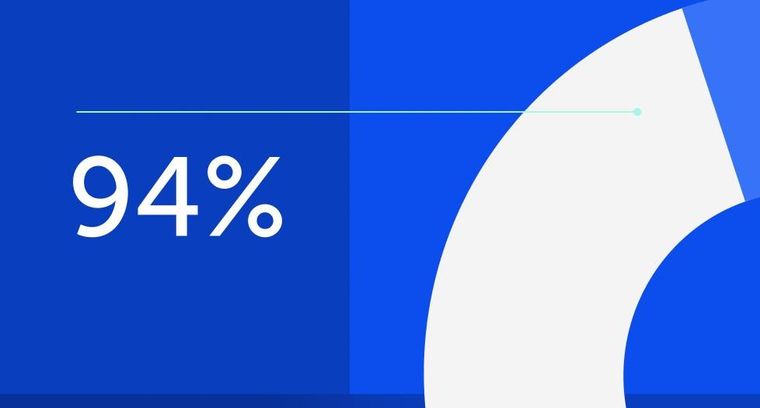
94% of researchers rate our articles as excellent or good
Learn more about the work of our research integrity team to safeguard the quality of each article we publish.
Find out more
ORIGINAL RESEARCH article
Front. Chem., 17 August 2023
Sec. Medicinal and Pharmaceutical Chemistry
Volume 11 - 2023 | https://doi.org/10.3389/fchem.2023.1238346
Aqueous extracts of Marrubium vulgare L. (M. vulgare) are widely used in traditional medicine for their therapeutic effects. Hence, this study aims to evaluate in vitro, in vivo, and in silico the biological activities of M. vulgare aqueous extract to further support their traditional use. Qualitative phytochemical tests of M. vulgare extracts showed the presence of primary and secondary metabolites, while quantitative analyses recorded revealed the contents of total phenols, flavonoids, and tannins, with values of 488.432 ± 7.825 mg/EAG gallic acid extract/g, 25.5326 ± 1.317 mg/EQ Quercetin extract/g and 23.966 ± 0.187 mg/EC catechin extract/g, respectively. Characterization of the phytochemical constituents of the extract revealed the presence of catechin and maleic acid as the most abundant while the evaluation of the antioxidant power revealed that the extract possesses significant antioxidant capacity, antimitotic potential, and antimicrobial properties against Streptococcus agalactiae and Staphylococcus epidermidis among many others. The antidiabetic activity of the extract showed a potent antihyperglycemic effect and a significant modulation of the pancreatic α-amylase activity as revealed by both in vitro and in vivo analysis, while an in silico evaluation showed that chemicals in the studied extract exhibited the aforementioned activities by targeting 1XO2 antimitotic protein, W93 antidiabetic protein and 1AJ6 antimicrobial protein, which revealed them as worthy of exploration in drug discovery odyssey. Conclusively, the result of this study demonstrates the numerous biological activities of M. vulgare and gives credence to their folkloric and traditional usage.
For decades, medicinal herbs have been identified as a rich source of natural compounds that can be used to treat and prevent a wide range of illnesses (Bencheikh et al., 2021). According to the World Health Organization, 80% of people worldwide use traditional medicine including plants (Singh et al., 2018). Lesser side effects, efficacy, economic feasibility, and accessibility are the most important reasons for these practices (Ekor, 2014; Taib et al., 2020). Interestingly, the use of medicinal herbs in drug therapies has recently gained prominence, with about 25% of the total drugs used in developing countries being derived from plants (Hosseinzadeh and Nassiri-Asl, 2015; Alami Merrouni et al., 2021).
Noteworthy, many aromatic and medicinal plants have been identified as possessing an array of pharmacological activities such as antioxidant, anti-inflammatory, anticancer, antidiabetic, and antimicrobial activities, with these activities being majorly conferred by the secondary metabolites present in these plants (Yuan et al., 2016). Among the plethora of medicinal plants distributed across different geographical locations of the world, some belonging to certain families including the Lamiaceae family often stand out due to the rich pharmacological properties of plants from such families. Natively, the body’s antioxidant defense system is responsible for assuaging the effect of reactive oxygen species (ROS), however, the imbalance between the production of the enzymes that mediate the process and the production of ROS result in OS (Porto et al., 2013). Hence, the intake of exogenous antioxidants, particularly those from medicinal plants, is often recommended.
Diabetes, defined by persistent hyperglycemia, is a metabolic syndrome that develops when the pancreas either secrete insufficient insulin or the body no longer effectively utilizes secreted insulin (Deepthi et al., 2017; Sisodia and Sisodia, 2018). Interestingly, OS has also been reported to mediate the pathogenesis and progression of diabetes (Bouhrim et al., 2021; Ouassou et al., 2021). Hence, ameliorating OS is considered a therapeutic strategy for the treatment of diabetes (Dra et al., 2019). Similarly, the inhibition of enzymes that play pertinent roles in the metabolism of glucose is also a widely utilized approach for the management of type 2 diabetes. Notable among these enzymes are the human pancreatic alpha-amylase (HPA) which mediates the initial step involved in the catabolism of glucose by functioning in the hydrolysis of the glycosidic bonds present in starch. Currently, there exist several inhibitors of this enzyme in clinical usage, and they were reported to be efficacious. However, their use is often concomitant with several side effects including nausea, bloating, liver disorder, and weakness among many others (Bouyahya et al., 2021). Cancer remains a major health challenge globally, causing significant morbidity and mortality (Xiaomei and Herbert, 2006; Bencheikh et al., 2022b). Traditional treatment approaches like chemotherapy and surgery face limitations, including toxicity and drug resistance (Bencheikh et al., 2022b; Fakchich and Elachouri., 2021). Therefore, the search for novel drugs against cancer continues. Medicinal plants are a valuable resource, as they have contributed to the development of many clinically used drugs. Similarly, antimicrobial resistance has become a global public health concern, leading to increased morbidity, mortality, and healthcare costs. Acquired resistance mechanisms involve genetic mutations, horizontal gene transfer, and misuse of antimicrobial drugs (Onesti et al., 2015).
Marrubium vulgare L. is an annual or perennial herb from the Lamiaceae family and has been reported to possess an array of pharmacological properties including anti-inflammatory and hemostatic effects, as well as antihypertensive, sedative potential, and antimicrobial properties (Yousefi et al., 2014). Furthermore, it has also been reported to possess a panoply of bioactive compounds including premarrubiin, peregrinol, vulgatol, marrubenol, marrubiol, verbascoside, and forsythoside B among many others (Karryev et al., 1976; Pukalskas et al., 2012; Yousefi et al., 2013).
As a result of the multi-pharmacological properties of this plant, it has emerged as an attractive option for exploration for the discovery of phyto-compounds that can be used in the treatment of a wide range of diseases and disease-inducing conditions. Prominent among the biological phenomena that drive diseases is oxidative stress (OS). Notably, OS has been identified as a driver of several diseases, including diabetes, Alzheimer’s disease, Parkinson’s disease, cancer, and cardiovascular diseases (Ladoh Yemeda et al., 2014; Loizzo et al., 2017).
M. vulgare L. has been reported to possess antioxidant properties in folkloric use, and the antioxidant power of a plant often varies based on the source of the plant. Medicinal plants, such as M. vulgare L., are being extensively studied for their bioactive compounds, as they offer potential as inhibitors and therapeutic agents. Developing alternatives to existing drugs is crucial in combating antimicrobial resistance, and medicinal plant compounds are being explored in this quest (Djahra, A. B. et al., 2013).
Therefore, this study aims to investigate the antioxidant, antimitotic, antimicrobial, and antidiabetic activities of M. vulgare leaves from Morocco. Additionally, the study aims to characterize the phytochemical constituents present in these leaves. By examining these properties and compounds, we aim to contribute to the ongoing search for effective treatments for cancer and antimicrobial-resistant infections.
The plant material utilized in this study consists of the leaves of M. vulgare and they were harvested from the region of Fez Meknes (Moulay Idriss Zerhoun) in April 2020 during the flowering period of the plant. Subsequently, the plant samples were carefully placed in ventilated bags and subjected to a thorough cleaning to eliminate any potential contaminants or debris that could compromise the purity of the plant samples, after which its identity was authenticated at the Department of Botany of the Scientific Institute of Rabat. Furthermore, the plant samples were subjected to a drying process to remove excess moisture; this drying was carried out in a well-ventilated room and away from sunlight at a temperature range of 30°C–40°C for 10 and 15 days. Table 1 presents information on the region where the plant was harvested while Figure 1 depicts Morphological appearance of the harvested plant.
FIGURE 1. Morphological appearance of the M. vulgare during harvest (April 2020, Region Fez Meknes, Morrocco).
Briefly, 5 g of the dried leaves were weighed into a pre-dried and tared crucible, after which the crucibles were placed into an oven at 103°C for 24 h. Subsequently, the crucibles were allowed to cool in a desiccator and were re-weighed to determine the final mass. This analysis was conducted in accordance with the guidelines outlined in the French association of standardization (AFNOR) standard (NF - V03-402 1985) (AFNOR, 1985). The results obtained from the analysis were expressed as a percentage of the dry matter content using the formula below:
Where:
TH%: humidity level
M0: initial mass of the plant leaves;
M1: mass obtained after drying.
To determine the pH of the leaves, 2 g of the leaves sample was placed in 10 ml of hot-filtered water. The resulting solution was purified by filtering and was allowed to undergo cooling. Subsequently, the electrode of a pH meter was immersed in the filtrate to determine its pH value.
The titratable acidity of the leaf samples, which represents the total acidity of a solution and encompasses the free minerals and organic acids, was determined by weighing 10 g of the powdered leaf samples into 50 ml of boiling distilled water and was subjected to stirring for 15 min. Subsequently, the volume of the resulting solution was adjusted to 100 ml using filtered water and was thereafter filtered. The filtrate was subjected to titration using a 0.01 N NaOH solution, with the addition of a few drops of phenolphthalein indicator. Noteworthy, the titration process was continued until a noticeable color change signified by the presence of a persistent pink hue occurred (Bergeron, 1995). The titer values were converted into its citric acid equivalent using the conversion factor below:
The method employed in this study was based on the calcination of the leaf samples in a muffle furnace at a temperature of 550°C until white ashes of constant weight were achieved in accordance with AFNOR standard (AFNOR, 1972). The organic matter content was quantified according to the equation below:
Where
MO%: Organic material
W1: Weight of capsule and sample before calcination
W2: Weight of capsule and sample after calcination
TS: Test simple
Following the determination of the organic matter content, the ash content was determined as follows using the equation below:
Ash %: Ash content
The leaf samples were investigated for the presence of heavy metals including arsenic (As), cadmium (Cd), chromium (Cr), iron (Fe), lead (Pb), antimony (Sb), and titanium (Ti). Noteworthy, there exists a specific contamination limit standard, which serves as a regulatory guideline, however, some exceptions are provided for medications whose primary ingredients have been established to accumulate significant levels of Cd. To determine the levels of the heavy metals, aqua regia (HNO3 + 3HCl) and the normalized mineralization, which helps to circumvent issues related to sample representativeness, were employed in accordance with the AFNOR guideline. To perform the analysis, aqua regia was firstly prepared by mixing 1 mL of nitric acid (HNO3; 99%) with 2 ml of hydrochloric acid (HCl; 37%) and heated to a temperature of 200°C, after which it was allowed to cool for 2 hours. Subsequently, the supernatant was carefully extracted and filtered through a 0.45 µm membrane filter. To ensure accuracy, the filtrate is then supplemented with 15 mL of distilled water. Following the preparation of the aqua regia, 0.1 g of the crushed plant leaves was combined with 3 mL of the prepared aqua regia, after which the concentration of the heavy metals was determined by using the inductively coupled plasma atomic emission spectroscopy (ICP-AES) utilizing the Ultima 2 Jobin Yvon instrument in accordance with protocols established in Özcan (Musa Özcan, 2006).
The antimicrobial activity of the plant extract was evaluated against nine bacterial strains and seven fungal strains known for their high resistance, invasiveness, and pathogenic properties. The bacterial strains are Staphylococcus epidermidis, Enterobacter cloacae, Streptococcus agalactiae (B), Klebsiella pneumoniae, wild Escherichia coli, ESBL Escherichia coli, Proteus mirabilis, Pseudomonas aeruginosa, and Staphylococcus aureus BLACT, while the fungal strains included Candida albicans, Candida krusei, Candida tropicalis, Candida parapsilosis, Candida dubliniensis, Saccharomyces cerevisiae and Aspergillus niger. Noteworthy, these microorganisms were obtained from the Mohamed V-Meknes Provincial Hospital and were initially stored in −80°C 20% glycerol stock. Before the utilization of the microorganisms, they were subcultured and grown on Mueller Hinton agar and Sabouraud agar for the bacterial and fungal strains, respectively.
30 g of powdered M. vulgare leaves was introduced into a 1-liter Erlenmeyer flask containing 600 mL of purified water. The mixture obtained was subjected to heat at 70°C for 1 h, after which it was filtered. Subsequently, the filtrate was subjected to an evaporation process under reduced pressure after cooling at room temperature. The residue obtained after the cooling was oven-dried at 50°C and subsequently weighed to calculate the extraction yield (Chavan et al., 2001).
To determine the phytochemicals present in M. vulgare leaves, various solvents including water, chloroform, methanol, and petroleum ether were utilized. To determine the presence of alkaloids, Dragendorff and Mayer reagents were employed, while Hydrochloric acid and isoamyl alcohol were used to detect the presence of catechin tannins, and tiasny’s reagent, sodium acetate, and ferric chloride were used to characterize gallic tannins. Also, the presence of sterols and triterpenes was detected using acetic anhydride and strong sulfuric acid, Magnesium chips, isoamyl alcohol, and diluted hydrochloric alcohol were used to detect the presence of flavonoids, while chloroform, dilute ammonia, and hydrochloric acid was used for the detection of quinone substances (Tamert et al., 2017).
To determine the number of total polyphenols in the M. vulgare extract, the Folin-Ciocalteu reagent was employed. 500 µL of freshly prepared 0.1X Folin-Ciocalteu reagent and 2 mL of sodium carbonate solution (20% Na2CO3) were mixed with 100 µL of the extract. The resulting mixture was incubated for 30 min at room temperature and the absorbance was measured at a wavelength of 760 nm with referce to a standard (Ali-Rachedi et al., 2018).
The quantification of the flavonoid content of M. vulgare extract was conducted using the AlCl3 method, as described by Barros et al. (Barros et al., 2011). To perform the quantification, 2 μL of the extract was mixed with 10 μL of 10% aluminum chloride solution and 2 mL of distilled water, after which 3 mL of absolute methanol was added to the mixture. Subsequently, the mixture was incubated for 2 hours in the dark, and the absorbance was measured at 433 nm.
50 µL of M. vulgare leaves extract was added to 1,500 µL of 4% vanillin/methanol workaround and subjected to vigorous mixing. Subsequently, 750 µL of concentrated HCl was added to the mixture, and the resulting mixture was allowed to react at room temperature for 20 min. Ultimately, the absorbance of the mixture formed after the reaction was measured at 550 nm concerning a blank (Lorrain et al., 2011).
The phytochemicals present in the M. vulgare extract were chromatographically characterized using high-performance liquid chromatography (HPLC) by employing an UltiMate 3000 system. Notably, the system contained a reverse-phase C18 column (250 mm × 4 mm, id 5 μm, Lichro CART, Lichrospher, Germany) with a sample changer, and the tests were conducted at a maintained temperature of 5°C. The temperature of the column was maintained at 40°C, while the mobile phase consisted of ultrasonically degassed solutions of 0.1% formic acid in water and 0.1% formic acid in acetonitrile and possessed gradient composition as follows: starting with 2% B at 0 min, followed by a linear change to 30% B at 20 min, 95% B at 25 min, and returning to 2% B at 26 and 30 min. Furthermore, the flow rate was set at 1 ml/min, and the injection volume was 20 µL. Detection of the compounds was performed using a Maxis Impact HD in MS/MS mode with negative electrospray ionization, while broadband collision-induced dissociation was employed for fragmentation. Additionally, UV detection was carried out using an L-2455 diode array detector, scanning in the wavelength range of 190–600 nm. Specific acquisition wavelengths of 280, 320, and 360 nm were utilized for analysis. Various parameter values were set for optimal performance, including a capillary voltage of 3000 V, the drying gas temperature of 200°C, dry gas flow rate of 8 L/min, vaporizing gas pressure of 2 bar, and an offset plate of −500 V. Nitrogen was used as the nebulization and desolvation gas, and mass spectrometry data were collected within the range of 50–750 m/z. Data acquisition and evaluation were performed using the Sysremsoftware program, specifically designed for chromatography data analysis in the Thermo ScientificTM ChromeleonTM 7.2 platform.
The free radical scavenging ability of the plant extract was determined using (2,2-diphenyl-1-picrylhydrazyl) assay protocol as detailed in (Bencheikh, et al., 2022a). To perform this assay, 200 µL of the extract at varying concentrations were placed in mixed with 2.8 mL of an ethanolic solvent containing DPPH (2.4 mg/100 mL) with an estimated absorbance of 0.6 and 0.7 at 517 nm. Subsequently, the resulting mixture was incubated for 30 min at ambient temperature and the absorbance was measured at 517 nm. To serve as positive controls for the experiment, solutions of ascorbic acid and butylhydroxyanisole (BHA) at the same concentrations as the plant extract was prepared while a blank experiment was also carried out using absolute ethanol. The results of the analysis were expressed as the percentage reduction in DPPH* (AA %) according to the equation below:
Where:
AA%: Inhibition percentage;
AControl: Absorbance of the mixture, which contains only the radical DPPH solution;
ASample: Absorbance of the samples to be assessed solution in the existence of DPPH; The relationship between the concentration of the plant extract and the percentage modification of the antioxidant activity (percentage of inhibition) was plotted on a graph from which the IC50 was determined.
The Iron reduction ability of M. vulgare extract was investigated using FRAP assay using the protocol described by Zovko et al. (Koncic et al., 2010). To perform the analysis, 2.5 mL of 1% potassium ferricyanide [K3Fe(CN)6] solution and 2.5 mL of phosphate buffer solution (pH = 6.6) were mixed with 0.5 mL of varying concentrations of the extract (same concentrations used in the DPPH test). The resulting mixtures were incubated in a water bath at 50°C for 20 min, after which the reaction was terminated by adding 2.5 mL of 10% trichloroacetic acid. Subsequently, the mixture was subjected to centrifugation at 3,000 revolutions per minute for 10 min. Aliquots from each concentration previously utilized were mixed with 2.5 mL of purified water and 0.5 mL of 0.1% FeCl3 solution. The absorbance of the mixtures was measured at 700 nm against a blank solution prepared using the same protocol, with distilled water replacing the plant extract. The calibration of the spectrophotometer was done using the blank solution, while the absorbance of standards including ascorbic acid, BHA, and BHT was also measured and compared.
The TAC of the extract was determined by evaluating its ability to reduce molybdenum (VI) to molybdenum (V) via a reaction that leads to the formation of a green-colored phosphate/Mo(V) complex under acidic pH conditions. To perform this assay, 100 μL of M. vulgare decoction was mixed with 3 ml of a solution containing sulfuric acid (0.6 N), sodium phosphate (28 mM), and ammonium molybdate (4 mM). The tubes containing the mixture were then incubated at 95°C for 90 min and were allowed to cool to room temperature. Subsequently, the absorbance was measured at 695 nm against a blank that contains only the reaction mixture without the plant extract. The TAC of the extract was expressed in milligrams of ascorbic acid equivalents per gram of extract (mg AAE/g) (Prieto et al., 1999).
The evaluation of the antimitotic activity of M. vulgare extract was done against Lepidium sativum seeds by preparing different concentrations of the extracts by dilution with distilled water. Initially, a 10 mg/ml concentration solution was prepared by diluting the extracts in 4 ml of distilled water. Subsequently, four dilutions were prepared with concentrations of 1,000, 100, 10, and 1 μg/mL. Control solutions including distilled water, colchicine (2.5 mg/ml), and methotrexate (5 mg/ml) were also prepared. Subsequently, these solutions were utilized to soak the watercress seeds for the assessment of antimitotic activity. After 72 h, the antimitotic activity of the extracts was determined by treating the Lepidium sativum seeds in Petri dishes with the respective plant decoction suspensions after which the length of the germinated Lepidium sativum rootlets was then measured using sterile forceps (Kumar and Singhalb, n.d.).
The activity of the extract is assessed through the quantification of the percentage inhibition of cell growth. Noteworthy, this quantification involved comparing the growth of cells treated with the extract to that of a control batch by employing the formula below:
Where:
LC: length of control rootlets
LT: length of treated rootlets
The mitosis index was determined by comparing the size of shoots from seeds soaked in the extract with those soaked in water (negative control). The measurements were conducted after 144 h of incubation in a dark and light-free environment, ensuring optimal conditions for observation and analysis (Mbayo et al., 2016). The value of the mitosis index was then determined using the formula below:
where:
Sextract (mm): Size of soaked seeds soaked in the extract;
Swater (mm): Size of soaked seeds soaked in water.
The Minimum Inhibitory Concentration (MIC) of the M. vulgare extract was determined using the microdilution method in a 96-well microplates, in accordance with the protocol utilized in the study by Kotan et al. (Kotan et al., 2008). The MIC represents the lowest concentration of the plant extract which visibly inhibit the growth of the microorganism being evaluated. To determine the MIC, standard solutions of the extracts were prepared by dilution in 10% dimethyl sulfoxide (DMSO) to obtain concentrations of extracting ranging from 75 to 2.35 mg/ml. For the evaluation of the bacteria susceptibility, the dilutions were incorporated into Mueller-Hinton basal medium, while for fungal susceptibility testing, the Sabouraud bouillon medium was utilized. Each well of the microplate was filled with 100 µL of the respective dilution, and subsequently, 100 µL of the inoculum containing a microbial count of 106 colony-forming units per milliliter (CFU/ml) was sequentially introduced into each well. The microplate was subjected to a 24-h incubation period at 37°C after which 10 µL of resazurin, a colorimetric indicator of bacterial growth, was introduced into each well. Subsequently, the microplates were further incubated for 2 hours at 37°C, after which color change from deep violet to pink, was monitored as it indicates the absence of microbial growth, signifying the MIC. Noteworthy, the experimental setup included growth and sterility control wells and the MIC determination process was performed twice for the M. vulgare extract. The determination of the MBC/MFC was performed by aseptically transferring 10 µL of the contents from wells without visible growth onto Mueller Hinton agar (MHA) and Sabouraud agar plates for bacteria and fungi respectively. Subsequently, the plates were incubated for 24 h at 37°C and 30°C for bacteria and fungi respectively, and the MBC and MFC were defined as the lowest concentration of the extract that resulted in a 99.9% reduction in CFU/ml compared to the control. Noteworthy, 250 mg of Terbinafine was utilized as the standard antifungal by dissolving in 10% DMSO. The evaluation of the antimicrobial efficacy of the varying concentrations of the extract was done by calculating the MBC/MIC or MFC/MIC ratio, where a ratio of less than 4 indicated a bactericidal/fungicidal effect, a ratio greater than 4 indicated a bacteriostatic/fungistatic effect, and a ratio equal to 4 suggested an indeterminate effect (Bissel et al., 2014).
The animal model employed in this study consisted of Wistar rats weighing between 195–260 g and Albino mice weighing between 20–30 g. The animals were housed in a dedicated animal facility located within a biological laboratory. The housing conditions provided a controlled environment with a photoperiod of 12 h of light followed by 12 h of darkness, and a temperature maintained at 22°C. The rodents were kept under standard breeding conditions, with unrestricted access to food and water.
The procedures used to perform this study agree with the international guidelines used for the use of laboratory animals. The ethical committee of the Faculty of Sciences of Meknes, Morocco, revised and approved this work under the ethical clearance 04/2019/LBEAS.
The dosage of M. vulgare extract which guarantees efficacy without toxicity in the short term evaluated in normal mice. The experimental design involved the use of albino mice weighing between 20–35 g, which were subjected to a 14-h fasting period before the experiment. The mice were randomly divided into five groups, with each group consisting of six mice (three males and three females). The mice were subjected to treatment based on their groups as follows:
• Group 1: This group served as the control group, and they were orally administered a dose of pure water at 10 mL/kg.
• Groups 2, 3, and 4: Those groups were orally administered M. vulgare extract at concentrations of 0.5 g/kg, 1 g/kg, and 2 g/kg, respectively.
Noteworthy, before the administration of the extract, the mice were weighed to ensure accurate dosage. Following the administration of the extract, the mice were meticulously monitored for 10 h to observe any signs of toxicity and were assessed regularly for any adverse effects or symptoms of poisoning. Subsequently, daily observations were carried out over 14 days to detect any delayed toxicity or long-term effects. The animals were carefully handled throughout this period and the experiment was conducted in strict compliance with the guidelines of the Organisation for Economic Co-operation and Development (OECD) (Tchoumtchoua et al., 2014).
The antihyperglycemic effect of the plant was evaluated in vivo by utilizing the oral glucose tolerance assay as described by Bouhrim et al. (Bouhrim et al., 2021). Normal rats were randomly distributed into three groups, with each group consisting of six rats (three males and three females). The groups were designated as follows:
Control group: Rats in this group were administered a dose of filtered water at 10 mL/kg.
Test group: Rats in this group were orally administered the plant extract at a dosage of 0.8 mL/kg.
Treated groups: Rats in this group were orally administered either the decocted extract at a concentration of 400 mg/mL or glibenclamide at a concentration of 2 mg/mL.
The evaluation of the oral glucose tolerance was conducted as follows: the measurement of the baseline glycemia was done at time zero, the sequel to the administration of their respective regimens (filtered water, M. vulgare, or glibenclamide). Subsequently, another blood glucose measurement was taken after 30 min, following the administration of an overload of D-glucose (2 mg/kg). Blood glucose levels were further monitored at 60, 90, and 150 min post-glucose overload. The oral glucose tolerance test was conducted in the following manner: Initially, baseline glycemia was measured at t0, immediately after the administration of the respective treatment (filtered water, plant extract, or glibenclamide). Subsequently, another blood glucose measurement was taken after 30 min, following the administration of an overload of D-glucose (2 mg/kg). Blood glucose levels were further monitored at 60, 90, and 150 min post-glucose overload. The oral glucose tolerance test was performed as follows: glycemia was measured at t0, immediately after treatment of the tested product (filtered water, plant extracts or glibenclamide). Another blood glucose measurement was taken 30 min later, just after the animals were overloaded with D-glucose (2 mg/kg). Blood glucose levels were then monitored at 60, 90, and 150 min.
The effect of the M. vulgare extract on the enzymatic activity of pancreatic α-amylase was investigated following the method described by Bouhrim et al. (Bouhrim et al., 2021). To perform the analysis, 200 µL of the plant extract solution at various concentrations (0.89, 0.45, 0.22, 0.11, and 0.06 mg/mL) or the acarbose solution at different concentrations (1, 0.8, 0.6, 0.4, and 0.2 mg/mL) was combined with 200 µL of a phosphate buffer solution (0.02 M, pH = 6.9). Subsequently, 13 IU of pancreatic α-amylase enzyme solution was added to all tubes, excluding a tube, which contained only the phosphate buffer instead of the enzyme solution. The tubes were then pre-incubated at 37°C for 10 min. Following the pre-incubation, a 200 µL volume of starch solution was introduced to each tube, and the mixture was further incubated at 37°C for 15 min. To halt the enzymatic reactions, 600 µL of DNSA (2.5%) was added to the tubes. The tubes were then immersed in a scalding water bath for 8 min. To terminate the reaction, a heat shock was applied by placing the tubes in an ice bath, followed by the addition of 1 mL of filtered water to each tube. A spectrophotometer was employed to determine the absorption spectrum at 540 nm in comparison to a blank that contained the buffer solution rather than the enzyme solution. The percentage inhibition of each extract and acarbose was assessed using the equation below:
Where;
Ab Control: Absorption of enzyme activity in the absence of an inhibitor;
Ab Test: Enzymatic activity absorption with the plant extract or acarbose.
The inhibitory effect of M. vulgare decoction on pancreatic α-amylase was evaluated in vivo in healthy rats by examining its impact on the activity of the intestinal lumen. Healthy rats weighing between 180–250 g were subjected to a 14-h fasting period and were divided into three groups, with a total of six rats in each group, and an equal distribution of males and females (♂/♀ = 1):
The control group was orally administered filtered water at a dose of 10 mL/kg, while the medicated groups were orally administered the decocted extract of M. vulgare at a dose of 400 mg/kg, or acarbose at a dose of 10 mg/kg. Assessment of the oral starch tolerance was carried out as follows: The test substance (distilled water, aqueous extract, or acarbose) was administered to the rats at time t = 0 min. After a 30-min interval, a blood glucose measurement was taken immediately following the administration of a starch overload (3 g/kg) to the rodents. The subsequent changes in blood glucose levels were monitored at 60, 90, and 120 min (Daoudi et al., 2020).
An in silico evaluation of the compounds derived from the M. vulgare decoction was done against antimitotic, antidiabetic, and antimicrobial targets. Following the identification of the targets, their respective structures were retrieved from the RCSB protein data bank using the PDB IDs 1XO2, 4W93, and 1AJ6. The preparation of the protein before molecular docking was done using BIOVIA’s Discovery Studio Visualizer (Accelrys Software Inc, 2005) and Autodock tools (Morris et al., 2009). Notably, the preparatory steps included the removal of heteroatoms, cognate ligands, and water molecules, and subsequent optimization of the structure. Similarly, the structure of the ligands was drawn in ChemDraw Ultra (CambridgeSoft, 2009a), and was subjected to preparatory steps including energy minimization using Chem3D Pro (CambridgeSoft, 2009b). Subsequently, the ligands were converted to pdbqt files (Zentgraf et al., 2007) using OpenBabel (Morris et al., 2009; Ferreira et al., 2015), while the molecular docking was performed using AutoDock Vina (Yusuf et al., 2008; Trott and Olson, 2010; Eberhardt et al., 2021). Following the formation of the complexes after docking, the interactions between the ligands and the protein were visualized and analyzed using BIOVIA’s Discovery Studio Visualizer.
The data presented in this study are expressed as means ± standard error of measurement (SEM). All statistical analysis was performed using one-way analysis of variance (ANOVA) followed by Tukey’s post hoc test. Noteworthy, significance levels were determined with p < 0.05, p < 0.01, and p < 0.001 considered as statistically significant.
The quality control assessments conducted on the retrieved leaves of M. vulgare included the determination of its moisture content (TH), pH, acidity, ash, and ICP-AVE. The results of this assessment are presented in Tables 2, 3. As evident in Table 2, the TH of M. vulgare leaves was found to be 22.56 ± 0.25%, indicating a high water content in the plant material. The pH of the M. vulgare extract was slightly acidic, with a value of approximately 5.945 ± 0.007, however, the pH value was in compliance with the quality standards of AFNOR (AFNOR, 1977; Kakoma et al., 2014). Determination of the titratable acidity revealed values of 0.1033 ± 0.9212, which was used to evaluate the characteristics, quality, absorption capacity, and solubility of many substances (Kroeze, 2020). Similarly, the percentage of ash in M. vulgare extract which provides information about the mineral content that remains after organic matter is volatilized at high temperatures was found to be 22.562 ± 0.25%. As presented in Table 3, analysis of the heavy metal content of the extract revealed Fe possessed the highest content with a value of 0.5498 mg/g, while Cu was the second-highest with a value of 0.0087 mg/g. Other heavy metals analyzed are presented in Table 3, interestingly, they were all found to be within the permitted range of FAO/WHO regulatory standards. Hence, rendering the extract suitable for direct consumption, as an ingredient in food processing, or for repackaging if necessary.
The qualitative screening of phytochemicals enables the identification of the classes of compounds present in an extract. To this end, qualitative reactions were employed to determine the phytochemicals present in the extract of M. vulgare, and the results are presented in Table 4. The results revealed the presence of primary metabolites including polysaccharides, reducing sugars (glucose and fructose), proteins, and lipids in varying concentrations. Similarly, the presence of secondary metabolites including flavonoids, tannins, mucilages, sterols and triterpenes, coumarins, and saponins were confirmed, however, alkaloids were found to be absent (Table 4). Interestingly, the results of this study are consistent with those of Akther et al. and Fayyad et al. who reported in their study that M. vulgare is rich in gallic tannins, catechin tannins, sterols, and triterpenes and flavonoids (Akther et al., 2013; Fayyad et al., 2014). Tannins and flavonoids are widely explored for their pharmacological properties including antioxidant, antiviral, antitumor, anti-inflammatory, anti-allergic and anti-cancer activities (Khanbabaee and van Ree, 2001; Crozier et al., 2009). Furthermore, numerous in vitro and in vivo studies show that polyphenols can modulate carbohydrate metabolism and have antidiabetic properties (Hanhineva et al., 2010; Martel et al., 2017).
Quantitative analysis of the classes of phytochemicals present in the aqueous extract of M. vulgare was conducted and the results are presented in Table 5. Notably, the yield of the aqueous extract was found to be significant, with a value of 17.673 ± 0.48. Classes of phytochemicals present in the extract include a high content of polyphenols (488.432 ± 7.825 mg/EAG/g), flavonoids (25.5326 ± 1.317 mg/EQ/g), and tannins (23.966 ± 0.187 mg/EV/g). The results of the quantitative analysis were found to be consistent with that of the qualitative analysis, with the values also being much higher than that reported by Matkowski et al. and Wojdylo et al. whose study revealed that the methanolic extracts of M. vulgare had polyphenolic contents of 63.4 ± 1.7 mg/EAG/g and 3.86 ± 0.05 mg/EAG/g respectively (Wojdyło et al., 2007; Matkowski et al., 2008). Conversely, the flavonoid content of the extract was found to be lower than that reported in the study of Elberry et al. in which they reported a value of 15.53 ± 0.67 mg/EAG/g study (Elberry et al., 2015). Variations in the values could be due to many intrinsic and extrinsic factors like genetic and environmental factors, and this could influence the quality and quantity of chemical composition of phenolic compounds in plant material. Other factors related to harvesting, drying, and processing may also be responsible (Ouedraogo et al., 2021).
The identification of the phenolic composition of the aqueous of M. vulgare leaves was conducted using HPLC/UV-ESI-MS analysis. The resulting chromatographic profile, which reveals the presence of 19 chemical compounds presented in Table 6, is depicted in Supplementary Figure S1 (supplementary provided). Notably, the major phyto-compounds present in the extract were catechin (12.03%), maleic acid (11.18%), luteolin (10.55%), apigenin (10.55%), salicylic acid (8.01%), biotin (7.24%), caffeic acid (6.36%), and vanillic acid (5.03%), these eight compounds accounted for a significant proportion, totaling 70.95% of the total identified compounds (96.78%). Interestingly, a study by Benzidane et al., 2020 on the methanolic extract of M. vulgare leaves collected in Algeria revealed the presence of ferulic acid (Nadia Benzidane, Ridha Smahi, Boudjemaa Zabouche, Abdelhalim Makrouf, 2020), however, the compounds were found to be present in the current study with a very low percentage (0.77%). They also reported the presence of catechin in the hexane extract, which is in tandem with the compounds identified in our aqueous extract. A recent study in Saudi Arabia reported that the methanolic extract of the leaves of M. vulgare contains luteolin-7-O-D-glucoside as the third major compound, however, luteolin was found to be present in this and was not linked to any sugar moiety. Furthermore, a study by Rezgui et al. in Tunisia corroborated the presence of several phenolic molecules in M. vulgare leaves, including apigenin, ferulic acid, coumaric acid, caffeic acid, and luteolin (Rezgui et al., 2020), which are in line with the compounds identified in our study. These compounds have been extensively investigated for their pharmacological properties, such as anticancer, anti-inflammatory, antibacterial, antiviral, and antiseptic activities, as documented in previous scientific studies (Karryev et al., 1976; Pukalskas et al., 2012; Yousefi et al., 2013). Noteworthy, the observed variation in the reported compared to that of other studies could be attributed to intrinsic and/or extrinsic factors.
The assessment of the antioxidant activity of plant extract was done using three different methods namely DPPH, FRAP, and TAC tests, the results of which are presented in Table 7. The results revealed a positive correlation between the inhibitory effect of the free radical DPPH and the quantity of the M. vulgare extract. The antioxidant activities of the extract and ascorbic acid were quantified by the determination of their IC50, which represents the concentration of the extract required to reduce 50% of the DPPH free radical. Noteworthy, a lower IC50 value indicates a higher antioxidant effect. Interestingly, the results of this study revealed that the decoction of M. vulgare exhibited significant antioxidant power (IC50 = 1.1815 ± 0.621 mg/mL), although lower than that of the ascorbic acid (IC50 = 0.323 ± 0.411 mg/mL). The results of this study differ slightly from those reported in a study by Boudjelal et al. in which an IC50 value of 0.49 ± 0.517 mg/mL was reported for the aerial part of the methanolic extract of M. vulgare harvested in M'Sila, southern Algeria (Boudjelal et al., 2013). Evaluation of the reducing power via measuring its ability to transfer an electron or a hydrogen atom, thereby reducing Fe (III) to Fe (II) in the presence of the K3Fe(CN)6 complex, was done and the results are presented in Figure 3B. Notably, a remarkable increase in the reducing power of M. vulgare was noticed in concomitant with the increasing concentrations of the extract. The ascorbic acid and the decoction exhibited effective concentrations with respective values of EC50 = (0.15 ± 0.24) mg/mL and EC50 = (1.5 ± 0.203) mg/mL. An earlier study on M. vulgare extract reported that it possessed significant antioxidant activity, with an EC50 of 0.51 ± 0.24 mg/mL (Ghedadba et al., 2014), this activity was attributed to the phenylpropanoid glycosides, which are reputed for their potent antioxidant activities. The TAC of M. vulgare extract was determined by the phosphomolybdate test which is based on the ability of the extract to reduce molybdenum Mo (VI) present as molybdate ions MoO42−, to molybdenum Mo(V) MoO42+, and the subsequent formation of a green complex [phosphate Mo(V)] at an acidic pH. The results of this study revealed that the decoction shows a TAC of 50.550 ± 3.746 mg EAA/gE, hence, indicative of the remarkable antioxidant capacity of the aqueous extract. However, the results of this study were not in tandem with that reported in a study by Amessis-Ouchemoukh et al., obtained from studying the TAC of the methanolic and acetone extracts of the aerial part of M. vulgare which showed high TAC with 101.82 ± 1.75 and 85.71 ± 1.35 (Amessis-Ouchemoukh et al., 2014). The antioxidant activities demonstrated by the aqueous extract of M. vulgare, as assessed through DPPH, FRAP, and TAC tests, hold potential applications in the food and pharmacological industries. These findings contribute to the understanding of the antioxidant properties of M. vulgare and its potential as a natural source of antioxidants.
The antimitotic effect of M. vulgare extract was carried out by the Lepidium sativum method which was proposed in 1972 by GAGIU for a preliminary selection of molecules acting on plant growth without presuming their precise place of action. Several authors have contributed to the study of the validity of this test, and it is widely used as it is relatively simple and fast. Additionally, the seed of Lepidium sativum has only one rootlet, the appreciable growth of which allows easy measurement. This test is conducted by measuring the length of the root of a germinated Lepidium seed placed in a medium containing the molecules to be tested. The monitored percentage of growth inhibition is estimated by comparison with a control. Colchicine, with an already-known antimitotic effect, was used as a positive control. The antimitotic activity of the M. vulgare decoction was evaluated by determining the inhibition index. As evident in Table 8, an increase in the concentration of the extract was concomitant with an increase in the percentage inhibition, and the extract was found to possess better antimitotic activity compared to colchicine with 90.184 ± 0.164% and 95.37 ± 0.19% at 10 mg/mL and 1.5 mg/mL respectively. Conversely, the IC50 values obtained for the decocted extract were higher for colchicine compared to the decoction with the values 1.8 ± 0.11 mg/mL and 3.688 ± 0.12 mg/mL respectively. The results of the mitotic index of the M. vulgare extract are presented in Table 9, with the extract exhibiting a higher value compared to the colchicine but lower compared to the negative control (distilled water). It is worth noting that a smaller mitotic index indicates a higher activity. Overall, the results of this study render the extract of M. vulgare fit for exploration as an antimitotic.
Evaluation of the MIC was conducted using the microdilution method, and the results are presented in Table 10. Notably, all the strains examined exhibited varying degrees of sensitivity to the aqueous extract of M. vulgare, as evident by the variability in the MIC values obtained. The classification of MICs was done based on the established criteria described in previous studies (Sartoratto et al., 2004; Duarte et al., 2007; de Oliveira Pedro et al., 2013; Wang et al., 2017). The antibacterial effect of M. vulgare aqueous extract was classified as high (MIC 75 μg/mL) against Klebsiella pneumoniae, Escherichia coli, Pseudomonas aeruginosa, and Staphylococcus epidermidis. A moderate antibacterial effect (MIC 37.5 μg/mL) was observed against Streptococcus agalactiae, while a low antibacterial effect (MIC 18.75 μg/mL) was observed against Proteus mirabilis. It is worth noting that the MIC values indicate the lowest concentration of the extract that inhibited the visible growth of the bacterial strains. Furthermore, the MBC values were found to be less than 75 μg/mL for all tested bacterial strains.
The antifungal impacts of M. vulgare aqueous extract were also investigated on certain fungal strains and the results are presented in Table 11. The results of the study revealed the extract exhibited significant antifungal activity and MFC of 37.5 μg/mL against the condidat strains; Candida tropicalis, Candida krusei, Candida albicans, Candida parapsilosis, Saccharomyces cerevisiae and Aspergillus niger, except Candida dubliniensis which was inhibited by a higher concetration with a MIC value of 75 μg/mL. Notably, the lowest MFC value of 9.375 μg/mL was observed against Candida parapsilosis, Saccharomyces cerevisiae, Aspergillus niger, which is indicative of their strong antifungal activity. A moderate MFC value of 18.75 μg/mL was observed against Candida krusei while the highest MFC values were found to be less than 75 μg/mL against Candida tropicalis, Candida dubliniensis, and Candida albicans.
This study demonstrated the antimicrobial properties of the aqueous extract of M. vulgare against the tested bacterial and fungal strains, this is attributable to the active constituents of this plant (Dib et al., 2021). Previous studies conducted on M. vulgare have identified catechin as the main component and it is present alongside a variety of bioactive substances including alkaloids, steroids, terpenes, and tannins (Meyre-Silva and Cechinel-Filho, 2010). Interestingly, the antibacterial activities of these compounds have been reported (Kurbatova et al., 2003; Djahra et al., 2013; Masoodi et al., 2015). Furthermore, previous studies have shown that M. vulgare extracts have antimicrobial activity against pathogenic bacterial and fungal strains (Molina-Salinas et al., 2006; Bouterfas et al., 2016; Al-Tohamy et al., 2018; Bouterfas et al., 2018).
The administration of M. vulgare aqueous extract (400 mg/kg) 30 min before glucose overload resulted in a significant reduction in postprandial hyperglycemia at 60 min (p < 0.01) and 90 min (p < 0.001). Similarly, glibenclamide, a reference antidiabetic drug, significantly inhibited postprandial hyperglycemia at 60 min (p < 0.01) and 90 min (p < 0.05) following glucose overload. By 150 min, as presented in Figure 2A, there were no significant differences in blood glucose levels among all rat groups compared to the normal rats at 60 min, 90 min, and 150 min. Furthermore, analysis of the area under the curves (AUC) showed a significant reduction (p < 0.01) in rats treated with M. vulgare aqueous extract compared to rats treated with distilled water. Similarly, the AUC of glibenclamide was significantly lower (p < 0.01) than that of animals treated with distilled water (Figure 2B). These findings suggest that the administration of M. vulgare extract effectively ameliorated elevated glucose levels in a manner similar to glibenclamide, indicating its potential antihyperglycemic activity. The observed decrease in elevated glucose levels is consistent with the results of a study in which they reported that ethanolic extracts of M. vulgare showed a promising antihyperglycemic effect. Noteworthy, the mechanisms that underpin the observed antihyperglycemic activity of M. vulgare are numerous and they include the modulation of insulin secretion by the beta cells of the islets of Langerhans as well as extrapancreatic and pancreatic effects of insulin. However, elucidating the precise mechanism is still an active area of research. HPLC analysis of M. vulgare extract revealed catechin, maleic acid, luteolin, apigenin, and salicylic acid as the main constituents. Interestingly, these bioactive compounds are likely responsible for the observed antihyperglycemic effect as they been reported possess antidiabetic properties and contribute to the regulation of glycemia (Olmedilla et al., 1997; Kumari et al., 2012). Quercetin, for instance, has been reported to exhibit insulin-like activity or increase insulin secretion (Khatware and Annapurna, 2014). Therefore, the synergistic effect of these bioactive compounds in M. vulgare extract likely contributes to its antihyperglycemic activity. In this regard, there is a clear antihyperglycemic effect of M. vulgare extract, which reinforces its traditional utilization in the control of diabetic patients.
FIGURE 2. Change in postprandial glycemic (A) and the post-prandial curve area (B) in healthy rats after taking the products test (decocted and glibenclamide). The values are SEM averages. (n = 6). ***p < 0.001; **p < 0.01: when compared to the control.
The impact of M. vulgare extract on the activity of pancreatic α-amylase is illustrated in Figure 3. The results demonstrate that the extract of M. vulgare significantly suppresses the activity of pancreatic α-amylase, with an IC50 value of 0.081 ± 0.013 mg/mL. This inhibitory effect on pancreatic α-amylase activity is even more pronounced compared to acarbose, a known α-amylase inhibitor, with an IC50 value of 0.37 ± 0.03 mg/mL. Pancreatic α-amylase plays a crucial role in the digestion of starch and glycogen. Inhibition of this enzyme’s activity involved in carbohydrate digestion can be an effective strategy for the management of carbohydrate assimilation disorders, such as diabetes mellitus (Ouassou et al., 2021). Therefore, the potent inhibitory activity of M. vulgare extract on pancreatic α-amylase, as demonstrated in this study, suggests its potential therapeutic use in the treatment of conditions related to abnormal carbohydrate metabolism. The observed activity of M. vulgare extract in inhibiting pancreatic α-amylase can be attributed to the presence of various bioactive substances within the extract. These bioactive compounds likely interfere with the formation of monosaccharides during carbohydrate digestion, leading to a reduction in blood glucose concentration and indicating a hypoglycemic activity. Further research is needed to identify and isolate the specific bioactive compounds responsible for the α-amylase inhibitory activity of M. vulgare extract and to explore their potential application in the management of carbohydrate-related disorders. Overall, the findings from this study highlight the potential of M. vulgare extract as a natural inhibitor of pancreatic α-amylase, offering prospects for the development of novel therapeutic approaches in the management of carbohydrate assimilation disorders such as diabetes mellitus.
FIGURE 3. Inhibitory effect on the activity of α-amylase by M. vulgare extract and acarbose, in vitro. The values are averages ± SEM, (n = 3).
To investigate the influence of the intestinal environment on the inhibitory activity of M. vulgare on α-amylase, an in vivo test was conducted. The administration of M. vulgare extract (400 mg/kg), 30 min before starch overload in healthy rodents. The results demonstrated a significant reduction in postprandial hyperglycemia in the rodents treated with M. vulgare extract compared to the group pretreated with distilled water. The reduction in blood glucose levels was observed at 60, 90, and 150 min (p < 0.001 for all time points). Similarly, the administration of acarbose also led to a significant decrease in postprandial hyperglycemia at 60, 90, and 150 min compared to the rats treated with water (p < 0.001 for all time points) (Figure 4A). Moreover, the area under the curve was substantially less (p < 0.001) in rodents given M. vulgare than in rats treated with distilled water. The area under the curve for acarbose was markedly (p < 0.001) low compared to the area under the curve in animals treated with water (Figure 4B). The results of the inhibitory effect of M. vulgare extract on pancreatic α-amylase were confirmed in vivo, which is in accordance with prior in vitro experiments. Pancreatic α-amylase is one of the major enzymes in the human body and its inhibition is considered an important strategy in managing blood glucose levels. Therefore, it might be the best strategy to manage type 2 diabetes (Dwek et al., 2002). In fact, the in vivo hypoglycemic effect found for our extract could be related to the bioactive molecules responsible for this effect. These findings suggest that the presence of polyphenolic molecules may have a potentially important role in diabetes management via the inhibition of α-amylase enzyme activities. Additionally, Costamagna et al. (2016) suggested that the hydrolysis of phenolic molecules during digestion contributes to the accumulation of shorter phenolic groups, which could improve their pharmacological properties (Costamagna et al., 2016). In addition, several flavonol glycosides have also been reported to have potent α-amylase inhibition activities in vivo and in vitro models (Braca et al., 2003; He et al., 2007).
FIGURE 4. Effect of M. vulgare L and acarbose on postprandial blood glucose variation in normal rats (A), with a representation as areas under the curves (B). The values are SEM averages (n = 6) ***p < 0.001: compared to the control.
The docking scores of the eight major components of M. vulgare extract, which represents their binding affinities for the antimitotic protein (1XO2), antidiabetic protein (4W93), and antimicrobial protein (1AJ6, 2023) are presented in Supplementary Table S2 (Supplementary provided). It is worth noting that a lower docking score corresponds to higher binding affinities. The results showed that the binding energies of the ligands vary depending on the protein target. For instance, Leotulin has the lowest binding energy with the antimitotic protein (1XO2) with a value of −9.3 kcal/mol, indicating a strong binding affinity, whereas Maleic Acid has the lowest binding energy with the antimicrobial protein (1AJ6) with a value of −4.3 kcal/mol, indicating a relatively weaker binding affinity. Interestingly, Leotulin consistently exhibits the lowest binding energy across all three protein targets, suggesting that it has a high potential to be an effective drug candidate for these protein targets. Conversely, Maleic Acid consistently shows the weakest binding affinity among the ligands. Apigenin and Vanillic Acid have relatively consistent binding energies across the three protein targets, whereas the other ligands have more varied binding energies depending on the protein target. For example, Catechin has significantly lower binding energy with the antidiabetic protein (4W93) than with the other two protein targets. Biotin shows moderate binding energies across all three protein targets, with a slightly stronger binding affinity to the antimitotic protein (1XO2) than to the other two protein targets. Salicylic Acid has a similar binding energy to the antidiabetic protein (4W93, 2023) and antimicrobial protein (1AJ6), whereas it has a slightly weaker binding affinity to the antimitotic protein (1XO2). Leotulin consistently exhibits the strongest binding affinity across all three protein targets, while Maleic Acid shows the weakest binding affinity. The other ligands have varying binding energies depending on the protein target, with some showing consistent binding affinities across all three protein targets. Major interactions with good results are shown in Table 12.
TABLE 12. Interaction of antimitotic protein target with the major components of M. vulgare extract ligands showing the 3D and 2D structural view. Full Table in Supplementary Table S4.
Supplementary Table S3 (Supplementary provided) shows the interaction of the antimitotic protein target (1XO2, 2023) with the major components of M. vulgare extract ligands, representing the binding pocket residue amino acids with distances and types of bonding interactions. Each ligand is listed with the amino acid residues that it interacts with in the binding pocket of the antimitotic protein, along with the distance between the ligand and the amino acid residues in Å. The types of bonding interactions are also listed, which can be either hydrogen bonding, π-bonding, alkyl bonding, or hydrophobic interactions. Apigenin interacts with several amino acid residues, including GLU52, ARG46, GLN48, LEU56, TYR24, and PRO55. The distances of these interactions range from 1.98 to 4.97 Å. The type of bonding interactions involved include H-bonds, π-bonds, and alkyl bonds. Biotin interacts with VAL27, LYS43, ALA162, and PHE98 amino acid residues. The distance between the ligand and these residues ranges from 4.19 to 5.44 Å. The type of bonding interactions involved are mainly alkyl bonds and hydrophobic interactions. Caffeic acid interacts with GLU61, VAL101, PHE98, VAL27, LYS43, and ALA162 amino acid residues. The distances between the ligand and these residues range from 2.56 to 5.24 Å. The type of bonding interactions involved includes H-bonds, alkyl bonds, and hydrophobic interactions. Catechin interacts with GLN48, ARG46, GLY53, and PRO55 amino acid residues. The distance between the ligand and these residues ranges from 2.16 to 5.33 Å. The type of bonding interactions involved includes H-bonds, alkyl bonds, and hydrophobic interactions. Leotulin interacts with several amino acid residues, including GLU21, ASP163, ASP104, ILE19, VAL27, LEU152, and others. The distances of these interactions range from 2.06 to 4.96 Å. The type of bonding interactions involved includes H-bonds, π-bonds, and alkyl bonds. Maleic acid interacts with ASP163, GLU99, and H-O amino acid residues. The distances of these interactions range from 2.10 to 2.87 Å. The type of bonding interactions involved includes H-bonds. Salicylic acid interacts with several amino acid residues, including LYS43, ASP163, GLU61, PHE98, VAL27, and others. The distances of these interactions range from 2.34 to 5.44 Å. The type of bonding interactions involved include H-bonds, π-bonds, hydrophobic bonds. Vanillic Acid showed interactions with ASP163 and GLU61 both have hydrogen bonds, while ALA162, VAL27 (both occurrences), and LEU152 have hydrophobic properties. Meanwhile, VAL77 has a π-bond, and VAL27 and LEU152 have alkyl bonds. All type of interactions is also shown in Supplementary Table S4 (Supplementary provided) as 3D and 2D. Major interactions with good results are shown in Table 13.
TABLE 13. Interaction of antidiabetic protein target with the major components of M. vulgare extract ligands showing 3D and 2D structural view. Full Table in Supplementary Table S5.
Supplementary Table S5 (Supplementary provided) shows the results of an experiment investigating the interaction between antidiabetic protein and the major components of M. vulgare extract ligands. The first ligand presented in the table is apigenin. The antidiabetic protein interacts with GLN63, GLU233, TRP59, and TYR62 amino acid residues of apigenin, and the distances between the amino acid residues and the ligand range from 2.47 to 5.23 Å. The bonding interactions between the protein and the apigenin ligand are mainly hydrogen bonds, except for a hydrophobic interaction and two types of alkyl bonds. The second ligand is biotin, and the antidiabetic protein interacts with ARG252, ARG421, PRO332, SER289, ASP402, and GLY334 amino acid residues of biotin. The distance between the ligand and the interacting amino acid residues ranges from 1.85 to 3.76 Å. The bonding interactions between the protein and biotin are mainly hydrogen bonds, except for two types of π-bonds. The third ligand is caffeic acid, and the antidiabetic protein interacts with ASP197 and TYR62 amino acid residues of caffeic acid. The distance between the ligand and the interacting amino acid residues ranges from 2.51 to 4.35 Å. The bonding interactions between the protein and caffeic acid are a hydrogen bond and an alkyl bond. The fourth ligand is catechin, and the antidiabetic protein interacts with ASP197, GLU233, TRP59, and TYR62 amino acid residues of catechin. The distance between the ligand and the interacting amino acid residues ranges from 2.05 to 4.66 Å. The bonding interactions between the protein and catechin are mainly hydrogen bonds, except for three types of alkyl bonds.
The fifth ligand is leotulin, and the antidiabetic protein interacts with GLN63, H-O, TRP59, and TYR62 amino acid residues of botulin. The distance between the ligand and the interacting amino acid residues ranges from 1.98 to 5.34 Å. The bonding interactions between the protein and leotulin are mainly hydrogen bonds, except for four types of alkyl bonds. The sixth ligand is maleic acid, and the antidiabetic protein interacts with ARG346, ASP317, and GLN302 amino acid residues of maleic acid. The distance between the ligand and the interacting amino acid residues ranges from 1.95 to 2.12 Å. The bonding interactions between the protein and maleic acid are all hydrogen bonds. The seventh and final ligand presented in the table is salicylic acid, and the antidiabetic protein interacts with ARG195, ASP197, GLU233, and TYR62 amino acid residues of salicylic acid. The distance between the ligand and the interacting amino acid residues ranges from 2.10 to 4.98. Vanillic Acid interacts with the ASP197 residue of the protein target through a hydrogen bond, with 2.32 Å. It also forms a second hydrogen bond with another ASP197 residue with 2.48 Å. Additionally; the ligand interacts with the GLU233 residue of the protein through a pi-bond, with 3.73 Å. Vanillic Acid binds to the TYR62 residue of the protein through an alkyl-bond, with 4.50 Å. Finally, the ligand interacts with the ALA198 and LEU162 residues of the protein through pi-bonds and alkyl-bonds, respectively, with distances of 3.75 and 4.98 Å. These interactions may play a role in the potential antidiabetic properties of the M. vulgare extract, as the ligand is able to interact with specific binding pocket residues of the protein target as shown in Supplementary Table 6S (Supplementary provided) as 3D and 2D representations. Major interactions with good results are shown in Table 14.
TABLE 14. Interaction of antimicrobial protein target with the major components of M. vulgare extract ligands showing 3D and 2D structural view. Full Table in Supplementary Table S7.
Supplementary Table S7 (Supplementary provided) lists the interaction of various ligands with the major components of M. vulgare extract and the antimicrobial protein target. Apigenin interacts with the antimicrobial protein target through hydrogen bonding and pi-bonding interactions with multiple amino acid residues, including GLY113, ILE186, HIS38, ARG190, LYS189, and ARG190. The distances between the ligand and the amino acid residues range from 1.8454 to 5.34399 Å. Biotin interacts with the antimicrobial protein target through hydrogen bonding and hydrophobic interactions with amino acid residues GLY77, THR165, ASP73, ALA100, and ILE78. The distances between the ligand and the amino acid residues range from 2.32752 to 5.36772 Å. Caffeic Acid interacts with the antimicrobial protein target through hydrogen bonding and pi-bonding interactions with amino acid residues THR165, ASP73, GLY77, GLU50, and ILE78. The distances between the ligand and the amino acid residues range from 2.04553 to 3.81553 Å. Catechin interacts with the antimicrobial protein target through hydrogen bonding and hydrophobic interactions with amino acid residues ARG76, GLU50, THR165, and ALA47. The distances between the ligand and the amino acid residues range from 2.61913 to 5.25295 Å.
Leotulin interacts with the antimicrobial protein target through hydrogen bonding, pi-bonding interactions, and hydrophobic interactions with amino acid residues ARG76, GLU50, THR165, ASN46, ILE78, and ALA47. The distances between the ligand and the amino acid residues range from 2.56108 to 5.05026 Å. Maleic Acid interacts with the antimicrobial protein target through hydrogen bonding and hydrophobic interactions with amino acid residues HIS99 and ALA100. The distances between the ligand and the amino acid residues range from 2.33271 to 2.67941 Å. Salicylic Acid interacts with the antimicrobial protein target through hydrogen bonding and hydrophobic interactions with amino acid residues ASP73, VAL120, and VAL167. The distances between the ligand and the amino acid residues range from 2.39911 to 5.39453 Å.Vanillic Acid interacts with the antimicrobial protein target through hydrogen bonding, pi-bonding interactions, and hydrophobic interactions with amino acid residues THR165, ASP73, VAL71, ASP73, THR165, ALA47, VAL43, VAL71, VAL167, and ILE78. The distances between the ligand and the amino acid residues range from 1.97698 to 5.36435 Å. All type of interactions is also shown in Supplementary Table S8 (Supplementary provided) as 3D and 2D.
In conclusion, the outcomes of the current study showed that the M. vulgare aqueous extract significantly exerts antioxidant, antimitotic, and antimicrobial activities. As well as a strong anti-hyperglycemic effect and pancreatic α-amylase inhibitory effect. These effects are due to its richness of a diversity of phenolic compounds. These findings support the folkloric use of M. vulgare decoction in the prevention and management of illnesses like diabetes, colds, and flu, and can also be used in the management of diabetes oxidative stress, and infectious diseases. Also, molecular docking studies revealed the compounds as viable potential drug candidates and could be explored in the discovery of antimitotic, antidiabetic, and antimicrobial protein targets. However, further experimental studies are needed to further validate the results of this study.
The raw data supporting the conclusion of this article will be made available by the authors, without undue reservation.
Conceptualization, writing the original draft, formal analysis, investigations: AG, HT, AD, AAs, FR, and SS. Funding acquisition, resources, project administration, reviewing and editing, data validation, and data curation: AAi, FS, and MB, data validation, and data curation, formal analysis, investigations. AS, LO, AM, and BE. Supervision: TZ. All authors contributed to the article and approved the submitted version.
The authors would like to extend their sincere appreciation to the Researchers Supporting Project, King Saud University, Riyadh, Saudi Arabia for funding this work through project number (RSP-2023R437).
BE was employed by the Laboratoires TBC.
The remaining authors declare that the research was conducted in the absence of any commercial or financial relationships that could be construed as a potential conflict of interest.
All claims expressed in this article are solely those of the authors and do not necessarily represent those of their affiliated organizations, or those of the publisher, the editors and the reviewers. Any product that may be evaluated in this article, or claim that may be made by its manufacturer, is not guaranteed or endorsed by the publisher.
The Supplementary Material for this article can be found online at: https://www.frontiersin.org/articles/10.3389/fchem.2023.1238346/full#supplementary-material
1AJ6, 20231AJ6 (2023). NOVOBIOCIN-RESISTANT mutant (R136H) of the N-terminal 24 kda fragment of dna gyrase B complexed with NOVOBIOCIN at 2.3 angstroms resolution. Available online: https//www.rcsb.org/1AJ6 (accessed on April 31, 2023).
1XO2, 20231XO2 (2023). Crystal structure of a human cyclin-dependent kinase 6 complex with a flavonol inhibitor, fisetin. Available online: https//www.rcsb.org/1XO2 (accessed on April 31, 2023).
4W93, 20234W93 (2023). Human pancreatic alpha-amylase in complex with montbretin A. Available online: https//www.rcsb.org/4W93 (accessed on April 31, 2023).
Accelrys Software Inc (2005). Discovery Studio visualizer, 2. San Diego, CA, USA: Accelrys Software Inc.
AFNOR (1985). NF V03-402. Épices et aromates - Détermination de la teneur en eau - Méthode par entraînement. » Afnor EDITIONS.
AFNOR (1972). NF V05-113. Fruits, légumes et produits dérivés - minéralisation des matières organiques - Méthode par incinération ». Afnor EDITIONS.
Akther, N., Shawl, A. S., Sultana, S., Chandan, B. K., and Akhter, M. (2013). Hepatoprotective activity of Marrubium vulgare against paracetamol induced toxicity. J. Pharm. Res. 7, 565–570. doi:10.1016/j.jopr.2013.06.023
Al-Tohamy, R., Ali, S. S., Saad-Allah, K., Fareed, M., Ali, A., El-Badry, A., et al. (2018). Phytochemical analysis and assessment of antioxidant and antimicrobial activities of some medicinal plant species from Egyptian flora. J. Appl. Biomed. 16, 289–300. doi:10.1016/j.jab.2018.08.001
Alami Merrouni, I., Kharchoufa, L., Bencheikh, N., and Elachouri, M. (2021). Ethnobotanical profile of medicinal plants used by people of North-eastern Morocco: cross-cultural and historical approach (part I). Ethnobot. Res. Appl. 21, 1–45. doi:10.32859/ERA.21.34.1-45
Ali-Rachedi, F., Meraghni, S., and Touaibia, N. (2018). Quantitative analysis of phenolic compounds of an Algerian endemic Scabiosa Atropurpurea sub. Maritima L. J. Bull. Société R. Des. Sci. Liège 87, 13–21.
Amessis-Ouchemoukh, N., Abu-Reidah, I. M., Quirantes-Piné, R., Madani, K., and Segura-Carretero, A. (2014). Phytochemical profiling, in vitro evaluation of total phenolic contents and antioxidant properties of marrubium vulgare (horehound) leaves of plants growing in Algeria. Ind. Crops Prod. 61, 120–129. doi:10.1016/j.indcrop.2014.06.049
Bissel, S. J., Winkler, C. C., Deltondo, J., Wang, G., Williams, K., and Wiley, C. A. (2014). Coxsackievirus B4 myocarditis and meningoencephalitis in newborn twins. Neuropathology 34, 429–437. doi:10.1111/neup.12121
Barros, L., Cabrita, L., Boas, M. V., Carvalho, A. M., and Ferreira, I. C. F. R. (2011). Chemical, biochemical and electrochemical assays to evaluate phytochemicals and antioxidant activity of wild plants. Food Chem. 127, 1600–1608. doi:10.1016/j.foodchem.2011.02.024
Bencheikh, N., Bouhrim, M., Merrouni, I. A., Boutahiri, S., Legssyer, A., Elachouri, M., et al. (2021). Antihyperlipidemic and antioxidant activities of flavonoid-rich extract of ziziphus lotus (L) lam fruits. Appl. Sci. 11, 7788. doi:10.3390/app11177788
Bencheikh, N., Elachouri, M., and Subhash, C., M. (2022a). Ethnobotanical, pharmacological, phytochemical, and clinical investigations on Moroccan medicinal plants traditionally used for the management of renal dysfunctions. J. Ethnopharmacol. 292, 115178. doi:10.1016/j.jep.2022.115178
Bencheikh, N., Ouahhoud, S., Cordero, M. A. W., Alotaibi, A., Fakchich, J., Ouassou, H., et al. (2022b). Nephroprotective and Antioxidant Effects of Flavonoid-Rich Extract of Thymelaea microphylla Coss. et Dur Aerial Part. Appl. Sci. 12, 9272. doi:10.3390/app12189272
Benzidane, N., Smahi, R., Zabouche, B., Abdelhalim Makrouf, L. A., and Arrar, L. (2020). Phytochemical study and antimicrobial activity of Algerian Marrubium vulgare leaf and stem extracts. J. Drug Deliv. Ther. 10, 70–74. doi:10.22270/jddt.v10i5.4353
Bergeron, L. (1995). Effet de la Teneur en Eau du Sol Sur le Rendement et la Qualité Des Fruits du Bleuet Nain; Université du Québec à Chicoutimi: Chicoutimi. QC, Canada. 978-1-4123-0614-0.
Boudjelal, A., Henchiri, C., Sari, M., Sarri, D., Hendel, N., Benkhaled, A., et al. (2013). Herbalists and wild medicinal plants in M’Sila (North Algeria): an ethnopharmacology survey. J. Ethnopharmacol. 148, 395–402. doi:10.1016/j.jep.2013.03.082
Bouhrim, M., Ouassou, H., Boutahiri, S., Daoudi, N. E., Mechchate, H., Gressier, B., et al. (2021). Opuntia dillenii (ker gawl) haw., seeds oil antidiabetic potential using in vivo, in vitro, in situ, and ex vivo approaches to reveal its underlying mechanism of action. Molecules 26, 1677. doi:10.3390/molecules26061677
Bouterfas, K., Mehdadi, Z., Elaoufi, M. M., Aouad, L., Latreche, A., and Benchiha, W. (2018). In vitro antibacterial activity of flavonoids extracts from three Algerian horehound (Marrubium vulgare L) leaves) leaves Orient. Pharm. Exp. Med. 18, 59–66. doi:10.1007/s13596-017-0287-5
Bouterfas, K., Mehdadi, Z., Elaoufi, M. M., Latreche, A., and Benchiha, W. (2016). Antioxidant activity and total phenolic and flavonoids content variations of leaves extracts of white Horehound (Marrubium vulgare Linné) from three geographical origins. Ann. Pharm. Fr. 74, 453–462. doi:10.1016/j.pharma.2016.07.002
Bouyahya, A., El Omari, N., Elmenyiy, N., Guaouguaou, F. E., Balahbib, A., Belmehdi, O., et al. (2021). Moroccan antidiabetic medicinal plants: Ethnobotanical studies, phytochemical bioactive compounds, preclinical investigations, toxicological validations and clinical evidences; challenges, guidance and perspectives for future management of diabetes worldwide. Trends Food Sci. Technol. 115, 147–254. doi:10.1016/j.tifs.2021.03.032
Braca, A., Politi, M., Sanogo, R., Sanou, H., Morelli, I., Pizza, C., et al. (2003). Chemical composition and antioxidant activity of phenolic compounds from wild and cultivated sclerocarya birrea (anacardiaceae) leaves. J. Agric. Food Chem. 51, 6689–6695. doi:10.1021/jf030374m
CambridgeSoft (2009b). Chem 3D Pro 12.0 (copyright) 1986 to 2009. Cambridge, MA, USA: CambridgeSoft Corp.
CambridgeSoft (2009a). Ultra 12.0 0 (copyright) 1986 to 2009. Cambridge, MA, USA: CambridgeSoft Corp.
Chavan, U., Shahidi, F., and Naczk, M. (2001). Extraction of condensed tannins from beach pea (Lathyrus maritimus L) as affected by different solvents) as affected by different solvents. Food Chem. 75, 509–512. doi:10.1016/s0308-8146(01)00234-5
Costamagna, M. S., Zampini, I. C., Alberto, M. R., Cuello, S., Torres, S., Pérez, J., et al. (2016). Polyphenols rich fraction from Geoffroea decorticans fruits flour affects key enzymes involved in metabolic syndrome, oxidative stress and inflammatory process. Food Chem. 190, 392–402. doi:10.1016/j.foodchem.2015.05.068
Crozier, A., Jaganath, I. B., and Clifford, M. N. (2009). Dietary phenolics: Chemistry, bioavailability and effects on health. Nat. Prod. Rep. 26, 1001–1043. doi:10.1039/b802662a
Daoudi, N. E., Bouhrim, M., Ouassou, H., Legssyer, A., Mekhfi, H., Ziyyat, A., et al. (2020). Inhibitory effect of roasted/unroasted Argania spinosa seeds oil on α-glucosidase, α-amylase and intestinal glucose absorption activities. South Afr. J. Bot. 135, 413–420. doi:10.1016/j.sajb.2020.09.020
De Oliveira Pedro, R., Takaki, M., Gorayeb, T. C. C., Bianchi, V. L. D., Thomeo, J. C., Tiera, M. J., et al. (2013). Synthesis, characterization and antifungal activity of quaternary derivatives of chitosan on Aspergillus flavus. Microbiol. Res. 168, 50–55. doi:10.1016/j.micres.2012.06.006
Deepthi, B., Sowjanya, K., Lidiya, B., Bhargavi, R., and Babu, P. (2017). A modern review of diabetes mellitus: an annihilatory metabolic disorder. J. Silico Vitr. Pharmacol. 3, 1–5. doi:10.21767/2469-6692.100014
Dib, K., Cherrah, Y., Rida, S., Filali-Maltouf, A., and Ennibi, O. (2021). In vitro antibacterial activity of myrtus communis L. And marrubium vulgare L. Leaves against aggregatibacter actinomycetemcomitans and eikenella corrodens. Altern. Med. 2021, 1–8. doi:10.1155/2021/8351332
Djahra, A. B., Bordjiba, O., and Benkherara, S. (2013). Extraction, séparationet activité antibactérienne des tanins de marrube blanc (Marrubium vulgare L). Phytotherapie 11, 348–352. doi:10.1007/s10298-013-0819-1
Dra, L. A., Sellami, S., Rais, H., Aziz, F., Aghraz, A., Bekkouche, K., et al. (2019). Antidiabetic potential of Caralluma europaea against alloxan-induced diabetes in mice. Saudi J. Biol. Sci. 26, 1171–1178. doi:10.1016/j.sjbs.2018.05.028
Duarte, M. C. T., Leme, E. E., Delarmelina, C., Soares, A. A., Figueira, G. M., and Sartoratto, A. (2007). Activity of essential oils from Brazilian medicinal plants on Escherichia coli. J. Ethnopharmacol. 111, 197–201. doi:10.1016/j.jep.2006.11.034
Dwek, R. A., Butters, T. D., Platt, F. M., and Zitzmann, N. (2002). Targeting glycosylation as a therapeutic approach. Nat. Rev. Drug Discov. 1, 65–75. doi:10.1038/nrd708
Eberhardt, J., Santos-Martins, D., Tillack, A. F., and Forli, S. (2021). AutoDock Vina 1.2.0: New docking methods, expanded force field, and Python bindings. J. Chem. Inf. Model. 61, 3891–3898. doi:10.1021/acs.jcim.1c00203
Ekor, M. (2014). The growing use of herbal medicines: issues relating to adverse reactions and challenges in monitoring safety. Front. Pharmacol. 4, 177–210. doi:10.3389/fphar.2013.00177
Elberry, A. A., Harraz, F. M., Ghareib, S. A., Gabr, S. A., Nagy, A. A., and Abdel-Sattar, E. (2015). Methanolic extract of Marrubium vulgare ameliorates hyperglycemia and dyslipidemia in streptozotocin-induced diabetic rats. Int. J. Diabetes Mellit. 3, 37–44. doi:10.1016/j.ijdm.2011.01.004
Fakchich, J., and Elachouri, M. (2021). An overview on ethnobotanico-pharmacological studies carried out in Morocco, from 1991 to 2015: Systematic review (part 1). J. Ethnopharmacol. 267, 113200–200. doi:10.1016/j.jep.2020.113200
Fayyad, A. G. S., Ibrahim, N., and Yaakob, W. A. (2014). Phytochemical screening and antiviral activity of Marrubium vulgare. J. Microbiol. 10, 106–111.
Ferreira, L. G., Dos Santos, R. N., Oliva, G., and Andricopulo, A. D. (2015). Molecular docking and structure-based drug design strategies. Molecules 20, 13384–13421. doi:10.3390/molecules200713384
Ghedadba, N., Bousselsela, H., Hambaba, L., Benbia, S., and Mouloud, Y. (2014). Évaluation de l’activité antioxydante et antimicrobienne des feuilles et des sommités fleuries de Marrubium vulgare L. Phytotherapie 12, 15–24. doi:10.1007/s10298-014-0832-z
Hanhineva, K., Törrönen, R., Bondia-Pons, I., Pekkinen, J., Kolehmainen, M., Mykkänen, H., et al. (2010). Impact of dietary polyphenols on carbohydrate metabolism. Int. J. Mol. Sci. 11, 1365–1402. doi:10.3390/ijms11041365
He, Q., Lv, Y., and Yao, K. (2007). Effects of tea polyphenols on the activities of α-amylase, pepsin, trypsin and lipase. Food Chem. 101, 1178–1182. doi:10.1016/j.foodchem.2006.03.020
Hosseinzadeh, H., and Nassiri-Asl, M. (2015). Pharmacological effects of Glycyrrhiza spp. and its bioactive constituents: update and review. Phytother. Res. 29 (12), 1868–1886. doi:10.1002/ptr.5487
Kakoma, P. K., Kadiebwe, D. M., Kayembe, A. M., Kashindi, P. M., Bugeme, M., and Mukuku, O. (2014). Diabetic ketoacidosis in adults in sendwe hospital lubumbashi: about 51 cases. Pan Afr. Med. J. 17, 324–325. doi:10.11604/pamj.2014.17.324.3545
Karryev, M., Bairyev, C., and Ataeva, A. (1976). Some therapeutic properties and phytochemistry of common horehound. J. Seriya Biol. Nauk. 3, 86–88.
Khanbabaee, K., and van Ree, T. (2001). Tannins: classification and definition. Nat. Prod. Rep. 18, 641–649. doi:10.1039/b101061l
Khatware, K., and Annapurna, A. (2014). The effect of Quercetin on blood glucose levels of normal and streptozotocin induced diabetic (type I & type ii) rats. J. Pharm. Chem. Biol. Sci. 4.
Koncic, M. Z., Kremer, D., Karlovic, K., and Kosalec, I. (2010). Evaluation of antioxidant activities and phenolic content of Berberis vulgaris L. and Berberis croatica Horvat. Food Chem. Toxicol. J. 48, 2176–2180. doi:10.1016/j.fct.2010.05.025
Kotan, R., Kordali, S., Cakir, A., Kesdek, M., Kaya, Y., and Kilic, H. (2008). Antimicrobial and insecticidal activities of essential oil isolated from Turkish Salvia hydrangea DC. ex Benth. Ex. Benth. Biochem. Syst. Ecol. 36, 360–368. doi:10.1016/j.bse.2007.12.003
Kumar, V., and Singhalb, A. (n.d.). Germinating seeds of the Mung bean, Vigna radiata (Fabaceae), as a model for the preliminary evaluation of cytotoxic effects of drugs. J. Biocell. 33, 19–24.
Kumari, S., Kumar, P., Wanjari, M., and Palani, S. (2012). Antidiabetic activity of Pandanus fascicularis Lamk - aerial roots in alloxan-induced hyperglycemic rats. Int. J. Nutr. Pharmacol. Neurol. Dis. 2, 105. doi:10.4103/2231-0738.95943
Kurbatova, N. V., Muzychkina, R. A., Mukhitdinov, N. M., and Parshina, G. N. (2003). Comparative phytochemical investigation of the composition and content of biologically active substances in Marrubium vulgare and M. alternidens. Chem. Nat. Compd. 39, 501–502. doi:10.1023/B:CONC.0000011128.64886.f4
Ladoh Yemeda, C. F., Dibong, S. D., Nyegue, M. A., Djembissi Talla, R., Mpondo, E., Yinyang, J., et al. (2014). Activité antioxydante des extraits méthanoliques de Phragmanthera capitata (Loranthaceae) récoltée sur Citrus sinensis. J. Appl. Biosci. 84, 7636–7643.
Loizzo, M. R., Leporini, M., Sicari, V., Falco, T., Pellicanò, T. M., and Tundis, R. (2017). Investigating the in vitro hypoglycaemic and antioxidant properties of Citrus × clementina Hort. juice. Juice. Eur. Food Res. Technol. 12, 523–534. doi:10.1007/s00217-017-2978-z
Lorrain, B., Chira, K., and Teissedre, P. L. (2011). Phenolic composition of merlot and cabernet-sauvignon grapes from bordeaux vineyard for the 2009-vintage: comparison to 2006, 2007 and 2008 vintages. Food Chem. 126, 1991–1999. doi:10.1016/j.foodchem.2010.12.062
Martel, J., Ojcius, D. M., Chang, C. J., Lin, C. S., Lu, C. C., Ko, Y. F., et al. (2017). Anti-obesogenic and antidiabetic effects of plants and mushrooms. J. Nat. Rev. Endocrinol. 13, 149–160. doi:10.1038/nrendo.2016.142
Masoodi, M., Ali, Z., Liang, S., Yin, H., Wang, W., and Khan, I. A. (2015). Labdane diterpenoids from Marrubium vulgare. Phytochem. Lett. 13, 275–279. doi:10.1016/j.phytol.2015.07.005
Matkowski, A., Tasarz, P., and Szypuła, E. (2008). Antioxidant activity of herb extracts from five medicinal plants from Lamiaceae, subfamily Lamioideae. J. Med. Plants Res. 2, 321–330.
Mbayo, M. K., Kalonda, E. M., Muya, R. K., Tshisand, P. T., Kanangila, A. B., Maseho, F. M., et al. (2016). Test d’activité antimitotique et étude chimique préliminaire de quelques Euphorbiaceae du Katanga méridional (RDC). Phytotherapie 2012, 1–13. doi:10.1007/s10298-016-1060-5
Meyre-Silva, C., and Cechinel-Filho, V. (2010). A review of the chemical and pharmacological aspects of the genus marrubium. Curr. Pharm. Des. 16, 3503–3518. doi:10.2174/138161210793563392
Molina-Salinas, G. M., Ramos-Guerra, M. C., Vargas-Villarreal, J., Mata-Cárdenas, B. D., Becerril-Montes, P., and Said-Fernández, S. (2006). Bactericidal activity of organic extracts from flourensia cernua DC against strains of Mycobacterium tuberculosis. Mycobacterium Tuberc. Arch. Med. Res. 37, 45–49. doi:10.1016/j.arcmed.2005.04.010
Morris, G. M., Huey, R., Lindstrom, W., Sanner, M. F., Belew, R. K., Goodsell, D. S., et al. (2009). AutoDock4 and AutoDockTools4: automated docking with selective receptor flexibility. J. Comput. Chem. 30, 2785–2791. doi:10.1002/jcc.21256
Musa Özcan, M. (2006). Determination of the mineral compositions of some selected oil-bearing seeds and kernels using Inductively Coupled Plasma Atomic Emission Spectrometry (ICP-AES). Grasas Aceites 57, 211–218. doi:10.3989/gya.2006.v57.i2.39
Olmedilla, B., Granado, F., Gil-Martinez, E., Blanco, I., and Rojas-Hidalgo, E. (1997). Reference values for retinol, tocopherol, and main carotenoids in serum of control and insulin-dependent diabetic Spanish subjects. Clin. Chem. 43, 1066–1071. doi:10.1093/clinchem/43.6.1066
Onesti, C. E., Vicier, C., and André, F. (2015). Qu'attendre de la génomique à haut débit dans les cancers du sein métastatiques ? Breast 24, S19–S22. doi:10.1016/j.breast.2015.07.006
Ouassou, H., Bouhrim, M., Bencheikh, N., Addi, M., Hano, C., Mekhfi, H., et al. (2021). In vitro antioxidant properties, glucose-diffusion effects, α-amylase inhibitory activity, and antidiabetogenic effects of C. Europaea extracts in experimental animals. Antioxidants 10, 1747. doi:10.3390/antiox10111747
Ouedraogo, S., Yoda, J., Traore, T. K., Nitiema, M., Sombie, B. C., Diawara, H. Z., et al. (2021). Production de matières premières et fabrication des médicaments à base de plantes médicinales. Int. J. Biol. Chem. Sci. 15, 750–772. doi:10.4314/ijbcs.v15i2.28
Porto, C. D., Porretto, E., and Decorti, D. (2013). Ultrasonics Sonochemistry Comparison of ultrasound-assisted extraction with conventional extraction methods of oil and polyphenols from grape (Vitis vinifera L) seeds. Ultrason. - Sonochemistry 20, 1076–1080. doi:10.1016/j.ultsonch.2012.12.002
Prieto, P., Pineda, M., and Aguilar, M. (1999). Spectrophotometric quantitation of antioxidant capacity through the formation of a phosphomolybdenum complex: specific application to the determination of vitamin E. Anal. Biochem. 269, 337–341. doi:10.1006/abio.1999.4019
Pukalskas, A., Venskutonis, P. R., Salido, S., Waard, P., and van Beek, T. A. (2012). Isolation, identification and activity of natural antioxidants from horehound (Marrubium vulgare L) cultivated in Lithuania) cultivated in Lithuania. Food Chem. 130, 695–701. doi:10.1016/j.foodchem.2011.07.112
Rezgui, M., Majdoub, N., Mabrouk, B., Baldisserotto, A., Bino, A., Ben Kaab, L. B., et al. (2020). Antioxidant and antifungal activities of marrubiin, extracts and essential oil from Marrubium vulgare L. against pathogenic dermatophyte strains. J. Mycol. Med. 30, 100927. doi:10.1016/j.mycmed.2020.100927
Sartoratto, A., Machado, A. L. M., Delarmelina, C., Figueira, G. M., Duarte, M. C. T., and Rehder, V. L. G. (2004). Composition and antimicrobial activity of essential oils from aromatic plants used in Brazil. Braz. J. Microbiol. 35, 275–280. doi:10.1590/S1517-83822004000300001
Singh, J., Singh, J., and Sharma, D. (2018). Traditional wisdom to treat the most common ailments in chopal region of Shimla district, Himachal Pradesh, India. Plant Arch. 18, 2759–2769.
Sisodia, D., and Sisodia, D. S. (2018). Prediction of diabetes using classification algorithms. Procedia Comput. Sci. 132, 1578–1585. doi:10.1016/j.procs.2018.05.122
Taib, M., Rezzak, Y., Bouyazza, L., and Lyoussi, B. (2020). Medicinal uses, phytochemistry, and pharmacological activities of quercus species. Evidence-based complement. Altern. Med. 2020, 20. doi:10.1155/2020/1920683
Tamert, A., Latreche, A., and Aouad, L. (2017). Criblage phytochimique et activité antimicrobienne des extraits de Thymus serpyllum et de Thymus vulgaris du mont de Tessala (Algérie occidentale). Phytotherapie 15, 384–394. doi:10.1007/s10298-017-1132-1
Tchoumtchoua, J., Mouchili, O. R., Ateba, S. B., Zingue, S., Halabalaki, M., Mbanya, J. C., et al. (2014). Safety assessment of the methanol extract of the stem bark of Amphimas pterocarpoides Harms: acute and subchronic oral toxicity studies in Wistar rats. Toxicol. Rep. 1, 877–884. doi:10.1016/j.toxrep.2014.10.003
Trott, O., and Olson, A. J. (2010). AutoDock Vina: improving the speed and accuracy of docking with a new scoring function, efficient optimization, and multithreading. J Comput. Chem. 31 (2), 455–461. doi:10.1002/jcc.21334
Wang, H., Wang, J., Qiu, C., Ye, Y., Guo, X., Chen, G., et al. (2017). Comparison of phytochemical profiles and health benefits in fiber and oil flaxseeds (Linum usitatissimum L). Food Chem. 214, 227–233. doi:10.1016/j.foodchem.2016.07.075
Wojdyło, A., Oszmiański, J., and Czemerys, R. (2007). Antioxidant activity and phenolic compounds in 32 selected herbs. Food Chem. 105, 940–949. doi:10.1016/j.foodchem.2007.04.038
Yousefi, K., Fathiazad, F., Soraya, H., Rameshrad, M., Maleki-Dizaji, N., and Garjani, A. (2014). Marrubium vulgare L. methanolic extract inhibits inflammatory response and prevents cardiomyocyte fibrosis in isoproterenol-induced acute myocardial infarction in rats. BioImpacts 4, 21–27. doi:10.5681/bi.2014.001
Yousefi, K., Soraya, H., Fathiazad, F., Khorrami, A., Hamedeyazdan, S., Maleki-Dizaji, N., et al. (2013). Cardioprotective effect of methanolic extract of Marrubium vulgare L. on isoproterenol-induced acute myocardial infarction in rats. Indian J. Exp. Biol. 51, 653–660. https://nopr.niscpr.res.in/handle/123456789/20478
Yuan, H., Ma, Q., Ye, L., and Piao, G. (2016). The traditional medicine and modern medicine from natural products. Molecules 21, 559. doi:10.3390/molecules21050559
Yusuf, D., Davis, A. M., Kleywegt, G. J., and Schmitt, S. (2008). An alternative method for the evaluation of docking performance: RSR vs. RMSD. J. Chem. Inf. Model. 48, 1411–1422. doi:10.1021/ci800084x
Keywords: Marrubium vulgare L., polyphenols, LC/MS, antioxidant, toxicity, antimitotic, antimicrobial, antidiabetic
Citation: Gourich AA, Touijer H, Drioiche A, Asbabou A, Remok F, Saidi S, Siddique F, Ailli A, Bourhia M, Salamatullah AM, Ouahmane L, Mouradi A, Eto B and Zair T (2023) Insight into biological activities of chemically characterized extract from Marrubium vulgare L. in vitro, in vivo and in silico approaches. Front. Chem. 11:1238346. doi: 10.3389/fchem.2023.1238346
Received: 11 June 2023; Accepted: 01 August 2023;
Published: 17 August 2023.
Edited by:
Kaushik Chanda, VIT University, IndiaReviewed by:
Cheng-Ting Zi, Yunnan Agricultural University, ChinaCopyright © 2023 Gourich, Touijer, Drioiche, Asbabou, Remok, Saidi, Siddique, Ailli, Bourhia, Salamatullah, Ouahmane, Mouradi, Eto and Zair. This is an open-access article distributed under the terms of the Creative Commons Attribution License (CC BY). The use, distribution or reproduction in other forums is permitted, provided the original author(s) and the copyright owner(s) are credited and that the original publication in this journal is cited, in accordance with accepted academic practice. No use, distribution or reproduction is permitted which does not comply with these terms.
*Correspondence: Touriya Zair, emFpckB1bWkuYWMubWE=; Mohammed Bourhia, Ym91cmhpYW1vaGFtbWVkQGdtYWlsLmNvbQ==
Disclaimer: All claims expressed in this article are solely those of the authors and do not necessarily represent those of their affiliated organizations, or those of the publisher, the editors and the reviewers. Any product that may be evaluated in this article or claim that may be made by its manufacturer is not guaranteed or endorsed by the publisher.
Research integrity at Frontiers
Learn more about the work of our research integrity team to safeguard the quality of each article we publish.