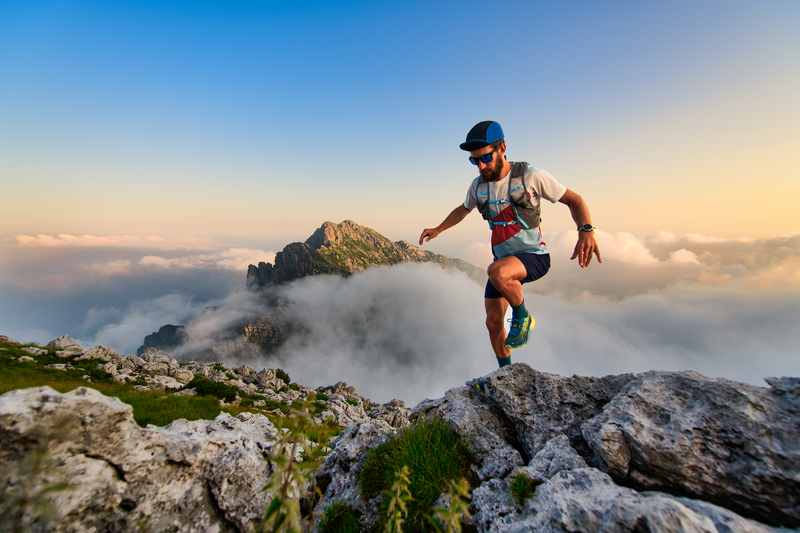
95% of researchers rate our articles as excellent or good
Learn more about the work of our research integrity team to safeguard the quality of each article we publish.
Find out more
ORIGINAL RESEARCH article
Front. Chem. , 01 August 2023
Sec. Analytical Chemistry
Volume 11 - 2023 | https://doi.org/10.3389/fchem.2023.1238631
This article is part of the Research Topic Chemical Sensing and Emerging Analysis of Environmental Contaminants View all 6 articles
Mercury ions (Hg2+) are widely found in the environment; it is considered a major pollutant. Therefore, the rapid and reliable detection of Hg2+ is of great technical interest. In this study, a highly fluorescent, sensitive, and selective fluorometric assay for detecting Hg2+ ions was developed using vancomycin functionalized and polyethyleneimine stabilized gold nanoparticles (PEI-f-AuNPs@Van). The as-made gold nanoparticles were highly fluorescent, with excitation and emission maxima occurring at 320 and 418 nm, respectively. The size of nanoparticles was ∼7 nm; a zeta potential of ∼38.8 mV was determined. The XRD analysis confirmed that the nanoparticles possessed crystalline structure with face centerd cubic symmetry. Using the PEI-f-AuNP@Van probe, the detection limit of Hg2+ ion was achieved up to 0.988 nM (within a linear range) by calculating the KSV. However, the detection limit in a natural environmental sample was shown to be 12.5 nM. Furthermore, the selectivity tests confirmed that the designed probe was highly selective to mercury (II) cations among tested other divalent cations. Owing to its sensitivity and selectivity, this approach for Hg2+ ions detection can be utilized for the analysis of real water samples.
Mercury ion (Hg2+) is an environmental metal contaminant; it is a nonessential and is toxic to lower organisms and humans. In case of human exposure, it can accumulate in body by the kidneys and resulting symptoms could include vomiting, diarrhea, hypovolemic shock, kidney failure, and potential death (Cowan, 1997; Park and Zheng, 2012; Fang et al., 2014; Gibb and O’Leary, 2014). It should also be noted that inorganic mercury ions and organic mercury ions (e.g., methyl mercuric (MeHg+), phenylmercuric (PhHg+), and ethyl mercuric (EtHg+) ions) can cause neurologic problems (e.g., ataxia, visual field constriction, hearing impairment, and blindness) (Hong et al., 2012; Dorea et al., 2013; Geier et al., 2014). As such, rapid and reliable mercury (II) ion detection is of technical interest. A variety of approaches such as direct mercury analysis (DMA) (Windmoller et al., 2017), Ion chromatography (IC) (Liu, 2010), as well as high-performance liquid chromatography (HPLC) (Yin et al., 2010; Cheng et al., 2014) have been demonstrated for mercury ion detection. It should be noted that these approaches involve complicated protocols such as sample pre-treatment steps, skilled technicians, and sophisticated tools. As such, the implementation of a low-cost and facile analytical approach for selective mercury ion detection is a major focus area in the field of analytical chemistry. Several types of nanoparticle assays that offer straightforward detection of Hg2+ ions have been evaluated; these approaches involve gold nanorod structures (Au-NR) (Placido et al., 2013), carbon nanoparticles (CNP) (Guo et al., 2013), silver nanoparticles (Ag-NPs) (Li et al., 2015), silver nano prism structures (Ag-NPR) (Chen et al., 2016), and gold nanoparticles (Au-NPs) (Chen et al., 2014; Sener et al., 2014; Chen et al., 2015). Due to inter-particle distance-dependent optical characteristics and high extinction coefficients, these colorimetric methods offer the capability of analyzing Hg2+ visually or via UV-Vis instrumentation.
Among various types of nanomaterials, gold nanoparticles (Au-NPs) have been recognized for their utility in various sensing and imaging applications. One of the desirable optical properties of Au-NPs is their localized surface plasmon resonance (LSPR) characteristics. Lorenz-Mie scattering was used to explain the SPR characteristics of spherical Au-NPs (Mulvaney, 1996; Sardar et al., 2009) and confirmed that fluorometric behavior of Au-NPs is depends on their particle shape, particle size, nanoscale geometry, refractive index of the medium, inter-particle distance, and aggregation state in a given solution. Any variation in these factors affects the plasmon-resonance frequency characteristics (Daniel and Astruc, 2004; Lin et al., 2011). These parameters may explain why Au-NPs having a size 5–20 nm appear deep red in color, while aggregates of small particles or larger particles appear deep blue to purple in color.
Considering the unique characteristics of Au-NPs, fluorometric sensing methods have attracted the research community due to the benefits, which include rapid analysis, cost-effective, and straightforward operation (Ma et al., 2017; Kim et al., 2018; Sedgwick et al., 2018; Vellaisamy et al., 2018; Wang et al., 2018; Wu et al., 2018). Further, Au-NPs have been widely used as a quenching material owing to their unique properties such as a large surface area to volume ratio, straightforward surface functionalization, and LSPR absorption characteristics in the visible light region (Furletov et al., 2017). The functionalization approach utilized with Au-NP materials involves surface modification with either ligands or receptor molecules using gold-sulfur (Au-S) and gold-nitrogen (Au-N) bonds. The use of a surface functionalization approach to alter the fluorescence activity of Au-NPs has been considered in this study. Under specific conditions (e.g., at high temperatures), PEI- mediated formation of gold nanoparticles over a relatively longer period of time has been previously discussed; however, we have for the first time described the rapid and controlled synthesis of gold nanoparticles under ambient conditions, in which there is an active role for PEI and formaldehyde/cyclohexanone (Pandey and Pandey, 2016; Pandey et al., 2017a; Pandey et al., 2017b). An advantage of the benign approach described in this study is that it is compatible with gene delivery mediators, templates, and stabilizers for modifying metal nanoparticles (Kumar et al., 2015). For example,. Amine groups may provide sufficient active sites for functionalization; these features of PEI can facilitate changes to the selectivity of various ions and biomolecules to deliver drugs and genes for breast cancer therapy and to deliver antimicrobial agents (Li et al., 2015; Kumar et al., 2015; Tiwari et al., 2020; Tiwari et al., 2022). Furthermore, Polyethyleneimine has been utilized for synthesizing noble metal nanoparticles (Tiwari et al., 2020; Tiwari et al., 2022; Pandey et al., 2023) and functionalization with curcumin (Pandey et al., 2023). These studies directed us to consider vancomycin, a potent agent used in the treatment of severe bacterial infections in hospitalized patients, which is a green-fluorescent analog. We described novel findings related to the formation of highly fluorescent gold nanoparticles via active participation of PEI, vancomycin, and formaldehyde; the fluorescent activity of the gold nanoparticles was entirely different from those of the respective precursors. Dramatically, as made fluorescent gold nanoparticles displayed selective variation of their fluorescent activity as a function of mercuric ions; findings on this topic are reported herein.
All of the materials and reagents were analytical quality. Vancomycin (purity ≥85%, CAS no. 1404-93-9) Polyethyleneimine (50% w/v in H2O; CAS no. 9002-98-6), tetra-chloroauric acid trihydrate (HAuCl4.3H2O; purity 99.9%, CAS no. 16961-25-4), and formaldehyde (≥36.0% in H2O) were purchased from Sigma Aldrich (Mumbai, India). Mercuric chloride (purity; ≥99.5%), potassium chloride (purity; 99%), sodium chloride (99%), magnesium chloride, and ammonium chloride were purchased from Merck (Bangalore, India). Other required glassware and plastic wares were purchased from Tarson (Mumbai, India). All of the experiments were performed using ultra-purified HPLC-grade water.
The PEI functionalized gold nanoparticles were synthesized per the procedure that was previously reported with slight modification (Pandey et al., 2023). Briefly, 400 μL (10 mM) of hydrogen tetra-chloroauric acid trihydrate (HAuCl4.3H2O) was placed in a 2 mL glass vial; this step was followed by the addition of an aqueous solution of vancomycin (40 μL, 2 mg/mL stock). The reaction mixture was then stirred on a magnetic stirrer for 5–10 min, followed by the addition of an aqueous solution of Polyethyleneimine (30 μL of 4 mg/mL stock solution). The reaction mixture continued to be mixed on the stirrer, followed by the addition of 30 μL of formaldehyde; this mixture continued to be stirred for the next 30–60 min to yield dark red-pink color PEI-f-AuNP@Van.
Several characterization methods were used to understand the synthesized fluorescent gold nanoparticles (Au-NPs). UV-VIS spectroscopy measurements were obtained using a Hitachi U-2900 spectrophotometer (Tokyo, Japan). Transmission electron microscopy (TEM) measurements were acquired using a FEI Tecnai G2 20 S Twin instrument (Hillsboro, Oregon, United States). The average length of gold nanoparticles was measured using ImageJ software (National Institutes of Health, Bethesda, MD, United States); a statistical graph was plotted on Origin 8.5 software (Northampton, MA, United States). The fluorescence emission (FL emission) spectra were obtained with a Hitachi F7000 fluorescence spectrophotometer (Tokyo, Japan). An X-ray photoelectron spectroscopy (XPS) instrument (Thermo Fisher Scientific, Waltham, MA, United States) with a K-alpha source was used to determine the binding energy and chemical composition of the samples. XRD spectra obtained using a Rigaku Mini-Flex 600 instrument (Tokyo, Japan). Time-resolved fluorescence spectra were acquired using a WiTec alpha 300 RA instrument (Ulm, Germany); a Malvern Nano Zeta Sizer (Malvern, United Kingdom) was used to obtain dynamic light scattering (DLS) zeta potential data.
All of the experiments were carried out in ultra-purified HPLC grade water. A 10 μL of synthesized gold nanoparticles were added to an aqueous solution containing various HgCl2 concentrations (ranging from 2 to 64 μM), which were placed in a 1 cm quartz cuvette; fluorescence spectra were recorded from these samples. All of the fluorescence spectra were obtained with a 5/10 slit width using an excitation of 320 nm. All of the measurements were repeated three times to assess if the results were accurate and the method was consistent.
A water sample was obtained from a pond at the institute campus (Indian Institute of Technology, BHU) Varanasi, India; this samples used immediately as an environmental sample without filtration. Various calculated spiked concentrations of HgCl2 (ranging from 0.5 to 32 μM) were added to a 1 cm quartz cuvette that contained a fixed volume of environmental sample and fluorescent Au-NPs; fluorescence spectra were recorded from these samples (Ma et al., 2019).
The prepared PEI-f-AuNPs were ∼7 nm in size using HAuCl4.3H2O As described in previous studies, Au- NPs synthesized by reducing HAuCl4 with citrate and other organic reducing agents (e.g., cyclohexanone) using a 3-glycedoxypropyltrimethoxysilane-mediated reduction were 10–33 nm (Rak et al., 2014; Mitra and Pandey, 2022); Au-NPs conjugated to branched Polyethyleneimine (PEI) with a molecular weight of 750 k Da under these conditions were much smaller (5–7 nm). As such, the mean Au-NP size was dependent on both the type and quantity of reducing agents, temperature, pH, and reaction time (Lin et al., 2013; Deraedt et al., 2014). A straightforward protocol was undertaken to reduce the gold cation in order to produce vancomycin-loaded gold nanoparticles; PEI was used as a stabilizing agent and formaldehyde was used as a reducing agent. The synthesized PEI-f-AuNP@Van were characterized using UV-Vis spectrophotometry; monitoring over time indicated a strong absorbance peak at 520 nm (Figure 1A), which was attributed to the synthesis of small-sized gold nanoparticles. TEM characterization confirmed the actual size of vancomycin-loaded gold nanoparticles was ∼7 nm (Figures 1C, D).
FIGURE 1. (A) Real time UV–visible spectra of vancomycin-loaded Polyethyleneimine functionalized Au- NPs synthesis, (B) corresponding X-ray differactogram, (C) TEM micrograph, and (D) size distribution plot.
The crystallinity and microstructure of the synthesized PEI-f-AuNP@Van were investigated using XRD from 10° to 80° 2θ degrees. Figure 1B shows the peaks for fluorescent Au-NPs as 38.4°, 44.6°, 64.6°, and 77.7°, corresponding to hkl values (111), (200), (220), (311) lattice planes, respectively. The peak at 38.4° was more intense than the other peaks, which suggested that Au in the fluorescent Au-NPs was in the face-cantered unit cell (FCC) structure. The hydrodynamic radii of fluorescent PEI-f-AuNP@Van were determined as ∼58 nm, which is larger than that of the bare Au-NPs (∼23 nm) as shown in Figure 2A. The increase in the hydrodynamic radii was associated with the loading of vancomycin. As shown in Figure 2B, the zeta potentials were to be ∼38.8 mV, indicating the presence of a positive charge on the surface of the fluorescent Au-NPs. Because of the greater magnitude of the zeta potential value, the dispersibility of the Au-NPs in water over many months was maintained, which indicates the stability of the fluorescent Au-NPs.
FIGURE 2. (A) Hydrodynamic radii of vancomycin-loaded and unloaded gold nanoparticles, (B) zeta potential, (C) fluorescent spectra of vancomycin and vancomycin-loaded gold nanoparticles excited at 270 nm, and (D) fluorescence screening of vancomycin-loaded gold nanoparticles at various excitation wavelengths (270–340 nm).
The fluorescence activity of vancomycin-loaded Au-NPs was evaluated to get a better understanding of the impact of the synthesis protocols on the applications of the materials. The emission spectra of the vancomycin-loaded gold nanoparticles at a given wavelength (λ = 280 nm) are shown in Figure 2C. It was revealed that the excitation of vancomycin at 270 nm was associated with an intense emission at 335 nm; when loaded on the gold nanoparticles, the emission shifts to 418 nm with significant fluorescence intensity. As shown in Figure 2C, the results indicate that the fluorescence activity was a function of vancomycin and the nanoscale geometry of gold. The fluorescence emission spectra indicate the PEI-f-AuNP@Van emits blue light when it is exposed to UV light with λ = 270 nm. A single absorption peak was noted at 520 nm from the dispersion of Au-NPs; an emission peak appeared at 418 nm after excitation at 270 nm. The fluorescent Au-NPs exhibited excitation-dependent activity over the 270–340 nm range as indicated in Figure 2D. During the early stages of the study, the wavelength of the excitation light decreased from 280 to 300 nm; the FL emission intensity of the light progressively increased without emission wavelength shifting. As the experiment progressed over time, the emission intensity progressively decreased. Our results indicate that diverse trap surface states cause an excitation-dependent emission pattern in the as-made fluorescent Au-NPs.
XPS measurements were undertaken to examine the chemical composition and the chemical bonding in the synthesized materials. Figure 3A shows the XPS survey spectrum of the fluorescent Au-NPs, which indicates that C, N, O, and Au were present. A deconvolution procedure on these peaks was undertaken to evaluate the chemical bonding as shown in Figures 3B–E. The XPS C1s, N1s, and O1s spectra are shown in Figures 2B, D, E, respectively. Figure 3C shows the XPS Au 4f core level spectrum. The oxidation state of the gold atom in HAuCl4 was initially Au3+; after PEI and formaldehyde were added to the HAuCl4 solution, the gold atom oxidation state decreased from Au3+ to Au0.
FIGURE 3. XPS spectra of the fluorescent Au-NPs: (A) survey scan showing the presence of various elements, (B) O1s spectra, (C) Au 4f spectra, (D) C1s spectra, and (E) N1s spectra.
The results shown in Figures 2C, D indicate the excellent fluorescent activity of vancomycin-loaded gold nanoparticles. We then evaluated the functionality of the as-made fluorescent gold nanoparticles on mercury (II) sensing using PEI-f-AuNP@Van as a probe (shown in Figure 4A). The results showed that the Hg2+ cation significantly quenched the fluorescence emission of the developed probe. After the concentration of the Hg2+ cation was raised from 2 to 64 μM, the fluorescence intensity of PEI-f- AuNP@Van was noted to be considerably quenched (as indicated in Figure 4A). It is again important to note the kinetic variation (if any) on the activity of PEI-f-AuNP@Van on Hg2+-based fluorescence quenching, which is based on the Stern–Volmer equation:
In this equation, F is the FL intensity as a function of various concentrations of the quencher [Hg2+], F0 is the FL emission intensity at [Hg2+] = 0, and KSV is the Stern–Volmer quenching constant. The relative kinetic variation determined using the above equation is plotted in Figure 4B. The kinetic parameters are incorporated into Figure 4B for the nanoparticles, which show the dependence of fluorescence quenching of PEI-f-AuNP@Van on Hg2+ sensing. The LOD was determined; it was noted that it might be as low as 0.988 nM (calculated at a DL = 3.3 × σ/S, where σ = slope and S = standard deviation). This finding demonstrates that PEI-f-AuNP@Van show promise for as a sensitive approach for Hg2+ detection.
FIGURE 4. (A) Dependence of Fluorescence emission intensity on Hg2+ concentration between 2 and 64 μM, and (B) Stern–Volmer (S–V) plot with the addition of Hg2+ under similar conditions into vancomycin loaded Au-NPs. The inset to (B) shows the kinetic parameters.
The physical characteristics of the developed probe were monitored by considering parameters such as the effect of Hg2+ ions on hydrodynamic radii, the effect on UV-Vis absorbance of the probe, as well as changes in crystallinity and zeta potential. The results showed that the hydrodynamic radii of synthesized gold nanoparticles were ∼58 nm (as shown in Figure 2A); the radii increased to ∼77 nm after a fixed concentration of mercury ions was added with decreasing count percent (Figure 5A). The increase in hydrodynamic radii indicated the binding of mercury ions to the gold nanoparticle surface. This binding could be mediated by the nitrogen atoms of PEI and vancomycin as suggested by a previous study that was conducted by Kim et al. (2018). Further, the N 1 s feature at 406.2 eV in Hg2+-PEI-AuNPs indicated the binding energy of the Hg2+-N bonds (Tao, 2012). Another study confirmed the binding of Hg2+ to the tertiary nitrogen of PEI-Au-NP; however, the role of vancomycin in the binding of Hg2+ is not confirmed yet except for making the gold nanoparticles highly fluorescent. The XRD pattern of Hg2+ ions added to fluorescent gold nanoparticles was obtained (Figure 5B); this result confirms the coordinated binding of Hg2+ ions to the nanoparticles. Intense XRD peaks of mercury were observed at the planes of (111), (200), (311), and (400) except for gold nanoparticles (as shown in Figure 5B). The UV-Vis spectrum of gold nanoparticles was recorded to observe any change in absorbance in PEI-f-AuNP@Van after the addition of Hg2+ ions (as indicated in Figure 5C). However, no such phenomena were observed. Further, the zeta potential of PEI-f- AuNP@Van recorded after adding Hg2+ increased significantly to ∼48.3 mV as shown in Figure 5D compared to bare PEI-f-AuNP@Van (Figure 2D; 38 mV). This increase in zeta potential enhanced the stability of the probe and confirmed once again the binding of Hg2+ to the probe.
FIGURE 5. (A) Changes in fundamental physical properties of vancomycin loaded Au-NP after the addition of Hg2+ under similar conditions, hydrodynamic radii, (B) XRD result, (C) UV-Visible spectrum, and (D) zeta potential.
The selectivity 0f the PEI-f-AuNP@Van assay was evaluated with 60 μM Hg2+ and various metal cations (100 μM Cr3+, Pb2+, As3+, Mg2+, and Co2+ ions). As shown in Figure 6, only Hg2+ showed significant fluorescence quenching behavior against the probe among the tested metal cations. The Hg2+ quenched the fluorescence of PEI-f-AuNP@Van around 70%; the other tested anions quenched only 10%–20%. The high quenching ratio of Hg2+ was associated with the aggregation of PEI-f-AuNP@Van; a low quenching ratio in the presence of other metal ions indicated the maintenance of well-dispersed forms of PEI-f-AuNP@Van. As such, the results suggest that the Hg2+ ion is selectively coordinated with sites on PEI-f-AuNP@Van.
The probe was assessed as a function of salt tolerance, pH, and the effect of time on the fluorescence property of the probe to optimize the sensitivity of the PEI-f-AuNP@Van probe for Hg2+. The fluorescence intensity of the PEI-f-AuNP@Van probe was modulated as a function of pH; this value was the highest at pH = 7.0 (Figure 7B). This optimum pH of the PEI-f-AuNP@Van probe is likely related to the conformation of PEI and the pKa of the tertiary amine (Curtis et al., 2016). At pH 7, the Hg2+ must be optimally coordinated with nitrogen atoms in PEI in its N-tetrahedral form, giving rise to highest sensitivity and selectivity for the probe (GaJney and Marley, 2014).
FIGURE 7. Stability of PEI-f-AuNP@Van in different salts (A) on variable pH (B) and effect of time on the fluorescence (C).
Pond water was used to evaluate the performance of PEI-f-Au-NP@Van for detecting Hg2+. The pond water samples were spiked with Hg2+ (ranging from 0.5 to 32 μM). The results indicate that the Hg2+ cation significantly quenched the fluorescence emission of the probe. After the concentration of the Hg2+ cation was increased from 0.5 to 32 μM, the fluorescence intensity of PEI-f-AuNP@Van was noted to be markedly quenched (Figure 8A). It is important to consider the potential role, if any, of kinetic variation on the performance of PEI-f-AuNP@Van on Hg2+ fluorescence quenching based on the Stern Volmer equation as provided above; the relative kinetic variation based on the Stern–Volmer equation is plotted as provided in Figure 8B. The kinetic parameters are inserted in Figure 8B for the nanoparticles, indicating the dependence of fluorescence quenching of PEI-f-AuNP@Van on Hg2+ sensing. The LOD was calculated; it might be as low as 12.5 nM (calculated at a DL = 3.3 × σ/S). This demonstrates that PEI-f-AuNP@Van is a promising material for sensitive Hg2+ detection. A detailed quantitative study is currently underway.
FIGURE 8. (A) Relationship between the fluorescence emission intensity and spiked Hg2+ concentration between 0.5 and 32 μM, and (B) Stern–Volmer (S–V) plot with addition of Hg2+ under similar conditions into vancomycin-loaded Au-NPs. The inset to (B) shows the kinetic parameters.
The fluorescence quenching of PEI-f-AuNP@Van in the presence of Hg2+ was evaluated using a time-resolved fluorescence decay analysis; this approach was used to determine quenching. The fluorescence decay can be evaluated using a double exponential function with the following equation:
In the equation, τi represents the fluorescence lifetimes of numerous fluorescent forms; D represents the fluorescence decay, and ai representing the associated pre-exponential factors (Raut et al., 2014). When no acceptor is present, the PEI-f-AuNP@Van shows an average lifetime of 3.25 ns (as indicated in Figure 9). The average lifetimes of 1.12 ns was significantly lower after addition of electron acceptor Hg2+, which indicates that PEI-f-AuNP@Van transfers electrons to Hg2+; it serves as an electron acceptor to lower the intensity of the fluorescence emission of PEI-f-AuNP@Van via the electron transfer pathway. In addition, it was noted that the count was substantially reduced, indicating the agglomeration of nanoparticles.
In conclusion, Polyethyleneimine mediated the controlled and rapid synthesis of vancomycin-functionalized fluorescent Au-NPs for detecting Hg2+ in environmental samples. The size of fluorescent Au-NPs (∼7 nm) and the zeta potential value (∼38 mV) suggested high fluorescence and stability. The physico-chemical properties of the fluorescent PEI-f-AuNP@Van were determined using X-ray photoelectron spectroscopy, UV–vis spectroscopy, X-ray diffraction, transmission microscopy, and photoluminescence spectroscopy. The synthesized fluorescent Au-NPs demonstrated a strong binding affinity for Hg2+, which was associated with a low detection limit of 0.988 nM and the capability for detecting Hg2+ in environmental samples with high selectivity and sensitivity. This study indicates possibilities for developing low-cost, ultra-sensitive, and straightforward detection methods for Hg2+.
The original contributions presented in the study are included in the article/Supplementary Material, further inquiries can be directed to the corresponding authors.
AT and PP conceived the experiments and designed the experiments. AT, HY, and MG conducted sample preparation, conducted the experiments, and performed the analyses. AT and PP wrote the manuscript. RN and PP oversaw the completion of the study and finally edited the manuscript. All authors contributed to the article and approved the submitted version.
The authors are thankful to the Central Instrument Facility, Indian Institute of Technology (BHU) for material characterization, Central Discovery Center (CDC), Banaras Hindu University, Varanasi for TRPL recording and Electron microscopy facility under SAIF at AIIMS, New Delhi, India for the TEM characterization of nanoparticles.
The authors declare that the research was conducted in the absence of any commercial or financial relationships that could be construed as a potential conflict of interest.
All claims expressed in this article are solely those of the authors and do not necessarily represent those of their affiliated organizations, or those of the publisher, the editors and the reviewers. Any product that may be evaluated in this article, or claim that may be made by its manufacturer, is not guaranteed or endorsed by the publisher.
Curtis, K. A., Miller, D., Millard, P., Basu, S., Horkay, F., and Chandran, P. L. (2016). Unusual salt and pH induced changes in polyethylenimine solutions. PLoS One 11, e0158147. doi:10.1371/journal.pone.0158147
Chen, L., Li, J., and Chen, L. (2014). Colorimetric detection of mercury species based on functionalized gold nanoparticles. ACS Appl. Mater. Interfaces 6, 15897–15904. doi:10.1021/am503531c
Chen, N., Zhang, Y., Liu, H., Wu, X., Miao, L., Shen, Z., et al. (2016). High-performance colorimetric detection of Hg2+ based on triangular silver nanoprisms. ACS Sensors 1 (5), 521–527. doi:10.1021/acssensors.6b00001
Chen, Z., Zhang, C., Ma, H., Zhou, T., Jiang, B., Chen, M., et al. (2015). A non-aggregation spectrometric determination for mercury ions based on gold nanoparticles and thiocyanuric acid. Talanta 134, 603–606. doi:10.1016/j.talanta.2014.11.065
Cheng, H., Wu, C., Shen, L., Liu, J., and Xu, Z. (2014). Online anion exchange column preconcentration and high-performance liquid chromatographic separation with inductively coupled plasma mass spectrometry detection for mercury speciation analysis. Anal. Chim. Acta 828, 9–16. doi:10.1016/j.aca.2014.04.042
Daniel, M. C., and Astruc, D. (2004). Gold nanoparticles: Assembly, supramolecular chemistry, quantum-size-related properties, and applications toward biology, catalysis, and nanotechnology. Chem. Rev. 104, 293–346. doi:10.1021/cr030698+
Deraedt, C., Salmon, L., Gatard, S., Ciganda, R., Hernandez, R., Ruiz, J., et al. (2014). Sodium borohydride stabilizes very active gold nanoparticle catalysts. Chem. Commun. 50, 14194–14196. doi:10.1039/c4cc05946h
Dorea, J. G., Farina, M., and Rocha, J. B. (2013). Toxicity of ethylmercury (and thimerosal): A comparison with methylmercury: Comparative toxicity of ethyl- and methylmercury. J. Appl. Toxi. 33, 700–711. doi:10.1002/jat.2855
Fang, Y., Sun, X., Yang, W., Ma, N., Xin, Z., Fu, J., et al. (2014). Concentrations and health risks of lead, cadmium, arsenic, and mercury in rice and edible mushrooms in China. Food Chem. 147, 147–151. doi:10.1016/j.foodchem.2013.09.116
Furletov, A. A., Apyari, V. V., Garshev, A. V., Dmitrienko, S. G., and Zolotov, Y. A. (2017). Triangular silver nanoplates as a spectrophotometric reagent for the determination of mercury (II). J. Anal. Chem. 72, 1203–1207. doi:10.1134/s1061934817120061
GaJney, J., and Marley, N. (2014). In-depth review of atmospheric mercury: Sources, transformations, and potential sinks. Energy Emiss. Control Technol. 2, 1–21.doi:10.2147/EECT.S37038
Geier, D. A., Hooker, B. S., Kern, J. K., King, P. G., Sykes, L. K., and Geier, M. R. (2014). A dose-response relationship between organic mercury exposure from thimerosal-containing vaccines and neurodevelopmental disorders. Int. J. Env. Res. Pub. Health 11, 9156–9170. doi:10.3390/ijerph110909156
Gibb, M., and O’Leary, K. G. (2014). Mercury exposure and health impacts among individuals in the artisanal and small-scale gold mining community: A comprehensive review. Environ. Health Perspect. 122 (7), 667–672. doi:10.1289/ehp.1307864
Guo, Y., Wang, Z., Shao, H., and Jiang, X. (2013). Hydrothermal synthesis of highly fluorescent carbon nanoparticles from sodium citrate and their use for the detection of mercury ions. Carbon 52, 583–589. doi:10.1016/j.carbon.2012.10.028
Hong, Y. S., Kim, Y. M., and Lee, K. E. (2012). Methylmercury exposure and health effects. J. Prev. Med. Public Health 45, 353–363. doi:10.3961/jpmph.2012.45.6.353
Kim, K. M., Nam, Y. S., Lee, Y., and Lee, K. B. (2018b). A highly sensitive and selective colorimetric Hg2+ ion probe using gold nanoparticles functionalized with polyethyleneimine. J. Anal. Methods Chem. 2018, 1–12. doi:10.1155/2018/1206913
Kim, T. I., Hwang, B., Lee, B., Bae, J., and Kim, Y. (2018a). Selective monitoring and imaging of eosinophil peroxidase activity with a J-aggregating probe. J. Am. Chem. Soc. 140, 11771–11776. doi:10.1021/jacs.8b07073
Kumar, V. V., Thenmozhi, M. K., Ganesan, A., Ganesan, S. S., and Anthony, S. P. (2015). Hyper-branched polyethylenimine-based sensor of multiple metal ions (Cu2+, Co2+ and Fe2+): Colorimetric sensing via coordination or AgNP formation. RCS Adv. 5, 88125–88132. doi:10.1039/c5ra13797g
Li, L., Gui, L., and Li, W. (2015a). A colorimetric silver nanoparticle based assay for Hg(II) using lysine as a particle-linking reagent. Microchim. Acta 182, 1977–1981. doi:10.1007/s00604-015-1536-2
Li, M., Li, Y., Huang, X., and Lu, X. (2015b). Captopril polyethyleneimine conjugate modified gold nanoparticles for co-delivery of drug and gene in anti-angiogenesis breast cancer therapy. J. Biomaterials Sci. Polym. Ed. 26, 813–827. doi:10.1080/09205063.2015.1057991
Lin, C., Tao, K., Hua, D., Ma, Z., and Zhou, S. (2013). Size Effect of gold nanoparticles in catalytic reduction of p-nitrophenol with NaBH4. Molecules 18, 12609–12620. doi:10.3390/molecules181012609
Lin, Y. W., Huang, C. C., and Chang, H. T. (2011). Gold nanoparticle probes for the detection of mercury, lead and copper ions. Analyst 136, 863–871. doi:10.1039/c0an00652a
Liu, Q. (2010). Determination of mercury and methylmercury in seafood by ion chromatography using photo-induced chemical vapor generation atomic fluorescence spectrometric detection. Microchem. J. 95, 255–258. doi:10.1016/j.microc.2009.12.010
Ma, D. L., Lin, S., Wang, W., Yang, C., and Leung, C. H. (2017). Luminescent chemosensors by using cyclometalated iridium (III) complexes and their applications. Chem. Sci. 8, 878–889. doi:10.1039/c6sc04175b
Ma, H., Xue, N., Wu, S., Li, Z., and Miao, X. (2019). Fluorometric determination of mercury (II) using positively charged gold nanoparticles, DNA-templated silver nanoclusters, T-Hg (II)-T interaction and exonuclease assisted signal amplification. Microchim. Acta 186, 317. doi:10.1007/s00604-019-3388-7
Mitra, M. D., and Pandey, P. C. (2022). Functional trialkoxysilane mediated controlled synthesis of fluorescent gold nanoparticles and fluoremetric sensing of dopamine. Opt. Mater. 132, 112810. doi:10.1016/j.optmat.2022.112810
Mulvaney, P. (1996). Surface plasmon spectroscopy of nanosized metal particles. Langmuir 12, 788–800. doi:10.1021/la9502711
Pandey, M., Singh, A., and Pandey, P. C. (2023). Synthesis and in vitro antibacterial behavior of curcumin-conjugated gold nanoparticles. J. Mater. Chem. B 11, 3014–3026. doi:10.1039/d2tb02256g
Pandey, P. C., Pandey, G., and Narayan, R. J. (2017a). Controlled synthesis of polyethylenimine coated gold nanoparticles: Application in glutathione sensing and nucleotide delivery: Controlled Synthesis of Gold Nanoparticles. J. Biomed. Mater. Res. Part B. 105, 1191–1199. doi:10.1002/jbm.b.33647
Pandey, P. C., Pandey, G., and Narayan, R. J. (2017b). Polyethylenimine-mediated synthetic insertion of gold nanoparticles into mesoporous silica nanoparticles for drug loading and biocatalysis. Biointerphases 12, 011005. doi:10.1116/1.4979200
Pandey, P. C., and Pandey, G. (2016). Novel synthesis of gold nanoparticles mediated by polyethylenimine and organic reducing agents for biomedical applications. Adv. Sci. Eng. Med. 8, 43–48. doi:10.1166/asem.2016.1805
Park, J. D., and Zheng, W. (2012). Human exposure and health effects of inorganic and elemental mercury. J. Prev. Med. Public Health 45, 344–352. doi:10.3961/jpmph.2012.45.6.344
Placido, T., Aragay, G., Pons, J., Comparelli, R., Curri, M. L., and Merkoci, A. (2013). Ion-directed assembly of gold nanorods: A strategy for mercury detection. ACS Appl. Mater. Interfaces 5 (3), 1084–1092. doi:10.1021/am302870b
Rak, M. J., Saade, N. K., Fri ´ ˇsci ˇ c, T., and Moores, A. (2014). Mechanosynthesis of ultra-small monodisperse amine-stabilized gold nanoparticles with controllable size. Green Chem. 16, 86–89. doi:10.1039/c3gc41827h
Raut, S., Rich, R., Fudala, R., Butler, S., Kokate, R., Gryczynski, Z., et al. (2014). Resonance energy transfer between fluorescent BSA protected Au nanoclusters and organic fluorophores. Nanoscale 6, 385–391. doi:10.1039/c3nr03886f
Sardar, R., Funston, A. M., Mulvaney, P., and Murray, R. W. (2009). Gold nanoparticles: Past, present, and future. Langmuir 25, 13840–13851. doi:10.1021/la9019475
Sedgwick, A. C., Dou, W. T., Jiao, J. B., Wu, L., Williams, G. T., Sessler, J. L., et al. (2018). An ESIPT probe for the ratiometric imaging of peroxynitrite facilitated by binding to aβ-aggregates. J. Am. Chem. Soc. 140, 14267–14271. doi:10.1021/jacs.8b08457
Sener, G., Uzun, L., and Denizli, A. (2014). Lysine-promoted colorimetric response of gold nanoparticles: A simple assay for ultrasensitive mercury (II) detection. Anal. Chem. 86, 514–520. doi:10.1021/ac403447a
Tao, F. F. (2012). Design of an in-house ambient pressure AP-XPS using a bench-top X-ray source and the surface chemistry of ceria under reaction conditions. Chem. Commun. 48, 3812–3814. doi:10.1039/c2cc17715c
Tiwari, A. K., Gupta, M. K., Pandey, G., Narayan, R. J., and Pandey, P. C. (2020). Molecular weight of polyethylenimine-dependent transfusion and selective antimicrobial activity of functional silver nanoparticles. J. Mater. Res. 35, 2405–2415. doi:10.1557/jmr.2020.183
Tiwari, A. K., Gupta, M. K., Pandey, G., Tilak, R., Narayan, R. J., and Pandey, P. C. (2022). Size and zeta potential clicked germination attenuation and anti-sporangiospores activity of PEI-functionalized silver nanoparticles against COVID-19 associated mucorales (rhizopus arrhizus). Nanomaterials 12, 2235. doi:10.3390/nano12132235
Vellaisamy, K., Li, G., Ko, C. N., Zhong, H. J., Fatima, S., Kwan, H. Y., et al. (2018). Cell imaging of dopamine receptor using agonist labeling iridium (III) complex. Chem. Sci. 9, 1119–1125. doi:10.1039/c7sc04798c
Wang, W., Wu, C., Yang, C., Li, G., Han, Q. B., Li, S., et al. (2018) A dual-functional luminescent probe for imaging H2S in living zebrafish and discrimination hypoxic cells from normoxic cells probe for imaging H2S in living zebrafish and discrimination hypoxic cells from normoxic cells. Sens. Actuators B Chem., 255, 1953–1959. doi:10.1016/j.snb.2017.08.222
Windmoller, C. C., Silva, N. C., Andrade, P. H. M., Mendes, L. A., and do Valle, C. M. (2017). Use of a direct mercury analyzer® for mercury speciation in different matrices without sample preparation. Anal. Methods 9, 2159–2167. doi:10.1039/c6ay03041f
Wu, C., Wu, K. J., Kang, T. S., Wang, H. M. D., Leung, C. H., Liu, J. B., et al. (2018). Iridium-based probe for luminescent nitric oxide monitoring in live cells. Sci. Rep. 8, 12467. doi:10.1038/s41598-018-30991-9
Yin, Y., Chen, M., Peng, J., Liu, J., and Jiang, G. (2010). Dithizone functionalized solid phase extraction– displacement elution high performance liquid chromatography–inductively coupled plasma mass spectrometry for mercury speciation in water samples. Talanta 81, 1788–1792. doi:10.1016/j.talanta.2010.03.039
Keywords: functional gold nanoparticles, polyethyleneimines, vancomycin-loaded gold nanoparticles, fluorescent gold nanoparticles, fluorometric sensing
Citation: Tiwari AK, Yadav HP, Gupta MK, Narayan RJ and Pandey PC (2023) Synthesis of vancomycin functionalized fluorescent gold nanoparticles and selective sensing of mercury (II). Front. Chem. 11:1238631. doi: 10.3389/fchem.2023.1238631
Received: 17 June 2023; Accepted: 24 July 2023;
Published: 01 August 2023.
Edited by:
Wenyue Gao, Shandong University, ChinaReviewed by:
Xiaojiang Xie, Southern University of Science and Technology, ChinaCopyright © 2023 Tiwari, Yadav, Gupta, Narayan and Pandey. This is an open-access article distributed under the terms of the Creative Commons Attribution License (CC BY). The use, distribution or reproduction in other forums is permitted, provided the original author(s) and the copyright owner(s) are credited and that the original publication in this journal is cited, in accordance with accepted academic practice. No use, distribution or reproduction is permitted which does not comply with these terms.
*Correspondence: Roger J. Narayan, cm9nZXJfbmFyYXlhbkB1bmMuZWR1; Prem C. Pandey, cGNwYW5kZXkuYXBjQGlpdGJodS5hYy5pbg==
Disclaimer: All claims expressed in this article are solely those of the authors and do not necessarily represent those of their affiliated organizations, or those of the publisher, the editors and the reviewers. Any product that may be evaluated in this article or claim that may be made by its manufacturer is not guaranteed or endorsed by the publisher.
Research integrity at Frontiers
Learn more about the work of our research integrity team to safeguard the quality of each article we publish.