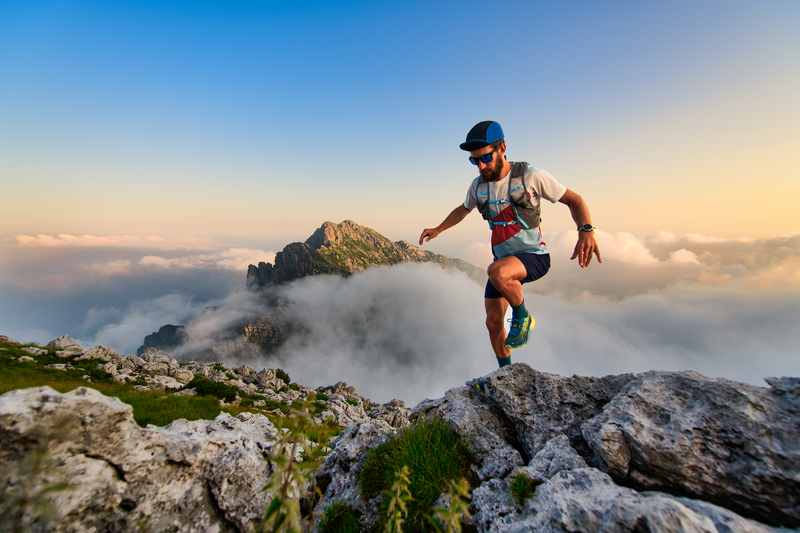
95% of researchers rate our articles as excellent or good
Learn more about the work of our research integrity team to safeguard the quality of each article we publish.
Find out more
ORIGINAL RESEARCH article
Front. Chem. , 03 October 2023
Sec. Supramolecular Chemistry
Volume 11 - 2023 | https://doi.org/10.3389/fchem.2023.1263440
A bis(aza-18-crown-6)-containing 2,5-di(benzylidene)cyclopentanone and a bis(ammoniopropyl) derivative of 1,2-di(4-pyridyl)ethylene in MeCN were found to form a supramolecular charge-transfer complex, which can act as an “off–on” fluorescent sensor for the Ca2+ and 1,12-dodecanediammonium ions. The molecular structure of this complex in solution was studied by density functional theory calculations.
Luminescent molecular sensors based on crown compounds are widely used to detect cations (De Silva et al., 1997; Ushakov et al., 2010; Li et al., 2017; Jiang et al., 2020; Golcs et al., 2021). The general concept of the design of these sensors is based mainly on the phenomena of changes in luminescence due to intramolecular processes in donor–acceptor systems, primarily such as photoinduced electron transfer and the transition of an excited molecule to a twisted state with internal charge transfer. The macrocyclic and luminophore units of the “off-on” luminescent molecular sensors should be connected in such a way that the substrate entering the macrocycle cavity be able to suppress the process responsible for luminescence quenching.
Organic charge-transfer complexes (Foster, 1969) attract attention of researchers owing to their wide use in various fields of materials science and chemistry such as development of organic solar cells (Günes et al., 2007; Huo et al., 2019), electromagnetic materials (Wu et al., 2021), organic semiconductors (Morita et al., 2013), photocatalysts (Yuan et al., 2020), and fluorescent sensors (Gromov et al., 2008; Vedernikov et al., 2011; Yao et al., 2017; Shakya and Khan, 2021), as model systems for studying ultrafast electron transfer reactions (Ushakov et al., 2004), and for the design of artificial light-harvesting antennas (Ke et al., 2017; Lian et al., 2020).
Molecular systems consisting of two or more structurally organized components that perform a common specific function are classified as molecular devices (Lehn, 1995; Balzani et al., 2003). Separately, each component (fragment) of the system perform only its particular action, but as a result of the cooperation of components, a more complex function arises. The molecular devices in which non-covalent interactions are used to combine components can be designated as supramolecular devices. Promising molecules for use as building blocks of supramolecular devices are biscrown-containing unsaturated compounds (Fery-Forgues and Al-Ali, 2004), which, in addition to the ability to form host–guest complexes between macrocyclic fragments and metal ions, also exhibit fluorescent properties due to the presence of an ethylene unit in the chromophore moiety. We have found that the formation of hydrogen bonds between a biscrown compound of this type and a π-electron acceptor molecule can provide not only a very high thermodynamic stability of the donor–acceptor complex, but also the spatial preorganization of the components for efficient charge-transfer interaction (Gromov et al., 2008). Therefore, two-component systems of this type can be attributed to such a class of photoactive supramolecular devices as supramolecular fluorescent sensors (Lehn, 1995; MakoRacicot and Levine, 2019).
In this communication, we present the results of experimental and theoretical studies of the supramolecular donor–acceptor complex between bis(azacrown)-containing dienone 1 (π-electron donor) and viologen analog, bis(ammoniopropyl) derivative of dipyridylethylene 2 (π-electron acceptor, Scheme 1). The complex is formed via hydrogen bonding and is characterized by intermolecular charge transfer in the ground state. Presumably, complex 1∙2 has a pseudocyclic structure (Scheme 1). Salt 3 was used in this study as the model diammonium compound containing no π-electron system.
SCHEME 1. Chemical structure of compounds 1–3 and formation of supramolecular pseudocyclic complex 1∙2.
MeCN (extra high purity, water content <0.03%, Cryochrom) was used to prepare solutions.
Compounds 1, 2 and 1,12-dodecanediammonium diperchlorate (compound 3) were prepared according to known procedures (refs: Fomina et al., 2020; Gromov et al., 1999; Gromov et al., 2009, respectively).
Complex [1∙2] was obtained as a dark red oil by mixing equimolar amounts of 1 and 2 in a minimum volume of acetonitrile. After evaporation of the solvent, the residue was washed with benzene and dried.
The stoichiometry of complex [1∙2] was confirmed by 1H NMR, 2D DOSY NMR (in MeCN-d3), IR (ATR), and elemental analysis data. Dark-red oil (14.9 mg, 97% yield); 1HNMR (500.13 MHz, MeCN-d3, 25°C) δ 1.31–1.37 (br.m, 4H), 2.77 (br.s, 4H), 2.78–2.83 (br.m, 4H), 3.51 (m, 8H), 3.68–3.72 (br.m, 22H), 3.76 (m, 24H), 4.20–4.24 (m, 4H), 7.08–7.13 (br.m, 6H), 7.33 (m, 4H), 7.70 (br.s, 2H), 8.15 (d, J = 6.7 Hz, 4H), 8.43 (d, J = 6.7 Hz, 4H); IR (ATR): 1,633 cm−1 (C=O), 3,125 cm−1 (N+−H). Analysis (calcd., found for C43H62N2O11·C19H30Cl4N4O16·2H2O): C (48.29, 47.99), H (6.24, 6.19) N (5.54, 5.61).
The 1H NMR spectra were recorded on Bruker Avance-500 and Bruker Avance-III 400 spectrometers (operating frequencies of 500.13 and 400.13 MHz, respectively) in MeCN-d3 at 25°C using the solvent signal as the internal standard (δH 1.96 ppm). The chemical shifts were determined with an accuracy of 0.01 ppm, and the spin–spin coupling constants were determined with an accuracy of 0.1 Hz. The NMR spectra were processed by TopSpin 3.2 software and diffusion coefficients were calculated using T1/T2 relaxation module.
IR spectra were recorded using a Nicolet iS5 Fourier transform spectrometer (Thermo Scientific) with an internal reflectance attachment and a diamond optical element for attenuated total reflectance (ATR, iD7) with 45° angle of incidence. The resolution was 4 cm−1, and the number of scans was 20.
UV-vis absorption spectra were measured on a Cary 4000 spectrophotometer in MeCN. Uncorrected fluorescence spectra were obtained on a Cary Eclipse spectrofluorimeter at room temperature. All manipulations with solutions of compounds 1 and 2 were performed in a darkroom under red light.
Fluorescence quantum yield measurements were carried out on a time-resolved fluorescence spectrometer FluoTime 300 (PicoQuant), equipped with a PDL-820 multichannel picosecond laser driver and an LDH-P-C-440 semiconductor pulsed laser as the excitation source (λ = 440 nm, pulse duration ∼80 ps).
Fluorescence quantum yields (φ) were determined using fluorescein in 0.1 N NaOH in water as the reference standard (φref = 0.92, Rurack, 2008) by the following equation:
where I is the integral intensity of the corrected fluorescence spectrum, D is the optical density of the solution at the excitation wavelength, n is the refractive index of the solvent.
The measured quantum yields are as follows: dienone 1, φ = 0.16; a mixture of 1 and 2 in 1:8 ratio, φ = 0.01; the 1:8 mixture after the addition of diammonium salt 3 in 15-fold excess over 1, φ = 0.18; the 1:8 mixture after the addition of Ca(ClO4)2 in 30-fold excess over 1, φ = 0.09.
Molecular mechanics was used to generate sets of low-energy conformations for compound 1 and complex 1∙2. Conformational searches were carried out using the MMFF94s force field (Halgren, 1999). The subsequent calculations by density functional theory (DFT) and time-dependent DFT (TDDFT) were performed using the Gaussian 16 program package (Frisch et al., 2016). The molecular mechanics geometries were re-optimized using the M06-2X density functional (Zhao and Truhlar, 2008) with the 6-31G(d) basis set (Hehre et al., 1972; Hariharan and Pople, 1973). The universal solvation model known as Solution Model Density (SMD) (Marenich et al., 2009) was employed to account for solvent effects. The default optimization criteria were tightened 3-fold using the internal option IOp (1/7 = 100). The geometry optimizations were followed by harmonic frequency calculations in order to verify the nature of stationary points and to derive thermochemical quantities. The thermochemical analysis was carried out using a scale factor of 0.9678 for harmonic frequencies (Kesharwani et al., 2015). The Gibbs free energy in solution (Gsoln) was calculated as follows:
where Esoln is the electronic energy in solution and ΔGcorr is the thermal correction to Gsoln, including the zero-point energy. To minimize basis set superposition errors for complexes, the Esoln values were derived from single-point calculations with the larger 6-311G(2df,2p) basis set (Krishnan et al., 1980; Frisch et al., 1984). The calculated Gsoln values were used to identify the most stable conformation for each molecular structure.
TDDFT calculations were carried out on the M06-2X/6-31G(d)/SMD geometries using the CAM-B3LYP functional (Yanai et al., 2004), the 6-311G(2d,p) basis set, and the SMD solvation model. This approach has been previously used to study the electronic transitions in a supramolecular charge-transfer complex involving bis(18-crown-6)azobenzene (Ushakov et al., 2020). It was shown that in the case of CAM-B3LYP, the energies of electronic transitions are usually significantly overestimated, but the positions of electronic transitions of different nature relative to each other are predicted quite well.
The natural transition orbitals (Martin, 2003) were derived from the TDDFT calculations as described in the Gaussian manual. The orbitals were visualized using the Chemcraft program (Zhurko, 2005).
Figure 1 shows the UV-vis absorption spectra of compounds 1 (λmax = 466 nm, εmax = 64,000 mol−1dm3cm−1), 2 (λmax = 321 nm, εmax = 44,000 mol−1dm3cm−1), and their equimolar mixture in MeCN, recorded at two different concentrations of the reactants. The fact that the absorption spectrum of the mixture measured at a low concentration of 1 and 2 (1 × 10−5 M) differs considerably from the sum of the spectra of separate components is indicative of the formation of a strong complex between 1 and 2. The complex formation induces a blue shift and a decrease in the intensity of the long-wavelength absorption band of 1 (λmax = 442 nm, εmax = 39,000 mol−1dm3cm−1), which is related to the electronic transition with charge transfer from the electron-donating phenylazacrown ether moieties to the electron-withdrawing cyclopentanone center. The increase in the energy of this transition is attributable to the decrease in the electron-donating effect of the phenylazacrown ether moieties due to hydrogen bonding with the ammonium groups of 2 (Scheme 1).
FIGURE 1. UV-vis absorption spectra in MeCN: (1) dienone 1, (2) diammonium compound 2, and (3) equimolar mixture of 1 and 2 (reactant concentrations of 1 × 10−5 M); the inset shows the spectra of the same systems but measured at reactant concentrations of 2 × 10−4 M.
A characteristic feature of the formation of organic donor–acceptor complexes with intermolecular charge transfer is the appearance of a new, low-intensity band (charge-transfer band) in the long-wavelength region of the UV-vis spectrum, which is absent in the spectra of the free donor and acceptor (Foster, 1969; Gromov et al., 2008). The absorption spectra of compounds 1 and 2 and their equimolar mixture measured at high component concentrations (2 × 10−4 M, see the inset in Figure 1) indicate that the formation of complex 1∙2, apart from inducing a blue shift of the dienone intense band, results in increased absorbance at the long-wavelength edge of the spectral curve. This fact can be due to the appearance of a weak, low-energy electronic transition characterized by intermolecular charge transfer. Parameters of the charge-transfer absorption band such as the peak position and molar absorptivity cannot be determined, as this band is superimposed by a broad intense band associated with a local transition in the dienone.
Figure 2 shows the fluorescence spectra of dienone 1 (λmax = 556 nm) and its mixture with 2 in MeCN. The formation of complex 1∙2 leads to almost complete quenching of fluorescence of 1, which supports the hypothesized charge-transfer nature of this complex. Previously, we studied the complex formation of 1 with a number of diammonium cations NH3+(CH2)nNH3+ with polymethylene chain length n = 2–12 (Fomina et al., 2020). A pseudocyclic complex was found to form in the case of the 1,12-dodecanediammonium ion 3. Good geometric matching of the components owing to the fact the distance between the ammonium groups in 3 is close to the distance between the binding sites in 1 provides rigid structure of complex 1∙3, which results in an increase in the fluorescence intensity of 1. Considering the geometric matching of the components of complex 1∙2, one could expect an increase in the fluorescence intensity of 1 upon complex formation. However, contrary to the expectations, the formation of complex 1∙2 leads to fluorescence quenching. The addition of excess diammonium salt 3 or calcium perchlorate to a solution of 1∙2 induces a fluorescence enhancement due to the destruction of the charge-transfer complex.
FIGURE 2. Uncorrected fluorescence spectra in MeCN (excitation at 428 nm): (1) dienone 1 (4 × 10−6 M), (2) a mixture of 1 and 2 in 1:8 ratio, (3) the 1:8 mixture in the presence of diammonium salt 3 in a concentration of 6 × 10−5 M, and (4) the 1:8 mixture in the presence of Ca(ClO4)2 in a concentration of 1.2 × 10−4 M.
The fluorescence quantum yields of the studied compounds are presented in Section 2.2. The main spectroscopic parameters (absorption and fluorescence) are given in Supplementary Table S1 (Supplementary Material).
The complex formation of dienone 1 with compounds 2 and 3 induces pronounced changes in the chemical shifts in the 1H NMR spectra (Figure 3). In a mixture of dienone 1 and dipyridylethylene derivative 2, most aliphatic and aromatic proton signals of 1 and 2 are shifted upfield, while in a mixture of dienone 1 with diammonium salt 3, most proton signals of compound 1 are shifted downfield. The most pronounced changes in the chemical shifts (0.11–0.38 ppm) are observed for an equimolar mixture of 1 and 2, which attests to the double-decker structure of complex 1∙2. In this complex, the central moieties of the components are located close to each other, which is responsible for the upfield shift of the signals of most protons due to mutual shielding.
FIGURE 3. 1H NMR spectra (aromatic proton region) in MeCN-d3 at 25°C: (A) compound 2, (B) equimolar mixture of dienone 1 and diammonium salt 3, and (C) equimolar mixture of 1 and 2; the reactant concentrations are 1 × 10−3 M.
More information about the structure of complex 1 with dipyridylethylene derivative 2 can be derived from the 2D NOESY spectrum of a saturated solution of a mixture of these compounds in MeCN-d3 (Supplementary Figure S6, Supplementary Material).
Figure 4 schematically shows intermolecular NOE interactions found for complex 1∙2. Apart from the intense intramolecular cross-peaks, there are peaks corresponding to the intermolecular through-space interaction of the ethylene protons and the pyridine ring protons of viologen analogue 2 with the 3-CH2 and 4-CH2 protons of the cyclopentanone ring, α-H protons of the ethylene bonds, and the 2′-H, 3′-H, 5′-H, and 6′-H benzene protons of dienone 1. All of the detected intermolecular NOE cross-peaks have low intensity compared to that of the intramolecular NOE interactions; therefore, the distances between the interacting protons can be roughly estimated to be in the 3.0–3.5 Å range.
Considering these interactions, we may propose the most probable structure for the complex of dienone 1 with dipyridylethylene derivative 2, which is shown in Scheme 1. The ammonium groups of viologen analogue 2 are bound simultaneously to two azacrown ether residues of dienone 1, and the pyridine rings and the ethylene unit of dipyridylethylene derivative 2 are located above (or near) the central moiety of molecule 1. In other words, the complex of dienone 1 with dipyridylethylene derivative 2 is a pseudocyclic structure.
The diffusion ordered NMR spectroscopy (DOSY), a sensitive method capable of distinguishing the spectra of single compounds in a mixture, is widely used in the host–guest chemistry (Gafni and Cohen, 1997; Frish et al., 1999; Frish et al., 2000; Avram, and Cohen, 2002; Cohen et al., 2005; Specht et al., 2005; Gulino et al., 2006). Compounds 1 and 2 have different diffusion coefficients (1.07−9 m2/s and 9.49−10 m2/s, respectively); a mixture of 1 and 2 shows only one diffusion coefficient (9.12−10 m2/s), which is lower than the diffusion coefficients of single 1 or 2, thus confirming the presence of a common discrete supramolecular system, complex 1·2 (the 2D DOSY spectra of 1, 2, and their equimolecular mixture are shown in Supplementary Figure S7 (Supplementary Material). Thus, analysis of 1H NMR and 2D DOSY spectra, in combination with elemental analysis data, indicates the formation of a strong supramolecular complex.
The molecular structures of dienone 1 and complex 1∙2 in MeCN were calculated using density functional theory (DFT), and the nature of the low-energy electronic transitions in these two structures was studied by time-dependent DFT (TDDFT). Details of the DFT and TDDFT calculations are given in Section 2.3, and the Cartesian coordinates for all atoms in the calculated structures are presented in the Supplementary Material (Supplementary Tables S2, S3).
Supplementary Figure S8 (Supplementary Material) shows the calculated structures of 1 and 1∙2. According to calculations, the most stable conformer of free dienone 1 in MeCN is a C2-symmetrical structure. The geometry of the dibenzylidenecyclopentanone residue of 1 is slightly non-planar; the maximal torsion angle in the cyclopentanone ring is 9.9°, and the torsion angles between the C=C bonds and the benzene rings are 8.4°.
The most stable conformer of complex 1∙2 in MeCN is an asymmetric pseudocyclic structure in which the dibenzylidenecyclopentanone residue of 1 and the dipyridylethylene residue of 2 are located one above the other. The complex formation leads to a significant distortion of the geometry of the dibenzylidenecyclopentanone residue; in the complex, this fragment looks noticeably bent and faces the dipyridylethylene residue of 2 with its convex side. The torsion angles between the C=C bonds and the benzene rings in the dibenzylidenecyclopentanone residue are 14.6° and −17.1°, and the maximal torsion angle in the cyclopentanone ring is 8.7°. In the dipyridylethylene residue of 2, the torsion angles between the C=C bond and the pyridinium rings are as low as 2.2° and 3.1°. In the calculated structure 1∙2, the intermolecular distances between the hydrogen atoms of the benzylidenecyclopentanone residue of 1 and the dipyridylethylene residue of 2 are consistent with the NOESY spectroscopy data (Section 3.2).
Table 1 shows the dominant natural transition orbital (NTO) pairs and their occupation numbers for the first excited singlet state of 1 and the first three excited singlet states of 1∙2, as derived from TDDFT calculations. It can be seen that the S0-S1 transition in free dienone 1 is accompanied by a significant charge redistribution: the electron density on the aniline residues decreases, while that in the central part of the molecule increases. The first two excited states of complex 1∙2 are characterized by intermolecular charge transfer, i.e., in both cases, excitation leads to the transition of an electron from an orbital located mainly on the donor moiety (dienone 1) to an orbital localized on the acceptor moiety (compound 2). The third excited state of 1∙2 results from a local electronic transition in the dienone moiety; this state is similar in nature to the S1 state of free dienone 1. Note that all the NTO pairs discussed here are characterized by high occupation numbers (0.92–0.98).
TABLE 1. Dominant natural transition orbital pairs and their occupation numbers for low-lying excited singlet states of dienone 1 and complex 1∙2 in MeCN; hydrogen atoms in structure 1∙2 are not shown except for those of the ammonium groups.
Table 2 presents the TDDFT-calculated energies and oscillator strengths for low-energy electronic transitions in dienone 1 and complex 1∙2. At a qualitative level, the theoretical results agree with the UV-vis absorption spectroscopy data (Section 3.1). First, the calculations predict a significant blue shift and a decrease in the intensity of the long-wavelength absorption band of dienone 1 upon the complexation with compound 2. Second, the weak S0-S1 transition in complex 1∙2 was calculated to occur at a lower energy than the intense S0-S1 transition in free dienone 1, which can explain the fact that the complex formation leads to some increase in the absorbance at the long-wavelength edge of the absorption spectrum of 1 (Section 3.1, the inset in Figure 1).
TABLE 2. Parameters of low-energy electronic transitions in dienone 1 and complex 1∙2, as calculated by TDDFT.
A novel donor–acceptor supramolecular system was developed, involving bis(azacrown)-containing dienone 1 and unsaturated viologen analog 2. The results of experimental and theoretical studies show that the pseudocyclic complex between 1 and 2, characterized by intra-supramolecular charge transfer, is a promising example of a supramolecular system capable of producing a strong fluorescence response upon the interaction with the Ca2+ and 1,12-dodecanediammonium ions. The results suggest the possibility of using complexes of this type as such photoactive supramolecular devices as supramolecular fluorescent sensors.
The original contributions presented in the study are included in the article/Supplementary Material, further inquiries can be directed to the corresponding author.
SG: Conceptualization, Funding acquisition, Project administration, Supervision, Writing–review and editing. MF: Data curation, Investigation, Visualization, Writing–original draft, Writing–review and editing. IZ: Investigation, Writing–review and editing. AF: Investigation, Visualization, Writing–review and editing. EU: Investigation, Visualization, Writing–review and editing.
The author(s) declare financial support was received for the research, authorship, and/or publication of this article. This work was supported by the Russian Science Foundation (Project No. 22-13-00064) and, in respect of DFT calculations, by the Ministry of Science and Higher Education of the Russian Federation (State Assignment No. AAAA-A19-119070790003-7), the synthesis of 1,12-dodecanediammonium diperchlorate 3 was done under financial support of the State assignment of FSRC «Crystallography and Photonics» RAS.
The authors are grateful to Dr. Artem I. Vedernikov for his help in the synthesis of compound 2.
The authors declare that the research was conducted in the absence of any commercial or financial relationships that could be construed as a potential conflict of interest.
All claims expressed in this article are solely those of the authors and do not necessarily represent those of their affiliated organizations, or those of the publisher, the editors and the reviewers. Any product that may be evaluated in this article, or claim that may be made by its manufacturer, is not guaranteed or endorsed by the publisher.
The Supplementary Material for this article can be found online at: https://www.frontiersin.org/articles/10.3389/fchem.2023.1263440/full#supplementary-material
Avram, L., and Cohen, Y. (2002). Complexation in pseudorotaxanes based on α-cyclodextrin and different α,ω-diaminoalkanes by NMR diffusion measurements. J. Org. Chem. 67, 2639–2644. doi:10.1021/jo016321q
Balzani, V., Credi, A., and Venturi, M. (2003). Molecular devices and machines – a journey into the nano world. Weinheim: Wiley-VCH Verlag GmbH & Co. KGaA. doi:10.1002/3527601600
Cohen, Y., Avram, L., and Frish, L. (2005). Diffusion NMR spectroscopy in supramolecular and combinatorial chemistry: An old parameter—new insights. Angew. Chem. Int. Ed. 44, 520–554. doi:10.1002/anie.200300637
De Silva, A. P., Gunaratne, H. Q. N., Gunnlaugsson, T., Huxley, A. J. M., McCoy, C. P., Rademacher, J. T., et al. (1997). Signaling recognition events with fluorescent sensors and switches. Chem. Rev. 97, 1515–1566. doi:10.1021/cr960386p
Fery-Forgues, S., and Al-Ali, F. (2004). Bis(azacrown ether) and bis(benzocrown ether) dyes: Butterflies, tweezers and rods in cation binding. J. Photochem. Photobiol. C 5, 139–153. doi:10.1016/j.jphotochemrev.2004.07.001
Fomina, M. V., Kurchavov, N. A., Freidzon, A.Ya., Nuriev, V. N., Vedernikov, A. I., Strelenko, Y. A., et al. (2020). Self-assembly involving hydrogen bonds. Spectral properties and structure of supramolecular complexes of bis-aza-18-crown-6-containing dienones with alkanediammonium salts. J. Photochem. Photobiol. A 402, 112801. doi:10.1016/j.jphotochem.2020.112801
Frisch, M. J., Pople, J. A., and Binkley, J. S. (1984). Self-consistent molecular orbital methods 25. Supplementary functions for Gaussian basis sets. J. Chem. Phys. 80, 3265–3269. doi:10.1063/1.447079
Frisch, M. J., Trucks, G. W., Schlegel, H. B., Scuseria, G. E., Robb, M. A., Cheeseman, J. R., et al. (2016). Gaussian 16, revision A.03. Wallingford, CT: Gaussian, Inc.
Frish, L., Matthews, S. E., Böhmer, V., and Cohen, Y. (1999). A pulsed gradient spin echo NMR study of guest encapsulation by hydrogen-bonded tetraurea calix[4]arene dimers. J. Chem. Soc. Perkin Trans. 2, 669–672. doi:10.1039/a900690g
Frish, L., Sansone, F., Casnati, A., Ungaro, R., and Cohen, Y. (2000). Complexation of a peptidocalix[4]arene, a vancomycin mimic, with alanine-containing guests by NMR diffusion measurements. J. Org. Chem. 65, 5026–5030. doi:10.1021/jo0001784
Gafni, A., and Cohen, Y. (1997). Complexes of macrocycles with γ-cyclodextrin as deduced from NMR diffusion measurements. J. Org. Chem. 62, 120–125. doi:10.1021/jo961758x
Golcs, Á., Vezse, P., Ádám, B. Á., Huszthy, P., and Tóth, T. (2021). Comparison in practical applications of crown ether sensor molecules containing an acridone or an acridine unit – A study on protonation and complex formation. J. Incl. Phenom. Macrocycl. Chem. 101, 63–75. doi:10.1007/s10847-021-01086-2
Gromov, S. P., Ushakov, E. N., Vedernikov, A. I., Lobova, N. A., Alfimov, M. V., Strelenko, Y. A., et al. (1999). A Novel optical sensor for metal ions based on ground-state intermolecular charge-transfer complexation. Org. Lett. 1, 1697–1699. doi:10.1021/ol990710d
Gromov, S. P., Vedernikov, A. I., Kuz’mina, L. G., Lobova, N. A., Basok, S. S., Strelenko, Y. A., et al. (2009). Stereoselective [2+2] photocycloaddition in bispseudosandwich complexes of bis(18-crown-6) stilbene with alkanediammonium ions. Russ. Chem. Bull. 58, 108–114. doi:10.1007/s11172-009-0016-4
Gromov, S. P., Vedernikov, A. I., Ushakov, E. N., and Alfimov, M. V. (2008). Unusual supramolecular donor—Acceptor complexes of bis(crown)stilbenes and bis(crown)azobenzene with viologen analogs. Russ. Chem. Bull. 57, 793–801. doi:10.1007/s11172-008-0118-4
Gulino, F. G., Lauceri, R., Frish, L., Evan-Salem, T., Cohen, Y., De Zorzi, R., et al. (2006). Noncovalent synthesis in aqueous solution and spectroscopic characterization of multi-porphyrin complexes. Chem. Eur. J. 12, 2722–2729. doi:10.1002/chem.200500825
Günes, S., Neugebauer, H., and Sariciftci, N. S. (2007). Conjugated polymer-based organic solar cells. Chem. Rev. 107, 1324–1338. doi:10.1021/cr050149z
Halgren, T. A. (1999). MMFF VI. MMFF94s option for energy minimization studies. J. Comp. Chem. 20, 720–729. doi:10.1002/(SICI)1096-987X(199905)20:7<720:AID-JCC7>3.0.CO;2-X
Hariharan, P. C., and Pople, J. A. (1973). The influence of polarization functions on molecular orbital hydrogenation energies. Theor. Chim. Acta 28, 213–222. doi:10.1007/BF00533485
Hehre, W. J., Ditchfield, R., and Pople, J. A. (1972). Self-consistent molecular orbital methods. XII. Further extensions of Gaussian-type basis sets for use in molecular orbital studies of organic molecules. J. Chem. Phys. 56, 2257–2261. doi:10.1063/1.1677527
Huo, Y., Zhang, H.-L., and Zhan, X. (2019). Nonfullerene all-small-molecule organic solar cells. ACS Energy Lett. 4 (6), 1241–1250. doi:10.1021/acsenergylett.9b00528
Jiang, C., Song, Z., Yu, L., Ye, S., and He, H. (2020). Fluorescent probes based on macrocyclic hosts: Construction, mechanism and analytical applications. TrAC Trends Anal. Chem. 133, 116086. doi:10.1016/j.trac.2020.116086
Ke, X.-S., Kim, T., Lynch, V. M., Kim, D., and Sessler, J. L. (2017). Flattened calixarene-like cyclic BODIPY array: A new photosynthetic antenna model. J. Am. Chem. Soc. 139, 13950–13956. doi:10.1021/jacs.7b08611
Kesharwani, M. K., Brauer, B., and Martin, J. M. L. (2015). Frequency and zero-point vibrational energy scale factors for double-hybrid density functionals (and other selected methods): Can anharmonic force fields be avoided? J. Phys. Chem. A 119, 1701–1714. doi:10.1021/jp508422u
Krishnan, R., Binkley, J. S., Seeger, R., and Pople, J. A. (1980). Self-consistent molecular orbital methods. XX. A basis set for correlated wave functions. J. Chem. Phys. 72, 650–654. doi:10.1063/1.438955
Li, J., Yim, D., Jang, W.-D., and Yoon, J. (2017). Recent progress in the design and applications of fluorescence probes containing crown ethers. Chem. Soc. Rev. 46, 2437–2458. doi:10.1039/c6cs00619a
Lian, Z., Jiang, M., Qiao, F., Chen, M.-N., Wang, R.-Zh., Zhuo, S., et al. (2020). Artificial light-harvesting supramolecular assemblies with different morphology formed by cucurbit[n]urils-based host-guest complexation. J. Photochem. Photobiol. A 386, 112135. doi:10.1016/j.jphotochem.2019.112135
Mako, T. L., Racicot, J. M., and Levine, M. (2019). Supramolecular luminescent sensors. Chem. Rev. 119, 322–477. doi:10.1021/acs.chemrev.8b00260
Marenich, A. V., Cramer, C. J., and Truhlar, D. G. (2009). Universal solvation model based on solute electron density and on a continuum model of the solvent defined by the bulk dielectric constant and atomic surface tensions. J. Phys. Chem. B 113, 6378–6396. doi:10.1021/jp810292n
Martin, R. L. (2003). Natural transition orbitals. J. Chem. Phys. 118, 4775–4777. doi:10.1063/1.1558471
Morita, Y., Murata, T., and Nakasuji, K. (2013). Cooperation of hydrogen-bond and charge-transfer interactions in molecular complexes in the solid state. Bull. Chem. Soc. Jpn. 86, 183–197. doi:10.1246/bcsj.20120241
Rurack, K. (2008). Fluorescence quantum yields: Methods of determination and standards. Resch-Genger U. Springer-Verlag Berl. Heidelb. 5, 101–145. doi:10.1007/4243_2008_019
Shakya, S., and Khan, I. M. (2021). Charge transfer complexes: Emerging and promising colorimetric real-time chemosensors for hazardous materials. J. Hazard. Mater. 403, 123537. doi:10.1016/j.jhazmat.2020.123537
Specht, A., Ziarelli, F., Bernard, P., Goeldner, M., and Peng, L. (2005). para-Sulfonated calixarenes used as synthetic receptors for complexing photolabile cholinergic ligand. Helv. Chim. Acta, 88, 2641–2653. doi, doi:10.1002/hlca.200590205
Ushakov, E. N., Alfimov, M. V., and Gromov, S. P. (2010). Crown ether-based optical molecular sensors and photocontrolled ionophores. Macroheterocycles 3 (4), 189–200. doi:10.6060/mhc2010.4.189
Ushakov, E. N., Martyanov, T. P., Vedernikov, A. I., Efremova, A. A., Moiseeva, A. A., Kuz’mina, L. G., et al. (2020). Highly stable supramolecular donor–acceptor complexes involving a bis(18-crown-6)azobenzene as weak donor: structure−property relationships. ACS Omega 5, 25993–26004. doi:10.1021/acsomega.0c03441
Ushakov, E. N., Nadtochenko, V. A., Gromov, S. P., Vedernikov, A. I., Lobova, N. A., Alfimov, M. V., et al. (2004). Ultrafast excited state dynamics of the bi- and termolecular stilbene-viologen charge-transfer complexes assembled via host–guest interactions. Chem. Phys. 298, 251–261. doi:10.1016/j.chemphys.2003.12.002
Vedernikov, A. I., Ushakov, E. N., Efremova, A. A., Kuz’mina, L. G., Moiseeva, A. A., Lobova, N. A., et al. (2011). Synthesis, structure, and properties of supramolecular charge-transfer complexes between bis(18-crown-6)stilbene and ammonioalkyl derivatives of 4,4′-bipyridine and 2,7-diazapyrene. J. Org. Chem. 76, 6768–6779. doi:10.1021/jo201172w
Wu, L., Wu, F., Sun, Q., Shi, J., Xie, A., Zhu, X., et al. (2021). A TTF–TCNQ complex: An organic charge-transfer system with extraordinary electromagnetic response behavior. J. of Mat. Chem C 9, 3316–3323. doi:10.1039/d0tc05230b
Yanai, T., Tew, D. P., and Handy, N. C. (2004). A new hybrid exchange–correlation functional using the Coulomb-attenuating method (CAM-B3LYP). Chem. Phys. Lett. 393, 51–57. doi:10.1016/j.cplett.2004.06.011
Yao, Q., Lü, B., Ji, C., Cai, Y., and Yin, M. (2017). Supramolecular host–guest system as ratiometric Fe3+ ion sensor based on water-soluble pillar[5]arene. ACS Appl. Matter. Interfaces 9, 36320–36326. doi:10.1021/acsami.7b12063
Yuan, Y.-q., Majumder, S., Yang, M.-h., and Guo, S.-r. (2020). Recent advances in catalyst-free photochemical reactions via electron-donor-acceptor (EDA) complex process. Tetrahedron Lett. 61, 151506. doi:10.1016/j.tetlet.2019.151506
Zhao, Y., and Truhlar, D. G. (2008). The M06 suite of density functionals for main group thermochemistry, thermochemical kinetics, noncovalent interactions, excited states, and transition elements: Two new functionals and systematic testing of four M06-class functionals and 12 other functionals. Theor. Chem. Acc. 120, 215–241. doi:10.1007/s00214-007-0310-x
Zhurko, G. A. (2005). Chemcraft – graphical program for visualization of quantum chemistry computations; Ivanovo, Russia. Available at: https://chemcraftprog.com.
Keywords: dienones, charge-transfer complexes, fluorescent sensor, NMR spectroscopy, electronic spectroscopy, quantum chemical calculations
Citation: Gromov SP, Fomina MV, Zdorovenko IP, Fakhrutdinov AN and Ushakov EN (2023) A novel fluorescent sensor for diammonium and metal ions based on a supramolecular charge-transfer complex of bis(aza-18-crown-6)-containing dienone. Front. Chem. 11:1263440. doi: 10.3389/fchem.2023.1263440
Received: 19 July 2023; Accepted: 20 September 2023;
Published: 03 October 2023.
Edited by:
Liming Huang, University of Nevada, United StatesReviewed by:
Arindam Mukhopadhyay, Idaho National Laboratory (DOE), United StatesCopyright © 2023 Gromov, Fomina, Zdorovenko, Fakhrutdinov and Ushakov. This is an open-access article distributed under the terms of the Creative Commons Attribution License (CC BY). The use, distribution or reproduction in other forums is permitted, provided the original author(s) and the copyright owner(s) are credited and that the original publication in this journal is cited, in accordance with accepted academic practice. No use, distribution or reproduction is permitted which does not comply with these terms.
*Correspondence: Sergey P. Gromov, c3Bncm9tb3ZAbWFpbC5ydQ==
Disclaimer: All claims expressed in this article are solely those of the authors and do not necessarily represent those of their affiliated organizations, or those of the publisher, the editors and the reviewers. Any product that may be evaluated in this article or claim that may be made by its manufacturer is not guaranteed or endorsed by the publisher.
Research integrity at Frontiers
Learn more about the work of our research integrity team to safeguard the quality of each article we publish.