- 1Department of Otolaryngology, Medical College of the University of Rzeszów, Rzeszów, Poland
- 2Department of Biochemistry and General Chemistry, Medical College of the University of Rzeszów, Rzeszów, Poland
- 3Students English Division Science Club, Medical College of the University of Rzeszów, Rzeszów, Poland
- 4Center for Innovative Research in Medical and Natural Sciences, Medical College of the University of Rzeszów, Rzeszów, Poland
- 5Department of Photomedicine and Physical Chemistry, Medical College of the University of Rzeszów, Rzeszów, Poland
Cancer is a main cause of death and preferred methods of therapy depend on the type of tumor and its location. Gliomas are the most common primary intracranial tumor, accounting for 81% of malignant brain tumors. Although relatively rare, they cause significant mortality. Traditional methods include surgery, radiotherapy and chemotherapy; they also have significant associated side effects that cause difficulties related to tumor excision and recurrence. Photodynamic therapy has potentially fewer side effects, less toxicity, and is a more selective treatment, and is thus attracting increasing interest as an advanced therapeutic strategy. Photodynamic treatment of malignant glioma is considered to be a promising additional therapeutic option that is currently being extensively investigated in vitro and in vivo. This review describes the application of photodynamic therapy for treatment of brain cancer. The mechanism of photodynamic action is also described in this work as it applies to treatment of brain cancers such as glioblastoma multiforme. The pros and cons of photodynamic therapy for brain cancer are also discussed.
1 Introduction
1.1 Brain cancer
Malignant gliomas are the second most common cause of cancer deaths among people under 35 years of age (Almammadov et al., 2022). Brain tumors can be divided into primary tumors, i.e., those that arise directly from the brain tissue, and metastatic tumors, which develop in other organs and spread to the brain (Kim and Lee, 2022). There are over 120 types of brain tumors, and approximately 45% of primary brain tumors are gliomas, the most common and aggressive of which is glioblastoma multiforme (GBM) (Kharroubi Lakouas et al., 2017). The classification system developed by the World Health Organization (WHO) is based on the histological features of brain tissues observed under a microscope and divides brain tumors into malignant and benign tumors (Kim and Lee, 2022). The treatment of brain tumors still poses many challenges, and one of the main limitations is the problem of drug delivery to the brain due to the presence of the selective blood-brain barrier formed by endothelial cells of the cerebral vessels which regulates the transport of nutrients and ions protecting the brain from neurotoxic molecules. For this reason, most drugs cannot cross the blood-brain barrier by physiological routes (Kim and Lee, 2022). One of the most commonly used methods of glioblastoma therapy is chemotherapy, which is characterized by high cytotoxicity, affecting both tumor cells and healthy cells of the body. Chemotherapy does not have the ability to distinguish cancer cells from healthy cells (Ladomersky et al., 2019; Meng et al., 2022). A common phenomenon in the treatment of brain gliomas is their multidrug resistance (Meng et al., 2022). The mainstay of treatment of high-grade brain tumors is still surgical resection in combination with radiotherapy and chemotherapy. The invasive growth pattern, especially in certain areas of the brain, often makes it difficult to completely remove the tumor and it is important to realize that not all of the tumor tissue will be resected. This forces us to search for new methods of brain tumor therapy, which will be characterized by both safety for the patient and high efficiency (Quirk et al., 2015). The healing potential of light was noticed in antiquity, when the treatment of various ailments, ranging from mood disorders to musculoskeletal disorders and skin diseases, was treated by exposing the patient to sunlight.
1.2 Photodynamic therapy (PDT)- mechanism
Photodynamic therapy (PDT) was first discovered over a century ago by medical student Oscar Raab, working with Professor Hermann von Tappeiner, who was the first to use the term “photodynamic therapy” (Niculescu and Grumezscu, 2021). Photodynamic therapy was originally developed as a treatment target for dermatological diseases. Skin lesions are easily exposed to light and are easily monitored by visual assessment. Thanks to the successes in the treatment of skin diseases, the so-called interstitial photodynamic therapy (iPDT), a promising treatment for deep tumors using optical fibers inserted in situ where targeting strategies aim to improve the delivery of photosensitizers (PS) to tumor tissues to simultaneously increases the selectivity and effectiveness of PDT are being sought. Photodynamic therapy is currently a method of treating various disease cases (Gunaydin et al., 2021). The main principle of operation is based on the localization of the PS molecule within cancer cells (Oniszczuk et al., 2016). The first stage is the excitation of the inflammatory/neoplastic area with light of a specific wavelength in the presence of oxygen and the PS (Baskaran et al., 2018; Kwiatkowski et al., 2018). Reactive products such as singlet oxygen and hydroxyl radical are generated at the light-exposed site (dos Santos et al., 2019). These free radicals interact with molecules of lipids, peptides, proteins and nucleic acids (Mansoori et al., 2019). They are able to directly kill cancer cells by apoptosis and necrosis, damage tumor vascular structures leading to hypoxia, and induce immune responses (Gunaydin et al., 2021). The group of reactive PDT products includes oxygen free radicals that are formed as a result of electron transfer (e.g., superoxide anion O2•−, hydroxyl radical HO•, hydroperoxyl radical HOO•), and as a result of energy transfer, singlet oxygen is obtained. Apart from light and oxygen, PS are an important component of photodynamic therapy. Figure 1 shows mechanism of PDT in brain cancer.
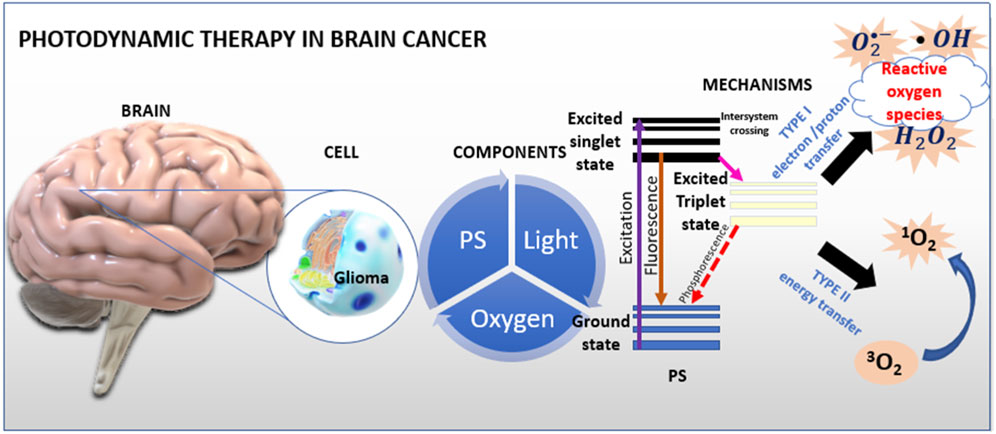
FIGURE 1. Mechanism of PDT. Photosensitizers accumulate in diseased cells and then are irradiated with laser light or visible light During light absorption, the accumulated PS molecules in the cells pass from the singlet ground state to the excited singlet state. Much of the energy is lost through direct photon emission in the excited state through fluorescence. A small part of the energy is used in the process of intersystem crossing to the excited triplet state. The active form of the PS in the triplet state, reacts with oxygen causing the formation of active oxygen species, i.e., singlet oxygen. These molecules induce apoptosis - programmed cell death. Figure 1 shows a schematic of photodynamic action. Interpretations of the entire procedure can be reduced (simplified) to the statement that PDT is the conversion of light energy into toxic, reactive oxygen species.
Photodynamic therapy involves administering PS that accumulates in the patient’s tissues. A PS is a non-toxic substance that has no negative effects on health in its inactive state. However, the PS exposed to irradiation becomes a source of free oxygen radicals that destroy surrounding cells. This means that the drug will act locally only in the area where it was activated by light, sparing healthy tissue. The described therapy model is already used in the case of numerous diseases, e.g., dermatological diseases or cancers and pre-cancerous lesions of the esophagus, and recently also in the treatment of brain tumors. Singlet oxygen is extremely reactive and has the ability to damage cellular proteins as well as DNA itself (Kostron, 2010; Agnez-Lima et al., 2012; Kwiatkowski et al., 2018; Chizenga et al., 2019; Fujii et al., 2023; Murotomi et al., 2023).
The most common features of a good PS are high selectivity towards the tissue we want to work on and high cytotoxicity in the presence of light (Dolmans, et al., 2003). Research is continuing on the search for new, more organ-specific PS. However, before they are introduced into practice, they must undergo a series of clinical trials (Dougherty and Marcus, 1992; Farokhzad and Langer, 2009). The type and concentration of the PS used, as well as the wavelength of the light source used, affect the extent and type of damage caused by PDT (Agostinis et al., 2011). In terms of superficial lesions on the mucous membranes and skin, the effectiveness of PDT has been clinically demonstrated and therefore it has already been introduced in the clinic (Lamberti et al., 2014; Bacellar et al., 2015; Baptista et al., 2017). Unfortunately, lesions located deeper in the tissues are still a problem because access to them is difficult, and the light also has a limited penetration depth (Zhao et al., 2018; Sun et al., 2019). The search for new, more tissue-specific PS is constantly ongoing. Research is also conducted on the modification of already available photosensitizing substances in order to minimize their effect on specific organs and eliminate the potentially harmful effect of PDT on healthy tissues (Thomas et al., 2017).
Photodynamic therapy is considered a less invasive therapy targeting all cells that contain the PS itself, while preserving adjacent normal tissues (Quirk et al., 2015; Akimoto, 2016; Leroy et al., 2021; Bartusik-Aebisher et al., 2022). Due to the nature and type of products generated during PDT, it has cytotoxic properties (Santos et al., 2020). Due to the extraordinary therapeutic properties of the method, this review discusses the mechanism of phototherapy, the application of the presented method (mainly in diseases of the nervous system and brain), and determining its advantages and disadvantages, both in preclinical and clinical trials (Agostinis, et al., 2011).
2 Methodology
In this paper, we reviewed the current preclinical and clinical studies showing the use of PDT in the treatment of brain tumors. Articles for individual sections have been collected from the following scientific databases: PubMed, ScienceDirect, Web of Science and Google Scholar. The main part presents an overview of in vitro and in vivo studies of PDT in brain tumors. Research papers, review papers and short essays from inception to 2023 were qualified for the review. The qualification scheme of individual articles is presented in Figure 2.
Additionally, the PICO criteria used for the selection of literature are presented in Table 1. The PICO criteria were: population, intervention, comparison and outcome of the research.
3 Application of photodynamic therapy in the treatment of brain tumors
3.1 Applied photosensitizers (PS)
The term “photosensitizer” means a substance that sensitizes the test site to light. There are many different PS known in medicine, which differ primarily in the extent of laser light absorption. In medicine, PS that cause more apoptosis and less inflammation are extremely desirable (Agostinis, et al., 2011). The key function of PDT is the ability to influence the immune system. The inflammatory response and necrotic cell death is one of the effects of PDT that characterizes the effectiveness of the therapy.
There are many different PS currently being tested and used in clinical trials (including in the treatment of brain tumors) (Mahmoudi et al., 2019). The PS is characterized by such properties as: toxicity, time and effectiveness of concentration in the tissue, the range of light absorption and the depth of tissue penetration (Correia et al., 2021). At the moment, there are no PS that meet all aspects, mainly in the treatment of brain diseases (Quirk et al., 2015).
Photosensitizers have been divided into three generations; the former consists of naturally occurring porphyrins, including hematoporphyrin. This group of molecules is characterized by strong absorption at a wavelength of about 400 nm. The second generation of PS includes compounds such as sodium thalaporfin and temoporfin, and 5-aminolevulinic acid (5-ALA), activated by wavelengths greater than 600 nm (Cramer and Chen, 2020). Third-generation PS are components of second-generation PS that include a nanoelement (Niculescu and Grumezescu, 2021). The first clinically approved PS was porfimer sodium, sold under the trade name Photofrin®. It is used in the treatment of non-small cell lung cancer, bladder cancer, esophageal cancer and brain cancer. Despite their wide application in medicine, first-generation PS are characterized by a number of unfavorable features; they have a low chemical purity and can be activated at wavelengths below 640 nm. Another disadvantage is their long half-life, which makes the skin hypersensitive to light. Patients treated with these PS are required to stay in a dark room for up to 6 weeks (Niculescu and Grumezescu, 2021). The absorption spectrum of PS belonging to the second generation is in the range of 650–800 nm, which allows for better absorption of light in deep tissue. Thanks to faster elimination from the body, they are characterized by fewer side effects and a shorter period of photosensitivity. The disadvantage of these compounds is their hydrophobicity, which is the reason for the aggregation of second-generation PS under physiological conditions, which in turn is the reason for the reduction of ROS production efficiency. The hydrophobic nature of these compounds also limits their intravenous administration, which forces the search for new methods of drug delivery (Kharroubi Lakouas et al., 2017; Park et al., 2018)]. Third generation photosensitizers are more stable and hydrophilic. They have favorable pharmacodynamic and pharmacokinetic parameters and are characterized by better biodistribution in vivo although as yet, no third generation PS is approved for clinical use. They have fewer side effects and less toxicity (Guo, et al., 2015).
The characteristics of the most popular photosensitizers used in PDT of brain tumors are presented below.
3.1.1 Porfimer sodium, photofrin, HPD
The first PS used clinically for the treatment of cancer was a hematoporphyrin derivative, now known as Photofrin® (Agostinis et al., 2011). This hematoporphyrin derivative has been used for PDT in oncology for the treatment of thousands of patients around the world for 40 years. Various forms are currently available on the market (Allison and Sibata, 2010). Sodium porfimer has an absorbance in the range of 630 nm [14]. Jiang studied the effect of PDT in combination with Photofrin® on human glioblastoma cell lines. The light source was a laser with a wavelength of 632 nm and the photosensitizer Photofrin® was applied in different doses. The aim of the study was to determine the degree of migration of glioblastoma cells post-PDT. The results of the experiment showed that PDT with Photofrin® inhibited the spread of cells lines U87 and U25ln. The authors concluded that PDT in combination with Photofrin® neutralizes and blocks the development and dynamics of glioblastoma cells (Jiang et al., 2002). In another study by Jiang et al., PDT was used in combination with liposome encapsulated Photofrin® in a human glioblastoma cell line injected into nude rats. The results of the experiment confirmed that PDT with the application of Photofrin® in a liposome led to greater tumor destruction. The conclusions of the study were as follows: PDT in combination with Photofrin® can aid in the treatment of brain tumors in humans (Jiang F et al., 1998)]. In turn, deCarvalho et al., applied PDT with Photofrin® to glioblastoma cells. In this experiment, the applied therapy changed the homeostasis of the tumor, becoming a suitable model to control the effect of PDT in the treatment of glioblastoma (deCarvalho et al., 2007). Photofrin® was also used in research by Lee et al. In their experiments, the authors studied the mechanisms of the cellular response in glioblastoma cells (Lee et al., 2010). In preclinical studies, a group of dogs was also noted as a study group in PDT. Whelan et al., performed PDT on a group of dogs with and without brain tumor disease (control group). Photofrin® was administered intravenously and exposed to light. The study showed that low doses of the photosensitizer initiated an apoptotic tumor response without significant persistent toxicity (Whelan et al., 1993). Figure 3 shows the structure of porfimer sodium.
3.1.2 5-aminolevulinic acid, 5-ALA
5-aminolevulinic acid (5-ALA) is a biosynthetic precursor of protoporphyrin IX and is a second generation PS. ALA in structure and physiology is analogous to amino acids. It is rapidly absorbed and then converted to heme in normal cells through a metabolic pathway. It is assumed that in various cancer cells, exogenous application of larger amounts of ALA increases the cellular level of PpIX, which results in greater accumulation of PpIX in cancer cells than in normal cells. One of the better examples of an article describing the process of transformation of 5-ALA into PpIX is the work by Kennedy and Pottier (Kennedy and Pottier, 1992). According to the authors and their review, after the application of 5-ALA there is increased activity of the bile ducts. Transport of the compound into the intestines induces dissemination to most organs and tissues. In turn, administration of 5-ALA to animals (mice) induces PpIX fluorescence in the parotid glands. Under constant and normal conditions, the process of heme synthesis is dependent on the process of its removal from the body. The liver, as the main site of heme synthesis, works on the principle of feedback. In this process, the presence of free heme inhibits the synthesis of 5-ALA. The dynamic and efficient course of heme removal and synthesis causes a reduction in the amount of free heme in the liver, so that the synthesis of 5-ALA will not be inhibited, on the contrary, the amount and concentration will increase. The increase in 5-ALA concentration contributes to the increase in heme synthesis. Therefore, under physiologically constant and normal conditions, the demand for heme controls the rate of heme synthesis and therefore the rate of PpIX synthesis. Currently, there are many steps that induce the synthesis of 5-ALA and heme. As previously mentioned, the main site of synthesis is the liver, but the feedback mechanism is also present in other tissues. Uroporphyrins I and III and coproporphyrin I and III are strong tissue sensitizing substances. They come from uroporphyrinogen and coproporphyrin from coproporphyrinogen, i.e., from the heme biosynthesis pathway, which leads to the accumulation of porphyrins. In the metabolic pathway of 5-ALA synthesis, each of the intermediates has a sufficient power reserve, without accumulation. This does not happen when the concentration is not controlled and the amount of exogenous 5-ALA is high. The rate of synthesis of the first intermediate in the metabolic pathway (porphobilinogen) depends primarily on the maximum efficiency of the enzyme system responsible for this particular step. Further stages are controlled and conditioned by the other processes involved. The authors report that if porphobilinogen is synthesized at a rate that exceeds the maximum capacity of the next step in the pathway (in this case, uroporphyrinogen synthesis), then porphobilinogen will accumulate. On the other hand, however, if the mechanism responsible for the synthesis of uroporphyrinogen still has some reserves even though porphobilinogen is produced at the maximum possible rate, then the rate of uroporphyrinogen synthesis will be limited by the rate of porphobilinogen synthesis. The rule of this principle is applicable to each subsequent step of the biosynthetic metabolic pathway. If the first step of decarboxylation of uroporphyrinogen is slower than the synthesis of uroporphyrinogen, then the accumulation of uroporphyrinogen and, subsequently, uroporphyrin will occur. If the conversion of PpIX to heme is slower than the rate of PpIX synthesis, then PpIX will accumulate. It is worth noting that although the maximum capacity to synthesize PpIX from protoporphyrinogen may greatly exceed the maximum capacity of the next iron-dependent step in which PpIX is converted to heme, PpIX will not accumulate if the even slower process is located anywhere upstream of PpIX in the heme biosynthetic pathway. The presence or absence of such a rate-limiting step may explain why only certain cell types accumulate PpIX when exposed to high concentrations of exogenous ALA.
ALA is analogous to amino acids in terms of structure and physiology. It is rapidly absorbed and then metabolically converted to heme in normal cells. It is assumed that in various cancer cells, exogenous administration of larger amounts of ALA increases the cellular level of PpIX, which results in greater accumulation of PpIX in cancer cells than in normal cells. It is worth noting, however, that the mechanism of accumulation and synthesis of PpIX from ALA is still not fully understood.
The maximum absorbance of this PS occurs at 630 nm (Agostinis et al., 2011). Currently, 5-ALA is one of the most commonly used photosensitizers in PDT. According to Mahmoudi et al., the application of 5-ALA in PDT improves tumor visibility through a specific metabolic process (Mahmoudi et al., 2019). Also, Lietke et al. applied 5-ALA to interstitial PDT (iPDT). The generated immune response and the production of reactive oxygen species resulted in a local cure of the disease. Interstitial photodynamic therapy is an innovative therapeutic approach consisting of direct introduction of light to the source of the tumor. 5-ALA is very often used in this method. At the moment, the use of 5-ALA is safe and effective in the treatment of brain tumors (Lietke et al., 2021). 5-ALA causes tumor cells to have a higher accumulation of protoporphyrin IX. According to Stepp and Stummer, the combination of 5-ALA with PDT is an innovative and non-toxic method of treatment (Stepp and Stummer, 2018). Due to its safety and negligible number of side effects, 5-ALA is also used in pediatric patients. In vitro studies conducted by Schwake et al. demonstrated that it is safe enough to be used as an agent to reduce the number of glioblastoma cells in children (Schwake et al., 2018). Omura et al., evaluated the efficacy of PDT in combination with 5-ALA on a human glioblastoma cell line. After the therapy, the effectiveness of the therapy was noticeable in all cell lines. The cell lines showed a lower tendency to tumorigenesis (Omura et al., 2023). Figure 4 shows the structure of 5-aminolevulinic acid.
3.1.3 Temoporfin (Foscan), mTHPC
Chlorins belong to the group of tetrapyrrole macrocycles. These photosensitizers are considered one of the most effective photosensitizers used in PDT. They absorb light in the range from 650 to 750 nm. They also have high quantum efficiency and generate large amounts of ROS, including singlet oxygen (Mata et al., 2023).
Temoporfin (Foscan®) is commercially available and is one of the strongest PSs and is most efficient at an absorption of 652 nm (Allison and Sibata, 2010). Temoporfin is one of the stronger photosensitizers used in PDT. Yakavets et al. reviewed the efficacy of PDT with temoporfin. The review confirmed that nanoplatforms used in conjunction temoporfin contribute to an increase in the effectiveness and penetration of treatment during PDT. This fact is an extremely promising treatment opportunity compared to conventional treatments (Yakavets et al., 2019). One of the challenges of the continued use of temoporfin in PDT in the treatment of brain disorders is relatively low water solubility. These limitations suggest that in some studies the production of reactive oxygen species is not sufficient (Marconi et al., 2023). There is as yet scant research describing the use of temoporfins in the treatment of brain diseases. Laboratory tests are still needed to analyze the effect of this photosensitizer on brain cancer cells. An example of such a study is the work of researchers Bœuf-Muraille et al. In this study, a temoporfin-containing complex applied to a murine glioblastoma cell line was analyzed. The encapsulated photosensitizer had a higher toxicity compared to the uncomplexed photosensitizer. In their conclusions, the authors showed that the main pathway of cancer cell destruction was apoptosis (Bœuf-Muraille et al., 2019). Another problem or challenge in the use of temoporfins in clinical trials is the problem of patient photosensitivity after therapy. One solution is to develop a method of delivering the photosensitizer inside the tumor. Mannino et al. used rats previously implanted with a tumor in their study. The authors confirmed that the application of complexed photosensitizer is more effective, because the time to reach the optimal concentration is shorter compared to the systemic application (Mannino et al., 2008). In a study by Vanacloch, et al., temoporfin was used as a second-generation photosensitizer after Photofrin®. During therapy with temoporfin, a laser with a wavelength of 652 nm was used. The photosensitizer itself was administered intravenously. Compared to the previous photosensitizer, PDT with temoporfin penetrated deeper into the brain tissue and the washout time from the body was shorter (Vanaclocha et al., 2015). Figure 5 shows the structure of Temoporfin.
3.1.4 Other chlorins
Chlorin e6 is also one of the strongest photosensitizers with low toxicity and quick dissolution and clearance from the body. According to the literature, this photosensitizer also has strong fluorescent properties. Due to the presented advantages, chlorin e6 is one of the most effective photosensitizers used in PDT (Shrestha et al., 2022).
PDT combined with chlorin e6 has antibacterial, anti-inflammatory and antimicrobial properties (Hur et al., 2022). Due to the fact that it belongs to the chlorine group of photosensitizers, it has no dark toxicity and is quickly removed from the body (Thapa Magar et al., 2023).
One of the disadvantages of chlorin e6 is its hydrophobicity, which correlates with poor biodistribution, consequently leading to the rapid removal of the photosensitizer from the body’s circulatory system (Hak et al., 2023).
According to Tereshkin et al., chlorin e6 also has other limitations, e.g., chlorin e6 is characterized by poor solubility in water, which leads to ineffective pharmacokinetics. Additionally, its effectiveness is lower compared to photosensitizers of the same generation. Moreover, this photosensitizer has a limited penetration depth (Tereshkina et al., 2022).
Chlorin e6 is the basic ingredient of talaporfin. Like other photosensitizers, chlorin e6 is used in the treatment of brain tumors. Teng et al., constructed cyanine-chlorine nanoclusters for the treatment of glioblastoma multiforme (Teng et al., 2020). They used a cell line that was administered an amount of photosensitizer and exposed to laser light. The study also included orthotopic models. The study showed that the concept of treating malignant gliomas, including fluorescence-guided resection with PDT adjuvant, is possible and feasible using the theragnostic nanocluster component.
3.1.5 Talaporfin (LS11, MACE, NPe6)
Talaporfin is chlorin derivative and has many names including MACE, NPe6, LS11. The light absorption range of talaporfin is in the range of 664 nm. It is worth noting that the accumulation time of PS in the tissue is about 4 h (Allison and Sibata, 2010). Muragaki, et al., in their study used talaporfin sodium for PDT in patients with malignant parenchymal changes in brain tumors. The research group consisted of 22 patients with a diagnosed and confirmed disease. According to the authors, the intraoperative form of PDT may prove to be an effective alternative therapeutic method. In addition, at the moment it turns out to be safe. The use of this type of therapy in the near future may significantly affect the therapy of patients with brain tumors (Muragaki et al., 2013). Another example is a study was that conducted by Namatame et al. Their experiment used a rat model in which glioblastoma cells were injected into the frontal lobe. A diode laser with a wavelength of 664 nm was used as the light source. The results of the experiment were as follows: the authors observed necrosis and a decrease in the migration of tumor cells. A few hours after treatment, increased activity and expression of cells responsible for cell death were noted. The applied therapy was the cause of the progressive apoptosis process in the glioblastoma cells (Namatame et al., 2008). Similar conclusions were presented in work by Akimoto (Akimoto et al., 2012), who stated that PDT in combination with talaporfin is a milestone and at the same time a breakthrough in the therapy of glioblastoma. Due to the selectivity of the therapy, it is a promising form of treating cancer cells in brain diseases (Akimoto, 2016). Three years later, Akimoto revisited PDT in combination with talaporfin in a clinical trial. The research group consisted of 47 patients. The results of the experiments also confirmed that talaporfin is an effective and useful photosensitizer (Akimoto et al., 2019). Miki et al., in turn, developed the NPe6-PDT nanocomplex in order to assess the effectiveness of the therapy. In vitro studies were performed on glioblastoma cell lines. Analysis of mitochondrial cell death pathways and cellular responses showed that the cell death observed was a result of the therapy. Cell morphology showed necrosis, which was a function of exposure time and dose (Miki et al., 2013). Figure 6 shows the structure of Talaporfin
3.1.6 Hypericin, HYP
One of the strongest natural photosensitizers used in photodynamic therapy is hypericin (HYP). It is commonly found in plants called St. John’s wort. Hypericin is a potential clinical anti-cancer agent as many studies have shown its strong anti-cancer effects. Its effectiveness depends on the type, concentration and dose of light applied (Agostinis, et al., 2002). Many studies have confirmed that HYP selectively accumulates in the neoplastic tissue of tumors. From scientific reports it can be concluded that chemotherapeutics significantly improves the already relatively high effectiveness of hypericin-supported PDT (Dong et al., 2021). Hypericin is the natural active ingredient of Hypericum perforatum (St. John’s wort). Hypericin has additional pharmaceutical and medical applications as it exhibits antidepressant, anticancer and antiviral effects (Liu et al., 2020). Hypericum perforatum is also a popular herbal remedy recommended for the treatment of depression. Due to the progressive rate of utilization of St. John’s wort on a global scale, it is one of the conventional antidepressants (Ng et al., 2017). Thanks to its active ingredients, hypericin is used in the treatment of mental and neurodegenerative disorders (Zirak, et al., 2019). Hypericin contains anthraquinone derivatives (hypericin and pseudohypericin), flavonoids, prenylated floroglucinols (hyperforin), tannins, phenolic acids and volatile oils. It has calming and astringent properties. It is used for excitability, neuralgia, anxiety and as a nerve tonic. St. John’s wort has a long history of traditional use in topical preparations for wound healing. It is now used in homeopathic products as well as in herbal products (Zirak, et al., 2019).
Hypericin, a natural small molecule extracted from H. perforatum, has been studied as a photosensitizer for photodynamic therapy and as an effective agent for necrosis targeted imaging and tumor therapy. Although the molecular targeting sites of hypericin are still under investigation, applications of hypericin for necrosis contrast agent development and necrosis targeted radiotherapy have increasingly gained attention. The development of an effective and efficient hypericin application model with a high safety standard is a challenge for the coming years (Rizzo et al., 2019; Han et al., 2020). In cases of brain tumors, hypericin has been used, among others, by Misuth et al., (Misuth et al., 2017). Rottlerin was also used in this study to test their synergistic effect. Cells from the U-87 MG line were treated in different ways: only hypericin, only rottlerin, or a combination thereof. The results showed that cells after exposure to laser light and after application of hypericin or its combination with rottlerin significantly reduced the metabolic activity of the cells’ mitochondria. In turn, irradiation of cells treated only with rottlerin did not reduce their mitochondrial metabolic activity. Additionally, after PDT, cells treated with 0.5 µM hypericin, 2 µM rottlerin and their combination significantly reduced the population of living cells.
Figure 7 shows the structure of Hypericin.
3.1.7 Phthalocyanines
Phthalocyanines are another group of photosensitizers used in PDT. These are aromatic, heterocyclic compounds. Its structure has 4 aromatic rings. They absorb light between 650 and 850 nm. Research has been conducted for several years on the use of phthalocyanine photosensitizers in PDT. Currently, they are being tested to increase selectivity, improve the process of accumulation in cancer cells, and in the development of new synergistic therapeutic strategies to increase the effectiveness of PDT.
Phthalocyanines undergo many types of chemical transformations, such as: coordination, polymerization, aggregation, oxidation and reduction reactions; they take an active part in the processes of catalysis, and photochemical transformations (Li et al., 2023).
Phthalocyanines have relatively low toxicity in the dark. However, after exposure to laser light, they become extremely photobiologically active (de Siqueira et al., 2022). Phthalocyanines, as a group of second-generation photosensitizers, have enhanced photochemical and photophysical properties compared to first-generation photosensitizers. The most important features characterizing phthalocyanines are their unique spectroscopic, luminescent (fluorescence and phosphorescence), magnetic (para- and diamagnetism) properties, as well as their thermal stability, photoconductivity, photoemission and surface activity. Phthalocyanines are very reactive compounds that undergo various types of reactions - coordination, polymerization, aggregation, formation of acids, bases and salts, redox reactions, catalysis, sorption and photochemical reactions (Santos et al., 2020). It turns out, for example, that phthalocyanine photosensitizers have a greater tendency to create radical species, while porphyrin photosensitizers tend to generate singlet oxygen.
Phthalocyanines have lipophilic elements in their structure, which makes their application in clinical trials difficult. To overcome this limitation, scientists are designing systems and platforms that facilitate their delivery (Miretti et al., 2021).
Conjugation of phthalocyanines with diamagnetic elements such as zinc, aluminum and silicon seem to improve the efficiency of singlet oxygen generation. Phthalocyanines combined with zinc are characterized by low toxicity in dark conditions. They are also characterized by a high therapeutic effect and low sensitivity of the skin and other organs. The disadvantage of this type of photosensitizer is poor water solubility. In turn, third-generation zinc (II) phthalocyanines, i.e., the so-called a second-generation photosensitizer, have been designed in combination with a carrier. Currently, photosensitizers with a carrier based on liposome, polymer micelles and nanoparticles are proposed in the literature. The use of this type of carrier improves the bioavailability of the applied photosensitizers and their transport to target sites. Roguin et al. attempted to analyze various liposomes and polymer micelles as carriers for photosensitizers based on zinc (II) phthalocyanines. Their research confirmed that in combination with carriers based on both liposome and polymer micelles, photosensitizers were more soluble. In the case of photodynamic activity, micelles were characterized by a higher activity coefficient towards cancer cells (Roguin et al., 2019).
In the treatment of brain tumors, phthalocyanines have been used, among others: by Castilho-Fernandes et al. (Castilho-Fernandes et al., 2017). The authors conducted an in vitro study using the U-87 MG line. The experiment used chloroaluminum phthalocyanine encapsulated in a new drug delivery system and designed as a nanoemulsion. After applying the nanomulsion to the cells and after exposure to laser light, the changes were observed under a microscope. The results were as follows: cells irradiated with 70 mJ/cm2 energy showed 56% ± 13% cell death, while doses of 140 and 250 mJ/cm2 caused 34% ± 15% and 33% ± 4% cell death, respectively, compared to untreated cells and control cells. So the dose of laser light was not directly proportional to the number of lethal cells. After PDT, glioma cells were clearly smaller and shrunken compared to cells from the control group. The results indicate that the proposed treatment model is effective and induces apoptosis. Figure 8 shows the structure of Phthalocyanines.
In turn Table 2 summarizes information regarding individual photosensitizers.
3.2 Clinical and preclinical studies of PDT in brain tumors–a review
Currently, PDT is undergoing intensive clinical trials (Kostron, 2010). The beginnings of PDT treatment of patients with brain cancer dates back to the 1980s. One of the first cases in which PDT was administered was in treatment of glioblastoma multiforme. During the procedure, resection of the glioblastoma cavity was performed. The photosensitizers used were porfimer sodium, 5-ALA and temoporfin. The light sources used were: dye lasers, diode lasers and lamps. The results of the therapy confirmed the hypotheses that PDT may be helpful in the treatment of brain tumors. Currently, PDT is being used in combination with other compatible therapies. The methods of adjuvant treatment include intraoperative diagnostics by means of photodetection (PD) and fluorescence-guided resection (FGR) (Stylli et al., 2005). An example of the application of PDT in the treatment of glioblastoma was the research conducted by Muller and Wilson. The authors presented the results of research conducted in Toronto, Canada. The study group consisted of 96 patients with supratentorial glioma who underwent PDT treatment with porfimer sodium (Muller and Wilson, 2006). During the research, the authors were aware that there was little clinical or even preclinical data related to the treatment of brain tumors with PDT. The aspect of tumor infiltration into adjacent tissues is extremely important. Achievements in the field of focused radiation are crucial in implementing further clinical trials (as the authors emphasize). This aspect is extremely important in the context of further clinical trials.
In turn, Stummer et al. (Stummer et al., 2006) presented the results of research conducted in Germany. The research group consisted of 322 patients diagnosed with malignant glioma. The photosensitizer used was 5-ALA. The aim of the study was to evaluate the effect of surgical resection with 5-ALA application. The results showed that during resection with the application of 5-ALA, the tumor was completely removed in 65% of patients. However, without the application of 5-ALA (only white light) the tumor was completely removed in only 36% of patients. It is worth noting that the authors were aware that maximum cytoreductive treatment of malignant gliomas is beneficial for patients, but the issue is still the subject of numerous discussions. The authors report that neurosurgeons are increasingly attempting to remove tumors under contrast enhancement. However, it is not 100% effective and such a procedure is only achieved with a small number of patients. To enhance this effect, intraoperative MRI, neuronavigation or ultrasound are often used.
An aim of the research conducted by Eljamel et al. was characterization of PDT in the treatment of glioblastoma in comparison with fluorescence-guided resection. The article clearly shows that both applied methods significantly prolonged patient’s lives (Eljamel et al., 2008). The main challenge of this study was the fluorescence of the photosensitizer Photofrin, because it is very similar in color to the background. The combination application of photosensitizers, i.e., ALA-induced PpIX and Photofrin®, eliminated this limitation. Potential side effects of the test include a temporary increase in liver enzyme levels. In the study by (Eljamel et al., 2008), these enzymes were not monitored and no clinically significant abnormalities in liver or kidney function were observed. Nevertheless, as the authors encourage, the presentation of the study is an encouragement to implement further test research protocols that have improved the therapeutic procedure.
In a study by Kostron and colleagues, 26 patients diagnosed with recurrent glioblastoma multiforme were enrolled in a PDT study with Foscan®. Before treatment, all tumors progressed and standard therapeutic options (irradiation, chemotherapy) were exhausted. After aggressive fluorescence-guided resection, intraoperative PDT was performed. Compared to matched controls, significantly better survival was demonstrated in the treatment group (Kostron et al., 2006; Kaneko et al., 2018). According to the authors, several specific side effects were observed in patients, such as increased skin sensitivity and swelling. In the context of further research, certain schemes can be developed that do not eliminate the presented side effects.
In a study by Han-Wen Guo et al., PDT was applied to a mouse model of human glioblastoma using an organic light-emitting diode and a single dose of 5-aminolevulinic acid (ALA) as a photosensitizer. Tumor volume was measured by bioluminescence imaging and the survival time of the animals was recorded. It was shown that in animals with a similar tumor volume before and immediately after irradiation, the average overall survival time of mice after PDT was significantly longer than in control mice (Guo et al., 2015). Testing metronomic PDT in small animals raises several problematic issues related to the maintenance and transportation of the lighting device. The authors used organic LEDs for this purpose. According to the authors, the study confirmed the feasibility of low fluence rate and long duration of ALA-PDT treatment using OLED without animal anesthesia. However, also taking into account such characteristics as dosimetric indicators, including photosensitizer fluorescence and the degree of tissue oxygenation, would enable understanding of certain therapeutic limitations and improve future treatment regimens.
In a 2013 study by Yoshihiro Muragaki et al., the potential efficacy and safety of iPDT with sodium talaporfin and semiconductor laser irradiation in patients with primary malignant parenchymal brain tumors was investigated. A single intravenous injection of talaporfin sodium was administered to 27 patients with suspected newly diagnosed or recurrent primary malignant parenchymal brain tumors 1 day before resection. Twenty-two patients with a histopathologically confirmed diagnosis of primary malignant parenchymal brain tumor were enrolled in the study. Intraoperative PDT with the use of sodium talaporfin and a semiconductor laser may be considered a potentially effective and sufficiently safe option for the adjuvant treatment of primary malignant parenchymal brain tumors (Muragaki et al., 2013).
One of the tests of newly developing therapies are preclinical studies. The conducted studies enable the selection of appropriate therapeutic regimens including light dose. According to the literature, longer exposure of laser light to lesions may increase the effectiveness of PDT in the brain in combination with other supportive therapies, such as LED light delivery (Kostron, 2010).
Preclinical studies also include in vitro studies on cell lines. An example is research on glioblastoma cell lines that were treated with PDT using HYP. Studies have confirmed that PDT with HYP was highly effective in reducing the number of glioblastoma cells and had an inhibitory effect on cell growth and reproduction. The cited studies indicate that hypericin-assisted PDT may become an effective method of treatment in various oncological diseases (Dong et al., 2021).
Despite the many advantages of PDT and its high efficiency in the treatment of brain tumors, there are still doubts about its use as a standard adjuvant therapy. The effects of PDT in both clinical and preclinical trials are satisfactory, however, additional studies targeting brain tumors are still needed to help take into account the pharmacokinetic aspects (Kim and Lee, 2022). Although there have been numerous studies demonstrating the use of PDT in the treatment of brain tumors (including malignant tumors), most of them are in the early stages. In order to effectively assess PDT, it is necessary to introduce certain criteria, such as: tumor subtype, supportive techniques, light sources or type of photosensitizer. Currently, it is difficult to obtain a general understanding of the current state of knowledge and assess the direction in which the treatment of malignant brain tumors with PTD is heading (Quirk et al., 2015).
The clinical studies described previously were described in publications of reputable journals. However, based on the publicly available clinical trials database ClinicalTrials.gov, nine clinical trials were registered in which patients with brain tumors were subjected to photodynamic therapy. The clinical studies cited are summarized in table 3 presenting their detailed characteristics. The number of registered clinical trials on the treatment of brain tumors with PDT is not large. This demonstrates the complexity of such tests, potential complications and long time durations. Table 3 presents the characteristics of 9 clinical trials officially registered in the ClinicalTrials.gov database. The search words were: condition: brain tumor, intervention: photodynamic therapy.
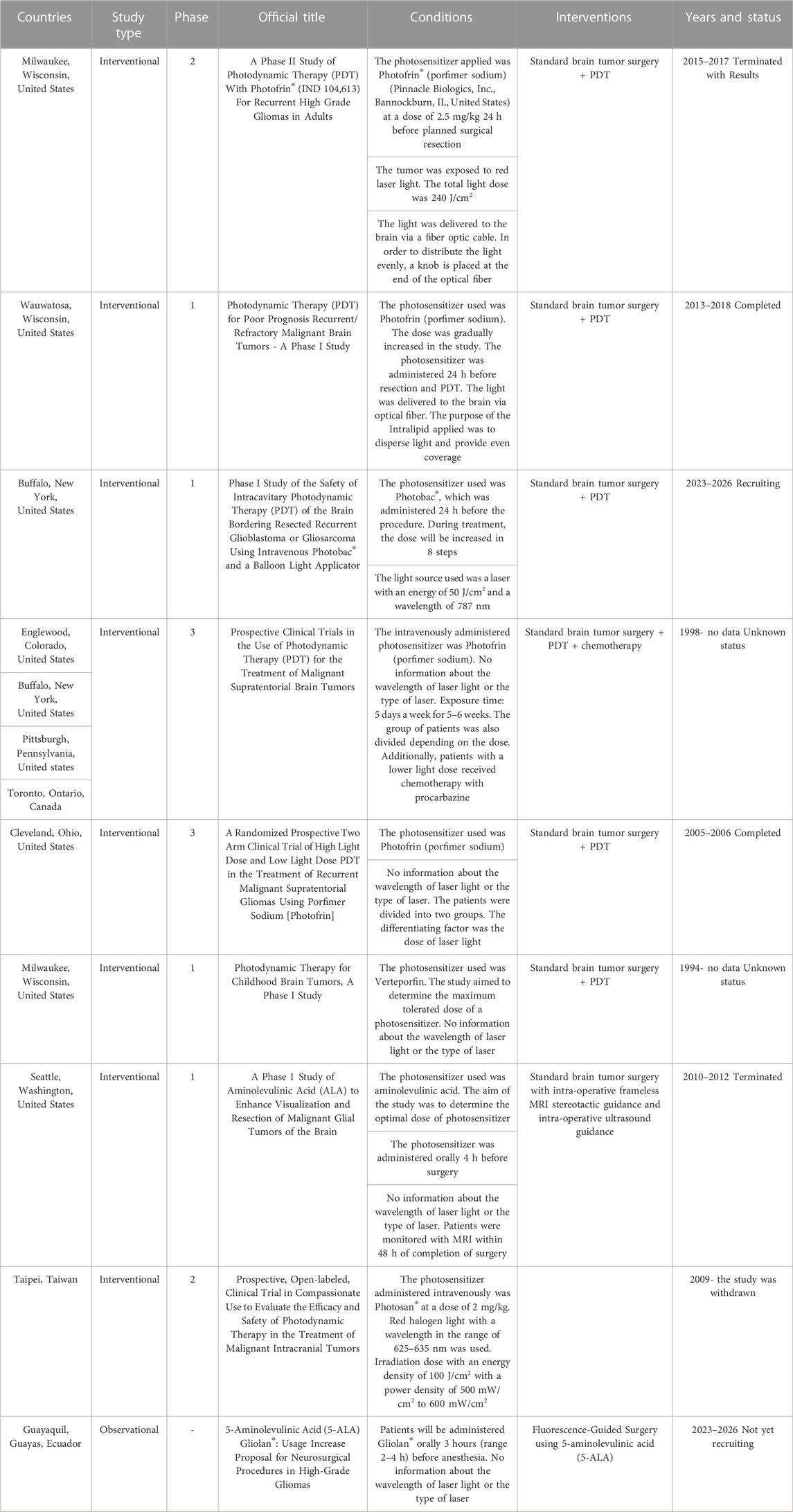
TABLE 3. Registered clinical trials in the database ClinicalTrials.gov.
4 Therapeutic challenges in PDT
4.1 Light sources
The main challenge in PDT is how to apply the light dose so that it is applied evenly and at the right depth to the treated area. In addition, the form of application of the photosensitizer and the method and time of accumulation in tissues are a challenge. Therefore, light and photosensitizer dosages are extremely complex and are key factors that determine the success of treatment (Quirk et al., 2015). The first sources of laser light were argon and xenon lasers (Quirk et al., 2015). Currently, laser and non-laser sources are applied. The group of laser light sources includes argon lasers, dye lasers, solid-state lasers, optical parametric oscillators and diode lasers. The group of non-laser light sources includes halogen, xenon, metal halide, sodium, fluorescent lamps as well as light-emitting diodes and femtosecond solid-state lasers (Gunaydin, et al., 2021). Choosing the right light source and the way it is applied is one of the challenges in PDT. Depending on the applied photosensitizer, red or blue light i useds, which have different penetration depths (Gunaydin, Gedik, Ayan, 2021). Currently, in therapy, light sources with a longer wavelength and greater penetration are sought, which can be used to treat internal organs. Light with absorption wavelengths between 600 and 800 nm are most often used (Cramer and Chen, 2020; Gunaydin, Gedik, Ayan, 2021). Photosensitizers also require the presence of appropriate oxygen species to be active (Gunaydin et al., 2021).
4.2 Hypoxic environment
One of the challenges of modern medicine is to increase the effectiveness of cancer treatment, which is one of the main causes of morbidity and mortality in people around the world. Despite the enormous amount of work of scientists, overcoming cancer still seems distant. The obstacle is not only the lack of effective compounds, but even those that show promising anticancer properties encounter additional difficulties that are not always taken into account in model studies and quite rarely in preclinical studies. One such aspect that may be responsible for the reduced effectiveness of PDT is the lack of oxygen in the tumor tissue, called hypoxia, which characterizes the microenvironment of solid and hematological tumors. Hypoxia has a double negative role. On the one hand, it may lead to the formation of a more aggressive phenotype of cancer cells, which are invasive and contribute to the formation of metastases. On the other hand, it reduces the effectiveness of oxygen-dependent chemotherapy and radiotherapy, as well as hinders the delivery of drugs to the tumor due to abnormal microcirculation. All these aspects encourage us to take a closer look at the phenomenon of hypoxia in cancer. To do this, you need, among others, markers that will identify areas of hypoxia. On the other hand, it is important that the effectiveness of the tested compounds as potential anticancer drugs is also checked under hypoxic conditions.
At the moment, there are few literature reports on how to deal with hypoxia in photodynamic therapy for the treatment of glioblastoma. The reason for this phenomenon may be the aspect of the complexity of this process, and its role and impact on PDT. Most of the publications describing hypoxia and its impact on the effectiveness of PDT are articles from 2021-2022, which confirms that this topic has been analyzed relatively recently. Additionally, research is still ongoing presenting the characteristics of this phenomenon in the context of PDT treatment and proposing solutions to limit its impact on the effectiveness of PDT.
In the field of hypoxia, research was also conducted by Ihata et al. In this experiment, they managed to confirm that the effectiveness of 5-ALA PDT under hypoxic conditions was not significantly reduced, even though the content of protoporphyrin produced was lower compared to standard PDT treatment conditions. The authors claim that oxygen concentrations below 5% initiate a series of cellular responses that induce hypoxia. According to the authors, the inclusion of various types of inhibitors in PDT will enhance the therapeutic effect of the destruction of brain tumor cells. Further studies (mainly in vitro) illustrating different variants of the tumor environment (with reduced or increased oxygen content) are necessary in order to select the most appropriate therapeutic method. Individual in vitro results do not make it possible to apply the presented innovative methods to clinical trials. Therefore, further research using cell lines and animal models testing ALA-PDT is needed (Ihata et al., 2022).
Albert et al., in an in vitro study, used two glioblastoma cell lines to analyze and characterize the effect of the level of oxygen partial pressure on the physiology of cancer cells and the effectiveness of PDT. The experiment showed that changing the partial pressure of oxygen from 19% to 9% did not change the intracellular accumulation of PpIX. However, reducing partial pressure changed the reactivity of glioma cells of 1 cell line. The therapeutic efficacy in the treatment of U-87 MG cells was not satisfactory at a pressure of 9%. The treatment of these cells required a pressure of 20% and a longer exposure time. This experiment illustrates that precise control of oxygen concentration is necessary, both in vivo and in vitro (Albert, Hefti, Luginbuehl, 2014).
Sunil et al., in order to reduce the impact of hypoxia proposed enhanced combination therapy using light-responsive oxygen generators that capture antigens. The designed model contained the Nutlin-3a molecule and the photosensitizer PpIX. Such a model can release oxygen when activated with laser light of a specific wavelength. The project assumes that generating oxygen on demand in hypoxic conditions leads to an increase in the tumor oxygenation level. By eliminating the phenomenon of hypoxia and stabilizing the level of partial pressure and oxygen concentration, the effectiveness of PDT may be higher (Sunil et al., 2021).
The ideas presented above to improve tumor oxygenation and thus improve the effectiveness of PDT are pioneering research. There is still a need to conduct research and thus implement further ideas that will be used in vivo research.
5 Conclusion
The science of photodynamic therapy has been on going for over 100 years, and its application in clinical practice began at least 35 years ago. Over the past 20 years, knowledge about the operation and use of PDT has greatly expanded. New proposals for photosensitizers, light sources and light delivery methods are some of the possibilities to improve this treatment technique. Molecular strategies based on nanotechnology are constantly being developed to increase the effectiveness and selectivity of PDT. Photodynamic therapy is a highly selective method of treating various malignant tumors of the skin and internal organs. It allows patients to avoid many adverse side effects associated with the use of such forms of treatment as radiotherapy or chemotherapy. Photodynamic therapy is characterized by a favorable profile of side effects, and the ability to enhance the anti-cancer immune response. At the moment, PDT has not yet reached its maximum potential due to the limitations of the classic photosensitizers used, including, i.e., light absorbance, penetration depth and cellular uptake. It is expected that intensive research on the use of photodynamic therapy in the treatment of cancer, including brain cancer, will soon make PDT one of the world standards of therapy in the treatment of these diseases.
Author contributions
WD: Conceptualization, Methodology, Validation, Formal Analysis, Resources, Writing–original draft, Writing–review and editing, Visualization. DB-A: Conceptualization, Methodology, Validation, Formal Analysis, Resources, Writing–original draft, Writing–review and editing, Visualization. IR: Methodology, Validation, Formal Analysis, Resources, Writing–original draft, Writing–review and editing, Visualization. KD: Methodology, Validation, Formal Analysis, Resources, Writing–original draft, Writing–review and editing, Visualization. KP: Methodology, Validation, Formal Analysis, Resources, Writing–original draft, Writing–review and editing, Visualization. DA: Conceptualization, Methodology, Validation, Formal Analysis, Resources, Writing–original draft, Writing–review and editing, Visualization, Supervision.
Funding
The author(s) declare that no financial support was received for the research, authorship, and/or publication of this article.
Conflict of interest
The authors declare that the research was conducted in the absence of any commercial or financial relationships that could be construed as a potential conflict of interest.
Publisher’s note
All claims expressed in this article are solely those of the authors and do not necessarily represent those of their affiliated organizations, or those of the publisher, the editors and the reviewers. Any product that may be evaluated in this article, or claim that may be made by its manufacturer, is not guaranteed or endorsed by the publisher.
References
Agnez-Lima, L. F., Melo, J. T., Silva, A. E., Oliveira, A. H., Timoteo, A. R., Lima-Bessa, K. M., et al. (2012). DNA damage by singlet oxygen and cellular protective mechanisms. Mutat. Res. Rev. Mutat. Res. 751 (1), 15–28. Epub 2012 Jan 14. PMID: 22266568. doi:10.1016/j.mrrev.2011.12.005
Agostinis, P., Berg, K., Cengel, K. A., Foster, T. H., Girotti, A. W., Gollnick, S. O., et al. (2011). Photodynamic therapy of cancer: an update. A Cancer J. Clin. 61 (4), 250–281. doi:10.3322/caac.20114
Agostinis, P., Vantieghem, A., Merlevede, W., and de Witte, P. A. (2002). Hypericin in cancer treatment: more light on the way. Int. J. Biochem. Cell Biol. 34 (3), 221–241. PMID: 11849990. doi:10.1016/s1357-2725(01)00126-1
Akimoto, J. (2016). Photodynamic therapy for malignant brain tumors. Neurol. Med. Chir. (Tokyo) 56 (4), 151–157. Epub 2016 Feb 16. PMID: 26888042; PMCID: PMC4831940. doi:10.2176/nmc.ra.2015-0296
Akimoto, J., Fukami, S., Ichikawa, M., Mohamed, A., and Kohno, M. (2019). Intraoperative photodiagnosis for malignant glioma using photosensitizer talaporfin sodium. Front. Surg. 6, 12. PMID: 30949484; PMCID: PMC6438081. doi:10.3389/fsurg.2019.00012
Akimoto, J., Haraoka, J., and Aizawa, K. (2012). Preliminary clinical report on safety and efficacy of photodynamic therapy using talaporfin sodium for malignant gliomas. Photodiagnosis Photodyn. Ther. 9 (2), 91–99. Epub 2012 Feb 28. PMID: 22594978. doi:10.1016/j.pdpdt.2012.01.001
Albert, I., Hefti, M., and Luginbuehl, V. (2014). Physiological oxygen concentration alters glioma cell malignancy and responsiveness to photodynamic therapy in vitro. Neurol. Res. 36 (11), 1001–1010. Epub 2014 Jun 13. PMID: 24923209. doi:10.1179/1743132814Y.0000000401
Allison, R. R., and Sibata, C. H. (2010). Oncologic photodynamic therapy photosensitizers: a clinical review. Photodiagnosis Photodyn. Ther. 7 (2), 61–75. Epub 2010 Apr 21. PMID: 20510301. doi:10.1016/j.pdpdt.2010.02.001
Almammadov, T., Elmazoglu, Z., Atakan, G., Kepil, D., Aykent, G., Kolemen, S., et al. (2022). Locked and loaded: β-galactosidase activated photodynamic therapy agent enables selective imaging and targeted treatment of glioblastoma multiforme cancer cells. ACS Appl. Bio Mater 5 (9), 4284–4293. Epub ahead of print. PMID: 36043987; PMCID: PMC9490748. doi:10.1021/acsabm.2c00484
Bacellar, I. O. L., Tsubone, T. M., Pavani, C., and Baptista, M. S. (2015). Photodynamic efficiency: from molecular photochemistry to cell death. ternational J. Mol. Sci. 16, 20523–20559. doi:10.3390/ijms160920523
Baptista, M. S., Cadet, J., Di-Mascio, P., Ghogare, A. A., Greer, A., Hamblin, M. R., et al. (2017). Type I and type II photosensitized oxidation reactions: guidelines and mechanistic pathways. Photochem. Photobiol. 93 (4), 912–919. doi:10.1111/php.12716
Bartusik-Aebisher, D., Żołyniak, A., Barnaś, E., Machorowska-Pieniążek, A., Oleś, P., Kawczyk-Krupka, A., et al. (2022). The use of photodynamic therapy in the treatment of brain tumors-A review of the literature. Molecules 27 (20), 6847. doi:10.3390/molecules27206847
Baskaran, R., Lee, J., and Yang, S. G. (2018). Clinical development of photodynamic agents and therapeutic applications. Biomater. Res. 22, 25. PMID: 30275968; PMCID: PMC6158913. doi:10.1186/s40824-018-0140-z
Bœuf-Muraille, G., Rigaux, G., Callewaert, M., Zambrano, N., Van Gulick, L., Roullin, V. G., et al. (2019). Evaluation of mTHPC-loaded PLGA nanoparticles for in vitro photodynamic therapy on C6 glioma cell line. Photodiagnosis Photodyn. Ther. 25, 448–455. Epub 2019 Jan 29. PMID: 30708089. doi:10.1016/j.pdpdt.2019.01.026
Castilho-Fernandes, A., Lopes, T. G., Primo, F. L., Pinto, M. R., and Tedesco, A. C. (2017). Photodynamic process induced by chloro-aluminum phthalocyanine nanoemulsion in glioblastoma. Photodiagnosis Photodyn. Ther. 19, 221–228. Epub 2017 Jul 4. PMID: 28599959. doi:10.1016/j.pdpdt.2017.05.003
Chizenga, E. P., Chandran, R., and Abrahamse, H. (2019). Photodynamic therapy of cervical cancer by eradication of cervical cancer cells and cervical cancer stem cells. Oncotarget 10 (43), 4380–4396. PMID: 31320992. doi:10.18632/oncotarget.27029
Correia, J. H., Rodrigues, J. A., Pimenta, S., Dong, T., and Yang, Z. (2021). Photodynamic therapy review: principles, photosensitizers, applications, and future directions. Pharmaceutics 13 (9), 1332. PMCID: PMC8470722. doi:10.3390/pharmaceutics13091332
Cramer, S. W., and Chen, C. C. (2020). Photodynamic therapy for the treatment of glioblastoma. Front. Surg. 6, 81. PMID: 32039232; PMCID: PMC6985206. doi:10.3389/fsurg.2019.00081
deCarvalho, A. C., Zhang, X., Roberts, C., Jiang, F., Kalkanis, S. N., Hong, X., et al. (2007). Subclinical photodynamic therapy treatment modifies the brain microenvironment and promotes glioma growth. Glia 55 (10), 1053–1060. doi:10.1002/glia.20525
de Siqueira, L. B. O., Dos Santos Matos, A. P., da Silva, M. R. M., Pinto, S. R., Santos-Oliveira, R., and Ricci-Júnior, E. (2022). Pharmaceutical nanotechnology applied to phthalocyanines for the promotion of antimicrobial photodynamic therapy: a literature review. Photodiagnosis Photodyn. Ther. 39, 102896. Epub 2022 May 4. PMID: 35525432. doi:10.1016/j.pdpdt.2022.102896
Dolmans, D. E., Fukumura, D., and Jain, R. K. (2003). Photodynamic therapy for cancer. Nat. Rev. Cancer 3 (5), 380–387. doi:10.1038/nrc1071
Dong, X., Zeng, Y., Zhang, Z., Fu, J., You, L., He, Y., et al. (2021). Hypericin-mediated photodynamic therapy for the treatment of cancer: a review. J. Pharm. Pharmacol. 73 (4), 425–436. doi:10.1093/jpp/rgaa018
dos Santos, A. F., Raquel Queiroz de Almeida, D., Ferreira Terra, L., and Maurício, S. (2019). Baptista, Leticia Labriola, Photodynamic therapy in cancer treatment - an update review. J. Cancer, Metastasis Treat. 5, 25. doi:10.20517/2394-4722.2018.83
Dougherty, T. J., and Marcus, S. L. (1992). Photodynamic therapy. Eur. J. Cancer. 28, 1734–1742. doi:10.1016/0959-8049(92)90080-l
Eljamel, M. S., Goodman, C., and Moseley, H. (2008). ALA and Photofrin fluorescence-guided resection and repetitive PDT in glioblastoma multiforme: a single centre Phase III randomised controlled trial. Lasers Med. Sci. 23 (4), 361–367. Epub 2007 Oct 10. PMID: 17926079. doi:10.1007/s10103-007-0494-2
Farokhzad, O. C., and Langer, R. (2009). Impact of nanotechnology on drug delivery. ACS Nano 3, 16–20. doi:10.1021/nn900002m
Fujii, J., Soma, Y., and Matsuda, Y. (2023). Biological action of singlet molecular oxygen from the standpoint of cell signaling, injury and death. Inj. Death. Mol. 28 (10), 4085. doi:10.3390/molecules28104085
Gunaydin, G., Gedik, M. E., and Ayan, S. (2021). Photodynamic therapy for the treatment and diagnosis of cancer-A review of the current clinical status. Front. Chem. 9, 686303. PMID: 34409014; PMCID: PMC8365093. doi:10.3389/fchem.2021.686303
Gunaydin, G., Gedik, M. E., and Ayan, S. (2021). Photodynamic therapy-current limitations and novel approaches. Front. Chem. 9, 691697. doi:10.3389/fchem.2021.691697
Guo, H. W., Lin, L. T., Chen, P. H., Ho, M. H., Huang, W. T., Lee, Y. J., et al. (2015). Low-fluence rate, long duration photodynamic therapy in glioma mouse model using organic light emitting diode (OLED). Photodiagnosis Photodyn. Ther. 12 (3), 504–510. Epub 2015 Apr 29. PMID: 25936596. doi:10.1016/j.pdpdt.2015.04.007
Hak, A., Ali, M. S., Sankaranarayanan, S. A., Shinde, V. R., and Rengan, A. K. (2023). Chlorin e6: a Promising Photosensitizer in Photo-Based Cancer Nanomedicine. ACS Appl. Bio Mater 6 (2), 349–364. Epub 2023 Jan 26. PMID: 36700563. doi:10.1021/acsabm.2c00891
Han, X., Taratula, O., Taratula, O., Xu, K., St Lorenz, A., Moses, A., et al. (2020). Biodegradable hypericin-containing nanoparticles for necrosis targeting and fluorescence imaging. Mol. Pharm. 17 (5), 1538–1545. Epub 2020 Apr 7. PMID: 32212709. doi:10.1021/acs.molpharmaceut.9b01238
Hur, G. H., Ryu, A. R., Kim, Y. W., and Lee, M. Y. (2022). The Potential Anti-Photoaging Effect of Photodynamic Therapy Using Chlorin e6-Curcumin Conjugate in UVB-Irradiated Fibroblasts and Hairless Mice. Pharmaceutics 14 (5), 968. doi:10.3390/pharmaceutics14050968
Ihata, T., Nonoguchi, N., Fujishiro, T., Omura, N., Kawabata, S., Kajimoto, Y., et al. (2022). The effect of hypoxia on photodynamic therapy with 5-aminolevulinic acid in malignant gliomas. Photodiagnosis Photodyn. Ther. 40, 103056. Epub 2022 Aug 6. PMID: 35944845. doi:10.1016/j.pdpdt.2022.103056
Jiang, F., Chopp, M., Katakowski, M., Cho, K. K., Yang, X., Hochbaum, N., et al. (2002). Photodynamic therapy with photofrin reduces invasiveness of malignant human glioma cells. Lasers Med. Sci. 17 (4), 280–288. doi:10.1007/s101030200041
Jiang, F., Lilge, L., Grenier, J., Li, Y., Wilson, M. D., and Chopp, M. (1998). Photodynamic therapy of U87 human glioma in nude rat using liposome-delivered photofrin. Lasers Surg. Med. 22 (2), 74–80. PMID: 9484699. doi:10.1002/(sici)1096-9101(1998)22:2<74::aid-lsm2>3.0.co;2-t
Kaneko, S., Fujimoto, S., Yamaguchi, H., Yamauchi, T., Yoshimoto, T., and Tokuda, K. (2018). Photodynamic therapy of malignant gliomas. Prog. Neurol. Surg. 32, 1–13. Epub 2018 Jul 10. PMID: 29990969. doi:10.1159/000469675
Kennedy, J. C., and Pottier, R. H. (1992). New trends in photobiology. J. Photochem Photobiol. B 14 (4), 275–292. PMID: 1403373]. doi:10.1016/1011-1344(92)85108-7
Kharroubi Lakouas, D., Huglo, D., Mordon, S., and Vermandel, M. (2017). Nuclear medicine for photodynamic therapy in cancer: planning, monitoring and nuclear PDT. Photodiagnosis Photodyn. Ther. 18, 236–243. doi:10.1016/j.pdpdt.2017.03.002
Kim, H. S., and Lee, D. Y. (2022). Nanomedicine in clinical photodynamic therapy for the treatment of brain tumors. Biomedicines 10 (1), 96. doi:10.3390/biomedicines10010096
Kostron, H. (2010). Photodynamic diagnosis and therapy and the brain. Methods Mol. Biol. 635, 261–280. doi:10.1007/978-1-60761-697-9_17
Kostron, H., Fiegele, T., and Akatuna, E. (2006). Combination of FOSCAN® mediated fluorescence guided resection and photodynamic treatment as new therapeutic concept for malignant brain tumors. Med. Laser Appl. 21 (Issue 4), 285–290. doi:10.1016/j.mla.2006.08.001
Kwiatkowski, S., Knap, B., Przystupski, D., Saczko, J., Kędzierska, E., Knap-Czop, K., et al. (2018). Photodynamic therapy - mechanisms, photosensitizers and combinations. Biomed. Pharmacother. 106, 1098–1107. Epub 2018 Jul 17. PMID: 30119176. doi:10.1016/j.biopha.2018.07.049
Ladomersky, E., Scholtens, D. M., Kocherginsky, M., Hibler, E. A., Bartom, E. T., Otto-Meyer, S., et al. (2019). The coincidence between increasing age, immunosuppression, and the incidence of patients with glioblastoma. Front. Pharmacol. 10, 200. doi:10.3389/fphar.2019.00200
Lamberti, M. J., Vittar, N. B., and Rivarola, V. A. (2014). Breast cancer as photodynamic therapy target: enhanced therapeutic efficiency by overview of tumor complexity. World J. Clin. Oncol. 5 (5), 901–907. doi:10.5306/wjco.v5.i5.901
Lee, S. Y., Luk, S. K., Chuang, C. P., Yip, S. P., To, S. S., and Yung, Y. M. (2010). TP53 regulates human AlkB homologue 2 expression in glioma resistance to Photofrin-mediated photodynamic therapy. Br. J. Cancer 103 (3), 362–369. doi:10.1038/sj.bjc.6605797
Leroy, H. A., Guérin, L., Lecomte, F., Baert, G., Vignion, A. S., Mordon, S., et al. (2021). Is interstitial photodynamic therapy for brain tumors ready for clinical practice? A systematic review. Photodiagnosis Photodyn. Ther. 36, 102492. Epub 2021 Aug 19. PMID: 34419674. doi:10.1016/j.pdpdt.2021.102492
Li, D., Cai, S., Wang, P., Cheng, H., Cheng, B., Zhang, Y., et al. (2023). Innovative design strategies advance biomedical applications of phthalocyanines. Adv. Healthc. Mater 12 (22), e2300263. Epub 2023 May 16. PMID: 37039069. doi:10.1002/adhm.202300263
Lietke, S., Schmutzer, M., Schwartz, C., Weller, J., Siller, S., Aumiller, M., et al. (2021). Interstitial photodynamic therapy using 5-ALA for malignant glioma recurrences. Cancers (Basel) 13 (8), 1767. PMCID: PMC8067827. doi:10.3390/cancers13081767
Liu, Q., Wackenhut, F., Hauler, O., Scholz, M., Zur Oven-Krockhaus, S., Ritz, R., et al. (2020). Hypericin: single molecule spectroscopy of an active natural drug. J. Phys. Chem. A 124 (12), 2497–2504. Epub 2020 Mar 11. PMID: 32126168. doi:10.1021/acs.jpca.9b11532
Mahmoudi, K., Garvey, K. L., Bouras, A., Cramer, G., Stepp, H., Jesu Raj, J. G., et al. (2019). 5-aminolevulinic acid photodynamic therapy for the treatment of high-grade gliomas. J. Neurooncol 141 (3), 595–607. Epub 2019 Jan 18. PMID: 30659522; PMCID: PMC6538286. doi:10.1007/s11060-019-03103-4
Mannino, S., Molinari, A., Sabatino, G., Ciafrè, S. A., Colone, M., Maira, G., et al. (2008). Intratumoral vs systemic administration of meta-tetrahydroxyphenylchlorin for photodynamic therapy of malignant gliomas: assessment of uptake and spatial distribution in C6 rat glioma model. Int. J. Immunopathol. Pharmacol. 21 (1), 227–231. doi:10.1177/039463200802100126
Mansoori, B., Mohammadi, A., Amin Doustvandi, M., Mohammadnejad, F., Kamari, F., Gjerstorff, M. F., et al. (2019). Photodynamic therapy for cancer: role of natural products. Photodiagnosis Photodyn. Ther. 26, 395–404. Epub 2019 May 4. doi:10.1016/j.pdpdt.2019.04.033
Marconi, A., Giugliano, G., Di Giosia, M., Marforio, T. D., Trivini, M., Turrini, E., et al. (2023). Identification of blood transport proteins to carry temoporfin: a domino approach from virtual screening to synthesis and in vitro PDT testing. Pharmaceutics 15 (3), 919. doi:10.3390/pharmaceutics15030919
Mata, A. I., Pereira, N. A. M., Cardoso, A. L., Nascimento, B. F. O., Pineiro, M., Schaberle, F. A., et al. (2023). Novel Foscan®-derived ring-fused chlorins for photodynamic therapy of cancer. Bioorg Med. Chem. 93, 117443. Epub 2023 Aug 12. PMID: 37634417. doi:10.1016/j.bmc.2023.117443
Meng, Z., Xue, H., Wang, T., Chen, B., Dong, X., Yang, L., et al. (2022). Aggregation-induced emission photosensitizer-based photodynamic therapy in cancer: from chemical to clinical. J. Nanobiotechnology 20 (1), 344. doi:10.1186/s12951-022-01553-z
Miki, Y., Akimoto, J., Yokoyama, S., Homma, T., Tsutsumi, M., Haraoka, J., et al. (2013). Photodynamic therapy in combination with talaporfin sodium induces mitochondrial apoptotic cell death accompanied with necrosis in glioma cells. Biol. Pharm. Bull. 36 (2), 215–221. Epub 2012 Nov 29. PMID: 23196427. doi:10.1248/bpb.b12-00567
Miretti, M., Prucca, C. G., Tempesti, T. C., and Baumgartner, M. T. (2021). Current phthalocyanines delivery systems in photodynamic therapy: an updated review. Curr. Med. Chem. 28 (26), 5339–5367. doi:10.2174/0929867328666210208111234
Misuth, M., Horvath, D., Miskovsky, P., and Huntosova, V. (2017). Synergism between PKCδ regulators hypericin and rottlerin enhances apoptosis in U87 MG glioma cells after light stimulation. Photodiagnosis Photodyn. Ther. 18, 267–274. Epub 2017 Mar 31. PMID: 28373118. doi:10.1016/j.pdpdt.2017.03.018
Muller, P. J., and Wilson, B. C. (2006). Photodynamic therapy of brain tumors-a work in progress. Lasers Surg. Med. 38, 384–389. doi:10.1002/lsm.20338
Muragaki, Y., Akimoto, J., Maruyama, T., Iseki, H., Ikuta, S., Nitta, M., et al. (2013). Phase II clinical study on intraoperative photodynamic therapy with talaporfin sodium and semiconductor laser in patients with malignant brain tumors. J. Neurosurg. 119 (4), 845–852. Epub 2013 Aug 16. PMID: 23952800. doi:10.3171/2013.7.JNS13415
Murotomi, K., Umeno, A., Shichiri, M., Tanito, M., and Yoshida, Y. (2023). Significance of singlet oxygen molecule in pathologies. Int. J. Mol. Sci. 24 (3), 2739. PMID: 36769060; PMCID: PMC9917472. doi:10.3390/ijms24032739
Namatame, H., Akimoto, J., Matsumura, H., Haraoka, J., and Aizawa, K. (2008). Photodynamic therapy of C6-implanted glioma cells in the rat brain employing second-generation photosensitizer talaporfin sodium. Photodiagnosis Photodyn. Ther. 5 (3), 198–209. Epub 2008 Oct 22. PMID: 19356656. doi:10.1016/j.pdpdt.2008.08.001
Ng, Q. X., Venkatanarayanan, N., and Ho, C. Y. (2017). Clinical use of Hypericum perforatum (St John's wort) in depression: a meta-analysis. J. Affect Disord. 210, 211–221. Epub 2017 Jan 3. PMID: 28064110. doi:10.1016/j.jad.2016.12.048
Niculescu, A. G., and Grumezescu, A. M. (2021). Photodynamic therapy—an up-to-date review. Appl. Sci. 11, 3626. doi:10.3390/app11083626
Omura, N., Nonoguchi, N., Fujishiro, T., Park, Y., Ikeda, N., Kajimoto, Y., et al. (2023). Ablation efficacy of 5-aminolevulinic acid-mediated photodynamic therapy on human glioma stem cells. Photodiagnosis Photodyn. Ther. 41, 103119. PMID: 36336324. doi:10.1016/j.pdpdt.2022.103119
Oniszczuk, A., Wojtunik-Kulesza, K. A., Oniszczuk, T., and Kasprzak, K. (2016). The potential of photodynamic therapy (PDT)-Experimental investigations and clinical use. Biomed. Pharmacother. 83, 912–929. Epub 2016 Aug 10. PMID: 27522005. doi:10.1016/j.biopha.2016.07.058
Park, W., Cho, S., Han, J., Shin, H., Na, K., Lee, B., et al. (2018). Advanced smart-photosensitizers for more effective cancer treatment. Biomater. Sci. 6, 79–90. doi:10.1039/c7bm00872d
Quirk, B. J., Brandal, G., Donlon, S., Vera, J. C., Mang, T. S., Foy, A. B., et al. (2015). Photodynamic therapy (PDT) for malignant brain tumors--where do we stand? Photodiagnosis Photodyn. Ther. 12 (3), 530–544. Epub 2015 May 8. PMID: 25960361. doi:10.1016/j.pdpdt.2015.04.009
Rizzo, P., Altschmied, L., Stark, P., Rutten, T., Gündel, A., Scharfenberg, S., et al. (2019). Discovery of key regulators of dark gland development and Hypericin biosynthesis in St. John’s Wort (Hypericum perforatum). Plant. Biotechnol. J. 17, 2299–2312. doi:10.1111/pbi.13141
Roguin, L. P., Chiarante, N., García Vior, M. C., and Marino, J. (2019). Zinc(II) phthalocyanines as photosensitizers for antitumor photodynamic therapy. Int. J. Biochem. Cell Biol. 114, 105575. Epub 2019 Jul 27. PMID: 31362060. doi:10.1016/j.biocel.2019.105575
Santos, K. L. M., Barros, R. M., da Silva Lima, D. P., Nunes, A. M. A., Sato, M. R., Faccio, R., et al. (2020). Prospective application of phthalocyanines in the photodynamic therapy against microorganisms and tumor cells: a mini-review. Photodiagnosis Photodyn. Ther. 32, 102032. Epub 2020 Oct 2. PMID: 33017659. doi:10.1016/j.pdpdt.2020.102032
Schwake, M., Nemes, A., Dondrop, J., Schroeteler, J., Schipmann, S., Senner, V., et al. (2018). In-vitro use of 5-ALA for photodynamic therapy in pediatric brain tumors. Neurosurgery 83 (6), 1328–1337. doi:10.1093/neuros/nyy054
Shrestha, R., Lee, H. J., Lim, J., Gurung, P., Thapa Magar, T. B., Kim, Y. T., et al. (2022). Effect of Photodynamic Therapy with Chlorin e6 on Canine Tumors. Life (Basel) 12 (12), 2102. PMCID: PMC9782963. doi:10.3390/life12122102
Stepp, H., and Stummer, W. (2018). 5-ALA in the management of malignant glioma. Lasers Surg. Med. 50 (5), 399–419. Epub 2018 May 8. PMID: 29737540. doi:10.1002/lsm.22933
Stummer, W., Pichlmeier, U., Meinel, T., Wiestler, O. D., Zanella, F., and Reulen, H. J.ALA-Glioma Study Group (2006). Fluorescence-guided surgery with 5-aminolevulinic acid for resection of malignant glioma: a randomised controlled multicentre phase III trial. Lancet Oncol. 7 (5), 392–401. PMID: 16648043. doi:10.1016/S1470-2045(06)70665-9
Stylli, S. S., Kaye, A. H., MacGregor, L., Howes, M., and Rajendra, P. (2005). Photodynamic therapy of high grade glioma - long term survival. J. Clin. Neurosci. 12 (4), 389–398. doi:10.1016/j.jocn.2005.01.006
Sun, M., Zhang, Y., He, Y., Xiong, M., Huang, H., Pei, S., et al. (2019). Green synthesis of carrier-free curcumin nanodrugs for light-activated breast cancer photodynamic therapy. Colloids Surfaces B Biointerfaces 180, 313–318. doi:10.1016/j.colsurfb.2019.04.061
Sunil, V., Mozhi, A., Zhan, W., Teoh, J. H., and Wang, C. H. (2021). Convection enhanced delivery of light responsive antigen capturing oxygen generators for chemo-phototherapy triggered adaptive immunity. Biomaterials 275, 120974. Epub 2021 Jun 16. PMID: 34166911. doi:10.1016/j.biomaterials.2021.120974
Teng, C. W., Amirshaghaghi, A., Cho, S. S., Cai, S. S., De Ravin, E., Singh, Y., et al. (2020). Combined fluorescence-guided surgery and photodynamic therapy for glioblastoma multiforme using cyanine and chlorin nanocluster. J. Neurooncol 149 (2), 243–252. Epub 2020 Sep 10. PMID: 32914293; PMCID: PMC7720701. doi:10.1007/s11060-020-03618-1
Tereshkina, Y. A., Kostryukova, L. V., Tikhonova, E. G., Khudoklinova, Y. Y., Orlova, N. A., Gisina, A. M., et al. (2022). Chlorin e6 Phospholipid Delivery System Featuring APN/CD13 Targeting Peptides: cell Death Pathways, Cell Localization, in vivo Biodistribution. Pharmaceutics 14 (10), 2224. doi:10.3390/pharmaceutics14102224
Thapa Magar, T. B., Mallik, S. K., Gurung, P., Lim, J., Kim, Y. T., Shrestha, R., et al. (2023). Chlorin E6-curcumin-mediated photodynamic therapy promotes an anti-photoaging effect in UVB-irradiated fibroblasts. Int. J. Mol. Sci. 24 (17), 13468. doi:10.3390/ijms241713468
Thomas, E., Colombeau, L., Gries, M., Peterlini, T., Mathieu, C., Thomas, N., et al. (2017). Ultrasmall AGuIX theranostic nanoparticles for vascular-targeted interstitial photodynamic therapy of glioblastoma. Int. J. Nanomed. 12, 7075–7088. doi:10.2147/ijn.s141559
Vanaclocha, V., Sureda, M., Azinovic, I., Rebollo, J., Cañón, R., Sapena, N. S., et al. (2015). Photodynamic therapy in the treatment of brain tumours. A feasibility study. Photodiagnosis Photodyn. Ther. 12 (3), 422–427. Epub 2015 Jun 11. PMID: 26073912. doi:10.1016/j.pdpdt.2015.05.007
Whelan, H. T., Schmidt, M. H., Segura, A. D., McAuliffe, T. L., Bajic, D. M., Murray, K. J., et al. (1993). The role of photodynamic therapy in posterior fossa brain tumors. A preclinical study in a canine glioma model. J. Neurosurg. 79 (4), 562–568. doi:10.3171/jns.1993.79.4.0562
Yakavets, I., Millard, M., Zorin, V., Lassalle, H. P., and Bezdetnaya, L. (2019). Current state of the nanoscale delivery systems for temoporfin-based photodynamic therapy: advanced delivery strategies. J. Control Release 304, 268–287. Epub 2019 May 25. PMID: 31136810. doi:10.1016/j.jconrel.2019.05.035
Zhao, X., Li, M., Sun, W., Fan, J., Du, J., and Peng, X. (2018). An estrogen receptor targeted ruthenium complex as a two-photon photody-namic therapy agent for breast cancer cells, Chem. Commun. 54(51), 7038–7041. doi:10.1039/c8cc03786h
Zirak, N., Shafiee, M., Soltani, G., Mirzaei, M., and Sahebkar, A. (2019). Hypericum perforatum in the treatment of psychiatric and neurodegenerative disorders: current evidence and potential mechanisms of action. J. Cell Physiol. 234 (6), 8496–8508. Epub 2018 Nov 21. PMID: 30461013. doi:10.1002/jcp.27781
Keywords: photodynamic therapy, brain cancer, photosensitizers, clinical studies, challenges
Citation: Domka W, Bartusik-Aebisher D, Rudy I, Dynarowicz K, Pięta K and Aebisher D (2023) Photodynamic therapy in brain cancer: mechanisms, clinical and preclinical studies and therapeutic challenges. Front. Chem. 11:1250621. doi: 10.3389/fchem.2023.1250621
Received: 29 September 2023; Accepted: 14 November 2023;
Published: 24 November 2023.
Edited by:
Jayachandran Jayakumar, National Tsing Hua University, TaiwanReviewed by:
Elamparuthi Ramasamy, University of Texas at Arlington, United StatesMingle Li, Shenzhen University, China
Copyright © 2023 Domka, Bartusik-Aebisher, Rudy, Dynarowicz, Pięta and Aebisher. This is an open-access article distributed under the terms of the Creative Commons Attribution License (CC BY). The use, distribution or reproduction in other forums is permitted, provided the original author(s) and the copyright owner(s) are credited and that the original publication in this journal is cited, in accordance with accepted academic practice. No use, distribution or reproduction is permitted which does not comply with these terms.
*Correspondence: Dorota Bartusik-Aebisher, ZGJhcnR1c2lrYWViaXNoZXJAdXIuZWR1LnBs; David Aebisher, ZGFlYmlzaGVyQHVyLmVkdS5wbA==