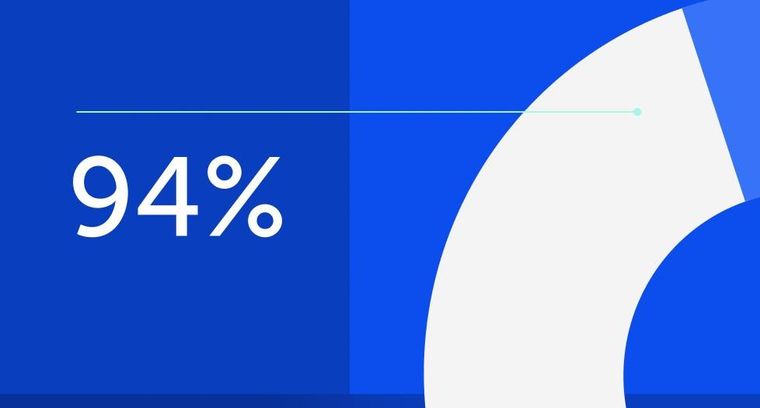
94% of researchers rate our articles as excellent or good
Learn more about the work of our research integrity team to safeguard the quality of each article we publish.
Find out more
ORIGINAL RESEARCH article
Front. Chem., 10 August 2023
Sec. Organic Chemistry
Volume 11 - 2023 | https://doi.org/10.3389/fchem.2023.1259609
This article is part of the Research TopicHigh Color Purity Boron-Based OLED MaterialsView all 5 articles
The design and synthesis of a type of [1 + 4 + 2] four-layer framework have been conducted by taking advantage of Suzuki–Miyaura cross-coupling and group-assisted purification (GAP) chemistry. The optimized coupling of double-layer diboronic esters with 1-bromo-naphth-2-yl phosphine oxides resulted in a series of multilayer folding targets, showing a broad scope of substrates and moderate to excellent yields. The final products were purified using group-assisted purification chemistry/technology, achieved simply by washing crude products with 95% EtOH without the use of chromatography and recrystallization. The structures were fully characterized and assigned by performing X-ray crystallographic analysis. UV–vis absorption, photoluminescence (PL), and aggregation-induced emission (AIE) were studied for the resulting multilayer folding products.
The layered organic structures, including chiral structures, play an important role in biological and material sciences (Moser et al., 1987; Gellman, 1998; Oh et al., 2001; Nakano, 2010; Knouse et al., 2018). The design of these targets is highly demanded to search for desired chemical, physical, and biological properties. This is particularly applicable to the research on multilayer monomers, oligomers, and polymers, which exhibit photoelectronic properties (Wu et al., 2019; Tang et al., 2022a; Tang et al., 2022b; Wang et al., 2022; Xia et al., 2023). For example, a through-space transfer through singlet fission (SF) was proven to involve the absorption of photons by two electronically interacting chromophores to generate a singlet exciton state, which is followed by the rapid formation of two triplet excitons (Chen et al., 2018). Meanwhile, charge-transfer pathways for hybridizing σ and π, and through-space interactions have been proven to be feasible by designing monomeric structures for poly- or copolymerizations (Shen and Chen, 2012; Chen and Shen, 2016; Kawashima et al., 2020; Stará and Starý, 2020; Fujise et al., 2021).
On the other hand, organophosphorus compounds, such as phosphine oxides, are widely applied in a wide range of fields, including medicinal chemistry (Alexandre et al., 2011; Dang et al., 2011), natural products (Kumar et al., 2010), biochemistry (George and Veis, 2008; Chen et al., 2012), catalysis (as catalysts and ligands) (Ackermann et al., 2005; Wang and Wan, 2011), and functional materials (Baumgartner and Réau, 2006; Kirumakki et al., 2009; Baumgartner, 2014). Considering these diverse applications, various methods have been developed for synthesizing these phosphorus-containing compounds (Yin and Buchwald, 2000; Murray et al., 2014; Zhou et al., 2014; Ji et al., 2020; Qian et al., 2020). Innovations in producing organophosphorus compounds, especially those associated with phosphine-containing axial skeletons, have become an attractive topic in chemical synthesis and industry.
In the past several years, our group has reported new multi-layer folding chirality of a series of molecules, including oligomers and polymers with structural flexibility, displaying physical properties on UV/Vis absorption, fluorescence, electrochemical performance, aggregation-induced emission (AIE) (Wu et al., 2019; Wu et al., 2019; Liu et al., 2020; Wu et al., 2020; Wu et al., 2021a; Jin et al., 2022a; Tang et al., 2022a; Tang et al., 2022b; Wang et al., 2022), and aggregation-induced polarization (AIP) (Tang et al., 2022c; Tang et al., 2022d). Among them are three-layer compounds (Scheme 1), in which electron-rich (Tang et al., 2022b) and electron-deficient (Jin et al., 2022a) bridges showed distinct impacts on UV–vis absorption and fluorescence behaviors. It is worth noting that many of these compounds showed fluorescence not only in solutions but also in solid states. Very recently, we have established the asymmetric catalytic approach to a [1 + 3+1] type of multi-layer 3D chirality containing the phosphine oxide moiety (Wu et al., 2021a) via chiral amide-phosphine ligands for Suzuki–Miyaura cross-couplings, in which a single asymmetric C–C bond formation led to the efficient control of three-layer chirality.
After achieving the synthesis of three-layer folding chiral targets, our attention is now focused on the design and assembly of four-layered compounds, starting from their racemic counterparts. In the new molecular framework, there are three planar units, including one naphthyl ring, four packed phenyl rings, and two parallel naphthyl rings, which are categorized as a type of [1 + 4 + 2] framework. This is inspired by our early work on the [1 + 3 + 1] framework, in which one packed plane is provided by the (P=O)Ph2 group (Wu et al., 2021a). Herein, we report our preliminary results on this endeavor based on new designs and modifications to reaction conditions (Scheme 1).
Retro-synthetic analysis (Corey and Cheng, 2009) revealed that there are several strategies to assemble the four-layer 3D molecular framework. These strategies are mainly based on utilizing dual Suzuki–Miyaura cross-couplings (Miyaura and Suzuki, 1995) as the key steps, as represented by the case of target 8a, in which two fragments (diboronic ester 1aa and bromide 1a) would be joined (Figure 1). In our previous synthesis, boronic esters proved to be more effective than boronic acids in multilayer synthesis via Suzuki–Miyaura couplings. Therefore, they were selected for the present assembly. We made many efforts to synthesizing diboronic ester 1aa for this purpose, but we failed. Similarly, low chemical yields were encountered during the synthesis of diphenyl(1-(4-(4,4,5,5-tetramethyl-1,3,2-dioxaborolan-2-yl)phenyl)naphthalen-2-yl)phosphine oxide 8a. For this reason, they are excluded from our RSA design. Two key precursors, 1,8-bis(4-(4,4,5,5-tetramethyl-1,3,2-dioxaborolan-2- yl)phenyl)naphthalene 6a and (1-bromonaphthalen-2-yl)diphenylphosphine oxide 7a, can be conveniently obtained, making us choose it as the major route (the top part of Figure 1) for this work. The precursor 6a was readily derived from the carbon–boron coupling of naphthalene-1,8-diylbis (4,1-phenylene) bis(trifluoromethanesulfonate) 5a, which originated from the dual Suzuki–Miyaura cross-couplings of 1,8-dibromonaphthalene 1a with (4-methoxyphenyl)boronic acid 2, both of which are commercially available.
The assembly was represented by the synthesis of targets 6a and 6b, in which different conditions are explored for two steps to achieve higher efficiencies (Scheme 2). The synthesis of the building block 6a was started from Suzuki–Miyaura coupling of 1,8-dibromonaphthalene 1a with (4-methoxyphenyl)boronic acid 2 by employing Pd(OAc)2 as a catalyst and K2CO3 as a base in the DMF/H2O co-solvent at 100°C, leading to the formation of 1,8-bis(4-methoxyphenyl)naphthalene 3a in an 85% yield. The precursor 3a was transformed into 4,4'-(naphthalene-1,8-diyl)diphenol 4a via demethylation in the presence of BBr3 in DCM by changing the temperature from −10°C to room temperature to afford an 88% yield. The precursor 4a was allowed to react with excess trifluoromethanesulfonic anhydride (Tf2O) by using pyridine and 4-dimethylaminopyridine (DMAP) as bases to yield naphthalene-1,8-diylbis (4,1-phenylene) bis(trifluoromethanesulfonate) 5a in a 96% yield. The reaction between 5a and bis(pinacolato)diboron (B2Pin2) in in situ catalytic systems involve using KOAc as a base additive and (1,1-bis(diphenylphosphino)ferrocene) dichloropalladium (II) as a catalyst in 1,4-dioxane at 80°C to afford 1,8-bis(4-(4,4,5,5-tetramethyl-1,3,2-dioxaborolan-2-yl)phenyl)naphthalene 6a as double-layer reactants (Scheme 2A). The synthesis of the building block of 5,6-bis(4-(4,4,5,5-tetramethyl-1,3,2-dioxaborolan-2-yl)phenyl)-1,2-dihydroacenaphthylene 6b was also started from Suzuki–Miyaura coupling by treating 5,6-dibromo-1,2-dihydroacenaphthylene 1b with (4-methoxyphenyl)boronic acid 2 by using Pd(PPh3)4 as the catalyst and Na2CO3 as the base in DMF/H2O as a mixed solvent at 100°C, to yield 5,6-bis(4-methoxyphenyl)-1,2-dihydroacenaphthylene 3b in a 57% yield. The precursor 3b was converted into 4,4'-(1,2-dihydroacenaphthylene-5,6-diyl)diphenol 4b via demethylation in the presence of BBr3 in DCM by gradually changing temperature from −78°C to room temperature to afford an 86% yield. The two steps shown in Scheme 2B were performed under the same conditions as the aforementioned synthetic route to yield (1,2-dihydroacenaphthylene-5,6-diyl)bis (4,1-phenylene) bis(trifluoromethanesulfonate) 5b and 5,6-bis(4-(4,4,5,5-tetramethyl-1,3,2-dioxaborolan-2-yl)phenyl)-1,2-dihydroacenaphthylene 6b chemical yields of 92% and 87%, respectively.
The synthesis of another key precursor is represented by the generation of (1-bromonaphthalen-2-yl)diarylphosphine oxides 7 by the following literature procedures (Wu et al., 2021a). It was started with the protection of 1-bromo-2-naphthol with Tf2O to yield 1-bromonaphthalen-2-yl trifluoromethanesulfonate in the presence of pyridine. The second step was conducted through the C-P coupling with diaryl phosphine oxide by taking advantage of Pd2 (dba)3 and 1,3-bis(diphenylphosphino)propane (DPPP) as the catalytic combination (Ji et al., 2020). In addition, substrates 7b–7o were synthesized starting with the nucleophilic substitution of diethyl phosphite with arylmagnesium bromide to yield bisaryl phosphine oxides, followed by subjecting to the catalytic coupling with 1-bromonaphthalen-2-yl trifluoromethanesulfonate.
The final step was to assemble the four-layer targets by treating 1,8-bis(4-(4,4,5,5-tetramethyl-1,3,2-dioxaborolan-2-yl)phenyl)naphthalene 6a with (1-bromonaphthalen-2-yl)diarylphosphine oxides 7a in the presence of a Pd(PPh3)4 catalyst as the key step, delivering various four-layered folding phosphine oxides 8a in good yields (Scheme 1). At this step, it is necessary to optimize the conditions since Suzuki–Miyaura coupling between the double-layer diboronic ester 6a and bromide 7a did not result in ideal yields under the aforementioned catalytic systems. Different catalysts, solvents, and bases were screened, and the results are shown in Table 1 (entries 1–9). In the beginning, the reaction of 6a and 7a in a 1:2.5 mol ratio was carried out in the presence of 10 mol% Pd(PPh3)4 and 3.0 equiv of K2CO3 in THF/H2O (5:1, v/v) at 90 °C for 48 h, and the desired [1 + 4+1] multilayered 3D product 8a was obtained in 86% yield through dual Suzuki–Miyaura couplings (entry 1). Other Pd catalysts, including Pd2 (dba)3, PdCl2, and Pd(OAc)2, were then examined together with K2CO3 in this transformation, but all yielded unsatisfactory results (entries 2–4). Similarly, experimentation with various solvent systems, such as toluene/H2O, DME/H2O, and 1,4-dioxane/H2O did not produce satisfactory results either (entries 5–7). We next attempted to optimize conditions by exploiting K3PO4 and Cs2CO3 as bases and found that both attempts did not show poor chemical yields of 37% and 40%, respectively (entries 8 and 9).
TABLE 1. Optimization of the reaction conditionsa.
Since there are two polar -POPh2 groups existing in the products, the purification of resulting crude products at this step can be readily obtained through the group-assisted purification (GAP) (Kaur et al., 2010; Kaur et al., 2011; An et al., 2015) chemistry/technology, eliminating the need for chromatography and recrystallization. The pure product 8a and its derivatives 8b—8p were conveniently obtained by simply washing the crude products with 95% EtOH, making this synthesis much greener and environmentally friendly.
Having established the optimal reaction conditions, we next investigated the scope of the double Suzuki–Miyaura cross-coupling reaction by using a variety of preformed 2-diarylphosphinyl-1-naphthyl bromide 7. As shown in Scheme 3, the influence of substituents in the aryl moiety of 7 was first evaluated. The reactions of 2-diarylphosphinyl-1-naphthyl bromide 7 with either electron-rich groups (Me 7b, OMe 7c, Ph 7d, SMe 7e, and NMe2 7f) or electron-poor groups (F 7g, Cl 7h, and OCF3 7i) at the para position of the aryl moiety of 7 could tolerate this reaction system, leading to the corresponding products 8b–8i in 54%–91% yield. Similarly, the meta-substituent of the aryl unit of 7 (Me 7j, OMe 7k, and F 7l) still showed a high reactivity profile, providing access to the corresponding multilayered 3D products 7j–7l in 62%–93% yield. It is noteworthy that the ortho-methyl substituted analog 7m was a suitable surrogate for this coupling reaction, which could work smoothly to deliver the product 8m in 52% yield. To our delight, both 3,5-dimethyl-substituted arylphosphine oxide 7n and 1-naphthyl-substituted phosphine oxide 7o were adopted to demonstrate the compatibility of this protocol and furnished the target products 8n–8o in 88% and 64% yields, respectively. Furthermore, the dihydroacenaphthylene-derived double-layer diboronic ester 6b was then allowed to react with 2-diphenylphosphinyl-1-naphthyl bromide 7a under standard conditions. As anticipated, the reactions proceeded smoothly, enabling the Pd-catalyzed coupling to yield the corresponding product 8p in 87% yield. The structures of all products were fully characterized by carbon and proton NMR spectroscopic and HRMS analyses.
Furthermore, the resulting multilayer framework has been unambiguously assigned by X-ray structural analysis of one of the products, 8a (Figure 2). This structure clearly presents two groups of nearly parallel units: four phenyl rings in the middle and two naphthyl rings at one end. These two planar units plus a single naphthyl ring anchor consist of a [1 + 4+2] multilayer framework, which added one more layer in the middle, as compared with our previous [1 + 3+1] type of multi-layer counterparts in the middle columns of their structures. It should be noted that our preliminary results on assembling a maximum of five-layer counterparts show promising results, leading to a [2 + 5+2] multilayer framework.
Among the products listed in Figure 3 and Figure 4, several representatives, 8a, 8c, 8g, 8i, and 8n, were examined for their behaviors on UV–vis absorption, photoluminescence (PL), and aggregation-induced emission (AIE). As shown in Figure 3A, UV-vis absorption spectra were recorded for these compounds with the same concentration in THF (Figure 3A). The highest absorptions of four samples (8a, 8g, 8i, and 8n) displayed wide absorption between 280 nm and 360 nm, except for molecule 8c. The wide absorption of 8c was observed to be between 260 and 210 nm; the highest position appeared at 280 nm, and the second highest position was around 340 nm.
FIGURE 3. (A) UV–vis absorbance of 8a, 8c, 8g, 8i, and 8n (0.1 mM) in THF; c = 0.1 mM. (B) Photoluminescence (PL) spectra of aforementioned five samples in THF; c = 0.1 mM; λex (8a, 8c, 8i, and 8g) = 344 nm; λex (8n) = 352 nm.
FIGURE 4. Photoluminescence (PL) spectra of 8n in cosolvents of THF/H2O with different fractions (fw); c = 0.1 mM; λex (8n) = 352 nm; inset: fluorescence photographs of 8n in the THF/water system.
The photoluminescence spectra of these compounds upon excitation exhibit bands at slightly different curves with regard to emission strengths and wavelengths (Figure 3B). Excitation wavelengths at 344 nm for 8a, 8c, 8i, and 8g and at 352 nm for 8n were utilized for measuring their photoluminescence. As shown in the spectrum, 8c displays its maximum emission at 400 nm. As compared with 8c, 8a displays its maximum emission at 430 nm shifted downfield and 8a, 8g, and 8i at 344 nm shifted upfield, respectively. The PL performance seems complicated, being attributed to solvent–target interactions and electronic and conformational steric effects of aromatic rings attached to the phosphorus center. Compared with 8a, which has no functional group on its two phenyl rings of the P=O center, the presence of both the electron-donating (OMe in 8c) and electron-withdrawing groups (CF3O and F in 8g and 8i, respectively) and the steric effect (two methyl groups in 8n) all resulted in upfield emission.
Fluorescence spectroscopic analysis was conducted using 8n as a representative for aggregation-induced emission (AIE). As shown in Figure X, the water fractions (fw) were increased from 0% to 60%, resulting in a steady emission enhancement from 352 nm to 555 nm. This emission change is attributed to the intermolecular packing of the molecular matrix, indicating the existence of aggregation-induced emission (AIE) by this four-layer compound. Although an obvious GAP exists between emission in fw = 0% (in pure THF) and the other four fw slots, the emission at the later four curves (10%–60%) does not display obvious differences.
As usual, the intermolecular aggregation largely suppresses the rotational motions of aromatic rings so that the exciton energy cannot be depleted by the radiation-less decay, thus making the present AIE observation possible. The intermolecular packing process would have an impact on the intramolecularly layered framework, but in a diluted environment, the movements between molecules would diminish as the poor solvent (water) became more prevalent. The intramolecular stacking would become more regular and predominantly controlled by suppressing the whole framework while water was added to the solvent mixture as soon as the acceptable saturation value of fw = 60% was reached. The partial AIE activities could also exist because of this compressed packing model’s contribution to more efficient through-space interactions. This result is in accordance with our earlier research on multilayer molecules (Wu et al., 2021a; Wu et al., 2021b; Wang et al., 2022).
An interesting shape of emission appeared in pure and transparent THF, which indicates some degrees of molecular aggregation exist in this system. This observation would benefit organic synthesis during condition modifications by taking advantage of aggregates. It should be noted that THF is among the most common solvents in organic synthesis, particularly in asymmetric synthesis and catalysis. Our laboratory has recently proven that chiral aggregates can enhance asymmetric control and can even switch the stereo configuration of resulting chiral products (Rouh et al., 2022; Tang et al., 2023). Chiral aggregates were directly confirmed by AIE, AIP (aggregation-induced polarization) (Tang et al., 2022c; Tang et al., 2022d), and dynamic light scattering (DLS) experiments in THF-water and THF-ethanol co-solvents. Both stoichiometric and catalytic asymmetric reactions have been carried out successfully, defined as aggregation-induced asymmetric synthesis (AIAS) (Tang et al., 2023) and aggregation-induced asymmetric catalysis (AIAC) (Jin et al., 2022b).
In summary, a new [1 + 4+2] framework of multilayer targets has been successfully designed and synthesized. Starting from commercial starting materials, more than 40 steps were performed for generating 16 multilayer folding products bearing various phosphine oxides. The synthesis takes advantage of modified dual Suzuki–Miyaura cross-couplings and GAP chemistry/technology simply by washing with 95% EtOH without the use of chromatography and recrystallization. The structures were fully characterized by spectroscopic analysis and assigned by X-ray crystallographic determination. The physical properties of UV–vis absorption, photoluminescence (PL), and aggregation-induced emission (AIE) were studied for the resulting multilayer folding products. Further research on the asymmetric synthesis and catalysis for generating the chiral [1 + 4+2] framework of four-layer counterparts and its attachment onto orientational chirality (Jin et al., 2022b; Jin et al., 2022c) is currently being conducted in our laboratories, and the results will be reported in due course.
The original contributions presented in the study are included in the article/Supplementary Material; further inquiries can be directed to the corresponding authors.
GL: supervision and writing–original draft. J-YW: supervision and writing–original draft. SZ: writing–original draft and investigation. DC: writing–original draft and investigation. SY: investigation and writing–original draft.
The authors declare that financial support was received for the research, authorship, and/or publication of this article from the Robert A. Welch Foundation (D-1361-20210327, USA) and the National Natural Science Foundation of China (nos 22071102 and 91956110).
The authors thank Yao Tang and Qingkai Yuan for their assistance.
The authors declare that the research was conducted in the absence of any commercial or financial relationships that could be construed as a potential conflict of interest.
The authors declared that they were an editorial board member of Frontiers, at the time of submission. This had no impact on the peer review process and the final decision.
All claims expressed in this article are solely those of the authors and do not necessarily represent those of their affiliated organizations, or those of the publisher, the editors, and the reviewers. Any product that may be evaluated in this article, or claim that may be made by its manufacturer, is not guaranteed or endorsed by the publisher.
The Supplementary Material for this article can be found online at: https://www.frontiersin.org/articles/10.3389/fchem.2023.1259609/full#supplementary-material
Ackermann, L., Born, R., Spatz, J. H., and Meyer, D. (2005). Efficient aryl-(hetero)aryl coupling by activation of C-Cl and C-F bonds using nickel complexes of air-stable phosphine oxides. Angew. Chem. Int. Ed. Engl. 44, 7216–7219. doi:10.1002/anie.200501860
Alexandre, F.-R., Amador, A., Bot, S., Caillet, C., Convard, T., Jakubik, J., et al. (2011). Synthesis and biological evaluation of aryl-phospho-indole as novel HIV-1 non-nucleoside reverse transcriptase inhibitors. J. Med. Chem. 54, 392–395. doi:10.1021/jm101142k
An, G., Seifert, C., and Li, G. (2015). N-Phosphonyl/phosphinyl imines and group-assisted purification (GAP) chemistry/technology. Org. Biomol. Chem. 13, 1600–1617. doi:10.1039/c4ob02254h
Baumgartner, T. (2014). Insights on the design and electron-acceptor properties of conjugated organophosphorus materials. Acc. Chem. Res. 47, 1613–1622. doi:10.1021/ar500084b
Baumgartner, T., and Réau, R. (2006). Organophosphorus pi-conjugated materials. Chem. Rev. 106, 4681–4727. doi:10.1021/cr040179m
Chen, C.-F., and Shen, Y. (2016). Helicene chemistry: From synthesis to applications. 1. Berlin, Germany: Springer. Available at: https://books.google.at/books?id=JkpxDQAAQBAJ.
Chen, M., Bae, Y. J., Mauck, C. M., Mandal, A., Young, R. M., and Wasielewski, M. R. (2018). Singlet fission in covalent terrylenediimide dimers: Probing the nature of the multiexciton state using femtosecond mid-infrared spectroscopy. J. Am. Chem. Soc. 140, 9184–9192. doi:10.1021/jacs.8b04830
Chen, X., Kopecky, D. J., Mihalic, J., Jeffries, S., Min, X., Heath, J., et al. (2012). Structure-guided design, synthesis, and evaluation of guanine-derived inhibitors of the eIF4E mRNA-cap interaction. J. Med. Chem. 55, 3837–3851. doi:10.1021/jm300037x
Corey, E. J., and Cheng, X.-M. (2009). The logic of chemical synthesis. Newy York: Wiley-Interscience.
Dang, Q., Liu, Y., Cashion, D. K., Kasibhatla, S. R., Jiang, T., Taplin, F., et al. (2011). Discovery of a series of phosphonic acid-containing thiazoles and orally bioavailable diamide prodrugs that lower glucose in diabetic animals through inhibition of fructose-1,6-bisphosphatase. J. Med. Chem. 54, 153–165. doi:10.1021/jm101035x
Fujise, K., Tsurumaki, E., Wakamatsu, K., and Toyota, S. (2021). Construction of helical structures with multiple fused anthracenes: Structures and properties of long expanded helicenes. Chemistry 27, 4548–4552. doi:10.1002/chem.202004720
George, A., and Veis, A. (2008). Phosphorylated proteins and control over apatite nucleation, crystal growth, and inhibition. Chem. Rev. 108, 4670–4693. doi:10.1021/cr0782729
Ji, W., Wu, H.-H., and Zhang, J. (2020). Axially chiral biaryl monophosphine oxides enabled by palladium/WJ-Phos-catalyzed asymmetric Suzuki–miyaura cross-coupling. ACS Catal. 10, 1548–1554. doi:10.1021/acscatal.9b04354
Jin, S., Wang, J.-Y., Tang, Y., Rouh, H., Zhang, S., Xu, T., et al. (2022a). Central-to-folding chirality control: Asymmetric synthesis of multilayer 3D targets with electron-deficient bridges. Front. Chem. 10, 860398. doi:10.3389/fchem.2022.860398
Jin, S., Wang, Y., Tang, Y., Wang, J.-Y., Xu, T., Pan, J., et al. (2022b). Orientational chirality, its asymmetric control, and computational study. Res. (Wash. D.C.) 2022. doi:10.34133/research.0012
Jin, S., Xu, T., Tang, Y., Wang, J.-Y., Wang, Y., Pan, J., et al. (2022c). A new chiral phenomenon of orientational chirality, its synthetic control and computational study. Front. Chem. 10, 1110240. doi:10.3389/fchem.2022.1110240
Kaur, P., Pindi, S., Wever, W., Rajale, T., and Li, G. (2010). Asymmetric catalytic N-phosphonyl imine chemistry: The use of primary free amino acids and Et2AlCN for asymmetric catalytic strecker reaction. J. Org. Chem. 75, 5144–5150. doi:10.1021/jo100865q
Kaur, P., Wever, W., Pindi, S., Milles, R., Gu, P., Shi, M., et al. (2011). The GAP chemistry for chiral N-phosphonyl imine-based Strecker reaction. Green Chem. 13, 1288. doi:10.1039/c1gc15029d
Kawashima, T., Matsumoto, Y., Sato, T., Yamada, Y. M. A., Kono, C., Tsurusaki, A., et al. (2020). Synthesis, structure, and complexation of an S-shaped double azahelicene with inner-edge nitrogen atoms. Chem. Eur. J. 26, 13170–13176. doi:10.1002/chem.202002405
Kirumakki, S., Huang, J., Subbiah, A., Yao, J., Rowland, A., Smith, B., et al. (2009). Tin(IV) phosphonates: Porous nanoparticles and pillared materials. J. Mater. Chem. 19, 2593–2603. doi:10.1039/B818618A
Knouse, K. W., deGruyter, J. N., Schmidt, M. A., Zheng, B., Vantourout, J. C., Kingston, C., et al. (2018). Unlocking P(V): Reagents for chiral phosphorothioate synthesis. Science 361, 1234–1238. doi:10.1126/science.aau3369
Kumar, T. S., Zhou, S.-Y., Joshi, B. V., Balasubramanian, R., Yang, T., Liang, B. T., et al. (2010). Structure−Activity relationship of (N)-methanocarba phosphonate analogues of 5′-AMP as cardioprotective agents acting through a cardiac P2X receptor. J. Med. Chem. 53, 2562–2576. doi:10.1021/jm9018542
Liu, Y., Wu, G., Yang, Z., Rouh, H., Katakam, N., Ahmed, S., et al. (2020). Multi-layer 3D chirality: New synthesis, AIE and computational studies. Sci. China Chem. 63, 692–698. doi:10.1007/s11426-019-9711-x
Miyaura, N., and Suzuki, A. (1995). Palladium-catalyzed cross-coupling reactions of organoboron compounds. Chem. Rev. 95, 2457–2483. doi:10.1021/cr00039a007
Moser, H. E., and Dervan, P. B. (1987). Sequence-specific cleavage of double helical DNA by triple helix formation. Science 238, 645–650. doi:10.1126/science.3118463
Murray, J. I., Woscholski, R., and Spivey, A. C. (2014). Highly efficient and selective phosphorylation of amino acid derivatives and polyols catalysed by 2-aryl-4-(dimethylamino)pyridine-N-oxides--towards kinase-like reactivity. Chem. Commun. (Camb.) 50, 13608–13611. doi:10.1039/c4cc05388e
Nakano, T. (2010). Synthesis, structure and function of π-stacked polymers. Polym. J. 42, 103–123. doi:10.1038/pj.2009.332
Oh, K., Jeong, K. S., and Moore, J. S. (2001). Folding-driven synthesis of oligomers. Nature 414, 889–893. doi:10.1038/414889a
Qian, Y., Dai, Q., Li, Z., Liu, Y., and Zhang, J. (2020). O-phosphination of aldehydes/ketones toward phosphoric esters: Experimental and mechanistic studies. Org. Lett. 22, 4742–4748. doi:10.1021/acs.orglett.0c01537
Rouh, H., Tang, Y., Xu, T., Yuan, Q., Zhang, S., Wang, J.-Y., et al. (2022). Aggregation-induced synthesis (AIS): Asymmetric synthesis via chiral aggregates. Res. (Wash. D.C.) 2022, 9865108. doi:10.34133/2022/9865108
Shen, Y., and Chen, C.-F. (2012). Helicenes: Synthesis and applications. Chem. Rev. 112, 1463–1535. doi:10.1021/cr200087r
Stará, I. G., and Starý, I. (2020). Helically chiral aromatics: The synthesis of helicenes by [2 + 2 + 2] cycloisomerization of π-electron systems. Acc. Chem. Res. 53, 144–158. doi:10.1021/acs.accounts.9b00364
Tang, Y., Jin, S., Zhang, S., Wu, G.-Z., Wang, J.-Y., Xu, T., et al. (2022a). Multilayer 3D chiral folding polymers and their asymmetric catalytic assembly. Res. (Wash. D.C.) 2022, 9847949. doi:10.34133/2022/9847949
Tang, Y., Wang, Y., Yuan, Q., Zhang, S., Wang, J.-Y., Jin, S., et al. (2023). Aggregation-induced catalysis: Asymmetric catalysis with chiral aggregates. Res. (Wash. D.C.) 6, 0163. doi:10.34133/research.0163
Tang, Y., Wu, G., Jin, S., Liu, Y., Ma, L., Zhang, S., et al. (2022b). From center-to-multilayer chirality: Asymmetric synthesis of multilayer targets with electron-rich bridges. J. Org. Chem. 87, 5976–5986. doi:10.1021/acs.joc.2c00234
Tang, Y., Yuan, Q., Wang, Y., Zhang, S., Wang, J.-Y., Jin, S., et al. (2022c). Aggregation-induced polarization (AIP) of derivatives of BINOL and BINAP. RSC Adv. 12, 29813–29817. doi:10.1039/d2ra05597j
Tang, Y., Zhang, S., Xu, T., Yuan, Q., Wang, J.-Y., Jin, S., et al. (2022d). Aggregation-induced polarization (AIP): Optical rotation amplification and adjustment of chiral aggregates of folding oligomers and polymers. Front. Chem. 10, 962638. doi:10.3389/fchem.2022.962638
Wang, H., and Wan, B. (2011). Cu-catalyzed coupling of aryl iodides with thiols using carbonyl-phosphine oxide ligands. Cuihua Xuebao/Chin. J. Catal. 32, 1129–1132. doi:10.1016/s1872-2067(10)60243-4
Wang, J.-Y., Tang, Y., Wu, G.-Z., Zhang, S., Rouh, H., Jin, S., et al. (2022). Asymmetric catalytic assembly of triple-columned and multilayered chiral folding polymers showing aggregation-induced emission (AIE). Chem. Eur. J. 28, e202104102. doi:10.1002/chem.202104102
Wu, G., Liu, Y., Rouh, H., Ma, L., Tang, Y., Zhang, S., et al. (2021a). Asymmetric catalytic approach to multilayer 3D chirality. Chem. Eur. J. 27, 8013–8020. doi:10.1002/chem.202100700
Wu, G., Liu, Y., Yang, Z., Jiang, T., Katakam, N., Rouh, H., et al. (2020). Enantioselective assembly of multi-layer 3D chirality. Natl. Sci. Rev. 7, 588–599. doi:10.1093/nsr/nwz203
Wu, G., Liu, Y., Yang, Z., Katakam, N., Rouh, H., Ahmed, S., et al. (2019). Multilayer 3D chirality and its synthetic assembly. Res. (Wash. D.C.) 2019, 6717104. doi:10.34133/2019/6717104
Wu, G., Liu, Y., Yang, Z., Ma, L., Tang, Y., Zhao, X., et al. (2021b). Triple-columned and multiple-layered 3D polymers: Design, synthesis, aggregation-induced emission (AIE), and computational study. Res. (Wash. D.C.) 2021, 3565791. doi:10.34133/2021/3565791
Xia, Q., Qin, A., and Tang, B. Z. (2023). Recent advances in chiral AIE polymers. J. Nanopart. Res. 25, 17. doi:10.1007/s11051-022-05657-3
Yin, J., and Buchwald, S. L. (2000). A catalytic asymmetric Suzuki coupling for the synthesis of axially chiral biaryl compounds. J. Am. Chem. Soc. 122, 12051–12052. doi:10.1021/ja005622z
Keywords: multilayer folding molecules, Suzuki–Miyaura coupling, GAP chemistry, phosphine oxides, AIE
Citation: Zhang S, Chen D, Wang J-Y, Yan S and Li G (2023) Four-layer folding framework: design, GAP synthesis, and aggregation-induced emission. Front. Chem. 11:1259609. doi: 10.3389/fchem.2023.1259609
Received: 16 July 2023; Accepted: 27 July 2023;
Published: 10 August 2023.
Edited by:
Zhendong Jin, The University of Iowa, United StatesCopyright © 2023 Zhang, Chen, Wang, Yan and Li. This is an open-access article distributed under the terms of the Creative Commons Attribution License (CC BY). The use, distribution or reproduction in other forums is permitted, provided the original author(s) and the copyright owner(s) are credited and that the original publication in this journal is cited, in accordance with accepted academic practice. No use, distribution or reproduction is permitted which does not comply with these terms.
*Correspondence: Jia-Yin Wang, d2p5Y2hlbUBjY3p1LmVkdS5jbg==; Guigen Li, Z3VpZ2VuLmxpQHR0dS5lZHU=
Disclaimer: All claims expressed in this article are solely those of the authors and do not necessarily represent those of their affiliated organizations, or those of the publisher, the editors and the reviewers. Any product that may be evaluated in this article or claim that may be made by its manufacturer is not guaranteed or endorsed by the publisher.
Research integrity at Frontiers
Learn more about the work of our research integrity team to safeguard the quality of each article we publish.