- 1Department of Medical Physics, University of Wisconsin, Madison, WI, United States
- 2Department of Radiology, University of Wisconsin, Madison, WI, United States
Introduction: 43Sc and 44gSc are both positron-emitting radioisotopes of scandium with suitable half-lives and favorable positron energies for clinical positron emission tomography (PET) imaging. Irradiation of isotopically enriched calcium targets has higher cross sections compared to titanium targets and higher radionuclidic purity and cross sections than natural calcium targets for reaction routes possible on small cyclotrons capable of accelerating protons and deuterons.
Methods: In this work, we investigate the following production routes via proton and deuteron bombardment on CaCO3 and CaO target materials: 42Ca(d,n)43Sc, 43Ca(p,n)43Sc, 43Ca(d,n)44gSc, 44Ca(p,n)44gSc, and 44Ca(p,2n)43Sc. Radiochemical isolation of the produced radioscandium was performed with extraction chromatography using branched DGA resin and apparent molar activity was measured with the chelator DOTA. The imaging performance of 43Sc and 44gSc was compared with 18F, 68Ga, and 64Cu on two clinical PET/CT scanners.
Discussion: The results of this work demonstrate that proton and deuteron bombardment of isotopically enriched CaO targets produce high yield and high radionuclidic purity 43Sc and 44gSc. Laboratory capabilities, circumstances, and budgets are likely to dictate which reaction route and radioisotope of scandium is chosen.
1 Introduction
An increasingly popular approach to cancer therapy is combining chemically similar pairs of radionuclides as radiolabels of diagnostic and therapeutic drugs (“theranostics”). Positron emission tomography (PET) of 68Ga- (t1/2 = 67.71 min; β+Eavg = 829.5 keV; β+intensity = 88.91%) labeled somatostatin analogs and prostate-specific membrane antigen targeting agents has been used to monitor response to 177Lu- (t1/2 = 6.644 days; β−EAvg = 133.6 keV; β-intensity = 100.0%) based treatment (Weineisen, et al., 2015; Grubmüller, et al., 2018; Heinzel, et al., 2019), to select potential patients for therapy (Gains, et al., 2011), and to detect lesions not found on 177Lu single photon emission tomography (SPECT) (Sainz-Esteban, et al., 2012). However, the high average positron energy and short half-life of 68Ga limit its clinical application. Because 68Ga’s half-life is too short for off-site transport, clinics require an on-site cyclotron or a68Ge/68Ga generator to provide patient doses.
Radioisotopes of scandium are promising alternatives to 68Ga. There are three clinically interesting radioisotopes of scandium: 43Sc (t1/2 = 3.891 h; β+Eavg = 476 keV; β+intensity = 88.1%), 44gSc (t1/2 = 3.97 h; β+Eavg = 632 keV; β+intensity = 94.27%), and 47Sc (t1/2 = 3.349 days; β−Eavg = 162 keV; β-intensity = 100.0%) (National Nuclear Data Center, Brookhaven National, 2022). Both 43Sc and 44gSc emit positrons with lower mean energies than 68Ga, have high positron branching ratios, and are chemically identical to the therapeutic radionuclide 47Sc. Additionally, the ionic radius and coordination behavior of scandium are better matched to lutetium than gallium (Robert, 1976); its positron-emitting radioisotopes are viable diagnostic analogues of 177Lu in radiopharmaceuticals. Proton bombardment of natural metallic calcium targets produces 44gSc (Severin, et al., 2012), but long-lived (t1/2 = 44 h–84 days) radionuclidic impurities (46Sc, 47Sc, and 48Sc) are co-produced due to the many stable isotopes of calcium (40Ca, 96.941%; 42Ca, 0.647%; 43Ca, 0.135%; 44Ca, 2.086%; 46Ca, 0.004%; 48Ca, and 0.187%). Producing 43Sc and 44gSc from isotopically enriched calcium minimizes the presence of long-lived radioisotopes of scandium. This work investigates the following reactions on isotopically enriched calcium targets: 42Ca(d,n)43Sc, 43Ca(p,n)43Sc, 43Ca(d,n)44gSc, 44Ca(p,n)44gSc, 44Ca(p,2n)43Sc, and 44Ca(d,2n)44gSc.
There are several methods for separating 43/44gSc from calcium targets. Following target dissolution, extraction chromatography resins such as UTEVA® (Valdovinos, et al., 2015), N,N,N′,N′-tetra-n-octyldiglycolamide (DGA) (Alliot, et al., 2015), and hydroxamate (Severin, et al., 2012) isolate 43/44gSc from the calcium target material. Additionally, adding ion exchange resins such as Dowex® 50WX8 in combination with extraction chromatography resin such as DGA further removes trace metal impurities (Rane et al., 1966; Müller, et al., 2013; van der Meulen, et al., 2015).
Irradiating titanium targets with protons also produces 43/44gSc (Loveless, et al., 2021; Şekerci̇ et al., 2023). Like the calcium targets, natTi targets are feasible for producing 43/44gSc, but production of high radionuclidic purity 43/44gSc requires enriched titanium (46Ti and 47Ti) targets due to reactions on other stable isotopes of titanium (46Ti, 8.25%; 47Ti, 7.44%; 48Ti, 73.72%; 49Ti, 5.41%; 50Ti, 5.18%). The 46Ti(p,α)43Sc and 47Ti(p,α)44gSc reaction routes have lower cross sections than proton-induced 43/44gSc reactions on calcium target material, but 46Ti and 47Ti are cheaper than the enriched calcium material, and may also handle higher currents (Chernysheva, et al., 2021). Domnanich et al., 2017a, reported a 90–120 μAh, 15.1 ± 1.9 MeV proton irradiation of 10 mg of 97.0% ± 0.02% isotopically enriched 46TiO2 targets produced 1.17–1.22 MBq/μAh 43Sc with 98.2% ± 0.3% radionuclidic purity. Similarly, a 183 µAh, 12.0 ± 2.3 MeV proton irradiation of 10 mg of 57.9% ± 1.8% isotopically enriched 43CaCO3 (12.36% 44CaCO3) produced 2.62 MBq/μAh 43Sc with 66.2% ± 1.5% radionuclidic purity (33.3% ± 1.5% 44gSc) (Domnanich, et al., 2017b). Production of the 33.3% ± 1.5% 44gSc was the result of the (p,n) reaction on the 12.36% 44CaCO3 present in the target material. The two routes achieved similar radionuclidic purities with the 46TiO2(p,α)43Sc reaction producing 99.7% ± 0.5% 43/44gSc and the 43CaCO3(p,n)43Sc reaction producing 99% ± 2% 43/44gSc at end of bombardment.
For sites without accelerators, a generator of 44gSc’s long-lived parent 44Ti (t1/2 = 60.0 year) (National Nuclear Data Center, Brookhaven National, 2022) has been developed (Gajecki and Marino., 2023; under review; Schumann et al., 2007; Loktionova, et al., 2010; Filosofov, et al., 2010; Pruszyński and Loktionova, 2010; Radchenko, et al., 2016; Radchenko, et al., 2017; Rane, et al., 2015; Kerdjoudj, et al., 2016; Müller, et al., 2018; Roesch, 2012)1. 44Ti’s long half-life, the potential for high efficiency elutions (>97%) (Filosofov, et al., 2010), and the lack of 44mSc make this system appealing. For a44Ti/44gSc generator to be clinically viable, the generator must have low 44Ti breakthrough, 44gSc elutions suitable for labeling (low volume, high activity concentration), and consistent elution results. Several published generator designs use AG 1-X8 anion exchange resins which result in large elution volumes and 44Ti breakthrough requiring reverse elution modes (Filosofov, et al., 2010; Pruszyński and Loktionova, 2010; Roesch, 2012; Kerdjoudj, et al., 2016). More recent designs use ZR hydroxamate resin which may increase generator lifetimes due to the increased number of elutions achieved before 44Ti breakthrough is observed (Gajecki and Marino, 2023). A 47Ca/47Sc generator system for the therapeutic radioisotope of scandium is also in development (Mausner, et al., 1998; Rane, et al., 2015; Starovoitova, et al., 2015; Misiak, et al., 2017; Carzaniga et al., 2019; Pawlak, et al., 2019). However, 47Ca production requires enriched 48Ca which is expensive and not widely available.
It is also possible to produce 47Sc through proton bombardment of titanium targets [natTi(p,x)43,44g,47Sc, 48Ti(p,2n)47Sc, 50Ti(p,α)47Sc]. These reactions suffer from co-production of 46Sc and 48Sc at high energies (>30–35 MeV) so lower proton energies are ideal (Chernysheva, et al., 2021). There are also two reaction routes to 47Sc from calcium target material: 48Ca(p,2n)47Sc and 44Ca(α,p)47Sc (Minegishi, et al., 2016; Sitarz, et al., 2018; Carzaniga et al., 2019; Wibowo et al., 2021; Aikawa, et al., 2022). However, neither 48Ca target material nor alpha accelerators are widely accessible, and these reactions routes will likely not have widespread use. Other alternative production paths to produce 47Sc include proton bombardment of natural vanadium targets [natV(p,x)47Sc] (Jafari, et al., 2019; Barbaro, et al., 2021; De Nardo, et al., 2021), photonuclear reactions via electron linear accelerators [48Ti(γ,p)47Sc and 51V(γ,α)47Sc] (Kazakov, et al., 2012; Rotsch, et al., 2018; Loveless, et al., 2019; Aliev, et al., 2020; Snow, et al., 2021) and reactor production with fast [46Ca(n,γ)47Ca → 47Sc] or thermal [47Ti(n,p)47Sc] neutrons (Domnanich, et al., 2017b; Jalilian, et al., 2021).
Once radiochemically separated from the target material, 44gSc has been successfully complexed and exhibited high in-vivo stability with the commonly used chelator 1,4,7,10-tetraazacyclododecane-1,4,7,10-tetraacetic acid (DOTA) (Huclier-Markai, et al., 2014). As an alternative to DOTA, novel chelators designed and optimized for scandium include the heptadendate AAZTA ligands, the nonmacrocyclic H4pypa chelator, and the triaza-macrocycle-based picolinate-functionalized chelator H3mpatcn that can be labeled with scandium at room temperature (Li, et al., 2020a; Vaughn, et al., 2020a; Li, et al., 2020b; Vaughn, et al., 2020b; Fersing, et al., 2022). In vivo and in vitro studies have investigated 44gSc labeled with DOTA-based peptides (Hernandez, et al., 2014; van der Meulen, et al., 2015; Eppard, et al., 2017; Singh, et al., 2017) and conjugated AAZTA-PSMA peptides (Sinnes, et al., 2020; Ghiani, et al., 2021). Additionally, 44gSc-labeled antibody fragments detected 4T1 murine breast cancer tumors and U87MG human glioblastoma tumors in murine models (Chakravarty, et al., 2014; Li, et al., 2020b).
Preclinical and clinical PET/CT studies have compared the image quality of 44gSc (Bunka, et al., 2016; Ferguson, et al., 2019; Rosar, et al., 2020; Lima, et al., 2021) and, less frequently, 43Sc (Lima, et al., 2021), to conventional PET radionuclides. These studies have shown that both 43Sc and 44gSc have favorable contrast and image resolution compared to 68Ga. 43Sc’s, and 44gSc’s relatively longer half-lives enable transportation to nearby clinics, increasing their accessibility.
Currently, enriched calcium is only available as CaCO3, a suboptimal material for cyclotron irradiation due to CaCO3’s low thermal conductivity and decarboxylation (and subsequent release of CO2 at high temperatures) in beam. Additionally, while previous works have investigated cross section measurements of various proton- and deuteron-induced reactions on calcium, there is a lack of comprehensive studies comparing the various radionuclidic purities and production yields for small cyclotron-produced 43Sc and 44gSc. The goal of this work is to investigate and optimize the possible production routes of both 43Sc and 44gSc using enriched calcium targets irradiated on a small cyclotron capable of accelerating 16 MeV protons and 8 MeV deuterons and compare their contrast on clinical PET/CT scanners.
2 Materials and methods
2.1 Chemicals
Enriched 42CaCO3, 43CaCO3, and 44CaCO3 materials were obtained from the Department of Energy National Isotope Development Center with isotopic purities of 93.58%, 83.93%, and 98.89%, respectively (Table 1). Solutions were prepared with optima grade HCl and HNO3 purchased from Fisher Chemical and 18 MΩ·cm−1 water. N,N,N′,N′-tetrakis-2-ethylhexyldiglycolamide (branched DGA) resin was purchased from Eichrom. DOTA chelator and DOTA-(Tyr3)-octreotate (DOTA-TATE) were purchased from Macrocyclics.
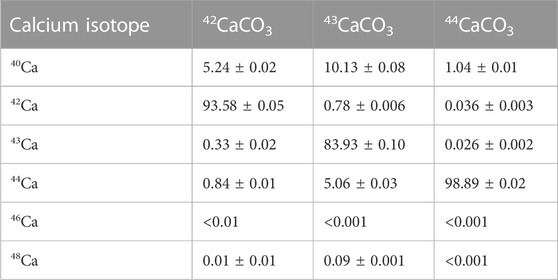
TABLE 1. Isotopic composition of enriched CaCO3 material received from the DOE National Isotope Development Center.
2.2 Target fabrication and cyclotron irradiation
Enriched CaCO3 targets have been reported to withstand proton currents up to 50 μA when kept relatively thin (0.1–0.2 mm) and of low mass (<10 mg) (van der Meulen, et al., 2015). However, this material is very hygroscopic and readily absorbs water from the atmosphere. Additionally, when sufficiently heated in beam, CaCO3 decarboxylates, forming CaO, offgassing CO2, potentially damaging the target, and reducing accelerator beam transmission. A transition from preclinical to clinical scale productions suggests need for larger target masses and/or higher beam currents to increase yield. To compensate for this increased power deposition, we used CaO as an alternative target material. CaCO3 was decarboxylated to CaO in a vertical tube furnace (Thermansys) heated in an inert atmosphere to 900°C–950°C. A graphite crucible containing approximately 150–300 mg of CaCO3 was lowered into a flat-bottomed quartz tube (the graphite crucible facilitated removal of the powder) and a compression fitting with an argon gas line was connected to the quartz tube to prevent degradation of the graphite crucible during heating. The crucible and quartz tube were placed in the preheated furnace under argon gas flow for 60–90 min. To confirm complete thermal decomposition of the CaCO3 material, the mass of the crucible and powder was measured before and after heating, and the decomposition of the graphite crucible was accounted for. Following the decarboxylation procedure, the CaO was quickly pressed into a 9.5 mm diameter niobium crucible with a hydraulic press (250 kg/cm2) and covered with a thin niobium cover foil to confine the target material and degrade the incident proton/deuteron energy (Figure 1). For the 44Ca(p,n)44gSc reaction, two incident energies were used. A 50 µm thick foil degraded the incident 16 MeV protons to 15.2 MeV and a 150 μm thick niobium cover foil degraded the incident energy to 13.6 MeV (calculated using SRIM) (Ziegler and Ziegler, 2010). Additionally, the 50 µm thick foil degraded the incident 8 MeV deuterons to 5.8 MeV. These energies were chosen to minimally degrade the 16 MeV proton beam (incident energy of 15.2 MeV) and 8 MeV deuteron beam (incident energy of 5.8 MeV) and to cover the peak of the cross-section curve (incident energy of 13.6 MeV). Figure 2 shows experimentally measured cross sections along with the energy range investigated. The target was then loaded on the University of Wisconsin, Madison’s GE PETtrace cyclotron or placed under vacuum in a furnace preheated to >100°C to keep the target from absorbing moisture. For the CaCO3 targets, 75–150 mg CaCO3 was first heated in a polypropylene centrifuge tube at 90°C for 30 min to ensure that the material was dry and then pressed directly into the niobium crucible. All targets were irradiated for 15°min to 1 h with 5–20 μA protons or 3–10 μA deuterons with water jet cooling on the back of the target.
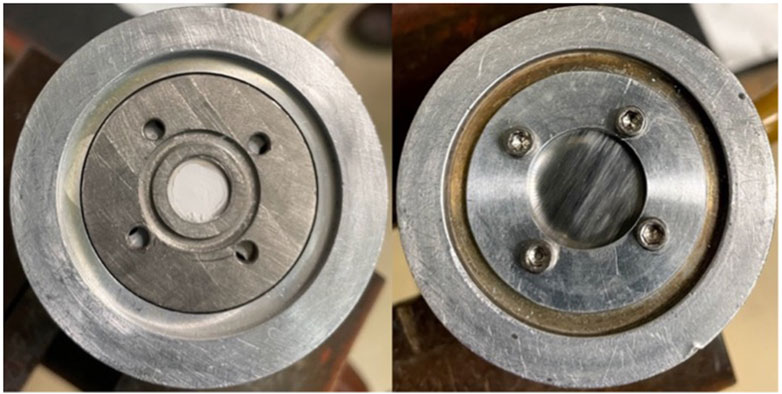
FIGURE 1. Niobium crucible containing ∼60 mg pressed CaO powder (left) and covered with 50 μm niobium cover foil (right).
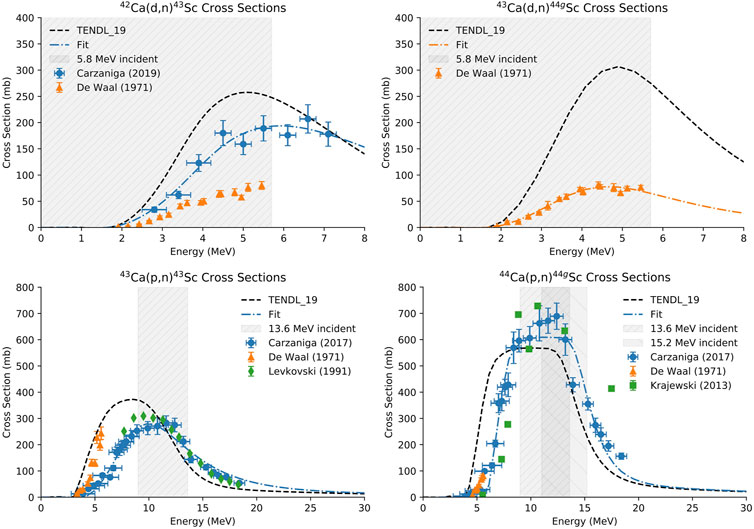
FIGURE 2. Cross sections for the 42Ca(d,n)43Sc, 43Ca(d,n)44gSc (top) (Carzaniga, et al., 2019; de Waal, et al., 1971a; Koning et al., 2012; de Waal, et al., 1971), 43Ca(p,n)43Sc, and 44Ca(p,n)44gSc (bottom) reactions. The energy range investigated is highlighted in gray and a fit of the experimental data is shown in blue.
To compare current tolerances, pressed powder natCaCO3 and natCaO targets of equal thickness were irradiated with 13.6 MeV protons. Cyclotron vacuum pressure was monitored during irradiation, and peak and stabilized pressures were recorded as the current was increased in 1 μA steps.
2.3 HPGe spectrometry
Targets were assayed via High Purity Germanium (HPGe) gamma spectrometry with an aluminum-windowed detector (Ortec) coupled to a Canberra Model C1519 research amplifier and multi-channel analyzer (full-width half-maximum at 1,333 keV = 1.8 keV) to quantify radionuclide activities 10–30 min after irradiation. 241Am, 133Ba, 152Eu, 137Cs, and 60Co check sources traceable to NIST were used to energy and efficiency calibrate the detector. Full targets were measured 200–400 cm from the detector face to quantify 43/44g/44mSc activity, and longer-lived radioisotopes of scandium (46Sc, 47Sc, and 48Sc) were quantified through 250 μL aliquots of the dissolved target (5 mL) measured overnight approximately 20 cm from the detector face. To determine end of bombardment (EoB) yields of long-lived scandium radioisotopes, the activity of 43Sc or 44gSc quantified in the aliquot provided a correction factor to obtain 46,47,48Sc full target activity.
2.4 Radiochemical isolation of 43/44gSc
Targets were dissolved in 5 mL 9 M HCl heated at 80°C with magnetic stir bar agitation for 30 min. Radiochemical separation of 43/44gSc from 42/43/44CaO target material used single-column extraction chromatography with branched DGA (132 ± 67 mg, 5.5 mm column diameter) resin pre-conditioned with 3 mL 0.01 M HCl followed by 5 mL 9 M HCl (Figure 3). The 5 mL dissolved target solution was loaded on to the conditioned resin using a peristaltic pump (1.6 mL/min) and collected for target recycling along with a 10 mL rinse of 6 M HCl. To remove trace metals and reduce the acidity in the column, a 12 mL 1 M HNO3 rinse was performed and 43/44gSc was subsequently eluted with four 500 μL fractions of 0.01 M HCl (Alliot, et al., 2015; Aluicio-Sarduy, et al., 2018). Activities were assayed in a Capintec CRC-15R dose calibrator (43Sc setting #825; 44gSc setting #938). Capintec dose calibrator settings were taken from the user’s manual and confirmed via cross-calibration with HPGe spectroscopy.
2.5 Recovery of enriched calcium
The enriched calcium target material was recovered through precipitation from the 5 mL load and 10 mL rinse solutions. The calcium was precipitated as CaCO3 through careful drop-wise addition of saturated (NH4)2CO3 solution (approximately 30 mL), allowing 10–30 s for the solution to settle and CO2 to be released between drops of (NH4)2CO3. The precipitate was then centrifuged, decanted, and dried under argon at 120°C. The dried CaCO3 could then be decarboxylated to CaO for future irradiations following the same procedure described above.
2.6 Apparent molar activity measurements and trace metals analysis
Trace metals analysis on the eluted 43/44gSc solutions was performed by microwave plasma-atomic emission spectrometry (MP-AES) using an Agilent Technologies 4,200 spectrometer. Calibration standards containing 10 ppb to 100 ppm of Fe, Zn, Cu, and Ca were prepared in 0.01 M HCl solutions from 1,000 mg/L standards purchased from Sigma-Aldrich.
The apparent molar activity of the eluted 43/44gSc solutions was determined through titration by reaction with varying concentrations of DOTA chelator. Polypropylene centrifuge tubes containing increasing concentrations of DOTA dissolved in 18 MΩ water (100 μL, 0–100 μg/mL) were prepared and small aliquots (10–15 μL, 3.7 ± 0.04 MBq) of 43/44gSc eluate were added to each vial. Additionally, 2–5 μL of 1.5 M NaOAc (pH = 4.5) buffer were added to each vial to reach a final pH between 4–4.5. The solutions were then vortexed and heated on a shaker plate at 80°C and 300°RPM for 30 min. The percent 43/44gSc complexed with DOTA was determined through thin layer chromatography (TLC) using aluminum backed silica gel plates (TLC Silica gel 60 F254, Sigma-Aldrich) in 50 mM ethylenediaminetetraacetic acid (EDTA). Plates were imaged with storage phosphor autoradiography (Packard Cyclone). Under these conditions, the radiolabeled 43/44gSc-DOTA stayed at the origin (retention factor, Rf = 0) while the free 43/44gSc travelled with the mobile front (Rf = 1). The apparent molar activity (MBq/µmol) of 43/44gSc with DOTA was found by dividing the activity of 43/44gSc in each vial by twice the amount of DOTA required to complex 50% of the activity.
Radiolabeling experiments were also performed with the DOTA-based peptide DOTA-TATE following the same radiolabeling conditions listed above for DOTA. Briefly, 100 μL 43/44gSc (37 ± 5 MBq) was vortexed with 2–3 μL DOTA-TATE (4.3–5.7°nmol, 0.2 mCi/nmol; 7.14°MBq/nmol) and 2–5 μL 1.5 M NaOAc pH 4.5 buffer and heated to 80°C while shaking at 300°RPM for 15–30 min. Percent complexation was determined through radio-TLC and high-performance liquid chromatography (HPLC) using a C18 column (4.6 × 250 mm, Dionex 5 μm 120 Å; ThermoFisher) and a water (0.1% trifluoroacetic acid, TFA) to acetonitrile gradient (5% MeCN, 0–3.5°min; 5%–35% MeCN, 3.5–13°min; 35%–90% MeCN, 13–15°min; 90% MeCN, 15–19°min; 90%–5% MeCN, 19–20°min; 5% MeCN, 20–25 min) flowing at 1 mL/min.
2.7 Clinical phantom scans
In this study, 43Sc, 44gSc, and a mixture of 43Sc and 44gSc (43/44gSc) were compared to other positron-emitting radionuclides (18F, 68Ga, and 64Cu) in a clinical Derenzo phantom (acrylic) purchased from Phantech (Madison, WI). The University of Wisconsin, Madison’s GE PETtrace cyclotron was used to produce 43Sc [42CaO(d,n)43Sc], 44gSc [44CaO(p,n)44gSc], 18F [H218O(p,n)18F], and 64Cu [64Ni(p,n)64Cu]. The mixture of 43/44gSc (88% 43Sc, 12% 44gSc by activity at end of chemistry) was obtained through 13.6 MeV proton bombardment of 83.93% isotopically enriched 43CaO targets containing 5.06% 44Ca. 68Ga was eluted from a GalliaPharm 68Ge/68Ga generator (5 mL, 0.1°M HCl, Eckert and Ziegler, Inc.) at the University of Wisconsin, Madison radiopharmaceutical production facility. Table 2 lists the physical decay characteristics of the radionuclides used in this study and the effective half-life, positron intensity, and mean positron energy for the 43/44gSc mixture.

TABLE 2. Physical decay characteristics and effective half-life and positron intensity of the radionuclides used in this study (National Nuclear Data Center, Brookhaven National, 2022).
The Derenzo phantom consisted of 6 sections of rods with diameters of 3.5, 4.0, 4.5, 5.0, 5.5, and 6.0°mm, and was filled with activities shown in Table 3. Filled activities were increased for low positron intensity radionuclides (64Cu) and short-lived radionuclides (68Ga and 18F) to keep scan times consistent (under 15 min). The concentrated activity solutions were prepared by first mixing 250 mL 18 MΩ water, 200 μL Tween® 80 (Sigma-Aldrich), 25 μL 6 M HCl (final pH 4.5), and then adding the radioactivity (2.74–19.97 MBq; 0.075–2.16 mCi). Tween® was used to prevent bubbles from forming in the linear Derenzo phantom and the acid was added to prevent the radiometals from sticking to the walls of the phantom. Additionally, 2–3 drops of food coloring were added to facilitate detection of air bubbles when filling the small rods of the Derenzo phantom. Radioactivity was drawn into 1 mL syringes and measured in a Capintec CRC-15R dose calibrator before and after adding to the prepared solutions.
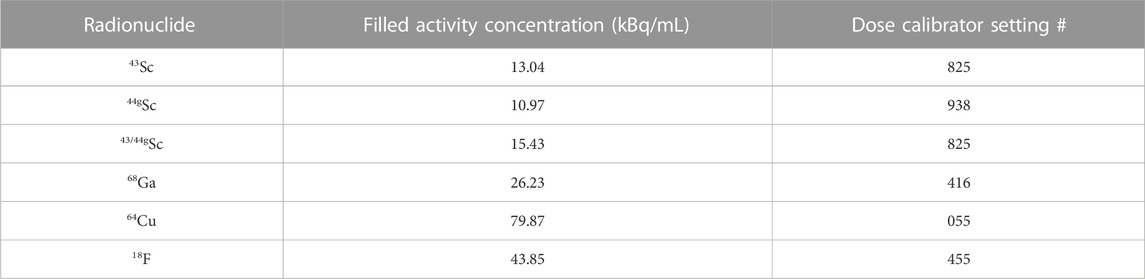
TABLE 3. Filled radioactivity derived from dose calibrator and HPGe recorded radioactivities and measured volumes.
The filled phantom was scanned on a clinical GE Discovery 710 and GE Discovery MI PET/CT (GE Healthcare, Waukesha, WI) scanner. The GE Discovery 710 has 13,824 lutetium-yttrium oxyorthosilicate (LYSO) scintillator crystals (4.2 × 6.3 × 25 mm3) optically connected to photomultiplier tubes without a light-guide (Yoon et al., 2015). The axial field of view is 15.7 cm, and the transaxial field of view is 70 cm. The energy window is 425–650 keV with a coincidence window of 4.9 ns. The GE Discovery MI has 19584 LYSO scintillator crystals (3.95 × 5.3 × 25 mm3) connected to arrays of silicon photomultiplier (SiPM) detectors through light-guides (Hsu et al., 2017). The axial field of view is 20 cm, and the transaxial field of view is 70 cm. The energy and coincidence windows are identical to the GE Discovery 710 at 425–650 keV and 4.9 ns, respectively. Ten million coincidences were collected regardless of which radionuclide the phantom was filled with. Data were reconstructed using an OSEM-TOF reconstruction algorithm (VPFX; without point spread function) with a 256 × 256 matrix size and 16 subsets, 8 iterations on the GE Discovery MI and 24 subsets, 5 iterations on the GE Discovery 710.
Contrast was compared across the six radionuclides. To determine contrast, a transverse slice in the center of the phantom was selected, and a line profile was drawn from the center of one outermost rod to the center of the opposing outermost rod. The maximum and minimum values were then used to calculate the contrast:
where
3 Results
3.1 Target fabrication and cyclotron irradiation
Pressed powder natCaCO3 and natCaO targets of approximately equal thickness (0.63 ± 0.02 mm; 158 ± 11 mg) were fabricated and irradiated with 13.6 MeV protons. The time required to reach 20 μA was 61 min for the natCaCO3 target and 23 min for the natCaO target. The natCaCO3 targets generated more gas load on the cyclotron’s vacuum system, slowing their progression to 20 μA. Following irradiation, the natCaO target sustained far less damage and did not suffer material losses while the natCaCO3 was fractured during irradiation (Figure 4).
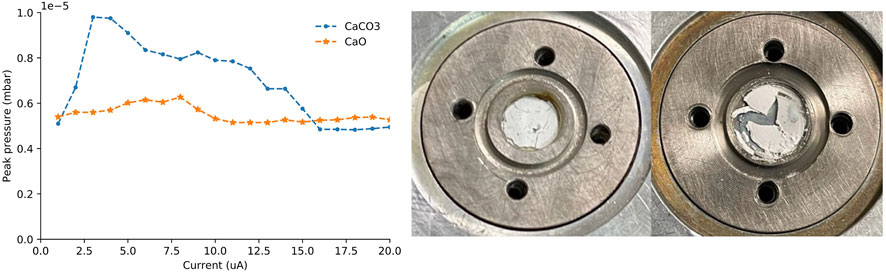
FIGURE 4. Peak pressure on the target as the current was increased (left). Pictures of the natCaO (middle) and natCaCO3 (right) targets immediately following 0–20 µA proton irradiation.
For deuteron production yield measurements (n = 3 per route), 42/43CaO targets that were thick to the 5.8 MeV deuteron beam were fabricated (0.25 ± 0.01 mm; 61 ± 3 mg) (Figure 2). For proton production yield measurements (n = 3 per route), 43/44CaO targets that were 4.7 MeV thick to the 13.6 MeV beam (0.62 ± 0.02 mm; 149 ± 2 mg) were fabricated to capture the flat region of the cross section curve for the 43Ca(p,n)43Sc and 44Ca(p,n)44gSc reactions (Figure 2) (Carzaniga, et al., 2017). Energy loss through the CaO targets was calculated using the Bethe-Bloch formula.
The 44Ca(p,2n)43Sc reaction has an energy threshold close to the maximum 16 MeV protons that the GE PETtrace can accelerate, so the thinner 50 μm niobium foil was used to minimally degrade the proton energy to 15.2 MeV (targets were kept the same mass and were consequently only 4.3 MeV thick for this reaction).
Targets were irradiated with either 5 μA protons or 3 μA deuterons with a total charge between 0.5 and 1.6 µAh. Production yields for the 42Ca(d,n)43Sc, 43Ca(p,n)43Sc, 43Ca(d,n)44gSc, 44Ca(p,n)44gSc, and 44Ca(p,2n)43Sc reactions are shown in Table 4. No 43Sc from the 44Ca(p,2n)43Sc was observed, indicating that 15.2 MeV is too close to the energy threshold for the reaction to produce significant quantities of 43Sc. This result agrees with previously reported data at 15.3 MeV (Carzaniga, et al., 2017).
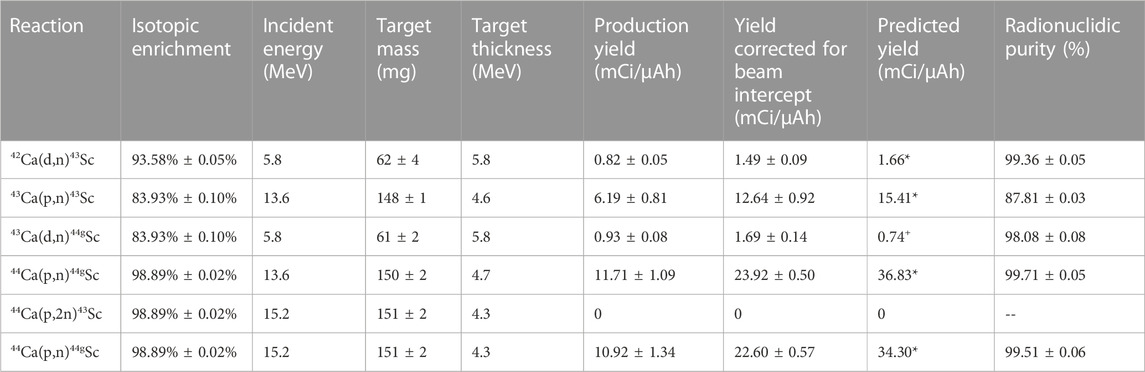
TABLE 4. Observed production yields for the 5 routes investigated along with target masses and energy thickness (Carzaniga, et al., 2017; Carzaniga, et al., 2019)* (de Waal, et al., 1971)+.
Targets were also evaluated for radionuclidic purity via HPGe spectrometry. Figure 5 shows HPGe-measured yield decay-corrected to EoB (mCi/μAh) for each radioisotope of scandium, and the radionuclidic purity as a percentage of total activity decay-corrected to EoB is listed in Table 4 (n = 3 per reaction route).
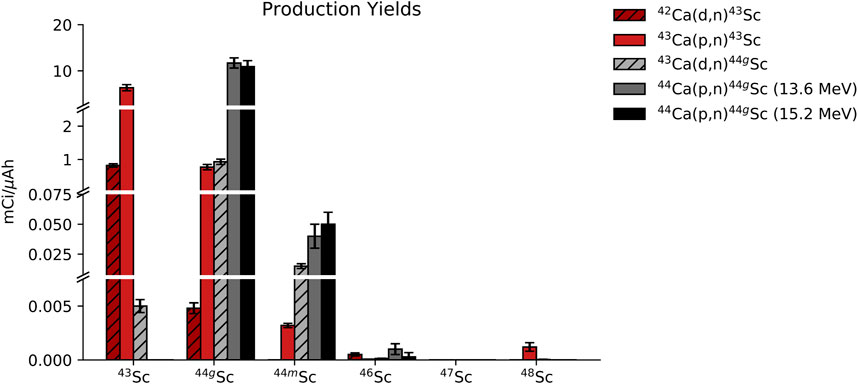
FIGURE 5. Activity of each scandium radioisotope that was present in the full target at EoB for each of the 5 reaction routes that were investigated.
The GE PETtrace beam spot on target is larger than the 9.5 mm target diameter, so the control system’s measured integrated charge on target (µA·h) overestimates integrated fluence on the 42/43/44Ca targets. To account for this, the niobium cover foil was used to determine the percentage of the beam that intercepted the target. After irradiation, a beam profile was obtained through autoradiography, and an ROI with a width of 9.5 mm was drawn and centered on the beam profile. A larger >20 mm diameter ROI was also drawn encompassing the entire beam spot. Additionally, four other 9.5 mm ROIs with an offset of up to 1.5 mm from center were drawn around the central region to determine the variance in beam intersection that may be caused from the beam drifting from the center. These values were independently measured for the proton and deuteron beams and were also evaluated before and after a cyclotron ion source puller electrode replacement that occurred during the measurement of these production yields. The measured beam intersections and the max difference between the offset regions are listed in Table 5 with a correction to the measured production yields listed in Table 4.
Figure 6 shows the predicted physical yields versus target thickness for a 13.6 MeV proton (left) and 5.8 MeV deuteron beam (right). Dashed lines are predicted yields taken from fits to published cross section values. The fitted cross sections used for this calculation are shown in blue in Figure 2. Experimentally measured, beam-spot corrected yields are additionally shown in Figure 6. Experimentally measured yields are similar to, but slightly lower than, predicted yields except in the case of the 43Ca(d,n)44gSc reaction where measured yields were higher than predicted. Predicted yields were based on cross-sections reported by Carzaniga (Carzaniga, et al., 2017; Carzaniga, et al., 2019) except for the 43Ca(d,n)44gSc reaction where the only experimental data available was reported in (de Waal et al., 1971a). For the other deuteron-induced reaction reported in (de Waal et al., 1971b) [42Ca(d,n)43Sc], the cross sections were lower than those reported by Carzaniga et al. (2019) for the same energy range. The discrepancy between cross sections reported by Carzaniga and de Waal are likely the cause of the difference in trends between the experimentally measured and predicted yields observed in this work.
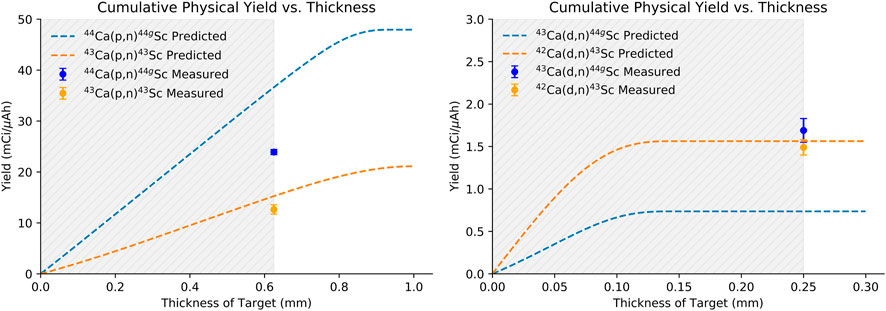
FIGURE 6. Measured production yields compared to literature reported cross sections fitted to TENDL_19 predicted curves for 13.6 MeV incident protons (left) and 5.8 MeV incident deuterons (right) (de Waal, et al., 1971b; Levkovski, 1991; Koning et al., 2012; Krajewski, et al., 2013; Carzaniga, et al., 2017; Carzaniga, et al., 2019).
Figure 3 illustrates the separation strategy for isolating the Sc+3 ions from the Ca+2 ions. The low affinity for Ca+2 ions on the branched DGA resin permitted efficient recycling from the 5 mL load and 10 mL 6 M HCl rinse stages of the separation (Alliot, et al., 2015). Other +2 metals (such as Cu+2) are also rinsed from the resin in this step. The 1 M HNO3 wash removes metals such as iron and zinc due to the low affinity for these ions in 1 M HNO3 and minimal loss of Sc+3 was observed as seen in the elution profile (Figure 7) (Alliot, et al., 2015). Elution with 2 mL of 0.01 M HCl effectively removes 92.8% ± 4.0% of the loaded scandium. However, there is large variation in the activity that is in each 0.01 M HCl elution fraction (n = 10), perhaps due to variations in volumes of liquid being used to elute the activity (residual liquid may be left in tubing or on walls in parts of the system). Following a 1 h, 5 μA, 13.6 MeV proton irradiation of a 150 mg 44CaO target, this chemical isolation procedure isolates 1.4 ± 0.3 GBq (38 ± 9 mCi) of 44gSc with 99.5% ± 0.05% radionuclidic purity in 2 mL 0.01 M HCl 2 h after EoB.
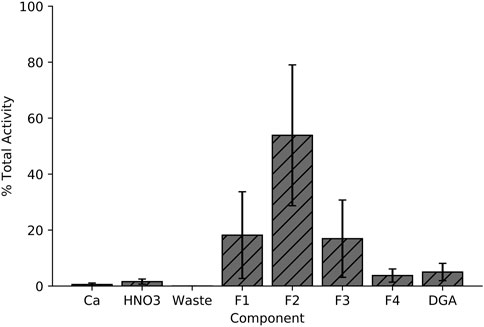
FIGURE 7. Elution profile for the Ca+2/Sc+3 radiochemical separation used in this work. Activity distribution in each of the 4 eluted fractions varies with each experiment, but the overall eluted fraction remains consistent.
3.2 Recovery of enriched calcium
42/43/44Ca was recovered in the form of 42/43/44CaCO3 through slow addition of (NH4)2CO3 and converted back to 42/43/44CaO through decarboxylation in a vertical tube furnace (900°C for 60–90 min) for future irradiations. The enriched 42/43/44CaCO3 target material was recovered with an efficiency of 95% ± 2% (n = 20). If argon gas is passed over the graphite crucible during the decarboxylation procedure, the crucible surface remains smooth and losses from transferring and heating are <1%.
3.3 Apparent molar activity measurements and trace metals analysis
The quality of the final 43/44gSc eluent was determined through titrimetric reactions with the chelator DOTA and the ability to label with the DOTA-based peptide DOTA-TATE. Molar activity of 43/44gSc produced through a 30–60 min, 13.6 MeV proton energy and 5 μA current irradiation of either 43CaO or 44CaO targets was 48 ± 11 GBq/μmol (1.3 ± 0.3 Ci/μmol) (n = 3) at end of chemistry. Additionally, 37 ± 5 MBq (1 ± 0.14 mCi) aliquots of 43/44gSc were labeled with DOTA-TATE at a molar activity of 7.14 MBq/nmol (0.2 mCi/nmol) and assayed using HPLC. Radio-HPLC chromatograms (Figure 8) show near quantitative labeling (>98%). Free 43/44gSc eluted with the solvent front (4 min) and 43/44gSc-DOTA-TATE eluted at 15.6 min.
Amounts of Ca and commonly seen metals such as Fe, Zn, and Cu that may affect Sc-labelling were measured through MP-AES and are listed in Table 6 (n = 5). The efficient removal of metals such as Fe, Zn, and Cu in the radiochemical isolation strategy resulted in the high apparent molar activity observed with Sc and DOTA. The mass of Ca that was co-loaded on to the column and seen in the eluent resulted in minimal enriched material loss (<1 mg).
3.4 Clinical phantom scans
The contrast, Crod, was compared between the 6 radionuclides on the two PET/CT scanners. Figure 9 shows a cross-sectional image of the reconstructed Derenzo phantom on the GE Discovery 710 (similar images were seen on the GE Discovery MI). Qualitative differences can be seen between shorter-range positron-emitters such as 64Cu and 18F compared to the higher-range positron-emitter 68Ga. All six sections of rods are easily distinguishable for 64Cu and 18F while the lower diameter rods (3.5 and 4 mm) are significantly blurred in the 68Ga image. Radioisotopes of scandium demonstrate image quality between the low and high positron range radionuclides where the smallest diameter rods are indistinguishable, but resolution improves with the 4 mm diameter rods. The calculated contrast values are shown in Figure 9 as well.
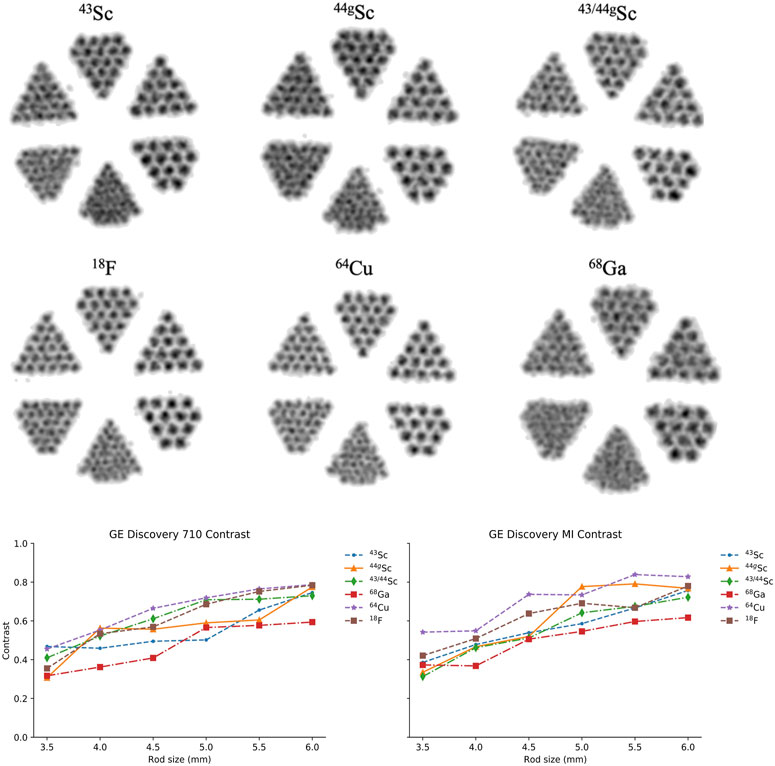
FIGURE 9. Reconstructed PET scans of the Derenzo phantom filled with each radionuclide on the GE Discovery 710 (top). Comparison of contrast (
On both scanners, 64Cu and 18F demonstrated the greatest contrast across the varying rod diameters while 68Ga performed the worst across all rod diameters which is consistent with what we would expect from the positron energies listed in Table 2. Between 43Sc, 44gSc, and 43/44gSc, there is no significant difference in contrast, suggesting minimal benefit in contrast from 43Sc’s softer positron energy using these clinical PET/CT systems.
4 Discussion
The goal of this study was to compare production yields, radionuclidic purity, and PET image quality between 43Sc and 44gSc. Among the six reactions that produce 43Sc and 44gSc with protons and deuterons on small cyclotrons, the 44Ca(p,2n)43Sc and 44Ca(d,2n)44gSc reactions are only possible above 15.2 MeV protons and 5.8 MeV deuterons, eliminating some commercial machines from consideration. The proton-induced reactions investigated here [43Ca(p,n)43Sc and 44Ca(p,n)44gSc] had significantly higher production yields (by approximately a factor of 10) than the deuteron-induced reactions [42Ca(d,n)43Sc and 43Ca(d,n)44gSc] but were performed with higher mass targets (approximately 150 mg versus 60 mg CaO) and higher incident energies (13.6 or 15.2 MeV protons versus 5.8 MeV deuterons). The peak of the cross-section curves for the deuteron-induced reactions were not fully captured with the energies and target thickness used here (Figure 2, gray area). Accelerators capable of accelerating higher energy deuterons would be able to produce higher yields through these routes. Thick target yields for the proton-induced reactions were not investigated in this work due to the large masses (∼250 mg CaO) required to degrade the proton energy in the target from 13.6 MeV to 4 MeV where the cross section approaches zero (Carzaniga, et al., 2017).
Due to the relatively low isotopic purity of available 43Ca (83.93%), an EoB radionuclidic purity of only 87.81% ± 0.03% 43Sc was achievable through proton bombardment of 43Ca. The main radioisotopic contaminants from this reaction are 44gSc at 12.16% ± 0.03% total activity and 48Sc (t1/2 = 43.71 h; β-mean = 220.5 keV; β+intensity = 100%) at 0.02% ± 0.01% total activity at EoB. The production of 44gSc results from a (p,n) reaction on the 5.06% 44Ca present in the enriched 43Ca target material and could be minimized through use of higher purity 43Ca target material. With 100% isotopically pure 43Ca, 44gSc would be removed and 43Ca(p,n)43Sc measured yields would increase to 288.6 MBq/μAh (7.38 mCi/μAh), or 557.2 MBq/μAh (15.06 mCi/μAh) when corrected for beam intercept. Considering the alternative deuteron-induced reaction, 42Ca(d,n)43Sc, the radionuclidic purity at EoB was 99.36% ± 0.05% 43Sc at EoB with no co-production of 44gSc’s metastable state 44mSc. However, this route will require longer irradiation times and a cyclotron capable of accelerating deuterons to produce the same EoB activity as the proton-induced route.
For producing 44gSc, the 44Ca(p,n)44gSc route results both in higher production yields and greater radionuclidic purity than the 43Ca(d,n)44gSc route with the conditions investigated in this work. This is partially due to the low purity of the 43Ca and the smaller mass of the target. Cyclotrons capable of accelerating higher energy deuterons (>8 MeV) along with higher purity and thicker 43Ca targets would increase the production yields compared to those reported here. However, the higher predicted cross sections for the 44Ca(p,n)44gSc reaction compared to 43Ca(d,n)44gSc (Figure 2) suggest that the highest yields of 44gSc would still be through proton bombardment of 44Ca. The presence of longer-lived radioisotopes of scandium such as 46Sc are also comparable in both reaction routes in the target thickness and energy range investigated herein: At EoB, there was 0.007% ± 0.003% 46Sc for 44Ca(p,n)44gSc and 0.013% ± 0.003% 46Sc for 43Ca(d,n)44gSc.
When considering translation to clinical studies, long-lived radioisotopes of scandium (46Sc, 47Sc, and 48Sc) should be minimized to avoid patient dose and handling of radioactive waste (De Nardo, et al., 2021). The threshold for 68Ge (t1/2 = 270.8 days) impurity set by the European Pharmacopoeia in 68Ge/68Ga generator elutions is 0.001% (Council of Europe, 2013, 2013). The presence of 46Sc (t1/2 = 83.79 days), 47Sc (t1/2 = 3.3492 days), and 48Sc (t1/2 = 43.71 h) for the reaction routes at the irradiation parameters investigated herein are lower than the 0.001% 68Ge limit at EoB with the exception of 43Ca(p,n)43Sc which results in 0.0012% 48Sc at the end of bombardment.
Radiochemical isolation with branched DGA resin was procedurally simple and achieved efficient target recycling and high radiochemical yields [95% ± 2% enriched calcium recovery (n = 20) and 92% ± 4% 43/44gSc activity in 2 mL 0.01 M HCl (n = 10)]. From the calcium mass that was measured via MP-AES, the Ca/Sc separation factor is on the order of ∼105. Quantitative labeling with DOTA-TATE and high apparent molar activities with DOTA were additionally achieved with this separation strategy and minimal trace metals were observed through MP-AES analysis.
Because PET resolution is impacted by positron range (energy), 43Sc may have better PET resolution than 44gSc. Additionally, it has been suggested that 44gSc’s higher energy gamma (1,157 keV; 99.9%) as opposed to 43Sc’s lower energy gamma (373 keV; 22.5%) may contribute negatively to image quality due to the potential to scatter within a PET scanner’s 511 keV window (Carzaniga, et al., 2017) and negatively to patient dosimetry. In this study we observed no significant difference in the contrast of the phantoms filled with 43Sc, 44gSc, or the mixture 43/44gSc.
5 Conclusion
This work demonstrated that isotopically enriched CaO targets can be used to produce high yield and high radionuclidic purity 43Sc and 44gSc through proton and deuteron bombardment. While several reaction routes produce either 43Sc or 44gSc, laboratory capabilities, circumstances, and budgets are more likely to dictate which reaction route is chosen. When comparing PET image quality, 43Sc and 44gSc contrast were comparable, and in some cases favorable compared to other conventional PET radionuclides. In addition, no significant difference in contrast was observed due to the lower energy of 43Sc’s positron compared to 44gSc. These results indicate that, while 44gSc’s higher energy gamma emission and co-produced metastable state (44mSc) may have dosimetric consequences for in-human studies in the future, with regards to yields, radionuclidic purity, and image contrast, 44gSc has superior or comparable characteristics compared to 43Sc.
Data availability statement
The raw data supporting the conclusion of this article will be made available by the authors, without undue reservation.
Author contributions
KB: Methodology, experimental investigation, analysis, experimental design, original draft writing, review and editing EA-S: Methodology, analysis, review and editing TB: Experimental investigation, analysis, review and editing SH: Analysis, review and editing AO: Analysis, review and editing KB: Analysis, review and editing JB: Resources, methodology, review PE: Methodology, analysis, review and editing TB: Resources, review and editing AP: Experimental design, resources, methodology, analysis, review and editing JE: Experimental design, methodology, analysis, review and editing.
Funding
Research reported in this publication was supported by the National Cancer Institute of the National Institutes of Health under Award Number T32CA009206. This work was supported by an award from the Department of Energy Isotope Program AO gratefully acknowledges support from NIH F31 Ruth L Kirschstein Predoctoral Individual National Research Service Award F31CA239617.
Conflict of interest
The authors declare that the research was conducted in the absence of any commercial or financial relationships that could be construed as a potential conflict of interest.
Publisher’s note
All claims expressed in this article are solely those of the authors and do not necessarily represent those of their affiliated organizations, or those of the publisher, the editors and the reviewers. Any product that may be evaluated in this article, or claim that may be made by its manufacturer, is not guaranteed or endorsed by the publisher.
Footnotes
1Kretowicz, M. N. (2023). Promising Scandium-44 radionuclides for nuclear medicine from a 44Ti/44gSc generator.
References
Aikawa, M., Hanada, Y., Ichinkhorloo, D., Haba, H., Takács, S., Ditrói, F., et al. (2022). Production cross sections of 47Sc via alpha-particle-induced reactions on natural calcium up to 29 MeV. Nucl. Instrum. Methods Phys. Res. Sect. B Beam Interact. Mater. Atoms 515, 1–6. doi:10.1016/j.nimb.2022.01.008
Aliev, R. A., Belyshev, S. S., Furkina, E. B., Khankin, V. V., Kuznetsov, A. A., Dzhilavyan, L. Z., et al. (2020). Photonuclear production of medically relevant radionuclide 47Sc. J. Radioanalytical Nucl. Chem. 326, 1099–1106. doi:10.1007/s10967-020-07400-5
Alliot, C., Kerdjoudj, R., Michel, N., Haddad, F., and Huclier-Markai, S. (2015). Cyclotron production of high purity 44m,44Sc with deuterons from 44CaCO3 targets. Nucl. Med. Biol. 42 (5), 524–529. doi:10.1016/j.nucmedbio.2015.03.002
Aluicio-Sarduy, E., Hernandez, R., Valdovinos, H. F., Kutyreff, C. J., Ellison, P. A., Barnhart, T. E., et al. (2018). Simplified and automatable radiochemical separation strategy for the production of radiopharmaceutical quality 86Y using single column extraction chromatography. Appl. Radiat. Isotopes 142, 28–31. doi:10.1016/j.apradiso.2018.09.016
Barbaro, F., Canton, L., Carante, M. P., Colombi, A., De Dominicis, L., Fontana, A., et al. (2021). New results on proton-induced reactions on vanadium for 47Sc production and the impact of level densities on theoretical cross sections. Phys. Rev. C 104, 044619. doi:10.1103/physrevc.104.044619
Bunka, M., Müller, C., Vermeulen, C., Haller, S., Türler, A., Schibli, R., et al. (2016). Imaging quality of 44Sc in comparison with five other PET radionuclides using Derenzo phantoms and preclinical PET. Appl. Radiat. Isotopes 110, 129–133. doi:10.1016/j.apradiso.2016.01.006
Carzaniga, T. S., Auger, M., Braccini, S., Bunka, M., Ereditato, A., Nesteruk, K. P., et al. (2017). Measurement of 43Sc and 44Sc production cross-section with an 18 MeV medical PET cyclotron. Appl. Radiat. Isotopes 129, 96–102. doi:10.1016/j.apradiso.2017.08.013
Carzaniga, T. S., and Braccini, S. (2019). Cross-section measurement of 44mSc,47Sc, 48Sc and 47Ca for an optimized 47Sc production with an 18 MeV medical PET cyclotron. Appl. Radiat. Isotopes 143, 18–23. doi:10.1016/j.apradiso.2018.10.015
Carzaniga, T. S., van der Meulen, N. P., Hasler, R., Kottler, C., Peier, P., Türler, A., et al. (2019). Measurement of the 43Sc production cross-section with a deuteron beam. Appl. Radiat. Isotopes 145, 205–208. doi:10.1016/j.apradiso.2018.12.031
Chakravarty, R., Goel, S., Valdovinos, H. F., Hernandez, R., Hong, H., Nickles, R. J., et al. (2014). Matching the decay half-life with the biological half-life: ImmunoPET imaging with 44Sc-labeled cetuximab fab fragment. Bioconjugate Chem. 25 (12), 2197–2204. doi:10.1021/bc500415x
Chernysheva, M., Loveless, S. C., Brossard, T., Becker, K., Cingoranelli, S., Aluicio-Sarduy, E., et al. (2021). Accelerator production of scandium radioisotopes: Sc-43, Sc-44, and Sc-47. Curr. Radiopharm. 14 (4), 359–373. doi:10.2174/1874471014999210112205535
Council of Europe (2013). Gallium (68Ga) edotreotide injection. European Directorate for the Quality of Medicines.
De Nardo, L., Mou, L., Furlanetto, D., Rosato, A., Esposito, J., Meléndez-Alafort, L., et al. 2021. Preliminary dosimetric analysis of DOTA-folate radiopharmaceutical radiolabelled with 47Sc produced through natV(p,x)47Sc cyclotron irradiation. Phys. Med. Biol., 66(2).025003, doi:10.1088/1361-6560/abc811
de Waal, T. J., Peisach, M., and Pretorius, R. (1971b). Activation cross sections for proton-induced reactions on calcium isotopes up to 5·6 MeV. J. Inorg. Nucl. Chem. 33 (9), 2783–2789. doi:10.1016/0022-1902(71)80038-6
de Waal, T. J., Peisach, M., and Pretorius, R. (1971a). Activation gross sections for deuteron-induced reactions on calcium isotopes up to 5.5 MeV. Radiochim. Acta 15, 123–127. doi:10.1524/ract.1971.15.3.123
Domnanich, K. A., Eichler, R., Müller, C., Jordi, S., Yakusheva, V., Braccini, S., et al. (2017a). Production and separation of 43Sc for radiopharmaceutical purposes. EJNMMi 2 (1), 14. doi:10.1186/s41181-017-0033-9
Domnanich, K. A., Müller, C., Benešová, M., Dressler, R., Haller, S., Köster, U., et al. (2017b). 47Sc as useful β–-emitter for the radiotheragnostic paradigm: A comparative study of feasible production routes. EJNMMI Radiopharm. Chem. 2 (5), 5. doi:10.1186/s41181-017-0024-x
Eppard, E., de la Fuente, A., Benešová, M., Khawar, A., Bundschuh, R. A., Gärtner, F. C., et al. (2017). Clinical translation and first in-human use of [44Sc]Sc-PSMA-617 for PET imaging of metastasized castrate-resistant prostate cancer. Theranostics 7 (18), 4359–4369. doi:10.7150/thno.20586
Ferguson, S., Jans, H. S., Wuest, M., Riauka, T., and Wuest, F. (2019). Comparison of scandium-44 g with other PET radionuclides in pre-clinical PET phantom imaging. EJNMMI Phys. 6 (23), 23. doi:10.1186/s40658-019-0260-0
Fersing, C., Masurier, N., Rubira, L., Deshayes, E., and Lisowski, V. (2022). AAZTA-derived chelators for the design of innovative radiopharmaceuticals with theranostic applications. Pharmaceuticals 15 (2), 234. doi:10.3390/ph15020234
Filosofov, D. V., Loktionova, N. S., and Rösch, F. (2010). A 44Ti/44Sc radionuclide generator for potential application of 44Sc-based PET-radiopharmaceuticals. rca-Radiochimica Acta 98 (3), 149–156. doi:10.1524/ract.2010.1701
Gains, J. E., Bomanji, J. B., Fersht, N. L., Sullivan, T., D'Souza, D., Sullivan, K. P., et al. (2011). 177Lu-DOTATATE molecular radiotherapy for childhood neuroblastoma. J. Nucl. Med. 52 (7), 1041–1047. doi:10.2967/jnumed.110.085100
Gajecki, L., Marino, C., Cutler, C. S., and Sanders, V. A. (2023). Evaluation of hydroxamate-based resins towards a more clinically viable 44Ti/44Sc radionuclide generator. Appl. Radiat. Isotopes 192, 110588. doi:10.1016/j.apradiso.2022.110588
Ghiani, S., Hawala, I., Szikra, D., Trencsényi, G., Baranyai, Z., Nagy, G., et al. (2021). Synthesis, radiolabeling, and pre-clinical evaluation of [44Sc]Sc-AAZTA conjugate PSMA inhibitor, a new tracer for high-efficiency imaging of prostate cancer. Eur. J. Nucl. Med. Mol. Imaging 48, 2351–2362. doi:10.1007/s00259-020-05130-0
Grubmüller, B., Senn, D., Kramer, G., Baltzer, P., D’Andrea, D., Grubmüller, K. H., et al. (2018). Response assessment using 68Ga-PSMA ligand PET in patients undergoing 177Lu-PSMA radioligand therapy for metastatic castration-resistant prostate cancer. Eur. J. Nucl. Med. Mol. Imaging 46, 1063–1072. doi:10.1007/s00259-018-4236-4
Heinzel, A., Boghos, D., Mottaghy, F. M., Gaertner, F., Essler, M., von Mallek, D., et al. (2019). 68Ga-PSMA PET/CT for monitoring response to 177Lu-PSMA-617 radioligand therapy in patients with metastatic castration-resistant prostate cancer. Eur. J. Nucl. Med. Mol. Imaging 46, 1054–1062. doi:10.1007/s00259-019-4258-6
Hernandez, R., Valdovinos, H. F., Yang, Y., Chakravarty, R., Hong, H., Barnhart, T. E., et al. (2014). 44Sc: An attractive isotope for peptide-based PET imaging. Mol. Pharm. 11 (8), 2954–2961. doi:10.1021/mp500343j
Hsu, D. F., Ilan, E., Peterson, W. T., Uribe, J., Lubberink, M., and Levin, C. S. (2017). Studies of a next-generation silicon-photomultiplier–based time-of-flight PET/CT system. J. Nucl. Med. 58 (9), 1511–1518. doi:10.2967/jnumed.117.189514
Huclier-Markai, S., Kerdjoudj, R., Alliot, C., Bonraisin, A., Michel, N., Haddad, F., et al. (2014). Optimization of reaction conditions for the radiolabeling of DOTA and DOTA-peptide with 44m/44Sc and experimental evidence of the feasibility of an in vivo PET generator. Nucl. Med. Biol. 41, e36–e43. doi:10.1016/j.nucmedbio.2013.11.004
Jafari, A., Aboudzadeh, M. R., Azizakram, H., Sadeghi, M., Alirezapour, B., Rajabifar, S., et al. (2019). Investigations of proton and deuteron induced nuclear reactions on natural and enriched Titanium, Calcium and Vanadium targets, with special reference to the production of 47Sc. Appl. Radiat. Isotopes 152, 145–155. doi:10.1016/j.apradiso.2019.07.007
Jalilian, A. R., et al. (2021). IAEA activities on 67Cu, 186Re, 47Sc theranostic radionuclides and radiopharmaceuticals. Curr. Radiopharm. 14 (4), 306–314. doi:10.2174/18744729mtewimjka0
Kazakov, A. G., Ekatova, T. Y., and Babenya, J. S. (2012). Photonuclear production of medical radiometals: A review of experimental studies. J. Radioanalytical Nucl. Chem. 328, 493–505. doi:10.1007/s10967-021-07683-2
Kerdjoudj, R., Pniok, M., Alliot, C., Kubíček, V., Havlíčková, J., Rösch, F., et al. (2016). Scandium(III) complexes of monophosphorus acid DOTA analogues: A thermodynamic and radiolabelling study with 44Sc from cyclotron and from a 44Ti/44Sc generator. Dalton Trans. 45, 1398–1409. doi:10.1039/c5dt04084a
Koning, A. J., and Rochman, D. (2012). Modern nuclear data evaluation with the TALYS code system. Nucl. Data Sheets 113 (12), 2841–2934. doi:10.1016/j.nds.2012.11.002
Krajewski, S., Cydzik, I., Abbas, K., Bulgheroni, A., Simonelli, F., Holzwarth, U., et al. (2013). Cyclotron production of 44Sc for clinical application. Radiochim. Acta 101, 333–338. doi:10.1524/ract.2013.2032
Levkovski, V. N. (1991). Cross sections of medium mass nuclide activation (A= 40-100) by medium energy protons and alpha-particles (E= 10-50 MeV). Tallinn: Inter-Vesi.
Li, L., Jaraquemada-Peláez, M. d. G., Aluicio-Sarduy, E., Xiaozhu, W., et al. (2020a). Coordination chemistry of [Y(pypa)]− and comparison immuno-PET imaging of [44Sc]Sc- and [86Y]Y-pypa-phenyl-TRC105. R. Soc. Chem. 49, 5547–5562. doi:10.1039/D0DT00437E
Li, L., Jaraquemada-Peláez, M. d. G., Aluicio-Sarduy, E., Wang, X., Jiang, D., Sakheie, M., et al. (2020b). [nat/44Sc(pypa)]−: Thermodynamic stability, radiolabeling, and biodistribution of a prostate-specific-membrane-antigen-targeting conjugate. Inorg. Chem. 59 (3), 1985–1995. doi:10.1021/acs.inorgchem.9b03347
Lima, T. V. M., Gnesin, S., Strobel, K., Pérez, M. d. S., Roos, J. E., Müller, C., et al. (2021). Fifty shades of scandium: Comparative study of PET capabilities using Sc-43 and Sc-44 with respect to conventional clinical radionuclides. Diagnostics 11 (10), 1826. doi:10.3390/diagnostics11101826
Loktionova, N., Dmitry, F., Marek, P., Frank, R., et al. (2010). Design and performance of a novel 5 mCi 44Ti/44Sc-radionuclide generator. J. Nucl. Med. 51, 1467.
Loveless, C. S., Blanco, J. R., Diehl, G. L., Elbahrawi, R. T., Carzaniga, T. S., Braccini, S., et al. (2021). Cyclotron production and separation of scandium radionuclides from natural titanium metal and titanium dioxide targets. J. Nucl. Med. 62 (1), 131–136. doi:10.2967/jnumed.120.242941
Loveless, C. S., Radford, L. L., Ferran, S. J., Queern, S. L., Shepherd, M. R., and Lapi, S. E. (2019). Photonuclear production, chemistry, and in vitro evaluation of the theranostic radionuclide 47Sc. EJNMMI Res. 9 (42), 42. doi:10.1186/s13550-019-0515-8
Mausner, L. F., Kolsky, K., Joshi, V., and Srivastava, S. (1998). Radionuclide development at BNL for nuclear medicine therapy. Appl. Radiat. Isotopes 49 (4), 285–294. doi:10.1016/s0969-8043(97)00040-7
Minegishi, K., Kotaro, N., Masami, F., Hisashi, S., Tomoyuki, O., Ming-Rong, Z., et al. 2016. Production of scandium-43 and -47 from a powdery calcium oxide target via the nat/44Ca(α,x)-channel. Appl. Radiat. Isotopes, Volume 116, pp. 8–12.doi:10.1016/j.apradiso.2016.07.017
Misiak, R., Walczak, R., Wąs, B., Bartyzel, M., Mietelski, J. W., and Bilewicz, A. (2017). 47Sc production development by cyclotron irradiation of 48Ca. J. Radioanalytical Nucl. Chem. 313, 429–434. doi:10.1007/s10967-017-5321-z
Müller, C., Bunka, M., Reber, J., Fischer, C., Zhernosekov, K., Türler, A., et al. (2013). Promises of cyclotron-produced 44Sc as a diagnostic match for trivalent β−-emitters: In vitro and in vivo study of a 44Sc-DOTA-Folate conjugate. J. Nucl. Med. 5 (12), 2168–2174. doi:10.2967/jnumed.113.123810
Müller, C., Domnanich, K. A., Umbricht, C. A., and van der Meulen, N. P. (2018). Scandium and terbium radionuclides for radiotheranostics: Current state of development towards clinical application. Br. J. Radiology 91 (1091), 20180074. doi:10.1259/bjr.20180074
National Nuclear Data Center, Brookhaven National , 2022. National nuclear data center, brookhaven national. Available at: https://www.nndc.bnl.gov/nudat3/[Accessed 05 April 2023].
Pawlak, D., Wojdowska, W., Parus, L. J., Cieszykowska, I., Zoltowska, M., Garnuszek, P., et al. (2019). Comparison of separation methods for 47Ca/47Sc radionuclide generator. Appl. Radiat. Isotopes 151, 140–144. doi:10.1016/j.apradiso.2019.05.020
Pruszyński, M., Loktionova, N. S., Filosofov, D., and Rösch, F. (2010). Post-elution processing of 44Ti/44Sc generator-derived 44Sc for clinical application. Appl. Radiat. Isotopes 68 (9), 1636–1641. doi:10.1016/j.apradiso.2010.04.003
Radchenko, V., Engle, J. W., Medvedev, D. G., Maassen, J. M., Naranjo, C. M., Unc, G. A., et al. (2017). Proton-induced production and radiochemical isolation of 44Ti from scandium metal targets for 44Ti/44Sc generator development. Nucl. Med. Biol. 50, 25–32. doi:10.1016/j.nucmedbio.2017.03.006
Radchenko, V., Meyer, C., Engle, J., Naranjo, C., Unc, G., Mastren, T., et al. (2016). Separation of 44Ti from proton irradiated scandium by using solid-phase extraction chromatography and design of 44Ti/44Sc generator system. J. Chromatogr. A 1477, 39–46. doi:10.1016/j.chroma.2016.11.047
Rane, A. T., and Bhatki, K. S. (1966). Rapid radiochemical separations of strontium-90-yttrium-90 and calcium-45-scandium-46 on a cation exchange resin. Anal. Chem. 38 (11), 1598–1601. doi:10.1021/ac60243a039
Rane, S., Harris, J. T., and Starovoitova, V. N. (2015). 47Ca production for 47Ca/47Sc generator system using electron linacs. Appl. Radiat. Isotopes 97, 188–192. doi:10.1016/j.apradiso.2014.12.020
Robert, S. D. (1976). Revised effective ionic radii and systematic studies of interatomic distances in halides and chalcogenides. Acta Crystallogr. Sect. A Cryst. Phys. Diffr. Theor. general Crystallogr. 32 (5), 751–767. doi:10.1107/s0567739476001551
Roesch, F. (2012). Scandium-44: Benefits of a long-lived PET radionuclide available from the 44Ti/44Sc generator system. Curr. Radiopharm. 5 (3), 187–201. doi:10.2174/1874471011205030187
Rosar, F., Buchholz, H. G., Michels, S., Hoffmann, M. A., Piel, M., Waldmann, C. M., et al. (2020). Image quality analysis of 44Sc on two preclinical PET scanners: A comparison to 68Ga. EJNMMI Phys. 7 (16), 16. doi:10.1186/s40658-020-0286-3
Rotsch, D. A., Brown, M. A., Nolen, J. A., Brossard, T., Henning, W. F., Chemerisov, S. D., et al. (2018). Electron linear accelerator production and purification of scandium-47 from titanium dioxide targets. Appl. Radiat. Isotopes 131, 77–82. doi:10.1016/j.apradiso.2017.11.007
Sainz-Esteban, A., Prasad, V., Schuchardt, C., Zachert, C., Carril, J. M., and Baum, R. P. (2012). Comparison of sequential planar 177Lu-DOTA-TATE dosimetry scans with 68Ga-DOTA-TATE PET/CT images in patients with metastasized neuroendocrine tumours undergoing peptide receptor radionuclide therapy. Eur. J. Nucl. Med. Mol. Imaging 39, 501–511. doi:10.1007/s00259-011-2003-x
Schumann, D., Horn, S., and Neuhausen, J. (2007). Design of a 44Ti/44Sc generator system. Annual Report. Villigen, Switzerland: Paul Scherrer Institut & Universitaet Bern, 41.
Şekerci̇, M., Özdoğan, H., and Kaplan, A. (2023). A study on the cross-section data of 43,44m,46,47Sc isotopes via (d,x) reactions on natural abundance targets under the effects of deuteron optical models. Appl. Radiat. Isotopes.94, 110714, doi:10.1016/j.apradiso.2023.110714
Severin, G. W., Engle, J., Valdovinos, H., Barnhart, T., and Nickles, R. (2012). Cyclotron produced 44gSc from natural calcium. Appl. Radiat. Isotopes 70 (8), 1526–1530. doi:10.1016/j.apradiso.2012.04.030
Singh, A., van der Meulen, N. P., Müller, C., Klette, I., Kulkarni, H. R., Türler, A., et al. (2017). First-in-Human PET/CT imaging of metastatic neuroendocrine neoplasms with cyclotron-produced 44Sc-dotatoc: A proof-of-concept study. Cancer Biotherapy Radiopharmeceuticals 32 (4), 124–132. doi:10.1089/cbr.2016.2173
Sinnes, J.-P., Bauder-Wüst, U., Schäfer, M., Sung Moon, E., et al. (2020). 68Ga, 44Sc and 177Lu-labeled AAZTA5-PSMA-617: Synthesis, radiolabeling, stability and cell binding compared to DOTA-PSMA-617 analogues. EJNMMI Radiopharm. Chem. 5 (28), 28. doi:10.1186/s41181-020-00107-8
Sitarz, M., Szkliniarz, K., Jastrzębski, J., Choiński, J., Guertin, A., Haddad, F., et al. (2018). Production of Sc medical radioisotopes with proton and deuteron beams. Appl. Radiat. Isotopes 142, 104–112. doi:10.1016/j.apradiso.2018.09.025
Snow, M. S., Foley, A., Ward, J. L., Kinlaw, M. T., Stoner, J., and Carney, K. P. (2021). High purity 47Sc production using high-energy photons and natural vanadium targets. Appl. Radiat. Isotopes 178, 109934. doi:10.1016/j.apradiso.2021.109934
Starovoitova, V. N., Cole, P. L., and Grimm, T. L. (2015). Accelerator-based photoproduction of promising beta-emitters 67Cu and 47Sc. J. Radioanalytical Nucl. Chem. 305, 127–132. doi:10.1007/s10967-015-4039-z
Valdovinos, H. F., Hernandez, R., Barnhart, T., Graves, S., Cai, W., and Nickles, R. (2015). Separation of cyclotron-produced 44Sc from a natural calcium target using a dipentyl pentylphosphonate functionalized extraction resin. Appl. Radiat. Isotopes 95, 23–29. doi:10.1016/j.apradiso.2014.09.020
van der Meulen, N. P., Bunka, M., Domnanich, K. A., Müller, C., Haller, S., Vermeulen, C., et al. (2015). Cyclotron production of 44Sc: From bench to bedside. Nucl. Med. Biol. 42 (9), 745–751. doi:10.1016/j.nucmedbio.2015.05.005
Vaughn, B. A., Aluicio-Sarduy, E., Devaraj, J., Olson, A. P., Jonathan, E., Boros, E., et al. (2020a). Chelation with a twist: A bifunctional chelator to enable room temperature radiolabeling and targeted PET imaging with scandium-44. R. Soc. Chem. 11, 333–342. doi:10.1039/C9SC04655K
Vaughn, B. A., Koller, A. J., Chen, Z., Ahn, S. H., Loveless, C. S., Cingoranelli, S. J., et al. (2020b). Homologous structural, chemical, and biological behavior of Sc and Lu complexes of the picaga bifunctional chelator: Toward development of matched theranostic pairs for radiopharmaceutical applications. Bioconjugate Chem. 32 (7), 1232–1241. doi:10.1021/acs.bioconjchem.0c00574
Weineisen, M., Schottelius, M., Simecek, J., Baum, R. P., Yildiz, A., Beykan, S., et al. (2015). 68Ga- and 177Lu-labeled PSMA I&T: Optimization of a PSMA-targeted theranostic concept and first proof-of-concept human studies. J. Nucl. Med. 56 (8), 1169–1176. doi:10.2967/jnumed.115.158550
Wibowo, F. A., and Kambali, I. (2021). Calculated cyclotron-based scandium-47 production yields via 48Ca(p, 2n)47Sc nuclear reaction. J. Phys. Conf. Ser. 1816 (1), 012099. doi:10.1088/1742-6596/1816/1/012099
Yoon, H. J., Jeong, Y. J., Son, H. J., Kang, D. Y., Hyun, K. Y., and Lee, M. K. (2015). Optimization of the spatial resolution for the GE Discovery PET/CT 710 by using NEMA NU 2-2007 standards. J. Korean Phys. Soc. 66, 287–294. doi:10.3938/jkps.66.287
Keywords: scandium, positron emission tomography (PET), cyclotron, radionuclide production, phantom imaging
Citation: Becker KV, Aluicio-Sarduy E, Bradshaw T, Hurley SA, Olson AP, Barrett KE, Batterton J, Ellison PA, Barnhart TE, Pirasteh A and Engle JW (2023) Cyclotron production of 43Sc and 44gSc from enriched 42CaO, 43CaO, and 44CaO targets. Front. Chem. 11:1167783. doi: 10.3389/fchem.2023.1167783
Received: 16 February 2023; Accepted: 18 April 2023;
Published: 26 April 2023.
Edited by:
Zeynep Talip, Paul Scherrer Institut (PSI), SwitzerlandReviewed by:
Gaia Pupillo, Legnaro National Laboratories (INFN), ItalyFerid Haddad, Université de Nantes, France
Copyright © 2023 Becker, Aluicio-Sarduy, Bradshaw, Hurley, Olson, Barrett, Batterton, Ellison, Barnhart, Pirasteh and Engle. This is an open-access article distributed under the terms of the Creative Commons Attribution License (CC BY). The use, distribution or reproduction in other forums is permitted, provided the original author(s) and the copyright owner(s) are credited and that the original publication in this journal is cited, in accordance with accepted academic practice. No use, distribution or reproduction is permitted which does not comply with these terms.
*Correspondence: Jonathan W. Engle, andlbmdsZUB3aXNjLmVkdQ==