced by electrospun polyurethane carbon nanotube scaffolds. Int. J. Nanomedicine 6, 2483–2497. doi:10.2147/ijn.s24073
Smalley, R. E. (1998). “Crystalline ropes of metallic carbon nanotubes,” in Supercarbon: Synthesis, properties and applications. Editors S. Yoshimura, and R. P. H. Chang (Berlin, Heidelberg: Springer), 31–40.
Song, X., Mei, J., Ye, G., Wang, L., Ananth, A., Yu, L., et al. (2019). In situ pPy-modification of chitosan porous membrane from mussel shell as a cardiac patch to repair myocardial infarction. Appl. Mater. Today 15, 87–99. doi:10.1016/j.apmt.2019.01.003
Stepniewski, J., Pacholczak, T., Skrzypczyk, A., Ciesla, M., Szade, A., Szade, K., et al. (2018). Heme oxygenase-1 affects generation and spontaneous cardiac differentiation of induced pluripotent stem cells. IUBMB Life 70 (2), 129–142. doi:10.1002/iub.1711
Stodolak-Zych, E., Benko, A., Szatkowski, P., Długoń, E., Nocuń, M., Paluszkiewicz, C., et al. (2016). Spectroscopic studies of the influence of CNTs on the thermal conversion of PAN fibrous membranes to carbon nanofibers. J. Mol. Struct. 1126, 94–102. doi:10.1016/j.molstruc.2016.01.022
Stoppel, W. L., Kaplan, D. L., and Black, L. D. (2016). Electrical and mechanical stimulation of cardiac cells and tissue constructs. Adv. Drug Deliv. Rev. 96, 135–155. doi:10.1016/j.addr.2015.07.009
Stout, D. A., Basu, B., and Webster, T. J. (2011). RETRACTED: Poly(lactic–co-glycolic acid): Carbon nanofiber composites for myocardial tissue engineering applications. Acta Biomater. 7 (8), 3101–3112. doi:10.1016/j.actbio.2011.04.028
Stout, D. A., and Webster, T. J. (2012). Carbon nanotubes for stem cell control. Mater. Today 15 (7–8), 312–318. doi:10.1016/s1369-7021(12)70136-0
Stout, D. A., Yoo, J., Santiago-Miranda, A. N., and Webster, T. J. (2012). Mechanisms of greater cardiomyocyte functions on conductive nanoengineered composites for cardiovascular application. Int. J. Nanomedicine 7, 5653–5669. doi:10.2147/ijn.s34574
Suhaeri, M., Subbiah, R., Kim, S.-H., Kim, C.-H., Oh, S. J., Kim, S.-H., et al. (2017). Novel platform of cardiomyocyte culture and coculture via fibroblast-derived matrix-coupled aligned electrospun nanofiber. ACS Appl. Mater. Interfaces 9 (1), 224–235. doi:10.1021/acsami.6b14020
Sun, H., Tang, J., Mou, Y., Zhou, J., Qu, L., Duval, K., et al. (2017). Carbon nanotube-composite hydrogels promote intercalated disc assembly in engineered cardiac tissues through β1-integrin mediated FAK and RhoA pathway. Acta Biomater. 48, 88–99. doi:10.1016/j.actbio.2016.10.025
Taheri, R. A., Akhtari, Y., Tohidi Moghadam, T., and Ranjbar, B. (2018). Assembly of gold nanorods on HSA amyloid fibrils to develop a conductive nanoscaffold for potential biomedical and biosensing applications. Sci. Rep. 8 (1), 9333. doi:10.1038/s41598-018-26393-6
Tallawi, M., Rosellini, E., Barbani, N., Cascone, M. G., Rai, R., Saint-Pierre, G., et al. (2015). Strategies for the chemical and biological functionalization of scaffolds for cardiac tissue engineering: a review. J. R. Soc. Interface 12 (108), 20150254. doi:10.1098/rsif.2015.0254
Tamimi, M., Rajabi, S., and Pezeshki-Modaress, M. (2020). Cardiac ECM/chitosan/alginate ternary scaffolds for cardiac tissue engineering application. Int. J. Biol. Macromol. 164, 389–402. doi:10.1016/j.ijbiomac.2020.07.134
Tandon, N., Cannizzaro, C., Chao, P.-H. G., Maidhof, R., Marsano, A., Au, H. T. H., et al. (2009). Electrical stimulation systems for cardiac tissue engineering. Nat. Protoc. 4 (2), 155–173. doi:10.1038/nprot.2008.183
Tao, Z.-W., Mohamed, M., Hogan, M., Gutierrez, L., and Birla, R. K. (2017). Optimizing a spontaneously contracting heart tissue patch with rat neonatal cardiac cells on fibrin gel. J. Tissue Eng. Regen. Med. 11 (1), 153–163. doi:10.1002/term.1895
Thevenot, P., Hu, W., and Tang, L. (2008). Surface chemistry influences implant biocompatibility. Curr. Top. Med. Chem. 8 (4), 270–280. doi:10.2174/156802608783790901
Thrivikraman, G., Boda, S. K., and Basu, B. (2018). Unraveling the mechanistic effects of electric field stimulation towards directing stem cell fate and function: A tissue engineering perspective. Biomaterials 150, 60–86. doi:10.1016/j.biomaterials.2017.10.003
Thrivikraman, G., Madras, G., and Basu, B. (2016). Electrically driven intracellular and extracellular nanomanipulators evoke neurogenic/cardiomyogenic differentiation in human mesenchymal stem cells. Biomaterials 77, 26–43. doi:10.1016/j.biomaterials.2015.10.078
Tirziu, D., Giordano, F. J., and Simons, M. (2010). Cell communications in the heart. Circulation 122 (9), 928–937. doi:10.1161/circulationaha.108.847731
Tran, P. A., Zhang, L., and Webster, T. J. (2009). Carbon nanofibers and carbon nanotubes in regenerative medicine. Adv. Drug Deliv. Rev. 61 (12), 1097–1114. doi:10.1016/j.addr.2009.07.010
Trombino, S., Curcio, F., Cassano, R., Curcio, M., Cirillo, G., and Iemma, F. (2021). Polymeric biomaterials for the treatment of cardiac post-infarction injuries. Pharmaceutics 13 (7), 1038. doi:10.3390/pharmaceutics13071038
Tzahor, E., and Poss, K. D. (2017). Cardiac regeneration strategies: Staying young at heart. Sci. (New York, N.Y.) 356 (6342), 1035–1039. doi:10.1126/science.aam5894
Ulmer, B. M., Stoehr, A., Schulze, M. L., Patel, S., Gucek, M., Mannhardt, I., et al. (2018). Contractile work contributes to maturation of energy metabolism in hiPSC-derived cardiomyocytes. Stem Cell Rep. 10 (3), 834–847. doi:10.1016/j.stemcr.2018.01.039
Van Dijk, A., Niessen, H., Ursem, W., Twisk, J., Visser, F., and Van Milligen, F. (2008). Accumulation of fibronectin in the heart after myocardial infarction: a putative stimulator of adhesion and proliferation of adipose-derived stem cells. Cell tissue Res. 332 (2), 289–298. doi:10.1007/s00441-008-0573-0
Van Vlierberghe, S., Graulus, G. J., Keshari Samal, S., Van Nieuwenhove, I., and Dubruel, P. (2014). “12 - porous hydrogel biomedical foam scaffolds for tissue repair,” in Biomedical foams for tissue engineering applications. Editor P. A. Netti (United States: Woodhead Publishing), 335–390.
Varzideh, F., Pahlavan, S., Ansari, H., Halvaei, M., Kostin, S., Feiz, M.-S., et al. (2019). Human cardiomyocytes undergo enhanced maturation in embryonic stem cell-derived organoid transplants. Biomaterials 192, 537–550. doi:10.1016/j.biomaterials.2018.11.033
Veldhuizen, J., Cutts, J., Brafman, D. A., Migrino, R. Q., and Nikkhah, M. (2020). Engineering anisotropic human stem cell-derived three-dimensional cardiac tissue on-a-chip. Biomaterials 256, 120195. doi:10.1016/j.biomaterials.2020.120195
Ventre, M., Causa, F., and Netti, P. A. (2012). Determinants of cell–material crosstalk at the interface: Towards engineering of cell instructive materials. J. R. Soc. Interface 9 (74), 2017–2032. doi:10.1098/rsif.2012.0308
Vial, S., Reis, R. L., and Oliveira, J. M. (2017). Recent advances using gold nanoparticles as a promising multimodal tool for tissue engineering and regenerative medicine. Curr. Opin. Solid State Mater. Sci. 21 (2), 92–112. doi:10.1016/j.cossms.2016.03.006
Vittorio, O., Raffa, V., and Cuschieri, A. (2009). Influence of purity and surface oxidation on cytotoxicity of multiwalled carbon nanotubes with human neuroblastoma cells. Nanomedicine Nanotechnol. Biol. Med. 5 (4), 424–431. doi:10.1016/j.nano.2009.02.006
Walker, B. W., Portillo Lara, R., Mogadam, E., Hsiang Yu, C., Kimball, W., and Annabi, N. (2019). Rational design of microfabricated electroconductive hydrogels for biomedical applications. Prog. Polym. Sci. 92, 135–157. doi:10.1016/j.progpolymsci.2019.02.007
Wang, L., Wu, Y., Hu, T., Guo, B., and Ma, P. X. (2017). Electrospun conductive nanofibrous scaffolds for engineering cardiac tissue and 3D bioactuators. Acta Biomater. 59, 68–81. doi:10.1016/j.actbio.2017.06.036
Wang, R. M., and Christman, K. L. (2016). Decellularized myocardial matrix hydrogels: In basic research and preclinical studies. Adv. Drug Deliv. Rev. 96, 77–82. doi:10.1016/j.addr.2015.06.002
Wang, W., Tan, B., Chen, J., Bao, R., Zhang, X., Liang, S., et al. (2018). An injectable conductive hydrogel encapsulating plasmid DNA-eNOs and ADSCs for treating myocardial infarction. Biomaterials 160, 69–81. doi:10.1016/j.biomaterials.2018.01.021
World Health Organization (2017). Cardiovascular diseases (CVDs). Available at: https://www.who.int/news-room/fact-sheets/detail/cardiovascular-diseases-(cvds) (Accessed March 07, 2020).
Wu, W.-q., Peng, S., Song, Z.-y., and Lin, S. (2019). Collagen biomaterial for the treatment of myocardial infarction: an update on cardiac tissue engineering and myocardial regeneration. Drug Deliv. Transl. Res. 9 (5), 920–934. doi:10.1007/s13346-019-00627-0
Wu, Y., Wang, L., Guo, B., and Ma, P. X. (2017). Interwoven aligned conductive nanofiber yarn/hydrogel composite scaffolds for engineered 3D cardiac anisotropy. ACS Nano 11 (6), 5646–5659. doi:10.1021/acsnano.7b01062
Yamato, M., Akiyama, Y., Kobayashi, J., Yang, J., Kikuchi, A., and Okano, T. (2007). Temperature-responsive cell culture surfaces for regenerative medicine with cell sheet engineering. Prog. Polym. Sci. 32 (8), 1123–1133. doi:10.1016/j.progpolymsci.2007.06.002
Yang, X., Rodriguez, M. L., Leonard, A., Sun, L., Fischer, K. A., Wang, Y., et al. (2019). Fatty acids enhance the maturation of cardiomyocytes derived from human pluripotent stem cells. Stem Cell Rep. 13 (4), 657–668. doi:10.1016/j.stemcr.2019.08.013
Yi, N., and Abidian, M. R. (2016). “10 - conducting polymers and their biomedical applications,” in Biosynthetic polymers for medical applications. Editors L. Poole-Warren, P. Martens, and R. Green (United States: Woodhead Publishing), 243–276.
Yoshida, S., Miyagawa, S., Fukushima, S., Kawamura, T., Kashiyama, N., Ohashi, F., et al. (2018). Maturation of human induced pluripotent stem cell-derived cardiomyocytes by soluble factors from human mesenchymal stem cells. Mol. Ther. 26 (11), 2681–2695. doi:10.1016/j.ymthe.2018.08.012
Yow, S.-Z., Lim, T. H., Yim, E. K. F., Lim, C. T., and Leong, K. W. (2011). A 3D electroactive polypyrrole-collagen fibrous scaffold for tissue engineering. Polymers 3 (1), 527–544. doi:10.3390/polym3010527
Yu, J., Lee, A. R., Lin, W. H., Lin, C. W., Wu, Y. K., and Tsai, W. B. (2014). Electrospun PLGA fibers incorporated with functionalized biomolecules for cardiac tissue engineering. Part A 20 (13-14), 1896–1907. doi:10.1089/ten.tea.2013.0008
Yue, K., Trujillo-de Santiago, G., Alvarez, M. M., Tamayol, A., Annabi, N., and Khademhosseini, A. (2015). Synthesis, properties, and biomedical applications of gelatin methacryloyl (GelMA) hydrogels. Biomaterials 73, 254–271. doi:10.1016/j.biomaterials.2015.08.045
Zhang, B., Wei, P., Zhou, Z., and Wei, T. (2016). Interactions of graphene with mammalian cells: Molecular mechanisms and biomedical insights. Adv. Drug Deliv. Rev. 105, 145–162. doi:10.1016/j.addr.2016.08.009
Zhang, L., Aboagye, A., Kelkar, A., Lai, C., and Fong, H. (2014). A review: Carbon nanofibers from electrospun polyacrylonitrile and their applications. J. Mater. Sci. 49 (2), 463–480. doi:10.1007/s10853-013-7705-y
Zhang, N., Stauffer, F., Simona, B. R., Zhang, F., Zhang, Z.-M., Huang, N.-P., et al. (2018). Multifunctional 3D electrode platform for real-time in situ monitoring and stimulation of cardiac tissues. Biosens. Bioelectron. 112, 149–155. doi:10.1016/j.bios.2018.04.037
Zhang, S., Crow, J. A., Yang, X., Chen, J., Borazjani, A., Mullins, K. B., et al. (2010). The correlation of 3D DT-MRI fiber disruption with structural and mechanical degeneration in porcine myocardium. Ann. Biomed. Eng. 38 (10), 3084–3095. doi:10.1007/s10439-010-0073-8
Zhao, G., Qing, H., Huang, G., Genin, G. M., Lu, T. J., Luo, Z., et al. (2018). Reduced graphene oxide functionalized nanofibrous silk fibroin matrices for engineering excitable tissues. NPG Asia Mater. 10 (10), 982–994. doi:10.1038/s41427-018-0092-8
Zhao, G., Zhang, X., Lu, T. J., and Xu, F. (2015). Recent advances in electrospun nanofibrous scaffolds for cardiac tissue engineering. Adv. Funct. Mater. 25 (36), 5726–5738. doi:10.1002/adfm.201502142
Zhao, J., Meng, F., Qian, J., Huang, Y., and Fan, Y. (2023). In vitro cell stretching devices and their applications: From cardiomyogenic differentiation to tissue engineering. Med. Nov. Technol. Devices 18, 100220. doi:10.1016/j.medntd.2023.100220
Zhao, Y., Rafatian, N., Feric, N. T., Cox, B. J., Aschar-Sobbi, R., Wang, E. Y., et al. (2019). A platform for generation of chamber-specific cardiac tissues and disease modeling. Cell 176 (4), 913–927.e18. doi:10.1016/j.cell.2018.11.042
Zhong, J., Yang, Y., Liao, L., and Zhang, C. (2020). Matrix stiffness-regulated cellular functions under different dimensionalities. Biomaterials Sci. 8 (10), 2734–2755. doi:10.1039/c9bm01809c
Zhu, K., Shin, S. R., van Kempen, T., Li, Y. C., Ponraj, V., Nasajpour, A., et al. (2017). Gold nanocomposite bioink for printing 3D cardiac constructs. Adv. Funct. Mater 27 (12), 1605352. doi:10.1002/adfm.201605352
Zimmermann, W.-H. (2020). Tissue engineered heart repair from preclinical models to first-in-patient studies. Curr. Opin. Physiology 14, 70–77. doi:10.1016/j.cophys.2020.02.001
Keywords: CVD (cardio vascular disease), cardiac tissue bioengineering, mechanostimulation, electrostimulation in vitro, bioactivity, biofuctionality, green materials, green design
Citation: Benko A and Webster TJ (2023) How to fix a broken heart—designing biofunctional cues for effective, environmentally-friendly cardiac tissue engineering. Front. Chem. 11:1267018. doi: 10.3389/fchem.2023.1267018
Received: 25 July 2023; Accepted: 04 September 2023;
Published: 12 October 2023.
Edited by:
Matteo Gigli, Ca’ Foscari University of Venice, ItalyReviewed by:
Giulia Guidotti, University of Bologna, ItalyDanielle Pretorius, University of Alabama at Birmingham, United States
Copyright © 2023 Benko and Webster. This is an open-access article distributed under the terms of the Creative Commons Attribution License (CC BY). The use, distribution or reproduction in other forums is permitted, provided the original author(s) and the copyright owner(s) are credited and that the original publication in this journal is cited, in accordance with accepted academic practice. No use, distribution or reproduction is permitted which does not comply with these terms.
*Correspondence: Aleksandra Benko, YWJlbmtvQGFnaC5lZHUucGw=