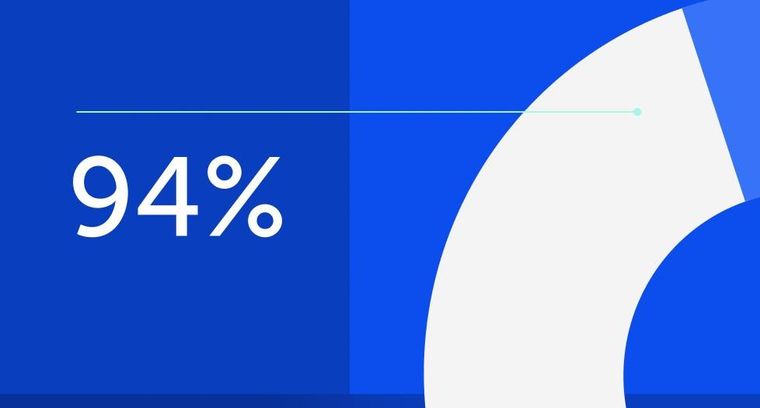
94% of researchers rate our articles as excellent or good
Learn more about the work of our research integrity team to safeguard the quality of each article we publish.
Find out more
REVIEW article
Front. Chem., 12 September 2023
Sec. Electrochemistry
Volume 11 - 2023 | https://doi.org/10.3389/fchem.2023.1253959
This article is part of the Research TopicElectrochemistry and Catalytic Reactions Editor's Pick 2024View all 10 articles
Electrochemical energy storage technology has attracted widespread attention due to its low cost and high energy efficiency in recent years. Among the electrochemical energy storage technologies, sodium ion batteries have been widely focused due to the advantages of abundant sodium resources, low price and similar properties to lithium. In the basic structure of sodium ion battery, the electrolyte determines the electrochemical window and electrochemical performance of the battery, controls the properties of the electrode/electrolyte interface, and affects the safety of sodium ion batteries. Organic liquid electrolytes are widely used because of their low viscosity, high dielectric constant, and compatibility with common cathodes and anodes. However, there are problems such as low oxidation potential, high flammability and safety hazards. Therefore, the development of novel, low-cost, high-performance organic liquid electrolytes is essential for the commercial application of sodium ion batteries. In this paper, the basic requirements and main classifications of organic liquid electrolytes for sodium ion batteries have been introduced. The current research status of organic liquid electrolytes for sodium ion batteries has been highlighted, including compatibility with various types of electrodes and electrochemical properties such as multiplicative performance and cycling performance of electrode materials in electrolytes. The composition, formation mechanism and regulation strategies of interfacial films have been explained. Finally, the development trends of sodium ion battery electrolytes in terms of compatibility with materials, safety and stable interfacial film formation are pointed out in the future.
Fossil fuels are the most widely used energy source in the world, however, its non-renewable and unsustainable nature makes it increasingly depleted, and the burning of fossil fuels causes a series of problems such as global warming and atmospheric pollution (Gao et al., 2020). Therefore, the development of renewable energy is becoming more and more important. However, the conversion of renewable energy into electrical energy is variable, intermittent and unpredictable (Cao et al., 2013), so it is necessary to develop energy storage technology to realize the scale of grid-connected storage of electrical energy and guarantee the continuous and stable electricity supply to users (Dunn et al., 2011). Electrochemical energy storage technologies have received much attention due to their high energy efficiency and high power density (Dunn et al., 2011; Yang et al., 2011). However, few, if any, electrochemical grid-scale energy storage technologies, when implemented at system-level, are currently “low cost” and “long lifetime”, especially when compared to standard natural gas peaker plants. Electrochemical energy storage technologies certainly have the potential to become “low cost” and “long lifetime” in future. More research is needed to achieve this goal and make it economically attractive compared to fossil fuels (Larcher and Tarascon, 2015).
As a mainstream electrochemical energy storage technology, lithium-ion batteries are widely used in our life by virtue of their high energy density and long cycle life. Additionally, the manufacturing scale of lithium-ion batteries continues to expand, which will inevitably cause huge consumption of lithium resources and soaring prices (Li et al., 2017). The element lithium is not abundant and unevenly distributed in the earth’s crust (Pan et al., 2013), and in China, it is 70% of the lithium used depends on imports (RONG et al., 2020). In order to avoid the problem of “neck” due to the shortage of resources, it is necessary to develop an energy storage technology that is comparable to lithium-ion batteries. In the periodic table, sodium and lithium are metal elements in the same group and posess similar physical and chemical properties. The earth is rich in sodium, with an elemental content of about 23,000 ppm (lithium content is only about 17 ppm), making it sixth place in terms of abundance. Sodium is distributed all over the world, completely free from resource and geographical constraints. Therefore, sodium-ion batteries have a greater promise than lithium-ion batteries. The research of sodium ion battery can mitigate resource problem of new energy battery development caused by the shortage of lithium resources. Sodium ion batteries (SIBs) include sodium-sulfur batteries, sodium-salt batteries (ZEBRA batteries), sodium-air batteries, organic-based sodium-ion batteries and aqueous-based sodium-ion batteries. Among them, sodium-sulfur batteries are based on the electrochemical reaction of sodium and sulfur to generate sodium polysulfide, and are characterized by high power and energy density, temperature stability, and low cost because of the abundant cost of its raw materials, and have already achieved large-scale production (Wen et al., 2008). ZEBRA batteries use common salt and nickel as the raw materials for the electrodes, and are combined with ceramic electrolyte and molten salt. This combination provides battery systems with high specific energy and power. ZEBRA battery technology has been industrialized for all types of electric cars and hybrid electric buses (Dustmann, 2004). Sodium-air batteries, organic sodium-ion batteries and aqueous sodium-ion batteries are still in the research phase. Sodium ion battery also has the advantages of low cost, excellent fast charging and low temperature performance, good safety performance, etc. The manufacturing of sodium ion battery can follow the production process and equipment of existing lithium ion battery, which is considered as one of the transformative technologies in the field of large-scale energy storage, and its industrialization prospect is quite optimistic and has important economic and strategic significance (Fang et al., 2018; Lu et al., 2018; ZHOU et al., 2020). Therefore, sodium ion batteries are called the “rising star” of the energy storage field.
Sodium ion battery is mainly composed of three parts: cathode, anode and electrolyte. The working principle is similar to that of lithium-ion battery. During the charging process, the cathode material loses electrons in the oxidation reaction and electrons move from the external circuit to the anode, while sodium ions (Na+) are removed from the cathode and enter the electrolyte, then migrate through the electrolyte to the vicinity of the anode and finally embedded in the anode material; during the discharge process, the anode electrode material loses electrons in the oxidation reaction and electrons move through the external circuit to the cathode, while Na+ is removed from the anode material and embedded in the anode material. In the process of discharge, the anode material loses electrons through oxidation and electrons move to the cathode through the external circuit (Pan et al., 2013). As one of the main components of sodium ion battery, electrolyte has an important role in conducting ions and participating in the redox reaction of cathode and anode (ZHU et al., 2016). Electrolyte is the “bridge” connecting cathode and anode. The performance of the electrolyte directly affects the performance of sodium ion batteries. During the charging and discharging process, the electrolyte itself decomposes or reacts with the electrode material to form an interface. The interfacial film on the anode is called the solid electrolyte interphase (SEI), and the interfacial film on the cathode is called the cathodic electrolyte interphase (CEI). CEI and SEI largely determine the electrochemical performance of the battery system (Lin et al., 2019). At present, sodium ion battery electrolyte system mainly includes aqueous electrolyte and non-aqueous electrolyte. Non-aqueous electrolyte contains organic liquid electrolyte and solid electrolyte. The recyclability of aqueous electrolyte is excellent but its electrochemical window is narrow and the overall energy density is low (LIU et al., 2018b). Solid electrolytes generally have higher impedance and polarization, leading to a decrease in battery capacity. Moreover, the solid electrolyte itself has a narrow electrochemical window, and the mismatch with high-voltage electrodes will cause side reactions, leading to the deterioration of battery cycling performance (Hu et al., 2020; Gao et al., 2021). In a comprehensive comparison, organic liquid electrolytes have good properties, such as electrochemical stability within a certain electrochemical window, sufficiently high ionic conductivity, and good compatibility with various electrode materials. Therefore, organic liquid electrolytes are the most promising electrolytes for sodium ion batteries in practical applications.
This paper summarizes the research progress of organic liquid electrolytes for sodium ion batteries by discussing the basic requirements and composition of organic electrolytes for sodium ion batteries, the current research status of organic liquid electrolytes, the composition and requirements of the interface between electrolytes and electrodes and the regulation strategies. Finally, the performance of organic electrolyte and the nature of interfacial film are synthesized, and some suggestions on the future development trend of organic electrolyte for sodium ion batteries are proposed, in order to provide some help to the research of sodium ion battery electrolyte and sodium ion battery energy storage science and technology.
As a bridge connecting cathode and anode, the electrolyte assumes the role of transporting ions between cathode and anode and is an important part of the battery, whichplays a vital role in the performance of the battery in terms of multiplicity, cycle life, safety and self-discharge. Organic liquid electrolyte is also customarily called organic electrolyte. Electrolyte is mainly composed of solvent, solute and additives (Figure 1), which together determine the properties of electrolyte.
FIGURE 1. Main components of sodium ion battery electrolyte (Hu et al., 2020).
Solvent is one of the important components of organic liquid electrolytes. Electrolyte solvents need to satisfy the most basic conditions such as stability, non-toxicity and cheapness. Besides, the electrolyte solvent should have a wide electrochemical stability window, sufficient sodium salt solubility, high dielectric constant, low viscosity and wide liquid range (i.e., low melting point and high boiling point). The solvent should also maintain electrochemical stability or promote the formation of a high-quality passivation layer during cell operation. However, these different and sometimes conflicting requirements for the same solvent are difficult to be met by a single solvent, and therefore multiple solvents are often used in combination. The main solvents currently used in sodium ion batteries are ester solvents and ether solvents. These two types of organic solvents have provided excellent performance in battery applications (Fenton, 1973; Armand et al., 2009; Monti et al., 2014; Hong et al., 2015; Ponrouch et al., 2015; Niu et al., 2019; Wang et al., 2020).
Ester solvents are a more commonly used class of solvents, especially cyclic [propylene carbonate (PC) and ethylene carbonate (EC)] and chain [dimethyl carbonate (DMC), diethyl carbonate (DEC) and methyl ethyl carbonate (EMC)] carbonates are most commonly used, and electrolytes of carbonate solvents tend to have the advantages of high ionic conductivity and good oxidation resistance (Ponrouch et al., 2012). The relevant physical and chemical properties of carbonate solvents are shown in Table 1. The dielectric constant of ether solvents is much lower than that of cyclic carbonates, but higher than that of chain carbonates. The resistance to oxidation is relatively poor, and they tend to decompose at high voltages. However, ether solvents generate thinner SEI on anode with higher initial Coulomb efficiency, and ether solvents are more compatible with anode such as metallic sodium and can co-embed graphite with sodium ions and show good reversibility, making graphite that cannot be embedded with sodium in ester solvents can be used as anode in this solvent system (Dubois et al., 1997). In the actual application process, the use of two or even a variety of solvent mix is a more common method (Komaba et al., 2011b), the ratio of different solvents is controlled, and the advantages of multiple solvents are integrated to maximize the performance of electrolytes.
TABLE 1. Physical and chemical properties of some commonly used organic solvents (Xu, 2004; Kamath et al., 2014; Vignarooban et al., 2016; Chen et al., 2019; Wang M. et al., 2022).
Sodium salts are another important component of organic liquid electrolytes and play a vital role in the performance of electrolytes (Che et al., 2017). For the selection of sodium salt. Firstly, the sodium salt should have sufficient solubility and dissociation ability in the solvent, and the dissociated cations should be free to move without obstacles to provide sufficient charge carriers. Secondly, the sodium salt should remain electrochemically stable within a certain electrochemical window without oxidation or reduction. The sodium salt and the solvent together determine the redox potential of the electrolyte, and the salt anion and the solvent are coupled by electrostatic interaction, which affects the oxidative stability of the electrolyte. In addition, the sodium salt should have good chemical stability as well as safety, remaining chemically inert to the diaphragm, solvent, electrode and collector fluid. If the sodium salt can effectively promote the formation of SEI film at the interface between electrode and electrolyte, it can better enhance the electrochemical performance of electrolyte, such as cycling stability (Strauss, 1993; Borodin and Jow, 2010; Ponrouch et al., 2015; Eshetu et al., 2019; Fadel et al., 2019). The commonly used sodium salts are sodium perchlorate (NaClO4), sodium tetrafluoroborate (NaBF4), sodium hexafluoroborate (NaPF6), sodium trifluoromethanesulfonate (NaCF3SO3, abbreviated as NaOTf), sodium bis(fluorosulfonyl)imide [Na(FSO2)2N, abbreviated as NaFSI] and sodium bis(trifluoromethanesulfonyl)imide [Na(CF3SO2)2N, abbreviated as NaTFSI] (Hu et al., 2020). The advantages and disadvantages of these sodium salts are shown in Table 2.
TABLE 2. Physical and chemical properties, advantages and disadvantages of commonly used sodium salts (Devlin and Herley, 1987; Ponrouch et al., 2012; Bhide et al., 2014; Evans et al., 2014; Ponrouch et al., 2015; Eshetu et al., 2016; Eshetu et al., 2019; Forsyth et al., 2019; Goktas et al., 2019; Minh Phuong et al., 2019; Hu et al., 2020; Wang et al., 2020).
Based on the fact that each of the commonly used sodium salts has its own advantages and disadvantages, which are difficult to overcome. Hybrid systems combining two or more sodium salts have also been investigated, and the aim of avoiding disadvantages is expected to be realized, but the results are not significant. Therefore, it is necessary to develop new sodium salts (Hu et al., 2020). Some new salts that have been synthesized and reported are sodium difluorooxalate borate (NaODFB), sodium 4,5-dicyano-2-(trifluoromethyl)imidazolate (NaTDI), sodium 4,5-dicyano-2-(pentafluoroethyl)imidazolate (NaPDI), sodium bisoxalate borate (NaBOB), sodium bis [salicylato (2-)]-borate (NaBSB), sodium salicylic benzylic acid borate (NaBDSB), sodium tetraphenyl borate (NaBPh4), etc.
Additives are the third main component of organic liquid electrolytes. Additives are components that are present in small amounts (less than 5%) in the electrolyte and are characterized by high specificity and small dosage. By adding a small amount of additives, it is possible to make up for the deficiencies of the original electrolyte and significantly optimize the specific performance of the battery without increasing the production cost or changing the production process (Xu, 2014; Wang et al., 2020). According to the function of additives, they are divided into film-forming additives, flame retardant additives, overcharge protection additives and other types of additives (Zhang, 2006).
The most studied are film-forming additives, which are usually easily consumed. During the initial activation cycle they participate and contribute to the formation of the interface between the electrode and the electrolyte, leaving a chemical signal only at the interface and not in the electrolyte itself. The ideal film-forming additives should have higher Fermi energy (Eg) located in the highest occupied molecular orbital-lowest unoccupied molecular orbital gap than solvents, electrolyte salts, etc., so that they preferentially undergo oxidation or reduction, which in turn improves the film-forming quality and efficiency of the SEI film and effectively enhances the electrochemical performance of the cell (Goodenough and Kim, 2010; Zhu et al., 2017; Niu et al., 2019). The mechanism of action of various additives is shown in Table 3. Other types of additives including acidity enhancers, impurity scavengers, viscosity reducers, free radical scavengers, etc., Also have potential applications in sodium ion batteries (Ponrouch et al., 2015).
Sodium ion battery organic liquid electrolytes are classified according to sodium salts and are mainly divided into sodium perchlorate (NaClO4)-based organic liquid electrolytes, sodium hexafluorophosphate (NaPF6)-based organic liquid electrolytes, sodium bis(fluorosulfonyl)imide (NaFSI)-based organic liquid electrolytes, sodium bis(trifluoromethylsulfonyl)imide (NaTFSI)-based organic liquid electrolytes, and sodium difluoroxalate borate (NaODFB)-based organic liquid electrolytes. Figure 2 shows the keyword clustering analysis of the literature related to organic liquid electrolytes for sodium ion batteries over the years. The overview of research in this field was presented, including electrodes, electrolytes, solid electrolyte interfacial films, and electrochemical properties. In addition to the traditional electrochemical testing of different electrode materials with matching electrolytes, the study of solid electrolyte interfacial membranes and the development of new organic liquid electrolytes are gradually attracting the attention of researchers in recent years. The following section will focus on the compatibility of different types of sodium ion battery electrolytes with each electrode.
FIGURE 2. Sodium batteries organic liquid electrolyte keywords co-occurrence analysis: (A) network visualization, (B) overlay visualization and (C) density visualization, produced by vosviewer software.
NaClO4-based organic liquid electrolyte is a widely used electrolyte for sodium ion batteries with good compatibility with common cathode materials (layered oxides, polyanionic compounds, and Prussian blue-like compounds). The battery system consisting of layered oxide and sodium perchlorate-based organic liquid electrolytes exhibited suitable reversible capacity, rate capability, and cycle life. The binary layered oxides P2-NaxCo0.7Mn0.3O2 (x ≈ 1) perform well in the electrolyte of NaClO4 dissolved in PC with FEC, which is easier to coordinate with the ClO4− on the cathode surface than the PC solvent and contributes to the formation of the NaF protective layer on the cathode surface (Cheng et al., 2019). Ternary layered oxides Na0.67Ni0.15Fe0.2Mn0.65O2 (N-NFM) CEI membranes formed in NaClO4 based electrolytes in EC/DEC contain more organic compounds but less inorganic compounds, leading to increased impedance. In addition, CEI membranes are sensitive to perchlorate, which has strong oxidizing properties. A small fraction of the CEI film peels off from the cathode surface, accelerating the dissolution of transition metal (TM) ions and leading to reactivation of electrolyte decomposition (Wang S. et al., 2021). The mechanism of action and subsequent solubilization of TM ions by CEI films is shown in Figure 3A. Therefore, further optimization of electrolyte replenishment is required. Polyanionic compounds have a very solid framework matched to NaClO4 electrolytes, allowing for higher cyclability and safety, and have been extensively studied by researchers. For example, Na3V2(PO4)3(NVP) showed high discharge capacity and coulomb efficiency with good cycling and rate capability, cycle life in 0.9 M NaClO4 dissolved in triethyl phosphate (TEP) electrolyte solution (Liu et al., 2019), and 1 M NaClO4 in PC added with 5 wt% [C3mpyr] [NTf2] IL (Manohar et al., 2018a). TEP electrolyte has non-flammable and high safety features. The IL additive makes the passivation layer organic and IL-containing and with sulfur in the surface film, and the surface film is more stable (Figures 3B,C). Na2VM(PO4)3 (M = Ga or Al) behaves differently in an electrolyte of 1 M NaClO4 with EC/PC = 1:1 v/v, FEC of 5 vol%, which is attributed to the fact that Na3VAl(PO4)3 possesses a larger diffusion bottleneck to transfer more electrons than Na3VGa(PO4)3 and exhibits a higher redox reaction potential (Wang Q. et al., 2021). Therefore, the V5+/V4+ redox reaction can be induced by the substitution of smaller-sized low-valent (≤+3) cations, which improves the utilization efficiency of the V5+/V4+ redox reaction. The Na4Fe3(PO4)2(P2O7) cathode formed a protective surface film in the EC/PC/DEC (5/3/2, v/v/v) electrolyte with 0.5 M NaClO4 added with FEC and prevented undesirable decomposition of the linear carbonate, leading to excellent cycling performance of the cathode (Figures 3D–F) (Lee et al., 2016). The discharge capacity, impedance, multiplicity performance, cycling stability, and capacity retention of Prussian blue-like cathode materials sodium hexacyanoferric (Fe-HCF) composites coated with polypyrrole (PPy) were greatly improved in the EC/PC electrolyte of NaClO4 (Figures 3G–I) (Tang et al., 2016). The insertion of Na+ in a series of Prussian blue compounds in organic carbonate electrolytes of NaClO4 was investigated. KFe [Fe(CN)6] provides the highest reversible capacity at ∼3.6 V for both the high-spin and low-spin Fe3+/Fe2+ couples (Lu et al., 2012; Wang Q. et al., 2022).
FIGURE 3. Compatibility of sodium NaClO4-based electrolytes with different cathode materials: (A) Schematic evolution of CEI film of Na0.67Ni0.15Fe0.2Mn0.65O2 (N-NFM) cathode in NaClO4-based electrolytes (Wang S. et al., 2021). (B) HR-TEM images of NVP/C electrodes after 50 cycles with organic electrolyte and organic + IL electrolyte respectively and (C) XPS C1s Peaks for NVP/C cathodes after 50cycles with Organic and Organic + IL electrolytes (Manohar et al., 2018a). (D) Diagram of the interaction, (E) cycling performance and (F) Coulombic efficiency of Na4Fe3(PO4)2(P2O7) electrode in NaClO4-based electrolyte with FEC (Lee et al., 2016). (G) Discharge capacities, (H) long-term cycling stability and (I) EIS spectra of Fe-HCF and Fe-HCF@PPy electrodes in NaClO4-EC/PC electrolyte (Tang et al., 2016).
There have also been a number of studies on the compatibility of sodium perchlorate-based organic liquid electrolytes with anode. The most commonly used anode materials are intercalation type materials (e.g., hard carbon). Hard carbon as the anode of sodium ion batteries showed good compatibility with NaClO4 electrolytes with solvent of EC, PC, DMC and DEC exhibiting high specific capacity and good capacity retention. The electrochemical properties of hard carbon in electrolyte are further enhanced by the action of some additives (e.g., 1-ethyl-3-methylimidazolium bis(fluoromethanesulfonyl)imide (EMImFSI) (Benchakar et al., 2020), N,N-diethyl-N-methoxyethylammonium bis(trifluoromethanesulfonyl)imide (DEMETFSI) (Egashira et al., 2012), FEC (Liu X. et al., 2018; Pan et al., 2018; Rangom et al., 2019)). For example, The reversibility of sodium insertion became evident at a volume content of 70% of DEMETFSI (Egashira et al., 2012). FEC promotes the formation of the initial sodiuming process SEI and improves the cycle life. By using added FEC and high salt-to-solvent molar ratio TMP electrolytes, it is possible to achieve both low RSEI and very small Rct on HC electrodes, thereby simultaneously inhibiting TMP decomposition and building thin and dense SEI membranes (Figure 4A). The electrolyte with a 5 vol% FEC ratio of 1:3 NaClO4/trimethyl phosphate (TMP) exhibited considerable reversible capacity (238 mAh g-1 at 20 mA g-1), long-term cycle life up to 1,500 cycles, 84% capacity retention at 200 mA g-1, and high rate capability (Liu X. et al., 2018). Graphite-based intercalated anode perform well in electrolytes with solvent combinations of EC/DEC (Luo et al., 2015), EC/DMC (Wang S.-W. et al., 2017), and PC (Wang et al., 2013). In addition to carbon materials, other inserted anodes have been investigated, such as TiO2, Na2Ti3O7. TiO2 in 1M NaClO4 in EC/PC electrolyte exhibits the best high-magnification performance of all titanium-based sodium ion anode materials reported so far (Wu et al., 2014). The SEI film generated in Na2Ti3O7@C anode material with sodium perchlorate (NaClO4)-based EC/DEC electrolyte contains more Na2CO3 and NaF, which is caused by the continuous decomposition of NaClO4 salt in carbonate solvent. This makes the SEI film thicker and the interfacial impedance greater, leading to a decrease in cell cycling performance, this is in contrast to the NaOTf electrolyte (Figure 4B) (Wen et al., 2023). Metal oxides/sulfides/phosphides are typical conversion electrodes in SIBs, which typically suffer from poor conversion reaction reversibility and shuttle effects. Similar to other electrode materials, stable cycling of conversion electrodes relies on dense SEIs to provide stability and suppress losses of high mechanical strength actives. Hollow γ-Fe2O3 nanoparticles in PC electrolytes of NaClO4 showed superior performance in terms of capacity retention, Coulomb efficiency, multiplicative performance, and cycle life (Figure 4C) (Koo et al., 2013).
FIGURE 4. Compatibility of sodium NaClO4-based electrolytes with different anode materials: (A) Schematic illustration of the behavior of the HC electrode in the different ratios NaClO4/TMP electrolytes (Liu X. et al., 2018). (B) Schematic evolution of SEI film of Na2Ti3O7@C with different electrolytes (Wen et al., 2023). (C) Capacity retention of hollow NP-based γ-Fe2O3 electrodes at different rates (Koo et al., 2013).
In general, NaClO4-based organic liquid electrolyte has been widely used as a relatively mature electrolyte for sodium ion batteries. NaClO4 electrolyte has contributed to the improvement of energy density of sodium ion batteries with its own stability and oxidation resistance along with some high-voltage cathode materials. However, the compatibility with some positive and negative electrodes is not very good, and it will promote the dissolution of transition metal ions as well as the generation of thick SEI films, which may require a combination of additives and different solvent combinations to optimize the electrolyte in the future.
NaPF6-based organic liquid electrolyte is also one of the commonly used electrolytes for sodium ion batteries. Studies on related cathode materials are often paired with NaPF6-based organic liquid electrolytes for a series of electrochemical tests. First is the layered oxide cathode material. The layered oxide exhibits excellent retention and outstanding multiplicative performance, specific capacity, capacity retention and long-term cycling stability in NaPF6-based electrolytes. Among them, The CEI film formed by Na0.67Ni0.15Fe0.2Mn0.65O2 (N-NFM) in NaPF6-based electrolyte is dense and homogeneous, which effectively inhibits the dissolution of transition metal ions and provides a low-energy barrier for Na+ transport (Wang S. et al., 2021). The NaNi1/3Fe1/3Mn1/3O2 cathode material, paired with a hard carbon anode, maintains up to 92.2% capacity after 1,000 cycles at 1C between 2.0 V and 3.8 V using an optimized electrolyte of 1 M NaPF6 dissolved in 1:1 (v/v) PC-EMC +2 wt% FEC, 1 wt% PST, and 1 wt% DTD. The PST and DTD additives promote the formation of a robust SEI on the anode and prevent the dissolution of transition metal ions by inducing the formation of a dense and dense electrolyte (Figure 5A) (Che et al., 2018). The second type of cathode material that is often adapted to NaPF6 electrolytes is the polyanionic compound cathode material. Polyanionic compounds matching NaPF6 ester and ether electrolytes have been reported. Na3(VOPO4)2F (NVOPF)/rGO 3D sub-microspheres and Na2Ti2O5 nanosheet anode electrode and NaPF6 diglyme electrolyte, the full cell was further designed with high initial Coulombic efficiency (90%), excellent multiplicative performance (40°C) and ultra-stable cycling performance (>4,000 cycles without degradation) (Figures 5C,D). The electrolyte defines a robust fluorine-rich inorganic-organic interface, which effectively improves the interface and promotes ultra-fast charge transfer (Figure 5B) (Ba et al., 2022). The SEI layer between Na3V2(PO4)3 and NaPF6/1-butyl-3-methylimidazolium bis (trifluoromethanesulfonyl) imide (BMITFSI) ionic liquid electrolyte consists of NaOH, Na2SO4, Na2S2O7 and NaF (Figure 5E). This is the reason for its good electrochemical properties (Wu et al., 2018). Half-cell tests of Na4Co3(PO4)2P2O7 in an electrolyte solution of EC/DEC with 1 M NaPF6 showed the formation of a double layer in the fully Na+ extracted state of charge, with semi-organic-rich material found in the subsurface region near the electrode and more organic material in the outermost surface region near the electrolyte. At the same time, an additional outermost inorganic cover layer consisting of sodium carbonate and sodium fluorophosphate was formed after complete Na+ insertion (Figure 5F). Therefore, the Na4Co3(PO4)2P2O7 cathode provided excellent cycling performance (Zarrabeitia et al., 2021). The third cathode material used to match the NaPF6-based electrolyte is a Prussian blue-like compound. The Prussian blue cathode electrode exhibits enhanced capacity retention in a volume ratio of 7: 3 of di-(2,2,2 trifluoroethyl) carbonate (TFEC)/fluoroethylene carbonate (FEC) consisting of 0.9 mol L-1 NaPF6. The electrolyte has excellent flame retardancy and good compatibility with sodium electrodes. The polycarbonate formed on the cathode surface plays an important role in the studied electrolyte system by enhancing the ionic conductivity and reducing the impedance of the solid electrolyte interphase (SEI) layer (Zeng et al., 2020).
FIGURE 5. Compatibility of sodium NaPF6-based electrolytes with different cathode materials: (A) Schematic summary on the role of PST and DTD additives in NFM/HC full cell (Che et al., 2018). (B) Illustration of effects of electrolyte system, (C) rate performance and (D) cycling performance on NVOPF@rGO//Na2Ti2O5 full cell (Ba et al., 2022). (E) The diagram of SEI layer formation mechanism on the Na3V2(PO4)3 cathode in NaPF6/BMITFSI IL electrolyte (Wu et al., 2018). (F) Schematic of the CEI on the Na4Co3(PO4)2P2O7 electrode after 1 st Na+ extraction and insertion (Zarrabeitia et al., 2021).
Researchers often choose NaPF6-based organic liquid electrolytes for the study of anode materials (intercalation-type, conversion-type materials, and non-metallic materials). Hard carbon HC performs well in NaPF6 electrolytes with ester and ether solvents. Among them, The TEGDME-based NaPF6 electrolyte can exhibit excellent rate capability, capacity retention and cyclic Coulombic efficiency at the HC anode. This is because the stable layer-by-layer SEI in the TEGDME-based electrolyte combined with the solvent layer “pseudo-SEI” on the HC facilitates high-performance Na+ ion storage in the HC and extends the cycle life of the HC anode material (Figure 6A) (Ma et al., 2021). In the EC/DMC electrolyte, the use of 3% FEC significantly increased the total capacity and capacity retention, while the use of DMCF additive had a negative impact on capacity but provided better cycling performance than the additive-free electrolyte (Figure 6B) (Fondard et al., 2020). The good performance can be attributed to the SEI composition consisting mainly of sodium ethylene dicarbonate NaO2CO-C2H4-OCO2Na (NEDC) and NaF. FEC as an additive promotes the production of NaF, which enhances the NEDC-rich SEI and results in a significant increase in capacity retention during cycling. Graphite-based materials have good reversible capacity and good cycling performance in NaPF6/diethylene glycol dimethyl ether (DEGDME) electrolytes (Han et al., 2015; Cabello et al., 2017; Nacimiento et al., 2019; Li Z. et al., 2021; Zheng et al., 2022). Transformation-based electrode materials (e.g., copper phosphorothioate (Cu3PS4) (Brehm et al., 2020), dandelion-shaped manganese sulfide (DS-MnS) (Duong Tung et al., 2018), tin phosphide (Sn4P3/C) electrodes (Luis Gomez-Camer et al., 2019), TiS2 (Tao et al., 2018), etc.) are also often studied matching NaPF6-based organic liquid electrolytes. The choice of solvent is often ether-based solvents (diethylene glycol dimethyl ether, DME), and to a lesser extent, esters. In these electrolytes the converted electrode materials exhibit high capacity, cycling performance, multiplicative performance, first turn Coulomb efficiency, etc. It indicates that the conversion-type electrodes have high compatibility with ether-based NaPF6 electrolytes. Non-metallic elemental anode materials [Micron Pb particles (Darwiche et al., 2016), and Bi electrodes (Wang C. et al., 2017; Li Y. et al., 2021)] exhibit good electrochemical performance in NaPF6-based diethylene glycol dimethyl ether electrolytes and good compatibility with NVP and NVPF cathode materials, and the full-cell test solution exhibited good performance. For the study of sodium metal electrodes in NaPF6-based electrolytes, EC/DMC is often chosen as the solvent. When FEC is added, the Na metal electrode forms a multilayer SEI structure, including an external NaF-rich amorphous phase and an internal Na3PO4 phase. This layered structure stabilizes the SEI and prevents further reactions between the electrolyte and the Na metal. Without FEC, the carbonate-based electrolyte containing NaPF6 reacts with the metal electrode to produce an unstable SEI, rich in Na2CO3 and Na3PO4, which continuously depletes the cell’s sodium reserves during cycling (Figures 6C,D) (Han et al., 2021). The Na metal deposition/dissolution efficiency increased with increasing NaDFP concentration when sodium difluorophosphate NaDFP additive was added. NaDFP suppressed the overpotential and interfacial resistance. A high multiplicative capacity and long cycle life of 76.3% capacity retention after 500 cycles were achieved with 1 wt% NaDFP. The NaDFP-containing electrolyte formed a more stable SEI layer than the pure electrolyte, thus mitigating further degradation of the electrolyte (Yang et al., 2021). In specific glyme (chain ether) electrolytes, the sodium-metal interface produces a thin, homogeneous inorganic SEI composed primarily of Na2O and NaF that may not support extensive or extreme cycling conditions, but the addition of FEC provides a more robust SEI to facilitate a large number of consistent sodium plating and stripping cycles (Seh et al., 2015; Sarkar et al., 2023).
FIGURE 6. Compatibility of sodium NaPF6-based electrolytes with different anode materials: (A) Schematic illustration of SEI and pseudo-SEI structures and chemistry for Na+ storage in HC anodes in NaPF6 based ester and ether electrolytes (Ma et al., 2021). (B) Cycle capacity for HC electrodes using 1 M NaPF6 in EC:DMC with addition of 1.5% and 3% of FEC or DMCF (Fondard et al., 2020). (C, D) Representation of free FEC and FEC electrolyte additives in tuning the microstructure of SEI on Na metals during cycling, respectively (Han et al., 2021).
In general, NaPF6-based organic electrolytes, as a commercially available electrolyte, have good compatibility with common cathode and anode materials. NaPF6 shows superior performance in ether solvents compared to other sodium salts. However, the study of NaPF6-based electrolyte body solutions (e.g., solvation structure, sodium ion transport kinetics) is still at the beginning stage and will be further enhanced to reveal the reasons for their superior electrochemical performance in the future.
The cathode materials matched to NaFSI electrolytes mainly include layered oxides, polyanionic compounds, and organics. For the compatibility study of layered oxide cathode materials with NaFSI-based organic liquid electrolytes, the solvents used with NaFSI are esters, ethers, ionic liquids. Among them, NaNi0.68Mn0.22Co0.10O2 (NaNMC) exhibited good long-cycle performance and capacity retention in the phosphate electrolyte NaFSI-TEP (Figure 7A). The stable cycling can be attributed to the formation of a stable CEI layer on the NaNMC cathode, which suppresses the surface reconstruction of the cathode, the dissolution of transition metals at the cathode, and the persistent side reactions at the electrolyte/electrode interface (Jin et al., 2022). NaFe0.4Ni0.3Ti0.3O2 matches well in electrolytes using IL solvents (e.g., C3C1pyrrFSI). This ionic liquid electrolyte enhances the formation of passivation layer on the surface of Al current collectors, stabilizes the surface to 5 V, and prevents Al corrosion even at 55°C (Otaegui et al., 2015). For the compatibility study of polyanionic compounds with NaFSI electrolytes, NVP in NaFSI electrolytes with ester solvents and ionic liquids has high Coulombic efficiency, fast charging capability, stable cycling, high reversible capacity and capacity retention (Jian et al., 2013; Manohar et al., 2018b; Zheng et al., 2018; Li et al., 2022). Among them, the ionic liquid can promote the formation of a stable and thin SEI layer on the surface, which improves the discharge capacity and cycling performance of NVP@C cathode materials (Figures 7C,D) (Manohar et al., 2018b).
FIGURE 7. Compatibility of sodium NaFSI-based electrolytes with different cathode materials: (A) Long-cycling performance of hard-carbon||NaNMC full cells in two electrolytes (Jin et al., 2022). (B) Organic and hybrid electrolyte compositions, (C) cycling performance and Coulombic efficiency of NVP@C with organic and hybrid electrolytes at 0.5C and (D) HR-TEM images of NVP@C electrodes after cycling with the organic and hybrid-2 electrolyte, respectively (Manohar et al., 2018b).
The anode materials matched to NaFSI electrolyte mainly include intercalation type materials, alloy type materials, conversion type materials and Na metal electrodes. The NaFSI electrolytes suitable for the study of intercalation-type materials include ester electrolytes, ether electrolytes, and ionic liquid electrolytes. Among them, in 3 mol dm-3 NaFSI/PC + EC electrolyte, an organic-inorganic equilibrium SEI (mainly composed of (CH2)n and NaF) was formed on the surface of the hard carbon electrode. This SEI not only enables easy charge transfer and fast Na+ transport, but also exhibits strong passivation ability and excellent durability (Patra et al., 2019). CMK half-cells exhibit extraordinary cycling stability and high reversible capacity in a 3.8 M NaFSI IL electrolyte in C3mpyrFSI (Figure 8A). This is due to the contribution of anionic decomposition species in ILs leading to inorganic SEI on mesoporous carbon CMK electrodes with high ionic conductivity, which promotes Na+ desolvation and diffusion kinetics. This rapid Na + migration facilitates improved reaction rates and cycling stability (Sun et al., 2021). Graphite materials do not perform well in the ether electrolyte of NaFSI, where side reactions occur between the electrolyte and the graphite electrode, and the formation of SEI films, which consist mainly of salt decomposition products and hydrocarbons, as shown in Figure 8B, leading to a low Coulomb efficiency of the studied cell system (Maibach et al., 2017; Goktas et al., 2019). For the study of Na metal electrodes in NaFSI-based organic electrolytes, electrolytes that have been reported are high concentration electrolytes with NaFSI and ester and ether electrolytes with additives. Among them, The Na|| Na3V2(PO4)3 cell is stable for nearly 1,400 cycles at 2 C in a highly concentrated electrolyte of DME with the addition of a small amount of SbF3 at 4 mol L-1 NaFSI and also exhibits excellent multiplicative performance of 80 mAh g-1 at 40°C. This is because the SbF3 additive forms a hard Na-Sb alloy layer, while the high concentration contributes to the formation of a dense NaF-rich SEI layer on the Na metal surface. This bilayer structure of the SEI layer effectively prevents dendrite growth and provides fast interfacial ion transport (Fang et al., 2020). The addition of NaFSI to methyl propylpyrrole dicyandiamide ([C3mpyr]DCA) ionic liquid produces a more stable SEI layer (Figure 8C) (Forsyth et al., 2019). The addition of 1,1,2,2-tetrafluoroethyl-2,2,3,3-tetrafluoropropylether (TTE) to 3.8 M NaFSI/DME electrolyte forms a localized high concentration electrolyte (LHCE), which helps to construct a stable SEI for SMBs. TTE also decomposes on Na metal anodes, synergistically forming dense SEI with low surface resistance and good mechanical properties, rich in NaF, which facilitates the transport of Na+ ions and inhibits the growth of Na dendrites (Figure 8D) (Wang Y. et al., 2021). Transformation-based anode materials (e.g., Cu1.8S/C (Li H. et al., 2021), SnP nanocrystals (NCs) (Liu J. et al., 2018), tin phosphide (Sn4P3) (Mogensen et al., 2017; Mogensen et al., 2018)) in FSI-based organic electrolytes exhibited stable cycling ability and high capacity. Both NaFSI and FEC additives contribute to the formation of a stable NaF-rich SEI on the anode surface.
FIGURE 8. Compatibility of sodium NaFSI-based electrolytes with different anode materials: (A) cycling stability tests of Na/CMK cells in carbonate and ionic liquid electrolytes (Sun et al., 2021). (B) Schematic representation of the surface processes occurring at this particular graphite/TEG-DME electrolyte interface (Maibach et al., 2017). (C) Schematic representation of the sodium-solvated and interfacial structures of NaFSI and NaDCA on sodium-metal surfaces in the [C3mpyr]DCA IL systems (Forsyth et al., 2019). (D) Cycling performance Na/NVP in NaFSI/DME electrolyte with TTE using STD, HCE, and LHCE, and schematic of Na+ solventization and formation of SEI on sodium metal surface (Wang Y. et al., 2021).
In general, NaFSI electrolytes dissolved in carbonate ester and ionic liquids exhibit better electrochemical performance and have better compatibility for hard carbon electrodes and sodium metal electrodes compared to other sodium salt electrolytes. In the future, combining different types of cathode and anode electrodes to assemble a complete battery for electrochemical testing, as well as contributing to the design and implementation of flame-retardant batteries, may be the trend for this electrolyte.
The cathode materials adapted to NaTFSI-based organic liquid electrolytes for electrochemical testing mainly include layered oxides and polyanionic compounds. Layered oxides (P2-type Na2/3Ni1/3Mn2/3O2 (Risthaus et al., 2018), Na0.45Ni0.22Co0.11Mn0.66O2 (Chagas et al., 2014), and Na0.44MnO2 (Stigliano et al., 2022)) exhibited high discharge capacity and capacity retention in the ionic liquid electrolyte with NaTFSI. For the polyanionic compound, NaFePO4/Na half-cells in sodium bis(trifluoromethanesulfonyl)imide (NaTFSI)-bonded butylmethylpyrrolidine (BMP)-TFSI ionic liquid (IL) electrolyte operate at 3 V. This IL electrolyte shows high thermal stability and non-flammability. NaFePO4 has the best capacity at 50 °C in 0.5 M NaTFSI mixed IL electrolyte (Wongittharom et al., 2014).
The most commonly used anode material for NaTFSI organic electrolyte is hard carbon. NaTFSI organic electrolytes used for hard carbon electrodes include ester electrolytes and ionic liquid electrolytes. Among them, hard carbon electrodes in 2 M NaTFSI/EC:DMC electrolyte provided the best initial reversible capacity, high electrochemical stability, and good cycling stability. In addition, a sharp capacity decay was observed after cycling in an ultra-high concentration electrolyte (5 M NaTFSI/EC: DMC) (Figures 9A,B) (Chen et al., 2020). In NaTFSI/EC: DMC electrolyte, the addition of FEC or DMCF was found to be beneficial for overall capacity and capacity retention during cycling (Figure 9C) (Fondard et al., 2020).
FIGURE 9. Compatibility of sodium NaFSI-based electrolytes with HCSs materials: (A) Schematic diagram of the interaction mechanism of HCSs electrodes in ultra-high concentration electrolytes and (B) cycling performance at 0.1C of HCSs in electrolytes with different salt concentrations (Chen et al., 2020). (C) Cycling capacity for HC electrodes using 1 M NaPF6 in EC:DMC with addition of 1.5% and 3% of FEC or DMCF(Fondard et al., 2020).
In addition, some applications of NaTFSI-based electrolytes in full batteries have been reported. For example, the full cell consisting of Na3V2(PO4)2F3 cathode and (Na2+xTi4O9/C) anode exhibited high capacity retention in a nonflammable low eutectic solvent (DES) including sodium bis(trifluoromethane) sulfonate (NaTFSI) dissolved in N-methylacetamide (NMA). The improved electrochemical stability was associated with a stronger surface film formed at the electrode/electrolyte interface (De Sloovere et al., 2022). P2-Na0.6Ni0.22Fe0.11Mn0.66O2 cathode and Nanostructured Sb-C composite anode cells in a 0.2M NaTFSIPyr14TFSI ionic liquid-based electrolyte exhibited high specific capacity for the full cell. The electrolyte has a high ionic conductivity and high thermal stability. The anodic stability of this electrolyte was up to 4.7 V vs. Na+/Na (Hasa et al., 2016).
In general, NaTFSI ionic liquid electrolytes show good electrochemical performance. However, there are some problems, such as corrosion of the aluminum foil, capacity decay and some disadvantages of the ionic liquid electrolyte itself (e.g., high viscosity, poor wettability to the electrode, etc.). Efforts are still needed to improve these shortcomings in the future.
Sodium difluoro (oxalato)borate (NaODFB) is a new chelated sodium salt discovered by researchers in recent years. Only a few articles have been published to study this electrolyte. Chen et al. (Chen et al., 2015) found that Na/Na0.44MnO2 half-cells combined with NaDFOB-based electrolytes exhibited greatly enhanced multiplicative capacity and cycling performance. Sun et al. (Sun et al., 2020) developed a high-capacity nanoconstrained FeF3 SIB-based cathode and found that the best cycling performance was achieved using NaDFOB salts in a ternary electrolyte (EC:DEC:DMC), with much higher cycling performance compared to the conventionally used NaClO4, which was associated with the formation of a thin and conformal CEI protective film on the cathode. They further predicted that the DFOB anion reduction-mediated radical oligomer/polymer pathway may be an important part of the formation of CEI films. As shown in Figure 10A. Wang et al. (2023) investigated the compatibility of NaODFB electrolyte with NVP cathode.1 M NaODFB-DME electrolyte contributed to the formation of thinner CEI film on the surface of NVP material with low content of B2O3, which resulted in high specific capacity and capacity retention of the cell. Zhao et al. (2022) investigated the compatibility of NaODFB ether electrolyte with HC anode at high temperature. The Na/HC half-cell with 1 M NaODFB in DME has a high reversible capacity of 249.9 mAh/g at 100 mA/g and 55°C, exhibiting excellent cycling stability attributed to the SEI membrane groups B-F and B-O containing inorganic substances. Gao et al. (2022) found that there was a dense and smooth SEI film on the surface of sodium sheets after cycling in NaODFB-based carbonate electrolyte, and the SEI film could effectively inhibit the growth of sodium dendrites. They provide insight into the underlying mechanism of the protective effect provided by SEI derived from sodium difluoro (oxalate)borate (NaDFOB) (Figure 10B). The pre-reduction of the DFOB− contributes to the formation of SEI and inhibits the decomposition of the carbonate solvent, and the DFOB− is gradually transformed into a borate- and fluoride-rich SEI with cycling. The protective effect of SEI is optimized at 50 cycles, resulting in a threefold increase in the lifetime of the sodium metal batteries.
FIGURE 10. Compatibility of sodium NaODFB-based electrolytes with different electrode materials: (A) Pathway of CEI film formation by FeF3 in NaODFB-based electrolyte (Chen et al., 2015). (B) Schematic illustrations of the NaDFOB-derived SEI structure on the surface of sodium metal (Gao et al., 2022).
In summary, NaDFOB has high compatibility with various common solvents used for NIBs, which means that NaDFOB may be very effective for various electrode materials for other NIBs. However, the preliminary work mainly focused on its application as an additive. We should optimize the electrolyte of NaODFB as the main salt and apply it to the full battery. Further studies on the complex interactions of NaDFOB electrolytes with different solvents with various electrode materials are also necessary. The goal of exploring its full potential as a emerging and high-performance electrolyte for sodium ion batteries will be realized in the future.
The study of the solid-liquid interface formed between electrolyte and electrode material is a hot research topic in the field of batteries. The solid-liquid interface film is formed between the electrolyte and electrode material during the first cycle of charging and discharging, and the presence of the interface film prevents the electrolyte from continuously contacting the electrode material and decomposing, thus allowing the electrochemical window of the electrolyte to be extended. Factors such as the denseness, thickness and components of the solid-liquid interfacial film have a great influence on the cycling performance of the battery, and obtaining a stable interfacial film with protective effect and stable Na+ transport has been the goal pursued by researchers.
In 1979, Peled (1979) found that alkali and alkaline earth metals in non-aqueous batteries form a surface film in contact with the electrolyte, which is an intermediate phase between the metal and the electrolyte and has the characteristics of an electrolyte, and hence the concept of “solid electrolyte interphase (SEI)” was introduced. At this time he considers the SEI model to be a simple two-dimensional passivation film structure. In 1997, Peled et al. (1997) suggested the mosaic model by arguing that insolubles generated from all types of reduction reactions of the electrolyte occurring simultaneously are deposited randomly mixed on the anode and stacked on each other to form a mosaic-like structure. In this model, grain boundaries and interfaces in SEI may act as electron conduction paths to promote the growth of dendrites and electron leakage. In 1999, Aurbach et al. (1999) proposed a multilayer structure of SEI films in lithium-ion battery systems using various means such as infrared spectroscopy, Raman spectroscopy, X-ray photoelectron spectroscopy, and electrochemical impedance spectroscopy, arguing that the passivation film formed at the beginning of the metallic Li surface is unstable and changes during the electrochemical process, with various types of substances forming one by one, and that traces of water in the electrolyte, solute Anion decomposition products also continue to influence the generated SEI film to form a multilayer film structure. This dynamic concept has also been applied to sodium ion batteries to derive bilayer and even multilayer structure models.
It is generally believed that the electrode-electrolyte interfacial film consists of the inorganic layer located on the inside connected to the electrode material and the organic layer located on the outside extending into the electrolyte. The inorganic layer is mostly inorganic with some sodium, and the organic layer is mostly organic with sodium formed by the reaction of solvent molecules with sodium. The formation of such a bilayer structure can be divided into two stages, namely, the formation of a bilayer on the surface when electrons flow into the anode and the participation of electrons in the reaction process. When the anode is filled with electrons, Na+ will be enriched on the electrode surface to form a bilayer. At the beginning the passivation film is thin and electron transfer is easy, so the double electron reaction occurs preferentially. The solvent molecules of the solubilized coordinated Na+ get electrons to be reduced and are more likely to produce inorganic products such as Na2CO3 and Na2O, which are precipitated on the electrode surface, while at the same time, sodium salt anions or additives may also participate in the reaction to produce NaF, NaCl, NaS, and Na2SO4, etc (Hu et al., 2020). The positive effect of NaF on dense SEI formation and unstable interphase growth control. Content increases appropriately to suppress the solubility of organic sodium carbonate (NaO2CO-C2H4-OCO2Na) and promote the conductivity of Na+ through the SEI layer, thus improving the electrochemical properties, whereas an increase in Na2CO3 content does not (Fondard et al., 2020). F-S or S=O species were also detected in the case of FSI− or bis(trifluoromethane)sulfonylimine (TFSI−) anions (Ding et al., 2019). However, as the thickness of the membrane increases, electron transfer is blocked and single-electron reactions begin to dominate, with organic species such as ROCO2Na (R is an organic group) organically accumulating on the inorganic layer to form an organic layer. The specific species of the organic and inorganic components depend on the reaction between the electrode surface and the electrolyte. Different electrode materials have different SEI components in different electrolyte systems. The thickness of the SEI film is usually between a few nanometers and tens of nanometers, which is mainly related to the electron tunneling distance, and if there is no surface damage or decomposition, after reaching the longest distance of electron tunneling, the solvent will not be able to continue to get electrons to be reduced and thus stop decomposing, and the thickness change of the SEI film will decrease and become an electron insulator and ion conductor, and stabilized (Hu et al., 2020). Recently, Cui et al. (Zhang et al., 2022) revealed the original structure and redefined the composition of SEI by using advanced cryo-electron microscopy to characterize the swelling state of SEI in various electrolytes, and showed that the swelling behavior depends on the electrolyte type and profoundly affects the ion transport in SEI. In the inorganic-rich SEI, the swelling rate is lower, resulting in a more stable electrochemical cycle of the cell.
The ideal SEI film should have the following characteristics: (i) good electronic insulator, preventing the electrolyte from being oxidized or reduced by charge transfer on the surface; (ii) good sodium ion conductivity, selectively allowing the passage of Na+ and preventing the solvent from entering the electrode material or directly contacting the electrode; (iii) good chemical and electrochemical stability, with no side reactions in the cell system; (iv) good thermal stability, stably adhering to the surface of the electrode material even at high temperatures; (v) homogeneous, dense and thin, possessing good mechanical properties and not easily flaking and dissolving (Hu et al., 2020).
The formation of the interfacial film is mainly the result of a combination of three factors: the energy polarization difference between the electrode and the electrolyte, the specific adsorption behavior and the ion solvation behavior, and the formation is accompanied by the interfacial growth and evolution. The specific formation mechanism is shown in Figure 11. The details of each part are developed below.
FIGURE 11. Schematic diagram of (A) the energy of the electrode and electrolyte (Gebresilassie Eshetu et al., 2018), (B) specific adsorption (Zhang and Zhao, 2009), (C) ion solventization (Li et al., 2020), (D) interface film formation, and (E) interfacial film growth and evolution.
The interfacial film arises from the difference in energy states between the two main parts of the electrode and the electrolyte (Gauthier et al., 2015). If the electrochemical potential μA of the anode is higher than the lowest unoccupied molecular orbital LUMO of the electrolyte, electrons are spontaneously transferred from the anode to the electrolyte, which leads to the reduction of the electrolyte and the formation of the interfacial film. Similarly, when the electrochemical potential μC of the cathode is lower than the highest occupied molecular orbital level HOMO of the electrolyte, electrons are transferred from the electrolyte to the anode and the solvent molecules of the electrolyte lose electrons leading to oxidation of the solvent, while the anode gains electrons and the transition metal cations (such as Mn4+, Ni4+, Co4+, etc.) in the material are reduced (Wang et al., 2020). The interfacial film on the surface of the anode, called SEI, is distinguished from the anode, and the products of electrolyte oxidation decomposition remain on the surface of the cathode, defined as the CEI passivation layer. To ensure a higher energy density, one chooses to initiate the redox reaction of the electrode at a voltage that exceeds the stability of the electrolyte. It is well known that non-aqueous electrolytes typically used in Li-ion batteries are oxidized if the operating voltage is higher than about 4.5 V with respect to Li+/Li. Given that the equilibrium potential of Na+/Na is 0.3 V higher than that of Li+/Li, some commonly used carbonate electrolytes will become thermodynamically unstable if the voltage in SIBs is higher than about 4.2 V (vs. Na+/Na) (You and Manthiram, 2018).
Specific adsorption behavior and ion solvation behavior are also the main factors affecting the formation of interfacial layers. The occurrence of specific adsorption behavior precedes the ion solvation behavior, i.e., the strong interaction of some substances with the electrode surface promotes the formation of interfacial films. The interfacial film model includes the inner Helmholtz plane (IHP) and the outer Helmholtz plane (OHP). In general, the specific absorption behavior of unsolvated molecules is mainly present in IHP, while ionic solvated structures are mainly present in OHP (Zhang and Zhao, 2009). The enrichment of specific substances initially adsorbed on the electrode surface determines the initial interfacial composition and structure, while the ion solvation structure subsequently acts to promote the growth of the interfacial layer (Yan et al., 2020). For ionic solventization behavior, the solventized structure is mainly related to the coordination of alkali metal cations (e.g., Na+) to electronegative atoms of the solvent molecule (e.g., carbonyl/ether oxygen) or anions (e.g., fluorine in NaPF6 salts). Their binding energy depends strongly on the type of cation, anion and solvent. Differences in the solvation structure and diffusion kinetics in different electrolytes subsequently lead to differences in the electrochemical properties of their organic and inorganic interfacial products. In addition, differences in ion solvation structures can lead to different degrees of changes in the electrolyte LUMO energy levels. The solventized Na+ structure at the molecular level in the electrolyte can change the preferential decomposition order of the solvent and the anion. As the salt concentration increases, the anions become more involved in the solventized shell layer, driving the transfer of LUMO from the solvent to the anion and forming an inorganic-rich interface (Xing et al., 2018). Thus, changes in the solventization environment will alter the previous order of solvent molecule or anion consumption, determining the initial composition formation of the internal interfacial layer, further affecting the organic/inorganic composition arrangement, structural evolution and overall ion transport capacity. The ionic solventization behavior is the main induction of surface interfacial phase formation and depends on the reduction/oxidation order of solvent molecules or anions (Li et al., 2020). The solvent-induced interfacial layer is dominated by the predominant organic matter in dilute electrolytes. However, the anion-induced interfacial layer in highly concentrated electrolytes consists of more inorganic species, such as NaF, NaCl and Na2CO3 (Zeng et al., 2018; Zheng et al., 2018; Yamada et al., 2019).
In addition to the regulation of specific adsorbed species during electrochemistry, the subsequent interfacial evolution is extremely important. The successive interfacial reactions are mainly driven by electron transfer based on a radical reaction mechanism. Usually, the outer organic layer is vulnerable to attack due to the preferential propagation of free radicals at the interface between the outer interfacial phase layer and the electrolyte, leading to organic polymerization (Soto et al., 2015). The evolutionary origin of the interfacial layer is therefore largely dependent on the electrochemical stability of the outer organic components. It determines which component is polymerized first and to what extent the dissolution and growth of the interfacial layer can occur. In particular, for some carbon anode materials with special microstructures (e.g., mesopores or nanopores), inward growth may occur inside the material (Bommier et al., 2016). The interfacial phase growth is related to the electrochemical reactivity of the components in the matrix electrolyte in addition to the interfacial phase components. In addition, the transport of sodium ions in the interfacial layer is a key factor affecting the evolution of the interfacial layer growth. The migration of Na+ ions in the interfacial layer is related to the desolvation process associated with the solventization behavior, the migration of Na+ ions through the interfacial reaction products, and the crystallinity and composition distribution of the interfacial layer. Among them, the desolvation behavior of Na+ at the electrode/electrolyte interface is a key step in determining the reaction rate. The ion desolvation energy potential depends on a combination of factors such as the strength of ion-solvent or ion-ion interactions, the choice of electrode, the presence of interfacial membrane, and the composition or structural condition of the interfacial membrane (Yamada and Yamada, 2015; Yan et al., 2020).
At present, the regulation strategy for interfacial film mainly includes four parts: electrolyte body regulation, concentration regulation, addition of functional additives and construction of artificial interfacial film (Figure 12). They are described as follows.
The actual state of the SEI actually depends on the choice of electrolyte composition, which determines to what extent the arrangement of inorganic and organic substances favors the final interfacial function. The current interfacial manipulation through the electrolyte ontology is mostly focused on the solventized structure of Na+, where the electrolyte solvent, salt anion, is involved (Hou et al., 2019), and a smaller percentage of external organic compounds by changing the solvent and sodium salt combination.
Solvents, as one of the participants in the solventized structure, mainly include esters, ethers and ionic liquids. For ester-based electrolytes, linear solvents can reduce the electrolyte viscosity and enhance the wettability, but usually linear solvents (DMC, DEC) are weakly coordinated with Na+ in the solventized structure, and therefore have high reactivity with the outer interfacial layer, which makes the interfacial layer unstable and may also increase the solubility of the interfacial layer (Xia et al., 2011; Ponrouch et al., 2013; Eshetu et al., 2016). The solventization of Na+ with cyclic EC and EC:PC is more favorable than that of linear solvents. Although the structural differences between EC and PC are small, the methyl group in PC may hinder the aggregation of reaction products from the perspective of long-term cell operation, resulting in insufficient formation of interphase layers (Takenaka and Nagaoka, 2019). In addition, since the LUMO level of Na+-solvent complexes decreases by 2–3 ev in varying degrees compared to a single solvent, the Na+-solvent complexes are more easily reduced on the anode surface and the increase in the HOMOLUMO energy band gap also leads to a longer operating window of the electrolyte. The addition of EC solvents to PC, DMC, EMC or DEC solvents results in the formation of a co-solvent, which reduces the band gap (Shakourian-Fard et al., 2015). Therefore, mixing different ratios of cyclic molecules (e.g., EC and PC) and chain molecules (DMC and DEC)) may lead to unexpected advantages. For ether solvents, such solvents can not only co-insert Na+ into graphite (Jache and Adelhelm, 2014; Kim et al., 2015; Cohn et al., 2016; Seidl et al., 2017), but also modulate the SEI passivation layer of the anode electrode material so that the interfacial phase composition has a better sodium ion transport rate and the generated passivation layer is thin and uniform (Soto et al., 2015; Wang C. et al., 2017; Zhang et al., 2017; He et al., 2018; Huang et al., 2019; Li et al., 2019). Among them, the special structure of amorphous Na2CO3 and NaF particles dispersed in polyether species improves the electrical conductivity of Na+ (Huang et al., 2019). However, the ether-derived interfacial phase is slightly lower than the ester-derived interfacial phase in terms of long-cycle performance. One optimizes the formation of the interfacial layer for high rate and long cycle performance by combining ester and ether, where the thick and loose ester-SEI is initially formed on the inside and the thin and dense ether-SEI is on the outside (Bai et al., 2018). ILs are actually salts in liquid state at room temperature compared to conventional molecular solvents (such as carbonates and ethers), which exhibit relatively low ionic conductivity due to strong interactions between their anions and cations, but they have the advantage of high electrochemical and thermal stability due to their low vapor pressure and low flammability. Therefore, hybrid electrolytes obtained by mixing ionic liquids with conventional molecular solvents such as organic carbonates can be used, thus combining all advantages to obtain interfacial layers with better performance (Monti et al., 2016; Manohar et al., 2018b).
Anionic salts, another major player in the solventized structure, play a crucial role in the oxidative decomposition of electrolytes along with the solvent. Some anions, such as BF4− and PF6−, have been found to reduce the oxidative stability of common carbonate solvents, such as EC, PC and DMC, through fluorine or proton transfer reactions (Borodin and Jow, 2010). In addition, oxidation between the salt anion and the solvent through electrostatic interactions may occur accompanied by charge transfer phenomena to reach the final coupled state. This determines the oxidative stability of the solventized salt (Fadel et al., 2019). When Na+ is transported through the interfacial layer, anions with lower donor numbers (e.g., PF6− and ClO4−) are more easily desolvated (Browning et al., 2017). Therefore, different anion-solvent complexes can have different effects on electrolyte oxidation stability during electrolyte decomposition on the cathode surface (Borodin and Jow, 2010; Xing et al., 2011; Cresce et al., 2015; Fadel et al., 2019). Different salts (e.g., NaClO4, NaFSI, NaTFSI) undergo different pathways when decomposed on the cathode surface, resulting in components with different properties that affect the performance of the interfacial layer. Therefore, mixed anions may provide additional benefits in regulating the interfacial chemistry.
The concentration effect is an important interfacial modulation strategy, and adjusting the optimized concentration can accordingly modulate the interfacial passivation chemistry to achieve an organic-inorganic equilibrium SEI layer. Traditionally, most electrolytes with optimized salt concentrations around 1 M exhibit the highest ionic conductivity (Yamada et al., 2019). Although the increase in salt concentration decreases the ionic conductivity, it exhibits special advantages in terms of enhanced interfacial properties and electrochemical behavior that conventional electrolytes do not possess. The decomposition order between solvent and salt differs with salt concentration. At conventional dilution concentrations the solvent decomposes preferentially, while at high concentrations, where there are almost no free solvent molecules left due to the urgent need to satisfy the dissolution of a large number of cations, the anion is forced to be decomposed first (Yamada and Yamada, 2015; 2017; Yamada et al., 2019; Yan et al., 2020). Li et al. (2020) found that, by reducing the sodium salt concentration (0.3 mol NaPF6/EC + PC (1:1 by volume)), the solvent molecules can fully occupy the Na+ solventized sheath layer, and the CEI and SEI films with high organic content (high C + O ratio) can be obtained on the cathode and anode sides. As the concentration of PF6− is reduced, the decomposition by-products such as F, which has a corrosive effect on the electrode materials, are reduced, and the obtained SEI and CEI films are more stable. On the contrary, when applying high salt concentration electrolyte, the Na+ solvated sheath layer (or ligand layer) is almost occupied by anions. This will bring some special advantages. For example, the interfacial mass transfer process is changed and fast reactions can be performed on the electrode, the electrolyte volatility is weakened, the thermodynamic stability is enhanced and the safety is improved, the good SEI film can be formed on the electrode, and the Al collector is protected from anion corrosion. Increasing sodium salt concentration has an effect on the properties of the Na+ solubilization environment (Wahlers et al., 2016; Chen et al., 2018; Kankanamge et al., 2018; Li et al., 2018), charge transport mechanism (Forsyth et al., 2016; Kankanamge et al., 2018), and Na+ conductivity (Forsyth et al., 2016; Kankanamge et al., 2018). In addition, the overall ionic conductivity of the ether solvent-based electrolytes showed a tendency to increase with increasing sodium salt concentration. Electrolytes based on ionic liquid solvents promote ionic movement with the continuous addition of salts (Chen et al., 2018). Therefore, there are concepts of hyperconcentration and localized high concentration electrolytes have been proposed in recent years. Superconcentration because it promotes preferential passivation of LUMO-level reducing anions, which in turn creates a more powerful inorganic SEI that can better mitigate the more severe solubility problems of organic components. Moreover, a lower percentage of free solvent molecules will mitigate the tendency of soluble components to dissolve into the electrolyte (Takada et al., 2017). Localized high electrolyte concentrations not only do not alter the local dissolution environment of the concentrated electrolyte, but also provide the advantage of interface enhancement or suppression of undesirable interface problems (Zheng et al., 2018; Yamada et al., 2019).
Additivity refers to the introduction of small doses of foreign molecules into the parent electrolyte. As one of the most economical interfacial modulation strategies, the addition of additives not only does not interfere with the overall properties of the electrolyte, but also significantly tunes the interfacial layer properties to better form films for interphase passivation for electrode protection and thus improve the overall electrochemical performance. Currently, FEC is the most widely reported and effective additive. Its energy gap, Eg is located between the HOMO-LUMO gap of salt and solvent, thus FEC can stabilize the interfacial layer by sacrificing decomposition in advance thus avoiding destructive decomposition of electrolyte (Wang et al., 2020). For example, the reason for the good performance in EC-based electrolytes containing FEC is that FEC has lower decomposition energy compared to EC solvents, and the presence of FEC also enhances the decomposition energy of EC molecules, so that its early decomposition in EC-based electrolytes promotes the generation of interfacial films (Kumar et al., 2016). In PC-based electrolytes, FEC also shows good film formation due to its still early decomposition compared to PC (Takenaka et al., 2015). It should be noted here that due to the strong electronegativity of F atoms, FEC attracts the positive charge of organic products, and excess FEC prevents the formation of dimers between organic monomer products, a phenomenon that causes undesired interfacial layer growth and adversely affects the stability of interfacial films (Takenaka et al., 2015; Simone et al., 2017; Bouibes et al., 2018). In addition to FEC, other additives as described above also contribute to the formation of stable interfacial films and need to be used in combination with different electrolyte and electrode systems for screening. In addition, the combined use of different additives may also be beneficial to achieve the desired stable interfacial film.
Usually, the electrode itself is attacked by the highly reactive electrolyte, which may undergo structural changes or cause surface defects, etc. This will lead to further decomposition of the electrolyte, resulting in lower first-loop Coulomb efficiency and thicker interfacial films. Therefore, surface coating of the electrode can effectively improve its surface properties and improve the compatibility with the electrolyte. In fact, some of the cladding work done at the cathode is also equivalent to artificially creating CEI films. By using atomic layer deposition (ALD) to coat metal oxides (AlO3, TiO2 and MgO), metal fluorides and even solid electrolytes on the surface of the cathode material, these coatings maintain the stability of the reversible phase change of the cathode material during charging and discharging, or prevent the erosion of the cathode material by by-products such as HF, or provide better channels for Na+ transport provides a better channel and acts as a CEI film. For example, Sun et al. (Hwang et al., 2017a) changed the interfacial properties of the cathode by coating a layer of nano-Al2O3 on the surface of Na[Ni0.6Co0.2Mn0.2]O2 cathode. On the one hand, nano-Al2O3 can react with F present in the electrolyte to reduce the content of HF and prevent the continuous accumulation of NaF as a by-product to hinder Na+ conduction; on the other hand, AlF3, the product of nano-Al2O3 and HF, can enhance the protection of the cathode material by CEI film as a good component of CEI film. With the synergistic effect of the two, the change of interfacial impedance of the coated cathode material during the cycling process was significantly smaller than that before the coating, and the presence of the coating layer also helped to reduce the leaching of transition metals from the active material. Ye et al. (2022) reported an in situ artificial CEI construction strategy based on a spontaneous redox reaction between a pre-sodiumed organic solvent and a polyvinylidene fluoride (PVDF) binder. Applying this strategy to PB cathodes, the chemically pretreated PB cathodes were successfully coated with a NaF-rich interfacial phase on the electrode surface to keep them away from electrolyte attack and maintain cycling stability. This artificial CEI based on the interaction between PVDF and organic solvents is not much affected by the surface properties of the cathode material and is expected to be applied to other cathode materials. Therefore, the construction of artificial interfacial film is also an effective means to improve the compatibility of electrode with electrolyte and avoid some side reactions.
In summary, there are four common effective strategies for the regulation of solid electrolyte interfacial film. In practice, it is necessary to consider the characteristics of the electrolyte and electrode materials to choose the appropriate regulation. If the electrolyte body has a great influence on the interfacial membrane, it can try to use different salt and solvent mixing to regulate the interfacial membrane. If the electrolyte interfacial film is regulated on the basis of not changing the electrolyte body, the concentration of electrolyte or functional additives can be changed to achieve the purpose of regulating the appropriate interfacial film. If the interfacial film formed inside the battery system is unstable and cannot be improved by adjusting the electrolyte body, concentration, or additives, a suitable artificial interfacial film can be constructed from the electrodes to realize excellent electrochemical performance.
With the advantages of abundant sodium resources and low cost, sodium ion batteries are a promising energy storage battery system. At present, researchers at home and abroad have developed a variety of feasible cathode and anode materials for sodium ion batteries. The electrolyte of sodium ion battery, as a medium for the cathode and anode materials to participate in the redox reaction, has an important influence on the thermodynamic and kinetic properties of the sodium ion battery system, such as the structural stability of the electrode materials, the composition and structure of the SEI, the multiplicative performance, cycling stability and thermal stability of the battery. Therefore, electrolyte is also the key to determine the battery performance. This paper introduces the research progress of organic liquid electrolytes for sodium ion batteries from the basic requirements and composition of organic liquid electrolytes, the current research status of organic liquid electrolytes, and the composition, requirements and regulation strategies of the interface between electrolytes and electrodes. First, an overview of organic liquid electrolytes is introduced, followed by the classification of organic liquid electrolytes from the perspective of sodium salts, and the compatibility and electrochemical properties of each sodium salt electrolyte with cathode and anode are introduced. Finally, the strategy for electrolyte regulation of interfacial film is explained. At present, organic liquid electrolytes for sodium ion batteries still have problems such as narrow electrochemical windows and poor stability of SEI films. The development of new, low-cost and high-performance sodium ion battery electrolytes is crucial for the commercialization of sodium ion batteries. Future research on organic liquid electrolytes for sodium ion batteries can be carried out from the following aspects.
(1) Optimization of each individual component of the organic liquid electrolyte, including its own physical and chemical properties such as viscosity, conductivity, stability, etc. The compatibility of the electrolyte with the electrode material is also crucial. For example, the commonly used ester electrolyte cannot be applied to graphite anode materials, but the ether electrolyte allows sodium ions to enter the interlayer energy storage in a solventized form. The selection of additives also needs to consider the compatibility with electrolyte and electrode materials as well. In addition, in the future, we should try to explore the internal energy balance of solvent molecules from the perspective of molecular dynamics simulation and analyze the sodium storage mechanism of sodium ion battery in combination with the special structure of electrode materials, which is more conducive to enhance the matching of electrolyte and electrode materials to achieve high capacity requirements. In conclusion, we should focus on the matching of electrode electrolyte and the development of new sodium salts and additives to achieve high performance of sodium ion batteries.
(2) Battery safety is the most important key indicator of market and customer concern. Commonly used organic electrolytes cannot operate properly in high temperature environments, so the use of stable Na salts and non-flammable solvents including ionic liquids and phosphate esters to replace traditional flammable solvents, as well as the addition of flame retardant additives and overcharge additives are within consideration to achieve sodium ion battery safety. In general, the use of stable Na salts increases the thermal stability of the electrolyte, and high concentrations of the electrolyte exhibit reduced flammability. The use of non-flammable solvents instead of traditional flammable solvents is attractive because they can make the electrolyte completely non-flammable. Electrolyte reformulation consisting of flame retardant additives and overcharge additives is economical and effective. However, the high cost required for nonflammable electrolytes is a significant limitation to their commercialization.
(3) The electrolyte of sodium ion battery, as a medium for the cathode and anode materials to participate in the redox reaction, its redox window, the migration and diffusion of sodium ions, the solventized structure of sodium ions, and the coupling correlation effect between sodium ions and anions or solvents are the key factors that determine the interfacial properties of electrode materials. In addition, the sensitive nature of the interfacial phase increases the difficulty of characterization and limits our understanding of the interfacial phase. Regulation of electrolyte proprieties, concentration effects, electrolyte additives, and artificial interfacial films are effective methods to manipulate interphase formation. To meet the requirements of applications, the enhancement of interfacial composition, structure, and stability requires more fundamental work, theoretical computational studies, and advanced testing and analysis methods.
In conclusion, in the context of the imminent commercialization of sodium-ion batteries, substantial progress has been made in the research on positive and negative electrode materials. For example, the anode materials currently used in commercialized sodium-ion batteries are all hard carbon. There are already examples of commercialized production of the three main types of cathode materials. However, the organic electrolyte system used is still the solvents (EC:PC, EC:DEC or PC as a single solvent) and the sodium salts (NaClO4 and NaPF6). There is still a long way to go for the commercialization of organic liquid electrolytes corresponding to specific scenarios (high voltage, wide temperature, non-flammable). In the future, for the development of organic liquid electrolytes, great efforts are still needed to design safer electrolytes and more stable interfaces for SIBs. The optimization of electrolyte and solid electrolyte interface films will further bring sodium ion batteries closer to practical applications, allowing them to be widely used in the direction of large-scale energy storage and promoting commercial applications.
JZ: Writing–original draft, Writing–review and editing. JL: Writing–review and editing. HW: Writing–review and editing. MW: Conceptualization, Supervision, Writing–review and editing.
The authors declare that the research was conducted in the absence of any commercial or financial relationships that could be construed as a potential conflict of interest.
All claims expressed in this article are solely those of the authors and do not necessarily represent those of their affiliated organizations, or those of the publisher, the editors and the reviewers. Any product that may be evaluated in this article, or claim that may be made by its manufacturer, is not guaranteed or endorsed by the publisher.
Armand, M., Endres, F., Macfarlane, D. R., Ohno, H., and Scrosati, B. (2009). Ionic-liquid materials for the electrochemical challenges of the future. Nat. Mater. 8, 621–629. doi:10.1038/nmat2448
Aurbach, D., Markovsky, B., Levi, M. D., Levi, E., Schechter, A., Moshkovich, M., et al. (1999). New insights into the interactions between electrode materials and electrolyte solutions for advanced nonaqueous batteries. J. Power Sources 81, 95–111. doi:10.1016/s0378-7753(99)00187-1
Ba, D., Gui, Q., Liu, W., Wang, Z., Li, Y., and Liu, J. (2022). Robust cathode-ether electrolyte interphase on interfacial redox assembled fluorophosphate enabling high-rate and ultrastable sodium ion full cells. Nano Energy 94, 106918. doi:10.1016/j.nanoen.2022.106918
Bai, P., He, Y., Xiong, P., Zhao, X., Xu, K., and Xu, Y. (2018). Long cycle life and high rate sodium-ion chemistry for hard carbon anodes. Energy Storage Mater. 13, 274–282. doi:10.1016/j.ensm.2018.02.002
Benchakar, M., Naejus, R., Damas, C., and Santos-Pena, J. (2020). Exploring the use of EMImFSI ionic liquid as additive or co-solvent for room temperature sodium ion battery electrolytes. Electrochimica Acta 330, 135193. doi:10.1016/j.electacta.2019.135193
Bhide, A., Hofmann, J., Dürr, A. K., Janek, J., and Adelhelm, P. (2014). Electrochemical stability of non-aqueous electrolytes for sodium-ion batteries and their compatibility with Na 0.7 CoO 2. Phys. Chem. Chem. Phys. 16, 1987–1998. doi:10.1039/c3cp53077a
Bommier, C., Leonard, D., Jian, Z., Stickle, W. F., Greaney, P. A., and Ji, X. (2016). New paradigms on the nature of solid electrolyte interphase formation and capacity fading of hard carbon anodes in Na-ion batteries. Adv. Mater. Interfaces 3, 1600449. doi:10.1002/admi.201600449
Borodin, O., and Jow, T. R. (2010). Quantum chemistry studies of the oxidative stability of carbonate, sulfone and sulfonate-based electrolytes doped with BF4-PF6- anions. ECS Transcations 33, 77–84. doi:10.1149/1.3563092
Bouibes, A., Takenaka, N., Fujie, T., Kubota, K., Komaba, S., and Nagaoka, M. (2018). Concentration effect of fluoroethylene carbonate on the formation of solid electrolyte interphase layer in sodium-ion batteries. Acs Appl. Mater. Interfaces 10, 28525–28532. doi:10.1021/acsami.8b07530
Brehm, W., Santhosha, A. L., Zhang, Z., Neumann, C., Turchanin, A., Martin, A., et al. (2020). Copper thiophosphate (Cu3PS4) as electrode for sodium-ion batteries with ether electrolyte. Adv. Funct. Mater. 30, 1910583. doi:10.1002/adfm.201910583
Browning, K. L., Sacci, R. L., and Veith, G. M. (2017). Energetics of Na+ transport through the electrode/cathode interface in single solvent electrolytes. J. Electrochem. Soc. 164, A580–A586. doi:10.1149/2.0311704jes
Cabello, M., Chyrka, T., Klee, R., Aragon, M. J., Bai, X., Lavela, P., et al. (2017). Treasure Na-ion anode from trash coke by adept electrolyte selection. J. Power Sources 347, 127–135. doi:10.1016/j.jpowsour.2017.02.065
Cao, J., Wan, Y., Tu, G., Zhang, S., Xia, A., Liu, X., et al. (2013). Information system architecture for smart grids. Chin. J. Comput. 36, 143–167. doi:10.3724/SP.J.1016.2013.00143
Chagas, L. G., Buchholz, D., Wu, L., Vortmann, B., and Passerini, S. (2014). Unexpected performance of layered sodium-ion cathode material in ionic liquid-based electrolyte. J. Power Sources 247, 377–383. doi:10.1016/j.jpowsour.2013.08.118
Che, H., Chen, S., Xie, Y., Wang, H., Amine, K., Liao, X.-Z., et al. (2017). Electrolyte design strategies and research progress for room-temperature sodium-ion batteries. Energy & Environ. Sci. 10, 1075–1101. doi:10.1039/c7ee00524e
Che, H., Yang, X., Wang, H., Liao, X.-Z., Zhang, S. S., Wang, C., et al. (2018). Long cycle life of sodium-ion pouch cell achieved by using multiple electrolyte additives. J. Power Sources 407, 173–179. doi:10.1016/j.jpowsour.2018.08.025
Chen, C., Wu, M., Liu, J., Xu, Z., Zaghib, K., and Wang, Y. (2020). Effects of ester-based electrolyte composition and salt concentration on the Na-storage stability of hard carbon anodes. J. Power Sources 471, 228455. doi:10.1016/j.jpowsour.2020.228455
Chen, F., Howlett, P., and Forsyth, M. (2018). Na-ion solvation and high transference number in superconcentrated ionic liquid electrolytes: A theoretical approach. J. Phys. Chem. C 122, 105–114. doi:10.1021/acs.jpcc.7b09322
Chen, J., Huang, Z., Wang, C., Porter, S., Wang, B., Lie, W., et al. (2015). Sodium-difluoro(oxalato)borate (NaDFOB): A new electrolyte salt for Na-ion batteries. Chem. Commun. 51, 9809–9812. doi:10.1039/c5cc02901e
Chen, M., Pan, Z., Jin, X., Chen, Z., Zhong, Y., Wang, X., et al. (2019). A highly integrated All-manganese battery with oxide nanoparticles supported on the cathode and anode by super-aligned carbon nanotubes. J. Mater. Chem. A 7, 4494–4504. doi:10.1039/c8ta11415c
Cheng, Z., Mao, Y., Dong, Q., Jin, F., Shen, Y., and Chen, L. (2019). Fluoroethylene carbonate as an additive for sodium-ion batteries: Effect on the sodium cathode. Acta Physico-Chimica Sin. 35, 868–875. doi:10.3866/pku.whxb201811033
Cohn, A. P., Share, K., Carter, R., Oakes, L., and Pint, C. L. (2016). Ultrafast solvent-assisted sodium ion intercalation into highly crystalline few-layered graphene. Nano Lett. 16, 543–548. doi:10.1021/acs.nanolett.5b04187
Cresce, A. V. W., Gobet, M., Borodin, O., Peng, J., Russell, S. M., Wikner, E., et al. (2015). Anion solvation in carbonate-based electrolytes. J. Phys. Chem. C 119, 27255–27264. doi:10.1021/acs.jpcc.5b08895
Darwiche, A., Dugas, R., Fraisse, B., and Monconduit, L. (2016). Reinstating lead for high-loaded efficient negative electrode for rechargeable sodium-ion battery. J. Power Sources 304, 1–8. doi:10.1016/j.jpowsour.2015.10.087
Darwiche, A., Marino, C., Sougrati, M. T., Fraisse, B., Stievano, L., and Monconduit, L. (2012). Better cycling performances of bulk Sb in Na-ion batteries compared to Li-ion systems: An unexpected electrochemical mechanism. J. Am. Chem. Soc. 134, 20805–20811. doi:10.1021/ja310347x
De Sloovere, D., Vanpoucke, D. E. P., Paulus, A., Joos, B., Calvi, L., Vranken, T., et al. (2022). Deep eutectic solvents as nonflammable electrolytes for durable sodium-ion batteries. Adv. Energy Sustain. Res. 3, 2100159. doi:10.1002/aesr.202100159
Devlin, D. J., and Herley, P. J. (1987). Thermal decomposition and dehydration of sodium perchlorate monohydrate. React. solids 3, 75–84. doi:10.1016/0168-7336(87)80019-0
Ding, Y., Guo, X., Qian, Y., Zhang, L., Xue, L., Goodenough, J. B., et al. (2019). A liquid-metal-enabled versatile organic alkali-ion battery. Adv. Mater. 31, 1806956. doi:10.1002/adma.201806956
Dubois, M., Ghanbaja, J., and Billaud, D. (1997). Electrochemical intercalation of sodium ions into poly(para-phenylene) in carbonate-based electrolytes. Synth. Met. 90, 127–134. doi:10.1016/s0379-6779(97)81261-1
Dunn, B., Kamath, H., and Tarascon, J.-M. (2011). Electrical energy storage for the grid: A battery of choices. Science 334, 928–935. doi:10.1126/science.1212741
Duong Tung, P., Sambandam, B., Kim, S., Jo, J., Kim, S., Park, S., et al. (2018). Dandelion-shaped manganese sulfide in ether-based electrolyte for enhanced performance sodium-ion batteries. Commun. Chem. 1, 83. doi:10.1038/s42004-018-0084-1
Dustmann, C.-H. (2004). Advances in ZEBRA batteries. J. power sources 127, 85–92. doi:10.1016/j.jpowsour.2003.09.039
Egashira, M., Tanaka, T., Yoshimoto, N., and Morita, M. (2012). Influence of ionic liquid species in non-aqueous electrolyte on sodium insertion into hard carbon. Electrochemistry 80, 755–758. doi:10.5796/electrochemistry.80.755
Eshetu, G. G., Diemant, T., Hekmatfar, M., Grugeon, S., Behm, R. J., Laruelle, S., et al. (2019). Impact of the electrolyte salt anion on the solid electrolyte interphase formation in sodium ion batteries. Nano Energy 55, 327–340. doi:10.1016/j.nanoen.2018.10.040
Eshetu, G. G., Grugeon, S., Kim, H., Jeong, S., Wu, L., Gachot, G., et al. (2016). Comprehensive insights into the reactivity of electrolytes based on sodium ions. Chemsuschem 9, 462–471. doi:10.1002/cssc.201501605
Evans, T., Olson, J., Bhat, V., and Lee, S.-H. (2014). Corrosion of stainless steel battery components by bis(fluorosulfonyl)imide based ionic liquid electrolytes. J. Power Sources 269, 616–620. doi:10.1016/j.jpowsour.2014.07.047
Fadel, E. R., Faglioni, F., Samsonidze, G., Molinari, N., Merinov, B. V., Goddard, W. A., et al. (2019). Role of solvent-anion charge transfer in oxidative degradation of battery electrolytes. Nat. Commun. 10, 3360. doi:10.1038/s41467-019-11317-3
Fang, W., Jiang, H., Zheng, Y., Zheng, H., Liang, X., Sun, Y., et al. (2020). A bilayer interface formed in high concentration electrolyte with SbF3 additive for long-cycle and high-rate sodium metal battery. J. Power Sources 455, 227956. doi:10.1016/j.jpowsour.2020.227956
Fang, Y., Xiao, L., Chen, Z., Ai, X., Cao, Y., and Yang, H. (2018). Recent advances in sodium-ion battery materials. Electrochem. Energy Rev. 1, 294–323. doi:10.1007/s41918-018-0008-x
Feng, J., Ci, L., and Xiong, S. (2015a). Biphenyl as overcharge protection additive for nonaqueous sodium batteries. Rsc Adv. 5, 96649–96652. doi:10.1039/c5ra19988c
Feng, J., Zhang, Z., Li, L., Yang, J., Xiong, S., and Qian, Y. (2015b). Ether-based nonflammable electrolyte for room temperature sodium battery. J. Power Sources 284, 222–226. doi:10.1016/j.jpowsour.2015.03.038
Fenton, D., Parker, J., and Wright, P. (1973). Complexes of alkali metal ions with poly (ethylene oxide). polymer 14, 589. doi:10.1016/0032-3861(73)90146-8
Fondard, J., Irisarri, E., Courreges, C., Palacin, M. R., Ponrouch, A., and Dedryvere, R. (2020). SEI composition on hard carbon in Na-ion batteries after long cycling: Influence of salts (NaPF6, NaTFSI) and additives (FEC, DMCF). J. Electrochem. Soc. 167, 070526. doi:10.1149/1945-7111/ab75fd
Forsyth, M., Hilder, M., Zhang, Y., Chen, F., Carre, L., Rakov, D. A., et al. (2019). Tuning sodium interfacial chemistry with mixed-anion ionic liquid electrolytes. Acs Appl. Mater. Interfaces 11, 43093–43106. doi:10.1021/acsami.9b12913
Forsyth, M., Yoon, H., Chen, F., Zhu, H., Macfarlane, D. R., Armand, M., et al. (2016). Novel Na+ ion diffusion mechanism in mixed organic-inorganic ionic liquid electrolyte leading to high Na+ transference number and stable, high rate electrochemical cycling of sodium cells. J. Phys. Chem. C 120, 4276–4286. doi:10.1021/acs.jpcc.5b11746
Gao, H., Grundish, N. S., Zhao, Y., Zhou, A., and Goodenough, J. B. (2021). Formation of stable interphase of polymer-in-salt electrolyte in all-solid-state lithium batteries. Energy Mater. Adv. 2020, 1–10. doi:10.34133/2020/1932952
Gao, L., Chen, J., Chen, Q., and Kong, X. (2022). The chemical evolution of solid electrolyte interface in sodium metal batteries. Sci. Adv. 8, eabm4606. doi:10.1126/sciadv.abm4606
Gao, Y., Chen, G., Wang, X., Bai, Y., and Wu, C. (2020). Safety of electrolytes for sodium-ion batteries:Strategies and progress. Energy Storage Sci. Technol. 9, 1309–1317. doi:10.19799/j.cnki.2095-4239.2020.0230
Gauthier, M., Carney, T. J., Grimaud, A., Giordano, L., Pour, N., Chang, H.-H., et al. (2015). Electrode-electrolyte interface in Li-ion batteries: Current understanding and new insights. J. Phys. Chem. Lett. 6, 4653–4672. doi:10.1021/acs.jpclett.5b01727
Gebresilassie Eshetu, G., Martinez-Ibanez, M., Sanchez-Diez, E., Gracia, I., Li, C., Rodriguez-Martinez, L. M., et al. (2018). Electrolyte additives for room-temperature, sodium-based, rechargeable batteries. Chemistry-an Asian J. 13, 2770–2780. doi:10.1002/asia.201800839
Goktas, M., Bolli, C., Buchheim, J., Berg, E. J., Novak, P., Bonilla, F., et al. (2019). Stable and unstable diglyme-based electrolytes for batteries with sodium or graphite as electrode. Acs Appl. Mater. Interfaces 11, 32844–32855. doi:10.1021/acsami.9b06760
Goodenough, J. B., and Kim, Y. (2010). Challenges for rechargeable Li batteries. Chem. Mater. 22, 587–603. doi:10.1021/cm901452z
Han, B., Zou, Y., Zhang, Z., Yang, X., Shi, X., Meng, H., et al. (2021). Probing the Na metal solid electrolyte interphase via cryo-transmission electron microscopy. Nat. Commun. 12, 3066. doi:10.1038/s41467-021-23368-6
Han, P., Han, X., Yao, J., Liu, Z., Cao, X., and Cui, G. (2015). Flexible graphite film with laser drilling pores as novel integrated anode free of metal current collector for sodium ion battery. Electrochem. Commun. 61, 84–88. doi:10.1016/j.elecom.2015.10.009
Hasa, I., Passerini, S., and Hassoun, J. (2016). Characteristics of an ionic liquid electrolyte for sodium-ion batteries. J. Power Sources 303, 203–207. doi:10.1016/j.jpowsour.2015.10.100
He, Y., Bai, P., Gao, S., and Xu, Y. (2018). Marriage of an ether-based electrolyte with hard carbon anodes creates superior sodium-ion batteries with high mass loading. Acs Appl. Mater. Interfaces 10, 41380–41388. doi:10.1021/acsami.8b15274
Hong, S., Kim, Y., Park, Y., Choi, A., Choi, N., and Lee, K. (2015). Charge carriers in rechargeable batteries: Na ions vs. Li ions. Energy Environ. Sci. 6, 2067. doi:10.1039/c3ee40811f
Hou, T., Yang, G., Rajput, N. N., Self, J., Park, S.-W., Nanda, J., et al. (2019). The influence of FEC on the solvation structure and reduction reaction of LiPF6/EC electrolytes and its implication for solid electrolyte interphase formation. Nano Energy 64, 103881. doi:10.1016/j.nanoen.2019.103881
Huang, J., Guo, X., Du, X., Lin, X., Huang, J.-Q., Tan, H., et al. (2019). Nanostructures of solid electrolyte interphases and their consequences for microsized Sn anodes in sodium ion batteries. Energy & Environ. Sci. 12, 1550–1557. doi:10.1039/c8ee03632b
Hwang, J.-Y., Myung, S.-T., Choi, J. U., Yoon, C. S., Yashiro, H., and Sun, Y.-K. (2017a). Resolving the degradation pathways of the O3-type layered oxide cathode surface through the nano-scale aluminum oxide coating for high-energy density sodium-ion batteries. J. Mater. Chem. A 5, 23671–23680. doi:10.1039/c7ta08443a
Hwang, J.-Y., Myung, S.-T., and Sun, Y.-K. (2017b). Sodium-ion batteries: Present and future. Chem. Soc. Rev. 46, 3529–3614. doi:10.1039/c6cs00776g
Jache, B., and Adelhelm, P. (2014). Use of graphite as a highly reversible electrode with superior cycle life for sodium-ion batteries by making use of Co-intercalation phenomena. Angew. Chemie-International Ed. 53, 10333–10337. doi:10.1002/ange.201403734
Jang, J. Y., Lee, Y., Kim, Y., Lee, J., Lee, S.-M., Lee, K. T., et al. (2015). Interfacial architectures based on a binary additive combination for high-performance Sn4P3 anodes in sodium-ion batteries. J. Mater. Chem. A 3, 8332–8338. doi:10.1039/c5ta00724k
Jian, Z., Han, W., Lu, X., Yang, H., Hu, Y.-S., Zhou, J., et al. (2013). Superior electrochemical performance and storage mechanism of Na3V2(PO4)3 cathode for room-temperature sodium-ion batteries. Adv. Energy Mater. 3, 156–160. doi:10.1002/aenm.201200558
Jin, Y., Xu, Y., Xiao, B., Engelhard, M. H., Yi, R., Vo, T. D., et al. (2022). Stabilizing interfacial reactions for stable cycling of high-voltage sodium batteries. Adv. Funct. Mater. 32. doi:10.1002/adfm.202204995
Kamath, G., Cutler, R. W., Deshmukh, S. A., Shakourian-Fard, M., Parrish, R., Huether, J., et al. (2014). In silico based rank-order determination and experiments on nonaqueous electrolytes for sodium ion battery applications. J. Phys. Chem. C 118, 13406–13416. doi:10.1021/jp502319p
Kankanamge, S. R. G., Li, K., Fulfer, K. D., Du, P., Jorn, R., Kumar, R., et al. (2018). Mechanism behind the unusually high conductivities of high concentrated sodium ion glyme-based electrolytes. J. Phys. Chem. C 122, 25237–25246. doi:10.1021/acs.jpcc.8b06991
Kim, H., Hong, J., Yoon, G., Kim, H., Park, K.-Y., Park, M.-S., et al. (2015). Sodium intercalation chemistry in graphite. Energy & Environ. Sci. 8, 2963–2969. doi:10.1039/c5ee02051d
Komaba, S., Ishikawa, T., Yabuuchi, N., Murata, W., Ito, A., and Ohsawa, Y. (2011a). Fluorinated ethylene carbonate as electrolyte additive for rechargeable Na batteries. Acs Appl. Mater. Interfaces 3, 4165–4168. doi:10.1021/am200973k
Komaba, S., Murata, W., Ishikawa, T., Yabuuchi, N., Ozeki, T., Nakayama, T., et al. (2011b). Electrochemical Na insertion and solid electrolyte interphase for hard-carbon electrodes and application to Na-ion batteries. Adv. Funct. Mater. 21, 3859–3867. doi:10.1002/adfm.201100854
Koo, B., Chattopadhyay, S., Shibata, T., Prakapenka, V. B., Johnson, C. S., Rajh, T., et al. (2013). Intercalation of sodium ions into hollow iron oxide nanoparticles. Chem. Mater. 25, 245–252. doi:10.1021/cm303611z
Kumar, H., Detsi, E., Abraham, D. P., and Shenoy, V. B. (2016). Fundamental mechanisms of solvent decomposition involved in solid-electrolyte interphase formation in sodium ion batteries. Chem. Mater. 28, 8930–8941. doi:10.1021/acs.chemmater.6b03403
Larcher, D., and Tarascon, J. M. (2015). Towards greener and more sustainable batteries for electrical energy storage. Nat. Chem. 7, 19–29. doi:10.1038/nchem.2085
Lee, Y., Lee, J., Kim, H., Kang, K., and Choi, N.-S. (2016). Highly stable linear carbonate-containing electrolytes with fluoroethylene carbonate for high-performance cathodes in sodium-ion batteries. J. Power Sources 320, 49–58. doi:10.1016/j.jpowsour.2016.04.070
Li, H., Chen, H., Shen, X., Liu, X., Fang, Y., Zhong, F., et al. (2022). High-voltage and intrinsically safe sodium metal batteries enabled by nonflammable fluorinated phosphate electrolytes. Acs Appl. Mater. Interfaces 14, 43387–43396. doi:10.1021/acsami.2c13295
Li, H., Zhang, H., Diemant, T., Behm, R. J., Geiger, D., Kaiser, U., et al. (2021a). Reversible copper sulfide conversion in nonflammable trimethyl phosphate electrolytes for safe sodium-ion batteries. Small Struct. 2, 2100035. doi:10.1002/sstr.202100035
Li, K., Kankanamge, S. R. G., Weldeghiorghis, T. K., Jorn, R., Kuroda, D. G., and Kumar, R. (2018). Predicting ion association in sodium electrolytes: A transferrable model for investigating glymes. J. Phys. Chem. C 122, 4747–4756. doi:10.1021/acs.jpcc.7b09995
Li, K., Zhang, J., Lin, D., Wang, D.-W., Li, B., Lv, W., et al. (2019). Evolution of the electrochemical interface in sodium ion batteries with ether electrolytes. Nat. Commun. 10, 725. doi:10.1038/s41467-019-08506-5
Li, Y., Lu, Y., Zhao, C., Hu, Y.-S., Titirici, M.-M., Li, H., et al. (2017). Recent advances of electrode materials for low-cost sodium-ion batteries towards practical application for grid energy storage. Energy Storage Mater. 7, 130–151. doi:10.1016/j.ensm.2017.01.002
Li, Y., Yang, Y., Lu, Y., Zhou, Q., Qi, X., Meng, Q., et al. (2020). Ultralow-concentration electrolyte for Na-ion batteries. Acs Energy Lett. 5, 1156–1158. doi:10.1021/acsenergylett.0c00337
Li, Y., Zhong, X., Wu, X., Li, M., Zhang, W., and Wang, D. (2021b). Bi/C nanosheet microspheres with an open pore structure as anodes for sodium ion batteries with high capacity, excellent rate performance and long cycle life. J. Mater. Chem. A 9, 22364–22372. doi:10.1039/d1ta06948a
Li, Z., Tian, Z., Zhang, C., Wang, F., Ye, C., Han, F., et al. (2021c). An AlCl3 coordinating interlayer spacing in microcrystalline graphite facilitates ultra-stable and high-performance sodium storage. Nanoscale 13, 10468–10477. doi:10.1039/d1nr01660a
Lin, Z., Xia, Q., Wang, W., Li, W., and Chou, S. (2019). Recent research progresses in ether- and ester-based electrolytes for sodium-ion batteries. Infomat 1, 376–389. doi:10.1002/inf2.12023
Liu, J., Wang, S., Kravchyk, K., Ibanez, M., Krumeich, F., Widmer, R., et al. (2018a). SnP nanocrystals as anode materials for Na-ion batteries. J. Mater. Chem. A 6, 10958–10966. doi:10.1039/c8ta01492b
Liu, S., Shao, L., Zhang, X., Tao, Z., and Chen, J. (2018b). Knockdown of long noncoding RNA (lncRNA) metastasis-associated lung adenocarcinoma transcript 1 (MALAT1) inhibits proliferation, migration, and invasion and promotes apoptosis by targeting miR-124 in retinoblastoma. Acta Physico-Chimica Sin. 34, 581–591. doi:10.3727/096504017X14953948675403
Liu, X., Jiang, X., Zeng, Z., Ai, X., Yang, H., Zhong, F., et al. (2018c). High capacity and cycle-stable hard carbon anode for nonflammable sodium-ion batteries. Acs Appl. Mater. Interfaces 10, 38141–38150. doi:10.1021/acsami.8b16129
Liu, X., Jiang, X., Zhong, F., Feng, X., Chen, W., Ai, X., et al. (2019). High-safety symmetric sodium-ion batteries based on nonflammable phosphate electrolyte and double Na3V2(PO4)(3) electrodes. Acs Appl. Mater. Interfaces 11, 27833–27838. doi:10.1021/acsami.9b07614
Lu, Y.-X., Zhao, C.-L., Rong, X.-H., Chen, L.-Q., and Hu, Y.-S. (2018). Research progress of materials and devices for room-temperature Na-ion batteries. Acta Phys. Sin. 67, 120601. doi:10.7498/aps.67.20180847
Lu, Y., Wang, L., Cheng, J., and Goodenough, J. B. (2012). Prussian blue: A new framework of electrode materials for sodium batteries. Chem. Commun. 48, 6544–6546. doi:10.1039/c2cc31777j
Luis Gomez-Camer, J., Acebedo, B., Ortiz-Vitoriano, N., Monterrubio, I., Galceran, M., and Rojo, T. (2019). Unravelling the impact of electrolyte nature on Sn4P3/C negative electrodes for Na-ion batteries. J. Mater. Chem. A 7, 18434–18441. doi:10.1039/c9ta04288a
Luo, X.-F., Yang, C.-H., and Chang, J.-K. (2015). Correlations between electrochemical Na+ storage properties and physiochemical characteristics of holey graphene nanosheets. J. Mater. Chem. A 3, 17282–17289. doi:10.1039/c5ta03687a
Ma, M., Cai, H., Xu, C., Huang, R., Wang, S., Pan, H., et al. (2021). Engineering solid electrolyte interface at nano-scale for high-performance hard carbon in sodium-ion batteries. Adv. Funct. Mater. 31, 2100278. doi:10.1002/adfm.202100278
Maibach, J., Jeschull, F., Brandell, D., Edstrom, K., and Valvo, M. (2017). Surface layer evolution on graphite during electrochemical sodium-tetraglyme Co-intercalation. Acs Appl. Mater. Interfaces 9, 12373–12381. doi:10.1021/acsami.6b16536
Manohar, C. V., Forsyth, M., Macfarlane, D. R., and Mitra, S. (2018a). Role of N-propyl-N-methyl pyrrolidinium bis(trifluoromethanesulfonyl)imide as an electrolyte additive in sodium battery electrochemistry. Energy Technol. 6, 2232–2237. doi:10.1002/ente.201800123
Manohar, C. V., Raj, A. K., Kar, M., Forsyth, M., Macfarlane, D. R., and Mitra, S. (2018b). Stability enhancing ionic liquid hybrid electrolyte for NVP@C cathode based sodium batteries. Sustain. Energy & Fuels 2, 566–576. doi:10.1039/c7se00537g
Minh Phuong, D., Bucher, N., Nagasubramanian, A., Markovits, I., Tian, B., Fischer, P. J., et al. (2019). Effect of conducting salts in ionic liquid electrolytes for enhanced cyclability of sodium-ion batteries. Acs Appl. Mater. Interfaces 11, 23972–23981. doi:10.1021/acsami.9b03279
Mogensen, R., Maibach, J., Brant, W. R., Brandell, D., and Younesi, R. (2017). Evolution of the solid electrolyte interphase on tin phosphide anodes in sodium ion batteries probed by hard x-ray photoelectron spectroscopy. Electrochimica Acta 245, 696–704. doi:10.1016/j.electacta.2017.05.173
Mogensen, R., Maibach, J., Naylor, A. J., and Younesi, R. (2018). Capacity fading mechanism of tin phosphide anodes in sodium-ion batteries. Dalton Trans. 47, 10752–10758. doi:10.1039/c8dt01068d
Monti, D., Jónsson, E., Palacín, M. R., and Johansson, P. (2014). Ionic liquid based electrolytes for sodium-ion batteries: Na+ solvation and ionic conductivity. J. Power Sources 245, 630–636. doi:10.1016/j.jpowsour.2013.06.153
Monti, D., Ponrouch, A., Rosa Palacin, M., and Johansson, P. (2016). Towards safer sodium-ion batteries via organic solvent/ionic liquid based hybrid electrolytes. J. Power Sources 324, 712–721. doi:10.1016/j.jpowsour.2016.06.003
Nacimiento, F., Cabello, M., Ortiz, G. F., Alcantara, R., Lavela, P., and Tirado, J. L. (2019). Morphological adaptability of graphitic carbon nanofibers to enhance sodium insertion in a diglyme-based electrolyte. Dalton Trans. 48, 5417–5424. doi:10.1039/c9dt00563c
Niu, Y. B., Yin, Y. X., and Guo, Y. G. (2019). Nonaqueous sodium-ion full cells: Status, strategies, and prospects. Small 15, e1900233. doi:10.1002/smll.201900233
Otaegui, L., Goikolea, E., Aguesse, F., Armand, M., Rojo, T., and Singh, G. (2015). Effect of the electrolytic solvent and temperature on aluminium current collector stability: A case of sodium-ion battery cathode. J. Power Sources 297, 168–173. doi:10.1016/j.jpowsour.2015.07.084
Pan, H., Hu, Y.-S., and Chen, L. (2013). Room-temperature stationary sodium-ion batteries for large-scale electric energy storage. Energy & Environ. Sci. 6, 2338–2360. doi:10.1039/c3ee40847g
Pan, K., Lu, H., Zhong, F., Ai, X., Yang, H., and Cao, Y. (2018). Understanding the electrochemical compatibility and reaction mechanism on Na metal and hard carbon anodes of PC-based electrolytes for sodium-ion batteries. Acs Appl. Mater. Interfaces 10, 39651–39660. doi:10.1021/acsami.8b13236
Patra, J., Huang, H.-T., Xue, W., Wang, C., Helal, A. S., Li, J., et al. (2019). Moderately concentrated electrolyte improves solid-electrolyte interphase and sodium storage performance of hard carbon. Energy Storage Mater. 16, 146–154. doi:10.1016/j.ensm.2018.04.022
Peled, E. (1979). The electrochemical-behavior of alkali and alkaline-earth metals in non-aqueous battery systems - the solid electrolyte interphase model. J. Electrochem. Soc. 126, 2047–2051. doi:10.1149/1.2128859
Peled, E., Golodnitsky, D., and Ardel, G. (1997). Advanced model for solid electrolyte interphase electrodes in liquid and polymer electrolytes. J. Electrochem. Soc. 144, L208–L210. doi:10.1149/1.1837858
Ponrouch, A., Dedryvere, R., Monti, D., Demet, A. E., Mba, J. M. A., Croguennec, L., et al. (2013). Towards high energy density sodium ion batteries through electrolyte optimization. Energy & Environ. Sci. 6, 2361–2369. doi:10.1039/c3ee41379a
Ponrouch, A., Marchante, E., Courty, M., Tarascon, J.-M., and Palacin, M. R. (2012). In search of an optimized electrolyte for Na-ion batteries. Energy & Environ. Sci. 5, 8572–8583. doi:10.1039/c2ee22258b
Ponrouch, A., Monti, D., Boschin, A., Steen, B., Johansson, P., and Palacin, M. R. (2015). Non-aqueous electrolytes for sodium-ion batteries. J. Mater. Chem. A 3, 22–42. doi:10.1039/c4ta04428b
Qian, J., Chen, Y., Wu, L., Cao, Y., Ai, X., and Yang, H. (2012). High capacity Na-storage and superior cyclability of nanocomposite Sb/C anode for Na-ion batteries. Chem. Commun. 48, 7070–7072. doi:10.1039/c2cc32730a
Qian, J., Wu, X., Cao, Y., Ai, X., and Yang, H. (2013). High capacity and rate capability of amorphous phosphorus for sodium ion batteries. Angew. Chem. 125, 4731–4734. doi:10.1002/ange.201209689
Rangom, Y., Gaddam, R. R., Duignan, T. T., and Zhao, X. S. (2019). Improvement of hard carbon electrode performance by manipulating SEI formation at high charging rates. Acs Appl. Mater. Interfaces 11, 34796–34804. doi:10.1021/acsami.9b07449
Risthaus, T., Zhou, D., Cao, X., He, X., Qiu, B., Wang, J., et al. (2018). A high-capacity P2 Na2/3Ni1/3Mn2/3O2 cathode material for sodium ion batteries with oxygen activity. J. Power Sources 395, 16–24. doi:10.1016/j.jpowsour.2018.05.026
Rong, X., Lu, Y., Qi, X., Zhou, Q., Kong, W., Tang, K., et al. (2020). Na-ion batteries: From fundamental research to engineering exploration. Energy Storage Sci. Technol. 9, 515–522. doi:10.19799/j.cnki.2095-4239.2020.0054
Sadan, M. K., Choi, S.-H., Kim, H. H., Kim, C., Cho, G.-B., Kim, K.-W., et al. (2018). Effect of sodium salts on the cycling performance of tin anode in sodium ion batteries. Ionics 24, 753–761. doi:10.1007/s11581-017-2243-2
Sarkar, S., Lefler, M. J., Vishnugopi, B. S., Nuwayhid, R. B., Love, C. T., Carter, R., et al. (2023). Fluorinated ethylene carbonate as additive to glyme electrolytes for robust sodium solid electrolyte interface. Cell Rep. Phys. Sci. 4, 101356. doi:10.1016/j.xcrp.2023.101356
Seh, Z. W., Sun, J., Sun, Y., and Cui, Y. (2015). A highly reversible room-temperature sodium metal anode. Acs Central Sci. 1, 449–455. doi:10.1021/acscentsci.5b00328
Seidl, L., Bucher, N., Chu, E., Hartung, S., Martens, S., Schneider, O., et al. (2017). Intercalation of solvated Na-ions into graphite. Energy & Environ. Sci. 10, 1631–1642. doi:10.1039/c7ee00546f
Shakourian-Fard, M., Kamath, G., Smith, K., Xiong, H., and Sankaranarayanan, S. K. R. S. (2015). Trends in Na-ion solvation with alkyl-carbonate electrolytes for sodium-ion batteries: Insights from first-principles calculations. J. Phys. Chem. C 119, 22747–22759. doi:10.1021/acs.jpcc.5b04706
Simone, V., Lecarme, L., Simonin, L., and Martinet, S. (2017). Identification and quantification of the main electrolyte decomposition by-product in Na-ion batteries through FEC: Towards an improvement of safety and lifetime. J. Electrochem. Soc. 164, A145–A150. doi:10.1149/2.0671702jes
Soto, F. A., Ma, Y., De La Hoz, J. M. M., Seminario, J. M., and Balbuena, P. B. (2015). Formation and growth mechanisms of solid-electrolyte interphase layers in rechargeable batteries. Chem. Mater. 27, 7990–8000. doi:10.1021/acs.chemmater.5b03358
Stigliano, P., Ferrara, C., Pianta, N., Gentile, A., Mezzomo, L., Lorenzi, R., et al. (2022). Physicochemical properties of Pyr(13)TFSI-NaTFSI electrolyte for sodium batteries. Electrochimica Acta 412, 140123. doi:10.1016/j.electacta.2022.140123
Strauss, S. H. (1993). The search for larger and more weakly coordinating anions. Chem. Rev. 93, 927–942. doi:10.1021/cr00019a005
Sun, J., O'dell, L. A., Armand, M., Howlett, P. C., and Forsyth, M. (2021). Anion-derived solid-electrolyte interphase enables long life Na-ion batteries using superconcentrated ionic liquid electrolytes. Acs Energy Lett. 6, 2481–2490. doi:10.1021/acsenergylett.1c00816
Sun, Z., Fu, W., Liu, M. Z., Lu, P., Zhao, E., Magasinski, A., et al. (2020). A nanoconfined iron(iii) fluoride cathode in a NaDFOB electrolyte: Towards high-performance sodium-ion batteries. J. Mater. Chem. A 8, 4091–4098. doi:10.1039/c9ta12853k
Takada, K., Yamada, Y., Watanabe, E., Wang, J., Sodeyama, K., Tateyama, Y., et al. (2017). Unusual passivation ability of superconcentrated electrolytes toward hard carbon negative electrodes in sodium-ion batteries. Acs Appl. Mater. Interfaces 9, 33802–33809. doi:10.1021/acsami.7b08414
Takenaka, N., and Nagaoka, M. (2019). Microscopic elucidation of solid-electrolyte interphase (SEI) film formation via atomistic reaction simulations: Importance of functional groups of electrolyte and intact additive molecules. Chem. Rec. 19, 799–810. doi:10.1002/tcr.201800137
Takenaka, N., Sakai, H., Suzuki, Y., Uppula, P., and Nagaoka, M. (2015). A computational chemical insight into microscopic additive effect on solid electrolyte interphase film formation in sodium-ion batteries: Suppression of unstable film growth by intact fluoroethylene carbonate. J. Phys. Chem. C 119, 18046–18055. doi:10.1021/acs.jpcc.5b04206
Tang, Y., Zhang, W., Xue, L., Ding, X., Wang, T., Liu, X., et al. (2016). Polypyrrole-promoted superior cyclability and rate capability of NaxFe Fe(CN)(6) cathodes for sodium-ion batteries. J. Mater. Chem. A 4, 6036–6041. doi:10.1039/c6ta00876c
Tao, H., Zhou, M., Wang, R., Wang, K., Cheng, S., and Jiang, K. (2018). TiS2 as an advanced conversion electrode for sodium-ion batteries with ultra-high capacity and long-cycle life. Adv. Sci. 5, 1801021. doi:10.1002/advs.201801021
Vignarooban, K., Kushagra, R., Elango, A., Badami, P., Mellander, B. E., Xu, X., et al. (2016). Current trends and future challenges of electrolytes for sodium-ion batteries. Int. J. Hydrogen Energy 41, 2829–2846. doi:10.1016/j.ijhydene.2015.12.090
Wen, Z., Cao, J., Gu, Z., Xu, X., Zhang, F., and Lin, Z. (2008). Research on sodium sulfur battery for energy storage. Solid State Ionics 179, 1697–1701. doi:10.1016/j.ssi.2008.01.070
Wahlers, J., Fulfer, K. D., Harding, D. P., Kuroda, D. G., Kumar, R., and Jorn, R. (2016). Solvation structure and concentration in glyme-based sodium electrolytes: A combined spectroscopic and computational study. J. Phys. Chem. C 120, 17949–17959. doi:10.1021/acs.jpcc.6b06160
Wang, C., Wang, L., Li, F., Cheng, F., and Chen, J. (2017a). Bulk bismuth as a high-capacity and ultralong cycle-life anode for sodium-ion batteries by coupling with glyme-based electrolytes. Adv. Mater. 29, 1702212. doi:10.1002/adma.201702212
Wang, E., Niu, Y., Yin, Y.-X., and Guo, Y.-G. (2020). Manipulating electrode/electrolyte interphases of sodium-ion batteries: Strategies and perspectives. ACS Mater. Lett. 3, 18–41. doi:10.1021/acsmaterialslett.0c00356
Wang, J., Lu, H., Zhang, J., and Li, S. (2023). Improved interfacial property of Na3V2(PO4)(3)@C cathode: Application of NaODFB-based ether electrolyte in sodium-ion batteries. J. Electrochem. Energy Convers. Storage 20. doi:10.1115/1.4054389
Wang, M., Wang, Q., Ding, X., Wang, Y., Xin, Y., Singh, P., et al. (2022a). The prospect and challenges of sodium-ion batteries for low-temperature conditions. Interdiscip. Mater. 1, 373–395. doi:10.1002/idm2.12040
Wang, Q., Gao, H., Li, J., Liu, G.-B., and Jin, H. (2021a). Importance of crystallographic sites on sodium-ion extraction from NASICON-structured cathodes for sodium-ion batteries. Acs Appl. Mater. Interfaces 13, 14312–14320. doi:10.1021/acsami.1c01663
Wang, Q., Li, J., Jin, H., Xin, S., and Gao, H. (2022b). Prussian-blue materials: Revealing new opportunities for rechargeable batteries. Infomat 4. doi:10.1002/inf2.12311
Wang, S.-W., Hu, H.-F., Wang, D.-Y., and Shen, C. (2017b). AFM investigation of solid electrolyte interphase on Hopg anode in sodium ion battery. J. Inorg. Mater. 32, 596–602. doi:10.15541/jim20160472
Wang, S., Li, C., Fan, X., Wen, S., Lu, H., Dong, H., et al. (2021b). Selection of sodium salt electrolyte compatible with Na0.67Ni0.15Fe0.2Mn0.65O2 cathode for sodium-ion batteries. Energy Technol. 9, 2100190. doi:10.1002/ente.202100190
Wang, Y.-X., Chou, S.-L., Liu, H.-K., and Dou, S.-X. (2013). Reduced graphene oxide with superior cycling stability and rate capability for sodium storage. Carbon 57, 202–208. doi:10.1016/j.carbon.2013.01.064
Wang, Y.-X., Lim, Y.-G., Park, M.-S., Chou, S.-L., Kim, J. H., Liu, H.-K., et al. (2014). Ultrafine SnO 2 nanoparticle loading onto reduced graphene oxide as anodes for sodium-ion batteries with superior rate and cycling performances. J. Mater. Chem. A 2, 529–534. doi:10.1039/c3ta13592f
Wang, Y., Jiang, R., Liu, Y., Zheng, H., Fang, W., Liang, X., et al. (2021c). Enhanced sodium metal/electrolyte interface by a localized high-concentration electrolyte for sodium metal batteries: First-principles calculations and experimental studies. Acs Appl. Energy Mater. 4, 7376–7384. doi:10.1021/acsaem.1c01573
Wen, S., Li, X., Zhang, J., Wang, J., Ding, H., Zhang, N., et al. (2023). Effects of sodium salts on compatibility between Na2Ti3O7@C anode and electrolyte for sodium-ion batteries. J. Alloys Compd. 930, 167380. doi:10.1016/j.jallcom.2022.167380
Wongittharom, N., Lee, T.-C., Wang, C.-H., Wang, Y.-C., and Chang, J.-K. (2014). Electrochemical performance of Na/NaFePO4 sodium-ion batteries with ionic liquid electrolytes. J. Mater. Chem. A 2, 5655–5661. doi:10.1039/c3ta15273a
Wu, F., Zhu, N., Bai, Y., Li, Y., Wang, Z., Ni, Q., et al. (2018). Unveil the mechanism of solid electrolyte interphase on Na3V2(PO4)(3) formed by a novel NaPF6/BMITFSI ionic liquid electrolyte. Nano Energy 51, 524–532. doi:10.1016/j.nanoen.2018.07.003
Wu, L., Buchholz, D., Bresser, D., Chagas, L. G., and Passerini, S. (2014). Anatase TiO2 nanoparticles for high power sodium-ion anodes. J. Power Sources 251, 379–385. doi:10.1016/j.jpowsour.2013.11.083
Xia, X., Obrovac, M. N., and Dahn, J. R. (2011). Comparison of the reactivity of NaxC6 and LixC6 with non-aqueous solvents and electrolytes. Electrochem. Solid State Lett. 14, A130–A133. doi:10.1149/1.3606364
Xing, L., Borodin, O., Smith, G. D., and Li, W. (2011). Density functional theory study of the role of anions on the oxidative decomposition reaction of propylene carbonate. J. Phys. Chem. A 115, 13896–13905. doi:10.1021/jp206153n
Xing, L., Zheng, X., Schroeder, M., Alvarado, J., Cresce, A. V. W., Xu, K., et al. (2018). Deciphering the ethylene carbonate-propylene carbonate mystery in Li-ion batteries. Accounts Chem. Res. 51, 282–289. doi:10.1021/acs.accounts.7b00474
Xu, K. (2014). Electrolytes and interphases in Li-ion batteries and beyond. Chem. Rev. 114, 11503–11618. doi:10.1021/cr500003w
Xu, K. (2004). Nonaqueous liquid electrolytes for lithium-based rechargeable batteries. Chem. Rev. 104, 4303–4417. doi:10.1021/cr030203g
Yabuuchi, N., Kubota, K., Dahbi, M., and Komaba, S. (2014). Research development on sodium-ion batteries. Chem. Rev. 114, 11636–11682. doi:10.1021/cr500192f
Yamada, Y., Wang, J., Ko, S., Watanabe, E., and Yamada, A. (2019). Advances and issues in developing salt-concentrated battery electrolytes. Nat. Energy 4, 269–280. doi:10.1038/s41560-019-0336-z
Yamada, Y., and Yamada, A. (2015). Review-superconcentrated electrolytes for lithium batteries. J. Electrochem. Soc. 162, A2406–A2423. doi:10.1149/2.0041514jes
Yamada, Y., and Yamada, A. (2017). Superconcentrated electrolytes to create new interfacial chemistry in non-aqueous and aqueous rechargeable batteries. Chem. Lett. 46, 1056–1064. doi:10.1246/cl.170284
Yan, C., Xu, R., Xiao, Y., Ding, J.-F., Xu, L., Li, B.-Q., et al. (2020). Toward critical electrode/electrolyte interfaces in rechargeable batteries. Adv. Funct. Mater. 30, 1909887. doi:10.1002/adfm.201909887
Yan, G., Reeves, K., Foix, D., Li, Z., Cometto, C., Mariyappan, S., et al. (2019). A new electrolyte formulation for securing high temperature cycling and storage performances of Na-ion batteries. Adv. Energy Mater. 9, 1901431. doi:10.1002/aenm.201901431
Yang, H., Hwang, J., Tonouchi, Y., Matsumoto, K., and Hagiwara, R. (2021). Sodium difluorophosphate: Facile synthesis, structure, and electrochemical behavior as an additive for sodium-ion batteries. J. Mater. Chem. A 9, 3637–3647. doi:10.1039/d0ta11689k
Yang, Z., Zhang, J., Kintner-Meyer, M. C. W., Lu, X., Choi, D., Lemmon, J. P., et al. (2011). Electrochemical energy storage for green grid. Chem. Rev. 111, 3577–3613. doi:10.1021/cr100290v
Ye, M., You, S., Xiong, J., Yang, Y., Zhang, Y., and Li, C. C. (2022). In-situ construction of a NaF-rich cathode-electrolyte interface on Prussian blue toward a 3000-cycle-life sodium-ion battery. Mater. Today Energy 23, 100898. doi:10.1016/j.mtener.2021.100898
You, Y., and Manthiram, A. (2018). Progress in high-voltage cathode materials for rechargeable sodium-ion batteries. Adv. Energy Mater. 8, 1701785. doi:10.1002/aenm.201701785
Zarrabeitia, M., Casas-Cabanas, M., and Munoz-Marquez, M. A. (2021). Understanding the electrode - electrolyte interphase of high voltage positive electrode Na4Co3(PO4)(2)P2O7 for rechargeable sodium-ion batteries. Electrochimica Acta 372, 137846. doi:10.1016/j.electacta.2021.137846
Zeng, G., Liu, Y., Gu, C., Zhang, K., An, Y., Wei, C., et al. (2020). A nonflammable fluorinated carbonate electrolyte for sodium-ion batteries. Acta Physico-Chimica Sin. 36. doi:10.3866/PKU.WHXB201905006
Zeng, Z., Murugesan, V., Han, K. S., Jiang, X., Cao, Y., Xiao, L., et al. (2018). Non-flammable electrolytes with high salt-to-solvent ratios for Li-ion and Li-metal batteries. Nat. Energy 3, 674–681. doi:10.1038/s41560-018-0196-y
Zhang, J., Wang, D.-W., Lv, W., Zhang, S., Liang, Q., Zheng, D., et al. (2017). Achieving superb sodium storage performance on carbon anodes through an ether-derived solid electrolyte interphase. Energy & Environ. Sci. 10, 370–376. doi:10.1039/c6ee03367a
Zhang, L. L., and Zhao, X. S. (2009). Carbon-based materials as supercapacitor electrodes. Chem. Soc. Rev. 38, 2520–2531. doi:10.1039/b813846j
Zhang, S. S. (2006). A review on electrolyte additives for lithium-ion batteries. J. Power Sources 162, 1379–1394. doi:10.1016/j.jpowsour.2006.07.074
Zhang, Z., Li, Y., Xu, R., Zhou, W., Li, Y., Oyakhire, S. T., et al. (2022). Capturing the swelling of solid-electrolyte interphase in lithium metal batteries. Science 375, 66–70. doi:10.1126/science.abi8703
Zhao, D., Lu, H., Li, S., Wang, P., and Fan, X. (2022). Boosting the cycling stability of hard carbon with NaODFB-based electrolyte at high temperature. Mater. Today Chem. 24, 100866. doi:10.1016/j.mtchem.2022.100866
Zheng, C., Chen, Y., Xu, X., Lin, Q., Wang, H., Xue, Q., et al. (2022). Diglyme-based electrolytes boosting high-rate and stable sodium-ion storage for three-dimensional VS4/Reduced graphene oxide hybrid anodes. J. Power Sources 526, 231098. doi:10.1016/j.jpowsour.2022.231098
Zheng, J., Chen, S., Zhao, W., Song, J., Engelhard, M. H., and Zhang, J.-G. (2018). Extremely stable sodium metal batteries enabled by localized high-concentration electrolytes. Acs Energy Lett. 3, 315–321. doi:10.1021/acsenergylett.7b01213
Zheng, X., Gu, Z., Liu, X., Wang, Z., Wen, J., Wu, X., et al. (2020). Bridging the immiscibility of an all-fluoride fire extinguishant with highly-fluorinated electrolytes toward safe sodium metal batteries. Energy & Environ. Sci. 13, 1788–1798. doi:10.1039/d0ee00694g
Zhou, Q., Xingguo, Q., Lu, Y., Rong, X., Tang, F., Kong, W., et al. (2020). The necessity of establishing Na-ion battery standards. Energy Storage Sci. Technol. 9, 1225–1233. doi:10.19799/j.cnki.2095-4239.2020.0085
Zhu, N., Wu, F., Wu, C., Bai, Y., and Li, Y. (2016). Recent Adv. electrolytes sodium-ion Batter. Energy Storage Sci. Technol. 5, 285–291. doi:10.3969/j.issn.2095-4239.2016.03.004
Keywords: sodium ion battery, organic liquid electrolyte, cathode, anode, solid electrolyte interphase (SEI)
Citation: Zhang J, Li J, Wang H and Wang M (2023) Research progress of organic liquid electrolyte for sodium ion battery. Front. Chem. 11:1253959. doi: 10.3389/fchem.2023.1253959
Received: 06 July 2023; Accepted: 23 August 2023;
Published: 12 September 2023.
Edited by:
Gioele Pagot, University of Padua, ItalyReviewed by:
Stephen Percival, Sandia National Laboratories (DOE), United StatesCopyright © 2023 Zhang, Li, Wang and Wang. This is an open-access article distributed under the terms of the Creative Commons Attribution License (CC BY). The use, distribution or reproduction in other forums is permitted, provided the original author(s) and the copyright owner(s) are credited and that the original publication in this journal is cited, in accordance with accepted academic practice. No use, distribution or reproduction is permitted which does not comply with these terms.
*Correspondence: Huaiyou Wang, d2h5QGlzbC5hYy5jbg==; Min Wang, d2FuZ21pbkBpc2wuYWMuY24=
Disclaimer: All claims expressed in this article are solely those of the authors and do not necessarily represent those of their affiliated organizations, or those of the publisher, the editors and the reviewers. Any product that may be evaluated in this article or claim that may be made by its manufacturer is not guaranteed or endorsed by the publisher.
Research integrity at Frontiers
Learn more about the work of our research integrity team to safeguard the quality of each article we publish.